DOI:
10.1039/D0QI00780C
(Research Article)
Inorg. Chem. Front., 2020,
7, 3379-3385
A water-stable terbium metal–organic framework as a highly sensitive fluorescent sensor for nitrite†
Received
30th June 2020
, Accepted 17th July 2020
First published on 21st July 2020
Abstract
A novel water-stable Tb-based metal–organic framework (Tb-MOF), namely {[Tb(CA)(OA)0.5(H2O)2]·H2O}n (H2CA = chelidonic acid; H2OA = oxalic acid), was synthesized via a solvothermal method. Tb-MOF was studied for its highly sensitive detection towards nitrite, which is a hazardous chemical in water and food. As a luminescent sensor, Tb-MOF displays a linear detection range between 0 and 15.6 μM, with a limit of detection of 28.25 nM. Dynamic quenching process was observed in this system and was studied in detail. A mechanism study revealed that the fluorescence response of Tb-MOF towards nitrite was due to the energy transfer from the sensitized Tb3+ ion to the nitrite.
1. Introduction
Recently, metal–organic frameworks (MOFs) have evoked intensive interests owing to their intrinsic features such as varied structures, permanent porosity and adjustable functionality.1–3 Inclusive applications in gas adsorption and separation,4,5 catalysis,6,7 sensors,8–11 magnetic materials,12 drug release13,14 and energy-related areas15–17 have been explored. For the MOFs with sensing-based applications,18–20 lanthanide MOFs (Ln-MOFs) have displayed excellent luminescence properties that can be taken into consideration.21,22 Through the reasonable adjustment of the host–guest interactions, Ln-MOFs have been applied in the chemical sensing of ions,23–25 organic small molecules,26,27 volatile organic compounds (VOCs)28 and gases,29–31 nitro explosives,32–34 biomolecules35,36 and so on.
Nitrite (NO2−) ion is widely used in pickled products but it has a great potential threat to human health because of the generation of carcinogenic N-nitrosamines in vivo from the ingested nitrite.37 Medical issues such as oesophageal cancer and birth defects have been reported due to the excessive consumption of nitrite ions.38 According to the World Health Organization guidelines, the maximum contaminant level (MCL) of nitrite ions in drinking water is 3 mg L−1 (65 μM).39 Traditional nitrite ion detection was performed using toxic naphthyl ethylenediamine via colorimetry assay.40 To avoid the usage of toxic materials, other methods including chromatography,41 electrochemical techniques42 and fluorescent technology43–45 have been developed. However, these methods need complicated instruments, tedious detection procedures, and are time-consuming. Among these methods, the fluorescence sensing technology include the advantages of rapid response, low cost and easy-operation.46
In this study, based on the fluorescence sensing mechanism and our experience on MOF-based sensing materials,47–56 a new water-stable Tb-based MOF (Tb-MOF), {[Tb(CA)(OA)0.5(H2O)2]·H2O}n (H2CA = chelidonic acid; H2OA = oxalic acid) was synthesized for highly sensitive nitrite detection. An isomorphic Gd-MOF was also synthesized for the mechanism studies. The dynamic quenching mechanism of Tb-MOF with nitrite provides a large quenching rate constant (kq) of 1.16 × 109 M−1 s−1. Both the high kq and the low limit of detection (LOD) of 28.25 nM indicate a high sensitivity of Tb-MOF towards nitrite ion.
2. Experimental
2.1 Materials and methods
All purchased chemicals were of reagent grade and were used directly. Fourier transform infrared (FT-IR) spectra were carried out on a Bruker ALPHA spectrophotometer. Elemental analyses (EA) for C and H were executed on a PerkinElmer 240 CHN elemental analyzer. Ultraviolet visible (UV–vis) spectra were acquired using a Shimadzu UV-2600 spectrophotometer. Powder X-ray diffraction (PXRD) patterns were recorded on a Rigaku Smartlab SE X-ray diffractometer. Thermogravimetric analysis (TGA) was performed on a Labsys NETZSCH TG209 Setaram apparatus in nitrogen atmosphere (at the heating rate of 10 °C min−1). Luminescence-related spectra were recorded on a FS-5 fluorescence spectrophotometer.
