DOI:
10.1039/D1QI00666E
(Research Article)
Inorg. Chem. Front., 2021,
8, 4052-4061
A bifunctional robust metal sulfide with highly selective capture of Pb2+ ions and luminescence sensing ability for heavy metals in aqueous media†
Received
25th May 2021
, Accepted 28th July 2021
First published on 29th July 2021
Abstract
Heavy metal ions represent hazardous and harmful contaminants for living organisms and the environment and thus, it is of urgent need to develop new materials sufficient to detect and remove them efficiently. Herein, we report the metal sulfide ion exchanger (MSIE) HxGaxGe4−xS8·yH2O (protonated UCR-20, p-UCR-20) which demonstrates exceptional capability for both detection and removal of heavy metals from aqueous samples. This material exhibited exceptional fast sorption kinetics (≤1 min), high sorption capacity (∼527 mg g−1) and remarkable selectivity for Pb2+ towards various common alkali and alkaline earth cations and in highly acidic to alkaline conditions. Even more importantly, p-UCR-20 in its composite form with calcium alginate was used as stationary phase (along with silica sand) in a fixed-bed ion exchange column and was found capable of eliminating traces of Pb2+ from a large volume of a wastewater simulant solution. Photophysical studies revealed that p-UCR-20 shows excellent luminescence sensing properties for Pb2+, achieving limits of detection below the acceptable Pb levels in water and such efficient sensing properties are largely retained even in the presence of several antagonistic species in large excess. In addition, p-UCR-20 displays highly efficient sensing properties for additional heavy metal ions, such as Ni2+ and Cd2+. Overall, the results derived from the current study reveal for the first time that MSIES may be a source not only of excellent sorbents but also exceptional luminescent sensors for heavy metal ions in aqueous media.
Introduction
Access to clean freshwater represents a key challenge for the upcoming decades; therefore, the treatment of wastewater is currently widely investigated to compensate for dwindling water supplies, as it is a reliable alternative water source. The presence of heavy metals in aqueous ecosystems poses a major threat, due to their high toxicity, non-biodegradability, discharge and accumulation in living beings.1–5 This issue is ever-increasing in less developed countries where many industrial residues containing toxic heavy metals are often released into the environment without suitable purification, due to the lack of proper regulations and the high cost of the treatment technologies. Lead (Pb), owing to its physical properties including high strength, high pliability and low melting point, is one of the most desirable industrial raw material. Lead is still being used in battery manufacturing, metal plating, painting, ceramic and glass industries printing, and mining activities.6 At the same time, Pb is considered as a very common and harmful element found in the environment, causing several undesired outcomes to brain, bones, kidneys, blood and also to cardiovascular, nervous and reproductive system.7 The World Health Organization – WHO and the European Environmental Agency-EEA have set the maximum contaminant limit for Pb to 10 ppb, with the US Environmental Protection Agency-US EPA proposing a limit value of 15 ppb, while the maximum contaminant level goal is zero.8 Since the use of Pb is still inevitable for the industrial activities, the efficient removal of Pb2+ ions from aqueous environment is of great importance. In the direction of achieving the zero limit, several state-of the-art methods and materials have been utilized for the removal of Pb2+ species from contaminated water. These methods include sorption,9,10 ion exchange,11–13 chemical precipitation,14,15 membrane filtration,16 and coagulation-flocculation.17 Among them, sorption and ion exchange are simply operated, efficient and economic methods. Sulfur-containing materials represent attractive Pb2+ sorbents, considering that Pb2+, as a soft acid, can form strong covalent bonds with soft S2− groups. Metal sulfide ion exchangers (MSIEs) are the leaders of this category, as they own soft S2− ligands and do not require additional functional groups into their frameworks.18 So far, MSIEs with layered structures have been extensively studied for Pb2+ ion exchange, indicating excellent sorption capability.18,19 In contrast, MSIEs with 3-D structures have been rarely studied for their heavy metal ion sorption properties.20
On the other hand, detection of heavy metal ions in water is also highly significant. Recent advances in optical technologies allow the design and construction of miniaturized, portable light detection devices. This fact, coupled with the ease and sensitivity with which light can be detected, has led to an increased interest in sensor systems based on luminescence.21–23 Such sensors rely on the recording of changes in the emission signal of a luminescent material (often termed as sensor element) upon its, usually reversible, interaction with a targeted analyte. These analyte-induced changes in luminescence may subsequently be processed by an electronic system thereby allowing the analyte to be recognized and quantified. The most important property of a good sensory material is its ability to selectively bind a targeted species in such a way as to induce a perturbation in its electronic properties, which, in turn, leads to measurable changes in the material's emission signal. The most widely studied luminescent sensory materials are those based on small molecules bearing analyte-binding moieties24,25 and porous fluorescent conjugated polymers.26 Recently, there has been an increasing interest in luminescent metal–organic frameworks (LMOFs) for sensing applications as these materials combine permanent porosity, and therefore the ability to bind guest species, with luminescence properties.27–29 Up to now, MSIE materials have not been investigated as sensors for heavy metals ions. This is not unexpected as only a handful of MSIEs are efficiently luminescent.
In an earlier study, Feng et al. reported a series of microporous and luminescent metal sulfides with the general formula (GaxGe4−xS8)x− (with the anionic charge of the framework balanced by the presence of organic ammonium cations), denoted as UCR-20.30 The K+-containing UCR-20 material has been recently studied for Cs+ ion exchange showing highly selective sorption capacity for this cation.31 However, no heavy metal ion sorption nor luminescence sensing investigations have been reported for such materials, even though their porous structures with plenty of S2− ligands make them particularly attractive for such investigations.
