DOI:
10.1039/D2MA01105K
(Review Article)
Mater. Adv., 2023,
4, 1831-1849
Green synthesis of silver nanoparticles: methods, biological applications, delivery and toxicity
Received
31st December 2022
, Accepted 9th March 2023
First published on 14th March 2023
Abstract
The advent of nanotechnology profoundly transformed the pharmaceutical sciences and greatly enhanced the diagnostics and treatment of various diseases that threaten human life. Several metallic nanoparticles are extensively used as nanomedicines due to their potential therapeutic applications. Among them, silver nanoparticles are remarkable due to their unique chemical and physical properties. This review discusses types of nanoparticles, and green synthesis methods along with their reduction mechanisms, involving economically viable reducing materials like algae, seaweeds and flowers. Apart from environment-friendly methods, several biological activities such as wound healing, antibacterial, antifungal, anti-tumour, anti-viral, etc., are described in detail. Consequently, we have focused on how silver nanoparticles enhance targeted drug delivery and the mechanism of drug release along with their toxic effects.
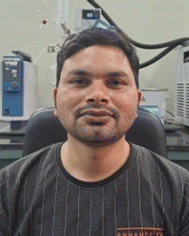
Vidyasagar
| Vidyasagar is a PhD student at Banaras Hindu University, India. He is pursuing his PhD in Chemistry under the supervision of Dr Meenakshi Singh. The area of his research is modern organic synthesis and incorporation of nanoparticles in the medical field. He completed his bachelor's degree at Dr Ram Manohar Lohia Avadh University, Faizabad and his master's degree in Chemistry at University of Lucknow. He is an awardee of a CSIR-JRF fellowship. |
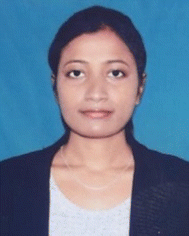
Ritu Raj Patel
| Ritu Raj Patel is a PhD student at Banaras Hindu University, India. She is pursuing her PhD in Microbiology under the supervision of Dr Meenakshi Singh. Her research interest is focused on the effect of green synthesized nanoparticles against Mycobacterium species, extremophile bacteria. She completed her Master of Science in Microbiology at Veer Bahadur Singh Purvanchal University, Jaunpur and Bachelor of Science in Medical Microbiology at ChhatrapatiShahu Ji Maharaj University, Kanpur. |
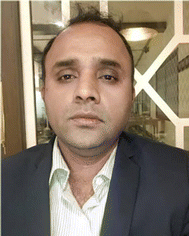
Sudhir Kumar Singh
| Dr Sudhir Kumar Singh is currently a Malaviya Postdoctoral fellow in the Department of Microbiology, Institute of Medical Sciences Banaras Hindu University, India. He earned his PhD in Protein biochemistry from the Jawaharlal Nehru University, New Delhi, India in 2015. Afterwards he pursued Post-Doctoral research at the Ben-Gurion University (BGU), Israel, for 4 years where he focused on the structural and functional characterization of kinesin proteins involved in eukaryote cell division. Currently, he is working on the role of antimicrobial proteins against multidrug resistant pathogenic bacteria and the development of protein based biosensors. He has 15 years of research experience with 20 peer-reviewed international publications and 2 Book chapters. |
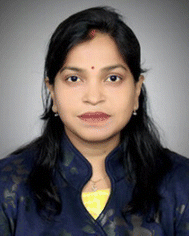
Meenakshi Singh
| Dr Meenakshi Singh is currently working as an Assistant Professor in the Department of Medicinal Chemistry, Faculty of Ayurveda, Institute of Medical Sciences, Banaras Hindu University, Varanasi, India. She earned her PhD Degree in Pharmaceutical Chemistry from Indian Institute of Technology, Banaras Hindu University in 2016. Afterwards she pursued Post-Doctoral research at the Ben-Gurion University (BGU), Israel, for more than 3 years where she focused on developing novel drug inhibitors that showed promising activity against DNA replisome machinery of Mycobacterium tuberculosis. Dr Meenakshi has 12 years of research experience with 35 peer-reviewed international publications, and 3 Book chapters. Currently, she is working on Herbal drug development, the phytochemical investigation of Natural products and the development of synthetic small molecules against cancerous cells & multi-drug resistant bacterial pathogens using novel avenues such as nanoparticles and nano delivery for their efficient abolition. |
1. Introduction
The enormous surge in multi-drug resistant pathogens has emerged as a vital challenge before scientists to develop effective therapeutics. Particles with a size range of 1–100 nm are considered nanoparticles (NPs), and silver nanoparticles (AgNPs) have been highly effective antimicrobial agents.1,2 The large surface area of AgNPs is the primary factor that results in better antimicrobial activity due to strong interaction with micro-organisms even at a lower concentration. As AgNPs release silver ions inside pathogens to kill them, many mechanisms have been proposed explaining the actions of AgNPs on bacteria, such as blocking the respiratory chain, protein denaturation due to strong bonding to functional groups, blocking transport of nutrients to the bacterial cell membrane, flowing out cellular contents by disrupting the cell membrane and blocking deoxyribonucleic acid (DNA) replication. Hence, AgNPs act as a potent killing agent against bacteria, including Gram-negative and Gram-positive.3
AgNPs have been synthesized by physical, chemical, photochemical, microemulsion, biological and microwave methods.4–7 However, the physical methods are costly, and chemical methods generally use reducing agents,8–11 which are hazardous chemicals. Hence, the research moved toward a new convenient, eco-friendly, high yield and cost-effective method called biological synthesis.12 In biological synthesis, for the reduction of silver nitrate (AgNO3), some parts of plants13–22 and microorganisms23–25 are used as reducing agents. The phytoconstituents responsible for reducing silver ions into AgNPs are glycosides, terpenoids, alkaloids, phenolics, etc.3 This process can be utilised for large scale production of AgNPs without harnessing any high pressure, energy or temperatures. But the drawback of the biological method is that it is a slow process compared to the chemical method.26,27 Hence, to overcome such problems, the microwave irradiation method has been used, which causes fast and uniform heating of the reaction mixture leading to the synthesis of AgNPs in a small span of time.28
Along with AgNPs, noble metals like gold, zinc and iron show an extensive range of material behaviour and their fabulous characteristic feature based on size, morphology and application in many diverse fields.29 Many capping agents are used to get non-agglomerated and uniform particle sizes, which bind on the surface of NPs and make them soluble in the desired solvent. Stable size of NPs can be used to build 2D and 3D structured materials representing a unique collection phenomenon.30 They are currently synthesized in many forms to catalyse reactions, protein separation, polymer preparation, sensor technology, optoelectronic recording media and heat propagation in thermal ablation therapy.31–35 Nowadays, various disease imaging systems like magnetic resonance imaging, positron emission tomography, surface-enhanced Raman spectroscopy (SERS) and optical imaging are in use based on the magnetic property of NPs.36 Wei et al. in 2015 opined that weak interaction with AgNPs causes oscillation of the conduction band electrons of AgNPs, and thereby, photon energy changes into thermal energy so that the SERS effect can be tamed to develop photothermal and thermolytic laser therapies.37 In a study conducted on the plasmonic properties of AgNPs, Lin et al. discussed that spherical AgNPs with a size range of 5–50 nm show yellow colour,38 and Tao et al. have suggested that it can be used to constitute 3D plasmonic crystals.39
Apart from the therapeutic role of AgNPs in disease treatment, they could also play a very vital role in developing new diagnostic approaches. Due to their high conductivity, catalytic activity, and plasmonic features, AgNPs are particularly appealing materials for diagnosis and can be used to enhance the performance of biosensors. A key element in detecting an analyte at low concentrations is the sensitivity of the biosensors. The sensitivity of the biosensor has been improved by using AgNPs to increase the electroactive area of the electrodes and, consequently, the electron transfer rate. Cheng et al. employed functionalized-AgNPs to develop a highly sensitive electrochemical biosensor for detecting exosomal miRNAs, which helps in the early diagnosis of cancer. In biological samples from humans, this biosensor showed a lower limit of 0.4 fM for detecting miRNA-21.40 In another work carried out by Chen et al., AgNPs were used for colorimetric detection of endogenous telomerase based on telomerase-regulated DNA “blunt-dangling” end conversion that causes AgNPs to disperse and change colour. The colour difference allowed the visual distinction between extracts from cancer cells and normal cells, and the detection limit was equal to 1 cell μL−1 of telomerase activity.41
Over the past decade, many reviews have focused on the green synthesis of AgNPs by various parts of plant extracts such as bark, stem, root, leaves, flower, oil, fruit peels, seed, seaweed and citrus lemon zest. The current review focuses on the green synthesis of AgNPs using extracts from flowers, algae and seaweed, their characterization techniques, and a possible mechanism of reduction of AgNO3 into AgNPs, along with drug delivery, biological application and the toxicity effect as well.
2. Classification of NPs
NPs can be categorized into organic or carbon-based, inorganic and hybrid NPs, as shown in Fig. 1.
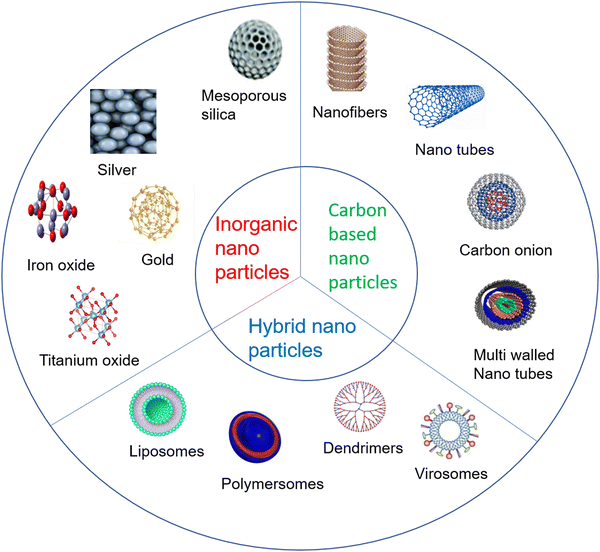 |
| Fig. 1 Types of NPs. | |
2.1. Carbon-based NPs
The carbon atom, being a more versatile element, has received increased attention from researchers towards taming its different types of hybridization states (sp, sp2 and sp3) and synthesizing a lot of its allotropes. These are fullerenes (C60), carbon nanotubes, carbon nanofibers, graphene, and carbon onions. Furthermore, single-walled carbon nanotubes, multiple-walled carbon nanotubes, quantum dots, and zero-dimensional dots have also been synthesized.42 These nanoparticles have biomedical applications like bioimaging, photoacoustic diagnostic, hemofiltration/hemodialysis, X-ray protection, etc.43
2.2. Inorganic NPs
Inorganic NPs can be classified as metals and metal oxides like aluminosilicates.44 Various NPs using inorganic metals like silver, gold, silicon, etc., and metal oxide NPs like iron oxide (Fe3O4), titanium oxide (TiO2), copper oxide (CuO), zinc oxide (ZnO), etc., have been synthesized. Other forms of gold NPs, like gold nano shells, have been synthesized45 and some advanced level synthesis methods of nano diamonds have also been reported.46
2.3. Hybrid/organic NPs
Inorganic and carbon-based NPs are combined to synthesize hybrid NPs. The hybrid NPs are synthesized to get multiple carriers and better drug delivery systems, such as lipid–polymer or mesoporous silica hybrid NPs with an inner polymeric core and a lipid shell that are used for a more promising drug carrier system.47 In addition, the physiochemical properties of hybrid nanoparticles can be harnessed for some medical applications like gene editing, biomedical imaging, dental implants, etc.48
3. Methods of AgNP preparation
There are many methods of preparation for AgNPs, which are classified into top-down, bottom-up, chemical and biological methods.
3.1. Miscellaneous methods
This section discusses several methods such as top-down, bottom-up, chemical and physical. In the top-down method, bulk materials are used as starting materials and many physical processes are used to fragment large particles into nano size particles (Fig. 2). In this method, we generally discuss the physical method involving mechanical milling, laser ablation, sputtering, etc. In mechanical milling, bulk materials are taken into a container and subjected to a high speed rotating ball to break it into a powder/particle form. In laser ablation, the solid material is placed directly under laser radiation to break it into nanoparticles49 (Fig. 3A). But this approach is not more suitable for synthesizing controlled metal NPs, especially when narrow size distribution or anisotropic morphologies are needed. Therefore, bottom-up methods like ball milling have been used for the size-controlled synthesis of NPs. In this method, some processes such as coalescence, atomic addition and oriented attachment are responsible for the atomic nucleation to synthesize the final NPs50 (Fig. 2). Whereas in chemical methods as shown in Fig. 3B, some reducing agents like sodium borohydride,7 hydrazine,8 ascorbic acid,9 and trisodium citrate10 were used for the reduction of AgNO3 into AgNPs. This method gives a high yield of AgNPs but it uses harmful chemicals that threaten living organisms.
