DOI:
10.1039/D1RA06030A
(Review Article)
RSC Adv., 2021,
11, 34688-34698
Anti-bacterial activity of gold nanocomposites as a new nanomaterial weapon to combat photogenic agents: recent advances and challenges
Received
9th August 2021
, Accepted 17th October 2021
First published on 26th October 2021
Abstract
Gold nanocomposites are being widely used in numerous biomedical applications owing to their excellent stability and miniaturization. Gold nanocomposites are notable because of their flexibility of functionalization and synthesis, ease of detection, and low toxicity. Cost-effectiveness, long-term stability, non-cytotoxicity, and biocompatibility are the main aspects of ideal nanocomposites. Antibacterial nanocomposites are being developed extensively in the food industry, environmental applications, and biological and medical devices. This review focuses on the applications of metal-based nanoparticles, mainly gold nanoparticles (AuNPs), as antibacterial agents in medical approaches. Additionally, the antibacterial mechanisms of AuNPs and their roles in fighting antibiotic-resistant microorganisms are highlighted in the present review.
1. Introduction
Antibacterial agents are crucial in several areas of science, such as medicine, industrial materials, food packaging, and water treatment.1 Antimicrobial activity can be defined as a collective term for all active principles (agents) that inhibit bacterial growth, prevent the formation of microbial colonies, and may destroy microorganisms.2,3 Organic compounds as conventional disinfectants are cytotoxic and also cause health problems. Therefore, a focus on developing inorganic disinfectants has gained extensive attention:1,4 for instance, nanoparticle (NP) enhanced antibacterial activity without creating toxicity to surrounding tissues. Over the past decade, different synthetic methods, such as the microemulsion technique, sol–gel method, and aerosol technique, have been applied to synthesizing nanoparticles.5,6 Their time-consuming nature, the high cost of production, and contamination with toxic chemicals have restricted the practical use of nanoparticles in biomedical applications. To overcome these limitations, low-cost green synthetic techniques were developed in which substances with low toxicity are utilized.7,8 Researchers have studied the antibacterial properties of nanoparticles for a long time. Among these, AuNPs are of high value because they have low toxicity. These unique properties have dramatically increased the use of AuNPs.9,10 Today, in addition to nanobiotechnology, AuNPs are used in medical, therapeutic, and drug delivery fields.10 One of the most attractive aspects of using AuNPs is producing medical implants, especially dental and orthopedic implants. It is revealed that AuNPs have excess potential for improving treatment results for maxillofacial and orthopedic and implant patients.11 In addition to antibacterial activities, AuNPs improve osteogenic differentiation of stem cells due to their photo-functionalizing properties.11 AuNPs were assembled on TiO2 nanotube (TN) arrays in an electrochemical deposition method to expand the surface features of TN arrays as an implant material.12 Also, it has been demonstrated that AuNPs have displayed huge potential in scaffolds, stem cells, medical imaging, delivery systems, and other parts.13 According to the obtained results, AuNPs, due to antibacterial activity and in various aspects of medicine, can be considered in the development of medical implants.14 The main elements of the present study are: (i) an overview of the antibacterial activity of metal-based nanocomposites; (ii) a particular review of the antibacterial activity of AuNPs along with the antibacterial mechanism; (iii) the introduction of advanced gold-based nanocomposites for antibacterial activity; and (iv) an overview of some bacteria that are most affected by AuNPs.
2. Antibacterial properties of metal-based nanocomposites
Metal-based nanoparticles act as non-specific antibacterial agents since they do not bind to a specific receptor in the bacterial cell.15,16 Moreover, metal-based nanoparticles prevent the development of resistance by bacteria and expand the range of antibacterial activity.15,16 The results show electrostatic interactions between the cell surface and nanoparticles as the primary step towards nanotoxicity, tracked by an increase in membrane permeability, morphological cell changes, and their accumulation in the cytoplasm.17 It is well understood that silver and copper nanoparticles have antibacterial activity against Bacillus subtilis (B. subtilis) and Staphylococcus aureus (S. aureus). Rupture of the plasma membrane, cell wall damage, and disturbing the biochemical process have been reported as the main mechanisms.18 Reduced enzymatic activity, the release of Cu2+, and changes in NADPH production were described as the antibacterial activity of Cu-doped TiO2 NPs toward Mycobacterium smegmatis (M. smegmatis).19 Adsorption and penetration of AgNPs and toxicity with electrostatic interaction were revealed as the underlying mechanisms against Klebsiella pneumoniae.20 For the eradication of Pseudomonas aeruginosa, ZnO NPs can be used appropriately. Applied nanoparticles could disrupt the membrane, generate reactive oxygen species (ROS), and disturb cell wall permeability.21 Electrostatic interaction altering bacterial attachment, damage to the bacterial cell wall, and improved permeability were demonstrated as the mode of action for Al2O3 nanocomposites as antibacterial agents.22 Growth inhibition, particularly in an aqueous medium, physical and mechanical stresses on cellular structural integrity, and significant damage to cellular functions are the main antibacterial activity points of NiO NPs against Escherichia coli (E. coli).23 Frameshift mutation and ROS generation are reported as the bacterial killing mechanism for TiO2 NPs against S. typhimurium.24 Disturbing the permeability and cell division, and interaction with sulfur- and phosphorus-containing compounds and the cell membrane are the mechanisms of Ag NPs against E. coli.25
Antimicrobial activity can be defined as a collective term for all active principles (agents) that inhibit the growth of bacteria, prevent the formation of microbial colonies, and may destroy microorganisms. As can be clarified from Table 1, the antimicrobial actions of metal-based nanocomposites can be categorized into three groups: (A) induction of toxicity and oxidative stress by the generation of ROS and free radicals to the bacterial surface, (B) disruption of the bacterial cell wall and plasma membrane with alteration in its permeability, and (C) modulation of signal transduction pathways.
Table 1 Antibacterial properties of some important metal-based nanocomposites
Nanocomposites |
Bacteria |
Mechanisms |
Ref. |
ZnO–Ag NPs |
B. subtilis |
Increases bacterial cell membrane permeability |
17 |
CuO–Ag NPs |
B. subtilis and S. aureus |
Rupture of the plasma membrane, cell wall damage, disturbs biochemical process |
18 |
Cu-doped TiO2 NPs |
M. smegmatis |
Reduced enzymatic activity, release of Cu2+, changes in NADPH production |
19 |
Ag NPs |
K. pneumoniae |
Adsorption, and penetration of NPs and toxicity with electrostatic interaction |
20 |
ZnO NPs |
P. aeruginosa |
Membrane disruption, ROS generation, and disturbance of permeability |
21 |
Al2O3 |
E. coli |
Electrostatic interaction and altered bacterial attachment, damage to the bacterial cell wall and increased permeability |
22 |
NiO NPs |
E. coli |
Growth inhibition, particularly in aqueous medium, physical and mechanical stresses on cellular structure integrity and major damage to cellular functions |
23 |
TiO2 |
S. typhimurium |
Frameshift mutation, ROS generation |
24 |
Ag |
E. coli |
Disturbed permeability and cell division, interacts with cell membrane and sulfur- and phosphorus-containing-compounds |
25 |
3. Gold nanoparticles
Gold nanoparticles (AuNPs) are considered the main candidate in numerous fields, such as nanobiotechnology, tissue engineering, and drug delivery. Chemical sensing and drug delivery technology are recognized as potential users of AuNPs due to the high affinity of AuNPs with organic species, and their high electrical conductivity (Fig. 1).