Herein we report the proton-containing version of UCR-20 material HxGaxGe4−xS8·yH2O (x = 3.25) (p-UCR-20). As a result of the replacement of bulky organic cations with the labile H+, p-UCR-20 displayed an exceptional performance towards Pb2+ uptake from aqueous media with extremely rapid sorption kinetics (≤1 min) and high sorption capacity (>500 mg g−1). Importantly, p-UCR-20, in its composite form with alginate, was utilized in an ion exchange column and showed capability for quantitative removal of Pb2+ traces (∼100 ppb) from large quantity of simulated wastewater. In addition, for the first time we demonstrate the highly efficient luminescence sensing properties of a metal sulfide ion exchanger. Thus, p-UCR-20 was found particularly effective for the luminescence based-detection of heavy metal ions in concentrations well below their acceptable levels in water, even in the presence of various competitive cationic species. These results indicate that MSIEs may constitute not only efficient sorbents, but also promising luminescent sensors for heavy metal ions.
Experimental section
Materials
All reagents and solvents were commercially available and used as received.
Synthesis of p-UCR-20.
The synthesis UCR-20GaGeS-AEP has been done as reported in literature.30 The synthesis of p-UCR-20 was achieved via treatment of UCR-20 with HCl acid. 20 mg (0.023 mmol) of UCR-20 are immersed into an aqueous solution of HCl (pH ∼ 2, 10 ml) and kept under stirring for 2 d. The brownish powder was isolated via centrifugation, rinsed several times with water and dried under vacuum. Yield: 19 mg.
Synthesis of p-UCR-20/CA composite.
20 mg of sodium alginate (SA) was dissolved in 40 mL of warm water. 96 mg (0.15 mmol) p-UCR-20 was dispersed in 8 ml of the SA solution. To this suspension, 96 mg (0.87 mmol) of CaCl2 was added under continuous stirring. The composite p-UCR-20-CA was immediately precipitated, collected by centrifugation, washed with water and acetone and dried under vacuum. Yield: 89 mg.
Characterization of p-UCR-20.
PXRD diffraction patterns were recorded on a Bruker D2 Phaser X-ray diffractometer (CuKα radiation, wavelength = 1.54184 Å). IR spectra were recorded on KBr pellets in the 4000–400 cm−1 range using a PerkinElmer Spectrum GX spectrometer. Thermogravimetric analyses (TGA) were performed on a PerkinElmer Diamond system. Thermal analysis was conducted from 30 to 600 °C in nitrogen atmosphere (100 mL min−1 flow rate) with a heating rate of 10 °C min−1. Energy dispersive spectroscopy (EDS) analyses were performed on a JEOL JSM6390LV scanning electron microscope (SEM) equipped with an Oxford INCA PentaFET-x3 energy dispersive X-ray spectroscopy (EDS) detector. Data acquisition was performed with an accelerating voltage of 20 kV and 120 s accumulation time.
Batch ion-exchange studies/Pb2+ ion-exchange experiments with p-UCR-20
Preparation of the Pb2+, Ni2+, Cd2+, Zn2+ and Cu2+ solutions.
A standard Pb2+ aqueous solution (C = 1000 ppm) was prepared via dissolution of 159.8 mg of Pb(NO3)2 in 100 ml distilled H2O. Likewise, we prepared standard aqueous solutions of Ni2+, Cd2+, Zn2+ and Cu2+, by using NiCl2, Cd(NO3)2, Zn(NO3)2·6H2O and Cu(NO3)2·3H2O, respectively. Metal ions solutions of 1 ppm concentration were prepared via dilution of the standard metal ions solutions.
Batch sorption kinetics.
For the determination of the sorption kinetics, Pb2+ ion-exchange experiments of various reaction times (1–20 min) have been performed. For each experiment, a 10 mL sample of Pb2+ solution (initial Pb2+ concentration = 1 ppm, pH = 7 ± 0.02) was added to each vial (containing 10 mg of p-UCR-20) and the mixtures were kept under magnetic stirring for the designated reaction times.
Sorption isotherms.
The Pb2+ uptake from solutions of various concentrations (1–1000 ppm) was studied by the batch method at V
:
m ∼ 1000 mL g−1, room temperature and 10 min contact. These data were used for the determination of Pb2+ sorption isotherms.
Effect of pH.
The variable pH ion exchange experiments were also carried out with the batch method at V
:
m ratio ∼ 1000 mL g−1, room temperature and 20 min contact (initial Pb2+ concentration = 1 ppm). The pH values were adjusted using HCl and NaOH aqueous solutions.
Selectivity study.
The selectivity of p-UCR-20 towards several common cations was investigated through individual and competitive ion-exchange experiments in distilled and natural spring water. The composition of natural spring water was the following: hardness: 257 ppm (as CaCO3); pH = 7.4; cations: Ca2+ = 100 ppm, Na+ = 2.82 ppm, Mg2+ = 1.83 ppm, K+ = 0.52 ppm, NH4+ < 0.013 ppm; anions: HCO3− = 281 ppm, Cl− < 8.26 ppm, SO42− = 12.9 ppm, NO3− = 8.65 ppm, NO2− < 0.06 ppm. For the competitive experiments, a mixture of 1 ppm each of Pb2+, Ni2+, Cd2+, Zn2+ and Cu2+ was added to 10 ml water, containing 10 mg of p-UCR-20.
Determination of metal content.
The suspensions from the various sorption reactions were filtrated and the resulting solutions were analysed for their Pb2+, Ni2+, Cd2+, Zn2+ and Cu2+ content with anodic stripping voltammetry, using Trace Metal Analyzer (797 VA Computrace, Metrohm AG Ltd, Switzerland). More specifically, a three-electrode system was used comprising Hanging Mercury Drop Electrode (HMDE) as working electrode, Platinum (Pt) as auxiliary electrode and Ag/AgCl/KCl (3 mol L−1) as reference electrode. The analysis has been done following the protocols developed by Metrohm (http://www.metrohm.com/en/applications/#). Each sorption experiment has been done at least twice and the reported sorption data represent the average of sorption results from the different sorption experiments. The difference between the concentrations of metal ions determined for the different sorption experiments was <2%.
Pb2+ leaching studies.
The Pb2+ leaching tests were conducted in distilled water and groundwater simulant solution at room temperature and 24 h contact. 100 mg of the loaded material were immersed in 100 ml distilled water (test 1) and 100 ml groundwater simulant solution (test 2) and kept under stirring for 24 h. The suspensions from both tests were filtrated and the resulting solutions were analysed for their Pb2+ content with anodic stripping voltammetry, using Trace Metal Analyzer (797 VA Computrace, Metrohm AG Ltd, Switzerland).