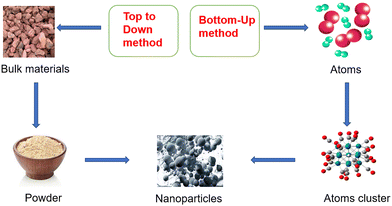 |
| Fig. 2 Top-down and bottom-up methods to synthesize NPs. | |
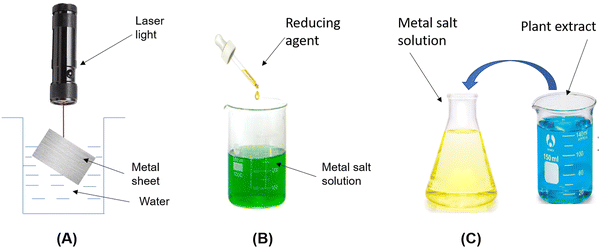 |
| Fig. 3 (A) Physical method, (B) chemical method and (C) biological method. | |
3.2. Biological method
The biological method requires plant extracts as a reducing agent instead of chemicals or a high radiation beam. In every biological method (Fig. 3C), plant extracts are used taken from bark, stems, roots, leaves, flowers, oil, fruit peels, seeds, seaweed, citrus lemon zest and microorganisms like fungi, bacteria, and yeast are used as well. In this review, we have mentioned the green synthesis of AgNPs using seaweed and algae extracts as shown in Table 1 and flower extracts in Table 2. So, biological synthesis has very broad methods of preparation of AgNPs. The plant extract is taken in small amounts, added to a high volume of AgNO3 solution, and put on a stirrer to change its colour from yellow to brown.51
Table 1 Seaweed and algae used for biological synthesis of AgNPs
S. no. |
Reducing agent seaweed (SD), algae (AL) |
Operating conditions |
Characterization |
Particle size (nm) |
Author/ref. |
#UV-Vis (UV-Vis spectroscopy), XRD (X-ray diffraction), FT-IR (Fourier-transform infrared spectroscopy), HR-TEM (high-resolution transmission electron microscopy), AFM (atomic force microscopy), SPR (surface plasmon resonance), FE-SEM (field emission scanning electron microscopy), EDX (energy dispersive X-ray), TEM (transmission electron microscopy), DLS (dynamic light scattering), TGA (thermo gravimetric analysis), SEM (scanning electron microscopy). |
1. |
Aqueous filtrate of Sargassum wightii (SD) |
AgNO3 conc. 1 mM, 5 mL/50 mL, incubated at 24 °C, 24 hours |
UV-Vis, XRD, FT-IR, HR-TEM, AFM |
15–20 |
52
|
2. |
Aqueous filtrate of Turbinaria conoides (SD) |
1 mM, Extract/AgNO3 : 10 mL/90 mL, incubated at rt |
UV-Vis, SPR, XRD, FT-IR, HR-TEM, FE-SEM |
2–17 |
53
|
3. |
Aqueous filtrate of Turbinaria ornate (SD) |
1 mM, 12 mL/88 mL, centrifuged at 10 000 rpm, 10 min |
UV-Vis, XRD, FT-IR, EDX |
20–32 |
54
|
4. |
Ethanol extract of Gracilaria birdie (SD) |
1 mM, 10 mL/100 mL, 30 min stirred, centrifuged at 3600 rpm for 15 min, pH= 7 |
UV-Vis, SPR, TEM, DLS |
20.2–94.9 |
55
|
5. |
Aqueous extract of Spyridia filamentosa (SD) |
1 mM, 20 mL/80 mL, incubated for 2 hours, centrifuged at 8000 rpm for 10 min |
UV-Vis, FT-IR, XRD, EDS, TEM |
20–30 |
21
|
6. |
Aqueous filtrate of Caulerpa serrulata (AL) |
1 mM, 5–25 mL/95–75 mL |
UV-Vis, HR-TEM, XRD |
10–100 |
56
|
7. |
Aqueous filtrate of Botryococcus braunii (AL) |
1 mM, 5 mL/45 mL, stirred 3 hours |
UV-Vis, FT-IR, SEM, XRD |
2–100 |
57
|
8. |
Aqueous filtrate of Cladophora glomerata (AL) |
5 mM, 5 mL/100 mL, stirred at 500 rpm |
UV-Vis, DLS, XRD, TEM, FT-IR |
8–11 |
58
|
9. |
Aqueous filtrate of Sargassum polycystum (SD) |
0.1 mM, 10 mL/90 mL, incubated 24–48 h, centrifuged at 13 000 rpm for 20 min |
UV, FT-IR, XRD, SEM, TEM |
— |
59
|
10. |
Aqueous extract of Enteromorpha compressa (SD) |
1 mM, 10/90 mL, incubation 1 hour |
UV-Vis, XRD, FT-IR, FE-SEM, HR-TEM |
2–24 |
60
|
11. |
Aqueous extract of Portieria hornemannii (SD) |
1 mM, 5 mL/45 mL |
UV-Vis, XRD, TEM, SEM, FT-IR |
60–70 |
61
|
12. |
Aqueous filtrate of Halymenia Porphyroides boergesen (SD) |
1 mM, 500 mg dry powder of seaweed in 250 mL of AgNO3, at pH = 5.09 |
UV-Vis, FT-IR, XRD, HR-TEM, SEM, TGA |
34.3–80.5 |
62
|
13. |
Aqueous extract of Gelidiella acerosa (SD) |
1 mM, 10 mL/100 mL |
UV-Vis, X-ray diffraction, FT-IR, AFM, SPR |
20–50 |
63
|
14. |
Aqueous extract of Fucus gardneri (SD) |
1 mM, 2 mL/10 mL |
UV-Vis, XRD, EDX, FT-IR, HR-TEM, SPR |
2–100 |
64
|
15. |
Polysaccharide of Caulerpa racemose (SD) |
1 mM, 10 mL/90 mL, stirred 30 min at 50 °C |
UV-Vis, SPR, DLS, FT-IR, SEM |
88 ± 0.5 |
65
|
16. |
Aqueous filtrate of Cystophora moniliformis (AL) |
1 mM, 1 mL/10 mL |
UV-Vis, SEM, EDX |
50–100 |
66
|
17. |
Aqueous filtrate of Chlorella vulgaris (AL) |
1 mM, 10 mL/90 mL, stirred for 3 hours, centrifuged for 20 min |
UV-Vis, FT-IR, XRD, SEM |
40–90 |
67
|
Table 2 Flowers used for biological synthesis of AgNPs
S. no. |
Reducing agent flower extract (FE) |
Operating conditions |
Characterization |
Particle size (nm) |
Author/ref. |
#UV-Vis (UV-Vis spectroscopy), XRD (X-ray diffraction), FT-IR (Fourier-transform infrared spectroscopy), HR-TEM (high-resolution transmission electron microscopy), AFM (atomic force microscopy), SPR (surface plasmon resonance), FE-SEM (field emission scanning electron microscopy), EDX (energy dispersive X-ray), TEM (transmission electron microscopy), DLS (dynamic light scattering), TGA (thermo gravimetric analysis), DTA (differential thermal analysis), SAED (selected area electron diffraction), SEM (scanning electron microscopy). |
1 |
Aqueous extract of Plumeria alba |
AgNO3 concentration – 1 mM, FE/AgNO3: 10 mL/70 mL |
UV-Vis, Zetasizer, FT-IR, TGA-DSC, XRD, SEM, EDX, DLS |
5–100 |
68
|
2 |
Ethanolic extract of Nyctanthe sarbor-tristis |
1 mM, 5% v/v into 500 μL stirred at 80 °C |
UV-Vis, TEM, XRD, TGA, DTA, FT-IR |
5–20 |
69
|
3 |
Aqueous extract of marigold |
1 mM, 6 mL/44 mL, centrifuged at 10 000 rpm for 10 min |
UV-Vis, FT-IR, XRD, TEM, |
10–90 |
70
|
4 |
Aqueous extract of Jatropha integerrima |
1 mM, different concentrations, centrifuged at 7000 rpm for 15 min |
UV-Vis, FESEM, TEM, XRD, FT-IR |
38.48–45.81 |
71
|
5 |
Aqueous extract of Couroupita guianensis |
1 mM, 5 mL/50 mL |
UV-Vis, FT-IR, XRD |
— |
72
|
6 |
Aqueous filtrate of Caesalpinia pulcherrima |
1 mM, 12 mL/38 mL |
UV-Vis, FT-IR, XRD, TEM |
3.3–18.28 |
73
|
7 |
Aqueous filtrate of Madhuca longifolia |
1 mM, 3 mL/30 mL, heated in water bath at 40 °C for 20 min, centrifuged at 11 000 rpm for 25 min |
UV-Vis, XRD, FT-IR, TEM, FE-SEM |
30–50 |
74
|
8 |
Aqueous filtrate of Fritillaria |
0.01 M, 10 mL/100 mL, incubated 24 hours |
UV-Vis, FT-IR, SEM, TEM, EDX |
9.49–30.63 |
75
|
9 |
Aqueous filtrate of Stachys lavandulifolia |
1 mM, 20 mL/20 mL, centrifuged at 4000 rpm for 20 min |
UV-Vis, FT-IR, TEM, EDS, SEM |
20–40 |
76
|
10 |
Aqueous filtrate of Ferulago macrocarpa |
1 mM, FE and AgNO3 ratio 1 : 1, 1 : 2, 1 : 4, 3 : 4 and 3 : 2, temperature 30 °C, 60 °C and 80 °C, at pH 4.5, 7, 9, and 11 respectively, centrifuged at 10 000 rpm for 20 min |
UV-Vis, FT-IR, EDX, SEM, XRF |
14–25 |
77
|
11 |
Aqueous filtrate of Moringa oleifera |
1 mM, different concentrations of FE and AgNO3, stirred for 30 min |
UV-Vis, FT-IR, XRD, TEM |
11–16 |
78
|
12 |
Aqueous filtrate of Calendula Officinalis |
1 mM, 5 mL/95 mL, shaken for 48 hours |
UV-Vis, FT-IR |
2–20 |
79
|
13 |
Ethanol extract of Lantana camara |
1 mM, 2 mL/18 mL |
UV-Vis, DLS, XRD, TEM, SAED |
16–40 |
80
|
14 |
Aqueous filtrate of Cassia auriculata |
1 mM, 10 mL/40 mL |
UV-Vis, XRD, FT-IR, HR-TEM |
10–28 |
81
|
15 |
Aqueous extract of Bauhinia purpurea |
1 mM, 4 mL/96 mL, centrifuged at 12 000 rpm for 15 min |
UV-Vis, FT-IR, SEM, EDS, XRD, TEM |
5–50 |
82
|
16 |
Aqueous filtrate of Thunbergia grandiflora |
1 mM, 10 mL/90 mL, heated at 80 °C for 10 min |
UV-Vis, XRD, FT-IR TEM, EDX, DLS |
20–50 |
83
|
17 |
Aqueous filtrate of Mangifera indica |
1 mM, 4 mL/96 mL, stirred for 30 min, centrifuged at 3000 rpm for 15 min |
UV-Vis, XRD, FT-IR, SEM, TEM |
10–20 |
84
|
18 |
Aqueous filtrate of Felty germander |
1–45 mM, different concentrations of FE and AgNO3 |
UV-Vis, XRD, FT-IR, DLS, FE-SEM, SEM, TEM |
10–100 |
85
|
19 |
Aqueous filtrate of Calotropis gigantea |
2 mM, 5 mM, and 9 mM, 10 mL/90 mL, stirred for 48 h |
UV-Vis, XRD, FT-IR, SPR-EDS, TEM |
87.85–227.65 |
86
|
20 |
Aqueous filtrate of Clitoria ternatea |
3 mM, 20 mL/4 mL, at pH 10, heated at 50 °C, centrifuged at 15 000 rpm for 15 min |
UV-Vis, TEM, zeta potential, FT-IR, XRD, EDX |
10–30 |
87
|
21 |
Aqueous filtrate of Aerva lanata |
— |
UV-Vis, TEM, AFM, EDX |
90 |
88
|
22 |
Aqueous filtrate of Catharanthus roseus |
1 mL, 1 mL/9 mL, kept for 3 days |
UV-Vis, SEM |
1–30 |
89
|
3.2. (i) Seaweed and algae extract used for biological synthesis of AgNPs.
