DOI:
10.1039/D3BM00387F
(Review Article)
Biomater. Sci., 2023,
11, 4774-4788
Structural and componential design: new strategies regulating the behavior of lipid-based nanoparticles in vivo
Received
4th March 2023
, Accepted 14th May 2023
First published on 15th May 2023
Abstract
Lipid-based nanoparticles have made a breakthrough in clinical disease as delivery systems due to their biocompatibility, thermal and long-term stability, high loading ability, simplicity of preparation, inexpensive production costs, and scalable manufacturing production. In particular, during the COVID-19 pandemic, this delivery system served as a vital vaccine component for virus confrontation. To obtain effective drug delivery, lipid-based nanoparticles should reach the desired sites with high efficiency, enter target cells, and release drugs. The structures and compositions of lipid-based nanoparticles can be modified to regulate these behaviors in vivo to enhance the therapeutic effects. Herein, we briefly review the development of lipid-based nanoparticles, from simple self-assembled nanovesicle-structured liposomes to multifunctional lipid nanoparticles. Subsequently, we summarize the strategies that regulate their tissue distribution, cell internalization, and drug release, highlighting the importance of the structural and componential design. We conclude with insights for further research to advance lipid-based nanotechnology.
 Mingqiang Li | Mingqiang Li is a professor of molecular medicine at Sun Yat-sen University. He received his B.S. degree from the University of Science and Technology of China in 2009 and obtained his Ph.D. degree under the supervision of Prof. Xuesi Chen from the Changchun Institute of Applied Chemistry, Chinese Academy of Sciences, in 2015. From 2015 to 2018, he carried out postdoctoral research with Prof. Kam W. Leong at Columbia University. He serves on the editorial board of Biomaterials, Journal of Tissue Engineering, Chinese Chemical Letters, and Med-X. His current research is mainly focused on biomaterials and nanomedicines. |
Introduction
Lipid-based nanoparticles, assembled from lipid molecules containing an alkyl tail coupled with a hydrophilic group, have received attention in drug delivery. These nanoparticles can transport hydrophobic and hydrophilic molecules to the target sites and increase the duration of drug action by prolonging the dug half-life through controlled release. Compared with other nanosized carriers like polymeric and inorganic nanoparticles, lipid-based nanoparticles are dominant in features such as low systemic toxicity and high water solubility. Meanwhile, they also exhibit the advantages of thermal and long-term stability, high loading ability, simple preparation, inexpensive production costs, and scalable manufacturing production.1–4 To date, lipid-based nanoparticles have served as the most common type of nanomedicine approved by the Food and Drug Administration (FDA). Notably, they have attracted attention as a key constituent of COVID-19 vaccines that efficiently protect and transport mRNAs into cells.5–7
Lipid-based nanoparticles have been studied for decades, with novel features added iteratively. Their earliest version is a liposome, which has a vesicular structure that can transport both hydrophobic and hydrophilic molecules. The second generation is mainly lipid nanoparticles (LNPs) with a micelle-like structure. Compared to liposomes, they have a complicated inner construction and improved physical stability to regulate the distribution and duration of drug action in vivo. The recently-advanced lipid-based nanoparticles, lipid–polymer hybrid nanoparticles (LPNPs), conceptively originating from liposomes and polymeric nanoparticles, are constructed as a core–shell nanostructure with strong designability for drug delivery. When applied in vivo, these nanoparticles reach the desired sites, enter targeted cells, and release drugs. Rational design of the structures and lipid components of lipid-based nanoparticles could regulate the efficiency of each process to optimize the drug efficacy sufficiently.8 Surface modifications, including target motifs with specific lipid components, can improve the targeting ability to specific sites, increasing the applicability of lipid-based nanoparticles. Meanwhile, incorporating specific head groups in lipid molecules may promote the lysosomal escape of nanoparticles to avoid degradation after endocytosis. Even fusogenic lipids enable the nanoparticles to fuse with the cell membrane to deliver cargo directly into the cytoplasm without passing lysosomes. Moreover, they can also be formulated using lipid molecules responsive to the external stimuli to improve drug release in a controllable manner.
Herein, this review provides an overview of the new strategies regulating the behavior of lipid-based nanoparticles in vivo, focusing on ways to improve their structural and componential design (Fig. 1). We summarize the development of lipid-based nanoparticles from simply self-assembled nanovesicle-structured liposomes to multifunctional lipid nanoparticles. Meanwhile, we also emphasize how to design the structures and compositions of lipid-based nanoparticles to regulate their tissue distribution, cell internalization, and drug release in vivo. We conclude with insights for further research to advance lipid-based nanotechnology.
 |
| Fig. 1 Strategies regulating the behavior of lipid-based nanoparticles in vivo. The surface modification of targeting ligands and the selection of specific lipid components can achieve the specific distribution in the target tissues. After reaching the targeted tissues, the intracellular delivery to the cytoplasm is designed by endocytosis, penetration, and membrane fusion. In the cytoplasm, the loaded drugs are released to unleash the therapeutic activity through endosomal escape and stimuli-responsive release. | |
Development of lipid-based nanoparticles
Bangham et al. discovered the first lipid-based nanoparticle when dispersing phospholipids in water for electron microscopy.9 These phospholipids naturally formed multi-layered vesicles in water, and each layer was a lipid bilayer with a thickness of about 4 nm. In the 1970s, liposomes were applied to convey active pharmaceutical agents for the first time by encapsulating hydrophobic or hydrophilic drugs with simple vesicular formulations due to their amphipathic properties.10 Subsequently, Gabizon et al. for the first time reported the formation of liposomal doxorubicin (DOX) for anticancer drug delivery,116 which was approved by the FDA in 1995 as a breakthrough in cancer nanomedicine. Subsequently, more and more cancer nanomedicines with liposomal or other lipid-based formulations have succeeded in clinical trials and been translated into commercial products.12 To date, more than twenty liposomal products have been approved, such as Doxil®, DaunoXome®, Depocyt®, Myocet®, Lipodox®, Onivyde®, Vyxeos®, and Lipoplatin™, in which different small molecule drugs are encapsulated. There are also many other liposomes indicated for chemotherapy in clinical trials currently, such as MBP-426®, ThermoDox®, MM-302, SPI-77, and OSI-211.12 Beyond the delivery of anticancer drugs, lipid-based nanoparticles also show promise for vaccine delivery because they can readily protect active vaccinal composites such as antigens, immunologic adjuvants, proteins, and RNAs, resulting in improved vaccine pharmacokinetics and targeted delivery.12,13 In 1974, liposomes were first used for vaccine delivery, and in 1989 a cationic lipid was reported to transport nucleic acids in vitro. The first-in-human trial for a lipid-based nanoparticle mRNA vaccine was initiated in 2014. In late 2020, lipid-based-nanoparticle-formulated vaccines shined in the COVID-19 pandemic, during which two COVID-19 mRNA vaccines, BNT162b2 and mRNA-1273, using two different ionizable lipids, respectively, obtained emergency use authorization against the virus.6,14 So far, lipid-based nanoparticles have been extended to deliver various bioactive pharmaceutical ingredients, including chemical therapeutics, proteins, antibodies, and nucleic acids.15
To accommodate these wide applications, the structures and compositions of lipid-based nanoparticles were engineered with intentional diversity for specific functionalities to contribute to drug loading and delivery. Liposomes, the first commonly-used lipid-based nanoparticles, are formulated with a multilayer-based vesicle structure composed of amphipathic natural or synthetic phospholipids and sphingolipids. These lipid molecules have a lipophilic tail and hydrophilic head. During manufacturing, the polar hydrophilic heads are oriented toward the aqueous medium. The hydrophobic lipophilic tails are assembled toward the interior, creating a membrane structure.16,17 This lipid membrane forms a closed vesicle that eventually encapsulates an interior aqueous region. Characterized by a hydrophilic lipid layer and an aqueous cavity, liposomes are suitable for delivering hydrophobic and hydrophilic drugs.18 Moreover, other membrane bilayer constituents can also be introduced to improve the particles’ in vivo performance.11 For example, introducing cholesterol into liposomes improves the fluidity and stability of the membranous bilayer in biological fluids and reduces the penetrability of soluble molecules into the interior.19 Polyethylene glycol (PEG) is usually incorporated into liposome surfaces to improve stability. It provides steric hindrance that reduces interactions with plasma proteins.20,21 Therefore, PEGylation prolongs liposome circulation, increasing their accumulation within targeted sites. Besides, natural substances, for instance, chitosan, can be incorporated into liposomes via an electrostatic interaction to provide high stability.22 Furthermore, some groups also reported cross-linked liposomes with covalently crosslinking inter-lipid bilayer or membrane bilayer constituents, showing greatly improved structural integrity and association of the lipid bilayer, enhanced serum-stability, and better drug release behavior, providing a more robust drug delivery system.23–26 Despite these efforts to enhance the performance, liposomes still have the disadvantages of insufficient stability, low loading encapsulation capacity, unavoidable usage of organic solvents, and uncontrollable drug release.
