Antibiotic removal by metal–organic framework and 2D nanomaterial based polyetherimide mixed matrix membranes using a membrane bioreactor†
Received
12th August 2022
, Accepted 24th October 2022
First published on 25th October 2022
Abstract
In the present research work, a metal–organic framework (MOF) and 2-dimensional (2D) nanoparticles are incorporated into a polyetherimide (PEI) mixed matrix membrane to enhance their antibiotic removal performance using the submerged membrane bioreactor process. The zinc imidazolate framework (ZIF-8) (MOF) and molybdenum disulfide (MoS2) and hexagonal boron nitride (h-BN) as 2D nanomaterials have been used to enhance the structural, physical and mechanical properties of the PEI mixed matrix membranes. The different properties of the PEI mixed matrix membranes are studied using FTIR, XRD, FE-SEM, water contact angle, tensile strength, AFM and TEM analysis. Favipiravir, azithromycin and ivermectin are used as model antibiotics for the removal study. The prepared ZIF-8/PEI, MoS2/PEI and h-BN/PEI mixed matrix membranes show significant removal of antibiotics such as 87.5%, 88.89% and 92.61% for favipiravir, azithromycin, and ivermectin, respectively. The antifouling performance of the PEI mixed matrix membranes is studied using a submerged membrane bioreactor. The h-BN/PEI mixed matrix membrane shows superior antifouling performance compared to other membranes. The stability and reusability performance of the prepared membranes are investigated. The results show that the h-BN/PEI mixed matrix membrane shows significant characteristics and long-term performance. From the overall study, it is found that the MOF and 2D nanomaterials in the PEI mixed matrix membranes have strong commercial potential for antibiotic wastewater treatment.
Water impact
The ZIF-8/PEI, MoS2/PEI and h-BN/PEI mixed matrix membranes are used for antibiotic wastewater treatment using a submerged MBR system. The different membrane characteristics, separation performance, antifouling performance, reusability and stability performance of the prepared mixed matrix membranes show superior performance and potential for commercial applications.
|
1. Introduction
Coronavirus disease 2019 (COVID-19), commonly known as SARS-CoV-2, was first discovered in the city of Wuhan, Central China, on December 31, 2019, and has now spread to 213 countries worldwide. More than 38 million people are infected with the virus, which resulted in over a million fatalities throughout the globe.1 Different antibiotics and pharmaceuticals are being used to treat the present COVID-19 outbreak. The detection of antibiotics in ecosystems, which could lead to potential risks to the aquatic environment arising from direct toxicity to aquatic organisms and the generation of antibiotic-resistance genes1–3 has caused global concern about the overuse of antibiotics for the treatment of diseases caused by virus infection. Antibiotics are mostly removed from wastewater treatment plants using activated sludge and microbial degradation. As a result, developing a potential method to improve antibiotic removal from the environment is crucial. The submerged membrane bioreactor (MBR) is a cutting-edge membrane-based treatment method for enhanced wastewater treatment.4,5 It integrates biological treatment processes with semipermeable membranes. For the removal of antibiotics from wastewater, functionalized mixed matrix membranes in the MBR offer remarkable advantages, including more excellent antibiotic removal, decreased fouling propensity, lower energy consumption, and lower operating costs.6,7
Conventional MBRs have operational and fouling issues. In this work, MOF and 2D nanomaterial functionalized polyetherimide (PEI) mixed matrix membranes are employed to improve existing wastewater treatment methods. Inadequate effluent, large land area, longer start-up period, limited MLSS concentrations, high sludge production, low shock loading endurance, more personnel required for operation and maintenance, and high sludge thickening and increasing are the limitations of traditional wastewater treatment processes. A submerged membrane bioreactor system with advanced PEI mixed matrix membranes is used to address antibiotic removal performance. Researchers are interested in functionalized mixed matrix membranes because they can combine the polymer's easy miscibility with fillers to obtain superior water separation. In this study, zinc imidazolate framework-8 (ZIF-8), molybdenum disulfide (MoS2), and hexagonal boron nitride (h-BN) functionalized PEI membranes are used to remove antibiotics from synthetic wastewater. The MOF's chemical composition and other characteristics make it suitable for nanocomposite membranes. The ZIF-8 nanomaterial is used to improve membrane separation and antifouling characteristics.8 ZIF-8 has a unique porous structure, high crystallinity, and good chemical and thermal stability. Due to its characteristics, ZIF-8 is suitable for membrane separation and wastewater treatment. Also, ZIF-8 is suitable for various applications, including catalysis, separation, and sensing.
The PEI polymer's chemical, physical, and structural characteristics make it suitable for fabricating membranes. PEI is a promising material for membrane separation due to its selectivity, high heat resistance, low cost, and excellent membrane-forming properties. The PEI polymer membrane has better fouling resistance than the other membranes. The PEI membrane matrix's hydrophilicity and porousness are also unique. 2-D MoS2 is used to functionalize PEI MMMs to improve separation and fouling resistance. The 2D crystal of MoS2 is a sulfur–molybdenum–sulfur trilayer having graphene-like nanosheets. MoS2 nanoparticles have been used as a fouling-resistant membrane coating because of their smooth, low-friction surface. The mechanical properties of MoS2 allow it to produce thin layers on membrane surfaces.9 h-BN has excellent temperature and pressure resistance, anti-corrosion properties, and a fine structure.10 The h-BN-functionalized PEI MMM improves antibiotic removal and membrane stability.9 The h-BN nanomaterial has excellent temperature and pressure resistance, high corrosion resistance, extreme thinness, and a flexible structure.10 During the functionalization of the MOF and 2D nanomaterials in membrane fabrication, nanoparticles agglomerate, affecting the membrane's separation performance. In the present study, nanoparticles are evenly dispersed over the membrane's effective surface to prevent agglomeration. The MOF and 2D nanomaterials could lead to a new generation of MMMs.
In addition, antibiotics such as favipiravir, ivermectin, and azithromycin are often used to treat COVID-19. In this work, the model antibiotics favipiravir (6-fluoro-3-hydroxypyrazine-2-carboxamide), ivermectin (22,23-dihydroavermectin B1a), and azithromycin (9-deoxy-9a-aza-9a-methyl-9a-homoerythromycin) are used, and their removal is examined using the submerged MBR technique. These antibiotics are widely used to treat SARS-CoV-19. Literature studies show that from the wastewater stream, the antibiotic removal is carried out using the MBR system.5,11,12 PAMAM-coated membranes are used to investigate the antibiotics lasalocid A, salinomycin, and semduramicin as ionophore antibiotics in pharmaceutical wastewater. For lasalocid A, salinomycin, and semduramicin, they reported 82.5, 87.3, and 91.3% removal, respectively. The visible light degradation of the macrolide antibiotic azithromycin by a novel ZrO2/Ag@TiO2 nanorod composite is investigated in ref. 13 and they also found a degradation efficiency of 81.05%, confirming the excellent detoxifying effectiveness. It is observed that very few literature studies are available for the removal of antibiotics using membrane bioreactors and polymer mixed matrix membranes.
In the present study, the removal performance for antibiotics is studied using synthetic wastewater. Different antibiotics are used to treat SARS-CoV-19. However, all have side effects. Favipiravir causes swelling, muscle discomfort, respiratory issues, gastrointestinal distress, miscarriage, hay fever, and allergic dermatitis. Favipiravir is used to treat SARS-CoV-19.14,15 Azithromycin causes nausea, diarrhea, vomiting, loss of appetite, headache, dizziness, and tiredness.16,17 Ivermectin causes sleepiness, lethargy, stomach pain, itching, and vomiting.18,19 Favipiravir, azithromycin, and ivermectin all fall under the antibiotic category. The present research study is focused on the preparation of uniform and homogeneous MOF and 2D nanomaterial functionalized PEI MMMs for the removal of antibiotics such as favipiravir, azithromycin, and ivermectin. PEI MMMs are produced using different MOF (ZIF-8) and 2-D nanomaterial (MoS2 and h-BN) weight percent loadings. The research focuses on antibiotic removal. Antibiotics are degraded by bacteria in synthetic wastewater and physically separated utilizing MOF and 2D nanomaterial functionalized PEI mixed matrix membranes. Table 1 lists the physicochemical characteristics of favipiravir, ivermectin, and azithromycin.
Table 1 Physicochemical characteristics of the antibiotics
Antibiotic |
Role |
Molecular formula |
Molecular weight |
Topological surface area |
Solubility |
Log k |
Structure |
Ref. |
Ivermectin |
Antiparasitic agent |
C48H74O14 |
875.1 |
170 Å2 |
Very freely soluble in ethanol, methanol, and acetone |
3.2 |
|
20
|
Azithromycin |
Antibiotic |
C38H72N2O12 |
789.0 |
180 Å2 |
Soluble in ethanol, minimally soluble in water |
4.02 |
|
21
|
Favipiravir |
Antiviral agent |
C5H4FN3O2 |
157.10 |
86.6 Å2 |
Slightly soluble in water |
5.1 |
|
22
|
The prepared MOF and 2D nanomaterial functionalized PEI mixed matrix membranes are characterized using different techniques like XRD, FTIR, FE-SEM, AFM, hydrophilicity, mechanical properties and TEM analysis. As per the author's knowledge, no research on the removal of favipiravir, ivermectin, and azithromycin as antibiotics during SARS-CoV-19 treatment has been reported using MOF and 2D nanomaterial functionalized PEI MMMs with the MBR system. Favipiravir, ivermectin, and azithromycin are used as model antibiotics to investigate their removal using the MBR system. The following are the objectives of the research study: (1) synthesis of ZIF-8 nanoparticles; (2) synthesis of MOF and 2D nanomaterial functionalized PEI mixed matrix membranes; (3) investigation of PEI mixed matrix membrane characteristics; (4) investigation of favipiravir, ivermectin, and azithromycin removal using the MBR system; (5) investigation of the antifouling properties of MMMs.