Seaweed Sargassum wightii was used for the green synthesis of AgNPs by Shanmugam et al. They have reported the synthesized AgNPs’ anti-bacterial activity against human pathogens. The UV-Visible spectroscopy (UV-Vis) revealed the optical properties, and the X-ray diffraction (XRD) results revealed the cubic size of AgNPs. Furthermore, scanning electron microscopy (SEM) and high-resolution transmission electron microscopy (HR-TEM) were used for characterizing the morphology and size of the synthesized AgNPs, against human pathogens, Staphylococcus aureus, Klebsiella pneumoniae and Salmonella typhi.52 AgNPs were used against a marine biofilm in 2014 by Vijayan et al., which were synthesized by using an aqueous filtrate of seaweed Turbinaria conoides as a reducing agent. The size range was 2–17 nm and it was efficient in controlling the formation of the biofilm. The maximum zone of inhibition was recorded against Escherichia coli, followed by Salmonella sp., Serratia liquefaciens and Aeromonas hydrophila. Weak adherence and disintegration of marine biofilm formation were confirmed by confocal laser scanning microscopic (CLSM) images when the bacterial strains were treated with AgNPs. The minimum inhibitory concentration (MIC) and minimum bactericidal concentration (MBC) were found to be (20–40 μg mL−1) and (40–60 μg mL−1), respectively. The synthesized colloidal AgNPs showed LC50 (lethal concentration) value 88.914 ± 5.04 μg mL−1 which was confirmed by Artemia cytotoxicity assay.53 In 2016, Paramasivam et al. reported green synthesis of AgNPs using Turbinaria ornate. The size of the AgNPs was confirmed by SEM having a range of 20–32 nm and was used against the fourth instar larvae of three mosquitoes. The lethal concentration values (μg mL−1): LC50 of 0.738, 1.134 and 1.494; and LC90 of 3.342, 17.982 and 22.475 were used for the three mosquitoes Aedes aegypti, Anopheles stephensi and Culex quinquefasciatus, respectively. The study gave a key route to develop an eco-friendly, low-cost pesticide.54 Aragão et al. synthesized AgNPs at pH 10 and 11 by stirring for 30 min and at temperature 90 °C using seaweed Gracilaria birdiae. The Fourier transform infrared (FT-IR) data was used to study the interaction between the NPs and functional biomolecules present in the polysaccharide. The size of the AgNPs synthesized had the range of 20.2–94.9 nm and no agglomeration was found after four months. The antimicrobial activity of the AgNPs was evaluated for MIC ranging from 5.15 μM to 137.5 μM and found to be active against Escherichia coli.55
Marine seaweed Spyridia filamentosa was used by Valarmathi et al. in 2019 to synthesize spherical shape AgNPs of size range 20–30 nm. The AgNPs showed anti-bacterial activity as 20 mM concentration of AgNPs inhibited 44.6% and 63.4% growth of Staphylococcus sp. and Klebsiella sp., respectively. They were also found to have anticancer activity against human breast cancer (MCF-7) cells as the viability of the cells decreased with an increase in the AgNP concentration (25, 50, 75 and 100 μg mL−1) analyzed by MTT assay.21 Furthermore, stable colloidal AgNPs were synthesized by Aboelfetoh et al. using green algae Caulerpa serrulata. During synthesis, many factors were considered, like algae extract concentration, contact time, temperature and pH value. Higher temperature leads to the formation of spherical AgNPs having an average size of 10 ± 2 nm. The AgNPs were used to study the catalytic reduction activity of congo red dye from aqueous solutions. The higher temperature showed higher catalytic activity and the reaction was found to be pseudo first order with respect to the dye concentration.56
Further on, Arya et al. used green algae Botryococcus braunii to synthesize AgNPs and study their anti-bacterial property. The X-ray diffraction technique revealed that the shape of AgNPs was face centred cubic. It was found to be highly toxic against pathogenic bacterial strains such as Klebsiella pneumoniae, Staphylococcus aureus, Pseudomonas aeruginosa and Escherichia coli as well as a fungal strain Fusarium oxysporum at a concentration of 500 μg mL−1.57 The effect of AgNPs in human colon cancer cells was reported by Acharya et al. in 2020, who synthesized AgNPs using green algae Cladophora glomerata with a size range of 8–11 nm. The AgNPs displayed specific cytotoxicity to human colon cancer cells (HCT116) with IC50 value 142 ± 0.45 μM at 72 hours, whereas nil or minimal effect was observed in the control human colon epithelial cell line cells. They have also demonstrated the role of AgNPs in inducing the p53-dependent apoptosis of human colon cancer cells.58
3.2. (ii) Various types of flower extract used for biological synthesis of AgNPs.
Flower extracts are also used as a reducing agent for the green synthesis of AgNPs. Plumeria alba flowers were used by Mata et al. in 2014 for the synthesis of AgNPs that had a face-centred cubic structure. The synthesized AgNPs were used for the reduction of 4-nitrophenol to 4-aminophenol within 8 min and biological activities are evaluated by means of anti-oxidant, anti-bacterial and cytotoxic effect. Two different pathogenic bacterial strains are used for the anti-bacterial effect and a growth kinetic study in Escherichia coli confirmed their bacteriostatic effect. The cytotoxic effect of AgNPs in COLO 205 was seen by MTT assay and the IC50 concentration was found to be 5.5 and 4 μg mL−1 after 24 and 48 hours, respectively, on incubation.68 A flower extract of Nyctanthes arbour-tristis was used by Gogoi et al. for AgNP synthesis and they were found to have anti-bacterial activity against the pathogenic strain of E. coli MTCC 443 with MIC value of 220.05 μg mL−1. A mouse fibroblastic cell line (1929) was used for cytotoxicity analysis of the synthesized AgNPs and the results showed non-toxic nature clearing it for biocompatibility with 83% cell viability at a concentration of 250 μg mL−1 after 24 hours of treatment.69 Padalia et al. synthesized AgNPs from marigold flower where TEM analysis revealed the particles as spherical, hexagonal and irregular having size range 10–90 nm. The antimicrobial activity of the AgNPs was analysed with different commercial antibiotics against Gram positive (Staphylococcus aureus and Bacillus cereus) and Gram negative (Escherichia coli and Pseudomonas) bacteria, and fungi (Candida glabrata, Candida albicans and Cryptococcus neoformans).70
A flower extract of Jatropha integerrima was used to synthesis AgNPs and anti-dengue activity was analysed by cytotoxicity assessment by Verma et al. in 2022. The synthesised AgNPs was tested against aquatic larval stages of Aedes aegypti which revealed maximum mortality with AgNPs in combination with leaf and flower extract of used plant.71 Singh et al. synthesised AgNPs using aqueous extract of flower Couroupita guianensis Aubl and reported its free radical scavenging activity through a DPPH.72 AgNPs synthesized by the flower extract of Caesalpinia pulcherrima gave 12 nm particle sizes as reported by Moteriya et al. They investigated the AgNPs for their antimicrobial activity against Bacillus subtilis and Corallium rubrum and the MIC value was found to be 10 mg mL−1. AgNPs were also tested for anti-oxidant and cytotoxic effects and further genotoxic study cleared their non-toxic nature at lower concentration.73
Flowers of Madhuca longifolia were used by Patil et al. to synthesize AgNPs, which gave a size range of 30–50 nm. Analysis of the antimicrobial activity of the AgNPs against Gram-negative bacteria (Escherichia coli, Salmonella typhimurium) and Gram-positive bacteria (Bacillus cereus, Staphylococcus saprophyticus) was done by collecting pathogenic bacterial cultures from the Korean Culture of Microorganisms (KCCM).74 The MIC value was found to be 60, 40, 90 and 80 μg mL−1 for Bacillus cereus ATCC13061, Staphylococcus saprophyticus KCTC3345, Escherichia coli KCTCI1682 and Salmonella typhimurium KCCM11862, respectively. Fritillaria flowers were used by Hemmati et al. to synthesize spherical shaped AgNPs and agar disk and agar well diffusion methods were used to determine the anti-bacterial properties of the AgNPs synthesized. Furthermore, the antimicrobial activity was analysed by a broth dilution assay. In this analysis, distilled water was used as a negative control whereas difloxacin, chloramphenicol, streptomycin, gentamicin, oxytetracycline, ampicillin and amikacin were used as positive controls. AgNPs at concentrations 1, 2, 4, and 8 mg mL−1 inhibited the growth of several bacteria including Bacillus subtilis, Escherichia faecalis, and Pseudomonas aeruginosa.75 Cutaneous wound-healing along with other properties like cytotoxicity, anti-oxidant and anti-bacterial were shown by AgNPs synthesized by Zangeneh et al. using an aqueous filtrate of Stachys lavandulifolia flowers in 2019. In anti-oxidant activity tests, the DPPH (1,1-diphenyl-2-picrylhydrazyl) free radical scavenging of effect of Stachys lavandulifolia and AgNPs indicated impressive prevention compared to that of butylated hydroxytoluene. For anti-bacterial analysis, several bacterial species were taken like Proteus mirabilis, Streptococcus pneumonia, Salmonella pneumonia, Shigella flexneri, Listeria monocytogenes, Klebsiella pneumonia, Pseudomonas aeruginosa, etc., from the Iranian Research Organisation for Science and Technology, which gave excellent results. The widest zone of inhibition was seen at 64 mg mL−1 through the disc diffusion method on an agar plate.76
A Ferulago macrocarpa flower extract was used by Azarbani et al. for green synthesis of AgNPs, and they analysed their anti-oxidant and anti-bacterial properties. The anti-oxidant activity was analysed by DPPH radical scavenging activity, and anti-bacterial activity was assayed against Escherichia coli, Bacillus cereus, Klebsiella pneumonia and Staphylococcus aureus by the disc diffusion method. The broth micro dilution method was used to evaluate MIC and it was found to be 62.5, 62.5, 250 and 250 μg mL−1 against Staphylococcus aureus, Bacillus cereus, Escherichia coli and Klebsiella pneumonia, respectively.77 Another group, Bindhu et al., studied the effect of AgNPs synthesized from flower extracts of Moringa oleifera against infection caused by Salmonella, Rhizopus and Escherichia coli bacteria.78
4. Mechanism of AgNO3 reduction
A plant extract has many molecules, like polyphenols, terpene derivatives, saccharides, alkaloids, etc., which cause the reduction of AgNO3 into Ag. The possible mechanism of such chemical reactions is shown in Fig. 4. Generally, these organic molecules’ functional groups (–OH, –COOH groups) interact with AgNO3. Since AgNO3 is a salt, it breaks up to give two ions, first Ag+ and second NO3− ions when dissolved into water. Since OH and COOH groups of phytoconstituents are acidic in nature, they furnish H+ and acquire a negative charge. The negative functional groups, such as O− in phenols and COO− in acids present in plant extracts form electrostatic linkage with Ag+. During the formation of such types of linkage, Ag+ ions get reduced. The second ion, NO3−, accepts H+ from OH of phenol or from COOH of acids of phytoconstituents to form HNO3. Since HNO3 is water soluble, this remains in the aqueous phase, whereas Ag survives in a free metallic state (Ag0) to form AgNPs.