Preparation of the column.
The stationary phase of the column comprised a mixture of 50 mg of p-UCR-20-CA and 5 g of sand (50–70 mesh SiO2, 0.7 cm ID column, height = 9 cm). The column sorption studies were carried out with a wastewater simulant solution containing: 3 mM NaHCO3, 0.143 mM NaNO3, 10−3 mM NaH2PO4·H2O, 5 × 10−2 mM NaF, 0.33 mM Na2SiO3·5H2O, 0.99 mM CaCl2·2H2O, 5 mM MgSO4·7H2O, 4.8 × 10−4 mM Pb2+.32 The pH of this solution was adjusted to 7 ± 0.02. Several bed volumes of the solution were passed through the column and the effluents collected at the bottom in glass vials and measured with anodic stripping voltammetry. The flow rate in the column sorption studies was 1 mL min−1.
Preparation of p-UCR-20 suspension for fluorescence titration measurements.
5 mg (0.008 mmol) of p-UCR-20 were dispersed in 5 mL H2O containing 50 mg (0.14 mmol) of Cetyltrimethylammonium Bromide (CTAB) and stirred for 24 h. Part of the p-UCR-20-CTAB solution was further diluted roughly by ten times in distilled water. The concentration was tuned to achieve a ∼10% absorption at the wavelength of excitation corresponding to a 1 cm path length. A 5 ml portion of the final solution was placed in a 1 × 1 × 5 cm quartz cuvette for the fluorescence quenching experiments and it was magnetically stirred continuously during the measurements. Details for the experimental setup (Fig. S1, ESI†) and the procedure for the fluorescence titration measurements are given in ESI.†
Results and discussion
Synthesis
As we have already mentioned in the introduction, 3-D MSIE materials have been rarely applied in heavy metal ion exchange.15 In addition, MSIEs in general have not been studied as luminescent sensors. Being motivated from this lack, we decided to investigate UCR-20, as a representative MSIE with a porous 3-D framework, for its ability not only to remove but also to determine a series of emergent contaminants. UCR-20 displays a 3-D four-connected network based on corner-sharing T2 (M4S10) supertetrahedral units.30 The large pores of UCR-20 are filled with bulky organic ammonium cations. Since we aimed in a material with fast sorption kinetics, we decided to replace the relatively large organic guests with proton ions, the smallest possible cationic species. To this end, we treated UCR-20 with an acidic aqueous solution (10−2 M HCl), resulting in the release of organic counterions and the insertion of proton species (Fig. 1). The isolated proton-containing material (p-UCR-20) was proved to be an excellent sorbent and highly efficient luminescence sensor for heavy metal ions, as it will be shown below.
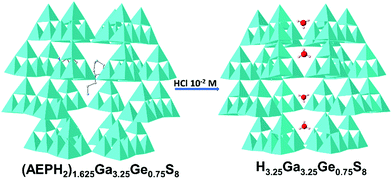 |
| Fig. 1 Schematic representation of the synthesis of p-UCR-20 by protonation of UCR-20. | |
Characterization of p-UCR-20 and Pb2+-loaded materials
The PXRD pattern of p-UCR-20 is very similar to that calculated from the crystal structure of UCR-20 (Fig. 2), indicating that the structure of pristine material remained intact after the protonation process. The characteristic IR peaks of AEP between 2700–3000 cm−1 are almost eliminated after protonation of UCR-20, which supports the near-complete replacement of AEPH22+ by H+ cations (Fig. 3). TGA data of p-UCR-20 revealed a sharp weight loss up to 120 °C, which is assigned to the release of water molecules (4.7 moles per formula unit). In contrast, pristine UCR-20 shows almost no weight loss in this temperature range, as its pores contain no water and are filled exclusively by AEPH22+ cations (Fig. 4).
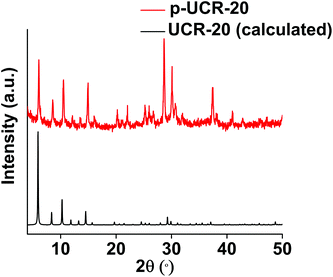 |
| Fig. 2 PXRD patterns of UCR-20 (calculated) and p-UCR-20. | |
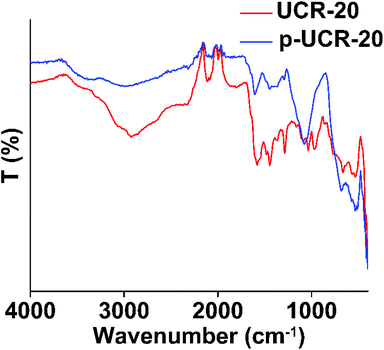 |
| Fig. 3 FTIR spectra of UCR-20 and p-UCR-20. | |
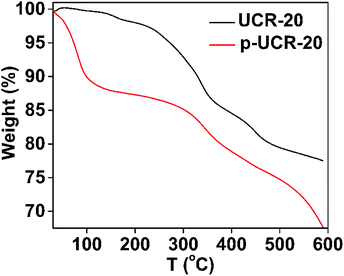 |
| Fig. 4 TGA data of UCR-20 and p-UCR-20. | |
The Pb2+-exchanged material was characterized with SEM-EDS and PXRD. EDS data and mapping images confirmed the presence of Pb2+ in the loaded material (Fig. S2 and 3†). PXRD data (Fig. S4a†) revealed that the structure of p-UCR-20 is preserved when the material is treated with low Pb2+ concentrations (up to 1 ppm), whereas the crystallinity of the compound is deteriorated upon its treatment with highly concentrated (1000 ppm) Pb2+ solutions (Fig. S4b†).
Pb2+ exchange studies of the p-UCR-20
Batch sorption kinetics.