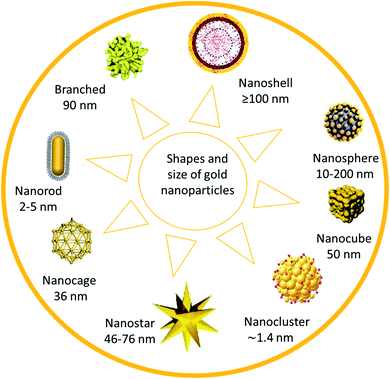 |
| Fig. 1 Shapes and size of gold nanoparticles.26 | |
AuNPs are used in many fields, including therapeutic, medical, and pharmaceutical applications. Gold nanorods were employed satisfactorily for photothermal tumor therapy.27 AuNPs were synthesized for the development of biosensors for the sensitive detection of Bacillus anthrax.28 Additionally, gold nanostars were applied for cancer therapy28,29 and biological labeling.30 Nanobelt AuNPs were used as transducers and resonators, and can be applied as a nanoscale sensor for medical approaches.28,31 Furthermore, nanoshells have potential for photonic crystals, fluorescent diagnostics, improving photoluminescent labels, catalysis, avoiding photodegradation, research into bioconjugates, and chemical and colloidal studies.32,33 Recently, AuNPs have been used extensively as antibacterial agents against a broad range of microorganisms.34 Their high impact, biocompatibility, low cost, and simple production are some advantages of AuNP-nanocomposites.35,36
3.1. Molecular mechanism of antibacterial activity of nanoparticles
The antibacterial activity of metal-based nanoparticles is promising in several fields, especially in medical areas. The size of the nanoparticles plays a fundamental role in their functional training, such as chemical and biological activity.37 Discovering the molecular mechanism of the antibacterial action of nanoparticles is an attractive aspect of nanobiotechnology. Inhibiting DNA replication, damaging the cell membrane, and inactivating proteins are the main antibacterial activity mechanisms of Ag NPs38 (Fig. 2). Affecting the purine metabolite pathway is another antibacterial mechanism of silver nanoparticles.39 Reactive oxygen species (ROS) aid as cell signaling molecules for regular biologic processes.40 However, the generation of ROS may damage many cellular and molecular processes.40 A high surface-area-to-volume ratio is one of the most essential properties of noble nanoparticles. So, nanoparticles enable a greater presence of atoms on the surface and suitable contact with the environment.41 Therefore, AgNPs make penetration through the cell membrane easier, cooperating with intracellular materials and allowing cell destruction.41 Accordingly, TiO2 and ZnO NPs could kill bacteria via ROS-production under UV irradiation.42 Carbon-based nanoparticles may cause mechanical damage or apply oxidants for antibacterial activity.43 Some crucial aspects that make the AuNPs remarkable in their antibacterial activities include facile synthesis methods,44 high functionalizability,45 strong interaction with the bacterial membrane,46 and their inherent biocidal activity.47
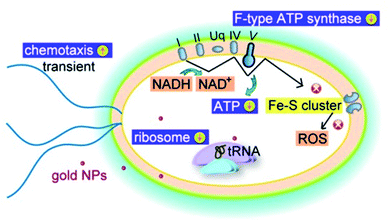 |
| Fig. 2 Schematic diagram of antibacterial activity of AuNPs against E. coli.26 | |
An interesting study has found that the antibacterial activity of AuNPs is not limited to ROS activity. It is revealed that AuNPs can also target the energy metabolism and transcription process of bacteria.26
Throughout these interesting phenomena, AuNPs are agglomerated on the bacterial surface and bound to the membrane protein due to the affinity of gold to the proteins. The formation of IB-AuNPs disrupts the bacterial membrane, and they are attached to cytoplasmic proteins with IB-AuNPs affinity, subsequently leading to the death of the bacterium. The mechanism discovered in this study is another example of the antibacterial activity of AuNPs, which is entirely independent of ROS creation (Fig. 3).
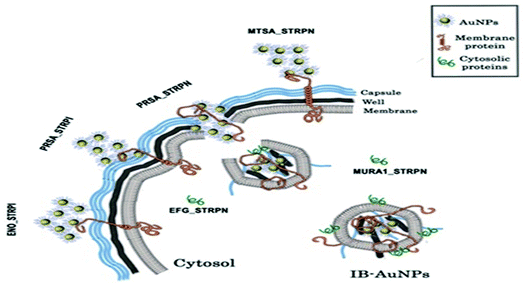 |
| Fig. 3 Antibacterial activity of AuNPs against S. pneumoniae.48 | |
In general, the main mechanism of antibacterial activity of AuNPs includes the effect on apoptosis, DNA damage, cell membrane damage, electron transfer chain damage, ROS production, and disruption of metabolic pathways, such as the ATP production pathway. Fig. 4 illustrates the main antibacterial activity of AuNPs against bacteria (Table 2).
