DOI:
10.1039/D2QI02461F
(Research Article)
Inorg. Chem. Front., 2023,
10, 1614-1622
Solvent-controlled synthesis of an Al12-oxo molecular ring and Al24-oxo truncated metallo-cube†
Received
21st November 2022
, Accepted 27th January 2023
First published on 28th January 2023
Abstract
Highly symmetrical molecules with beautiful geometries are ubiquitous in nature. It has inspired creative ideas of the perfect combination of geometry and molecular structural chemistry. Some metal clusters with regular geometric polyhedra have been reported, but such highly symmetric polyhedra for Al-oxo clusters are really scarce on account of the fast hydrolysis of Al3+ ions. Herein, a [Al12(CH3O)24(NAP)12]·4DMF·2H2O·2CH3OH (Al12, NAP− = 2-naphthoic acid) nanoring was synthesized by the solvothermal reaction of AlCl3·6H2O, 2-naphthoformic acid (HNAP) and triethylamine (Et3N) in CH3OH and DMF. Interestingly, the regulation from ring-shaped Al12 to [Al24(OH)32(CH3O)22(CH3OH)2(NAP)12]·6Cl·2H2O·2CH3OH (Al24) metallocage is realized by only changing the reactive solvents. The Al24 metallocage can be seen as one of 13 Archimedean polyhedra, a truncated cube composed of eight Al3 triangles and six Al8 octagons by sharing vertical Al3+ ions. In addition, high-resolution electrospray ionization mass spectrometry (HR-ESI-MS) reveals that the metallic skeletons of Al12 and Al24 can be maintained stable in CH3OH and CH2Cl2. Furthermore, Al12 and Al24 emit blue luminescence and exhibit photocurrent responses under LED light illumination.
Introduction
Highly symmetrical polyhedra have intrigued various professional researchers in the fields of mathematics, biology, aesthetics, architecture, and chemistry for thousands of years.1–6 The classical polyhedra contain 5 platonic polyhedra constructed by one kind of regular convex polygon with the same number of faces at each vertex, and 13 Archimedean polyhedra composed of two or more types of regular polygons.7 In chemistry, these exemplifications have been found in coordination molecular nanocages and nanoclusters of coordination chemistry.8 For coordination nanocages, based on the construction rules of polyhedra, suitable organic ligands with specific configurations are often selected for their syntheses.9–14 However, the nanoclusters assembled from simple ingredients are elusory and the acquisition of geometric structures lacks the presupposition. As a famous polyhedral cluster, C60 is constructed by 12 pentagons and 20 hexagons, which is a classical Archimedean polyhedron, truncated icosahedron, inspiring various creative ideas for building fullerene-like nanopolyhedral metal clusters.15 After long-term unremitting efforts, transition/lanthanide metal clusters with Platonic and Archimedean polyhedra have been isolated, such as V24,16 Co32,17 Cd66,18 Mo240,19 Pd145,20 Ag180,21 and Ln104.22
As the most abundant metal element in the earth's crust, Al clusters with lightweight and high stability have potential applications in environmental science, geochemistry, biology, optical, and adsorption materials.23,24 Aqueous aluminum chemistry presents a series of typical aluminum oxo-hydroxo polycation aggregations, as exemplified by flat-Al13 [Al13(OH)24(H2O)24]15+,25,26 isomers of the Al13-Keggin cluster [Al13O4(OH)24(H2O)12]7+,24,27 and larger Al30 clusters [Al30O8(OH)56(H2O)26]18+.24,28 They were produced from hydrolytic processes of Al3+ ions in aqueous solutions, which is actually intricate and affected by olation reactions, formation of precursors, aggregation, nucleation and crystallization.24,27 An effective route is to choose suitable trapping ligands, such as Al3 and Al8 protected by the trisilanol ligand,29 a supramolecular zeotype Al15 constructed using hpdta (H5hpdta = HOCH2[CH2N(CH2COOH)2]2).30 Although many efforts have been made to investigate the aqueous aluminum chemistry of hydrolysis and polymerization of Al3+ ions, the relationship of solution equilibria and the existence of polynuclear species is still complex and unpredictable.31 For this dilemma, recently, a new strategy known as coordination delayed hydrolysis has been adopted by Zhang et al. to synthesize a series of Al-oxo clusters by choosing monodentate carboxylic acid ligands and organic aluminium salts under solvothermal conditions.32–35 Most of these Al-oxo clusters feature the structures of molecular rings from Al8 to the largest Al20 constructed using different large conjugated carboxylic acids.33,34,36 The different peripheral ligands realize luminescence modulation of Al20 molecular rings from blue to green. Besides, other Al clusters also display luminescence properties, which originated from the luminescence of the corresponding ligands.37 Nevertheless, highly symmetric aluminum-oxo clusters are rare because of the rapid hydrolysis of Al3+ ions and the difficult precipitation of high-quality and determinable single crystals.36 Many efforts will be made to further explore the assembly and luminescence properties of aluminum-oxo clusters.
Based on these considerations, enlightened by the simple one-pot solvothermal synthesis method of transition/lanthanide metal clusters accompanied by adding alkali to control the hydrolysis of metal ions, a ring Al12 dodecagon with the formula, [Al12(CH3O)24(NAP)12]·4DMF·2H2O·2CH3OH was obtained in CH3OH–DMF by the reaction of monodentate carboxylic acid and inorganic aluminium salt with Et3N to adjust the alkalinity of the solution. When using CH3OH–CH3CN as the solvent, a new Archimedean polyhedron Al24, [Al24(OH)32(CH3O)22(CH3OH)2(NAP)12]·6Cl·2H2O·2CH3OH was isolated. It features a truncated-cube metallocage containing 8 Al3 triangles and 6 Al8 octagons. This work successfully realizes the regulation of Al-oxo skeletons from molecular ring to metallocage. Moreover, the metallic cores of Al12 and Al24 have good stability in methanol and dichloromethane with the ligand exchange. Furthermore, the photocurrent response and the temperature-responsive luminescence behaviour of the Al12 and Al24 were investigated.
Experimental
Materials and physical measurements
Chemicals and solvents were purchased without further purification. On an ABB Bomen MB 102 series FT-IR (KBr pellets) spectrometer, IR spectra were recorded in the 4000–400 cm−1 region. The elemental analyses were conducted on a Vario EL cube analyzer. Powder X-ray diffraction (PXRD) data were obtained on the Rigaku SmartLab X-ray diffractometer. Thermogravimetric (TG) curves were measured from ambient temperature to 800 °C using the SDT Q600 analyzer. A Puxi Tu-1901 spectrophotometer was used to measure UV-vis spectra. A Bruker Impact II high-definition mass spectrometer quadrupole and time-of-flight (Q/TOF) modules were used to record the mass spectra (HR-ESI-MS). The photocurrent experiments were performed on a CHI660E electrochemistry workstation. The 10 L of naphthol (0.5 wt%) and 5 mg samples of Al12 or Al24 were combined with 0.5 mL of ethanol and then subjected to a 30-minute sonication process. The coated film was produced by pipetting 150 μL solution onto the cleaned ITO glass after evaporation. A Pt wire served as the counter electrode, an Ag/AgCl electrode served as the reference electrode, and the produced ITO glass film served as the working electrode. The medium was an aqueous solution of Na2SO4 (0.2 M). An F-4700 fluorescence spectrometer was used to measure the room-temperature fluorescence. The Edinburgh spectrofluorometer (FLS980), with a cryostat to regulate temperature, was used to collect variable-temperature fluorescence data. After a 10-minute homeothermy, each data was collected and quantum yield data were obtained on the integrating sphere, and the luminescence lifetimes were determined on the same device using a time-correlated single-photon counting method (FLS980).