The sorption investigations of the p-UCR-20 towards Pb2+ were initiated with the determination of sorption kinetics through variable time experiments from 1 to 20 min. Surprisingly, no residues of Pb2+ were detected at the solutions, even with an extremely short (1 min) treatment of p-UCR-20 with the Pb2+ solution (initial concentration = 1 ppm, pH = 7 ± 0.02). It is worth mentioning that the detection limit of anodic stripping voltammetry, used for the determination of Pb2+, is as low as 100 ppt. Consequently, p-UCR-20 displays extraordinary fast sorption kinetics, as it is capable to remove ≥99.9999% of the initial Pb2+ content within only 1 minute of contact.
Afterwards, we examined the sorption kinetics of the pristine UCR-20 under the same conditions. The pristine material displayed also rapid kinetics with 99.97% of the initial Pb2+ content been removed within only 1 minute, but the remaining Pb2+ content (∼30 ppb) was higher than the acceptable limit for water (10–15 ppb). Even after 20 min of contact we were still able to detect ∼2 ppb of Pb2+ at the solution. The above data confirmed our initial hypothesis that the protonation will enhance the sorption kinetics of the UCR-20 material, as a result of the inclusion of smaller size and more labile extra-framework cations (i.e. H+ ions). Note that hard H+ ions may interact weakly with the soft S2− ions of the metal sulfide framework, thus being particularly amenable to be replaced by soft heavy metal ions.
Effect of pH.
The Pb2+ exchange studies for p-UCR-20 were mainly carried out at pH = 7 ± 0.02, to reproduce the conditions usually found in natural waters. However, the Pb2+ removal ability of p-UCR-20 was also examined in additional pH values ranging from 0 to 10 (Fig. 6). Specifically, p-UCR-20 retains high removal capability for Pb2+ in pH range from 1 to 10 (±0.02), with more than ∼97% of the initial Pb2+ content being removed. Surprisingly, the removal percentage in pH = 0 was estimated to be ∼40% (initial Pb concentration = 1 ppm), indicating an exceptional sorbent even under extreme acidic conditions. This is attributed to the soft S2− ligands of p-UCR-20 framework showing weak interactions with the hard H+ ions.
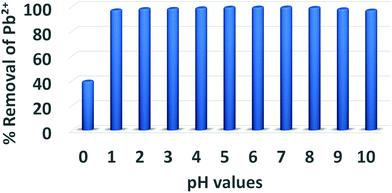 |
| Fig. 6 % Pb2+ removal by p-UCR-20vs. pH. | |
Effect of ionic strength-selectivity studies.
We have also investigated the effect of ionic strength on the Pb2+ sorption capability of p-UCR-20. To this end, we have studied Pb2+ sorption from natural spring water solutions artificially contaminated with Pb2+ (initial concentration = 1 ppm). Note that these solutions contained several alkaline and alkaline earth cations in relatively high concentrations and large excess (up to 500-fold) in relation to the Pb content (the composition of natural spring water samples is given in Experimental section). The results revealed that p-UCR-20 was able to remove 99.4% of the initial Pb2+ amount from these samples, thus indicating no effect of the high ionic strength on Pb2+ sorption. In addition, we have examined the selectivity of p-UCR-20 for Pb2+ over other heavy metal ions. Experiments were conducted in 10 ml distilled water containing a mixture of Pb2+, Ni2+, Cd2+, Zn2+ and Cu2+ (each with an initial concentration of 1 ppm) and 10 mg of p-UCR-20. The removal percentages were calculated to be 99.95%, 93%, 99.74%, 77% and 82.36% for Pb2+, Ni2+, Cd2+, Zn2+ and Cu2+, respectively (Table 1). As a further step to our investigations, we repeated the above experiments using natural spring water samples intentionally contaminated by 1 ppm for each of the examined heavy metal ions, to simulate the real-world wastewater conditions. The results showed removal percentages of 99.4%, 95.5%, 99.7%, 76.1% and 84.5% for Pb2+, Ni2+, Cd2+, Zn2+ and Cu2+, respectively (Table 1). The above results indicated p-UCR-20 as an excellent sorbent for Pb2+ even in the presence of several competitive cations. Importantly, in all competitive sorption experiments, the final Pb concentrations were found well-below the acceptable Pb levels in water. In parallel, p-UCR-20 was also highly efficient for removal of additional toxic metal ions such as Cd2+ and Ni2+.
Table 1 Results of the ion exchange selectivity studies
Sample |
C
0 (ppb) |
C
e (ppb) |
% removal |
Distilled water.
Natural spring water (its composition is provided in Experimental section.
Initial concentration of each ion.
|
Mixture of ionsa |
1000c |
0.5(Pb), 2.54(Cd), 67(Ni), 176(Cu), 230(Zn) |
99.95(Pb), 99.74(Cd), 93(Ni), 82.36(Cu), 77(Zn) |
Mixture of ionsb |
1000c |
6(Pb), 3(Cd), 45(Ni), 155(Cu), 239(Zn) |
99.4(Pb), 99.7(Cd), 95.5(Ni), 84.5(Cu), 76.1 (Zn) |
Column ion exchange study.
The promising results obtained from batch ion exchange studies motivated us to explore the capability of p-UCR-20 to uptake Pb2+ using fixed-bed ion exchange columns, which are usually employed in industrial wastewater processes. Note that MSIEs reported so far in literature have been rarely studied for heavy metal ion sorption under continuous flow conditions. In fact, as-prepared MSIEs cannot be used as stationary phase in ion exchange columns due to the small size of their particles (with a few exceptions)35,36 and their tendency to form fine water suspensions.20,37 Indeed, the material p-UCR-20 is isolated as very light powder that forms fine suspensions in the water, thus flowing out of the prepared columns. To overcome this drawback and utilize p-UCR-20 as a stationary phase, we transformed it into a composite material with calcium alginate (CA) which is not easily dispersed in water, since its particles are covered by CA shell.20,37,38 The composite p-UCR-20-CA was prepared with only 4 wt% of CA. Thus, the alginate shell was not thick enough to prevent the diffusion of ions into the frameworks’ pores and thus, the material retained its exceptional sorption kinetics.