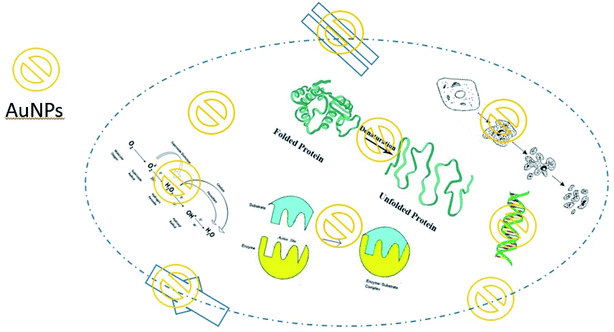 |
| Fig. 4 Main molecular mechanisms of AuNP antibacterial activities. | |
Table 2 Molecular mechanisms of metal-based nanoparticle antibacterial activity
NPs |
Molecular mechanisms |
Ref. |
Ag |
Inhibiting DNA replication, damaging the cell membrane, and inactivation of proteins |
38 |
Ag |
Affecting the purine metabolite pathway is another antibacterial mechanism |
39 |
Ag |
Makes penetration through cell membrane easier, cooperating with intracellular materials and allowing cell destruction |
41 |
TiO2/ZnO |
Reactive oxygen species (ROS) aid as cell signaling molecules for regular biological processes and damage many cellular and molecular processes |
42 |
C-based |
Mechanical damage or apply oxidant |
43 |
AuNPs |
AuNPs target the energy metabolism and transcription process of bacteria |
26 |
AuNPs |
Formation of IB-AuNPs, and disruption of the bacterial cell membrane |
48 |
3.2. The role of nanocomposites in enhancing the antibacterial properties of gold nanoparticles
Numerous materials are used in the composition of nanocomposites based on AuNPs, which are applied for different purposes. In other words, nanocomposites are used to achieve more effective compositions. In the following, some of the most important ones will be introduced. It has been reported that chitosan-based AuNP composites have better stability against aggregation compared to citrate-stabilized AuNPs.49,50 Additionally, chitosan increases the biodegradability and cytocompatibility of the composite, whereas AuNPs improve the electronic, optical, mechanical, and catalytic properties of the composite.49,50 Chitosan can perform as a reducing agent and control the hydrolysis rate in producing oligosaccharide-based nanocomposites. Moreover, in aqueous solutions, oligosaccharides have greater mobility than polymers. Therefore, Au(III) is more dispersed in the presence of chitosan oligosaccharides, inhibiting aggregation events.51 Chitosan derivatives with electron-donor groups show higher efficiency in coordinating with Au(III) ions than unmodified chitosan. Besides, unmodified chitosan can reduce Au(III) through a complex procedure via hydrolysis.52 In recent years, metal-based nanoparticles, such as copper, gold, silver, platinum, and palladium, have been extensively used in medical approaches.53,54 Though, along with their advantages, metal-based nanoparticles have deficiencies that limit their helpful application. For instance, platinum and palladium nanoparticles are bacteriostatic, but their high cost and material shortage are the main problems.55,56 Additionally, silver nanoparticles have different antibacterial mechanisms, but their cytotoxicity restricts their practical application.12,56 Combinations of metal nanoparticles may improve these limitations and disadvantages.12 As an illustration, due to the action of solid electron ligands, the interaction between gold and silver nanoparticles can increase their antibacterial activity and enhance the effective concentration range.12 Surprisingly, adding a third metal can sometimes provide more improvement.12 Therefore, according to several studies, the simultaneous use of several nanoparticles with AuNPs increases the antibacterial activity of nanocomposites and significantly reduces the toxicity of the nanoparticles.
3.3. Development of antibacterial gold nanocomposite
AuNPs using Galaxaura elongata showed antibacterial activity in a critical study. In this work, AuNPs show a strong biocidal effect against some bacteria such as E. coli and K. pneumoniae.57 Fig. 5 reveals a nanozyme for antibacterial therapy using ultra-small AuNPs/metal–organic frameworks (UsAuNPs/MOFs hybrid). The formed nanocomposites exhibited acceptable peroxidase-like activity and acted as a valuable antibacterial agent against Gram-positive (S. aureus) and Gram-negative (E. coli) bacteria.58
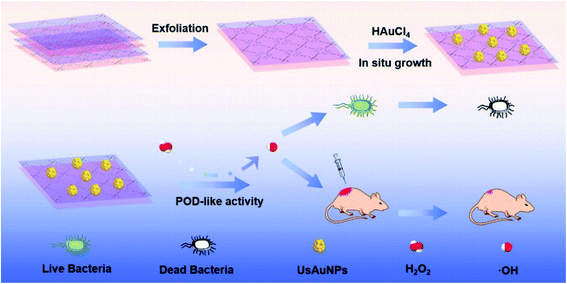 |
| Fig. 5 Schematic illustration of the preparation of UsAuNPs/MOFs hybrid for antibacterial therapy.58 | |
Green synthesis methods were applied and showed good activity against some Gram-negative bacterial strains.59 Good antibacterial activity was described from MnFe2O4@SiO2@Au nanocomposites against P. aeruginosa, K. pneumoniae and Proteus mirabilis bacteria. The recovery of photocatalytic activity of MnFe2O4@SiO2@Au after five cycles of operation seems remarkable. Fig. 6 illustrates the preparation steps used.60
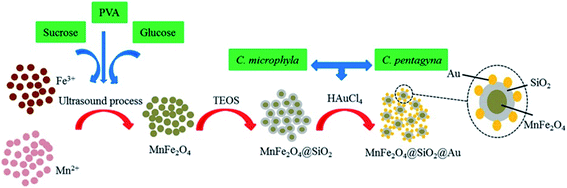 |
| Fig. 6 Schematic illustration of nanocomposite preparation for antibacterial activity.60 | |
The addition of Allium noeanum extract increased the solubility of AuNPs. It showed antibacterial properties against a wide range of bacteria, including Pseudomonas aeruginosa, Streptococcus pneumoniae, Bacillus subtilis, Salmonella typhimurium, Staphylococcus aureus, E. coli (O157:H7), Staphylococcus saprophyticus, and Shigella flexneri. In other words, gold nanocomposites increase the solubility and antibacterial and antioxidant properties of the A. noeanum extract.61 Photothermal and photodynamic antibacterial activities of Bi2S3 increased after coating with AuNPs. Hyperthermia and ROS are the main reasons for the antibacterial activity of the nanocomposites. The developed nanocomposites are applicable for environmental remedies and medical treatment with high antibacterial properties toward Staphylococcus aureus and E. coli.62 A photo-reduction protocol/hydrothermal approach was used for the synthesis of polydimethylsiloxane (PDMS-ZnO/Au). The produced nanostructure demonstrated exceptional anti-adhesive and antibacterial activity against E. coli.63 AuNPs-COOH/AgNO3 was produced for medical usage with great stability and low cytotoxicity. The antibacterial activity of the synthesized nanocomposites was evaluated against E. coli and S. aureus bacterial strains.64 A photocatalytic system comprising TNTs/Au/CDs (Fig. 7) was developed for a bactericidal approach. The proposed system produces ROS resulting in converting NIR light into light of 500–600 nm and transferring electrons and improving the surface plasmon resonance (SPR) effect of AuNPs to yield additional local photothermy.65
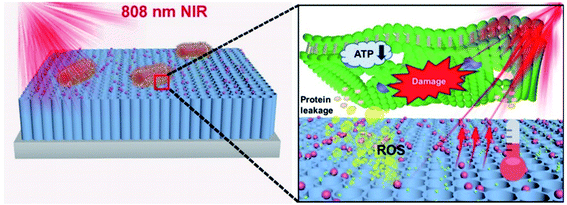 |
| Fig. 7 A schematic image of the antibacterial mechanism of TNTs/Au/CDs under 808 nm NIR light irradiation within 15 min.65 | |