Synthesis of Al24
A mixture of 2-naphthoformic acid (HNAP, 172.2 mg, 1 mmol), AlCl3·6H2O (500 mg, 2 mmol), were dissolved in a mixed solvent of CH3OH (3 mL)
:
CH3CN (8 mL) in a 23 mL Teflon-lined reaction vessel, then 0.5 mL triethylamine (Et3N) were added into the mixture under stirring, finally kept at 100 °C for 4 days. When slowly cooled to room temperature, washed with CH3OH, and dried in the air, colorless block crystals were obtained (yield 35.62% based on AlCl3·6H2O). Elemental analyses calcd. (found) for Al24: C158H202Al24Cl6O84: C, 44.08 (43.89); H, 4.73 (4.92)%. Selected IR peaks (cm−1): 3450 (s), 3060 (w), 2950 (m), 2840 (w), 2670 (w),1610 (s), 1570 (m), 1480 (s), 1430 (s), 1070 (m), 982 (m), 870 (m), 796 (s), 594 (s), 501 (w).
Synthesis of Al12
The Al12 is similar to Al24 except for CH3OH
:
CH3CN (11 mL, v
:
v = 3
:
8) was replaced by CH3OH and DMF (10 mL, v
:
v = 1
:
1), colorless lamellar crystals were obtained (yield 40.56% based on AlCl3·6H2O). Elemental analyses calcd. (found) for Al12: C170H196Al12N4O56: C, 58.09 (57.87); H, 5.62 (5.78); N, 1.59 (1.62)%. Selected IR peaks (cm−1): 3434 (s), 2964 (m), 2925 (m), 2856 (m), 2296 (w), 1629 (s), 1381 (m), 1080 (m), 1043 (m), 929 (w), 879 (w), 565 (w).
X-ray crystallography
Single crystals of Al24 and Al12 with the appropriate dimensions were selected under an optical microscope, coated fast into high vacuum grease, and put on a glass fiber for single-crystal data collection with Cu Kα radiation on the Rigaku XtaLAB MM007 CCD diffractometer. OLEX38 and SHELXL-97 were used to directly solve the structures.39 Based on the relevant atoms and refined with predetermined temperature factors, all hydrogen atoms were theoretically hydrogenated. To ensure that no extra symmetry could be given to the models, the Addsym function of PLATON40 was used to check each structure. The SQUEEZE command was used to eliminate the disorganized solvent molecules. The Cambridge Crystallographic Data Centre (CCDC) has received the crystallographic information from this article (CCDC: 2163632 (Al12); 2163633 (Al24)).† Table S6† provides the pertinent crystallographic data. Table S7† displays the selected bond lengths and angles.
Results and discussion
Crystal structure of Al12 cluster
Single-crystal X-ray diffraction analysis revealed that the Al12 cluster crystallizes in the triclinic P
space group and it contains 12 Al3+, 12 NAP−, and 24 CH3O− (Fig. 1a). Twelve Al3+ ions form a complanate dodecagon with the Al–Al distances in the range of 2.8621–2.8781 Å and every Al3+ ion is located on the vertex of a dodecagon. The diameter of the Al12 metal ring is about 9.93 Å. The whole Al12 cluster is stabilized by 12 NAP− and 24 CH3O−, which can be divided into three layers (Fig. 1b). The peripheral ligands of the middle layer contain six NAP− ligands and six CH3O−, which alternately array to form a large outer ring parallel to the Al12 metal dodecagon (Fig. 1c). The inner part of the middle layer is filled with parallel six CH3O−, in which three face up and three faces down. The upper and lower two ligand layers vertical to the Al12 metal dodecagon are identical and every layer comprises three NAP− and six CH3O− (Fig. 1d). Every two methanol molecules are sandwiched between two NAP− ligands. All Al3+ ions of Al12 cluster adopt six-coordinated distorted octahedral geometry with six O atoms from 2 NAP− and 4 CH3O−. The NAP− and CH3O− respectively adopt μ2–η1:η1 and μ2 connection modes. The Al–O distances and O–Al–O angles are in the range of 1.850–1.946 Å and 77.01–175.27°, respectively.
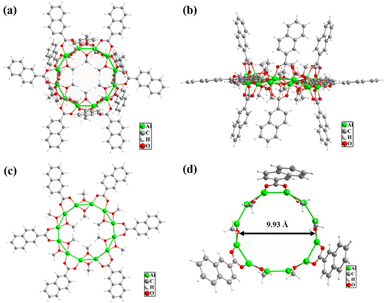 |
| Fig. 1 (a) and (b) Molecular structures of Al12 at different view directions. (c) and (d) The ligand arrangement of the middle and upper/lower layers of Al12 (green: Al; red: O; grey: C; white: H). | |
Crystal structure of Al24 cluster
Similar to the synthesis of Al12, one new Al24 cluster with the formula [Al24(OH)32(CH3O)22(CH3OH)2(NAP)12]·6Cl·2H2O·2CH3OH was obtained by using the solvent of CH3OH–CH3CN. It crystallizes in the monoclinic P21/n space group and the asymmetric unit contains half an Al24 cluster. As shown in Fig. 2a, the Al24 molecular skeleton is composed of eight [Al3(OH)(CH3O)3] in the truncated positions and six open [Al8(OH)4(CH3O)4(NAP)4] windows. The [Al3(OH)(CH3O)3] building block is coordinated by 3 CH3O− capped on the Al–Al sides and 1 OH− stamped over the centre of the triangle (Fig. 2b). The [Al8(OH)4(CH3O)4(NAP)4] consists of four NAP− and OH−, both capped on the interval four sides, and the remaining four sides are fixed by four CH3O− (Fig. 2c). The Al24 is a cation cluster with six free Cl− ions in the centre of octagonal windows bonded to the Al24 frame by hydrogen-bond interactions, which are formed with four μ2-OH− in the interior of the octagon with the Cl⋯O distances in the range of 3.174–3.302 Å and the Cl⋯H–O angles in the range of 161.16–165.06° (Table S4†). This Cl− template interaction is frequently found in the lanthanide clusters, such as Dy76,41 Ln48,42–44 and Ln15.45–47 The Al24 metallic inner features a truncated cube (Fig. 2d) with a small 7 Å diameter, though the total molecular Al24 cluster with a larger 2.0 nm diameter due to the peripheral big NAP− ligands. The Al–Al interactions are in the range of 2.853(3)–2.976(3) Å.