Another formulation of lipid-based nanoparticles, LNPs, was designed in the 1990s. They have a micelle-like structure, encapsulating therapeutic drugs in the hydrophobic lipid core. Solid lipid nanoparticles (SLNs) are the first generation of LNPs, and the nanostructured lipid carrier (NLC) is the second. SLNs, regarded as lipospheres or solid lipid nanospheres, are formulated using a sort of lipids with a solid phase at room temperature or body temperature.27 SLNs have shown great potential in drug delivery due to their easy preparation, physical stability, labile ingredient protection, and controllable release.28 However, they have low encapsulation of hydrophilic drugs due to inadequate dissolution within the melted lipid droplets.29 They also display nonuniform drug release due to the burst release effect, a quick premier release of a large proportion of drugs following a slow and imperfect release. To avoid burst release, NLCs were developed in 1999. Unlike SLNs, NLCs are prepared by mixing solid and liquid lipids or oil.27 Incorporating liquid lipids into the solid lipid matrix remarkably improves the drug loading ability and avoids drug immobilization. Thus, these lipidic mixtures allow poorly-structured lipid crystals to form a homogeneous phase, leading to uniform drug encapsulation without rapid leakage from the carriers.30 The complicated inner lipid constructions allow LNPs to be more favorable as loading agents with temporal stability and provide a platform for incorporating functional units to endow LNPs with desired properties, such as stimuli-responsive degradation.
More recently, advanced new generation of lipid-based nanoparticles, LPNPs, which combine the properties of polymeric nanoparticles and liposomes, has garnered significant interest. These LPNPs have a core–shell structure. The core comprises a polymer encapsulating therapeutic substances surrounded by a lipid layer shell.31–33 The lipid layer shell confers biocompatibility to the LPNPs and prevents the encapsulated contents from leaking. The stealthy PEGylated lipid on the surfaces can also enhance their in vivo circulation. Due to their hybrid structure, LPNPs exhibit great structural uniformity, stability, and controllable release because of the polymeric core, as well as good biocompatibility and bioavailability attributed to the lipid shells.34 More importantly, LPNPs can even be designed with an attractive membrane-fusion capacity using specific lipid composites to directly deliver cargoes into the cytoplasm.35 This new type of LPNPs is distinguished from any current lipid-based nanoparticles, which traffick into cells through an endocytosis pathway. Despite the great promise, LPNPs remain under exploration and are not ubiquitous. It is difficult to produce consistent LPNPs with controlled size and homogeneity on a large scale. It is also difficult to maintain the physical characteristics after long-term storage. The development of these lipid-based nanoparticles is summarized in Fig. 2 and Table 1.
 |
| Fig. 2 Schematic representation of four different types of lipid-based nanoparticles: liposomes, solid lipid nanoparticles, nanostructured lipid carriers, and lipid–polymer hybrid nanoparticles. | |
Table 1 Summary of the main characteristics of lipid-based nanoparticles
Particle |
Composition |
Preparation |
Advantages |
Disadvantages |
Ref. |
Liposomes |
Phospholipids, cholesterol |
Solvent evaporation, solvent dispersion, reverse phase evaporation |
Solubility for hydrophobic drugs, high bioavailability and biodistribution |
Insufficient stability, low encapsulation capacity, uncontrollable drug release |
16–18
|
Solid lipid nanoparticles |
Phospholipids, solid lipids |
High-pressure homogenization, emulsion/solvent evaporation, double emulsion, phase inversion |
Solubility for hydrophobic drugs, high bioavailability and biodistribution |
Low loading of hydrophilic drugs, nonuniform drug release |
27–29
|
Nanostructured lipid carriers |
Phospholipids, solid lipids, liquid lipids |
High-pressure homogenization, emulsion/solvent evaporation, double emulsion, phase inversion |
Improved drug retention, enhanced drug loading capacity |
Optimization required of the ratio of solid/liquid lipids |
27 and 30 |
Lipid–polymer hybrid nanoparticles |
Ionizable lipids, phospholipids, cholesterol, PEGylated lipids, polymeric core |
Film hydration, emulsification–solvent-evaporation, nanoprecipitation |
High structural integrity, great stability, controlled release, high biocompatibility and bioavailability |
Uncontrolled size and homogeneity, unstable long-term storage |
27, 30–32 and 34 |
Distribution regulation
In general, the distribution of lipid-based nanoparticles is an important factor deserving careful consideration as it determines the effect of loaded drugs. Initially, the parameters of lipid-based nanoparticles are taken into account to optimize their behavior in the body. Prolonged circulation is beneficial for biodistribution and enhances passive accumulation in targeted sites to increase the effectiveness.11 Subsequently, engineering lipid-based nanoparticles with specific targets has become an attractive strategy not only to increase therapeutic selectivity to target tissues but also to minimize toxicity against other sites. Endowing lipid-based nanoparticles with targeting abilities currently rely on modifying surface targeting motifs, and specific lipid composition doping is a rising targeting approach.36,37
Nanoparticle characteristic regulation
The intrinsic characteristics and properties of lipid-based nanoparticles affect their distribution in a passive way. Size is one of the most key parameters which regulates the distribution and clearance of lipid-based nanoparticles. There are rapid urinary excretion and renal elimination when the hydrodynamic diameter of particles is below 5.5 nm.38 When the hydrodynamic diameter of particles is over 200 nm, its intravascular clearance occurs via splenic filtration and hepatic sequestration. In most cases, the size is optimized between 100 and 300 nm to show acceptable circulation and accumulation.11 To control the size, there are lots of techniques available including sonication, freeze–thaw, homogenization, and extrusion.16 Moreover, to reduce the clearance known as opsonization, PEGylation is applied to lipid-based nanoparticles to decrease the nonspecific binding of plasma proteins to enhance the circulation half-life.30 Surface charge is another important factor that affects the distribution of lipid-based nanoparticles. It was reported that the surface charge controls the biodistribution of nanoparticles by influencing their stability and interaction with the microenvironment. Specifically, the nanoparticles would aggregate when close to neutral, while they are easily phagocytosed by macrophages with a high percentage of positive charge.41 Hence, the negatively charged lipid-based nanoparticles may exhibit better results. In addition, the morphology of nanoparticles also affects their distribution via determining the stability, size, and size distribution.41,42 In conclusion, the distribution of lipid-based nanoparticles needs to consider comprehensively multiple parameters determining the characteristics and properties under diverse conditions.
Targeting ligand modification
Traditional specific targeting is achieved by grafting the ligand on the surface of lipid-based nanoparticles, which could recognize the molecules present on the specific cells in a selective way. These targeting ligands include peptides, antibodies, antibody fragments, nanobodies, aptamers, nucleic acids, and small molecules.36,43 The choice of ligands relies on the targeted cell types, properties of lipid-based nanoparticles, and molecular size. The ways to graft ligands onto lipid nanoparticles commonly consist of noncovalent or covalent surface modification. Noncovalent techniques to decorate lipid nanoparticles with targeting ligands include lipid anchoring, ionic interactions, and biological interactions.44 Lipid anchoring is based on inserting a lipid composition previously coupled with a ligand-capping polymer onto the lipid nanoparticles to achieve surface modification. These lipid anchors mainly contain phospholipids, cholesterol, and single-chain fatty acids.45 Another standard method of ionic interaction is adsorbing these targeting motifs on the lipid-based nanoparticle surfaces through electrostatic or chelating interaction. In electrostatic adsorption, charged nanoparticles are paired with an oppositely charged ligand.46,47 Chelating interaction relies on the binding between nickel ions (Ni2+) with His-tags connected with a targeting ligand.48 Biological interaction relies on the specific recognition of biotin–streptavidin49 and folate–folate binding proteins.50 Covalent grafting of the targeting ligands on lipid-based nanoparticles is more stable and adjustable compared with noncovalent coupling. Covalent surface modification is achieved through reactions between ligands and reactive head groups at the end of PEGylated lipids to form a bond–bond association.51 Bond–bond links are formed through reactions including amide-bonds between an amine and a carboxylic group,52 thioether-bond via the Michael addition reaction,53 secondary amine bond reduced by Schiff bases with aldehyde groups,54 isothiourea bonds by reaction of an aldehyde group with an isothiocyanate,55 triazole bonds formed by click chemistry.56 Using a cross-linking agent is also a strategy to conjugate lipid nanoparticles with ligands from two adapted reactive groups.58 Both of these two grafting techniques have their merits. Noncovalent modification is easy and simple to handle and could be accomplished under mild conditions. However, ionic interactions are unstable and have risks of dissociation, toxicity, and clearance. Lipid anchoring and biological interactions are more stable but require coupling beforehand with ligands which may impair their biological characteristics. Compared with noncovalent modification strategies, covalent modifications rely on the specific reaction conditions to conjugate the ligands and offers certain selectivity and specificity. Meanwhile, covalent modifications are more flexible for targeted ligands.