For the preparation of ionic liquid functionalized AuNPs, a green synthesis methodology was employed for antibacterial and anticancer applications. In this system, an Ag–Au/CeO2 nanostructure was produced successfully with a maximum zone of inhibition against S. aureus and E. coli strains.66 Although pure gold and platinum nanoparticles have no specific antibacterial properties, their composition in the structure of nanocomposites without surface modification revealed antibacterial activity. The lack of ROS production is the most critical feature of the compound, which significantly reduces its toxicity to human cells, and its production promises a safe antibacterial compound.67 Nanohybrids composed of titanium dioxide/gold (TiO2/Au) nanoparticles were created for an antibacterial approach. The produced nanohybrid showed acceptable antibacterial activity and was applicable for marine antifouling paint and sewage treatment.68 Other nanohybrids include the TiO2–Au platform, which was developed for antibacterial and photocatalytic activity.69 Iron oxide gold nanocomposites (Fe3O4–Au NCs) were proposed for pharmaceutical applications and in the biomedical field. Planned nanosystems exhibit antibacterial activity toward a wide range of Gram-positive and Gram-negative pathogens.70 Crataegus monogyna leaf extract with AuNPs (CML@X-NPs, X = Ag, Au) revealed anticancer and antibacterial activity. The non-toxicity, and rapid, green and facile synthesis are the remarkable aspects of the created nanosystem.71 Hierarchical ZnO microspheres (ZMS) with AuNPs demonstrated antibacterial and photocatalytic activities. The produced nanohybrid displayed promising potential applications in environmental protection.72 Without ROS production, AuNPs and titania nanotubes (Au@TiO2-NT) were assembled for a durable antibacterial approach. Au@TiO2-NT was light-independent and applicable to the dark environment inside tissues, such as for orthopedic devices and implants.73 Liquid medium-based nanocomposites comprising propane-1,2,3-triol and silver/AuNPs were developed against E. coli and S. epidermidis. The results showed that AuNP4-6 have antibacterial activity and are able to prevent the growth of S. epidermidis.74 Coleus aromaticus has been applied as a bio-reductant for the synthesis of Au–Ag-based nanocomposites. In this work, the essential oil was used for the synthesis of nanocomposites against S. aureus and E. coli.75 To improve Au@Ag antibacterial activity, protein-coated graphene oxide was successfully applied in the nanosystem. The developed antibacterial nanocomposites revealed enhanced activity against E. coli, and were applicable to environmental samples.76 The elimination of biofilms and intracellular bacteria is one of the most important challenges in medical bacteriology. An antibacterial nanocomposite was developed against biofilms and intracellular bacteria. In this regard, phosphatidylcholine-decorated AuNPs loaded with gentamicin (GPA NPs) was used as a favorable antibacterial agent for the effective treatment of chronic infections.77 A green chemistry method was used to synthesize watermelon-based nanocomposites to eradicate E. coli and Staphylococcus epidermidis. The produced system showed acceptable antibacterial activity and potential pharmaceutical applications.78 Surface plasmon resonance (SPR) was employed for the synthesis of Au/TiO2 hetero-nanostructure composites. The established nanoscale system exhibited maximum photocatalytic activity and was applicable against E. coli, both in the dark and under visible light illumination.79 A nanofluid-based nanosystem including Au/Pt/Ag presented improved antibacterial activity against some microorganisms. An advanced tri-metallic composite (Fig. 8) can be utilized in several fields, such as pharmaceutical industries, environmental sciences, and medical examination.80
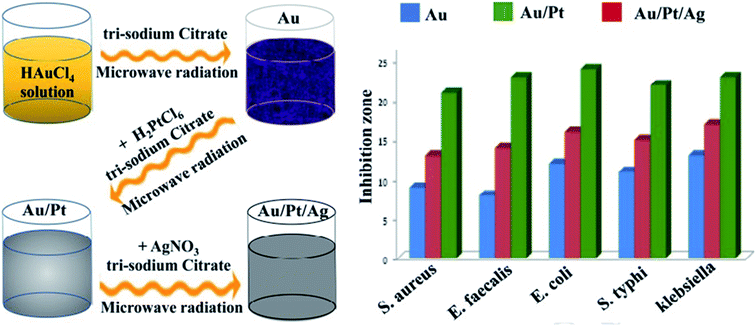 |
| Fig. 8 Schematic illustration of the antibacterial activity of synthesized trimetallic nanocomposites.80 | |
Anti-inflammatory and antibacterial activity was confirmed from TiO2 nanotubes mixed with AuNPs. Because of its improved anti-inflammatory and antibacterial aspects, the prepared nanosystem is a promising material to raise the success rates of orthopedic and dental implants.81 Light-activated AuNPs with methylene blue and silicone were assembled as an antibacterial agent. The findings revealed that the mixed nanoparticles act as a bactericidal component against E. coli and S. epidermidis.82 A cellulose paper based antibacterial platform coated in gold and silver nanoparticles was developed for bactericidal purposes. The advanced system was applicable for different applications, such as wound dressings, food packaging, personal care products and clothing.83 Anticancer, anti-inflammatory, antifungal, and antibacterial activities have been perceived in trimetallic TiO2@ZnO@Au nanocomposites. The proposed nanostructure may be utilized in several industries against Candida albicans, S. aureus and E. coli.84 The produced lipopeptide biosurfactant (LPB) via Acinetobacter junii B6 was employed for the production of gold-based nanocomposites. The results demonstrated that LPB significantly improved the antibacterial activity of the AuNPs.85 Recently, anticancer and antibacterial activities were described from AuNP-based nanocomposites. A mixture of aragonite and AuNPs showed acceptable stability and antibacterial activities.86 A research study revealed that chitosan–gold hybrid nanoparticles (CS–AuNPs) presented a synergetic affect against methicillin–resistant S. aureus.87 Hydrothermal synthesis of pseudo-nanocubes and hematite porous nanorods and their usage in antibacterial applications and visible-light driven photocatalysis were described. In this work, nanostructures were decorated equally with gold nanoparticles to improve their optical activity in visible-light illumination.88 This finding indicated that AgNPs possess high antibacterial activity only against E. coli. Whereas, AuNPs display only slight antibacterial activity against S. aureus and E. coli.89 Interestingly, synthesized encapsulated Au and Ag NPs showed both antibacterial and antifungal activities.90 Bio-planned gold nanoparticles showed antibacterial and cytotoxicity activities against Enterobacter cloacae, S. haemolyticus, and S. petrasii.91 Innovative research revealed that synthesized nanocomposites (Ag/Pt, Ag/Ir, Ag/PtIr, Au/Pt, Au/Ir, and Au/PtIr) presented antibacterial and cytotoxic activity.92 High antibacterial activity was presented by AuNPs synthesized via epigallocatechin 3-gallate. The produced nanocomposites showed antibacterial properties against S. aureus, Enterococcus faecalis, P. aeruginosa and E. coli (Table 3).93