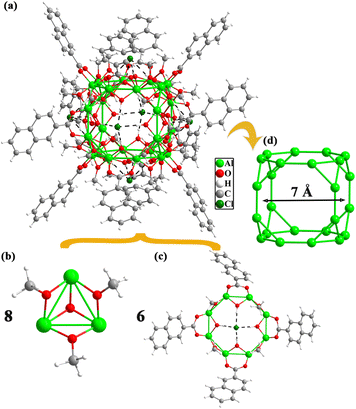 |
| Fig. 2 (a) Molecular structures of Al24. (b) and (c) The [Al3(OH)(CH3O)3] and [Al8(OH)4(CH3O)4(NAP)4] building blocks. (d) The 24-nuclearity Al metallic skeleton with a truncated hexahedral geometry (green: Al; red: O; grey: C; dark green: Cl; white: H). | |
The Al clusters are also a kind of metallic hydroxide clusters. The simplified Al/O core of Al24 (Fig. 3c) is composed of the simplified trigonal [Al3(OH)O3] and octagonal [Al8(OH)8O4] (Fig. 3a and b) by sharing the sides of the triangles. As depicted in Fig. 3f, the further simplified metal skeleton belongs to one of the 13 Archimedean polyhedra, a truncated cube comprising 8 Al3 triangles on the truncated positions of the cube (Fig. 3d) and 6 Al8 octagons on the six faces (Fig. 3e) with the strong Al–Al interactions in the range of 2.850–2.978 Å. This Archimedean polyhedron is rare in the metal nanoclusters, compared with the truncated tetrahedron (Ag37),48 truncated octahedron (Co32),49 truncated icosahedron (Ln60),50 whereas some similar M24 coordination molecular cages with the organic ligands as the linkers have been reported.51,52
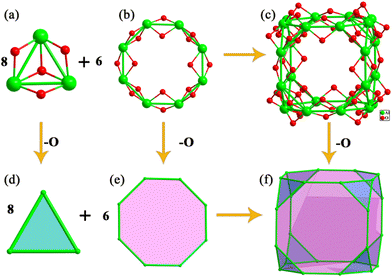 |
| Fig. 3 (a) and (b) [Al3(OH)O3] and [Al8(OH)8O4] building units. (c) The Al/O core of Al24. (d) and (e) The simplified Al3 triangle (blue) and Al8 octagon (purple). (f) The Archimedean polyhedron of Al24 metallic core (green: Al; red: O). | |
All Al3+ ions in the Al24 cluster adopt six-coordinated distorted octahedral geometry with six O atoms from 3 OH−, 1 NAP− and 2 CH3O−. The Al–O contacts and O–Al–O angles are in the range of 1.823–1.987 Å and 74.97–171.3°, respectively. All 12 NAP− ligands are situated at the sides of the octagons by adopting a μ2–η1:η1 connection mode (Fig. S1a†). The 32 OH− can be divided into two types, namely, 24 μ2-OH− is uniformly oriented to the centre of 6 octagon (Fig. S1b†), which are at the opposite positions of every NAP− ligand, whereas the residual 8 μ3-OH− are capped on the centre of the 8 trigonal Al3 building blocks (Fig. S1c†). For the CH3O− ligands, all are located on the sharing Al–Al sides of triangular Al3 and octagonal Al8 building blocks (Fig. S1d†).
Chemical stability and TG analyses
The stability of Al24 and Al12 was measured by immersing their single crystals in water and typical organic solvents. According to the comparison of the experimental and simulated powder X-ray diffraction (PXRD) patterns (Fig. S9†), Al24 can be kept stable in CH3OH, EtOH, CH3CN, and H2O at least for a day. For Al12, it displays very good stability in CH3OH, EtOH, CH3CN, H2O, and DMF for a month (Fig. S10†). The above findings demonstrate that Al24 and Al12 exhibit high chemical stability in some solvents and H2O. As shown in Fig. S8c and S8d,† the experimental IR spectra of Al12 and Al24 after immersing their single crystals in water and typical organic solvents were measured to further show their stabilities. Additionally, dry solid samples of Al24 and Al12 were used to study the thermal stability under the N2 environment from 30 to 800 °C (Fig. S11†). Two H2O and two CH3OH molecules were removed from Al24 during a slight weight loss of 2.33% up to 210 °C (calcd 2.35%). The mass loss from 210 to 285 °C is due to the departure of 24 coordinated methanol molecules (exp 17.98%, calcd 17.34%). As the temperature increased to 615 °C, the naphthalene rings of the organic NAP− ligands began to break down and the mass fraction of 39.50% was lost (calcd 39.00%). For Al12, the first weight loss below 185 °C was ascribed to the removal of four DMF, two H2O, and two CH3OH molecules (exp 11.16%, calcd 11.21%). Next, the weight loss of 47.86% from 260 to 440 °C is ascribed to the removal of the naphthalene rings from the NAP− ligands (calcd 47.51%). The third weight loss from 500 to 615 °C corresponds to the decomposition of coordinated methanol molecules.
Solution behaviours of Al24 and Al12 clusters
The high-resolution electrospray ionization mass spectrometry (ESI-MS) can be used to determine the fragment composition and charge state of the metal clusters.21,53–60 The negative-mode ESI-MS of Al24 was investigated by dissolving the crystals in CH3OH–CH2Cl2 to analyse its solution stability. As shown in Fig. 4, there are two sets of peaks in the mass-to-charge (m/z) ratio ranges of 2000–2100 (1) with −2 charge and 4000–4200 (2) with −1 charge, respectively. For 1 group, the distances between two neighbouring peaks are disparate with 6.51, 7.51, 6.55, 7.47, 7.00, and 7.51 having no uniform variation trend for the seven species (1a–1g). Their compositions, at the positions: m/z = 2045.78, 2052.20, 2059.79, 2066.22, 2073.79, 2080.79, and 2088.20, can be identified by matching the experimental and simulated isotopic distributions with the corresponding formulas, [Al24(OH)34(CH3O)26(NAP)11(H2O)4Cl3]2−, [Al24(OH)34(CH3O)23(NAP)11(H2O)4Cl6]2−, [Al24(OH)32(CH3O)28(NAP)11(H2O)4Cl3]2−, [Al24(OH)32(CH3O)25(NAP)11(H2O)4Cl6]2−, [Al24(OH)31(CH3O)30(NAP)11(H2O)1Cl4H2]2−, [Al24(OH)31(CH3O)31(NAP)11Cl4H3]2−, [Al24(OH)34(CH3O)23(NAP)11(H2O)6Cl7H1]2− (Table S1†). This set of signals indicates that the metal skeleton of Al24 cluster is stable in CH3OH–CH2Cl2, whereas the protection groups of OH−, CH3O−, and NAP− exist in the coordination–disassociation equilibrium. The most dominant peak (1d) with the composition of [Al24(OH)32(CH3O)25(NAP)11(H2O)4Cl6]2− at m/z = 2066.22 is approximate to the parent cluster except for one NAP− replaced with one CH3O−. For the 2 groups, 2a–2c has similar distances of 14.02 corresponding to [+CH3O−-H2O + H+] with the formulas, [Al22(OH)23(CH3O)25(NAP)12(H2O)2Cl7]−, [Al22(OH)23(CH3O)26(NAP)12(H2O)1Cl7H1]−, [Al22(OH)23(CH3O)27(NAP)12Cl7H2]− at m/z = 4097.45, 4111.47 and 4125.48, suggesting the presence of the Al22 fragments in the CH3OH–CH2Cl2 of Al24.