2.2 Synthesis
The synthesis of {[Tb(CA)(OA)0.5(H2O)2]·H2O}n: Terbium nitrate hexahydrate (0.0453 g, 0.1 mmol), chelidonic acid (0.0272 g, 0.15 mmol) and CH3CN/H2O (v
:
v = 3
:
1; 8 mL in total) were successively placed in a 25 mL Teflon-lined stainless steel reactor. The reactor was heated at 95 °C for three days with an additional one day cooling to 30 °C. Light yellow crystals were selected and washed with water and acetonitrile with 28.9% yield based on terbium nitrate hexahydrate. EA calculated: C, 21.01%; H, 2.19%. Found: C, 21.42%; H, 2.12%. IR data (cm−1): 3603 (s), 3175 (br), 1717 (s), 1618 (d), 1363 (t), 1212 (s), 1122 (s), 925 (d), 792 (s), 716 (s), 537 (m), 442(s).
Gd-MOF was obtained via the same method used for Tb-MOF by replacing terbium nitrate with gadolinium nitrate.
2.3 X-ray crystallography
Using an Agilent Technologies SuperNova single-crystal diffractometer equipped with graphite-monochromatic Mo-Kα radiation (λ = 0.71073 Å), the crystal data of Tb-MOF was collected at 120 K. The structure was solved by SHELXS (direct methods) and refined by SHELXL (full matrix least-squares techniques) in the Olex2 package.57,58 The parameters, data collection, and refinements of the single crystal are summarized in Table S1.† CCDC 2011050† contains the details of the crystal structure.
2.4 Luminescence measurements
Tb-MOF (30 mg) was finely ground. This powder was then scattered in distilled water (100 mL). An aqueous suspension of Tb-MOF was obtained after ultrasonicating it for 10 min. After the suspension was stabilized, luminescence tests were performed using 3 mL of supernatant. The luminescence spectra of Tb-MOF suspension were measured in situ with freshly prepared 0.4 mM analyte solutions.
3. Results and discussion
3.1 Structural description and characterizations
Crystallized in the P
space group, the asymmetric unit of Tb-MOF contains one Tb3+ ion, two coordinated water molecules, one solvent water molecule, one CA2− ligand and a half OA2− ligand. Eight oxygen atoms coordinate to the Tb3+ ion, completing a triangular dodecahedron configuration (Fig. 1a and b). Four oxygen atoms are from two CA2−, including three carboxylate oxygen atoms (Tb–O bond lengths ranging from 2.304(10) Å to 2.428(10) Å) and a carbonyl oxygen atom (the bond length of Tb–O is 2.366(9) Å). Two oxygen atoms are from two coordinated water molecules (Tb–O bond lengths of 2.380(10) Å and 2.407(10) Å). Rest of the two oxygen atoms are from the carboxylate oxygen atoms of the oxalates derived from the partial decomposition of H2CA (Tb–O bond lengths of 2.399(9) Å and 2.402(9) Å). Tb3+ ions are connected by CA2− ions in chains (Fig. 1c) and these chains are connected into a layer by CA2− (Fig. 1d). The layers are further connected by OA2− into a three-dimensional framework (Fig. 1e). Taking OA2−, CA2− and Tb3+ ions as two, four and five-connected nodes, respectively, the framework is simplified as a (2,4,5)-connected trinodal net with a short (Schläfli) vertex symbol of {44·62·84}2{44·62}2{8} (Fig. S1†).