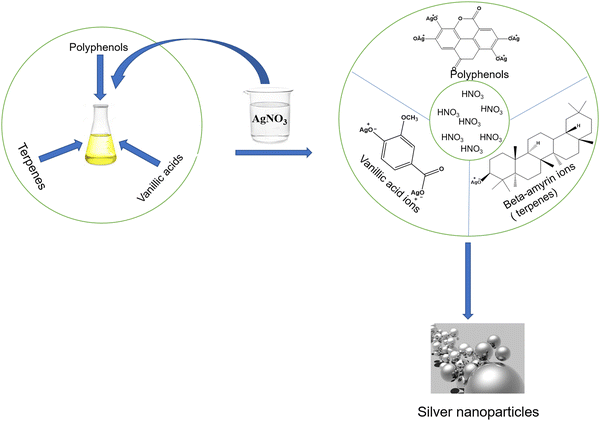 |
| Fig. 4 Mechanism of AgNO3 reduction into AgNPs. | |
5. Biological applications
People have employed silver and its compounds for thousands of years as anti-bacterial and medicinal agents. Hippocrates utilized silver remedies to treat ulcers and speed up the healing of wounds. For the treatment of burns and wound infections, silver preparations were used at the start of the 19th century. Since the 1940s, when antibiotics were first developed, they have been abused, leading to bacterial resistance that has become a global issue, especially since the 1980s. With the advent of nanotechnology at the beginning of this century, silver has gained attention once more.90 Due to their distinctive physicochemical features, AgNPs have been one of the most appealing nanomaterials in biomedicine. AgNPs are well known for their powerful, broad-spectrum anti-bacterial, anticancer and other properties as shown in Fig. 5. AgNPs have also been studied for their potential biological effects against diabetes, bone healing, and wound healing.91 In addition, they could improve vaccination immunogenicity.92
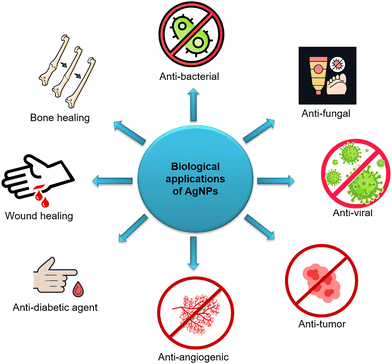 |
| Fig. 5 Various biological applications of AgNPs. | |
According to studies, AgNPs are effective against a wide range of pathogens, including bacteria, fungi, and viruses. Additionally, nematodes and worms can be successfully killed by AgNPs. Numerous researchers have tested hypotheses, albeit the precise processes behind AgNPs’ anti-bacterial and anticancer effects are still unknown.93 By causing membrane deterioration, reactive oxygen species (ROS) production, DNA damage, enzyme inactivation, and protein denaturation, AgNPs can either slow or stop the development of bacteria.94
5.1. Anti-bacterial activity
Resistance of bacteria from the genera Streptococcus, Salmonella, Escherichia, Pseudomonas, etc., to numerous common antibiotics is a severe medical issue that requires immediate attention. AgNPs have been discovered to be a promising field in the fight against diseases in searching for novel bio medicines. The vast majority of studies concur that the potential of AgNPs can prevent growth and cause the death of harmful bacteria that cause many forms of common human diseases. Due to their high surface-to-volume ratios and crystallographic surface structure, AgNPs are promising anti-bacterial agents. The ground-breaking study published by Sondi and co-workers illustrated the anti-bacterial action of AgNPs against Escherichia coli. AgNPs accumulated in the bacterial cell wall and formed “pits” in the treated Escherichia coli cells.95 Plant extracts are now an endless source of AgNP manufacturing. Plants are the main focus of research in this field since they frequently possess unique medicinal capabilities and generate AgNPs in a particular fashion. Despite most often using plant extracts to synthesize AgNPs, bacteria themselves also function as “biofactories” for creating AgNPs. Brevibacterium casei,96Streptomyces albogriseolus,97Salmonella typhimurium,98Acinetobacter calcoaceticus,99Sporosarcina koreensis,100 and Aeromonas sp.101 are among the bacteria that serve as a bacterial “plant” for AgNPs with an inhibitory effect against pathogenic pathogens. In experimental medicine, AgNPs are used in conjunction with various antibiotics as anti-bacterial agents. However, not all antibiotics have this synergistic effect.102
5.2. Anti-fungal activity
Immunosuppressed patients are more likely to contract fungi, and treating fungi-mediated illnesses can be difficult due to the scarcity of anti-fungal medications on the market right now.103 Hence, there is an unavoidable and urgent need to produce anti-fungal medicines that are biocompatible, non-toxic, and environmentally friendly. AgNPs currently serve a significant role as anti-fungal agents against many diseases caused by fungi. The anti-fungal properties of AgNPs and their mode of action were examined in the work carried out by Kim and colleagues. They elucidated the anti-fungal mode of action of the AgNPs by assessing the change in membrane dynamics of Candida albicans using 1,6-diphenyl-1,3,5-hexatriene (DPH) as a probe and employing techniques such as flow cytometry analysis, and transmission electron microscopy and a glucose-release test. The findings imply that AgNPs may operate as an anti-fungal agent by altering the composition of the cell membrane and preventing proper budding because the integrity of the membrane is compromised.104
5.3. Anti-viral activity
Diseases caused by viruses have become the most difficult and challenging task to overcome. Despite their structural simplicity, viruses pose a significant threat in the form of deadly diseases like human immunodeficiency virus (HIV), Ebola, the Spanish flu, and finally the COVID-19 virus that caused the pandemic in 2020 resulting in the death of millions of people all over the world. This demonstrates how little we understand about combating viruses. Although AgNPs have been shown to be effective against viruses, the precise mechanism by which they do this is still unknown.105 While establishing infection, viral surface components interact with ligands and proteins on the cell membrane, allowing the virus to attach itself to host cells and enter into it. The greatest approach in formulating new anti-viral agents is to disrupt the interaction between the virus and cell membrane, preventing the virus from adhering and entering the cells. Data available based on previous research suggests that the possible mechanisms of anti-viral activity of AgNPs could be due to (i) binding of AgNPs to the outer coat of the virus resulting in the inhibition of viral attachment on the cell surface receptors or (ii) binding of AgNPs to the viral DNA or RNA and suppressing its replication inside the host cell.106 Speshock et al. evaluated the interaction of AgNPs with Tacaribe virus and found a significant reduction in the viral RNA production due to inhibition of the replication process.107 Furthermore, Xiang et al. investigated the interaction of AgNPs with H1N1 influenza A virus and demonstrated the anti-H1N1 influenza A virus activity of AgNPs.108 Galdiero et al. showed that herpes simplex virus types 1 and 2 and type 3 of the human parainfluenza virus interact with AgNPs in a size-dependent manner. They demonstrated that it is possible to produce AgNPs from several fungi, and that the manufacturing method affects the anti-viral activity of these particles.109
5.4. Anti-tumor activity
Cancer is a life threatening disease and leads to many deaths worldwide. The World Health Organization (WHO) projects that there will be 22 million new cases of cancer by 2032, up from 14 million in 2012.110 Consequently, for many years, researchers have been working to develop new anticancer medications and successful clinical techniques for the treatment of cancer. AgNPs are widely used as possible cancer diagnostic and therapeutic agents. In a work carried out by Gopinath and his team, the molecular mechanism of AgNP-mediated cytotoxicity was investigated and it was revealed that the programmed cell death in both cancer and non-cancer cells depends on the concentration of AgNPs. They also studied the synergistic effect of AgNPs on the uracil phosphoribosyl transferase expression system and found that the cells treated with 5-fluorouracil became more sensitised.111 AgNPs could also inhibit the growth of acute myeloid leukemia (AML) cells. Guo et al. investigated the cytotoxic effect of AgNPs on AML cells and found that DNA damage, ROS production, and loss of mitochondrial membrane potential (MMP) results in the apoptosis of AML cells.112 Al-Sheddi et al. used the Nepeta deflersiana plant to reduce silver ions in a single step, demonstrating for the first time the biosynthesis of AgNPs and their anticancer potential against human cervical carcinoma (HeLa) cells. Their findings demonstrated that HeLA cells were cytotoxically affected by biosynthesized AgNPs (NDAgNPs) in a concentration-dependent manner.113
5.5. Anti-angiogenic activity
Angiogenesis is a complicated process that involves several gene products expressed by various cell types, all of which contribute to a coordinated series of events. However, an imbalance of the growth factors involved in this process leads to the acceleration of several diseases, such as inflammatory, neoplastic, and ophthalmic conditions. A possible approach to slow the spread of these disorders is to inhibit angiogenesis by interfering with its mechanism.114 AgNPs are involved in angiogenesis, but the mechanism is still unknown. Various studies have shown the anti-angiogenic and anticancer characteristics of AgNPs employing both in vitro and in vivo models.
Kemp et al. developed AgNPs reduced with diaminopyridinyl (DAP)-derivatized heparin (HP) polysaccharides that effectively inhibited angiogenesis. The synthesized AgNPs had a higher anti-angiogenesis efficacy when conjugated with DAPHP.115 It is known that nitric oxide synthase (NOS) upregulation encourages the production of several angiogenic factors, including vascular endothelial growth factor (VEGF) and fibroblast growth factor (FGF2). In rat models, suppression of inducible nitric oxide synthase (iNOS) was reported to stop angiogenesis.116 Kitimu et al. showed that biogenic AgNPs made from neem plant (Azadirachta indica) blocked the VEGF-NO and VEGF/VEGF-R pathways and further obstructed cell migration and metastasis, hence reducing angiogenesis.117
5.6. Anti-diabetic activity
Nearly 422 million people worldwide have diabetes; the majority of them reside in low and middle-income countries. Diabetes also causes 1.6 million fatalities globally each year.118 In recent years, diabetes diagnosis and treatment have benefited greatly from nanotechnology. There is a long history of using medicinal plants to treat various diseases. Plant extracts have been utilised in the development of AgNPs and drawn great attention over the past decade as they are eco-friendly and cost effective. Certain bioactive compounds such as flavonoids, anti-oxidants, and phenolic chemicals, are abundant in medicinal plants and are recognised as a crucial source in the development of medications against Type II diabetes mellitus.119 Bagyalakshmi and Haritha synthesized AgNPs using Pterocarpus marsupium bark and wood extract and found them to be effective for in vitro anti-diabetic activity.120 The metabolism of carbohydrate involves two main enzymes, α-amylase and α-glucosidase that are responsible for the breakdown of carbohydrates into monosaccharides resulting in elevated blood sugar levels. Thus, inhibition of these enzymes is one of the most important strategies for diabetes therapy.16 Perumalsamy and Krishnad have synthesized AgNPs and tested their anti-diabetic abilities using a hydroethanolic extract of Myristica fragrans seeds (MFHE). The α-amylase and α-glucosidase enzymes were significantly inhibited by MFHENP. Additionally, MFHENP delayed the transport of glucose across the membrane, which was measured by glucose diffusion and glucose uptake assays.121
5.7. Wound repair
The body's largest organ and primary barrier against viruses, poisons, and injuries is the skin.122 A wound is formed when the skin's structural integrity is compromised; a disease, an unintentional injury, or a deliberate act may cause this disruption.123 The hemostasis/inflammatory phase, proliferative phase, and remodelling phase are three overlapping phases of the wound healing process that focus on restoring tissue integrity and functionality. The care of chronic wounds must include the control of infections.124 Even though wounds and skin flora naturally include bacteria, these bacteria have been proposed as the crucial threshold between colonisation and clinically meaningful illness. In skin that is already injured, bacteria can inflame the tissues beneath it, releasing proteases and ROS from inflammatory cells. Increased bacterial endotoxins raise pro-inflammatory cytokine levels, which slows down and delays collagen deposition in wounds and growth factor production. Therefore, many absorbent dressings are impregnated with silver ions or particles, the most popular substance to obtain antimicrobial effects and to reduce bacteria and the risk of infection.125 Mariam et al. investigated the combined effect of mint and AgNP hydrogel films as wound-healing agents in diabetic rats. They discovered that it significantly increased the rate at which the wounds healed.126
5.8. Bone healing
Bone is a living tissue with the capacity for regeneration and restoration. Infectious diseases, genetic disorders, degenerative diseases, malignancies, and fractures are a few of the varied and complex pathologies impacting millions of individuals each year.127 Bacterial infection in bone defects typically compromises the bone's capacity to heal. However, significant defects typically caused by severe trauma, tumour removal, or genetic abnormality are frequently replaced or restored with the help of bone grafts.128 AgNPs have intrinsic anti-bacterial action with a larger spectrum than standard antibiotics. AgNPs can suppress or impede the development of mature biofilms or mature biofilms in the case of antibiotic-resistant bacteria, such as methicillin-resistant Staphylococcus aureus.129 For artificial bone scaffolds, AgNPs can be utilised as doping agents. Strong anti-bacterial activity against both Gram-positive and Gram-negative bacterial strains is demonstrated by AgNP-implanted crystalline hydroxyapatite (HA) or titanium scaffolds.130 A study conducted by Zhang et al. revealed that AgNPs could enhance osteogenesis and mesenchymal stem cell (MSC) proliferation to speed up the healing of bone fractures.131
6. Drug delivery via AgNPs
The majority of medicinal drugs have poor solubility, quick blood clearance, poor targeting, and frequently inadequate ability to cross cell membranes. However, drug delivery systems (DDSs) are able to get around some of these obstacles and improve drug delivery to the intended target, like inside cancer cells, as shown in Fig. 6.
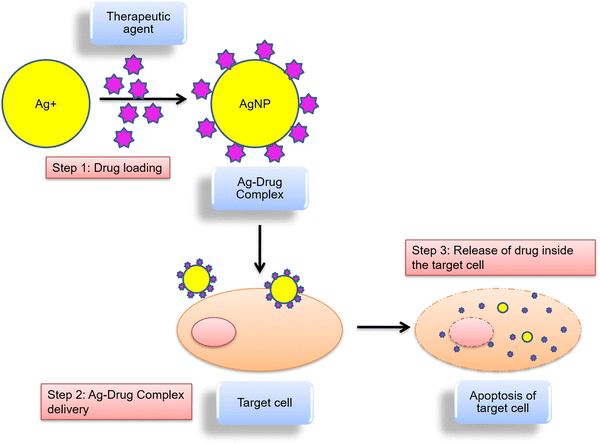 |
| Fig. 6 Systemic drug delivery in the target cell. | |
The delivery of NPs can be done either through an active or passive process. In passive delivery systems, NPs are transported across the leaky tumor capillary to tumor cells and the interstitium through passive diffusion. Whereas an active delivery system is based on molecular recognition, that delivers the NPs to a specific site. The cellular uptake of drugs can be aided by receptor-mediated endocytosis.132 For example, coupling of NPs to a ligand, such as peptides, lectins or monoclonal antibodies, allows it to interact with its receptor at the target cell site and increases the rate of cellular internalization.133 Our understanding of the mechanism of action of NPs and their underlying interactions with medicines, biomembranes, and other biological molecules has improved as a result of complementary experimental and computational studies.
Based on numerous published literatures, AgNPs are a suitable and promising drug to stop the development of cancer cells using several mechanistic methods. Fig. 7 displays the possible mechanisms of uptake and cytotoxic effects of AgNPs.