2. Materials
Polyetherimide (PEI) pellets (density = 1.27 g cm−3 at 25 °C) are used and supplied by Sigma Aldrich, Mumbai. PEI is dried at 45 °C overnight before using for membrane preparation. The solvent used is N-methyl-2 pyrrolidone (NMP), which is a polar aprotic solvent and has strong dissolving power for many polymers. NMP with high purity (>99%) is provided by Sigma-Aldrich. It has a normal boiling point of 153 °C and a density of 0.944 g cm−3 at 20 °C. DI water (H2O) is used as a coagulant additive to strengthen the non-solvent. The MOF (ZIF-8) is prepared in the laboratory using zinc nitrate hexahydrate [Zn(NO3)2·6H2O] and 2-methyimidazole (2-MeIm) supplied by Sigma Aldrich in Mumbai, India. The h-BN nanoparticles with high purity (99%) are provided by Loba Chemie, India. MoS2 nanoparticles (Mw 160.07 g mol−1) with a purity of above 98% are supplied by Sigma-Aldrich, India. Favipiravir is supplied by Xenon Pharma, Delhi, India. Also, ivermectin is provided by Bennet Pharmaceuticals, Vadodara, Gujarat, India. Azithromycin is supplied by Matrix Healthcare, Ahmedabad, India.
2.1 Synthesis of ZIF-8 nanoparticles
ZIF-8 is synthesized using the method described in our previous study.23 The ZIF-8 nanoparticles are made up of 2.205 g Zn (NO3)2·6H2O and 2.435 g 2-MeIm in 300 mL methanol. These solutions mix effectively at room temperature. This would be continued with 1 h of continuous mixing at 50 °C to 60 °C with zinc nitrate hexahydrate solution. The blended solution is then stored for 2 days to crystallize. The obtained particles are then centrifuged for 15 min at 10
000 rpm. The ZIF-8 nanoparticles are then rinsed in DI water and dried at 70 °C for 24 h.
2.2 Fabrication of membranes
Pure PEI and MOF and 2D nanomaterial functionalized PEI MMMs are fabricated by the phase inversion technique. PEI is dissolved in the solvent NMP (16 wt%) with stirring. Each ZIF-8 (0.5 wt%), MoS2 (1.5 wt%), and h-BN (2.5 wt%) are dispersed in the PEI solution using ultrasonication (50 kHz, 250 W) for 15 min to prepare the functionalized PEI mixed matrix membranes. The fabrication of functionalized PEI MMMs is carried out using different weight percent loadings of MOF and 2D nanomaterials. The best-optimized composition of MOF and 2D nanomaterial functional PEI membranes is used in the present study (see Table 2). To achieve complete dissolution of PEI, the solution is mixed overnight and PEG is added as a pore-forming agent in the dope solution. Next, the solution is sonicated for 25 min to remove the air bubbles from the polymer dope solution. Next, the casting solution is dispersed onto a glass plate using a stainless-steel casting knife set at 100 μm, and then immersed in the water bath. Afterward, the membrane is withdrawn, carefully cleaned, and stored for further use. The MOF and 2D nanomaterial functionalization in PEI MMMs is schematically presented in Fig. 1. Throughout the manuscript, the prepared membrane type is indicated as follows: P1 (pure PEI), P2 (ZIF-8/PEI), P3 (MoS2/PEI), and P4 (h-BN/PEI) mixed matrix membranes.
Table 2 Composition of the pure PEI and PEI-based MMMs
Membrane type |
PEI (wt%) |
PEG (wt%) |
NMP (wt%) |
ZIF-8 (wt%) |
MoS2 (wt%) |
h-BN (wt%) |
P1 |
16 |
4 |
79.44 |
|
|
|
P2 |
16 |
4 |
79.50 |
0.5 |
|
|
P3 |
16 |
4 |
78.50 |
|
1.5 |
|
P4 |
16 |
4 |
77.50 |
|
|
2.5 |
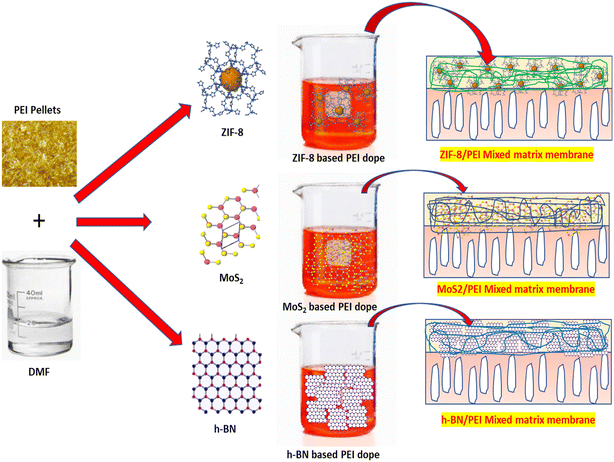 |
| Fig. 1 Schematic mechanism of addition of the MOF (ZIF-8) and 2D nanomaterials (MoS2 and h-BN) in PEI MMMs. | |
2.3 Experimental section
The antibiotic removal performance is studied using synthetic wastewater. To investigate the removal performance for antibiotics, a lab-scale membrane bioreactor setup having a capacity of 15 L has been used in the present study (see Fig. 2). For the experimental study, the antibiotics are separately added into the synthetic wastewater. After the addition of each antibiotic into the synthetic wastewater its initial concentration in wastewater is measured. The synthetic wastewater characteristics (physical–chemical parameters) are as follows: chemical oxygen demand (COD) (789 ± 82.6 mg L−1), total Kjeldahl nitrogen (TKN) (11.4 ± 6.3 mg L−1), total phosphorous (2 ± 0.62 mg L−1), mixed liquor suspended solids (MLSS) (4362.42 ± 640 mg L−1), total suspended solids (TSS) (490 ± 29 mg L−1), flux (12–14 L m−2 h−1 bar−1), sludge retention time (35 days), and hydraulic retention time (HRT) (8 h). The MBR system's maximum operating temperature is 29.8 °C. The membrane bioreactor system is operated for 9 min of filtration and 2 min of relaxation period. After the stability period of 23 days, the removal performance for favipiravir, ivermectin, and azithromycin is studied. The initial concentration of antibiotics in feed wastewater is 10.7 mg L−1, 13.71 mg L−1 and 11.8 mg L−1 for favipiravir, azithromycin and ivermectin, respectively. As per the Central Pollution Control Board (CPCB), India, the final concentration of these antibiotics in the treated water should be above 85%. Hence, the target for removing antibiotics from the feed wastewater is set above 85% and accordingly an experimental study is performed. The antibiotic concentration is determined using the BMG Labtech spectrophotometer (India).
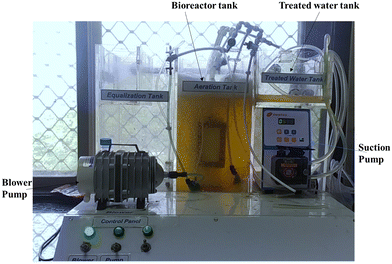 |
| Fig. 2 Experimental setup of the membrane bioreactor system. | |
2.4 Characterization of pure PEI and MMMs
The hydrophilicity of PEI MMMs is studied using Data Physics Instruments (OCA 15EC and SCA software, Germany). The 3-dimensional surface morphology and roughness of the membranes are characterized by atomic force microscopy (AFM, NanoScope IV controller microscope). The membrane sample's roughness is studied over a scan size of 2 μm × 2 μm and a scan rate of 1 Hz. The average roughness (Sa), root mean square of Z data (Sq), and the height difference between the highest peak and lowest valley (Sy) are all the surface roughness parameters. The mechanical properties, tensile strength and fracture strain, are studied using the tensile machine (UTM, INSTRON 5967, UK). The morphology of nanomaterials and their dispersion in PEI MMMs are studied using transmission electron microscopy (TEM) (JEOL, JEM 2100). The porosity (ε) of the membranes is determined using the gravimetric technique. Eqn (1) is then used to compute the porosity of the PEI MMMs: |  | (1) |
where Ww and Wd are the wet and dry membrane weights (g), A (cm2) is the membrane surface area, dw (0.988 g cm−3) is the water density, and membrane thickness is l (cm). The PEI-based membrane functional groups are studied using the ATR-FTIR (8400S SHIMADZU). The XRD analysis (Siemens XRD D5000 diffractometer, Germany) of the prepared membrane samples is carried out in the range from 10 to 80°. The surface imaging and cross-sectional morphology of the membrane samples are studied using scanning electron microscopy (SEM, FEI Quanta 400 FEG, 20 kV).