Table 3 Antibacterial activity of AuNPs in some studiesa
Nanocomposites |
Bacteria |
Synthesis method |
Application |
Ref. |
NA: not available, (UsAuNPs/MOFs hybrid): ultra-small AuNPs/metal–organic frameworks, (PDMS-ZnO/Au): polydimethylsiloxane, (Fe3O4–Au NCs): iron oxide gold nanocomposites, (CML@X-NPs, X = Ag, Au): Crataegus monogyna leaf extract with gold nanoparticles, (LPB): lipopeptide biosurfactant, (CS–AuNPs): chitosan–gold hybrid nanoparticles, (MOCVD): metal–organic chemical vapor deposition, (EGCG): epigallocatechin 3-gallate. |
AuNPs |
Staphylococcus aureus and Pseudomonas aeruginosa |
Green synthesis method |
Environmental/biological |
57 |
UsAuNPs/MOFs hybrid |
S. aureus and E. coli |
Hydrothermal |
Wound healing, clinical |
94 |
Au, Ag, and Pd nanoparticles-carbon composite |
Gram-negative bacterial strains |
Green synthesis method |
Electrochemical energy storage applications |
59 |
MnFe2O4@SiO2@Au |
P. aeruginosa, Klebsiella pneumoniae and Proteus mirabilis bacteria |
Ultrasound-assisted precipitation |
Treatment of bacterial and fungal diseases |
61 |
Au@Bi2S3 |
S. aureus and E. coli |
Schottky junction via intermediate layer conversion method |
Environmental remedy and medical treatment |
62 |
PDMS-ZnO/Au |
E. coli |
Photo-reduction protocol/hydrothermal |
Biological contamination for catheter, medical paint, and implant tools |
63 |
AuNPs-COOH/AgNO3 |
E. coli and S. aureus |
Seeding method |
Nanomedicine engineering |
64 |
TNTs/Au/CDs |
S. aureus and E. coli |
NA |
Public health and medical disinfection |
65 |
Ag–Au/CeO2 |
S. aureus and E. coli strains |
Ionic liquid functionalized green synthesized |
Biological |
66 |
Au–Pt |
E. coli, P. aeruginosa, K. pneumoniae, and Salmonella choleraesuis |
Co-reduction |
Clinical |
67 |
Titania/TiO2 |
E. coli |
Hydrothermal method |
Marine antifouling paint sewage and treatment |
68 |
Au/TiO2 |
Bacillus subtilis |
NA |
Biological |
69 |
Fe3O4–Au NCs |
Gram-positive and Gram-negative pathogens |
Reduction method |
Water samples, pharmaceutical and medical applications |
70 |
CML@X-NPs, X = Ag, Au |
S. aureus, Enterococcus faecalis, P. aeruginosa, Acinebacter baumannii, E. coli, K. pneumoniae and P. mirabilis |
Green synthesis |
Biological, clinical |
71 |
ZMS-Au |
S. aureus and E. coli strains |
Layer-by-layer self-assembly |
Environmental protection |
72 |
Au@TiO2-NT |
S. aureus |
NA |
Orthopedic devices and implants |
73 |
Propane-1,2,3-triol and Ag/AuNPs |
E. coli and Staphylococcus epidermidis |
Size-controlled synthesis |
Environmental |
74 |
Au/Ag alloy nanoparticles using Coleus aromaticus |
S. aureus and E. coli |
NA |
Biological fields |
75 |
GO@Au@Ag |
E. coli |
NA |
Environmental |
76 |
GPA NPs |
Biofilms and intracellular bacteria |
Green synthesis |
Treatment of chronic infections |
77 |
Watermelon extract-AuNPs |
E. coli and S. epidermidis |
Biogenic synthesis |
Pharmaceutical applications |
78 |
Au/TiO2 |
E. coli |
Solvothermal method |
Biological fields |
79 |
Trimetallic Au/Pt/Ag |
Some microorganisms |
Chemical reduction method |
Pharmaceutical applications, environmental and medical |
80 |
TiO2–Au |
Bacterial adhesion and biofilm |
NA |
Orthopedic and dental implants |
81 |
Silicone-Au-Mb |
E. coli and S. epidermidis |
Swell-encapsulation-shrink |
NA |
82 |
Cellulose paper-Au-AgNPs |
E. coli |
NA |
Wound dressings, food packaging, personal care products and clothing |
83 |
TiO2@ZnO |
Candida albicans, S. aureus and E. coli |
NA |
Biological applications |
84 |
LPB-AuNPs |
Gram-positive and 4 Gram-negative bacterial strains |
NA |
Biological applications |
85 |
Aragonite-AuNPs |
Gram-negative e.g., Pseudomonas putida (P. putida), anti-biofilm |
Hydrothermal synthesis |
Medical application |
86 |
CS–AuNPs |
Methicillin–resistant S. aureus |
Co-reduction |
Medical application |
87 |
(α-Fe2O3)-AuNPs |
E. coli |
Hydrothermal |
Wastewater remediation applications |
88 |
Ag/AuNPs |
S. aureus and E. coli |
Citrate-capped nanoparticle synthesis |
Biological, clinical |
89 |
Ag/AuNPs |
Antibiotic-resistant bacteria and C. albicans |
Green synthesis |
Pharmaceutical applications, environmental and medical |
90 |
AuNPs |
Enterobacter cloacae, Staphylococcus haemolyticus and Staphylococcus petrasii |
Green synthesis |
Cell line studies, pharmaceutical applications |
91 |
Ag/Pt, Ag/Ir, Ag/PtIr, Au/Pt, Au/Ir, and Au/PtIr |
S. epidermidis, S. aureus, Streptococcus pyogenes, P. aeruginosa and Enterococcus faecium |
MOCVD |
Pharmaceutical and medical applications |
92 |
AuNPs-EGCG |
S. aureus, E. faecalis, P. aeruginosa and E. coli |
Green synthesis |
Biomedical and biological |
93 |
4. Conclusion
AuNPs are among the main nanomaterials utilized in nanobiotechnology. According to the above examples, gold nanocomposites have antibacterial properties. The most important factors in the development of antibacterial gold-based nanocomposites can be considered to be: (1) the facile fabrication with low cost is one of the most fundamental factors in the development of nanobiotechnology; therefore, the use of low-cost materials, such as carbon-based compounds, along with AuNPs should be considered. (2) The cytotoxicity of AuNPs is one of the most pressing challenges in its application in an in vivo environment; therefore, researchers should focus on reducing their toxicity. (3) Various studies have shown that gold nanoparticles in combination with different materials exhibited different antibacterial activities. For this reason, the choice of nanocomposite components is essential. (4) The biodegradability of nanocomposites is a prominent feature that should be considered in their development. (5) The size and morphology of AuNPs are directly related to their antibacterial activity. (6) The present review shows that the antibacterial activity of AuNPs has been studied against a small number of bacteria, so it is recommended that their antibacterial activity against important pathogenic bacteria should be considered.