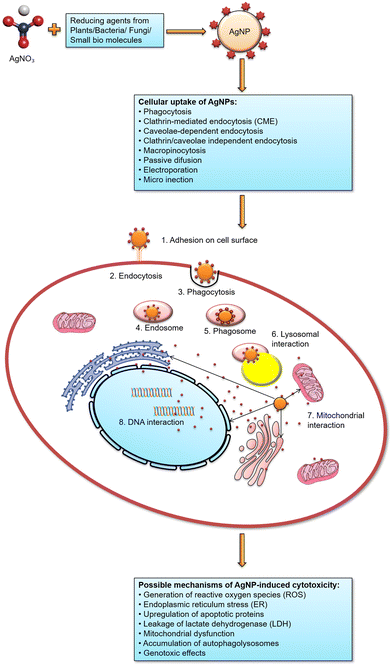 |
| Fig. 7 Cellular uptake of AgNPs and hypothesized mechanism of AgNP-induced cytotoxic effects resulting in apoptosis of the target cell. | |
7. Toxicity
AgNPs have several benefits that make them ideal for biomedical applications, although it has some harmful effects, as mentioned in Fig. 8. AgNPs are generally advertised as extremely efficient anti-bacterial agents with minimal adverse side effects on healthy mammalian cells. However, several in vitro investigations in rat hepatocytes, neuronal cells, murine stem cells, and human lung epithelial cells showed AgNP-related harmful effects. Roda et al. assessed the effect of AgNPs in rat liver and lungs. Intratracheal instillation of AgNPs (50 μg per rat) resulted in lung parenchyma injury and diffuse hepatocyte injury accompanied by inflammation.134 Xu et al. examined the toxic effects of AgNPs (20 nm) on rat cortical cells. They reported inhibition of neurite outgrowth and reduced cell viability of glial and neuron cells at a concentration of 50 μg mL−1.135 In an interesting work done by Foldbjerg and colleagues, the toxic effects of AgNPs on human lung epithelial cell line A549 were investigated through gene expression profiling. They observed alteration in the regulation of more than 1000 genes following the exposure of cell lines to 12.1 μg mL−1 of AgNPs.136 The effect of AgNPs on the proliferation and self-renewal ability of mouse embryonic stem cells (mESCs) has also been evaluated. It was reported that at a 50 μg mL−1 concentration, AgNPs could induce cell cycle arrest at G1 and S phase in mESCs.137 Pinzaru et al. investigated the in vitro toxicological profile of AgNPs on human keratinocytes – HaCat cells at different concentrations. They found no effect on the number of viable cells at lower concentrations, i.e. 0.1, 0.3, 1 and 3 μM; however, at a concentration of 10 and 50 μM, significant cytotoxic effects were observed.138
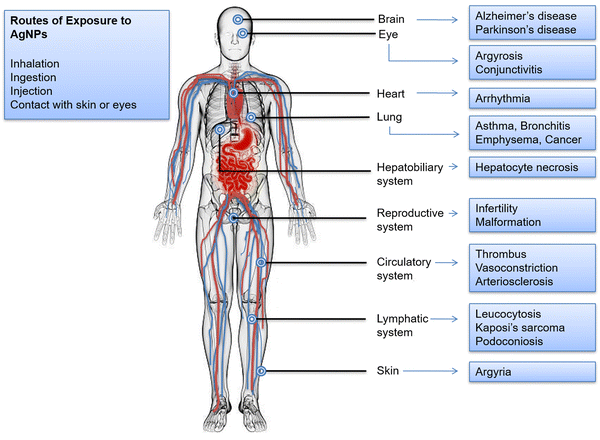 |
| Fig. 8 An illustration of AgNPs’ possible toxicities in the human body. AgNPs can be exposed through skin contact, intravenous injection, oral ingestion, and pulmonary inhalation. Eye, brain, skin, nerves, respiratory, immunological, hepatobiliary, and reproductive systems are among the organs that are impacted. AgNP-induced illnesses or pathological alterations are listed. | |
Humans consume between 0.4 to 30 g of silver daily from natural sources in food and drink.139 Studies on AgNPs harmful effect on biological systems, including bacteria, viruses, and human cells, are currently accessible, although they produce conflicting and inconsistent findings.140 The blood–brain and blood–testis barriers, for example, are easily crossed by the small-sized AgNPs, which may then cause potential cytotoxicity once within the body. AgNPs may also be delivered to several organs through blood circulation in addition to the directly exposed tissues. The absorbed AgNPs are dispersed throughout many systems, including the dermis, respiratory, spleen, digestive, urinary, nervous, immune, and reproductive systems. The primary distribution sites are the spleen, liver, kidneys, and lungs, with little AgNP deposition seen in the teeth and bones. In a study conducted by Huo et al., endoplasmic reticulum (ER) stress was employed as a sensitive and early biomarker to assess AgNPs potential for toxicity in three different human cell lines, both in vitro and in vivo in mice. According to mouse investigations, different tissues responded differently to intra-tracheal instillation exposure to AgNPs. Only the lungs and kidneys revealed evidence of apoptosis by the terminal deoxynucleotidyl transferase dUTP nick end labeling (TUNEL) assay, but the liver and kidneys both significantly showed significant ER stress responses.141 According to the toxicity experiments carried out in a rat ear model, AgNP exposure caused severe mitochondrial dysfunction and, depending on the inoculation dose, either temporary or permanent hearing loss. In addition, AgNPs were absorbed by retinal cells at even lower concentrations, and the increased number of cells that experienced oxidative stress led to significant structural damage.142
Numerous studies demonstrated that exposure to AgNPs can cause a reduction in cell viability via various biological pathways. The activation of the apoptosis mechanism and the expression of genes associated with apoptosis are examples of one of these mechanisms. Additionally, it has been demonstrated that AgNPs can alter the permeability of the mitochondrial membrane, produce ROS and accumulate inside cells, and damage DNA.143,144 AgNPs can potentially cause severe oxidative damage to cellular membranes and organelles such as the nucleus, mitochondria, and lysosomes, directly causing necrotic or apoptotic events145 as shown in Fig. 7. AgNP-induced oxidative stress can trigger inflammatory reactions, such as the activation of innate immunity and an increase in endothelial cell permeability.146
8. Conclusion and future outlook
In all facets of human existence, recent developments in nanoscience and nanotechnology have fundamentally altered how we identify, treat, and prevent different diseases. The unique qualities of AgNPs, their size and shape-dependent biomedical applications, have made them one of the most analysed nanostructures created from nanotechnology during the past several years. Various applications of green synthesized AgNPs and their improved antimicrobial properties have attracted a lot of attention of researchers worldwide. The plant extract has been proven to be highly efficient among various kinds of natural extract from biocomponents like plants, yeast, fungi, etc., used for synthesizing AgNPs. This method has decreased the use of hazardous chemicals to synthesize the AgNPs. The applications of AgNPs are broader than those of other NPs as they have displayed immense and dynamic roles in the medical field, demonstrating anti-fungal, anti-bacterial, anti-diabetic, anti-cancer effects, etc. Excellent biological activity with low toxicity to healthy cells and significant toxicity to cancer cells has been achieved by plant-based AgNPs.
Additionally, targeted drug delivery systems using AgNPs are a possible alternative for cancer treatment in the future. Their physicochemical characteristics have been studied for developing biosensors, particularly for biomedical applications that will aid in diagnosing several ailments. In conclusion, future research and development of the potential green synthesis of nanoparticles should focus on scaling up laboratory-based work to an industrial scale while taking into account current challenges, particularly the consequences on human health and the environment.
Author contributions
VS and RRP performed the literature survey and writing of the manuscript. The compilation of the manuscript was carried out by VS, RRP and SKS. MS designed and conceptualized the work. SKS and MS did the final editing of the manuscript.
Abbreviations
NPs | Nanoparticles |
AgNPs | Silver nanoparticles |
AgNO3 | Silver nitrate |
AL | Algae |
SD | Seaweed |
FE | Flower extract |
Conflicts of interest
The authors declare that they have no known competing financial interests or personal relationships that could have appeared to influence the work reported in this paper. The authors declare that they have no conflicts of interest.
Acknowledgements
This work was supported by University Grant Commission (UGC), Start-up grant, New Delhi, India. VS is grateful to the CSIR, New Delhi for providing the JRF-NET Fellowship. RRP is grateful to Banaras Hindu University (BHU), Varanasi, India for providing the UGC Non-NET Fellowship. SKS is grateful to the Institute of Eminence, BHU, Varanasi, India, for providing the Malaviya Postdoctoral Fellowship (MPDF).
References
- J. Chen, J. Wang, X. Zhang and Y. Jin, Microwave-assisted green synthesis of silver nanoparticles by carboxymethyl cellulose sodium and silver nitrate, Mater. Chem. Phys., 2008, 108(2–3), 421–424, DOI:10.1016/j.matchemphys.2007.10.019.
- G. A. Martínez-Castañón, N. Niño-Martínez, F. Martínez-Gutierrez, J. R. Martínez-Mendoza and F. Ruiz, Synthesis and antibacterial activity of silver nanoparticles with different sizes, J. Nanoparticle Res., 2008, 10(8), 1343–1348, DOI:10.1007/s11051-008-9428-6.
- V. Dhand, L. Soumya, S. Bharadwaj, S. Chakra, D. Bhatt and B. Sreedhar, Green synthesis of silver nanoparticles using Coffea arabica seed extract and its antibacterial activity, Mater. Sci. Eng., C, 2016, 58, 36–43, DOI:10.1016/j.msec.2015.08.018.
- S. Joseph and B. Mathew, Microwave-assisted facile synthesis of silver nanoparticles in aqueous medium and investigation of their catalytic and antibacterial activities, J. Mol. Liq., 2014, 197, 346–352, DOI:10.1016/j.molliq.2014.06.008.
- S. Kumar Ghosh, S. Kundu, M. Mandal, S. Nath and T. Pal, Studies on the Evolution of Silver Nanoparticles in Micelle by UV-Photoactivation, J. Nanoparticle Res., 2003, 5(5/6), 577–587, DOI:10.1023/B:NANO.0000006100.25744.fa.
- I. Pastoriza-Santos and L. M. Liz-Marzán, Formation of PVP-Protected Metal Nanoparticles in DMF, Langmuir, 2002, 18(7), 2888–2894, DOI:10.1021/la015578g.
- W. Zhang, X. Qiao and J. Chen, Synthesis and characterization of silver nanoparticles in AOT microemulsion system, Chem. Phys., 2006, 330(3), 495–500, DOI:10.1016/j.chemphys.2006.09.029.
- U. T. Khatoon, G. V. S. Nageswara Rao, K. M. Mohan, A. Ramanaviciene and A. Ramanavicius, Antibacterial and antifungal activity of silver nanospheres synthesized by tri-sodium citrate assisted chemical approach, Vacuum, 2017, 146, 259–265, DOI:10.1016/j.vacuum.2017.10.003.
- Y. Qin, X. Ji, J. Jing, H. Liu, H. Wu and W. Yang, Size control over spherical silver nanoparticles by ascorbic acid reduction, Colloids Surf., A, 2010, 372(1–3), 172–176, DOI:10.1016/j.colsurfa.2010.10.013.
- K. C. Song, S. M. Lee, T. S. Park and B. S. Lee, Preparation of colloidal silver nanoparticles by chemical reduction method, Korean J. Chem. Eng., 2009, 26(1), 153–155, DOI:10.1007/s11814-009-0024-y.
- A. Taleb, C. Petit and M. P. Pileni, Synthesis of Highly Monodisperse Silver Nanoparticles from AOT Reverse Micelles: A Way to 2D and 3D Self-Organization, Chem. Mater., 1997, 9(4), 950–959, DOI:10.1021/cm960513y.
- V. K. Sharma, R. A. Yngard and Y. Lin, Silver nanoparticles: Green synthesis and their antimicrobial activities, Adv. Colloid Interface Sci., 2009, 145(1–2), 83–96, DOI:10.1016/j.cis.2008.09.002.
- D. Elangovan, H. B. H. Rahman and R. Dhandapani,
et al., Coating of wallpaper with green synthesized silver nanoparticles from Passiflora foetida fruit and its illustrated antifungal mechanism, Process Biochem., 2022, 112, 177–182, DOI:10.1016/j.procbio.2021.11.027.
- P. Hanachi, Z. Gharari, H. Sadeghinia and T. R. Walker, Synthesis of bioactive silver nanoparticles with eco-friendly processes using Heracleum persicum stem extract and evaluation of their antioxidant, antibacterial, anticancer and apoptotic potential, J. Mol. Struct., 2022, 1265, 133325, DOI:10.1016/j.molstruc.2022.133325.
- L. N. Khanal, K. R. Sharma, H. Paudyal, K. Parajuli, B. Dahal, G. C. Ganga, Y. R. Pokharel and S. K. Kalauni,
et al., Green Synthesis of Silver Nanoparticles from Root Extracts of Rubus ellipticus Sm. and Comparison of Antioxidant and Antibacterial Activity, J. Nanomater., 2022, 2022, 1832587, DOI:10.1155/2022/1832587.
- Y. Khane, K. Benouis and S. Albukhaty,
et al., Green Synthesis of Silver Nanoparticles Using Aqueous Citrus limon Zest Extract: Characterization and Evaluation of Their Antioxidant and Antimicrobial Properties, Nanomaterials, 2022, 12(12), 2013, DOI:10.3390/nano12122013.
- S. Palithya, S. A. Gaddam and V. S. Kotakadi,
et al., Green synthesis of silver nanoparticles using flower extracts of Aerva lanata and their biomedical applications, Part. Sci. Technol., 2022, 40(1), 84–96, DOI:10.1080/02726351.2021.1919259.