Structures and composition optimization
Introducing active targeting ligands to lipid-based nanoparticles can precisely deliver cargo to specific sites in vivo. However, this causes complexity in the delivery system, lowering the manufacturing pace.59 Hence, significant efforts have been extended to exploit the lipid delivery systems without targeting ligands. Typically, lipid-based nanoparticles for drug delivery contain four lipid components: phospholipid, cholesterol, PEGylated lipid, and ionizable lipid. By optimizing their structures and ratios, selective organ targeting can be achieved. Xu's team reported a variety of LNPs that could specifically target the spleen (Fig. 3),39 liver,60 lymph nodes,61 and lungs,62 respectively through library screening approaches rather than targeting ligands. They established the library of synthetic ionizable lipids with different chemical structures and added them to the other three components (phospholipids, cholesterol, and PEGylated lipids). They found that the amine heads and the hydrophobic chain length in the ionizable lipids played important roles in mRNA transportation efficacy in vivo. The insertion of imidazole-based synthetic lipidoids tended to deliver mRNAs into the spleen, O-series LNPs that contained an ester bond in the tail selectively accumulated mRNAs in the liver, while N-series LNPs which included an amide bond in the tail preferentially targeted mRNAs to the lungs.62 In the study of lymph node-targeting delivery, they ulteriorly developed the lipid molecule libraries according to the structure of side groups in head amines, linker types, tail lengths, and tail combinations.15,61 There was a higher expression in the lymph nodes delivered by lipidoids containing a shorter length tail than that with a longer tail. At the same time, the lipidoids which contained the ester bond linkers had an enhanced delivery efficacy compared with amide bond linkers. Moreover, the methyl group of the head amine could enhance the target capacity of LNPs compared with other groups.61 In addition, the ratios of these four constituents should be optimized for improved delivery efficacy.
 |
| Fig. 3 The primary T lymphocyte targeted delivery by selected lipidoid with specific chemical structures. (A) Schematic illustration of selecting imidazole-containing lipidoids for the primary T cell delivery through structure-based rough-to-detailed screening. (B) Screening of lipid nanoparticles (LNPs) from lipidoids library based on the structures of head groups and tails. (C) mRNA delivery and biodistribution of selected LNPs in vivo. Reproduced with permission.39 Copyright 2020, Wiley-VCH. | |
Rather than conceiving the chemical structure and optimizing four lipid components, adding a fifth component can also alter the in vivo organ-targeting properties of LNPs. Cheng et al. added a fifth selective-organ-targeting lipid molecule to allow LNPs to deliver to mice's lungs, spleen, and livers (Fig. 4).40 After screening the structures of these molecules and optimizing their ratios, the lipid-based nanoparticles with tissue-specific delivery were developed, including a liver-targeted LNP containing 20% 1,2-dioleoyl-3-dimethylammonium-propane (DODAP), lung-targeted LNP composed of 50% 1,2-dioleoyl-3-trimethylammonium-propane (DOTAP), and spleen-targeted LNP doped with 30% 1,2-dioleoyl-sn-glycero-3-phosphate (18PA). The chemical structure of these selective organ targeting molecules uniquely impacted the specific blood proteins recruited onto the nanoparticle surfaces. These proteins adsorbed on the surface governed their ultimate fate in the body through the interaction with homologous receptors expressed on the cells in targeting tissue, thereby facilitating effective mRNA delivery towards these specific organs.63 With the identification of these critical parameters, organ-targeted LNPs could be tuned with more accuracy of organ-selective delivery. Luozhong et al. introduced 1,2-dioleoyl-sn-glycero-3-phospho-L-serine (DOPS) to a classical four-constituent MC3 LNP to form a secondary lymphoid organ (SLO)-targeting formulation (Fig. 5).57 Phagocytes recognized the membrane molecule phosphatidylserine, allowing the LNP system to be actively internalized by specific cells in SLOs, resulting in biomimetic and effective SLO-targeting mRNA delivery.
 |
| Fig. 4 Selective organ targeting LNPs for tissue-specific mRNA delivery. (A) Schematic illustration of tissue-specific activity through adding fifth selective organ targeting molecules to conventional four-component LNPs. (B) Tissue-targeted delivery is achieved by selective organ-targeting molecules based on general biophysical properties rather than exact chemical structures. (C) Selective organ-targeting LNPs enable delivery to mediate organ-specific gene editing. Reproduced with permission.40 Copyright 2020, Springer Nature. | |
 |
| Fig. 5 Specific delivery to secondary lymphoid organs (SLOs) using phosphatidylserine LNP (PS-LNP). (A) Schematic illustration of PS-LNP achieving effective SLO-targeting mRNA delivery. (B) Fluorescent images show the specific distribution of PS-LNP in the spleens and lymph nodes after systemic delivery. Reproduced with permission.57 Copyright 2022, American Chemical Society. | |
Intracellular delivery
Besides the selectivity toward target tissues, the ability of lipid-based nanoparticles to cross the cell membrane to access the cytoplasm is also associated with the eventual therapeutic efficiency. The intracellular delivery of lipid-based nanoparticles is mainly accomplished in three ways including endocytosis, penetration, and membrane fusion.
Endocytosis
Endocytosis is the most common type of lipid-based nanoparticle uptake. Researchers have tried various means to promote endocytosis to increase the intracellular delivery of therapeutics. Cationic lipids among the four lipid components of lipid-based nanoparticles facilitate endocytosis. The cationic-lipid-induced positive charge offers a hydrated lipid headgroup to maintain the bilayer structure stabilization and benefits endocytosis due to the robust interaction with the negatively charged cell surfaces.65,66 The first cationic lipid, N-[1-(2,3-dioleyloxy)propyl]-N,N,N-trimethylammonium chloride (DOTMA), was reported for delivering mRNA in 1989,67 and afterward, more cationic lipids have been synthesized with all kinds of headgroups including quaternary amine-based, tertiary amine-based, univalent and multivalent cationic headgroups.68 However, PEG-lipids incorporated into lipid-based nanoparticles will reduce the tendency of positively-charged lipid-based nanoparticles to associate with negatively-charged cell surfaces by the stealth function, hindering their endocytosis. To overcome this phenomenon, PEG-lipids’ hydrophobic anchor is shortened to enable the rapid dissociation of the PEG shield from lipid-based nanoparticles in circulation, decreasing the interference in subsequent intracellular delivery.69,70 Moreover, specific cell-targeting ligands anchoring on lipid-based nanoparticles, summarized previously, not only ensure loaded cargo to deliver into desired tissues but can also enhance the endocytosis efficacy.36,71 Although endocytosis is the most classical intracellular delivery, the overall therapeutic efficacy mediated by this route is limited since the loaded drugs were often trapped in endosomes or lysosomes, leading to partial degradation. Therefore, endocytosis-independent delivery strategies can serve as available alternatives and have unlimited potential in future biomedical applications, such as penetration and membrane fusion.
Penetration
Intracellular delivery can also be achieved by cell penetration, a membrane-disruption-based approach usually mediated by cell-penetrating peptides (CPPs). Conjugation of CPPs on lipid-based nanoparticles results in the penetration of nanoparticles across the membranes of live cells and delivery of different types of cargoes to cells or even directly to subcellular organelles.72 Such CPP-based penetration mainly involves two routes. One is dependent on the binding between the positively-charged CPPs with the negatively-charged membrane constituents or phospholipids, inducing pore formation or membrane destabilization for nanoparticle entrance.73 Another depends on binding guanidinium groups of extracellular CPPs with fatty acids on cells, nucleating a transient toroidal pore at high membrane pH to mediate their transport across the plasma membrane.73 Despite the effective delivery of small and midsize molecules, CPP-mediated penetration still has some shortcomings impeding the development, including disruption of the membrane, concurrent penetration, endocytosis, and specific trigger conditions.73–75
Membrane-fusion
Fusion with the cell membrane is an alternative recently-developed strategy to achieve intracellular delivery of lipid-based nanoparticles. This membrane-fusion-based transportation strategy is inspired by the natural process of viruses sending their genome into the cytosol to transfect the hosts.35,76,77 Through membrane fusion, the lipid composites of lipid-based nanoparticles merge with the lipid layer of cell membranes, and the loaded agents are directly transported into the cytoplasm, bypassing the endocytic pathway with lysosomal entrapment. The surface modification of fusion-related motifs and fusogenic lipid composites are the two strategies that regulate the membrane-fusion effect of lipid-based nanoparticles.