5. Future prospects
Certainly, antibiotic resistance is one of the most important challenges in medicine today. Despite significant advances in the development of multiple antibiotics, the antibiotic resistance of bacteria is increasing. The present study shows that nanoparticles can be considered as suitable tools to overcome antibiotic status. Despite the exceptional properties of the antibacterial activity of nanoparticles, there are serious challenges that need to be overcome and considered in future studies. These challenges include: (1) although different types of gold nanocomposites prepared from AuNPs with different morphologies and sizes have been confirmed as antimicrobial agents, their antimicrobial activities still need to be improved. (2) Although the antibacterial properties of noble metal nanoparticles and their nanocomposites, especially gold nanocomposites, have been proven, these compounds are expensive and in some cases unusable, even for research projects. (3) The antimicrobial activity of AuNPs can be improved by the use of a synergistic effect between AuNPs and antimicrobial surface ligands. (4) According to the results, AuNPs bind non-specifically to the surface of the bacterial cell. In other words, they do not have any specific receptors for connection. This poses a significant challenge to the uptake of nanoparticles into the bacterial cell. (5) To date, there are several mechanisms to describe the antimicrobial performance of AuNPs. As a result, theoretical and experimental investigations of the metabolisms of AuNPs in bacteria are still unclear and require comprehensive study for a correct understanding of their antimicrobial activity. (6) Finally, serious work needs to be completed to expand the antimicrobial activity of AuNPs towards clinical application. In other words, we believe that gold nanocomposites can be used as antimicrobial agents in the clinic in the near future.
Conflicts of interest
The authors declare that they have no known competing financial interests or personal relationships that could have influenced the work reported in this paper.
Acknowledgements
The authors would like to thank the Aging Research Institute and the Physical Medicine Rehabilitation Research Center of Tabriz University of Medical Sciences.
References
- M. J. Hajipour, K. M. Fromm, A. A. Ashkarran, D. J. de Aberasturi, I. R. de Larramendi, T. Rojo, V. Serpooshan, W. J. Parak and M. Mahmoudi, Trends Biotechnol., 2012, 30, 499–511 CrossRef CAS PubMed.
- M. Yousefi, M. Dadashpour, M. Hejazi, M. Hasanzadeh and B. A. Behnam, Mokhtarzadeh. Mater Sci Engin: C, 2017, 74, 568–581 CrossRef CAS PubMed.
- U. Meyer-Hoffert, M. W. Hornef, B. Henriques-Normark, L.-G. Axelsson, T. Midtvedt, K. Pütsep and M. J. G. Andersson, Gut, 2008, 57, 764–771 CrossRef CAS PubMed.
- R. Dastjerdi and M. Montazer, Colloids Surf., B, 2010, 79, 5–18 CrossRef CAS PubMed.
- M. Sajid and J. Płotka-Wasylka, Microchem. J., 2020, 154, 104623 CrossRef CAS.
- P. Singh, A. Garg, S. Pandit, V. Mokkapati and I. Mijakovic, Nanomaterials, 2018, 8, 1009 CrossRef PubMed.
- I. Khan, K. Saeed and I. Khan, Arabian J. Chem., 2019, 12, 908–931 CrossRef CAS.
- H. Moustafa, A. M. Youssef, N. A. Darwish and A. I. Abou-Kandil, Composites, Part B, 2019, 172, 16–25 CrossRef CAS.
- M. Noruzi, Bioprocess Biosyst. Eng., 2015, 38, 1–14 CrossRef CAS PubMed.
- L. Freitas de Freitas, G. H. C. Varca, J. G. dos Santos Batista and A. Benévolo Lugão, Nanomaterials, 2018, 8, 939 CrossRef PubMed.
- Y. Elkhidir, R. Lai and Z. Feng, Heliyon, 2018, 4, e00662 CrossRef PubMed.
- Y. Bai, Y. Bai, C. Wang, J. Gao and W. Ma, J. Mater. Sci.: Mater. Med., 2016, 27, 31 CrossRef PubMed.
- H. Li, S. Pan, P. Xia, Y. Chang, C. Fu, W. Kong, Z. Yu, K. Wang, X. Yang and Z. Qi, J. Biol. Eng., 2020, 14, 14 CrossRef CAS PubMed.
- T. Russo, A. Gloria, R. De Santis, U. D'Amora, G. Balato, A. Vollaro, O. Oliviero, G. Improta, M. Triassi and L. Ambrosio, Bioact. Mater., 2017, 2, 156–161 CrossRef CAS PubMed.
- G. R. Rodrigues, C. López-Abarrategui, I. de la Serna Gómez, S. C. Dias, A. J. Otero-González and O. L. Franco, Int. J. Pharm., 2019, 555, 356–367 CrossRef CAS PubMed.
- L. Viganor, O. Howe, P. McCarron, M. McCann and M. Devereux, Curr. Top. Med. Chem., 2017, 17, 1280–1302 CrossRef CAS PubMed.
- R. Sinha, R. Karan, A. Sinha and S. K. Khare, Bioresour. Technol., 2011, 102, 1516–1520 CrossRef CAS PubMed.
- J. P. Ruparelia, A. K. Chatterjee, S. P. Duttagupta and S. Mukherji, Acta Biomater., 2008, 4, 707–716 CrossRef CAS PubMed.
- B. Wu, R. Huang, M. Sahu, X. Feng, P. Biswas and Y. J. Tang, Sci. Total Environ., 2010, 408, 1755–1758 CrossRef CAS PubMed.
- S. S. Khan, A. Mukherjee and N. Chandrasekaran, Colloids Surf., B, 2011, 87, 129–138 CrossRef CAS PubMed.
- K. Feris, C. Otto, J. Tinker, D. Wingett, A. Punnoose, A. Thurber, M. Kongara, M. Sabetian, B. Quinn and C. Hanna, Langmuir, 2010, 26, 4429–4436 CrossRef CAS PubMed.
- W. Jiang, H. Mashayekhi and B. Xing, Environ. Pollut., 2009, 157, 1619–1625 CrossRef CAS PubMed.
- Z. Wang, Y.-H. Lee, B. Wu, A. Horst, Y. Kang, Y. J. Tang and D.-R. Chen, Chemosphere, 2010, 80, 525–529 CrossRef CAS PubMed.
- A. Kumar, A. K. Pandey, S. S. Singh, R. Shanker and A. Dhawan, Chemosphere, 2011, 83, 1124–1132 CrossRef CAS PubMed.
- J. R. Morones, J. L. Elechiguerra, A. Camacho, K. Holt, J. B. Kouri, J. T. Ramírez and M. J. Yacaman, Nanotechnology, 2005, 16, 2346 CrossRef CAS PubMed.
- Y. Cui, Y. Zhao, Y. Tian, W. Zhang, X. Lü and X. Jiang, Biomaterials, 2012, 33, 2327–2333 CrossRef CAS PubMed.