- S. Periasamy, U. Jegadeesan, K. Sundaramoorthi, T. Rajeswari, V. N. B. Tokala, S. Bhattacharya, S. Muthusamy, M. Sankoh and M. K. Nellore,
et al., Comparative Analysis of Synthesis and Characterization of Silver Nanoparticles Extracted Using Leaf, Flower, and Bark of Hibiscus rosasinensis and Examine Its Antimicrobicidal Activity, J. Nanomater., 2022, 2022, 8123854, DOI:10.1155/2022/8123854.
- G. Rajkumar and R. Sundar, Biogenic one-step synthesis of silver nanoparticles (AgNPs) using an aqueous extract of Persea americana seed: Characterization, phytochemical screening, antibacterial, antifungal and antioxidant activities, Inorg. Chem. Commun., 2022, 143, 109817, DOI:10.1016/j.inoche.2022.109817.
- M. M. Riyanto, L. Asysyafiiyah, M. I. Sirajuddin and N. Cahyandaru, Direct synthesis of lemongrass (Cymbopogon citratus L.) essential oil-silver nanoparticles (EO-AgNPs) as biopesticides and application for lichen inhibition on stones, Heliyon, 2022, 8(6), e09701, DOI:10.1016/j.heliyon.2022.e09701.
- N. Valarmathi, F. Ameen, A. Almansob, P. Kumar, S. Arunprakash and M. Govarthanan, Utilization of marine seaweed Spyridia filamentosa for silver nanoparticles synthesis and its clinical applications, Mater. Lett., 2020, 263, 127244, DOI:10.1016/j.matlet.2019.127244.
- M. T. Yassin, A. A. F. Mostafa, A. A. Al-Askar and F. O. Al-Otibi, Facile Green Synthesis of Silver Nanoparticles Using Aqueous Leaf Extract of Origanum majorana with Potential Bioactivity against Multidrug Resistant Bacterial Strains, Crystals, 2022, 12(5), 603, DOI:10.3390/cryst12050603.
- P. Abishad, J. Vergis and V. Unni,
et al., Green Synthesized Silver Nanoparticles Using Lactobacillus Acidophilus as an Antioxidant, Antimicrobial, and Antibiofilm Agent Against Multi-drug Resistant Enteroaggregative Escherichia Coli, Probiotics Antimicrob. Proteins, 2022, 14(5), 904–914, DOI:10.1007/s12602-022-09961-1.
- A. Ahmad, P. Mukherjee and S. Senapati,
et al., Extracellular biosynthesis of silver nanoparticles using the fungus Fusarium oxysporum, Colloids Surf., B, 2003, 28(4), 313–318, DOI:10.1016/S0927-7765(02)00174-1.
- S. R. Waghmare, M. N. Mulla, S. R. Marathe and K. D. Sonawane, Ecofriendly production of silver nanoparticles using Candida utilis and its mechanistic action against pathogenic microorganisms, 3 Biotechnol., 2015, 5(1), 33–38, DOI:10.1007/s13205-014-0196-y.
- M. Khan, P. Karuppiah and H. Z. Alkhathlan,
et al., Green Synthesis of Silver Nanoparticles Using Juniperus procera Extract: Their Characterization, and Biological Activity, Crystals, 2022, 12(3), 420, DOI:10.3390/cryst12030420.
- M. N. Nadagouda, T. F. Speth and R. S. Varma, Microwave-Assisted Green Synthesis of Silver Nanostructures, Acc. Chem. Res., 2011, 44(7), 469–478, DOI:10.1021/ar1001457.
- S. Joseph and B. Mathew, Microwave-assisted green synthesis of silver nanoparticles and the study on catalytic activity in the degradation of dyes, J. Mol. Liq., 2015, 204, 184–191, DOI:10.1016/j.molliq.2015.01.027.
- J. Jeevanandam, A. Barhoum, Y. S. Chan, A. Dufresne and M. K. Danquah, Review on nanoparticles and nanostructured materials: history, sources, toxicity and regulations, Beilstein J. Nanotechnol., 2018, 9(1), 1050–1074, DOI:10.3762/bjnano.9.98.
- M. M. Oliveira, D. Ugarte, D. Zanchet and A. J. G. Zarbin, Influence of synthetic parameters on the size, structure, and stability of dodecanethiol-stabilized silver nanoparticles, J. Colloid Interface Sci., 2005, 292(2), 429–435, DOI:10.1016/j.jcis.2005.05.068.
- A. Corma and H. Garcia, Supported gold nanoparticles as catalysts for organic reactions, Chem. Soc. Rev., 2008, 37(9), 2096, 10.1039/b707314n.
- D. H. Gracias, J. Tien, T. L. Breen, C. Hsu and G. M. Whitesides, Forming Electrical Networks in Three Dimensions by Self-Assembly, Science, 2000, 289(5482), 1170–1172, DOI:10.1126/science.289.5482.1170.
- H. Gu, K. Xu, C. Xu and B. Xu, Biofunctional magnetic nanoparticles for protein separation and pathogen detection, Chem. Commun., 2006,(9), 941, 10.1039/b514130c.
- D. Moura, M. T. Souza and L. Liverani,
et al., Development of a bioactive glass-polymer composite for wound healing applications, Mater. Sci. Eng., C, 2017, 76, 224–232, DOI:10.1016/j.msec.2017.03.037.
- S. F. Shaikh, R. S. Mane, B. K. Min, Y. J. Hwang and O. S. Joo,
D-sorbitol-induced phase control of TiO2 nanoparticles and its application for dye-sensitized solar cells, Sci. Rep., 2016, 6(1), 20103, DOI:10.1038/srep20103.
- Z. Ashikbayeva, A. Aitkulov, T. S. H. Atabaev, W. Blanc, V. J. Inglezakis and D. Tosi, Green-Synthesized Silver Nanoparticle–Assisted Radiofrequency Ablation for Improved Thermal Treatment Distribution, Nanomaterials, 2022, 12(3), 426, DOI:10.3390/nano12030426.
- L. Wei, J. Lu, H. Xu, A. Patel, Z. S. Chen and G. Chen, Silver nanoparticles: synthesis, properties, and therapeutic applications, Drug Discovery Today, 2015, 20(5), 595–601, DOI:10.1016/j.drudis.2014.11.014.
- M. H. Lin, H. Y. Chen and S. Gwo, Layer-by-Layer Assembly of Three-Dimensional Colloidal Supercrystals with Tunable Plasmonic Properties, J. Am. Chem. Soc., 2010, 132(32), 11259–11263, DOI:10.1021/ja103722p.
- A. R. Tao, D. P. Ceperley, P. Sinsermsuksakul, A. R. Neureuther and P. Yang, Self-Organized Silver Nanoparticles for Three-Dimensional Plasmonic Crystals, Nano Lett., 2008, 8(11), 4033–4038, DOI:10.1021/nl802877h.
- W. Cheng, J. Ma and P. Cao,
et al., Enzyme-free electrochemical biosensor based on double signal amplification strategy for the ultra-sensitive detection of exosomal microRNAs in biological samples, Talanta, 2020, 219, 121242, DOI:10.1016/j.talanta.2020.121242.
- W. Chen, L. Wang, R. He, X. Xu and W. Jiang, Convertible DNA ends-based silver nanoprobes for colorimetric detection human telomerase activity, Talanta, 2018, 178, 458–463, DOI:10.1016/j.talanta.2017.09.057.
- P. R. Riley and R. J. Narayan, Recent advances in carbon nanomaterials for biomedical applications: a review, Curr. Opin. Biomed. Eng., 2021, 17, 100262, DOI:10.1016/j.cobme.2021.100262.
- D. Holmannova, P. Borsky, T. Svadlakova, L. Borska and Z. Fiala, Carbon Nanoparticles and Their Biomedical Applications, Appl. Sci., 2022, 12(15), 7865, DOI:10.3390/app12157865.
- L. Loomba and T. Scarabelli, Metallic nanoparticles and their medicinal potential. Part II: aluminosilicates, nanobiomagnets, quantum dots and cochleates, Ther. Delivery, 2013, 4(9), 1179–1196, DOI:10.4155/tde.13.74.
- K. Maier-Hauff, F. Ulrich and D. Nestler,
et al., Efficacy and safety of intratumoral thermotherapy using magnetic iron-oxide nanoparticles combined with external beam radiotherapy on patients with recurrent glioblastoma multiforme, J. Neurooncol., 2011, 103(2), 317–324, DOI:10.1007/s11060-010-0389-0.
- C. Lei, L. Zhang, K. Yang, L. Zhu and D. Lin, Toxicity of iron-based nanoparticles to green algae: Effects of particle size, crystal phase, oxidation state and environmental aging, Environ. Pollut., 2016, 218, 505–512, DOI:10.1016/j.envpol.2016.07.030.
- Y. Wang, Q. Zhao and N. Han,
et al., Mesoporous silica nanoparticles in drug delivery and biomedical applications, Nanomed. Nanotechnol. Biol. Med., 2015, 11(2), 313–327, DOI:10.1016/j.nano.2014.09.014.
- W. Park, H. Shin, B. Choi, W. K. Rhim, K. Na and D. Keun Han, Advanced hybrid nanomaterials for biomedical applications, Prog. Mater. Sci., 2020, 114, 100686, DOI:10.1016/j.pmatsci.2020.100686.
- P. G. Jamkhande, N. W. Ghule, A. H. Bamer and M. G. Kalaskar, Metal nanoparticles synthesis: An overview on methods of preparation, advantages and disadvantages, and applications, J. Drug Delivery Sci. Technol., 2019, 53, 101174, DOI:10.1016/j.jddst.2019.101174.
- P. F. M. de Oliveira, R. M. Torresi, F. Emmerling and P. H. C. Camargo, Challenges and opportunities in the bottom-up mechanochemical synthesis of noble metal nanoparticles, J. Mater. Chem. A, 2020, 8(32), 16114–16141, 10.1039/D0TA05183G.
- C. Singh, J. Kumar and P. Kumar,
et al., Green synthesis of silver nanoparticles using aqueous leaf extract of Premna integrifolia (L.) rich in polyphenols and evaluation of their antioxidant, antibacterial and cytotoxic activity, Biotechnol. Biotechnol. Equip., 2019, 33(1), 359–371, DOI:10.1080/13102818.2019.1577699.
- N. Shanmugam, P. Rajkamal and S. Cholan,
et al., Biosynthesis of silver nanoparticles from the marine seaweed Sargassum wightii and their antibacterial activity against some human pathogens, Appl. Nanosci., 2014, 4(7), 881–888, DOI:10.1007/s13204-013-0271-4.
- S. R. Vijayan, P. Santhiyagu, M. Singamuthu, N. Kumari Ahila and R. Jayaraman, Ethiraj K. Synthesis and Characterization of Silver and Gold Nanoparticles Using Aqueous Extract of Seaweed, Turbinaria conoides, and Their Antimicrofouling Activity, Sci. World J., 2014, 2014, 1–10, DOI:10.1155/2014/938272.
- P. Deepak, R. Sowmiya, R. Ramkumar, G. Balasubramani, D. Aiswarya and P. Perumal, Structural characterization and evaluation of mosquito-larvicidal property of silver nanoparticles synthesized from the seaweed, Turbinaria ornata (Turner) J. Agardh 1848, Artif. Cells, Nanomed., Biotechnol., 2017, 45(5), 990–998, DOI:10.1080/21691401.2016.1198365.
- A. P. de Aragão, T. M. de Oliveira and P. V. Quelemes,
et al., Green synthesis of silver nanoparticles using the seaweed Gracilaria birdiae and their antibacterial activity, Arabian J. Chem., 2019, 12(8), 4182–4188, DOI:10.1016/j.arabjc.2016.04.014.
- E. F. Aboelfetoh, R. A. El-Shenody and M. M. Ghobara, Eco-friendly synthesis of silver nanoparticles using green algae (Caulerpa serrulata): reaction optimization, catalytic and antibacterial activities, Environ. Monit. Assess., 2017, 189(7), 349, DOI:10.1007/s10661-017-6033-0.
- A. Arya, K. Gupta, T. S. Chundawat and D. Vaya, Biogenic Synthesis of Copper and Silver Nanoparticles Using Green Alga Botryococcus braunii and Its Antimicrobial Activity, Bioinorg. Chem. Appl., 2018, 2018, 1–9, DOI:10.1155/2018/7879403.
- D. Acharya, S. Satapathy, J. J. Thathapudi, P. Somu and G. Mishra, Biogenic synthesis of silver nanoparticles using marine algae Cladophora glomerata and evaluation of apoptotic effects in human colon cancer cells, Mater. Technol., 2022, 37(8), 569–580, DOI:10.1080/10667857.2020.1863597.
- R. Thirurunavukkarau, S. Shanmugam, K. Subramanian, P. Pandi, G. Muralitharan, M. Arokiarajan, K. Kasinathan, A. Sivaraj, R. Kalyanasundaram and S. Yousef AlOmar, Silver nanoparticles synthesized from the seaweed Sargassum polycystum and screening for their biological potential, Sci. Rep., 2022, 12, 14757, DOI:10.1038/s41598-022-18379-2.