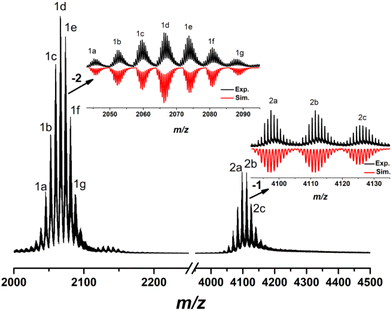 |
| Fig. 4 The negative-ion ESI-MS of Al24 in CH3OH/CH2Cl2. Inset: the experimental isotropic pattern (block) and simulated (red) data. | |
The positive-ion ESI-MS data of Al24 was also monitored at m/z = 500–6000 (Fig. 5) by dissolving its crystals in CH3OH–CH2Cl2. There are four groups (1a–1f, 2a–2g, 3a–3g, and 4a–4e) of experimental peaks at m/z = 1950–2250 with +2 charge (see inset in Fig. S2†). By matching the experimental and simulated isotopic distributions, all formulas of fragments are listed in Table S2.† The formulas of 2c–2f ([Al24(OH)32(CH3O)24(NAP)12Cl2(H2O)x(CH3OH)3−x]2+, x = 3, 2, 1, 0), 3a–3f ([Al24(OH)32(CH3O)24(NAP)12Cl2(H2O)5(CH3CN)1(CH3OH)1]2+ (3a); [Al24(OH)32(CH3O)24(NAP)12Cl2(H2O)3(CH3CN)3]2+ (3b); [Al24(OH)32(CH3O)24(NAP)12Cl2(H2O)2(CH3CN)3(CH3OH)1]2+ (3c); [Al24(OH)32(CH3O)24(NAP)12Cl3(H2O)4(CH3OH)3H1]2+ (3d); [Al24(OH)32(CH3O)24(NAP)12Cl4(H2O)1(CH3OH)4H2]2+ (3e); [Al24(OH)32(CH3O)24(NAP)12Cl4(CH3OH)5H2]2+ (3f)) and 4a–4e ([Al24(OH)32(CH3O)24(NAP)12Cl2(H2O)x(CH3CN)4(CH3OH)6−x]2+, x = 3, 2, 1, 0) are consistent with the molecular formulas of Al24 ([Al24(OH)32(CH3O)22(CH3OH)2(NAP)12]·6Cl·2H2O·2CH3OH) except for the different number of Cl− and solvent molecules, indicating the stability of total Al24 structure in CH3OH and CH2Cl2 again. Meanwhile, 1b–1f ([Al24(OH)32(CH3O)26(NAP)10Cl5(H2O)x(CH3OH)4−xH3]2+, x = 4, 3, 2, 1, 0), 2a ([Al24(OH)32(CH3O)26(NAP)10Cl5(H2O)1(CH3CN)2(CH3OH)3H3]2+), 2b ([Al24(OH)32(CH3O)26(NAP)10Cl5(CH3CN)2(CH3OH)4H3]2+) and 3g ([Al24(OH)32(CH3O)25(NAP)11Cl4(H2O)3(CH3CN)4(CH3OH)3H2]2+) also show the ligand exchange between CH3O− and NAP−.
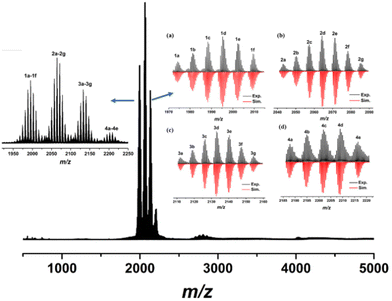 |
| Fig. 5 The positive-ion ESI-MS of Al24 in CH3OH/CH2Cl2. Left inset: the experimental isotropic pattern. Right inset: the experimental isotropic pattern (block) and simulated (red) data. | |
Similarly, the positive-mode ESI-MS obtained by dissolving the crystals of Al12 in CH3OH–CH2Cl2 was also monitored at m/z = 1000–6000 (Fig. 6a). Three groups of experimental peaks at m/z = 1950–2020 (1a–1j, +2), 2100–2160 (2a–2f, +2) and 2290–2330 (3a–3f, +2) can be clearly assigned by matching the experimental and simulated isotopic distributions (Fig. 6b–d). Two neighbouring peaks of the three groups of experimental data have the same distances with 14.02 corresponding to [+CH3O−-H2O + H+] and all formulas of fragments are listed in Table S3.† Regrettably, the molecular ion signal was not detected. 1a–1e assigned to [Al12(CH3O)17(NAP)17(H2O)8]2+ (1a); [Al12(CH3O)16(NAP)18(H2O)1]2+ (1b); [Al12(CH3O)17(NAP)18H1]2+ (1c); [Al12(CH3O)20(NAP)17(H2O)5H3]2+ (1d); [Al12(CH3O)21(NAP)17(H2O)4H4]2+ (1e), and 2c–2f assigned to [Al12(CH3O)14(NAP)20(H2O)5]2+ (2c); [Al12(CH3O)15(NAP)20(H2O)4H1]2+ (2d); [Al12(CH3O)16(NAP)20(H2O)3H2]2+ (2e); [Al12(CH3O)17(NAP)20(H2O)2H3]2+ (2f) coincide with the metallic core of Al12 whereas the numbers of CH3O− and NAP− are different with the molecular formulas, indicating the occurrence of ligand exchange between CH3O−, NAP− and H2O. 1f–1i assigned to [Al11(CH3O)12(NAP)19(H2O)3]2+, [Al11(CH3O)13(NAP)19(H2O)2H1]2+, [Al11(CH3O)14(NAP)19(H2O)1H2]2+, and [Al11(CH3O)15(NAP)19H3]2+ lost a metal ion compared with Al12 and 1j assigned to [Al10(CH3O)8(NAP)20(H2O)5]2+ has two fewer metal ions than Al12. Interestingly, the fragments of 2a–2b with the corresponding attribution of [Al13(CH3O)20(NAP)19(H2O)1H2]2+ and [Al13(CH3O)21(NAP)19H3]2+, and 3a–3f with the corresponding attribution of [Al14(CH3O)20(NAP)20(H2O)9]2+, [Al14(CH3O)21(NAP)20(H2O)8H1]2+, [Al14(CH3O)22(NAP)20(H2O)7H2]2+, [Al14(CH3O)23(NAP)20(H2O)6H3]2+, [Al14(CH3O)24(NAP)20(H2O)5H4]2+, [Al14(CH3O)25(NAP)20(H2O)4H5]2+ display the increase of a and two Al3+ ions for Al12. The above analysis result indicates that the metal skeleton of the Al12 cluster can be partly stable in CH3OH and CH2Cl2, while some fragments with adjacent nuclear numbers, e.g., Al10, Al11, Al13, Al14 exist in CH3OH and CH2Cl2. Alternatively, the number of CH3O− is less than the theoretical 24, whereas that of NAP− is more. Thus, we surmise that NAP− firstly coordinated with Al3+ ions in the assembly process of Al12, and was later replaced by CH3O−. Besides, the negative-ion ESI-MS data of Al12 obtained by dissolving its crystals in CH3OH–CH2Cl2 was monitored at m/z = 500–6000 (Fig. S2†). Only a small fragment peak at m/z = 863.15 was detected at m/z = 500–6000 and there are no more pieces of information.