As we have done in previous studies with alginate composites,20,37–39 the stationary phase in the column consisted of the composite material p-UCR-20-CA and silica sand (p-UCR-20-CA : sand mass ratio = 1
:
100). The column study was conducted with a ground water simulant solution (its composition is given in the Experimental section) containing traces of Pb2+ (initial Pb2+ concentration = 100 ppb). Besides Pb2+ cations, the simulant solution contained a variety of competitive cations with concentrations up to 104 times higher than that of Pb2+. The results indicated that 0.95 L of the solution passed through the column exhibited a concentration <10 ppb, i.e. below the allowed WHO and EU levels for Pb2+ in water (Fig. 7). Furthermore, no traces of Pb2+ were detected before ∼0.6 L of simulant solution passed through the column. These results are particularly significant considering that (a) only 50 mg of the p-UCR-20 were efficient to decontaminate almost 1 L of wastewater and (b) industrial wastewater treatment processes demand materials that can be utilized successfully in ion exchange columns.
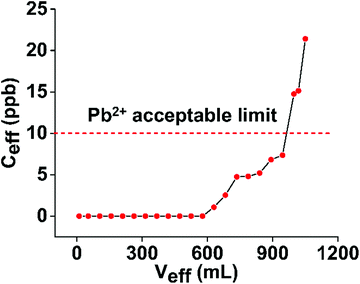 |
| Fig. 7 Column sorption data with a wastewater simulant solution (flow rate ∼1.0 mL min−1, one bed volume = 3.5 mL, stationary phase p-UCR-20-CA/sand = 0.05 g : 5 g, initial Pb concentration = 100 ppb). Veff and Ceff represent the volume (mL) and Pb2+ concentration (ppb) of the solution passed through the column (i.e. effluent) respectively. | |
Mechanism of Pb2+ sorption by p-UCR-20
The results from the sorption studies indicate that the process proceeds via a rapid ion exchange between the labile H+ and Pb2+ species (see eqn (5)). The capture of Pb2+ by p-UCR-20 is facilitated by the highly porous structure of this material and the presence of numerous S2− ligands. The latter constitute binding sites for soft heavy metal ions such as Pb2+, as revealed by several studies with MSIES.20 In addition, the sorption of Pb2+ by p-UCR-20 in either batch or continuous flow conditions is not affected by the presence of several cationic species such as H+, Na+, Ca2+, Mg2+etc. This is attributed to the particularly weak interactions of hard cations with the soft sulfide-based framework of p-UCR-20. In contrast, the latter interacts particularly strongly with the soft Pb2+, thus leading to the exceptional selectivity of p-UCR-20 towards this cation.
Comparison of p-UCR-20 with other Pb2+ sorbents
A comparison of the sorption capacities, as well as other important sorption properties, of various Pb2+ sorbents are provided in Table S2.† It can be seen that the maximum sorption capacity of p-UCR-20 (527 mg g−1) compares well with those of most efficient Pb2+ sorbents.40–42 In addition, p-UCR-20 represents the most effective Pb2+ sorbent among the metal sulfide ion exchangers, since it displays the fastest sorption kinetics (the equilibrium is reached within only 1 min) as well the highest sorption capacity.18,43 Furthermore, p-UCR-20 is the only MSIE tested so far for the removal of Pb2+ under flow conditions, i.e. under conditions simulating the practical wastewater treatment.
Reusability-Pb2+ leaching studies
The regeneration of p-UCR-20 after the Pb2+ sorption was investigated by treatment with dilute acidic solutions (0.01–0.1 M HCl); however, this process was not effective in removing Pb from the metal sulfide. Treatment with more concentrated acidic media (e.g. ≥1 M HCl) may result in the decomposition of the material. Thus, p-UCR-20 is not reusable, as happens with almost all known MSIES.20 This disadvantage, however, is compensated by the high sorption capacity of the material (>50% heavy metal by weight). In addition, the strong binding of heavy metal ion into the UCR-20 framework and the no detectable Pb2+ leaching upon treatment of the loaded material with either distilled water or groundwater simulant solution indicate that the Pb2+-laden material could be considered as solid waste safe for disposal. In fact, not always regeneration of sorbents is desirable, as this results in large amounts of secondary waste. In contrast, solid waste occupies much less space and can be safely handled and stored if it shows negligible leaching of toxic species.
Luminescence sensing properties
The excellent sorption properties of p-UCR-20 towards Pb2+ ions coupled with its documented photoemission properties30 prompted us to test the material as a luminescent sensor for Pb2+ ions. At this point, we should mention that as synthesized p-UCR-20 could not form a stable suspension in water, which is a requirement for luminescence titration investigations. After testing various ionic surfactants and hydrophilic organic polymers, we have found that CTAB produces sufficiently stable p-UCR-20 aqueous suspensions for our fluorescence titration investigations. In agreement with the results originally reported by Feng et al.,30 irradiation of an aqueous suspension of p-UCR-20 at 380 nm (see ESI† for experimental details) with a laser beam gives rise to a broad emission signal with maximum at ca. 470 nm. Time-resolved emission measurements revealed that the emission of p-UCR-20 shows a single exponential decay with a time constant of 4.1 ± 0.3 ns which falls well within the fluorescence range.