- G. Von Maltzahn, J.-H. Park, A. Agrawal, N. K. Bandaru, S. K. Das, M. J. Sailor and S. N. Bhatia, Cancer Res., 2009, 69, 3892–3900 CrossRef CAS PubMed.
- A. Gupta, S. Pandey and J. S. Yadav, Adv. Pharm. Bull., 2020, 11, 10–27 CrossRef PubMed.
- J. Krawinkel, U. Richter, M. L. Torres-Mapa, M. Westermann, L. Gamrad, C. Rehbock, S. Barcikowski and A. Heisterkamp, J. Nanobiotechnol., 2016, 14, 1–14 CrossRef PubMed.
- C.-A. J. Lin, T.-Y. Yang, C.-H. Lee, S. H. Huang, R. A. Sperling, M. Zanella, J. K. Li, J.-L. Shen, H.-H. Wang and H.-I. Yeh, ACS Nano, 2009, 3, 395–401 CrossRef CAS PubMed.
- Z. L. Wang, Annu. Rev. Phys. Chem., 2004, 55, 159–196 CrossRef CAS PubMed.
- D. Sikdar, I. D. Rukhlenko, W. Cheng and M. Premaratne, Nanoscale Res. Lett., 2013, 8, 1–5 CrossRef PubMed.
- T. A. Erickson and J. W. Tunnell, Mixed Metal Nanomaterials, 2009, 3, 1–44 Search PubMed.
- Y. Kherde, Z. P. Aguilar, L. Zystein, H. M. Rodgers and W. Hamilton, Frontiers in Bioscience, 2014, 19, 1320–1344 CrossRef PubMed.
- K. Parveen, V. Banse and L. Ledwani, AIP Publishing LLC, 2016, 1724, 020048 Search PubMed.
- M. A. Dheyab, A. A. Aziz, P. M. Khaniabadi, M. S. Jameel, N. M. Ahmed and A. T. Ali, Mater. Res. Express, 2021, 8, 015009 CrossRef CAS.
- K. Lewis and A. M. Klibanov, Trends Biotechnol., 2005, 23, 343–348 CrossRef CAS PubMed.
- K. Chaloupka, Y. Malam and A. M. Seifalian, Trends Biotechnol., 2010, 28, 580–588 CrossRef CAS PubMed.
- L. Cui, P. Chen, S. Chen, Z. Yuan, C. Yu, B. Ren and K. Zhang, Anal. Chem., 2013, 85, 5436–5443 CrossRef CAS PubMed.
- R. L. Auten and J. M. Davis, Pediatr. Res., 2009, 66, 121–127 CrossRef CAS PubMed.
- M. P. Patil and G.-D. Kim, Appl. Microbiol. Biotechnol., 2017, 101, 79–92 CrossRef CAS PubMed.
- N. Jones, B. Ray, K. T. Ranjit and A. C. Manna, FEMS Microbiol. Lett., 2008, 279, 71–76 CrossRef CAS PubMed.
- S. Kang, M. Herzberg, D. F. Rodrigues and M. Elimelech, Langmuir, 2008, 24, 6409–6413 CrossRef CAS PubMed.
- S. Kumar, K. Gandhi and R. Kumar, Ind. Eng. Chem. Res., 2007, 46, 3128–3136 CrossRef CAS.
- B.-K. Pong, H. I. Elim, J.-X. Chong, W. Ji, B. L. Trout and J.-Y. Lee, J. Phys. Chem. C, 2007, 111, 6281–6287 CrossRef CAS.
- H. H. Lara, N. V. Ayala-Núnez, L. d. C. I. Turrent and C. R. Padilla, World J. Microbiol. Biotechnol., 2010, 26, 615–621 CrossRef CAS.
- Y. Zhang, T. P. Shareena Dasari, H. Deng and H. Yu, J. Environ. Sci. Health, Part C: Environ. Carcinog. Ecotoxicol. Rev., 2015, 33, 286–327 CrossRef CAS PubMed.
- E. A. Ortiz-Benítez, N. Velázquez-Guadarrama, N. V. Durán Figueroa, H. Quezada and J. d. J. Olivares-Trejo, Metallomics, 2019, 11, 1265–1276 CrossRef PubMed.
- X. Guo, Q. Zhuang, T. Ji, Y. Zhang, C. Li, Y. Wang, H. Li, H. Jia, Y. Liu and L. Du, Carbohydr. Polym., 2018, 195, 311–320 CrossRef CAS PubMed.
- U. A. Fahmy, AAPS PharmSciTech, 2018, 19, 3454–3461 CrossRef CAS PubMed.
- A. B. da Silva, K. B. Rufato, A. C. de Oliveira, P. R. Souza, E. P. da Silva, E. C. Muniz, B. H. Vilsinski and A. F. Martins, Int. J. Biol. Macromol., 2020, 161, 977–998 CrossRef CAS PubMed.
- N. J. Vickers, Curr. Biol., 2017, 27, R713–R715 CrossRef CAS PubMed.
- M. Ovais, A. T. Khalil, M. Ayaz, I. Ahmad, S. K. Nethi and S. Mukherjee, Int. J. Mol. Sci., 2018, 19, 4100 CrossRef PubMed.
- A. A. Yaqoob, K. Umar and M. N. M. Ibrahim, Appl. Nanosci., 2020, 10, 1369–1378 CrossRef CAS.
- J.-K. Sun and Q. Xu, Energy Environ. Sci., 2014, 7, 2071–2100 RSC.
- Z. Wang, K. Dong, Z. Liu, Y. Zhang, Z. Chen, H. Sun, J. Ren and X. Qu, Biomaterials, 2017, 113, 145–157 CrossRef CAS PubMed.
- N. Abdel-Raouf, N. M. Al-Enazi and I. B. Ibraheem, Arabian J. Chem., 2017, 10, S3029–S3039 CrossRef CAS.
- W.-C. Hu, M. R. Younis, Y. Zhou, C. Wang and X.-H. Xia, Small, 2020, 16, 2000553 CrossRef CAS PubMed.
- P. Anjana, M. Bindhu, M. Umadevi and R. Rakhi, Appl. Surf. Sci., 2019, 479, 96–104 CrossRef CAS.
- M. Shirzadi-Ahodashti, M. A. Ebrahimzadeh, S. M. Ghoreishi, A. Naghizadeh and S. Mortazavi-Derazkola, Appl. Organomet. Chem., 2020, 34, e5614 CAS.
- M. Shirzadi-Ahodashti, M. A. Ebrahimzadeh, S. M. Ghoreishi, A. Naghizadeh and S. Mortazavi-Derazkola, Appl. Organomet. Chem., 2020, 34, e5614 CAS.
- W.-N. Wang, P. Pei, Z.-Y. Chu, B.-J. Chen, H.-S. Qian, Z.-B. Zha, W. Zhou, T. Liu, M. Shao and H. Wang, Chem. Eng. J., 2020, 397, 125488 CrossRef CAS.