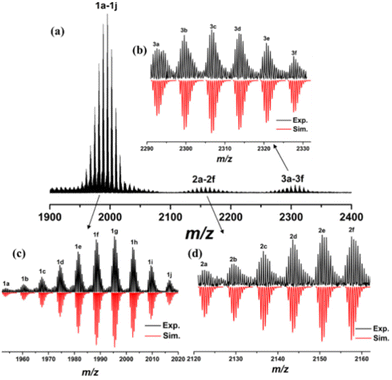 |
| Fig. 6 (a) The positive-ion ESI-MS of Al12 in CH3OH/CH2Cl2. The experimental isotropic pattern (block) and simulated (red) data for 1 group (c), 2 group (d), and 3 group (b). | |
UV–Vis spectra and photocurrent measurements of Al24 and Al12
The solid-state ultraviolet-visible absorption spectra (UV-Vis) of HNAP, Al24, and Al12 were recorded at room temperature. As shown in Fig. S3a and S3b,† the HNAP ligand exhibits absorption at 210 nm, whereas Al24 and Al12 have a similar broad absorption band at 250–400 nm. The red-shifted phenomenon may be caused by Al-perturbed π → π* transition of the ligand.61 The band gaps (Eg) of Al24 and Al12 are 3.48 and 3.44 eV calculated based on the Kubelka–Munk function, respectively (Fig. S4†),62 which are much smaller than 9 eV of Al2O3.63 This reveals that the aggregation of aluminum ions into aluminum-oxo clusters affects the band gap structure, including the widening of the absorption edge and the narrowing of the band gap.
The photocurrent responses of Al24 and Al12 under LED light were further examined by the typical three-electrode system. The working electrode was indium tin oxide (ITO) glass, the auxiliary electrode was platinum wire, and the reference electrode was Ag/AgCl, maintaining the bias voltage of 0.6 V in the meantime. As shown in Fig. 7, upon on–off cycling exposure with LED light (λ = 365 nm and 420 nm; 10 s intervals; 50 W), obvious photocurrent reactions were observed, and the photocurrent densities exhibit a promptly rising or fast falling under light irradiation or no irradiation conditions. This indicates that Al12 and Al24 both have fast response speeds to LED light. No matter whether the irradiations wavelengths are at 420 nm or 365 nm, Al12 generates higher photocurrent values than these of Al24, which demonstrates that more efficient generation and separation of photoinduced electron/hole pairs were observed in ITO electrodes of Al12 under the LED light. This could be explained on the basis of the lower band gap of Al12 at 3.44 eV.64 The above experimental results exhibiting Al12 and Al24 are a kind of potential photoelectric materials.
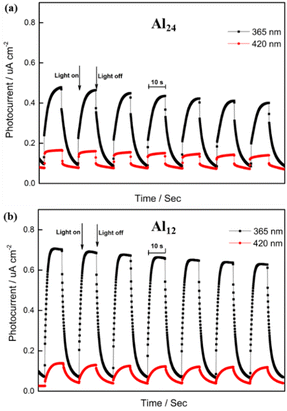 |
| Fig. 7 Transient photocurrent responses of Al24 (a) and Al12 (b). | |
Luminescence properties of Al24 and Al12
At room temperature, the solid-state luminescence properties of HNAP, Al24, and Al12 were observed (Fig. S5†). The maximum emission of HNAP was at 372 nm under a maximum excitation wavelength of 319 nm (Fig. S5a and 5b†), which originated from the intramolecular π–π* transition of the HNAP organic ligand. As illustrated in Fig. S5c and 5d,† the Al24 cluster exhibits the ligand-centered blue fluorescence with emission at 374 nm under 319 nm excitation. A similar blue fluorescence from ligand-centered emission is also observed in Al12 with the maximum emission wavelength at 389 nm under 352 nm excitation (Fig. S5e and 5f†).
To determine their potential applications as optical thermometers, the operating range for the temperature-dependent luminescence spectra of Al24 and Al12 was from 300 to 120 K. The temperature was changed from 300 K to 120 K, but the emission location of the Al24 cluster remained fixed at x = 0.15 and y = 0.06 in the CIE space (Fig. S6a and Table S5†) in the blue-light region (Fig. 8a). Furthermore, the emission position of Al12 is slightly blue-shifted from 394.5 nm to 387.5 nm, as the temperature drops from 300 K to 120 K (Fig. 8b) while the CIE coordinates of the emission positions change from x = 0.16, y = 0.05 to x = 0.17, y = 0.07 (Fig. S6b and Table S5†). The probable reason is the restricted rotation of ligands in Al12 at lower temperatures. In addition, the emission intensity of Al24 and Al12 gradually increased with decreasing temperature on account of the reduction of non-radiative decay.
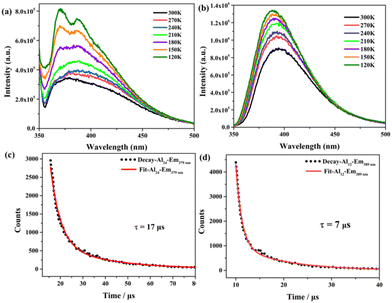 |
| Fig. 8 The temperature-dependent luminescence spectra of Al24 (a) and Al12 (b) from 300 K to 120 K. Luminescence lifetimes of Al24 (c, λem = 379 nm) and Al12 (d, λem = 389 nm) at room temperature. | |
The time-resolved decay curves of the Al24 and Al12 clusters were recorded at room temperature, and their fitting results show the double exponential functions. The lifetimes of Al24 and Al12 are 17 μs (λem = 379 nm; τ1 = 4.44, 37%; τ2 = 24.4, 63%; Fig. 8c) and 7 μs (λem = 389 nm; τ1 = 1.26, 35%; τ2 = 10.26, 65%; Fig. 8d). The absolute solid-state quantum yields of the Al24 and Al12 are 25.11% and 18.42%, respectively. The different luminescence behaviours of Al24 and Al12 can be attributed to the different ligand environments in the crystalline structures, generating the different constraints on the rotation of the NAP− ligands.