The first membrane-fusion strategy includes using a pair of fusion-recognitive molecules to modify the liposomes and cell membranes simultaneously. The recognition and combination of these molecules will facilitate the fusion of liposomes and cells. Kros's group has designed a pair of complementary coiled-coil lipopeptides, (KIAALKE)4 (K4) and (EIAALEK)4 (E4), which could bind together to generate a coiled-coil construction to initiate membrane fusion.79 By embedding them on the liposomal layers and cell membranes, the cargos encapsulated in the liposomes were delivered into the cytoplasm via membrane fusion.79,80 Based on these complementary lipopeptides, Huang et al. designed a controlled membrane-fusogenic liposome using a light-responsive coiled-coil structure.81 They modified K4 with a light-sensitive cholesterol-ortho-nitrobenzyl-PEG and inserted it in the lipid layer. Under the ultraviolet (UV) radiation, the PEG shielding blocks were removed due to the cleavage of the nitrobenzyl groups, exposing K4 that recognizes E4 peptides on liposomes to recover membrane fusion. Apart from these coiled-coil lipopeptides, the DNA sequences were also applied as fusion-regulatory molecules because of complementary base pairing. Sun et al. reported a membrane-fusogenic approach by using DNA hybridization to guide the effective and direct delivery of proteins into the cellular cytoplasm.82 This membrane-fusion delivery was achieved using a zipper-like construction formed by the hybridization of 3′-cholesterol-functionalized and the complementary 5′-cholesterol-functionalized single-stranded DNA. Peruzzi et al. also utilized the specificity and orthogonality of DNA oligonucleotide hybridization to deliver cargos into various vesicles via a membrane fusion.83
The membrane fusion through artificial dual modifications of liposome/cell surfaces is challenged by in vivo applications because of the complexity and insufficient efficiency for in vivo operation. To develop a membrane-fusion-based process relying on biomimetic materials, lipid-based nanoparticles are investigated to simulate the natural membrane-fusion phenomenon containing lipid blending to directly deliver therapeutic components into the targeting cell cytoplasm. Considering the similarity of chemical structures, fluidity, and phase-transition properties of some lipid molecules with the membranous lipid bilayer, altering suitable lipids could result in non-physiological fusion. Shen's team designed a membrane-fusion lipid coating technique to induce a membrane-fusion-dependent intracellular delivery (Fig. 6).64,84 These coating lipids contained 1,2-dioleoyl-sn-glycero-3-phosphoethanolamine (DOPE), negatively-charged cholesteryl hemisuccinate (CHEMS), and 1,2-distearoyl-sn-glycero-3-phosphoethanolamine-N-polyethylene glycol (DSPE-PEG) in a fixed ratio of 7.65/2/1.35. Through membrane fusion, this liposome achieved a lysosome-independent intracellular delivery of the encapsulated polyplex encapsulating tumor necrosis factor-related apoptosis-inducing ligand (TRAIL)-encoding DNA and USP22 shRNA for A549 tumor and hepatocellular carcinoma treatment, respectively.64,84
 |
| Fig. 6 Fusogenic lipid coating to induce membrane-fusion-dependent delivery. (A) Schematic illustration of fusogenic lipopolyplex for systemic gene delivery. (B) Confocal laser scanning microscopy images indicate efficient escape of the fusogenic polyplexes from the lysosomes. Scale bar, 10 μm. Reproduced with permission.64 Copyright 2016, Wiley-VCH. | |
In addition, Kim's group has also designed a membrane-fusogenic lipid layer of core–shell liposomes. The lipid layer was made up of 1,2-dimyristoyl-sn-glycero-3-phosphocholine (DMPC), DOTAP, and DSPE-PEG with a specific ratio of 76.2/20/3.8 (Fig. 7).78,85 Such membrane fusion lipid-based nanoparticles successfully deliver the oligonucleotide and siRNA loaded in the silica core into the cytoplasm of macrophages to improve bacterial clearance. Due to high efficiency and repeatability, this membrane-fusogenic liposome (DMPC/DSPE-PEG/DOTAP) has been extended to other ingredients with similar structures of phospholipids, PEGylated lipids, and cationic lipids for membrane-fusion delivery. Zheng et al. designed a core–shell fusogenic liposome to engineer the tumor surface glycans for improved natural killer (NK) cell-based tumor elimination (Fig. 8). This liposome had the lipid components of DMPC, LeX trisaccharide-modified DSPE-PEG and DOTAP with a similar ratio of 75/5/19. Through membrane fusion, they could deliver sialyltransferase inhibitor, encapsulated in the core, into the cytoplasm of tumor cells, and simultaneously present LeX trisaccharide onto the cell surface, reversing the NK-suppressing tumor surface to be NK-activating, arousing sensitive NK-cell-based recognition and destruction.86 Shi et al. designed a fusogenic liposome to fuse with T cells to modulate and measure their activity by utilizing their surface redox degree as a chemical target.87 These liposomes consisted of 1,2-dioleoyl-sn-glycero-3-phosphoethanolamine (DOPE), DSPE-PEG, and DOTAP. The maximized fusion efficiency was obtained when the DOTAP content reached 50%. The roles of these lipids in membrane fusion were also investigated. The neutrally charged lipids with a slightly low phase transition point near room temperature should occupy the major portion to obtain appropriate fluidity. The cationic lipids provide a strong interaction with the cell membranes, while PEGylated modification is responsible for dehydrating the contact surface.88 Distinguishing from this design principle, Lin et al. also reported another spherical nucleic acid-based liposome with membrane-fusion-based transport.89 The liposome was composed of dipalmitoyl-phosphocholine (DPPC), dioleoyl-phosphatidylcholine (DOPC), cholesterol, and DOTAP with a ratio of 1/1/2/0.2 in the absence of necessary PEG (Fig. 9). The membrane-fusion mechanism needs further research and identification. However, there is no doubt that fusion-based intracellular delivery is promising to improve intracellular drug delivery efficiency.
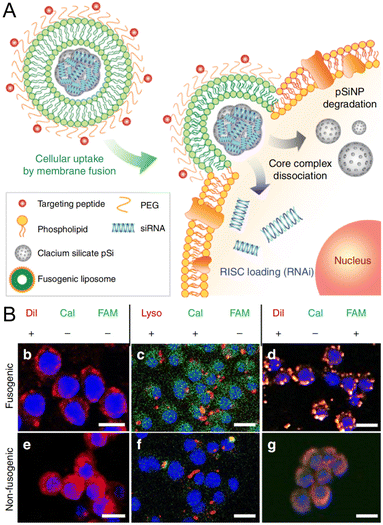 |
| Fig. 7 Membrane-fusion system for siRNA delivery to improve immunogene therapy. (A) Schematic illustration of fusogenic systems delivering siRNA loaded in the silica core into the cytoplasm of macrophages. (B) Confocal microscopy images show the fusogenic nanoparticles fuse with the cell membrane of macrophages and deliver the payload into the cytoplasm. Scale bar, 20 μm. Reproduced with permission.78 Copyright 2018, Springer Nature. | |
 |
| Fig. 8 Membrane-fusogenic liposomes to engineer tumor cell surface glycans for natural killer cell therapy. (A) Schematic illustration of membrane-fusogenic liposome engineering targets in/on the cytoplasm and surface simultaneously through membrane-fusion. (B) Confocal laser scanning microscopy images show the successful engineering of tumor cells through membrane fusion after intratumoral injection. (C) Antitumor efficacy mediated by in situ membrane-fusogenic liposome therapy. Reproduced with permission.86 Copyright 2022, Wiley-VCH. | |
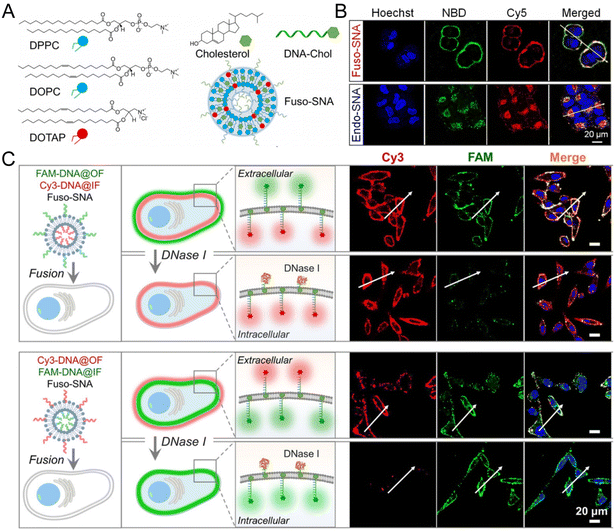 |
| Fig. 9 Fusogenic spherical nucleic acid for spatially-controllable cell membrane modification. (A) Formulation of the fusogenic spherical nucleic acid. (B) Confocal laser scanning microscopy images verify membrane-delivery by fusogenic spherical nucleic acid. (C) Fusogenic spherical nucleic acids engineer the external and internal cell membranes. Reproduced with permission.89 Copyright 2022, Wiley-VCH. | |
Drug release
Endosomal escape