2.5 Membrane fouling experiment
At room temperature fouling studies are carried out in triplicate for the fabricated pure PEI, as well as MOF and 2D nanomaterial functionalized PEI mixed matrix membranes. Compaction, fouling, and cleaning are the three processes of the experimental methodology. The membranes are compacted at the start by filtering deionized water until the permeate flow is stable. At a pressure of 4.30 bar, the permeate flow is stabilized. The pressure is then reduced to provide the appropriate starting clean water flow, which is maintained during all fouling trials. The antibiotic-contaminated synthetic wastewater is employed as a foulant feed solution after compaction, with an antibiotic-contaminated synthetic wastewater TSS content of 490 mg L−1. Filtration takes place at the same pressure for 30 min, with aeration applied to the membrane surface to facilitate hydraulic shearing and wastewater flux is measured (JF). The fouled membrane is hydraulically cleaned with DI water for 10 min and then washed thoroughly with DI water at the end of the fouling process. The permeate flow is then measured again after resuming filtration (Jii). The membrane water flux Ji is calculated by eqn (2): |  | (2) |
where A and t indicate the membrane surface area (m2) and permeation period (h), respectively. V is the permeate volume collected (L). Rt is the total fouling resistance of the fouled membrane during filtration of antibiotic-contaminated synthetic wastewater as a feed solution, and Rm is the total intrinsic membrane resistance, Rir is the irreversible resistance and Rr is the reversible resistance of the membrane samples. The total fouling resistance of the membrane is calculated using eqn (3): |  | (4) |
| 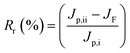 | (5) |
where TMP is the transmembrane pressure (bar) and μ is the water viscosity (Pa s). The flux recovery ratio (FRR) is a quantitative assessment of a membrane's reversible vs. irreversible fouling resistance after cleaning. It is the ratio flux of the membrane sample after cleaning and the clean membrane flux (eqn (4)):8 | 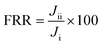 | (7) |
2.6 Removal performance for antibiotics using pure PEI and functionalized PEI MMMs
Antibiotics including favipiravir, ivermectin, and azithromycin have been used to investigate the ability of MOF and 2D nanomaterial functionalized MMMs to remove antibiotics. PEI-based mixed matrix membranes are used to treat antibiotic-contaminated synthetic wastewater. The antibiotic removal performance of each functional PEI MMM is studied using a UV-spectrophotometer. Favipiravir, ivermectin, and azithromycin have their absorption determined at 323 nm, 275 nm, and 248 nm, respectively. The antibiotic's initial and final concentrations are monitored using a UV-spectrophotometer. The 4.30 bar transmembrane pressure is applied for the filtration of wastewater. The sample of antibiotics obtained after each experiment is completely free of contaminants. The sample is collected after filtration through the respective functional PEI membrane and used for analysis using a spectrophotometer. In the present experiment, the biological transformation and possible metabolites of antibiotics are not taken into account because after degradation, some parts of the antibiotics form another degradation metabolite, which is critical for analysis using analytical methods. The removal efficiency of antibiotics is calculated using eqn (8): |  | (8) |
where Ci and Cf are the initial and final antibiotic concentrations (mg L−1).
3. Results and discussion
3.1 FTIR analysis of the PEI mixed matrix membranes
The FTIR spectrum of the prepared membranes (Fig. 3) shows that C
O asymmetric stretching vibrations can be observed at 1978 cm−1 for the pure PEI membrane.24,25 The bands detected at 1313 cm−1 and 744 cm−1 correspond to C–N stretching and imide group bending.23 Similarly, the red color spectra of the ZIF-8 functional PEI membrane represent the different functional groups. The aromatic C–H stretch band of imidazole is observed at the peak at 1590 cm−1. The characteristic peak at 1350 cm−1 relates to the stretching of the imidazole ring.23
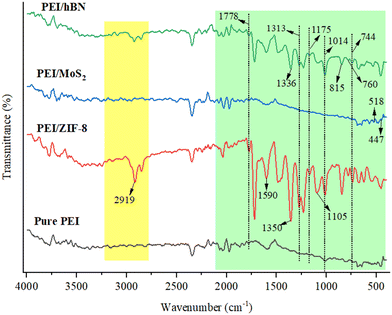 |
| Fig. 3 FTIR spectrum of the prepared membrane samples. | |
On the other hand, the intensity of all the PEI membrane characteristic peaks has disappeared and become less visible as a result of the incorporation of MoS2 into the membrane sample (blue color curve in Fig. 3). Furthermore, the 1778 cm−1 band observed in pure PEI disappears after the addition of MoS2, suggesting that MoS2 interacts with the carbonyl (C
O) and ether (C–O–C) groups of PEI, able to convert most of the vibrations in the membrane and terminating the production of new functional groups. As a result, the peaks are only slightly changing, and a few new peaks have appeared in the modified membrane. A strong peak is observed at 815 cm−1 in the h-BN functional PEI membrane, which is consistent with out-of-plane B–N–B bending vibration, suggesting the honeycomb structure of h-BN.10,23
Furthermore, all MOF and 2D nanomaterial functionalized PEI MMMs show a decrease in the absorption peak intensity corresponding to C–H stretching. The effective modification of the PEI membrane by ZIF-8, h-BN and MoS2 particles is confirmed by the changes in the C–N stretching and the C
O asymmetric stretching.
3.2 XRD pattern of the PEI mixed matrix membranes
The PEI-based MMM characteristics peaks are shown using the XRD pattern in Fig. 4. The “Match” software is used to identify the crystalline phases of the obtained MOF and 2D nanomaterial functionalized mixed matrix membranes. In the case of a metal–organic framework, the tension caused by the metal and organic linker combination may be a limiting factor for particle development during the synthesis and self-assembly of nanoparticles. The diffraction patterns of the MOF and 2D nanomaterial functionalized MMMs (corresponding to the crystalline phase) are enlarged compared to the pure PEI membrane, as seen in Fig. 4. In the crystalline structure, the smaller crystal shows that more atoms will be out of optical coherence, leading to a higher diffraction peak. The MOF and 2D nanomaterials exhibit a sharp crystalline pattern in the prepared PEI MMMs. Furthermore, the remarkable properties of MOF and 2D nanomaterials have been seen in the PEI mixed matrix membranes due to the desired weight percent loading and relative high crystallinity of these nanomaterials.23,26 The nanoparticle's crystalline structure indicates that the presently used nanoparticles have the same structure as previously reported.23 In the diffraction pattern of ZIF-8, MoS2 and h-BN-based PEI mixed matrix membranes, strong characteristic peaks are observed due to the coexistence of ZIF-8 (JCPDS data card No. 00-062-1030), MoS2 (JCPDS data card No. 37-1492), and h-BN (JCPDS data card No. 14-0033). In addition, the strong crystalline characteristics of ZIF-8, MoS2, and h-BN are observed because of the optimum concentration of these nanomaterials in the mixed matrix PEI membranes.
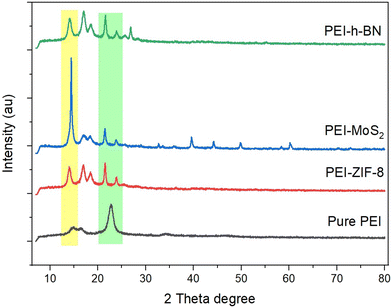 |
| Fig. 4 XRD spectra of the PEI-based mixed matrix membranes. | |
3.3 PEI mixed matrix membrane morphology
Fig. 5 shows the SEM images of pure PEI, and MOF and 2D nanomaterial functionalized PEI-based mixed matrix membranes. PEI membranes have a surface pore size of less than 50 nm, which is similar to that of ultrafiltration membranes, as illustrated in Fig. 5. The thin, dense active layer supported with finger-like pores and macrospaces are visible in the cross-section SEM images. The membrane pore structure is significantly modified by the functionalization of nanomaterials in the PEI MMMs. As shown in Fig. 5, the pure PEI membrane shows a small pore structure than the MOF and 2D nanomaterial functionalized PEI mixed matrix membranes. The number of finger-like pores in the prepared mixed matrix membranes is more uniform and has effectively developed in the membrane structure.27 In the MOF and 2D nanomaterial functionalized PEI mixed matrix membranes, a sponge-like structure is observed due to the transition zone and a large number of finger-like channels. These modifications could be attributed to the increased exchange rate of solvent and non-solvent in the presence of nanoparticles.
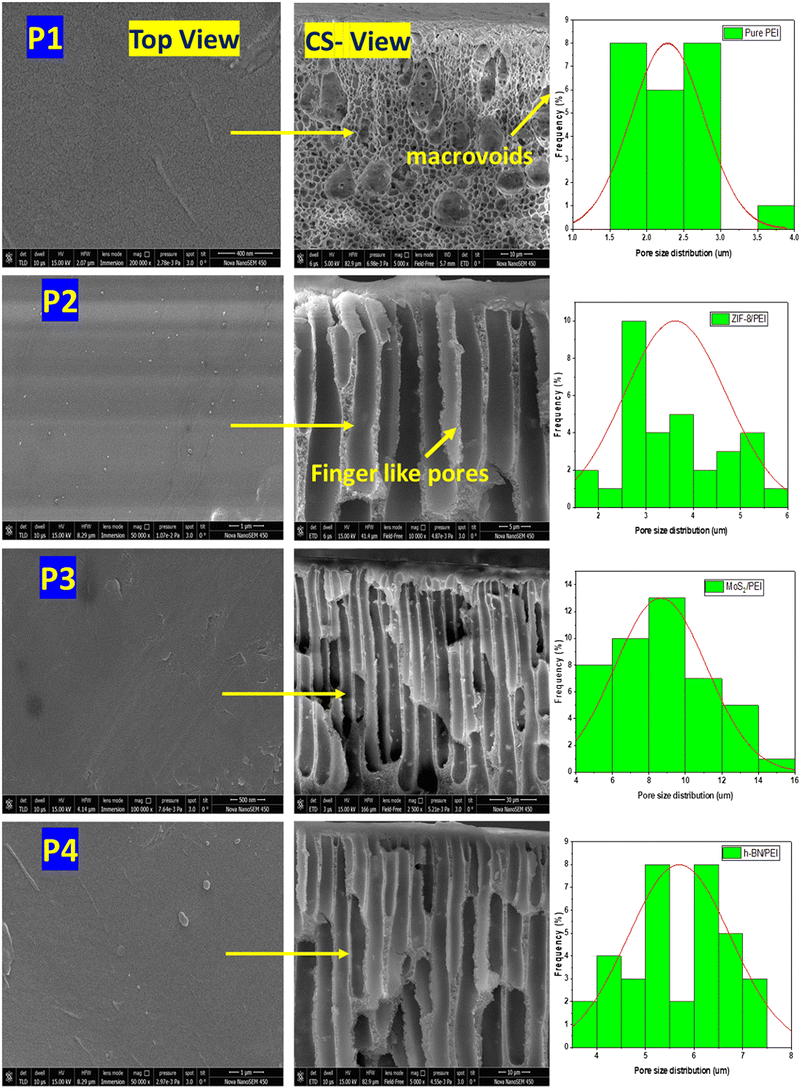 |
| Fig. 5 The top surface, cross-section morphology, and pore size distribution of the membranes. | |
Fig. 6 shows the EDS mapping of the prepared MOF and 2D nanomaterial functionalized PEI MMMs. The EDS analysis shows the existence of the MOF and 2D nanomaterials in the prepared PEI MMMs. Different elements of the MOF and 2D nanomaterials such as Zn, Mo, S, B, N, and O are observed in the EDS spectra of the PEI mixed matrix membranes. Furthermore, elemental mapping of the MOF (Zn) and 2D nanomaterials (Mo, S, B, N, and O) reveals that they are found and evenly distributed all over the PEI MMMs.