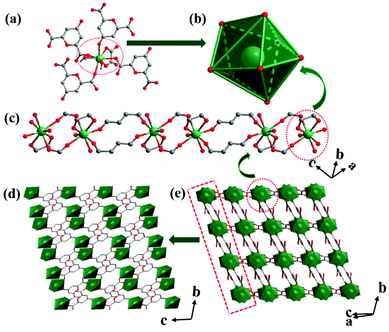 |
| Fig. 1 Coordination environment (a) and coordination geometry (b) of Tb3+ ion; (c) the connection mode of TbO8; (d) 2D layer of Tb-MOF along the a axis; (e) 3D framework of Tb-MOF. Atom codes: Tb (green), O (red), C (gray). Hydrogen atoms are removed for clarity. | |
PXRD showed that the diffraction peaks of the as-synthesized Tb-MOF are accorded with the calculated ones from the crystal data (Fig. S2†). This result confirms the high phase purity of the as-synthesized Tb-MOF. In addition, the diffraction peaks of Tb-MOF after immersion in water for 36 h agree well with that of the primitive data. The results also indicate the high water-stability of Tb-MOF, which benefits from the suitable coordination models and rigid framework of Tb-MOF. The TGA of Tb-MOF displays a weight loss of 11.96% below 195 °C, which corresponds to the release of three water molecules (Fig. S3,† calc. 11.81%). Further heating causes a dramatic weight loss, indicating the disintegration of Tb-MOF.
3.2 Luminescence study and detection of nitrite
The luminescence spectrum is shown in Fig. 2. The excitation spectrum was recorded at a wavelength of 544 nm, which exhibited a broad band with a peak at 295 nm. Excited by 295 nm ultraviolet light, Tb-MOF displayed four well-resolved characteristic emissions at 489, 545, 585 and 621 nm, corresponding to the 5D4 → 7FJ (J = 6, 5, 4, and 3) transitions of Tb3+ ions, respectively. The schematic energy transfer process within Tb-MOF is shown in Fig. S4.† The singlet and triplet levels of the ligands were calculated through the UV-Vis spectrum (Fig. S5a†) and phosphorescence spectrum of the isomorphic Gd-MOF at 77 K (Fig. S5b†). The singlet level of H2OA is observed at 39
582 cm−1 (252 nm), in accordance with our previous study,52 while the singlet level of H2CA is at 33
630 cm−1 (273 nm). The triplet levels of OA2− and CA2− are 24
390 cm−1 (410 nm) and 22
831 cm−1 (438 nm), respectively. The energy gaps (ΔE) between the singlet-state and triplet-state energy levels of the ligands are 12
647 cm−1 and 10
799 cm−1, respectively. The values of ΔE are much higher than 5000 cm−1, indicating effective intersystem crossing processes using the Reinhold's empirical rule.59,60 Hence, the gaps of the triplet level of the ligands and the 5D4 energy level of the Tb3+ ion (20
500 cm−1) are 3890 cm−1 (H2OA) and 2331 cm−1 (H2CA) in the range of the most capable values (2500–4500 cm−1),61 which indicates an effective energy transfer process from CA2−/OA2− to Tb3+ ion, giving rise to a bright green emission when exposed to 254 nm UV light (Fig. 2, inset).
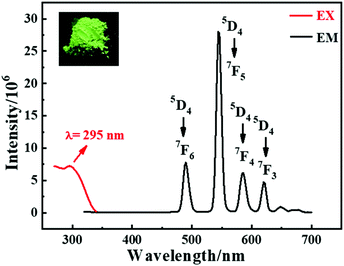 |
| Fig. 2 The luminescence spectra of Tb-MOF. The inset picture is Tb-MOF excited under a 254 nm ultraviolet lamp. | |
For studying the selectivity of Tb-MOF in water, the quenching effects of different salts were studied. The luminescence intensities of Tb-MOF with other salts have almost no change except that with the nitrite salt (Fig. 3a). A certain degree of fluorescence quenching of Tb-MOF occurs in the presence of Fe3+, Cu2+ and PO43−. However, when nitrite was added, a clear quenching effect occurred, indicating selectivity of Tb-MOF towards nitrite ions. The quenching efficiencies were calculated by (I0 − I)/I0, as shown in Fig. 3b, where I0 and I are intensities at 544 nm of Tb-MOF dispersions without or with the addition of different salts, respectively. In particular, nitrite ions exhibited a quenching efficiency of 82.3%. Compared with Fe3+, Cu2+ and PO43−, the much higher quenching efficiency of NaNO2 indicated the excellent selectivity of Tb-MOF towards nitrite ions. The results indicated that Tb-MOF can be used for the specific detection of nitrite.