The eventual therapeutic effect of lipid-based nanoparticles is still determined by effective cargo release from the lysosomal trap and the nanoparticle interior. The cell penetration and membrane fusion mechanism could easily deliver cargoes into the cytoplasm, while the endocytosis pathway still encounters endosomal entrapment. Facilitating endosomal escape of lipid-based nanoparticles to achieve cytosolic delivery of cargoes is vital to avoid degradation and unleash therapeutic activity.90 The strategy of promoting the endosomal escape of lipid-based nanoparticles is destabilizing the endosomal membrane using cationic lipids through strong electrostatic interactions. Primarily, the cationic lipids were applied to facilitate cellular uptake and endosomal release of cargo through electrostatic interactions with the anion. Nevertheless, the high density of positive charge is also cytotoxic to the body in vivo.66 Therefore, ionizable cationic lipids were exploited to solve these safety issues. Such ionizable cationic lipids are almost neutrally charged in the physiological environment (pH 7.4), ensuring biosafety and extending body circulation superior to cationic lipids. After exposure to acidic endosomal environments, these ionizable cationic lipids become positively charged and bind to the negative lipid layers of endosomal membranes. This binding destabilizes the initial endosomal membrane balance, forming a non-bilayer hexagonal (HII) structure to induce endosomal destruction followed by the cargo escape.91,92 The first ionizable lipids generated are DODAP, 1,2-dioleyloxy-N,N-dimethyl-3-aminopropane (DODMA), 1,2-dilinoleyloxy-N,N-dimethyl-3-aminopropane (DLinDMA) and dilinoleylmethyl-4-dimethylaminobutyrate (DLin-MC3-DMA).93–96 The research related to novel ionizable lipids is growing, motivating a lot of academies and industries to design new combinatorial reaction conceptions to synthesize various promising lipid materials for trials to promote material discovery.97 Several helper lipids are also found in inducing endosome destabilization through modulating lipid fluidity and enhancing efficacy by improving lipid phase transitions.19 DOPE presents a cone-like shape since its structure includes a relatively small headgroup, phosphoethanolamine, and two bulky and unsaturated oleoyl chains. This lipid conformation tends to form the non-bilayer HII phase to facilitate endosomal membrane fusion and/or bilayer disruption to promote endosomal escape.98 Cholesterol is commonly used in lipid-based nanoparticles because of the stabilization of lipid bilayers via padding in cranny between phospholipids. It was reported that the high percentages of cholesterol could enhance the activity of cationic lipids to facilitate endosomal escape by inducing bilayer destabilization.66 Anionic lipids, such as linoleic acid, CHEMS, and oleic acid (OA), can form ion pairs with cationic lipids when incorporated into lipid-based nanoparticles, which facilitate endosomal release by bilayer disruption.99
Stimuli-responsive release
Endosomal escape can avoid drug efficacy loss. By using stimuli-sensitive components or units and incorporating responsive ligands, lipid-based nanoparticles are formed to release cargo in various microenvironments.100 We summarize the strategy to regulate cargo release to the cytoplasmic microenvironment under stimulation by pH, enzyme, and oxidation and reduction effects (redox).
The pH-responsive release depends on lipid membrane destabilization induced by the characteristic change of lipid-based nanoparticles at low pH values. This mechanism is similar to endosomal escape due to endosomal membrane destabilization induced by charge changes.101,102 Conformational flip in acidic media is also used for the formulation of pH-sensitive lipid-based nanoparticles by incorporating trans-2-morpholinocyclohexanol-based lipids in lipid components.103 The headgroup conformational transition triggered by protonation can produce a transient filling crack in the lipid tail to enhance cargo leakage. In addition, pH-sensitive lipid-based nanoparticles could be also prepared via encapsulating bicarbonate ions, which could generate CO2 bubbles when acidified in the inner compartment, thereby forming pores to collapse the liposomes.104
Enzyme-responsive release often relies on the cleavage of linkers in lipid-based nanoparticles by various enzymes in lysosomes or the cytoplasm. Commonly, the controllable release of the therapeutic agents from enzyme-responsive delivery systems is achieved by cleaving amides or esters in a short peptide via proteases or esterases, respectively.105 For example, Moon et al. formulated multilamellar vesicles via covalent cross-linking, which released cargo slowly under serum-containing conditions but were rapidly degraded in the presence of lipases.26 Yingyuad and co-workers synthesized PEG-peptidyl lipids which responded to elastase to facilitate the release of oligonucleotides from the endosome.107,108 Song et al. introduced enzymatically cleavable peptide linkers (GFLG), which could be degraded by cathepsin B to boost the transfection efficiency.109
Redox has also been reported to trigger responsive drug release by destabilizing lipid-based nanoparticles via charge changes using chemical reducing agents or lipid phase transitions due to cleaving reducible linkers in highly reducible intracellular space environments.110 The typical redox-responsive strategy is introducing disulfide linkages which could be cleaved by strong thiolytic reducing reagents. The reduced amphiphilic coproducts will be commonly increased, leading to fragmentation, aggregation, and subsequent cargo release.110 Ling et al. developed a disulfide cross-linked liposomes that were assembled from a dimeric lipoic acid-glycerophosphorylcholine (di-LA-PC) conjugation and further cross-linked by the addition of dithiothreitol (DTT) with the lipoyl units in lipoic acid. The nanoparticles exhibited high serum stability and triggered intracellular reduction-responsive release in tumor cells.24 Candiani et al. designed a liposome comprising redox-responsive triazine-based surfactant, which promoted lipid disruption by cleaving the surfactant to single-chain amphiphiles responding to a reducing environment.111 Systematically, Xu's group synthesized a group of disulfide-bond containing lipids, which were degradable in the presence of thiol-containing biomolecules (Fig. 10).106,112–114 In addition, a quinone propionic acid moiety was also used for the preparation of lipid-based nanoparticles to induce the ruinous aggregation via quinone reduction.115 Except for these single-stimuli-responsive strategies, multiple-stimuli-responsive delivery systems have been designed for combinational activation in the cellular environment.100,114 The cargos could be released more precisely and effectively under synergetic effects of certain intracellular factors, such as multiple enzymes and a combination of pH, enzymes or redox.
 |
| Fig. 10 Bioreducible LNPs enable gene editing in vivo. (A) Schematic illustration of bioreducible LNPs consisting of disulfide bond-containing hydrophobic tails for gene editing. (B) Bioreducible LNPs show an effective knockout effect in vitro. (C) The biodistribution and gene editing efficacy of bioreducible LNPs in vivo. Reproduced with permission.106 Copyright 2019, Wiley-VCH. | |
Perspectives and future directions
The lipid-based nanoparticles that carry chemotherapeutics and nucleic acids have made breakthroughs in clinical trials and demonstrate promise in application in a range of diseases. Recently, LNP-based vaccines against COVID-19 have gained emergency FDA approval and were administered to millions of people worldwide, attracting attention in scientific research and biomedical applications. However, there are only a limited amount of lipid-based nanoparticle formulations successfully coming out to the market, indicating challenges remaining in clinical translation. To promote industrialization, many effective strategies have been used to optimize the properties of lipid-based nanoparticles, including safety, encapsulating capacity, stability, pharmacokinetics, biodistribution, and therapeutic efficacy. Through surface modification, structural design, and composition modulation, lipid-based nanoparticles have become more effective in meeting various requirements. Moreover, there are still several aspects that can be further investigated. First, based on the chemical structures including head groups, linker groups, and hydrophobic tails, more innovative lipid materials and derivatives should be conceived and synthesized. The corresponding high-throughput screening technology can be used to search for available lipid components to setup libraries. In addition, the preparation of lipid-based nanoparticles could be tuned and optimized for different requirements. The roles of each component or impact of diverse ratios require further studies. Thirdly, the mechanisms regulating the behavior of lipid-based nanoparticles in vivo should be further investigated. The specific mechanisms governing membrane-fusion or endosomal escape are lacking. High-precision instruments or molecular dynamics simulations may reveal the molecular details of many basic biological processes. Furthermore, the models can be honed to better recapitulate human physiology to verify the functionality and clinical translation potential of the lipid-based nanoparticles. This will accelerate the translation of lipid-based nanoparticles from preclinical animal models to humans. Finally, the cost of manufacture, transportation, and storage of lipid-based nanoparticles is also considerable. It is an urgent requirement to find ways to develop nanoparticles with controllable size and homogeneity at large-scale production while maintaining their physical properties intact during long-term storage. With cooperative efforts between academia and industry, these challenges can be overcome to develop a series of advanced lipid-based nanoparticles that can translate to improve human health.