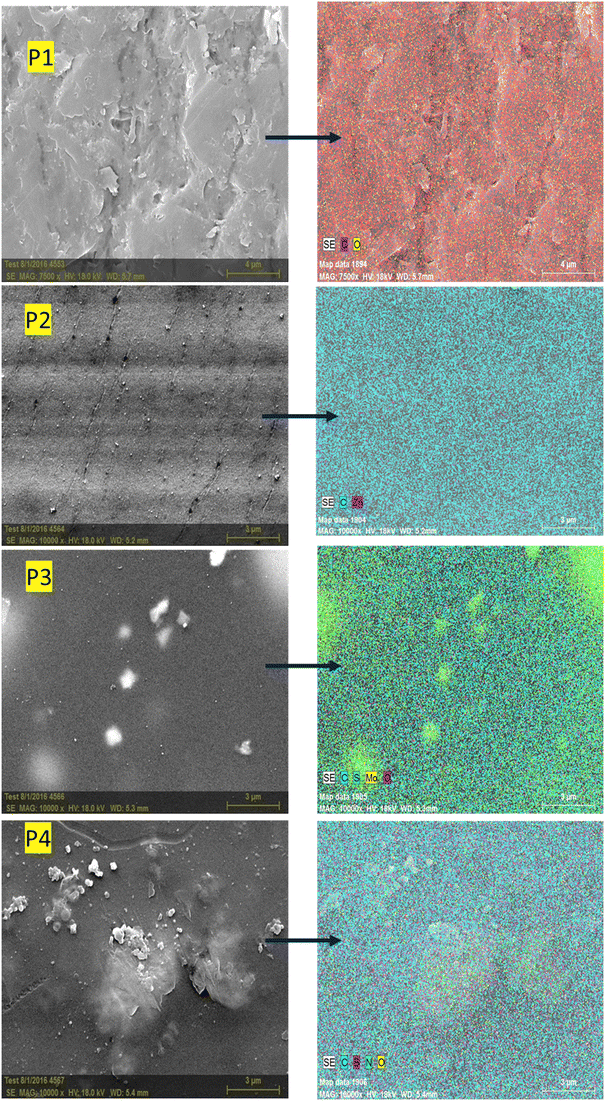 |
| Fig. 6 EDX of pure PEI and the prepared PEI-based MMMs. | |
3.4 Hydrophilicity of the PEI mixed matrix membranes
The water contact angle analysis of the membranes shows the hydrophilic or hydrophobic characteristics of the membranes. The resulting polymer membrane surface hydrophilicity is increased after functionalization using the MOF and 2D nanomaterials.24 The membrane configuration, phase and fabrication step are the critical parameters used to determine the membrane's hydrophilicity. Because pure PEI has low surface energy, it is difficult to wet with water, resulting in a wide contact angle of 69°. The h-BN/PEI membrane is more hydrophilic than the ZIF-8/PEI and MoS2/PEI MMMs. The prepared MOF and 2D nanomaterial based PEI membranes exhibit a hydrophilicity of 59°, 55°, and 48°, respectively. The bonds of a hydrophilic structure are interconnected by covalent bonds, whereas the surface of a nanoparticle has van der Waals forces responsible for its wettability. These findings are associated with the surface of the nanoparticle structure controlled by van der Waals forces on the nanomaterials.28 The surface properties of the MOF and 2D nanomaterial functionalized MMMs are greatly stimulated because nanoparticles include many hydroxyl groups. Functionalization using the MOF and 2D nanoparticles improves the hydrophilicity of PEI MMMs, which facilitates membrane permeation and antifouling properties.25
The h-BN/PEI mixed matrix membrane's surface hydrophobicity decreases compared to the other membranes. The surface hydrophilicity of the resulting membrane is influenced by the van der Waals forces. In addition, the MOF (ZIF-8) nanoparticles are very unstable in the water system. The stability of ZIF-8 nanoparticles is substantially increased by adding them to the PEI membrane matrix. The MOF (ZIF-8) is incorporated in the PEI membrane matrix after the exfoliation, which increases the stability of ZIF-8 nanoparticles and enhances the hydrophilic characteristics of PEI MMMs.
The polar covalent interaction between boron and nitrogen atoms promotes these polar atoms to contribute additional surface area to the membrane, resulting in a greater polar surface area.10 The hydrophobic properties of the pure PEI membrane are reduced, becoming hydrophilic due to its high polar surface. Consequently, the h-BN/PEI membrane is found to be more hydrophilic than the ZIF-8/PEI and MoS2/PEI membranes. The pore sizes of pure PEI, and MOF and 2D nanomaterial functionalized PEI MMMs are observed at 2.28 ± 0.5 μm, 3.619 ± 1.0 μm, 4.649 ± 3.0 μm, and 5.696 ± 1.1 μm, respectively. The membrane porosity improved by 68 ± 3, 73 ± 3, 82 ± 5, and 81 ± 6, respectively, when the MOF and 2D nanomaterials are added to the casting solution. This is in line with the modification in the pore structure found in the SEM examination (Fig. 5). Also, the measured porosity values are similar to those reported for composite membranes prepared using nanoparticles or additive materials.10
3.5 Surface roughness properties of the PEI mixed matrix membranes
The surface topography of the MOF and 2D nanomaterial functionalized PEI membranes is presented in Fig. 7. The corresponding average surface roughness of the prepared mixed matrix membranes are listed in Table 3. The average surface roughness of the prepared membranes such as pure PEI, and MOF and 2D nanomaterial functionalized PEI membranes are found to be 11.3 nm, 14.1 nm, 17.6 nm, and 13.8 nm, respectively. The minimum Sa values and the EDS mapping results (Fig. 6) suggest that the MOF and 2D nanoparticles are well dispersed throughout the PEI membrane matrix. MoS2/PEI appears to have more roughness than the other membranes. This is owing to the increased hydrophilicity of MoS2, which causes a higher diffusion between solvent phases in the water bath. The fast exchange rate between NMP and water relatively increases the surface energy of the PEI matrix; due to the surface energy difference between the PEI matrix and MoS2 nanoparticles, the roughness of the membrane increases. In addition, the MoS2 nanoparticles have higher surface energy than the other nanoparticles.