- V. S. Ramkumar, A. Pugazhendhi and K. Gopalakrishnan,
et al., Biofabrication and characterization of silver nanoparticles using aqueous extract of seaweed Enteromorpha compressa and its biomedical properties, Biotechnol. Rep., 2017, 14, 1–7, DOI:10.1016/j.btre.2017.02.001.
- R. Fatime, M. Priya, L. Indurthi, V. Radhakrishnan and R. Sudhakaran, Biosynthesis of silver nanoparticles using red algae Portieria hornemannii and its antibacterial activity against fish pathogens, Microb. Pathog., 2020, 138, 103780, DOI:10.1016/j.micpath.2019.103780.
- V. K. Manam and M. Subbaiah, Biosynthesis and characterization of silver nanoparticles from marine macroscopic red seaweed halymenia Porphyroides boergesen (crypton) and its antifungal efficacy against dermatophytic and non-dermatophytic fungi, Asian J. Pharm. Clin. Res., 2020, 174–181, DOI:10.22159/ajpcr.2020.v13i8.38262.
- L. Satish, S. Santhakumari, S. Gowrishankar, S. K. Pandian, A. V. Ravi and M. Ramesh, Rapid biosynthesized AgNPs from Gelidiella acerosa aqueous extract mitigates quorum sensing mediated biofilm formation of Vibrio species—an in vitro and in vivo approach, Environ. Sci. Pollut. Res., 2017, 24(35), 27254–27268, DOI:10.1007/s11356-017-0296-4.
- K. F. Princy and A. Gopinath, Green synthesis of silver nanoparticles using polar seaweed Fucus gardeneri and its catalytic efficacy in the reduction of nitrophenol, Polar Sci., 2021, 30, 100692, DOI:10.1016/j.polar.2021.100692.
- T. Kathiraven, A. Sundaramanickam, N. Shanmugam and T. Balasubramanian, Green synthesis of silver nanoparticles using marine algae Caulerpa racemosa and their antibacterial activity against some human pathogens, Appl. Nanosci., 2015, 5(4), 499–504, DOI:10.1007/s13204-014-0341-2.
- T. N. V. K. V. Prasad, V. S. R. Kambala and R. Naidu, Phyconanotechnology: synthesis of silver nanoparticles using brown marine algae Cystophora moniliformis and their characterisation, J. Appl. Phycol., 2013, 25(1), 177–182, DOI:10.1007/s10811-012-9851-z.
- A. Mahajan, A. Arya and T. S. Chundawat, Green synthesis of silver nanoparticles using green alga (Chlorella vulgaris) and its application for synthesis of quinolines derivatives, Synth. Commun., 2019, 49(15), 1926–1937, DOI:10.1080/00397911.2019.1610776.
- R. Mata, J. Reddy Nakkala and S. Rani Sadras, Catalytic and biological activities of green silver nanoparticles synthesized from Plumeria alba (frangipani) flower extract, Mater. Sci. Eng., C, 2015, 51, 216–225, DOI:10.1016/j.msec.2015.02.053.
- N. Gogoi, P. J. Babu, C. Mahanta and U. Bora, Green synthesis and characterization of silver nanoparticles using alcoholic flower extract of Nyctanthes arbortristis and in vitro investigation of their antibacterial and cytotoxic activities, Mater. Sci. Eng., C, 2015, 46, 463–469, DOI:10.1016/j.msec.2014.10.069.
- H. Padalia, P. Moteriya and S. Chanda, Green synthesis of silver nanoparticles from marigold flower and its synergistic antimicrobial potential, Arabian J. Chem., 2015, 8(5), 732–741, DOI:10.1016/j.arabjc.2014.11.015.
- A. Verma and S. Preet, Novel Green Synthesis of Combinational Silver Nanoparticles using Jatropha integerrima for Dengue Vector Control and Cytotoxicity Assessment, Proc. Natl. Acad. Sci., India, Sect. B, 2022, 92(4), 747–757, DOI:10.1007/s40011-022-01373-z.
- R. Singh, C. Hano and F. Tavanti, Biogenic Synthesis and Characterization of Antioxidant and Antimicrobial Silver Nanoparticles Using Flower Extract of Couroupita guianensis Aubl, Materials, 2021, 14, 6854, DOI:10.3390/ma14226854.
- P. Moteriya and S. Chanda, Synthesis and characterization of silver nanoparticles using Caesalpinia pulcherrima flower extract and assessment of their in vitro antimicrobial, antioxidant, cytotoxic, and genotoxic activities, Artif. Cells, Nanomed., Biotechnol., 2017, 45(8), 1556–1567, DOI:10.1080/21691401.2016.1261871.
- M. P. Patil, R. D. Singh and P. B. Koli,
et al., Antibacterial potential of silver nanoparticles synthesized using Madhuca longifolia flower extract as a green resource, Microb. Pathog., 2018, 121, 184–189, DOI:10.1016/j.micpath.2018.05.040.
- S. Hemmati, A. Rashtiani, M. M. Zangeneh, P. Mohammadi, A. Zangeneh and H. Veisi, Green synthesis and characterization of silver nanoparticles using Fritillaria flower extract and their antibacterial activity against some human pathogens, Polyhedron, 2019, 158, 8–14, DOI:10.1016/j.poly.2018.10.049.
- M. M. Zangeneh, Z. Joshani, A. Zangeneh and E. Miri, Green synthesis of silver nanoparticles using aqueous extract of Stachys lavandulifolia flower, and their cytotoxicity, antioxidant, antibacterial and cutaneous wound-healing properties, Appl. Organomet. Chem., 2019, 33(9), e5016, DOI:10.1002/aoc.5016.
- F. Azarbani and S. Shiravand, Green synthesis of silver nanoparticles by Ferulago macrocarpa flowers extract and their antibacterial, antifungal and toxic effects, Green Chem. Lett. Rev., 2020, 13(1), 41–49, DOI:10.1080/17518253.2020.1726504.
- M. R. Bindhu, M. Umadevi, G. A. Esmail, N. A. Al-Dhabi and M. V. Arasu, Green synthesis and characterization of silver nanoparticles from Moringa oleifera flower and assessment of antimicrobial and sensing properties, J. Photochem. Photobiol., B, 2020, 205, 111836, DOI:10.1016/j.jphotobiol.2020.111836.
- S. K. Chidambaram, R. Maheswari and M. S. Muzammil, Efficacy of Green Synthesis of Silver Nanoparticles
using Flowers of Calendula Officinalis, Chem. Sci. Trans., 2014, 3(2), 773–777, DOI:10.7598/cst2014.815.
- B. Kumar, S. Kumari, L. Cumbal and A. Debut, Lantana camara berry for the synthesis of silver nanoparticles, Asian Pac. J. Trop. Biomed., 2015, 5(3), 192–195, DOI:10.1016/S2221-1691(15)30005-8.
- K. Muthu and S. Priya, Green synthesis, characterization and catalytic activity of silver nanoparticles using Cassia auriculata flower extract separated fraction, Spectrochim. Acta, Part A, 2017, 179, 66–72, DOI:10.1016/j.saa.2017.02.024.
- S. Chinnappan, S. Kandasamy, S. Arumugam, K. K. Seralathan, S. Thangaswamy and G. Muthusamy, Biomimetic synthesis of silver nanoparticles using flower extract of Bauhinia purpurea and its antibacterial activity against clinical pathogens, Environ. Sci. Pollut. Res., 2018, 25(1), 963–969, DOI:10.1007/s11356-017-0841-1.
- T. Varadavenkatesan, R. Selvaraj and R. Vinayagam, Green synthesis of silver nanoparticles using Thunbergia grandiflora flower extract and its catalytic action in reduction of Congo red dye, Mater. Today Proc., 2020, 23, 39–42, DOI:10.1016/j.matpr.2019.05.441.
- F. Ameen, P. Srinivasan and T. Selvankumar,
et al., Phytosynthesis of silver nanoparticles using Mangifera indica flower extract as bioreductant and their broad-spectrum antibacterial activity, Bioorg. Chem., 2019, 88, 102970, DOI:10.1016/j.bioorg.2019.102970.
- S. Ghojavand, M. Madani and J. Karimi, Green Synthesis, Characterization and Antifungal Activity of Silver Nanoparticles Using Stems and Flowers of Felty Germander, J. Inorg. Organomet. Polym. Mater., 2020, 30(8), 2987–2997, DOI:10.1007/s10904-020-01449-1.
- P. Kemala, R. Ramli, M. Khairan, Z. Jalil, G. Mauer Idroes, T. Ekawati Tallei, Z. Helwani, E. Safitri, M. Iqhrammullah and R. Nasution, Green Synthesis and Antimicrobial Activities of Silver Nanoparticles Using Calotropis gigantea from Ie Seu-Um Geothermal Area, Aceh Province, Indonesia, Molecules, 2022, 27, 5310, DOI:10.3390/molecules27165310.
- D. Khwannimit, R. Maungchang and P. Rattanakit, Green synthesis of silver nanoparticles using Clitoria ternatea flower: an efficient catalyst for removal of methyl orange, Int. J. Environ. Anal. Chem., 2020, 102(17), 5247–5263, DOI:10.1080/03067319.2020.1793974.
- P. Kanniah, J. Radhamani and P. Chelliah,
et al., Green Synthesis of Multifaceted Silver Nanoparticles Using the Flower Extract of Aerva lanata and Evaluation of Its Biological and Environmental Applications, ChemistrySelect, 2020, 5(7), 2322–2331, DOI:10.1002/slct.201903228.
- M. Kandiah and K. N. Chandrasekaran, Green Synthesis of Silver Nanoparticles Using Catharanthus roseus Flower Extracts and the Determination of Their Antioxidant, Antimicrobial, and Photocatalytic Activity, J. Nanotechnol., 2021 DOI:10.1155/2021/5512786.
- J. W. Alexander, History of the Medical Use of Silver, Surg. Infect., 2009, 10(3), 289–292, DOI:10.1089/sur.2008.9941.
- G. D. Saratale, R. G. Saratale and G. Benelli,
et al., Anti-diabetic Potential of Silver Nanoparticles Synthesized with Argyreia nervosa Leaf Extract High Synergistic Antibacterial Activity with Standard Antibiotics Against Foodborne Bacteria, J. Cluster Sci., 2017, 28(3), 1709–1727, DOI:10.1007/s10876-017-1179-z.
- R. Cohan, A. Shoari and F. Baghbani-Arani,
et al., Green synthesis and evaluation of silver nanoparticles as adjuvant in rabies veterinary vaccine, Int. J. Nanomed., 2016, 11, 3597–3605, DOI:10.2147/IJN.S109098.
- S. Hamedi, M. Ghaseminezhad, S. Shokrollahzadeh and S. A. Shojaosadati, Controlled biosynthesis of silver nanoparticles using nitrate reductase enzyme induction of filamentous fungus and their antibacterial evaluation, Artif. Cells, Nanomed., Biotechnol., 2017, 45(8), 1588–1596, DOI:10.1080/21691401.2016.1267011.
- W. R. Li, X. B. Xie, Q. S. Shi, H. Y. Zeng, Y. S. OU-Yang and Y. B. Chen, Antibacterial activity and mechanism of silver nanoparticles on Escherichia coli, Appl. Microbiol. Biotechnol., 2010, 85(4), 1115–1122, DOI:10.1007/s00253-009-2159-5.
- I. Sondi and B. Salopek-Sondi, Silver nanoparticles as antimicrobial agent: a case study on E. coli as a model for Gram-negative bacteria, J. Colloid Interface Sci., 2004, 275(1), 177–182, DOI:10.1016/j.jcis.2004.02.012.
- K. Kalishwaralal, V. Deepak and S. Ram Kumar Pandian,
et al., Biosynthesis of silver and gold nanoparticles using Brevibacterium casei, Colloids Surf., B, 2010, 77(2), 257–262, DOI:10.1016/j.colsurfb.2010.02.007.
- A. Samundeeswari, S. P. Dhas, J. Nirmala, S. P. John, A. Mukherjee and N. Chandrasekaran, Biosynthesis of silver nanoparticles using actinobacterium S treptomyces albogriseolus and its antibacterial activity: Biosynthesis of nanoparticles using actinobacteria, Biotechnol. Appl. Biochem., 2012, 59(6), 503–507, DOI:10.1002/bab.1054.
- H. R. Ghorbani, Biosynthesis of silver nanoparticles using Salmonella typhirium, J. Nanostruct. Chem., 2013, 3(1), 29, DOI:10.1186/2193-8865-3-29.
- B. A. Chopade, R. Singh and P. Wagh,
et al., Synthesis, optimization, and characterization of silver nanoparticles from Acinetobacter calcoaceticus and their enhanced antibacterial activity when combined with antibiotics, Int. J. Nanomed., 2022, 8, 4277–4290, DOI:10.2147/IJN.S48913.
- P. Singh, H. Singh, Y. J. Kim, R. Mathiyalagan, C. Wang and D. C. Yang, Extracellular synthesis of silver and gold nanoparticles by Sporosarcina koreensis DC4 and their biological applications, Enzyme Microb. Technol., 2016, 86, 75–83, DOI:10.1016/j.enzmictec.2016.02.005.