Conclusions
In summary, we synthesized an Al12 molecular ring and a rare Al24 metallocage based on the NAP− ligand obtained by modulating the solvent in a simple one-pot solvothermal reaction. The Al12 is a dodecagonal molecular ring and the Al24 metallocage can be seen as a truncated cube composed of eight Al3 triangles and six Al8 octagons formed by sharing vertical Al3+ ions, realizing the complete change of Al-oxo skeletons. Interestingly, the Al12 and Al24 clusters exhibited the photocurrent-generating capacity and luminescent behaviour, indicating that the Al clusters are potentially photocatalytic and luminescent materials.
Conflicts of interest
There are no conflicts to declare.
Acknowledgements
This work was supported by the National Natural Science Foundation of China (no. 22101148) and the Natural Science Foundation of Shandong Province (no. ZR2021QB008).
References
- S. Schein and J. M. Gayed, Fourth class of convex equilateral polyhedron with polyhedral symmetry related to fullerenes and viruses, Proc. Natl. Acad. Sci. U. S. A., 2014, 111, 2920–2925 CrossRef CAS PubMed.
- C. Stump, AI aids intuition in mathematical discovery, Nature, 2021, 600, 44–45 CrossRef CAS PubMed.
- I. Severcan, C. Geary, A. Chworos, N. Voss, E. Jacovetty and L. Jaeger, A polyhedron made of tRNAs, Nat. Chem., 2010, 2, 772–779 CrossRef CAS PubMed.
- R. Twarock and A. Luque, Structural puzzles in virology solved with an overarching icosahedral design principle, Nat. Commun., 2019, 10, 4414 CrossRef PubMed.
- R. Iinuma, Y. Ke, R. Jungmann, T. Schlichthaerle, J. B. Woehrstein and P. Yin, Polyhedra Self-Assembled from DNA Tripods and Characterized with 3D DNA-PAINT, Science, 2014, 344, 65–69 CrossRef CAS PubMed.
- J.-P. Lang, Q.-F. Xu, Z.-N. Chen and B. F. Abrahams, Assembly of a Supramolecular Cube, [(Cp*WS3Cu3)8Cl8(CN)12Li4] from a Preformed Incomplete Cubane-like Compound [PPh4][Cp*WS3(CuCN)3], J. Am. Chem. Soc., 2003, 125, 12682–12683 CrossRef CAS PubMed.
- S. R. Seidel and P. J. Stang, High-Symmetry Coordination Cages via Self-Assembly, Acc. Chem. Res., 2002, 35, 972–983 CrossRef CAS PubMed.
- A. V. Virovets, E. Peresypkina and M. Scheer, Structural Chemistry of Giant Metal Based Supramolecules, Chem. Rev., 2021, 121, 14485–14554 CrossRef CAS PubMed.
- D. Fujita, Y. Ueda, S. Sato, N. Mizuno, T. Kumasaka and M. Fujita, Self-assembly of tetravalent Goldberg polyhedra from 144 small components, Nature, 2016, 540, 563–566 CrossRef CAS PubMed.
- X. Z. Li, C. B. Tian and Q. F. Sun, Coordination-Directed Self-Assembly of Functional Polynuclear Lanthanide Supramolecular Architectures, Chem. Rev., 2022, 122, 6374–6458 CrossRef CAS PubMed.
- S. J. Bao, Z. M. Xu, T. C. Yu, Y. L. Song, H. Wang, Z. Niu, X. Li, B. F. Abrahams, P. Braunstein and J. P. Lang, Flexible Vertex Engineers the Controlled Assembly of Distorted Supramolecular Tetrahedral and Octahedral Cages, Research, 2022, 2022, 9819343 CrossRef CAS PubMed.
- S. J. Bao, Z. M. Xu, Y. Ju, Y. L. Song, H. Wang, Z. Niu, X. Li, P. Braunstein and J. P. Lang, The Covalent and Coordination Co-Driven Assembly of Supramolecular Octahedral Cages with Controllable Degree of Distortion, J. Am. Chem. Soc., 2020, 142, 13356–13361 CrossRef CAS PubMed.
- Z. H. Wei, C. Y. Ni, H. X. Li, Z. G. Ren, Z. R. Sun and J. P. Lang, [PyH][{TpMo(μ3-S)4Cu3}4(μ12-I)]: a unique tetracubane cluster derived from the S-S bond cleavage and the iodide template effects and its enhanced NLO performances, Chem. Commun., 2013, 49, 4836–4838 RSC.
- L. Yang, X.-Y. Wang, X.-Y. Tang, M.-Y. Wang, C.-Y. Ni, H. Yu, Y.-L. Song, B. F. Abrahams and J.-P. Lang, Temperature-dependent chloride-mediated access to atom-precise silver thiolate nanoclusters, Sci. China: Chem., 2022, 65, 1094–1099 CrossRef CAS.
- H. W. Kroto, J. R. Heath, S. C. O'Brien, R. F. Curl and R. E. Smalley, C60: Buckminsterfullerene, Nature, 1985, 318, 162–163 CrossRef CAS.
- J. Spandl, I. Brüdgam and H. Hartl, Solvothermal Synthesis of a 24-Nuclear, Cube-Shaped Squarato-oxovanadium(IV) Framework: [N(nBu)4]8[V24O24(C4O4)12(OCH3)32], Angew. Chem., Int. Ed., 2001, 40, 4018–4020 CrossRef CAS PubMed.
- Y. Bi, S. Du and W. Liao, Thiacalixarene-based nanoscale polyhedral coordination cages, Coord. Chem. Rev., 2014, 276, 61–72 CrossRef CAS.
- S. P. Argent, A. Greenaway, M. d. C. Gimenez-Lopez, W. Lewis, H. Nowell, A. N. Khlobystov, A. J. Blake, N. R. Champness and M. Schroder, High-nuclearity metal-organic nanospheres: a Cd66 ball, J. Am. Chem. Soc., 2012, 134, 55–58 CrossRef CAS PubMed.
- J. Lin, N. Li, S. Yang, M. Jia, J. Liu, X. M. Li, L. An, Q. Tian, L. Z. Dong and Y. Q. Lan, Self-Assembly of Giant Mo240 Hollow Opening Dodecahedra, J. Am. Chem. Soc., 2020, 142, 13982–13988 CrossRef CAS PubMed.
- N. T. Tran, D. R. Powell and L. F. Dahl, Nanosized Pd145(CO)x(PEt3)30 Containing a Capped Three-Shell 145-Atom Metal-Core Geometry of Pseudo Icosahedral Symmetry, Angew. Chem., Int. Ed., 2000, 39, 4121–4125 CrossRef CAS PubMed.
- Z. Wang, H. F. Su, Y. Z. Tan, S. Schein, S. C. Lin, W. Liu, S. A. Wang, W. G. Wang, C. H. Tung, D. Sun and L. S. Zheng, Assembly of silver Trigons into a buckyball-like Ag180 nanocage, Proc. Natl. Acad. Sci. U. S. A., 2017, 114, 12132–12137 CrossRef CAS PubMed.