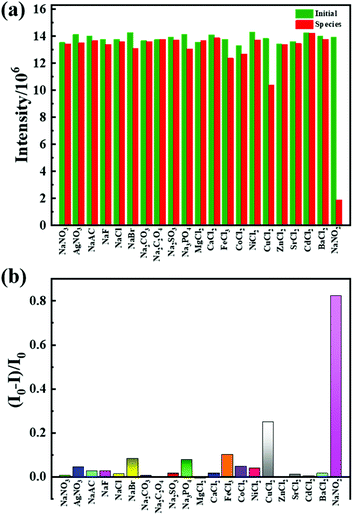 |
| Fig. 3 (a) Fluorescence intensities of Tb-MOF without or with different species. (b) the quenching efficiency of different salts. | |
To improve the accuracy for the detection of nitrite ions, three different fluorescence titration experiments were performed by adding different volumes of nitrite ions (3 μL, 5 μL, and 10 μL). Considering the first one for example, the luminescence intensities of Tb-MOF with successive additions of 3 μL nitrite are shown in Fig. S6.† The fluorescence quenching result of Tb-MOF is quantitatively mustered using the Stern–Volmer (S–V) eqn (1):
where
KSV is the S–V quenching constant (M
−1), [
C] is the nitrite ion concentration, and
I0 and
I are fluorescence intensities without and with adding nitrite ions in dispersions, respectively. In Fig. S7,
† the linear equation is well fitted with
I0/
I − 1 = 0.474[
C] + 0.016 in the range of 0–6 μM with a
R2 of 0.999. The
KSV value of Tb-MOF is 4.74 × 10
5 M
−1. The
KSV value is higher than that of most MOF-based ion sensing materials (usually 10
3–10
4 M
−1), indicating a high sensitivity of Tb-MOF towards nitrite.
23–25 The results of the three sets of fluorescence titrations (Fig. S6–S11
†) were combined in
Fig. 4. The linear correlation was further modified as
I0/
I − 1 = 0.482[
C] + 0.002 with a
R2 of 0.999 in the range of 0–15.6 μM, with the
KSV value of 4.82 × 10
5 M
−1.
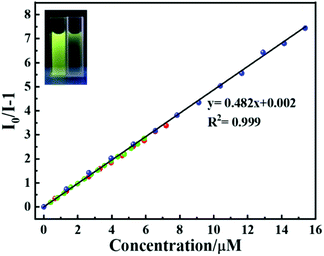 |
| Fig. 4 Summary of S–V plots at 544 nm of Tb-MOF towards nitrite ions with different volumes, 3 μL (green plots), 5 μL (red plots), 10 μL (blue plots). Inset is the picture of Tb-MOF suspension excited by 254 nm ultraviolet light before and after adding 20 μL nitrite aqueous solution (4 mM). | |
The LOD was calculated using the 3σ IUPAC criteria by eqn (2) and (3):
|  | (2) |
where
Sd is the standard deviation of the replicate fluorescence intensities of Tb-MOF dispersions performed twenty times (Fig. S12
†).
Ii is the fluorescence intensity at 544 nm without nitrite, and

is the average of

. The slope value was calculated from
eqn (1) and the LOD was calculated to be 28.25 nM, which is far below the criterion of the World Health Organization for drinking water.