Conclusions
In conclusion, we reviewed the strategies to design the structures and lipid compositions of lipid-based nanoparticles in vivo for specific biological effects. For in vivo distribution control, the traditional approach is based on surface grafting the particles with targeting ligands. The advanced method is aimed at optimizing the lipid compositions and ratios to target selective tissue. Besides endocytosis and cell penetration pathways, the innovative membrane-fusion method was also emphasized in direct cytosolic delivery. The cargo loaded can be released controllably through endosomal escape and stimuli-sensitive activation. Future studies are needed to understand how lipid-based nanoparticles interact with biological systems both in vitro and in vivo. We hope this review offers new insights into the engineering of lipid-based nanoparticles to advance nanotechnology for biomedical applications.
Conflicts of interest
There are no conflicts to declare.
Acknowledgements
This work is supported by the National Key Research and Development Program of China (2019YFA0111300), the National Natural Science Foundation of China (22277155, 52103197, 51903256, 21907113), the Science and Technology Program of Guangzhou (202102010225), the Guangdong Provincial Science and Technology Program (International Scientific Cooperation, 2018A050506035), the China Postdoctoral Science Foundation (2020M680133, 2022T150747), the China Primary Health Care Foundation (2022-003), the Thousand Talents Plan, and the Guangdong Provincial Pearl River Talents Program (2019QN01Y131).
References
- J. Wang, M. Zhu and G. Nie, Adv. Drug Delivery Rev., 2021, 178, 113974 CrossRef CAS PubMed
.
- L. Xu, X. Wang, Y. Liu, G. Yang, R. J. Falconer and C.-X. Zhao, Adv. NanoBiomed Res., 2022, 2, 2100109 CrossRef CAS
.
- M. J. Mitchell, M. M. Billingsley, R. M. Haley, M. E. Wechsler, N. A. Peppas and R. Langer, Nat. Rev. Drug Discovery, 2021, 20, 101–124 CrossRef CAS PubMed
.
- X. Hou, T. Zaks, R. Langer and Y. Dong, Nat. Rev. Mater., 2021, 6, 1078–1094 CrossRef CAS PubMed
.
- L. Miao, Y. Zhang and L. Huang, Mol. Cancer, 2021, 20, 41 CrossRef CAS PubMed
.
- Y. Zhang, C. Sun, C. Wang, K. E. Jankovic and Y. Dong, Chem. Rev., 2021, 121, 12181–12277 CrossRef CAS PubMed
.
- K. Kaygisiz and C. V. Synatschke, Biomater. Sci., 2020, 8, 6113–6156 RSC
.
- Y. Zeng, O. Escalona-Rayo, R. Knol, A. Kros and B. Slütter, Biomater. Sci., 2023, 11, 964–974 RSC
.
- A. D. Bangham and R. W. Horne, J. Mol. Biol., 1964, 8, 660–668 CrossRef CAS PubMed
.
- D. D. Lasic, Trends Biotechnol., 1998, 16, 307–321 CrossRef CAS PubMed
.
- P. Yingchoncharoen, D. S. Kalinowski and D. R. Richardson, Pharmacol. Rev., 2016, 68, 701–787 CrossRef CAS PubMed
.
- T. T. H. Thi, E. J. A. Suys, J. S. Lee, D. H. Nguyen, K. D. Park and N. P. Truong, Vaccines, 2021, 9, 359 CrossRef CAS PubMed
.
- E. Keles, Y. Song, D. Du, W. J. Dong and Y. Lin, Biomater. Sci., 2016, 4, 1291–1309 RSC
.
- C. Wang, Y. Zhang and Y. Dong, Acc. Chem. Res., 2021, 54, 4283–4293 CrossRef CAS PubMed
.
- Y. Li, Z. Ye, H. Yang and Q. Xu, Acta Pharm. Sin. B, 2022, 12, 2624–2639 CrossRef CAS PubMed
.
- S. Shah, V. Dhawan, R. Holm, M. S. Nagarsenker and Y. Perrie, Adv. Drug Delivery Rev., 2020, 154–155, 102–122 CrossRef CAS PubMed
.
- T. Peng, W. Xu, Q. Li, Y. Ding and Y. Huang, Biomater. Sci., 2022, 11, 62–75 RSC
.
- D. E. Large, R. G. Abdelmessih, E. A. Fink and D. T. Auguste, Adv. Drug Delivery Rev., 2021, 176, 113851 CrossRef CAS PubMed
.
- S. T. Yang, A. J. B. Kreutzberger, J. Lee, V. Kiessling and L. K. Tamm, Chem. Phys. Lipids, 2016, 199, 136–143 CrossRef CAS PubMed
.
- Y. Liu, K. M. C. Bravo and J. Liu, Nanoscale Horiz., 2021, 6, 78–94 RSC
.
- X. He, L. Li, H. Su, D. Zhou, H. Song, L. Wang and X. Jiang, Int. J. Nanomed., 2015, 10, 1791–1804 CAS
.
- S. Kumar, J. Dutta, P. K. Dutta and J. Koh, Int. J. Biol. Macromol., 2020, 160, 470–481 CrossRef CAS PubMed
.
- S. Liu and D. F. O'Brien, J. Am. Chem. Soc., 2002, 124, 6037–6042 CrossRef CAS PubMed
.
- L. Ling, M. Ismail, Y. Du, C. Yao and X. Li, Int. J. Pharm., 2019, 560, 246–260 CrossRef CAS PubMed
.
- K. I. Joo, L. Xiao, S. Liu, Y. Liu, C. L. Lee, P. S. Conti, M. K. Wong, Z. Li and P. Wang, Biomaterials, 2013, 34, 3098–3109 CrossRef CAS PubMed
.
- J. J. Moon, H. Suh, A. Bershteyn, M. T. Stephan, H. Liu, B. Huang, M. Sohail, S. Luo, S. H. Um, H. Khant, J. T. Goodwin, J. Ramos, W. Chiu and D. J. Irvine, Nat. Mater., 2011, 10, 243–251 CrossRef CAS PubMed
.
- C. P. Costa, J. N. Moreira, J. M. S. Lobo and A. C. Silva, Acta Pharm. Sin. B, 2021, 11, 925–940 CrossRef CAS PubMed
.
- H. Mu and R. Holm, Expert Opin. Drug Delivery, 2018, 15, 771–785 CrossRef CAS PubMed
.
- Y. Mirchandani, V. B. Patravale and B. S, J. Controlled Release, 2021, 335, 457–464 CrossRef CAS PubMed
.
- R. Tenchov, R. Bird, A. E. Curtze and Q. Zhou, ACS Nano, 2021, 15, 16982–17015 CrossRef CAS PubMed
.
- E. Elhassan, N. Devnarain, M. Mohammed, T. Govender and C. A. Omolo, J. Controlled Release, 2022, 351, 598–622 CrossRef CAS PubMed
.
- N. Rajana, A. Mounika, P. S. Chary, V. Bhavana, A. Urati, D. Khatri, S. B. Singh and N. K. Mehra, J. Controlled Release, 2022, 352, 1024–1047 CrossRef CAS PubMed
.
- J. Yuan, M. Guo, S. Zhao, J. Li, X. Wang, J. Yang, Z. Jin and X. Song, Chin. Chem. Lett., 2023, 34, 107943 CrossRef CAS
.
- A. Mukherjee, A. K. Waters, P. Kalyan, A. S. Achrol, S. Kesari and V. M. Yenugonda, Int. J. Nanomed., 2019, 14, 1937–1952 CrossRef CAS PubMed
.
- H. Kong, K. Yi, C. Zheng, Y. H. Lao, H. Zhou, H. F. Chan, H. Wang, Y. Tao and M. Li, J. Mater. Chem. B, 2022, 10, 6841–6858 RSC
.
- Y. Xu, T. Fourniols, Y. Labrak, V. Préat, A. Beloqui and A. des Rieux, ACS Nano, 2022, 16, 7168–7196 CrossRef CAS PubMed
.
- L. Khalili, G. Dehghan, N. Sheibani and A. Khataee, Int. J. Biol. Macromol., 2022, 213, 166–194 CrossRef CAS PubMed
.
- H. S. Choi, W. Liu, P. Misra, E. Tanaka, J. P. Zimmer, B. Itty Ipe, M. G. Bawendi and J. V. Frangioni, Nat. Biotechnol., 2007, 25, 1165–1170 CrossRef CAS PubMed
.
- X. Zhao, J. Chen, M. Qiu, Y. Li, Z. Glass and Q. Xu, Angew. Chem., Int. Ed., 2020, 59, 20083–20089 CrossRef CAS PubMed
.
- Q. Cheng, T. Wei, L. Farbiak, L. T. Johnson, S. A. Dilliard and D. J. Siegwart, Nat. Nanotechnol., 2020, 15, 313–320 CrossRef CAS PubMed
.