- J. B. Peng, X. J. Kong, Q. C. Zhang, M. Orendac, J. Prokleska, Y. P. Ren, L. S. Long, Z. Zheng and L. S. Zheng, Beauty, symmetry, and magnetocaloric effect-four-shell keplerates with 104 lanthanide atoms, J. Am. Chem. Soc., 2014, 136, 17938–17941 CrossRef CAS PubMed.
- S. L. Heath, P. A. Jordan, I. D. Johnson, G. R. Moore, A. K. Powell and M. Helliwell, Comparative X-ray and 27Al NMR spectroscopic studies of the speciation of aluminum in aqueous systems: Al(III) complexes of N(CH2CO2H)2(CH2CH2OH), J. Inorg. Biochem., 1995, 59, 785–794 CrossRef CAS.
- S. Abeysinghe, D. K. Unruh and T. Z. Forbes, Crystallization of Keggin-Type Polyaluminum Species by Supramolecular Interactions with Disulfonate Anions, Cryst. Growth Des., 2012, 12, 2044–2051 CrossRef CAS.
- W. Wang, K. M. Wentz, S. E. Hayes, D. W. Johnson and D. A. Keszler, Synthesis of the hydroxide cluster [Al13(μ3-OH)6(μ-OH)18(H2O)24]15+ from an aqueous solution, Inorg. Chem., 2011, 50, 4683–4685 CrossRef CAS PubMed.
- B. L. Fulton, C. K. Perkins, R. H. Mansergh, M. A. Jenkins, V. Gouliouk, M. N. Jackson, J. C. Ramos, N. M. Rogovoy, M. T. Gutierrez-Higgins, S. W. Boettcher, J. F. Conley, D. A. Keszler, J. E. Hutchison and D. W. Johnson, Minerals to Materials: Bulk Synthesis of Aqueous Aluminum Clusters and Their Use as Precursors for Metal Oxide Thin Films, Chem. Mater., 2017, 29, 7760–7765 CrossRef CAS.
- S. E. Smart, J. Vaughn, I. Pappas and L. Pan, Controlled step-wise isomerization of the Keggin-type Al13 and determination of the γ-Al13 structure, Chem. Commun., 2013, 49, 11352–11354 RSC.
- L. Allouche, C. Gérardin, T. Loiseau, G. Férey and F. Taulelle, Al30: A Giant Aluminum Polycation, Angew. Chem., Int. Ed., 2000, 39, 511–514 CrossRef CAS PubMed.
- K. S. Lokare, N. Frank, B. Braun-Cula, I. Goikoetxea, J. Sauer and C. Limberg, Trapping Aluminum Hydroxide Clusters with Trisilanols during Speciation in Aluminum(III)-Water Systems: Reproducible, Large Scale Access to Molecular Aluminate Models, Angew. Chem., Int. Ed., 2016, 55, 12325–12329 CrossRef CAS PubMed.
- W. Schmitt, E. Baissa, A. Mandel, C. E. Anson and A. K. Powell, [Al15(μ3-O)4(μ3-OH)6(μ-OH)14(hpdta)4]3−—A New Al15 Aggregate Which Forms a Supramolecular Zeotype, Angew. Chem., Int. Ed., 2001, 40, 3577–3581 CrossRef PubMed.
- E. A. Cochran, K. N. Woods, D. W. Johnson, C. J. Page and S. W. Boettcher, Unique chemistries of metal-nitrate precursors to form metal-oxide thin films from solution: materials for electronic and energy applications, J. Mater. Chem. A, 2019, 7, 24124–24149 RSC.
- Y. J. Liu, Q. H. Li, D. J. Li, X. Z. Zhang, W. H. Fang and J. Zhang, Designable Al32-Oxo Clusters with Hydrotalcite-like Structures: Snapshots of Boundary Hydrolysis and Optical Limiting, Angew. Chem., Int. Ed., 2021, 60, 4849–4854 CrossRef CAS PubMed.
- Y. Li, C. Zheng, S. T. Wang, Y. J. Liu, W. H. Fang and J. Zhang, Record Aluminum Molecular Rings for Optical Limiting and Nonlinear Optics, Angew. Chem., Int. Ed., 2022, 61, e202116563 CAS.
- S. T. Wang, Y. J. Liu, C. C. Feng, W. H. Fang and J. Zhang, The largest aluminum molecular rings: Phenol-thermal synthesis, photoluminescence, and optical limiting, Aggregate, 2022, e264 Search PubMed.
- Y. J. Liu, Y. F. Sun, S. H. Shen, S. T. Wang, Z. H. Liu, W. H. Fang, D. S. Wright and J. Zhang, Water-stable porous Al24 Archimedean solids for removal of trace iodine, Nat. Commun., 2022, 13, 6632 CrossRef CAS PubMed.
- L. Geng, C. H. Liu, S. T. Wang, W. H. Fang and J. Zhang, Designable Aluminum Molecular Rings: Ring Expansion and Ligand Functionalization, Angew. Chem., Int. Ed., 2020, 59, 16735–16740 CrossRef CAS PubMed.
- X. Z. Zhang, X. F. Wang, W. H. Fang and J. Zhang, Synthesis, Structures, and Fluorescence Properties of Dimeric Aluminum Oxo Clusters, Inorg. Chem., 2021, 60, 7089–7093 CrossRef CAS PubMed.
- O. V. B. Dolomanov, L. J. Bourhis, R. J. Gildea, J. A. K. Howard and H. Puschmann, OLEX2: a complete structure solution, refinement and analysis program, J. Appl. Crystallogr., 2009, 42, 339–341 CrossRef CAS.
- G. M. Sheldrick, A short history of SHELX, Acta Crystallogr., Sect. A: Found. Crystallogr., 2008, 64, 112–122 CrossRef CAS PubMed.
- A. L. Spek, Structure validation in chemical crystallography, Acta Crystallogr., Sect. D: Biol. Crystallogr., 2009, 65, 148 CrossRef CAS PubMed.
- X. Y. Li, H. F. Su, Q. W. Li, R. Feng, H. Y. Bai, H. Y. Chen, J. Xu and X. H. Bu, A Giant Dy76 Cluster: A Fused Bi-Nanopillar Structural Model for Lanthanide Clusters, Angew. Chem., Int. Ed., 2019, 58, 10184–10188 CrossRef CAS PubMed.
- M. Wu, F. Jiang, D. Yuan, J. Pang, J. Qian, S. A. Al-Thabaiti and M. Hong, Polymeric double-anion templated Er48 nanotubes, Chem. Commun., 2014, 50, 1113–1115 RSC.
- F.-S. Guo, Y.-C. Chen, L.-L. Mao, W.-Q. Lin, J.-D. Leng, R. Tarasenko, M. Orendáč, J. Prokleška, V. Sechovský and M.-L. Tong, Anion-Templated Assembly and Magnetocaloric Properties of a Nanoscale {Gd38} Cage versus a {Gd48} Barrel, Chem. – Eur. J., 2013, 19, 14876–14885 CrossRef CAS PubMed.