To investigate the practical application, Tb-MOF was employed to measure the nitrite concentration in tap water. The related results of spike-and-recovery experiences are shown in Table S2.† The recoveries were found to be 97.7–100.9%. Moreover, the anti-interference of Tb-MOF was also evaluated (Fig. S13†). With coexisting anions and cations, the degree of the quenched fluorescence of Tb-MOF stayed almost the same after the addition of nitrite, indicating that Tb-MOF displayed a considerable anti-interference ability. The recycling performance of Tb-MOF was also studied (Fig. 5). The suspensions with nitrite ions were centrifuged and washed with water and acetonitrile for several times after the sensing experiments. It was found that the luminescence intensity can be recycled at least 5 times. All these results indicate that Tb-MOF has potential applications in the nitrite ion detection in polluted water and food preservatives.
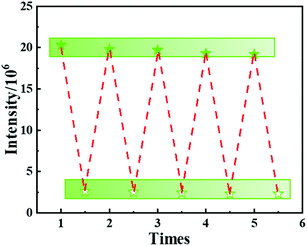 |
| Fig. 5 Reproducibility of Tb-MOF with five continuous quenching cycles. | |
3.3 Sensing mechanism
To the best of our knowledge, this sensing material provides the lowest LOD (28.25 nM) for the nitrite ion detection amongst the reported MOF-based fluorescence sensing materials (Table S3†). To figure out the quenching behaviour towards nitrite ions, the sensing mechanism was investigated. First, the PXRD of Tb-MOF immersed in 4 mM nitrite ions for 4 h showed similar results to that obtained with the calculated data (Fig. S14†), indicating the structural stability of Tb-MOF with nitrite ions. As shown in Fig. 4, the S–V plots show a linear relationship at the completed concentration range, which is different from that of the concentration controlled by a self-absorption process.51–55 The luminescence lifetimes of Tb-MOF were measured at 544 nm with different concentrations of the nitrite ions (Fig. S15 and Table S4†). The lifetimes decrease with the increase in the concentration of nitrite ions, and the lifetime-based S–V curve provides a same KSV of 4.82 × 105 M−1 (Fig. S16†) with that of the S–V curve, indicating a dynamic quenching progress. The quenching rate constant (kq) can be calculated using eqn (4):where KSV is lifetime-based S–V quenching constant (M−1), and τ0 is the luminescence lifetime (s−1) of Tb-MOF without nitrite ions. The kq value was 1.16 × 109 M−1 s−1, which is 10 times lower than that of the purely diffusion-controlled quenching process (about 1010 M−1 s−1)62 possibly due to the steric hindrance of Tb-MOF, limiting the number of collisional quenching occurring.23,63 The decrease in lifetimes indicates the presence of other relaxation pathway that can depopulate the excited state. To further understand the specific energy transfer between Tb-MOF and nitrite ions, the singlet and triplet energy levels of NaNO2 were obtained as 48
077 cm−1 (208 nm) and 19
084 cm−1 (524 nm) (Fig. S17 and S18†), which are similar to the results reported by Fujio.64,65 The triplet level of NaNO2 is slightly lower than the 5D4 energy level of the Tb3+ ion (20
500 cm−1) with a gap of 1416 cm−1, which is lower than 1850 cm−1. This result indicates that energy transfer between Tb-MOF and NaNO2 is possible.61,66 In addition, no obvious overlap was observed between the UV-Vis spectra of the ligands and NaNO2 (Fig. S5a and S17†), which excludes the possibility of the competitive absorption mechanism.
Based on the above analysis, the mechanism of the sensing function is shown in Fig. 6. Excited at 295 nm, the Tb3+ ion is sensitized by antenna effect and displays characteristic emissions; when nitrite ion was added, dynamic quenching behaviour between Tb-MOF and nitrite ion happened. In this process, energy transfer between Tb-MOF and nitrite ion occurred, leading to the decrease in the emission intensity.