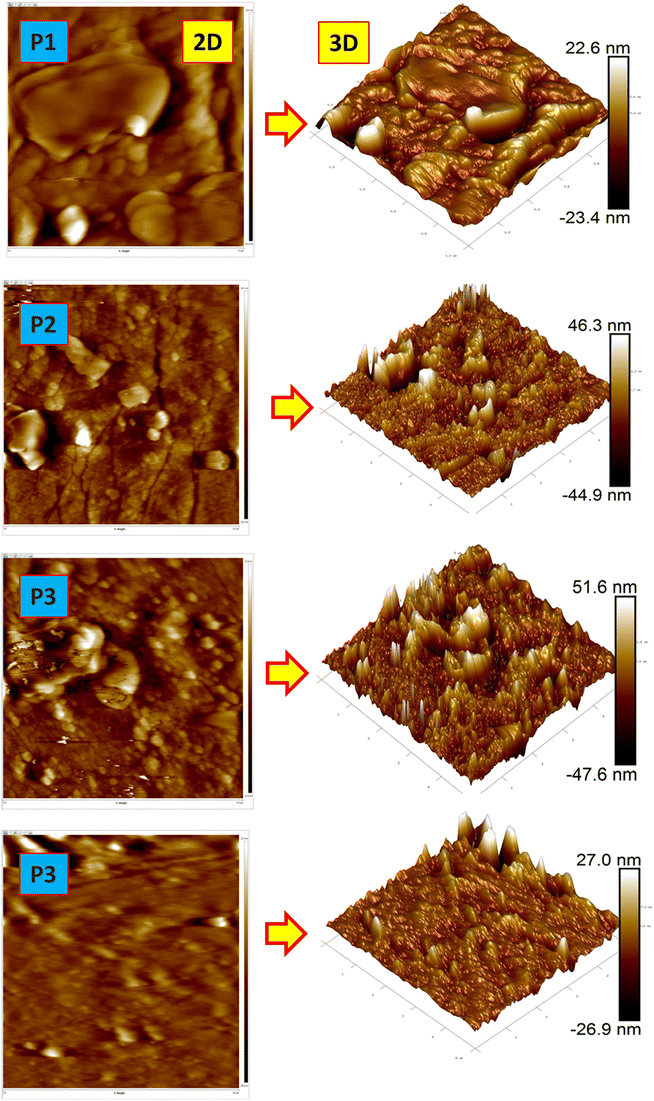 |
| Fig. 7 Surface roughness images of pure PEI and PEI-based MMMs. | |
Table 3 The surface roughness characteristics of the PEI-based MMMs
Membrane type |
S
a (nm) |
S
q (nm) |
S
y (nm) |
P1 |
11.3 |
12.1 |
128 |
P2 |
14.1 |
20.4 |
162 |
P3 |
17.6 |
21.7 |
183 |
P4 |
13.8 |
19.4 |
179 |
The higher Sa in the MoS2/PEI membrane could potentially be attributed to the presence of MoS2 in the active layer.28 The h-BN/PEI surface has a lower Sa value than the ZIF-8 and MoS2 functionalized PEI mixed matrix membranes because of the alteration in the interaction of polymer chains in the presence of h-BN.27
3.6 Tensile strength analysis of the PEI mixed matrix membranes
In the ESI,† Fig. S1 shows the mechanical properties of the MOF and 2D nanomaterial functionalized PEI MMMs. Also, ESI† Table S1 lists the tensile strength and fracture strain properties of the prepared MOF and 2D nanomaterial functionalized PEI MMMs. Before the tensile strength analysis, the membrane samples are cut for the test according to the ASTM standard D882-18. In the narrow region, the samples are cut 100 mm long and 15 mm wide. At room temperature, the tensile machine programmed elongation rate is set at 10 mm min−1. At least five tensile tests are performed on each sample, and the mean value is reported. The mixed matrix membrane separation performance and stability entirely depend on the compatibility and quality of the MOF and 2D nanomaterials with even distribution in PEI MMMs. It has been found that as the MOF and 2D nanomaterial's weight percent loading increases, the mechanical strength of the MMMs increases. The h-BN/PEI MMMs have a higher tensile strength of around 18.99 MPa at a concentration of 2.5 wt%. The tensile strengths of the ZIF-8/PEI and MoS2/PEI membranes are found to be 14.53 MPa and 18.96 MPa, respectively. The functionalization of the MOF and 2D nanomaterials in the PEI MMMs improved the chain interaction between the polymer matrix and nanoparticles, which directly enhanced the mechanical strength of MMMs.29 Next, the MOF and 2D nanoparticles change the polymer chain structure in the membrane matrix by increasing the pores, causing the chain structure to interlock more tightly. As a result of the introduction of nano-filler, the membrane matrix structure is improved, resulting in enhanced mechanical characteristics.23
3.7 TEM analysis of the PEI mixed matrix membranes
The TEM characterization of the MOF and 2D nanomaterial functionalized PEI MMMs is carried out to explore the dispersion and structure of the nanoparticles in the mixed matrix membranes. The TEM examination shows that the MOF and 2D nanomaterials are successfully functionalized in the PEI MMMs as shown in Fig. 8(a–c). The TEM analysis of the ZIF-8 metal organic framework revealed that it has a maximum size of about 6.871 nm. In the mixed matrix membranes, several sizes of ZIF-8 nanoparticles have been detected; including 4.145 nm, 5.743 nm, 5.778 nm, and 5.801 nm; these are similar to the previous literature.30
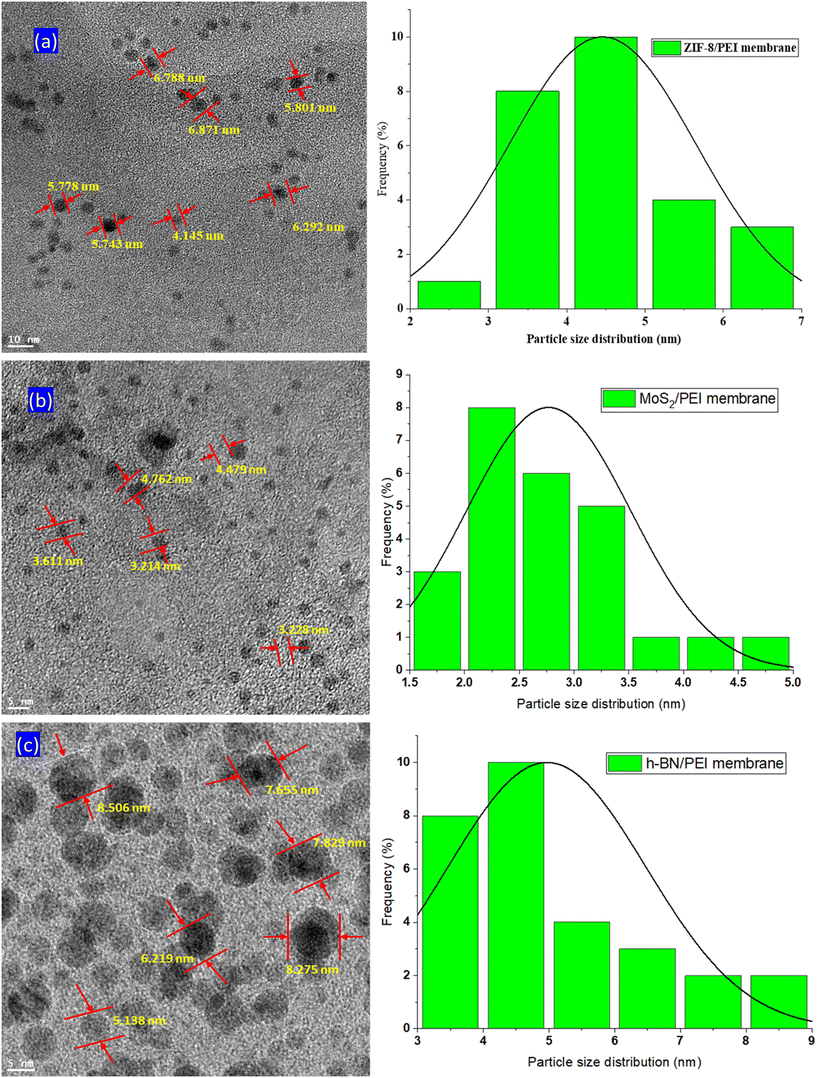 |
| Fig. 8 TEM image of (a) ZIF-8/PEI, (b) MoS2/PEI, and (c) h-BN/PEI MMMs. | |
Furthermore, the ZIF-8 nanoparticles are discovered to be in the 3 nm to 6 nm range. It is also worth mentioning that the particle shapes and sizes of all ZIF-8 nanoparticles are almost identical. The average particle size of the ZIF-8 MOF is 4.458 ± 0.198 nm.31 The TEM investigation of the MoS2 heterostructure found in the MoS2/PEI MMM is shown in Fig. 8(b). The TEM characterization of MoS2 nanoparticles reveals that the average length of the nanoparticles is about 2.767 ± 0.751 nm. In the MoS2/PEI MMMs, several sizes of MoS2 nanoparticles are detected, including 3.011 nm, 3.214 nm, 4.479 nm, and 3.228 nm; these are similar to the previous literature.32 The h-BN nanoparticles are introduced into PEI MMMs, and their uniform distribution and nanoparticle size are investigated by TEM analysis (Fig. 8(c)). h-BN is observed in the PEI MMMs in various diameters in the TEM image, including 5.158 nm, 6.219 nm, and 7.655 nm.33 The homogeneous distribution of the h-BN lattice is observed in Fig. 8(c), which is similar to that reported in the literature.34
4. Antibiotic removal efficiency
In the present research study, favipiravir, azithromycin and ivermectin are selected because these antibiotics are less absorbed in the human body and are abundantly used for treatment during the SARS-Cov-19 pandemic. The maximum concentration of the mentioned antibiotics is accumulated in the kidney system. Hence, due to the bioaccumulation and non-biodegradable nature, these antibiotics are considered particularly hazardous to the environment, including aquatic species, wild animals, and humans.35 In the submerged MBR system, different parameters influence the removal efficiency of antibiotics. The age of the sludge and its concentration, the aerobic environment in the membrane compartments, wastewater contaminants, and other physical parameters such as temperature and pH are all biological variables that impact the functioning of an MBR. The antibiotic removal efficiency from synthetic wastewater is affected due to the different parameters such as the use of the MOF and 2D nanomaterial functionalized PEI MMM characteristics, antibiotic physicochemical properties, MBR operating parameters and wastewater characteristics (pH, organic matter content, and ionic strength), in the MBR systems.35–37
The physical retention of antibiotics by the MOF and 2D nanomaterial functionalized PEI membranes, biodegradation, and sorption are the three phenomena affecting their removal performance for antibiotics using the MBR system. Biodegradation of polar antibiotics or contaminants is the primary process, and sorption is a relatively secondary process for antibiotic removal and is very limited. Sorption is followed by membrane physical separation, which is the primary route of antibiotic removal for non-polar antibiotics. In terms of sorption removal, it is determined by the antibiotic's hydrophobicity, which is assessed by water partition coefficients (log
k).37 As per the different literature studies, if the water partition coefficient (log
k) value is more significant than 3.1, the antibiotic is primarily removed by sorption and biodegradation.38 In the present study, antibiotics used for the removal study have log
k-values greater than 3.1 (see Table 2). Fig. 9(a–c) show the removal of favipiravir, azithromycin, and ivermectin using MOF and 2D nanomaterial functionalized PEI mixed matrix membranes with the MBR system.