- Y. Tang, H. Sun, Z. Qin, S. Yin, L. Tian and Z. Liu, Chem. Eng. J., 2020, 398, 125575 CrossRef CAS.
- S. Panicker, I. Ahmady, C. Han, M. Chehimi and A. Mohamed, Mater. Today Chem., 2020, 16, 100237 CrossRef CAS.
- C. Jin, K. Su, L. Tan, X. Liu, Z. Cui, X. Yang, Z. Li, Y. Liang, S. Zhu and K. W. K. Yeung, Mater. Des., 2019, 177, 107845 CrossRef CAS.
- P. Nithya and M. Sundrarajan, J. Photochem. Photobiol., B, 2020, 202, 111706 CrossRef CAS PubMed.
- Y. Zhao, C. Ye, W. Liu, R. Chen and X. Jiang, Angew. Chem., Int. Ed., 2014, 53, 8127–8131 CrossRef CAS PubMed.
- Y. Tang, H. Sun, Y. Shang, S. Zeng, Z. Qin, S. Yin, J. Li, S. Liang, G. Lu and Z. Liu, J. Colloid Interface Sci., 2019, 535, 516–523 CrossRef CAS PubMed.
- L. Armelao, D. Barreca, G. Bottaro, A. Gasparotto, C. Maccato, C. Maragno, E. Tondello, U. L. Štangar, M. Bergant and D. Mahne, Nanotechnology, 2007, 18, 375709 CrossRef.
- D. A. Jency, K. Sathyavathi, M. Umadevi and R. Parimaladevi, Mater. Lett., 2020, 258, 126795 CrossRef.
- M. Shirzadi-Ahodashti, S. Mortazavi-Derazkola and M. A. Ebrahimzadeh, Surf. Interfaces, 2020, 21, 100697 CrossRef CAS.
- Y. Wang, H.-B. Fang, Y.-Z. Zheng, R. Ye, X. Tao and J.-F. Chen, Nanoscale, 2015, 7, 19118–19128 RSC.
- G. Wang, H. Feng, W. Jin, A. Gao, X. Peng, W. Li, H. Wu, Z. Li and P. K. Chu, Appl. Surf. Sci., 2017, 414, 230–237 CrossRef CAS.
- J. Siegel, K. Kolářová, V. Vosmanská, S. Rimpelová, J. Leitner and V. Švorčík, Mater. Lett., 2013, 113, 59–62 CrossRef CAS.
- V. Vilas, D. Philip and J. Mathew, J. Mol. Liq., 2016, 221, 179–189 CrossRef CAS.
- H. Wang, J. Liu, X. Wu, Z. Tong and Z. Deng, Nanotechnology, 2013, 24, 205102 CrossRef PubMed.
- H. Mu, J. Tang, Q. Liu, C. Sun, T. Wang and J. Duan, Sci. Rep., 2016, 6, 1–9 CrossRef PubMed.
- W. Chums-ard, D. Fawcett, C. C. Fung and G. E. J. Poinern, International Journal of Research in Medical Sciences, 2019, 7, 2499–2505 CrossRef.
- J. Zhang, X. Suo, J. Zhang, B. Han, P. Li, Y. Xue and H. Shi, Mater. Lett., 2016, 162, 235–237 CrossRef CAS.
- N. Yadav, A. K. Jaiswal, K. K. Dey, V. B. Yadav, G. Nath, A. K. Srivastava and R. R. Yadav, Mater. Chem. Phys., 2018, 218, 10–17 CrossRef CAS.
- W. Xu, M. Qi, X. Li, X. Liu, L. Wang, W. Yu, M. Liu, L. A. Yang, Y. Zhou and Y. Song, J. Electroanal. Chem., 2019, 842, 66–73 CrossRef CAS.
- S. Perni, C. Piccirillo, A. Kafizas, M. Uppal, J. Pratten, M. Wilson and I. P. Parkin, J. Cluster Sci., 2010, 21, 427–438 CrossRef CAS.
- T. T. Tsai, T. H. Huang, C. J. Chang, N. Yi-Ju Ho, Y. T. Tseng and C. F. Chen, Sci. Rep., 2017, 7, 3155 CrossRef PubMed.
- C. Pragathiswaran, C. Smitha, H. Barabadi, M. M. Al-Ansari, L. A. Al-Humaid and M. Saravanan, Inorg. Chem. Commun., 2020, 121, 108210 CrossRef CAS.
- M. Ohadi, H. Forootanfar, G. Dehghannoudeh, T. Eslaminejad, A. Ameri, M. Shakibaie and A. Najafi, BioNanoScience, 2020, 10, 899–908 CrossRef.
- A. Samanta, S. Podder, M. Kumarasamy, C. K. Ghosh, D. Lahiri, P. Roy, S. Bhattacharjee, J. Ghosh and A. K. Mukhopadhyay, Mater. Sci. Eng., C, 2019, 103, 109734 CrossRef CAS PubMed.
- M. A. M. Hussein, M. Grinholc, A. S. A. Dena, I. M. El-Sherbiny and M. Megahed, Carbohydr. Polym., 2021, 256, 117498 CrossRef CAS PubMed.
- E. Alp, R. İmamoğlu, U. Savacı, S. Turan, M. K. Kazmanlı and A. Genç, 2021, 852, 157021.
- J. Gouyau, R. E. Duval, A. Boudier and E. Lamouroux, Int. J. Mol. Sci., 2021, 22, 1905 CrossRef CAS PubMed.
- D. G. Téllez-de-Jesús, N. S. Flores-Lopez, J. A. Cervantes-Chávez and A. R. Hernández-Martínez, Surf. Interfaces, 2021, 27, 101456 CrossRef.
- R. Vinayagam, M. Santhoshkumar, K. E. Lee, E. David and S. G. Kang, Bioprocess Biosyst. Eng., 2021, 44, 1253–1262 CrossRef CAS PubMed.
- S. I. Dorovskikh, E. S. Vikulova, E. V. Chepeleva, M. B. Vasilieva, D. A. Nasimov, E. A. Maksimovskii, A. R. Tsygankova, T. V. Basova, D. S. Sergeevichev and N. B. Morozova, 2021, 9, 851.
- S. R. R. Avila, G. P. D. Schuenck, L. P. C. e. Silva, W. J. Keijok, L. M. Xavier, D. C. Endringer, J. P. Oliveira, R. P. Schuenck and M. C. C. Guimarães, J. Mater. Res., 2021, 36, 518–532 CrossRef CAS.
- W. C. Hu, M. R. Younis, Y. Zhou, C. Wang and X. H. Xia, Small, 2020, 16, 2000553 CrossRef CAS PubMed.
|
This journal is © The Royal Society of Chemistry 2021 |