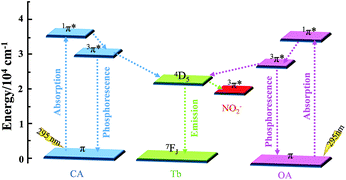 |
| Fig. 6 The schematic energy transfer process of Tb-MOF in the presence of nitrite. | |
4. Conclusions
In summary, a water-stable Tb-based MOF was synthesized as a sensitive and selective nitrite ion sensor. The sensing mechanism studies show that in the presence of nitrite ions, a part of the energy for the emission of Tb-MOF is transferred to nitrite ion, hence decreasing the emission intensity. To the best of our knowledge, the sensor provides the lowest LOD (28.25 nM) for the nitrite ion detection compared with the recently reported MOF-based fluorescence sensing materials for nitrite. The satisfactory recyclability, sensitivity, selectivity, and stability indicate a promising utilization of Tb-MOF for nitrite detection in water.
Conflicts of interest
There are no conflicts to declare.
Acknowledgements
This work was supported by the National Natural Science Foundation of China (grant numbers 21931004 and 21861130354), the Natural Science Foundation of Tianjin (18JCJQJC47200), the Fundamental Research Funds for the Central Universities, Nankai University (63201016 and 63201043) and the Ministry of Education of China (grant number B12015). W. S. acknowledges the receipt of a Newton Advanced Fellowship from Royal Society.
References
-
O. M. Yaghi, M. J. Kalmutzki and C. S. Diercks, Introduction to Reticular Chemistry, Metal-Organic Framework and Covalent Organic Frameworks, 2019, WILEY-VCH Search PubMed.
- G. Maurin, C. Serre, A. Cooper and G. Férey, The new age of MOFs and of their porous-related solids, Chem. Soc. Rev., 2017, 46, 3104 RSC.
- B. Li, H. Wen, Y. Cui, W. Zhou, G. Qian and B. Chen, Emerging Multifunctional Metal-Organic Framework Materials, Adv. Mater., 2016, 28, 8819 CrossRef CAS.
- D. Xue, Q. Wang and J. Bai, Amide-functionalized metal–organic frameworks: Syntheses, structures and improved gas storage and separation properties, Coord. Chem. Rev., 2017, 378, 2 CrossRef.
- M. S. Derry, Jr., J. C. Moreton, L. Benz and S. M. Cohen, Metal–organic frameworks for membrane-based separations, Nat. Rev. Mater., 2016, 1, 16078 CrossRef.
- R. Zhang, B. Wu, Q. Li, L. Lu, W. Shi and P. Cheng, Design strategies and mechanism studies of CO2 electroreduction catalyst based on coordination chemistry, Coord. Chem. Rev., 2020, 422 DOI:10.1016/j.ccr.2020.213436.
- L. Chen and Q. Xu, Metal-Organic Framework Composites for Catalysis, Matter, 2019, 1, 57 CrossRef.
- J. Liu, Z. Luo, Y. Pan, A. K. Singh, M. Trivedi and A. Kumar, Recent developments in luminescent coordination polymers: Designing strategies, sensing application and theoretical evidences, Coord. Chem. Rev., 2020, 406, 213145 CrossRef CAS.
- S.-Y. Zhang, Z.-Y. Wang, J. Gao, K. Wang, E. Gianolio, S. Aime, W. Shi, P. Cheng and M. J. Zaworotko, A Gadolinium(III) Zeolite-like Metal-Organic Framework-based Magnetic Resonance Thermometer, Chem, 2019, 5, 1609 CAS.
- Y. Cui, J. Zhang, H. He and G. Qian, Photonic functional metal-organic frameworks, Chem. Soc. Rev., 2018, 47, 5740 RSC.
- F. Yi, D. Chen, M. Wu, L. Han and H. Jiang, Chemical Sensors Based on Metal−Organic Frameworks, ChemPlusChem, 2016, 81, 675 CrossRef CAS.