- M. Wacker, Int. J. Pharm., 2013, 457, 50–62 CrossRef CAS PubMed
.
- J. Wang, J. D. Byrne, M. E. Napier and J. M. DeSimone, Small, 2011, 7, 1919–1931 CrossRef CAS PubMed
.
- M. Qiu, H. Sun, F. Meng, R. Cheng, J. Zhang, C. Deng and Z. Zhong, J. Controlled Release, 2018, 272, 107–113 CrossRef CAS PubMed
.
- K. Piorecka, J. Kurjata, M. Stanczyk and W. A. Stanczyk, Biomater. Sci., 2018, 6, 2552–2565 RSC
.
- C. C. Hanna, J. Kriegesmann, L. J. Dowman, C. F. W. Becker and R. J. Payne, Angew. Chem., Int. Ed., 2022, 61, e202111266 CrossRef CAS PubMed
.
- E. Ho, Y. Deng, D. Akbar, K. Da, M. Létourneau, C. M. Morshead, D. Chatenet and M. S. Shoichet, ACS Appl. Mater. Interfaces, 2023, 15, 91–105 CrossRef CAS PubMed
.
- Y. L. Lin, N. M. Tsai, C. H. Chen, Y. K. Liu, C. J. Lee, Y. L. Chan, Y. S. Wang, Y. C. Chang, C. H. Lin, T. H. Huang, C. C. Wang, K. H. Chi and K. W. Liao, J. Nanobiotechnol., 2019, 17, 25 CrossRef PubMed
.
- V. Platt, Z. Huang, L. Cao, M. Tiffany, K. Riviere and F. C. Szoka Jr., Bioconjugate Chem., 2010, 21, 892–902 CrossRef CAS PubMed
.
- M. M. Kuijten, M. Hannah Degeling, J. W. Chen, G. Wojtkiewicz, P. Waterman, R. Weissleder, J. Azzi, K. Nicolay and B. A. Tannous, Sci. Rep., 2015, 5, 17220 CrossRef CAS PubMed
.
- X. Pan and R. J. Lee, Int. J. Pharm., 2007, 336, 276–283 CrossRef CAS PubMed
.
- E. Nogueira, A. C. Gomes, A. Preto and A. Cavaco-Paulo, Colloids Surf., B, 2015, 136, 514–526 CrossRef CAS PubMed
.
- V. P. Torchilin, T. S. Levchenko, A. N. Lukyanov, B. A. Khaw, A. L. Klibanov, R. Rammohan, G. P. Samokhin and K. R. Whiteman, Biochim. Biophys. Acta, 2001, 1511, 397–411 CrossRef CAS PubMed
.
- A. C. Khayrani, H. Mahmud, A. K. K. Oo, M. H. Zahra, M. Oze, J. Du, M. J. Alam, S. M. Afify, H. A. A. Quora, T. Shigehiro, A. S. Calle, N. Okada, A. Seno, K. Fujita, H. Hamada, Y. Seno, T. Mandai and M. Seno, Int. J. Mol. Sci., 2019, 20, 1042 CrossRef CAS PubMed
.
- G. K. Sinhmar, N. N. Shah, S. U. Rawal, N. V. Chokshi, H. N. Khatri, B. M. Patel and M. M. Patel, Artif. Cells, Nanomed., Biotechnol., 2018, 46, 565–578 CrossRef CAS PubMed
.
- L. Zhang, S. Wu, Y. Qin, F. Fan, Z. Zhang, C. Huang, W. Ji, L. Lu, C. Wang, H. Sun, X. Leng, D. Kong and D. Zhu, Nano Lett., 2019, 19, 4237–4249 CrossRef CAS PubMed
.
- E. O. Blenke, G. Klaasse, H. Merten, A. Pluckthun, E. Mastrobattista and N. I. Martin, J. Controlled Release, 2015, 202, 14–20 CrossRef PubMed
.
- S. Luozhong, Z. Yuan, T. Sarmiento, Y. Chen, W. Gu, C. McCurdy, W. Gao, R. Li, S. Wilkens and S. Jiang, Nano Lett., 2022, 22, 8304–8311 CrossRef CAS PubMed
.
- A. S. Manjappa, K. R. Chaudhari, M. P. Venkataraju, P. Dantuluri, B. Nanda, C. Sidda, K. K. Sawant and R. S. Murthy, J. Controlled Release, 2011, 150, 2–22 CrossRef CAS PubMed
.
- K. T. Magar, G. F. Boafo, X. Li, Z. Chen and W. He, Chin. Chem. Lett., 2022, 33, 587–596 CrossRef
.
- M. Qiu, Z. Glass, J. Chen, M. Haas, X. Jin, X. Zhao, X. Rui, Z. Ye, Y. Li, F. Zhang and Q. Xu, Proc. Natl. Acad. Sci. U. S. A., 2021, 118, e2020401118 CrossRef CAS PubMed
.
- J. Chen, Z. Ye, C. Huang, M. Qiu, D. Song, Y. Li and Q. Xu, Proc. Natl. Acad. Sci. U. S. A., 2022, 119, e2207841119 CrossRef CAS PubMed
.
- M. Qiu, Y. Tang, J. Chen, R. Muriph, Z. Ye, C. Huang, J. Evans, E. P. Henske and Q. Xu, Proc. Natl. Acad. Sci. U. S. A., 2022, 119, e2116271119 CrossRef CAS PubMed
.
- S. A. Dilliard, Q. Cheng and D. J. Siegwart, Proc. Natl. Acad. Sci. U. S. A., 2021, 118, e2109256118 CrossRef CAS PubMed
.
- X. Liu, J. Xiang, D. Zhu, L. Jiang, Z. Zhou, J. Tang, X. Liu, Y. Huang and Y. Shen, Adv. Mater., 2016, 28, 1743–1752 CrossRef CAS PubMed
.
- M. J. Hope, Ther. Delivery, 2014, 5, 663–673 CrossRef CAS PubMed
.
- X. Cheng and R. J. Lee, Adv. Drug Delivery Rev., 2016, 99, 129–137 CrossRef CAS PubMed
.
- R. W. Malone, P. L. Felgner and I. M. Verma, Proc. Natl. Acad. Sci. U. S. A., 1989, 86, 6077–6081 CrossRef CAS PubMed
.
- S. C. Semple, A. Akinc, J. Chen, A. P. Sandhu, B. L. Mui, C. K. Cho, D. W. Sah, D. Stebbing, E. J. Crosley, E. Yaworski, I. M. Hafez, J. R. Dorkin, J. Qin, K. Lam, K. G. Rajeev, K. F. Wong, L. B. Jeffs, L. Nechev, M. L. Eisenhardt, M. Jayaraman, M. Kazem, M. A. Maier, M. Srinivasulu, M. J. Weinstein, Q. Chen, R. Alvarez, S. A. Barros, S. De, S. K. Klimuk, T. Borland, V. Kosovrasti, W. L. Cantley, Y. K. Tam, M. Manoharan, M. A. Ciufolini, M. A. Tracy, A. de Fougerolles, I. MacLachlan, P. R. Cullis, T. D. Madden and M. J. Hope, Nat. Biotechnol., 2010, 28, 172–176 CrossRef CAS PubMed
.
- S. C. Semple, T. O. Harasym, K. A. Clow, S. M. Ansell, S. K. Klimuk and M. J. Hope, J. Pharmacol. Exp. Ther., 2005, 312, 1020–1026 CrossRef CAS PubMed
.
- L. Y. Song, Q. F. Ahkong, Q. Rong, Z. Wang, S. Ansell, M. J. Hope and B. Mui, Biochim. Biophys. Acta, 2002, 1558, 1–13 CrossRef CAS PubMed
.
- R. Lehner, X. Wang, S. Marsch and P. Hunziker, Nanomedicine, 2013, 9, 742–757 CrossRef CAS PubMed
.
- M. Qiu, J. Ouyang, Y. Wei, J. Zhang, Q. Lan, C. Deng and Z. Zhong, Adv. Healthcare Mater., 2019, 8, e1900500 CrossRef PubMed
.
- G. Guidotti, L. Brambilla and D. Rossi, Trends Pharmacol. Sci., 2017, 38, 406–424 CrossRef CAS PubMed
.
- S. Futaki and I. Nakase, Acc. Chem. Res., 2017, 50, 2449–2456 CrossRef CAS PubMed
.
- E. Soprano, E. Polo, B. Pelaz and P. Del Pino, J. Nanobiotechnol., 2022, 20, 538 CrossRef CAS PubMed
.
- R. Jahn, T. Lang and T. C. Südhof, Cell, 2003, 112, 519–533 CrossRef CAS PubMed
.
- W. Wickner and R. Schekman, Nat. Struct. Mol. Biol., 2008, 15, 658–664 CrossRef CAS PubMed
.