- X.-Y. Li, Y. Jing, J. Zheng, H. Ding, Q. Li, M.-H. Yu and X.-H. Bu, Two Luminescent High-Nuclearity Lanthanide Clusters Ln48 (Ln = Eu and Tb) with a Nanopillar Structure, Cryst. Growth Des., 2020, 20, 5294–5301 CrossRef CAS.
- R. Wang, Z. Zheng, T. Jin and R. J. Staples, Coordination Chemistry of Lanthanides at “High” pH: Synthesis and Structure of the Pentadecanuclear Complex of Europium(III) with Tyrosine, Angew. Chem., 1999, 111, 1929–1932 CrossRef.
- W. Huang, Z. Zhang, Y. Wu, W. Chen, D. A. Rotsch, L. Messerle and Z. Zheng, A systematic study of halide-template effects in the assembly of lanthanide hydroxide cluster
complexes with histidine, Inorg. Chem. Front., 2021, 8, 26–34 RSC.
- D. T. Thielemann, A. T. Wagner, Y. Lan, P. Ona-Burgos, I. Fernandez, E. S. Rosch, D. K. Kolmel, A. K. Powell, S. Brase and P. W. Roesky, Peptoid-ligated pentadecanuclear yttrium and dysprosium hydroxy clusters, Chem. – Eur. J., 2015, 21, 2813–2820 CrossRef CAS PubMed.
- X. Y. Li, H. F. Su, K. Yu, Y. Z. Tan, X. P. Wang, Y. Q. Zhao, D. Sun and L. S. Zheng, A platonic solid templating Archimedean solid: an unprecedented nanometre-sized Ag37 cluster, Nanoscale, 2015, 7, 8284–8288 RSC.
- Y. Bi, X.-T. Wang, W. Liao, X. Wang, X. Wang, H. Zhang and S. Gao, A {Co32} Nanosphere Supported by p-tert-Butylthiacalix[4]arene, J. Am. Chem. Soc., 2009, 131, 11650–11651 CrossRef CAS PubMed.
- X.-J. Kong, Y. Wu, L.-S. Long, L.-S. Zheng and Z. Zheng, A Chiral 60-Metal Sodalite Cage Featuring 24 Vertex-Sharing [Er4(μ3-OH)4] Cubanes, J. Am. Chem. Soc., 2009, 131, 6918–6919 CrossRef CAS PubMed.
- Y. Domoto, M. Abe and M. Fujita, A Highly Entangled (M3L2)8 Truncated Cube from the Anion-Controlled Oligomerization of a pi-Coordinated M3L2 Subunit, J. Am. Chem. Soc., 2021, 143, 8578–8582 CrossRef CAS PubMed.
- K. Su, M. Wu, D. Yuan and M. Hong, Interconvertible vanadium-seamed hexameric pyrogallol[4]arene nanocapsules, Nat. Commun., 2018, 9, 4941 CrossRef PubMed.
- L. Tang, A. Ma, C. Zhang, X. Liu, R. Jin and S. Wang, Total Structure of Bimetallic Core-Shell [Au42Cd40(SR)52]2− Nanocluster and Its Implications, Angew. Chem., Int. Ed., 2021, 60, 17969–17973 CrossRef CAS PubMed.
- S. Lee, M. S. Bootharaju, G. Deng, S. Malola, W. Baek, H. Hakkinen, N. Zheng and T. Hyeon, [Cu32(PET)24H8Cl2](PPh4)2: A Copper Hydride Nanocluster with a Bisquare Antiprismatic Core, J. Am. Chem. Soc., 2020, 142, 13974–13981 CrossRef CAS PubMed.
- J. J. Li, Z. J. Guan, Z. Lei, F. Hu and Q. M. Wang, Same Magic Number but Different Arrangement: Alkynyl-Protected Au25 with D3 Symmetry, Angew. Chem., Int. Ed., 2019, 58, 1083–1087 CrossRef CAS PubMed.
- Y.-K. Deng, H.-F. Su, J.-H. Xu, W.-G. Wang, M. Kurmoo, S.-C. Lin, Y.-Z. Tan, J. Jia, D. Sun and L.-S. Zheng, Hierarchical Assembly of a {MnII15MnIII4} Brucite Disc: Step-by-Step Formation and Ferrimagnetism, J. Am. Chem. Soc., 2016, 138, 1328–1334 CrossRef CAS PubMed.
- X. Y. Li, H. F. Su, M. Kurmoo, C. H. Tung, D. Sun and L. S. Zheng, Structure, solution assembly, and electroconductivity of nanosized argento-organic-cluster/framework templated by chromate, Nanoscale, 2017, 9, 5305–5314 RSC.
- X. Y. Li, H. F. Su, Q. W. Li, R. Feng, H. Y. Bai, H. Y. Chen, J. Xu and X. H. Bu, A Giant Dy76 Cluster: A Fused Bi-Nanopillar Structural Model for Lanthanide Clusters, Angew. Chem., Int. Ed., 2019, 58, 10184–10188 CrossRef CAS PubMed.
- H. Zheng, M.-H. Du, S.-C. Lin, Z.-C. Tang, X.-J. Kong, L.-S. Long and L.-S. Zheng, Assembly of a Wheel-Like Eu24Ti8 Cluster under the Guidance of High-Resolution Electrospray Ionization Mass Spectrometry, Angew. Chem., Int. Ed., 2018, 57, 10976–10979 CrossRef CAS PubMed.
- Y.-Y. Zhang, D.-S. Zhang, T. Li, M. Kurmoo and M.-H. Zeng, In Situ Metal-Assisted Ligand Modification Induces
Mn4 Cluster-to-Cluster Transformation: A Crystallography, Mass Spectrometry, and DFT Study, Chem. – Eur. J., 2020, 26, 721–728 CrossRef CAS PubMed.
- J. H. Weber, Complexes of pyrrole-derivative ligands. I., Planar nickel(II) complexes with tetradentate ligands, Inorg. Chem., 1967, 6, 258–262 CrossRef CAS.
- Y. Mu, D. Wang, X.-D. Meng, J. Pan, S.-D. Han and Z.-Z. Xue, Construction of Iodoargentates with Diverse Architectures: Template Syntheses, Structures, and Photocatalytic Properties, Cryst. Growth Des., 2020, 20, 1130–1138 CrossRef CAS.
- K. Matsunaga, T. Mizoguchi, A. Nakamura, T. Yamamoto and Y. Ikuhara, Formation of titanium-solute clusters in alumina: A first-principles study, Appl. Phys. Lett., 2004, 84, 4795–4797 CrossRef CAS.
- A. Kongkanand, K. Tvrdy, K. Takechi, M. Kuno and P. V. Kamat, Quantum Dot Solar Cells. Tuning Photoresponse through Size and Shape Control of CdSe–TiO2 Architecture, J. Am. Chem. Soc., 2008, 130, 4007–4015 CrossRef CAS PubMed.
|
This journal is © the Partner Organisations 2023 |
Click here to see how this site uses Cookies. View our privacy policy here.