Titration of a stirred suspension of p-UCR-20 with aliquots of a 1.0 ppm standard solution of Pb2+ ions in distilled water, results in the gradual quenching of the material's emission (Fig. 8). We observe stabilization of the emission signal at Pb2+ concentrations above ca. 25 ppb with the final emission intensity being about 60% of the initial emission signal. The plot of signal intensity versus Pb2+ concentration shows excellent linearity up to ca. 20 ppb (R2 = 0.980) with a slope of S = −0.0171 ± 0.0006 ppb−1 which, combined with the standard deviation of SD = 0.012 for the measurement of the luminescence signal, leads to limits of detection and quantification of 2.1 and 7.0 ppb respectively (Fig. S5 and Table S3†). These values illustrate the extremely high sensitivity of p-UCR-20 towards Pb2+ as both the LOD and LOQ values are below the EEA and US EPA acceptable levels of Pb2+ in water (10 and 15 ppb respectively).8
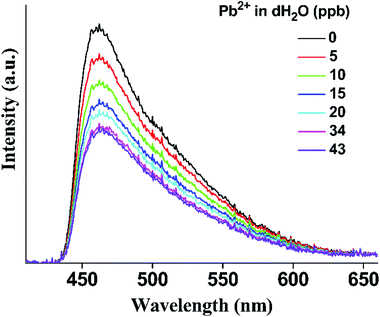 |
| Fig. 8 The fluorescence titration (λexc = 380 nm) of a stirred suspension of p-UCR-20 in water (0.1 mg mL−1) upon the addition of aliquots from a Pb2+ standard stock solution (concentration = 1000 ppb) in distilled water. | |
To further assess the sensing performance of p-UCR-20 towards Pb2+ in samples which contain relatively large concentrations of other, potentially inhibiting, metal ions we performed titration experiments with potable water (containing 78.7 ppm Ca2+, 3.45 ppm Mg2+, 3.3 ppm Na+, 1.04 ppm K+) spiked with 1 ppm of Pb2+. The results, shown in Fig. 9 indicate that the emission of p-UCR-20 gradually quenches with a stabilization at ca. 60% of the intensity of the original signal albeit with a considerably less steep slope compared to the case of the Pb2+ standard solution in distilled water. Nevertheless, the LOD and LOQ values being 6.4 and 21.2 ppb respectively are still comparable to the EU and USA acceptable levels of Pb2+ in water (vide supra) (Fig. S5†).
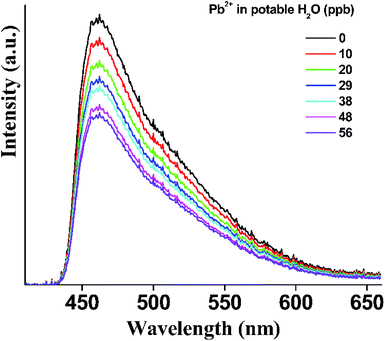 |
| Fig. 9 The fluorescence titration (λexc = 380 nm) of a stirred suspension of p-UCR-20 in water (0.1 mg mL−1) upon the addition of aliquots from a Pb2+ standard stock solution (concentration = 1000 ppb) in potable water. | |
An analysis of the p-UCR-20 emission quenching data in terms of the Stern–Volmer equation (eqn (4)) shows excellent linearity up to a Pb2+ concentration of ca. 0.12 μM in both the cases of distilled and potable water (R2 > 0.98) with Stern–Volmer constants, KSV, values of 5.3 × 106 and 2.0 × 106 M−1 respectively (Fig. S6†).
| 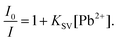 | (4) |
Additionally, we observed that the luminescence lifetime of p-UCR-20 remains unchanged at all Pb2+ concentrations. These observations point towards one dominating quenching mechanism which, most possibly involves the entry of Pb2+ within the framework of p-UCR-20, which in turn causes static quenching of the material's emission possibly because the guest ions act as non-radiative exciton recombination centres.44 This is in good agreement with the sorption data (vide supra) which demonstrated that Pb2+ ions enter the material's framework replacing the weakly bound protons most possibly driven by the favorable S2−⋯Pb2+ interactions. The markedly lower KSV in the case of potable water suggests that the presence of hard metal ions in much larger concentrations can have an inhibitory effect on the interaction between p-UCR-20 and Pb2+ which however remains strong.
Analogous sensing experiments were performed with Cd2+ and Ni2+ (Fig. S7–10†) which also show quenching of the emission of p-UCR-20 albeit to a smaller extent. The results, in comparison to those of Pb2+, are summarized in Table S3.†
It is worth emphasizing that with this study we demonstrate for the first time that a luminescent MSIE material can be attractive as a sensor as it combines the ability to selectively and efficiently sorb metal ions within its framework with a signal generating property.
Conclusions
In summary, we have isolated p-UCR-20, a proton-containing MSIE with a 3-D open framework structure. This material was proved to be a first-rate Pb2+ sorbent, with extremely rapid sorption kinetics (equilibrium time ≤1 min) and excellent maximum sorption capacity (527 mg g−1), comparable to the sorption capacities of the leading Pb2+ sorbent materials. Furthermore, p-UCR-20 in its composite form with calcium alginate was utilized, in a mixture with sand, as stationary phase in an ion exchange column, which was found highly efficient for the removal of traces of Pb2+ from a large volume of wastewater simulant solution.
In addition to the sorption study, photophysical investigations set out in depth revealed that p-UCR-20 represents also a very promising luminescent sensor, a property first time demonstrated for MSIEs. The material was able to detect Pb2+ cations at concentrations quite lower than its safe upper limit in water, even in the presence of several competitive species in large excess. We should also note that p-UCR-20 was effective to detect additional heavy metal cations, such as Ni2+ and Cd2+. Overall, the results presented here, indicate the high efficiency of MSIES not only for sorption but also for luminescence sensing of heavy metal ions, thus expanding the potential applications of these materials in environmental monitoring besides wastewater treatment.
Author contributions
A. D. Pournara: investigation, formal analysis, writing-original draft, C-G. Bika: investigation, formal analysis, X. Chen: investigation, formal analysis, T. Lazarides: investigation, formal analysis, writing-review &editing, S. Kaziannis: investigation, formal analysis, writing-review & editing, Pingyun Feng: resources, formal analysis, writing-review & editing, M. J. Manos: conceptualization, supervision, resources, writing-original draft.
Conflicts of interest
There are no conflicts to declare.
Acknowledgements
We thank the Central Laser Facility at the University of Ioannina.
References
- R. Singh, N. Gautam, A. Mishra and R. Gupta, Heavy metals and living systems: An overview, Indian J. Pharmacol., 2011, 43, 246 CrossRef CAS.
- Z. Lin, Y. Hu, Y. Yuan, B. Hu and B. Wang, Comparative analysis of kinetics and mechanisms for Pb(II) sorption onto three kinds of microplastics, Ecotoxicol. Environ. Saf., 2021, 208, 111451 CrossRef CAS.