- H. Singh, J. Du and T. H. Yi, Biosynthesis of silver nanoparticles using Aeromonas sp. THG-FG1.2 and its antibacterial activity against pathogenic microbes, Artif. Cells, Nanomed., Biotechnol., 2017, 45(3), 584–590, DOI:10.3109/21691401.2016.1163715.
- A. F. Tăbăran, C. T. Matea and T. Mocan,
et al., Silver Nanoparticles for the Therapy of Tuberculosis, Int. J. Nanomed., 2020, 15, 2231–2258, DOI:10.2147/IJN.S241183.
- K. J. Kim, W. S. Sung, S. K. Moon, J. S. Choi, J. G. Kim and D. G. Lee, Antifungal effect of silver nanoparticles on dermatophytes, J. Microbiol. Biotechnol., 2008, 18(8), 1482–1484 CAS.
- K. J. Kim, W. S. Sung and B. K. Suh,
et al., Antifungal activity and mode of action of silver nano-particles on Candida albicans, Biometals, 2009, 22(2), 235–242, DOI:10.1007/s10534-008-9159-2.
- E. O. Mikhailova, Silver Nanoparticles: Mechanism of Action and Probable Bio-Application, J. Funct. Biomater., 2020, 11(4), 84, DOI:10.3390/jfb11040084.
- A. Salleh, R. Naomi and N. D. Utami,
et al., The Potential of Silver Nanoparticles for Antiviral and Antibacterial Applications: A Mechanism of Action, Nanomaterials, 2020, 10(8), 1566, DOI:10.3390/nano10081566.
- J. L. Speshock, R. C. Murdock, L. K. Braydich-Stolle, A. M. Schrand and S. M. Hussain, Interaction of silver nanoparticles with Tacaribe virus, J. Nanobiotechnol., 2010, 8(1), 19, DOI:10.1186/1477-3155-8-19.
- D. X. Xiang, Q. Chen, L. Pang and C. L. Zheng, Inhibitory effects of silver nanoparticles on H1N1 influenza A virus in vitro, J. Virol. Methods, 2011, 178(1–2), 137–142, DOI:10.1016/j.jviromet.2011.09.003.
- S. Galdiero, M. Rai and A. Gade,
et al., Antiviral activity of mycosynthesized silver nanoparticles against herpes simplex virus and human parainfluenza virus type 3, Int. J. Nanomed., 2013, 8, 4303–4314, DOI:10.2147/IJN.S50070.
- S. McGuire, World Cancer Report 2014. Geneva, Switzerland: World Health Organization, International Agency for Research on Cancer, WHO Press, 2015, Adv Nutr., 2016, 7(2), 418–419, DOI:10.3945/an.116.012211.
- P. Gopinath, S. K. Gogoi, A. Chattopadhyay and S. S. Ghosh, Implications of silver nanoparticle induced cell apoptosis for in vitro gene therapy, Nanotechnology, 2008, 19(7), 075104, DOI:10.1088/0957-4484/19/7/075104.
- D. Guo, L. Zhu and Z. Huang,
et al., Anti-leukemia activity of PVP-coated silver nanoparticles via generation of reactive oxygen species and release of silver ions, Biomaterials, 2013, 34(32), 7884–7894, DOI:10.1016/j.biomaterials.2013.07.015.
- E. S. Al-Sheddi, N. N. Farshori and M. M. Al-Oqail,
et al., Anticancer Potential of Green Synthesized Silver Nanoparticles Using Extract of Nepeta deflersiana against Human Cervical Cancer Cells (HeLA), Bioinorg. Chem. Appl., 2018, 2018, 1–12, DOI:10.1155/2018/9390784.
- S. Gurunathan, K. J. Lee, K. Kalishwaralal, S. Sheikpranbabu, R. Vaidyanathan and S. H. Eom, Antiangiogenic properties of silver nanoparticles, Biomaterials, 2009, 30(31), 6341–6350, DOI:10.1016/j.biomaterials.2009.08.008.
- M. M. Kemp, A. Kumar and S. Mousa,
et al., Gold and silver nanoparticles conjugated with heparin derivative possess anti-angiogenesis properties, Nanotechnology, 2009, 20(45), 455104, DOI:10.1088/0957-4484/20/45/455104.
- S. Nematollahi, M. Nematbakhsh, S. Haghjooyjavanmard, M. Khazaei and M. Salehi, Inducible nitric oxide synthase modulates angiogenesis in ischemic hindlimb of rat, Biomed. Pap., 2009, 153(2), 125–129, DOI:10.5507/bp.2009.021.
- S. R. Kitimu, P. Kirira and A. A. Abdille,
et al., Anti-Angiogenic and Anti-Metastatic Effects of Biogenic Silver Nanoparticles Synthesized Using Azadirachta indica, Adv. Biosci. Biotechnol., 2022, 13(04), 188–206, DOI:10.4236/abb.2022.134010.
-
WHO. Diabetes-overview, symptoms and treatments, World Health Organization, 2021 Search PubMed.
- H. Perera, Antidiabetic Effects of Pterocarpus marsupium (Gammalu), Eur. J. Med. Plants, 2016, 13(4), 1–14, DOI:10.9734/EJMP/2016/23930.
- J. Bagyalakshmi and H. Haritha, Green Synthesis and Characterization of Silver Nanoparticles Using Pterocarpus marsupium and Assessment of its In vitro Antidiabetic Activity, Am. J. Adv. Drug Delivery, 2017, 5(3) DOI:10.21767/2321-547x.1000019.
- R. Perumalsamy and L. Krishnadhas, Anti-Diabetic Activity of Silver Nanoparticles Synthesized from the Hydroethanolic Extract of Myristica fragrans Seeds, Appl. Biochem. Biotechnol., 2022, 194(3), 1136–1148, DOI:10.1007/s12010-022-03825-8.
- C. You, Q. Li and X. Wang,
et al., Silver nanoparticle loaded collagen/chitosan scaffolds promote wound healing via regulating fibroblast migration and macrophage activation, Sci. Rep., 2017, 7(1), 10489, DOI:10.1038/s41598-017-10481-0.
- F. H. Zulkifli, F. S. J. Hussain and S. S. Zeyohannes, Rasad MSBA, Yusuff MM. A facile synthesis method of hydroxyethyl cellulose-silver nanoparticle scaffolds for skin tissue engineering applications, Mater. Sci. Eng., C, 2017, 79, 151–160, DOI:10.1016/j.msec.2017.05.028.
- A. Hendi, Silver nanoparticles mediate differential responses in some of liver and kidney functions during skin wound healing, J. King Saud Univ., Sci., 2011, 23(1), 47–52, DOI:10.1016/j.jksus.2010.06.006.
- Y. Yang and H. Hu, A Review on Antimicrobial Silver Absorbent Wound Dressings Applied to Exuding Wounds, J. Microb. Biochem. Technol., 2015, 07(04), 228–233, DOI:10.4172/1948-5948.1000212.
- M. Mojally, E. Sharmin and Y. Alhindi,
et al., Hydrogel films of methanolic Mentha piperita extract and silver nanoparticles enhance wound healing in rats with diabetes Type I, J. Taibah. Univ. Med. Sci., 2022, 16(1), 308–316, DOI:10.1080/16583655.2022.2054607.
- T. R. Correia, D. R. Figueira and K. D. de Sá,
et al., 3D Printed scaffolds with bactericidal activity aimed for bone tissue regeneration, Int. J. Biol. Macromol., 2016, 93, 1432–1445, DOI:10.1016/j.ijbiomac.2016.06.004.
- P. N. Soucacos, E. O. Johnson and G. Babis, An update on recent advances in bone regeneration, Injury, 2008, 39, S1–S4, DOI:10.1016/S0020-1383(08)70009-3.
- H. Lu, Y. Liu, J. Guo, H. Wu, J. Wang and G. Wu, Biomaterials with Antibacterial and Osteoinductive Properties to Repair Infected Bone Defects, Int. J. Mol. Sci., 2016, 17(3), 334, DOI:10.3390/ijms17030334.
- E. Marsich, F. Bellomo, G. Turco, A. Travan, I. Donati and S. Paoletti, Nano-composite scaffolds for bone tissue engineering containing silver nanoparticles: preparation, characterization and biological properties, J. Mater. Sci.: Mater. Med., 2013, 24(7), 1799–1807, DOI:10.1007/s10856-013-4923-4.
- R. Zhang, P. Lee and V. C. H. Lui,
et al., Silver nanoparticles promote osteogenesis of mesenchymal stem cells and improve bone fracture healing in osteogenesis mechanism mouse model, Nanomed. Nanotechnol. Biol. Med., 2015, 11(8), 1949–1959, DOI:10.1016/j.nano.2015.07.016.
- M. Jeyaraj, M. Rajesh and R. Arun,
et al., An investigation on the cytotoxicity and caspase-mediated apoptotic effect of biologically synthesized silver nanoparticles using Podophyllum hexandrum on human cervical carcinoma cells, Colloids Surf., B, 2013, 102, 708–717, DOI:10.1016/j.colsurfb.2012.09.042.
-
M. D. Blanco, C. Teijon, R. M. Olmo and J. M. Teijo, Targeted Nanoparticles for Cancer Therapy, in Sezer A. D., ed. Recent Advances in Novel Drug Carrier Systems, InTech, 2012. DOI:10.5772/51382.
- E. Roda, M. Bottone, M. Biggiogera, G. Milanesi and T. Coccini, Pulmonary and hepatic effects after low dose exposure to nanosilver: Early and long-lasting histological and ultrastructural alterations in rat, Toxicol Rep., 2019, 6, 1047–1060, DOI:10.1016/j.toxrep.2019.09.008.
- F. Xu, C. Piett, S. Farkas, M. Qazzaz and N. I. Syed, Silver nanoparticles (AgNPs) cause degeneration of cytoskeleton and disrupt synaptic machinery of cultured cortical neurons, Mol. Brain, 2013, 6(1), 29, DOI:10.1186/1756-6606-6-29.
- R. Foldbjerg, E. S. Irving and Y. Hayashi,
et al., Global Gene Expression Profiling of Human Lung Epithelial Cells After Exposure to Nanosilver, Toxicol. Sci., 2012, 130(1), 145–157, DOI:10.1093/toxsci/kfs225.
- P. Rajanahalli, C. J. Stucke and Y. Hong, The effects of silver nanoparticles on mouse embryonic stem cell self-renewal and proliferation, Toxicol Rep., 2015, 2, 758–764, DOI:10.1016/j.toxrep.2015.05.005.
- I. Pinzaru, D. Coricovac and C. Dehelean,
et al., Stable PEG-coated silver nanoparticles – A comprehensive toxicological profile, Food Chem. Toxicol., 2018, 111, 546–556, DOI:10.1016/j.fct.2017.11.051.
- N. Hadrup and H. R. Lam, Oral toxicity of silver ions, silver nanoparticles and colloidal silver – A review, Regul. Toxicol. Pharmacol., 2014, 68(1), 1–7, DOI:10.1016/j.yrtph.2013.11.002.
- R. Vazquez-Muñoz, B. Borrego and K. Juárez-Moreno,
et al., Toxicity of silver nanoparticles in biological systems: Does the complexity of biological systems matter, Toxicol. Lett., 2017, 276, 11–20, DOI:10.1016/j.toxlet.2017.05.007.
- L. Huo, R. Chen and L. Zhao,
et al., Silver nanoparticles activate endoplasmic reticulum stress signaling pathway in cell and mouse models: The role in toxicity evaluation, Biomaterials, 2015, 61, 307–315, DOI:10.1016/j.biomaterials.2015.05.029.
- J. J. Antony, P. Sivalingam and B. Chen, Toxicological effects of silver nanoparticles, Environ. Toxicol. Pharmacol., 2015, 40(3), 729–732, DOI:10.1016/j.etap.2015.09.003.
- C. A. Dos Santos, M. M. Seckler and A. P. Ingle,
et al., Silver Nanoparticles: Therapeutical Uses, Toxicity, and Safety Issues, J. Pharm. Sci., 2014, 103(7), 1931–1944, DOI:10.1002/jps.24001.
- A. J. Kora and R. B. Sashidhar, Biogenic silver nanoparticles synthesized with rhamnogalacturonan gum: antibacterial activity, cytotoxicity and its mode of action, Arabian J. Chem., 2018, 11(3), 313–323, DOI:10.1016/j.arabjc.2014.10.036.
- M. Bin-Jumah, M. AL-Abdan, G. Albasher and S. Alarifi, Effects of Green Silver Nanoparticles on Apoptosis and Oxidative Stress in Normal and Cancerous Human Hepatic Cells in vitro, Int. J. Nanomed., 2020, 15, 1537–1548, DOI:10.2147/IJN.S239861.
- T. Zhang, L. Wang, Q. Chen and C. Chen, Cytotoxic Potential of Silver Nanoparticles, Yonsei Med. J., 2014, 55(2), 283, DOI:10.3349/ymj.2014.55.2.283.
|
This journal is © The Royal Society of Chemistry 2023 |