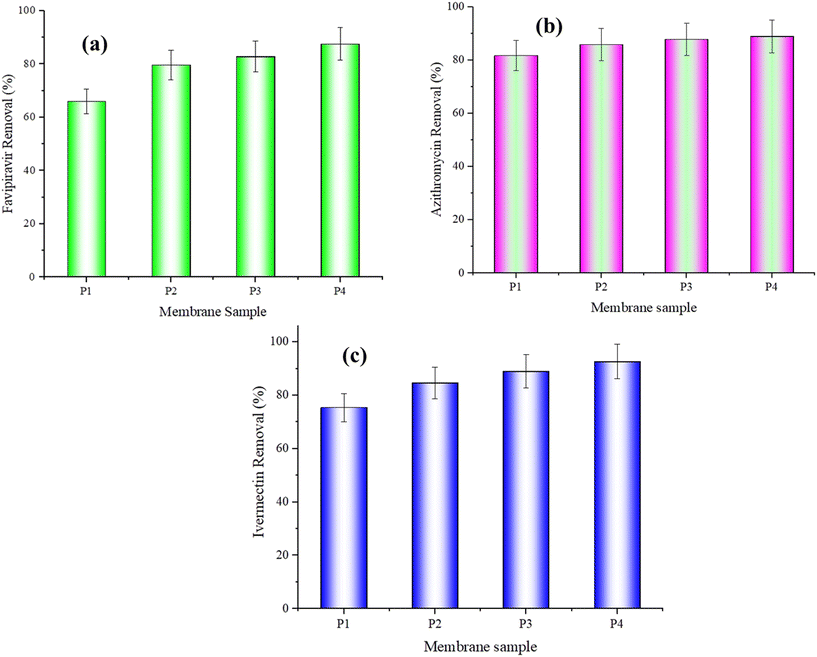 |
| Fig. 9 Antibiotic removal performance: (a) favipiravir, (b) azithromycin and (c) ivermectin. | |
On the other hand, biodegradation of antibiotics using microorganisms is a well-known biological phenomenon in which microorganisms may break down the redox-dependent micropollutants in the wastewater system.39 In the present research study, the removal of antibiotics focused on the sorption mechanism. In the bioreactor system, microorganisms are present in the wastewater stream. Hence, biodegradation of antibiotics using microorganisms may lead to the formation of different by-products because antibiotics and microorganisms have a similar negative charge. The formation of different by-products due to biodegradation is difficult to analyze using a spectrophotometer. But due to the biodegradation reaction, some concentrations of antibiotics may decrease, which helps to study the removal of antibiotics using the sorption mechanism. As a result, Fig. 9(a) shows the favipiravir removal performance of the MBR systems using pure PEI and functionalized PEI mixed matrix membranes.
The favipiravir removal by the sorption mechanism is found to be 66.67%, 79.63%, 82.82%, and 87.5% using pure PEI (P1), ZIF-8/PEI (P2), MoS2/PEI (P3), and h-BN/PEI (P4) MMMs in the experiment. In addition, the initial concentration of favipiravir is 10.7 mg L−1, which can be decreased to 0.01 by using the MOF and 2D nanomaterial functionalized PEI-based mixed matrix membranes by the sorption mechanism. Azithromycin removal performance is also studied using the pure PEI and MOF and 2D nanomaterial functionalized PEI mixed matrix membranes. The removal efficiency of azithromycin using pure PEI, ZIF-8/PEI, MoS2/PEI, and h-BN/PEI mixed matrix membranes is obtained at around 81.71%, 85.83%, 87.78%, and 88.89%, respectively (Fig. 9(b)). The initial azithromycin concentration in the synthetic wastewater is 13.71 mg L−1 and decreases to roughly 0.7 mg L−1 after the removal experiment. The effectiveness of the submerged membrane bioreactor system, pure PEI, and MOF and 2D nanomaterial functionalized PEI-based mixed matrix membranes in removing ivermectin is also investigated.
Before the experiment, the ivermectin initial concentration is around 11.8 mg L−1. After the experiment, the ivermectin concentration is determined to be 0.33 mg L−1. Fig. 9(c) shows that the ivermectin removal performance using pure PEI, ZIF-8/PEI, MoS2/PEI, and h-BN/PEI MMMs is 75.43%, 84.67%, 88.98%, and 92.61%, respectively. According to the results obtained for favipiravir, azithromycin, and ivermectin removal, it has been discovered that a submerged membrane bioreactor with pure PEI and PEI-based mixed matrix membranes successfully removed the highest concentration of antibiotics using the sorption mechanism.39,40 Favipiravir, azithromycin and ivermectin removal using MOF and 2D nanomaterial functionalized PEI MMMs are shown in ESI† Fig. S2. The phase change in different antibiotics is shown with anecdotic beakers.
5. Membrane antifouling performance
5.1 Pure water flux
Fig. 10 shows the pure water flux for pure PEI and MOF and 2D nanomaterial functionalized PEI MMMs. The addition of the MOF (ZIF-8) and 2D nanomaterials (MoS2 and h-BN) into PEI membranes considerably enhanced the membrane's water permeability: from 21 L m−2 h−1 bar−1 for pure PEI to 26 L m−2 h−1 bar−1, 31 L m−2 h−1 bar−1, and 37 L m−2 h−1 bar−1 for ZIF-8/PEI, MoS2/PEI, and h-BN/PEI membranes, respectively. The high flux is observed in the functionalized PEI mixed matrix membranes because of the porosity and wettability. These two parameters cause the increased pure water flux of the membranes. The membrane hydrophilicity increases the attraction between the membrane surface and a water molecule, resulting in more hydrophilic membrane properties. Also, the optimum weight percent loading of the MOF and 2D nanomaterials increases the membrane's effective filtration area and porosity, which shows an increasing trend of flux.41 Next, nanomaterials in the polymer membrane improve the polymer chain morphology, which allows the H2O molecules to be easily filtered by the MMMs. The roughness of the MOF and 2D nanomaterial functionalized PEI MMMs has been greatly improved, resulting in more area for filtration and, as a result, a better flux of the MMMs is obtained.23,25,42
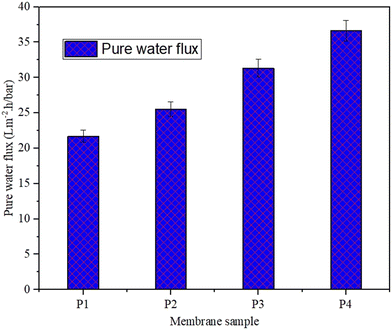 |
| Fig. 10 Pure water flux of the pure PEI and PEI-based MMMs. | |
5.2 Fouling resistance
Antibiotic-contaminated synthetic wastewater is used as a model foulant to evaluate membrane fouling behaviour. The feed wastewater containing a total suspended solids concentration of 490 mg L−1 is filtered under constant pressure. Fig. S3 (ESI†) shows the flux of the pure PEI and ZIF-8/PEI, MoS2/PEI, and h-BN/PEI membranes during the 30 min wastewater fouling step. During the filtration of antibiotic-contaminated synthetic wastewater, the flow of the pure PEI membranes decreased significantly. Nonetheless, at the completion of the wastewater filtration stage, the MOF and 2D nanomaterial functionalized PEI membranes have a considerably smaller flux drop with normalized flux than the pure PEI membrane.
Analysis of fouling layer resistance reveals a considerable decrease in reversible and irreversible fouling resistance of the membranes (Fig. 11), as estimated by eqn (3)–(7). The ZIF-8/PEI, MoS2/PEI, and h-BN/PEI membranes had lower reversible and irreversible wastewater fouling layer resistance than pure PEI membranes. The lower Rr and Rir reveal a lower deposition of foulants on its surface. This is due to increased electrostatic repulsion between the membrane and suspended molecules and improved membrane hydrophilicity, which lowers hydrophobic interactions between wastewater molecules and the membrane surface.28
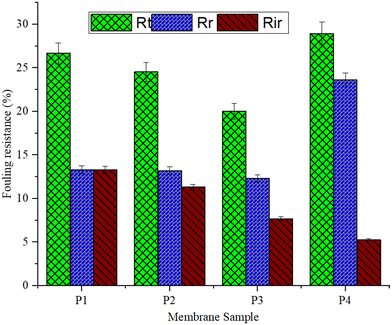 |
| Fig. 11 Antifouling performance of the membranes. | |
The flux recovery ratio (FRR) obtained after DI water cleaning corresponds with the results in ESI† Fig. S4. Due to the h-BN nanoparticle's high concentration and surface roughness in the PEI membrane, the FRR of the h-BN/PEI membrane is obtained at around 94.74%, which is significantly higher than the other PEI MMMs. The total fouling ratio (Rt), reversible fouling ratio (Rr), and irreversible fouling ratio (Rir) are calculated to determine the antifouling properties of the pure PEI and PEI-based MMMs. Fig. 11 shows the antifouling performance of the PEI-based MMMs. Using the MBR system backwashing operation, the particles of foulants deposited inside the membrane's pores could be easily removed.
5.3 PEI-based mixed matrix membrane reusability and stability performance
The reusability and stability performance of the pure PEI (P1), ZIF-8/PEI (P2), MoS2/PEI (P3) and h-BN/PEI (P4) MMMs are studied according to the same experimental conditions for antibiotic wastewater treatment shown in Fig. 12. In the present experimental study, the reusability and stability performance of the PEI MMMs are assessed in five successive cycles using antibiotic wastewater under the same experimental conditions. Further, the removal efficiency and stability of each PEI mixed matrix membrane are studied and calculated after each experiment. As shown in Fig. 12, the removal efficiency and stability of the h-BN/PEI mixed matrix membrane show a very stable and efficient performance for antibiotic wastewater treatment. This is because of the uniform distribution in the PEI membrane matrix, compatibility, chemical inertness, and thermal stability of h-BN nanoparticles.