- B. Hu, H. Wang, R. Liu and M. Qiu, Highly efficient U (VI) capture by amidoxime/carbon nitride composites, Chemosphere, 2021, 274, 129743 CrossRef CAS.
- S. Li, L. Dong, Z. Wei, G. Sheng, K. Du and B. Hu, Adsorption and mechanistic study of the invasive plant-derived biochar functionalized with CaAl-LDH for Eu(III) in water, J. Environ. Sci., 2020, 96, 127–137 CrossRef.
- S. Dai, N. Wang, C. Qi, X. Wang, Y. Ma, L. Yang, X. Liu, Q. Huang, C. Nie, B. Hu and X. Wang, Preparation of core-shell structure Fe3O4@C@MnO2 nanoparticles for efficient elimination of U (VI) and Eu(III) ions, Sci. Total Environ., 2019, 685, 986–996 CrossRef CAS.
- H. Sone, B. Fugetsu and S. Tanaka, Selective elimination of lead(II) ions by alginate/polyurethane composite foams, J. Hazard. Mater., 2009, 162, 423–429 CrossRef CAS.
- L. Järup, Hazards of heavy metal contamination, Br. Med. Bull., 2003, 68, 167–182 CrossRef PubMed.
- E.PA. US, National primary drinking water regulations: Long Term 1 Enhanced Surface Water Treatment Rule. Final rule, Fed. Regist., 2002, 67, 1811–1844 Search PubMed.
- J. Goel, K. Kadirvelu, C. Rajagopal and V. K. Garg, Removal of lead(II) by adsorption using treated granular activated carbon: Batch and column studies, J. Hazard. Mater., 2005, 125, 211–220 CrossRef CAS.
- X. Liu, H. Pang, X. Liu, Q. Li, N. Zhang, L. Mao, M. Qiu, B. Hu, H. Yang and X. Wang, Orderly porous covalent organic frameworks-based materials: superior adsorbents for pollutants removal from aqueous solutions, The Innovation, 2021, 2, 100076 CrossRef.
- A. Lalmi, K.-E. Bouhidel, B. Sahraoui and C. El H. Anfif, Removal of lead from polluted waters using ion exchange resin with Ca(NO3)2 for elution, Hydrometallurgy, 2018, 178, 287–293 CrossRef CAS.
- X. Cai, J. Li, Z. Zhang, F. Yang, R. Dong and L. Chen, Novel Pb2+ Ion Imprinted Polymers Based on Ionic Interaction via Synergy of Dual Functional Monomers for Selective Solid-Phase Extraction of Pb2+ in Water Samples, ACS Appl. Mater. Interfaces, 2014, 6, 305–313 CrossRef CAS.
- L. Mi, H. Hou, Z. Song, H. Han and Y. Fan, Polymeric Zinc Ferrocenyl Sulfonate as a Molecular Aspirator for the Removal of Toxic Metal Ions, Chem. – Eur. J., 2008, 14, 1814–1821 CrossRef CAS PubMed.
- F. Fu, L. Xie, B. Tang, Q. Wang and S. Jiang, Application of a novel strategy—Advanced Fenton-chemical precipitation to the treatment of strong stability chelated heavy metal containing wastewater, Chem. Eng. J., 2012, 189–190, 283–287 CrossRef CAS.
- M. M. Matlock, B. S. Howerton and D. A. Atwood, Chemical Precipitation of Lead from Lead Battery Recycling Plant Wastewater, Ind. Eng. Chem. Res., 2002, 41, 1579–1582 CrossRef CAS.
- M. Soylak, Y. E. Unsal, N. Kizil and A. Aydin, Utilization of membrane filtration for preconcentration and determination of Cu(II) and Pb(II) in food, water and geological samples by atomic absorption spectrometry, Food Chem. Toxicol., 2010, 48, 517–521 CrossRef CAS.
- A. R. Karbassi and S. Nadjafpour, Flocculation of dissolved Pb, Cu, Zn and Mn during estuarine mixing of river water with the Caspian Sea, Environ. Pollut., 1996, 93, 257–260 CrossRef CAS.
- M. J. Manos and M. G. Kanatzidis, Sequestration of heavy metals from water with layered metal sulfides, Chem. – Eur. J., 2009, 15, 4779–4784 CrossRef CAS PubMed.
- J.-R. Li, X. Wang, B. Yuan, M.-L. Fu and H.-J. Cui, Robust removal of heavy metals from water by intercalation chalcogenide [CH3NH3]2xMnx Sn3−x S6·0.5H2O, Appl. Surf. Sci., 2014, 320, 112–119 CrossRef CAS.
- M. J. Manos and M. G. Kanatzidis, Metal sulfide ion exchangers: Superior sorbents for the capture of toxic and nuclear waste-related metal ions, Chem. Sci., 2016, 7, 4804–4824 RSC.
-
R. Rigler, M. Orrit and T. Basché, Single molecule spectroscopy nobel conference lectures: Preface, Springer Series in Chemical Physics, 2001, vol. 67 Search PubMed.
- B. Kuswandi, Nuriman, J. Huskens and W. Verboom, Optical sensing systems for microfluidic devices: A review, Anal. Chim. Acta, 2007, 601, 141–155 CrossRef CAS.
- N. M. M. Pires, T. Dong, U. Hanke and N. Hoivik, Recent developments in optical detection technologies in lab-on-a-chip devices for biosensing applications, Sensors, 2008, 14, 15458–15479 CrossRef PubMed.
- A. P. De Silva, Luminescent photoinduced electron transfer (PET) molecules for sensing and logic operations, J. Phys. Chem. Lett., 2011, 2, 2865–2871 CrossRef CAS.
- J. K. Tusa and H. He, Critical care analyzer with fluorescent optical chemosensors for blood analytes, J. Mater. Chem., 2005, 15, 2640–2647 RSC.
- T. M. Swager, Iptycenes in the design of high performance polymers, Acc. Chem. Res., 2008, 41, 1181–1189 CrossRef CAS PubMed.