- B. Kim, H. B. Pang, J. Kang, J. H. Park, E. Ruoslahti and M. J. Sailor, Nat. Commun., 2018, 9, 1969 CrossRef PubMed
.
- J. Yang, A. Bahreman, G. Daudey, J. Bussmann, R. C. Olsthoorn and A. Kros, ACS Cent. Sci., 2016, 2, 621–630 CrossRef CAS PubMed
.
- J. Yang, J. Tu, G. E. M. Lamers, R. C. L. Olsthoorn and A. Kros, Adv. Healthcare Mater., 2017, 6, 1700759 CrossRef PubMed
.
- F. Huang, R. Duan, Z. Zhou, M. Vazquez-Gonzalez, F. Xia and I. Willner, Chem. Sci., 2020, 11, 5592–5600 RSC
.
- L. Sun, Y. Gao, Y. Wang, Q. Wei, J. Shi, N. Chen, D. Li and C. Fan, Chem. Sci., 2018, 9, 5967–5975 RSC
.
- J. A. Peruzzi, M. L. Jacobs, T. Q. Vu, K. S. Wang and N. P. Kamat, Angew. Chem., Int. Ed., 2019, 58, 18683–18690 CrossRef CAS PubMed
.
- S. Xu, S. Ling, Q. Shan, Q. Ye, Q. Zhan, G. Jiang, J. Zhuo, B. Pan, X. Wen, T. Feng, H. Lu, X. Wei, H. Xie, S. Zheng, J. Xiang, Y. Shen and X. Xu, Adv. Sci., 2021, 8, 2003042 CrossRef CAS PubMed
.
- B. Kim, Q. Yang, L. W. Chan, S. N. Bhatia, E. Ruoslahti and M. J. Sailor, Nanoscale Horiz., 2021, 6, 330–340 RSC
.
- C. Zheng, Q. Zhong, W. Song, K. Yi, H. Kong, H. Wang, Y. Tao, M. Li and X. Chen, Adv. Mater., 2022, 14, 2206989 Search PubMed
.
- C. Shi, Q. Zhang, Y. Yao, F. Zeng, C. Du, S. Nijiati, X. Wen, X. Zhang, H. Yang, H. Chen, Z. Guo, X. Zhang, J. Gao, W. Guo, X. Chen and Z. Zhou, Nat. Nanotechnol., 2023, 18, 86–97 CrossRef CAS PubMed
.
- B. Kim, S. Sun, J. A. Varner, S. B. Howell, E. Ruoslahti and M. J. Sailor, Adv. Mater., 2019, 31, e1902952 CrossRef PubMed
.
- M. Lin, Y. Chen, S. Zhao, R. Tang, Z. Nie and H. Xing, Angew. Chem., Int. Ed., 2022, 61, e202111647 CAS
.
- L. I. Selby, C. M. Cortez-Jugo, G. K. Such and A. P. R. Johnston, Wiley Interdiscip. Rev.: Nanomed. Nanobiotechnol., 2017, 9, e1452 Search PubMed
.
- M. Schlich, R. Palomba, G. Costabile, S. Mizrahy, M. Pannuzzo, D. Peer and P. Decuzzi, Bioeng. Transl. Med., 2021, 6, e10213 CAS
.
- S. Guo, K. Li, B. Hu, C. Li, M. Zhang, A. Hussain, X. Wang, Q. Cheng, F. Yang, K. Ge, J. Zhang, J. Chang, X.-J. Liang, Y. Weng and Y. Huang, Exploration, 2021, 1, 35–49 CrossRef
.
- S. C. Semple, S. K. Klimuk, T. O. Harasym, N. Dos Santos, S. M. Ansell, K. F. Wong, N. Maurer, H. Stark, P. R. Cullis, M. J. Hope and P. Scherrer, Biochim. Biophys. Acta, 2001, 1510, 152–166 CrossRef CAS PubMed
.
- J. Heyes, L. Palmer, K. Bremner and I. MacLachlan, J. Controlled Release, 2005, 107, 276–287 CrossRef CAS PubMed
.
- M. Jayaraman, S. M. Ansell, B. L. Mui, Y. K. Tam, J. Chen, X. Du, D. Butler, L. Eltepu, S. Matsuda, J. K. Narayanannair, K. G. Rajeev, I. M. Hafez, A. Akinc, M. A. Maier, M. A. Tracy, P. R. Cullis, T. D. Madden, M. Manoharan and M. J. Hope, Angew. Chem., Int. Ed., 2012, 51, 8529–8533 CrossRef CAS PubMed
.
- A. Algarni, E. H. Pilkington, E. J. A. Suys, H. Al-Wassiti, C. W. Pouton and N. P. Truong, Biomater. Sci., 2022, 10, 2940–2952 RSC
.
- N. Chaudhary, D. Weissman and K. A. Whitehead, Nat. Rev. Drug Discovery, 2021, 20, 817–838 CrossRef CAS PubMed
.
- Y. Hattori, S. Suzuki, S. Kawakami, F. Yamashita and M. Hashida, J. Controlled Release, 2005, 108, 484–495 CrossRef CAS PubMed
.
- G. Shi, W. Guo, S. M. Stephenson and R. J. Lee, J. Controlled Release, 2002, 80, 309–319 CrossRef CAS PubMed
.
- Y. Lee and D. H. Thompson, Wiley Interdiscip. Rev.: Nanomed. Nanobiotechnol., 2017, 9, e1450 Search PubMed
.
- M. V. Barbosa, L. O. Monteiro, G. Carneiro, A. R. Malagutti, J. M. Vilela, M. S. Andrade, M. C. Oliveira, A. D. Carvalho-Junior and E. A. Leite, Colloids Surf., B, 2015, 136, 553–561 CrossRef CAS PubMed
.
- H. Xu, M. Hu, X. Yu, Y. Li, Y. Fu, X. Zhou, D. Zhang and J. Li, Eur. J. Pharm. Biopharm., 2015, 91, 66–74 CrossRef CAS PubMed
.
- N. M. Samoshina, X. Liu, B. Brazdova, A. H. Franz, V. V. Samoshin and X. Guo, Pharmaceutics, 2011, 3, 379–405 CrossRef CAS PubMed
.
- J. Liu, H. Ma, T. Wei and X. J. Liang, Chem. Commun., 2012, 48, 4869–4871 RSC
.
- E. Fleige, M. A. Quadir and R. Haag, Adv. Drug Delivery Rev., 2012, 64, 866–884 CrossRef CAS PubMed
.
- J. Liu, J. Chang, Y. Jiang, X. Meng, T. Sun, L. Mao, Q. Xu and M. Wang, Adv. Mater., 2019, 31, e1902575 CrossRef PubMed
.
- P. Yingyuad, M. Mével, C. Prata, S. Furegati, C. Kontogiorgis, M. Thanou and A. D. Miller, Bioconjugate Chem., 2013, 24, 343–362 CrossRef CAS PubMed
.
- P. Yingyuad, M. Mével, C. Prata, C. Kontogiorgis, M. Thanou and A. D. Miller, J. RNAi Gene Silencing, 2014, 10, 490–499 Search PubMed
.
- S. J. Song, S. Lee, Y. Lee and J. S. Choi, Eur. J. Pharm. Biopharm., 2016, 91, 20–30 CAS
.
- R. L. McCarley, Annu. Rev. Anal. Chem., 2012, 5, 391–411 CrossRef CAS PubMed
.
- G. Candiani, D. Pezzoli, L. Ciani, R. Chiesa and S. Ristori, PLoS One, 2010, 5, e13430 CrossRef PubMed
.
- M. Wang, K. Alberti, A. Varone, D. Pouli, I. Georgakoudi and Q. Xu, Adv. Healthcare Mater., 2014, 3, 1398–1403 CrossRef CAS PubMed
.
- Y. Li, R. Jarvis, K. Zhu, Z. Glass, R. Ogurlu, P. Gao, P. Li, J. Chen, Y. Yu, Y. Yang and Q. Xu, Angew. Chem., Int. Ed., 2020, 59, 14957–14964 CrossRef CAS PubMed
.
- M. Qiu, Y. Li, H. Bloomer and Q. Xu, Acc. Chem. Res., 2021, 54, 4001–4011 CrossRef CAS PubMed
.
- M. F. Mendoza, N. M. Hollabaugh, S. U. Hettiarachchi and R. L. McCarley, Biochemistry, 2012, 51, 8014–8026 CrossRef CAS PubMed
.
- A. Gabizon, D. Goren, A. T. Horowitz, D. Tzemach, A. Lossos and T. Siegal, Adv. Drug Delivery Rev., 1997, 24, 337–344 CrossRef CAS
.
Footnote |
† These authors contributed equally. |
|
This journal is © The Royal Society of Chemistry 2023 |
Click here to see how this site uses Cookies. View our privacy policy here.