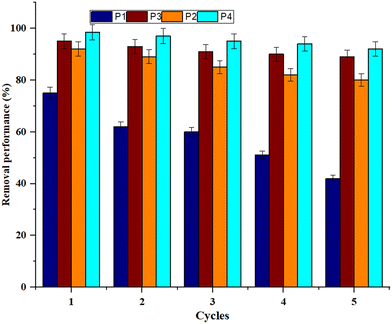 |
| Fig. 12 Reusability and stability performance of the PEI-based MMMs. | |
5.4 The comparison of the previous literature with the present study considering permeability and removal efficiency
In the SARS-CoV-19 pandemic, various types of antibiotics are used, but their retention in the human body and adverse effects on health are some side effects on human health. Some literature studies that reported antibiotic removal efficiencies are listed in Table 4, which shows the comparison between different nanocomposite membranes used for different antibiotic removal studies.
Table 4 Comparison with the previous literature
Membrane type |
Permeability (L m−2 h−1 bar−1) |
Type of antibiotic |
Removal efficiency (%) |
Ref. |
GA/PEI nanofiltration membrane |
78.6 |
Azithromycin |
71.5 |
43
|
74.3 |
Clindamycin phosphate |
81.3 |
68.2 |
Cephalexin |
85.3 |
MoS2/O-MWCNT/PES membrane |
41.3 |
Tobramycin |
96.2 |
44
|
64.1 |
Rifampicin |
97.5 |
PVDF–P(L-DOPA)–ZnO membrane |
60 |
Oxytetracycline |
71 |
45
|
GO/PES membrane |
75 |
Penicillin-G-procaine |
97 |
46
|
ZnO/N-g-C3N4/PVDF composite membrane |
— |
Tetracycline |
71.7 |
47
|
Ciprofloxacin |
80 |
Ofloxacin |
77 |
Pure PEI
|
21
|
Favipiravir
|
87.50
|
This study
|
ZIF-8/PEI
|
25.54
|
Azithromycin
|
88.89
|
MoS
2
/PEI
|
31.33
|
Ivermectin
|
92.61
|
h-BN/PEI
|
36.63 (for 25 cm
2
membrane area)
|
|
|
6. Conclusions
The preparation and functionalization of PEI membranes are carried out by incorporating a high nanoporous MOF (ZIF-8) and 2D nanomaterials (MoS2 and h-BN). The prepared MOF and 2D nanomaterial functionalized PEI membranes are used to study the antibiotic removal performance from synthetic wastewater. The functionalization of PEI membranes using MOF and 2D nanomaterials could alter the membranes' properties, including porosity, pore structure and shape, hydrophilicity, mechanical strength, dispersion of nanomaterials, and surface roughness. As a result, the ZIF-8/PEI, MoS2/PEI, and h-BN/PEI MMMs significantly increased water permeability compared to pure PEI membranes. Compared with the pure PEI membrane, the MOF and 2D nanomaterial functionalized PEI mixed matrix membranes improved membrane antifouling performance with low concentration in the casting solution (0.5 wt% ZIF-8, 1.5 wt% MoS2, and 2.5 wt% h-BN). The MBR system has achieved substantial antibiotic removal efficiency by employing functionalized PEI MMMs. The h-BN/PEI membrane shows excellent removal performance for favipiravir, azithromycin, and ivermectin. The h-BN/PEI membrane is proven to be a more significant membrane for favipiravir, azithromycin, and ivermectin removal based on the membrane's above characteristics and antibiotic removal performance.
Data availability
The data that support the findings of this study are available from the corresponding author upon reasonable request.
Author contributions
Amol V. Sonawane: conceptualization, methodology, software, validation, formal analysis, investigation, writing – review & editing. Z. V. P. Murthy: corresponding author, supervision, conceptualization, methodology, resources, writing – review & editing, data curation, project administration.
Conflicts of interest
The authors declare that they have no known competing financial interests or personal relationships that could have appeared to influence the work reported in this paper.
Acknowledgements
The authors declare that no funds, grants, or other support were received during the preparation of this manuscript. The first author (AVS) acknowledges the Ministry of Education, Government of India, for a doctoral fellowship. The authors acknowledge the Sophisticated Analytical Instrument Facility (SAIF), IIT Bombay, FIST (Physics)–IRCC SPM Central Facility of IIT Bombay, and Malaviya National Institute of Technology (MNIT) Jaipur for providing the characterization facilities.
References
- M. Achak, S. Alaoui Bakri, Y. Chhiti, F. E. M'hamdi Alaoui, N. Barka and W. Boumya, SARS-CoV-2 in hospital wastewater during outbreak of COVID-19: A review on detection, survival and disinfection technologies, Sci. Total Environ., 2021, 761, 143192, DOI:10.1016/j.scitotenv.2020.143192.
- A. Majumder, A. K. Gupta, P. S. Ghosal and M. Varma, A review on hospital wastewater treatment: A special emphasis on occurrence and removal of pharmaceutically active compounds, resistant microorganisms, and SARS-CoV-2, J. Environ. Chem. Eng., 2021, 9, 104812, DOI:10.1016/j.jece.2020.104812.
- T. Rasheed, M. Bilal, A. A. Hassan, F. Nabeel, R. N. Bharagava, L. F. Romanholo Ferreira, H. N. Tran and H. M. N. Iqbal, Environmental threatening concern and efficient removal of pharmaceutically active compounds using metal-organic frameworks as adsorbents, Environ. Res., 2020, 185, 109436, DOI:10.1016/j.envres.2020.109436.
- X. L. Yang, J. Y. Xu, H. L. Song, X. Wang and T. Li, Enhanced removal of antibiotics in wastewater by membrane bioreactor with addition of rice straw, Int. Biodeterior. Biodegrad., 2020, 148, 104868, DOI:10.1016/j.ibiod.2019.104868.
- Y. X. Lu, H. L. Song, H. Chand, Y. Wu, Y. L. Yang and X. L. Yang, New insights into the role of molecular structures on the fate and behavior of antibiotics in an osmotic membrane bioreactor, J. Hazard. Mater., 2022, 423, 127040, DOI:10.1016/j.jhazmat.2021.127040.
- D. Cheng, H. H. Ngo, W. Guo, Y. Liu, S. W. Chang, D. D. Nguyen, L. D. Nghiem, J. Zhou and B. Ni, Anaerobic membrane bioreactors for antibiotic wastewater treatment: Performance and membrane fouling issues, Bioresour. Technol., 2018, 267, 714–724, DOI:10.1016/j.biortech.2018.07.133.
- F. Parveen and N. Hankins, Integration of Forward Osmosis Membrane Bioreactor (FO-MBR) and Membrane Distillation (MD) units for water reclamation and regeneration of draw solutions, J. Water Process Eng., 2021, 41, 102045, DOI:10.1016/j.jwpe.2021.102045.
- D. D. Kachhadiya and Z. V. P. Murthy, Preparation and characterization of ZIF-8 and ZIF-67 incorporated poly (vinylidene fluoride) membranes for pervaporative separation of methanol/water mixtures, Mater. Today Chem., 2021, 22, 100591, DOI:10.1016/j.mtchem.2021.100591.
- A. R. Kamble, C. M. Patel and Z. V. P. Murthy, Different 2D materials based polyetherimide mixed matrix membranes for CO2/N2 separation, J. Ind. Eng. Chem., 2020, 81, 451–463, DOI:10.1016/j.jiec.2019.09.035.
- A. R. Kamble, C. M. Patel and Z. V. P. Murthy, Effects of inorganic additive of two-dimensional hexagonal boron nitride on the gas separation/permeation for PVDF-derived membranes, Sep. Sci. Technol., 2019, 54, 1489–1501, DOI:10.1080/01496395.2019.1577451.
- Z. Xu, X. Song, Y. Li, G. Li and W. Luo, Removal of antibiotics by sequencing-batch membrane bioreactor for swine wastewater treatment, Sci. Total Environ., 2019, 684, 23–30, DOI:10.1016/j.scitotenv.2019.05.241.
- V. Cao, A. Yunessnia lehi, M. Bojaran and M. Fattahi, Treatment of Lasalocid A, Salinomycin and Semduramicin as ionophore antibiotics in pharmaceutical wastewater by PAMAM-coated membranes, Environ. Technol. Innovation, 2020, 20, 101103, DOI:10.1016/j.eti.2020.101103.
- S. Naraginti, Y. Y. Yu, Z. Fang and Y. C. Yong, Visible light degradation of macrolide antibiotic azithromycin by novel ZrO2/Ag@TiO2 nanorod composite: Transformation pathways and toxicity evaluation, Process Saf. Environ. Prot., 2019, 125, 39–49, DOI:10.1016/j.psep.2019.02.031.
- S. Joshi, J. Parkar, A. Ansari, A. Vora, D. Talwar, M. Tiwaskar, S. Patil and H. Barkate, Role of favipiravir in the treatment of COVID-19, Int. J. Infect. Dis., 2021, 102, 501–508, DOI:10.1016/j.ijid.2020.10.069.
- K. Huraimel, M. Alhosani, S. Kunhabdulla and M. H. Stietiya, SARS-CoV-2 in the environment: Modes of transmission, early detection and potential role of pollutions, Sci. Total Environ., 2020, 744, 140946, DOI:10.1016/j.scitotenv.2020.140946.
- M. L. V. Maier and R. S. Tjeerdema, Azithromycin sorption and biodegradation in a simulated California river system, Chemosphere, 2018, 190, 471–480, DOI:10.1016/j.chemosphere.2017.10.008.
- A. M. A. Adam, H. A. Saad, A. M. Alsuhaibani, M. S. Refat and M. S. Hegab, Charge-transfer chemistry of azithromycin, the antibiotic used worldwide to treat the coronavirus disease (COVID-19). Part I: Complexation with iodine in different solvents, J. Mol. Liq., 2021, 325, 115187, DOI:10.1016/j.molliq.2020.115187.
- Y. Wang, M. Gong, X. Wang, X. Peng, Y. Wang, J. Guan, D. Cheng, C. Weng and Y. Zheng, Efficient degradation of ivermectin by newly isolated Aeromonas taiwanensis ZJB-18,044, Biodegradation, 2020, 31, 275–288, DOI:10.1007/s10532-020-09909-8.