- M. D. Allendorf, C. A. Bauer, R. K. Bhakta and R. J. T. Houk, Luminescent metal-organic frameworks, Chem. Soc. Rev., 2009, 38, 1330–1352 RSC.
- L. Shi, N. Li, D. Wang, M. Fan, S. Zhang and Z. Gong, Environmental pollution analysis based on the luminescent metal organic frameworks: A review, TrAC, Trends Anal. Chem., 2021, 134, 116131 CrossRef CAS.
- S. A. Diamantis, A. Margariti, A. D. Pournara, G. S. Papaefstathiou, M. J. Manos and T. Lazarides, Luminescent metal-organic frameworks as chemical sensors: Common pitfalls and proposed best practices, Inorg. Chem. Front., 2018, 5, 1493–1511 RSC.
- N. Zheng, X. Bu, B. Wang and P. Feng, Microporous and photoluminescent chalcogenide zeolite analogs, Science, 2002, 298, 2366–2369 CrossRef CAS PubMed.
- H. Yang, M. Luo, L. Luo, H. Wang, D. Hu, J. Lin, X. Wang, Y. Wang, S. Wang, X. Bu, P. Feng and T. Wu, Highly Selective and Rapid Uptake of Radionuclide Cesium Based on Robust Zeolitic Chalcogenide via Stepwise Ion-Exchange Strategy, Chem. Mater., 2016, 28, 8774–8780 CrossRef CAS.
- F. Pinakidou, E. Kaprara, M. Katsikini, E. C. Paloura, K. Simeonidis and M. Mitrakas, Sn(II) oxy-hydroxides as potential adsorbents for Cr(VI)-uptake from drinking water: An X-ray absorption study, Sci. Total Environ., 2016, 551–552, 246–253 CrossRef CAS PubMed.
- M. J. Manos, N. Ding and M. G. Kanatzidis, Layered metal sulfides: Exceptionally selective agents for radioactive strontium removal, Proc. Natl. Acad. Sci. U. S. A., 2008, 105, 3696–3699 CrossRef CAS.
- G. P. Jeppu and T. P. Clement, A modified Langmuir-Freundlich isotherm model for simulating pH-dependent adsorption effects, J. Contam. Hydrol., 2012, 129–130, 46–53 CrossRef CAS.
- X.-H. Qi, K.-Z. Du, M.-L. Feng, J.-R. Li, C.-F. Du, B. Zhang and X.-Y. Huang, A two-dimensionally microporous thiostannate with superior Cs+ and Sr2+ ion-exchange property, J. Mater. Chem. A, 2015, 3, 5665–5673 RSC.
- Y.-J. Gao, H.-Y. Sun, J.-L. Li, X.-H. Qi, K.-Z. Du, Y.-Y. Liao, X.-Y. Huang, M.-L. Feng and M. G. Kanatzidis, Selective Capture of Ba2+, Ni2+, and Co2+ by a Robust Layered Metal Sulfide, Chem. Mater., 2020, 32, 1957–1963 CrossRef.
-
M. G. Kanatzidis, D. Sarma and M. Manos, Column material for the capture of heavy metal and precious metal ions, U.S. Patent No10,549,270, 2020 Search PubMed.
- S. Rapti, A. Pournara, D. Sarma, I. T. Papadas, G. S. Armatas, A. C. Tsipis, T. Lazarides, M. G. Kanatzidis and M. J. Manos, Selective capture of hexavalent chromium from an anion-exchange column of metal organic resin–alginic acid composite, Chem. Sci., 2016, 7, 2427–2436 RSC.
- S. Rapti, A. Pournara, D. Sarma, I. T. Papadas, G. S. Armatas, Y. S. Hassan, M. H. Alkordi, M. G. Kanatzidis and M. J. Manos, Rapid, green and inexpensive synthesis of high quality UiO-66 amino-functionalized materials with exceptional capability for removal of hexavalent chromium from industrial waste, Inorg. Chem. Front., 2016, 3, 635–644 RSC.
- A. D. Pournara, A. Margariti, G. D. Tarlas, A. Kourtelaris, V. Petkov, C. Kokkinos, A. Economou, G. S. Papaefstathiou and M. J. Manos, A Ca2+ MOF combining highly efficient sorption and capability for voltammetric determination of heavy metal ions in aqueous media, J. Mater. Chem. A, 2019, 7, 15432–15443 RSC.
- N. Wang, X.-K. Ouyang, L.-Y. Yang and A. M. Omer, Fabrication of a Magnetic Cellulose Nanocrystal/Metal–Organic Framework Composite for Removal of Pb(II) from Water, ACS Sustainable Chem. Eng., 2017, 5, 10447–10458 CrossRef CAS.
- C. Yu, X. Han, Z. Shao, L. Liu and H. Hou, High Efficiency and Fast Removal of Trace Pb(II) from Aqueous Solution by Carbomethoxy-Functionalized Metal–Organic Framework, Cryst. Growth Des., 2018, 18, 1474–1482 CrossRef CAS.
- L. Ma, Q. Wang, S. M. Islam, Y. Liu, S. Ma and M. G. Kanatzidis, Highly Selective and Efficient Removal of Heavy Metals by Layered Double Hydroxide Intercalated with the MoS42− Ion, J. Am. Chem. Soc., 2016, 138, 2858–2866 CrossRef CAS PubMed.
- C. Xue, X. Fan, J. Zhang, D. Hu, X.-L. Wang, X. Wang, R. Zhou, H. Lin, Y. Li, D.-S. Li, X. Wei, D. Zheng, Y. Yang, K. Han and T. Wu, Direct observation of charge transfer between molecular heterojunctions based on inorganic semiconductor clusters, Chem. Sci., 2020, 11, 4085–4096 RSC.
Footnote |
† Electronic supplementary information (ESI) available: Details of fluorescence experiments, determination of LODs, Stern–Volmer plots, EDS-elemental mapping. See DOI: 10.1039/d1qi00666e |
|
This journal is © the Partner Organisations 2021 |