- F. K. Kosyna, M. Nagel, L. Kluxen, K. Kraushaar and R. Depping, The importin α/β-specific inhibitor Ivermectin affects HIF-dependent hypoxia response pathways, Biol. Chem., 2015, 396, 1357–1367, DOI:10.1515/hsz-2015-0171.
- D. N. Rani, K. S. Kokilambigai and K. S. Lakshmi, Multivariate calibration technique for the spectrophotometric quantification of Ivermectin in pharmaceutical formulation, Asian J. Pharm. Clin. Res., 2019, 29416, DOI:10.22159/ajpcr.2019.v12i2.29416.
- A. Yazdani and M. H. Sayadi, Sonochemical degradation of azithromycin in aqueous solution, Environ. Health Eng. Manage. J., 2018, 5, 85–92, DOI:10.15171/ehem.2018.13.
- R. P. Nippes, P. D. Macruz, G. N. da Silva and M. H. Neves Olsen Scaliante, A critical review on environmental presence of pharmaceutical drugs tested for the covid-19 treatment, Process Saf. Environ. Prot., 2021, 152, 568–582, DOI:10.1016/j.psep.2021.06.040.
- A. V. Sonawane and Z. V. P. Murthy, Synthesis, characterization, and application of ZIF-8/Ag3PO4, MoS2/Ag3PO4, and h-BN/Ag3PO4 based photocatalytic nanocomposite polyvinylidene fluoride mixed matrix membranes for effective removal of drimaren orange P2R, J. Membr. Sci., 2022, 641, 119939, DOI:10.1016/j.memsci.2021.119939.
- U. Sathya and M. Nithya, Keerthi, Fabrication and characterization of fine-tuned Polyetherimide (PEI)/WO3 composite ultrafiltration membranes for antifouling studies, Chem. Phys. Lett., 2020, 744, 137201, DOI:10.1016/j.cplett.2020.137201.
- S. Benkhaya, S. M'rabet, R. Hsissou and A. el Harfi, Synthesis of new low-cost organic ultrafiltration membrane made from Polysulfone/Polyetherimide blends and its application for soluble azoic dyes removal, J. Mater. Res. Technol., 2020, 9, 4763–4772, DOI:10.1016/j.jmrt.2020.02.102.
- A. Karimi, A. Khataee, V. Vatanpour and M. Safarpour, The effect of different solvents on the morphology and performance of the ZIF-8 modified PVDF ultrafiltration membranes, Sep. Purif. Technol., 2020, 253, 117548, DOI:10.1016/j.seppur.2020.117548.
- A. V. Sonawane and Z. V. P. Murthy, Synthesis and characterization of ZIF-8-based PVDF mixed matrix membranes and application to treat pulp and paper industry wastewater using a membrane bioreactor, Environ. Sci.: Water Res. Technol., 2022, 8, 881–896, 10.1039/d2ew00011c.
- S. Saki and N. Uzal, Preparation and characterization of PSF/PEI/CaCO3 nanocomposite membrane for oil/water separation, Environ. Sci. Pollut. Res., 2018, 25, 25315–25326, DOI:10.1007/s11356-018-2615-9.
- A. Karimi, A. Khataee, V. Vatanpour and M. Safarpour, High-flux PVDF mixed matrix membranes embedded with size-controlled ZIF-8 nanoparticles, Sep. Purif. Technol., 2019, 229, 115838, DOI:10.1016/j.seppur.2019.115838.
- Y. Zhang, L. Luo, Z. Shi, X. Shen, C. Peng, J. Liu, Z. Chen, Q. Chen and L. Zhang, Synthesis of MoS2/CdS Heterostructures on Carbon-Fiber Cloth as Filter-Membrane-Shaped Photocatalyst for Purifying the Flowing Wastewater under Visible-Light Illumination, ChemCatChem, 2019, 11, 2855–2863, DOI:10.1002/cctc.201900542.
- R. Chandra and M. Nath, Controlled synthesis of AgNPs@ZIF-8 composite: Efficient heterogeneous photocatalyst for degradation of methylene blue and congo red, J. Water Process Eng., 2020, 36, 101266, DOI:10.1016/j.jwpe.2020.101266.
- T. P. Nguyen and I. T. Kim, Ag nanoparticle-decorated MoS2 nanosheets for enhancing electrochemical performance in lithium storage, Nanomaterials, 2021, 11, 1–13, DOI:10.3390/nano11030626.
- Z. Shi, G. Lu, P. Yang, T. Wu, W. Yin, C. Zhang, R. Jiang and X. Xie, Controlled synthesis of uniform multilayer hexagonal boron nitride films on Fe2B alloy, RSC Adv., 2019, 9, 10155–10158, 10.1039/c9ra00595a.
- H. Zhou, J. Zhu, Z. Liu, Z. Yan, X. Fan, J. Lin, G. Wang, Q. Yan, T. Yu, P. M. Ajayan and J. M. Tour, High thermal conductivity of suspended few-layer hexagonal boron nitride sheets, Nano Res., 2014, 7, 1232–1240, DOI:10.1007/s12274-014-0486-z.
- L. Goswami, R. Vinoth Kumar, S. N. Borah, N. Arul Manikandan, K. Pakshirajan and G. Pugazhenthi, Membrane bioreactor and integrated membrane bioreactor systems for micropollutant removal from wastewater: A review, J. Water Process Eng., 2018, 26, 314–328, DOI:10.1016/j.jwpe.2018.10.024.
- T. K. Q. Vo, X. T. Bui, S. S. Chen, P. D. Nguyen, N. D. T. Cao, T. D. H. Vo, T. T. Nguyen and T. B. Nguyen, Hospital wastewater treatment by sponge membrane bioreactor coupled with ozonation process, Chemosphere, 2019, 230, 377–383, DOI:10.1016/j.chemosphere.2019.05.009.
- T. T. Nguyen, X. T. Bui, V. P. Luu, P. D. Nguyen, W. Guo and H. H. Ngo, Removal of antibiotics in sponge membrane bioreactors treating hospital wastewater: Comparison between hollow fiber and flat sheet membrane systems, Bioresour. Technol., 2017, 240, 42–49, DOI:10.1016/j.biortech.2017.02.118.
- M. Yao, L. Duan, Y. Song and S. W. Hermanowicz, Degradation mechanism of Ibuprofen via a forward osmosis membrane bioreactor, Bioresour. Technol., 2021, 321, 124448, DOI:10.1016/j.biortech.2020.124448.
- M. Yao, L. Duan, J. Wei, F. Qian and S. W. Hermanowicz, Carbamazepine removal from wastewater and the degradation mechanism in a submerged forward osmotic membrane bioreactor, Bioresour. Technol., 2020, 314, 123732, DOI:10.1016/j.biortech.2020.123732.
- T. T. Zhu, Z. X. Su, W. X. Lai, Y. B. Zhang and Y. W. Liu, Insights into the fate and removal of antibiotics and antibiotic resistance genes using biological wastewater treatment technology, Sci. Total Environ., 2021, 776, 145906, DOI:10.1016/j.scitotenv.2021.145906.
- P. Zhu, M. Duan, R. Wang, J. Xu, P. Zou and H. Jia, Facile synthesis of ZnO/GO/Ag3PO4 heterojunction photocatalyst with excellent photodegradation activity for tetracycline hydrochloride under visible light, Colloids Surf., A, 2020, 602, 125118, DOI:10.1016/j.colsurfa.2020.125118.
- V. Vatanpour, A. Ghadimi, A. Karimi, A. Khataee and M. E. Yekavalangi, Antifouling polyvinylidene fluoride ultrafiltration membrane fabricated from embedding polypyrrole coated multiwalled carbon nanotubes, Mater. Sci. Eng., 2018, 89, 41–51, DOI:10.1016/j.msec.2018.03.026.
- X. Q. Cheng, Z. X. Wang, Y. Zhang, Y. Zhang, J. Ma and L. Shao, Bio-inspired loose nanofiltration membranes with optimized separation performance for antibiotics removals, J. Membr. Sci., 2018, 554, 385–394, DOI:10.1016/j.memsci.2018.03.005.
- S. Arefi-Oskoui, A. Khataee, S. J. Behrouz, V. Vatanpour, S. H. Gharamaleki, Y. Orooji and M. Safarpour, Development of MoS2/O-MWCNTs/PES blended membrane for efficient removal of dyes, antibiotic, and protein, Sep. Purif. Technol., 2022, 280, 119822, DOI:10.1016/j.seppur.2021.119822.
- A. Popa, D. Toloman, M. Stefan, A. Petran, S. Macavei, S. Ulinici, M. Stan, L. Barbu-Tudoran, M. Vlassa and O. Pana, Hybrid PVDF-P(L-DOPA)-ZnO membranes for dyes and antibiotics removal through simultaneous action of adsorption and photocatalysis processes, J. Environ. Chem. Eng., 2021, 9, 106812, DOI:10.1016/j.jece.2021.106812.
- S. Valizadeh, L. Naji and M. Karimi, Controlling interlayer spacing of graphene oxide membrane in aqueous media using a biocompatible heterobifunctional crosslinker for Penicillin-G Procaine removal, Sep. Purif. Technol., 2021, 263, 118392, DOI:10.1016/j.seppur.2021.118392.
- F. Wang, Z. Chen, Z. Zhu and J. Guo, Construction of visible light responsive ZnO/N-g-C3N4 composite membranes for antibiotics degradation, J. Mater. Res. Technol., 2022, 17, 1696–1706, DOI:10.1016/j.jmrt.2022.01.140.
|
This journal is © The Royal Society of Chemistry 2023 |