- K. Liu, X. Zhang, X. Meng, W. Shi, P. Cheng and A. K. Powell, Constraining the coordination geometries of lanthanide centers and magnetic building blocks in frameworks: a new strategy for molecular nanomagnets, Chem. Soc. Rev., 2016, 45, 2423 RSC.
- W. Cai, J. Wang, C. Chu, W. Chen, C. Wu and G. Liu, Metal Organic Framework-Based Stimuli-Responsive Systems for Drug Delivery, Adv. Sci., 2019, 6, 1801526 CrossRef.
- M. Giménez-Marqués, T. Hidalgo, C. Serre and P. Horcajada, Nanostructured metal–organic frameworks and their bio-related applications, Coord. Chem. Rev., 2016, 307, 342 CrossRef.
- L. Jiao and H. Jiang, Metal-Organic-Framework-Based Single-Atom Catalysts for Energy Applications, Chem, 2019, 5, 786 CAS.
- J. W. Liu, D. X. Xie, W. Shi and P. Cheng, Coordination compounds in lithium storage and lithium-ion transport, Chem. Soc. Rev., 2020, 49, 1624 RSC.
- Y. Wang, Q. Li, W. Shi and P. Cheng, The application of metal-organic frameworks in electrocatalytic nitrogen reduction, Chin. Chem. Lett., 2020, 31, 1768 CrossRef CAS.
- W. P. Lustig and J. Li, Luminescent metal–organic frameworks and coordination polymers as alternative phosphors for energy efficient lighting devices, Coord. Chem. Rev., 2018, 373, 116 CrossRef CAS.
- J. Hao, X. Xu, H. Fei, L. Li and B. Yan, Functionalization of Metal–Organic Frameworks for Photoactive Materials, Adv. Mater., 2018, 30, 1705634 CrossRef.
- W. P. Lustig, S. Mukherjee, N. D. Rudd, A. V. Desai, J. Li and S. K. Ghosh, Metal–organic frameworks: functional luminescent and photonic materials for sensing applications, Chem. Soc. Rev., 2017, 46, 3242 RSC.
-
P. Cheng, Lanthanide Metal-Organic Frameworks, Springer, 2015 Search PubMed.
- S. Zhao, G. Wang, D. Poelman and P. V. D. Voort, Luminescent Lanthanide MOFs: A Unique Platform for Chemical Sensing, Materials, 2018, 11, 572 CrossRef.
- F. M. Ebrahim, T. N. Nguyen, S. Shyshkanov, A. Gładysiak, P. Favre, A. Zacharia, G. Itskos, P. J. Dyson and K. C. Stylianou, Selective, Fast-Response, and Regenerable Metal–Organic Framework for Sampling Excess Fluoride Levels in Drinking Water, J. Am. Chem. Soc., 2019, 141, 3052 CrossRef CAS.
- H. Chen, P. Fan, X. Tu, H. Min, X. Yu, X. Li, J. L. Zeng, S. Zhang and P. Cheng, A Bifunctional Luminescent Metal–Organic Framework for the Sensing of Paraquat and Fe3+ Ions in Water, Chem. – Asian J., 2019, 14, 3611 CrossRef CAS.
- Z. Yang, M. Wang, X. Wang and X. Yin, Boric-Acid-Functional Lanthanide Metal–Organic Frameworks for Selective Ratiometric Fluorescence Detection of Fluoride Ions, Anal. Chem., 2017, 89, 1930 CrossRef CAS.
- T. Song, K. Yuan, W. Qian, Y. Shi, J. Dong, H. Gao, X. Yang, J. Cui and B. Zhao, Water Stable [Tb4] Cluster-Based Metal–Organic Framework as Sensitive and Recyclable Luminescence Sensor of Quercetin, Anal. Chem., 2019, 91(4), 2595 CrossRef CAS.
- Y. Gao, G. Yu, K. Liu and B. Wang, Luminescent mixed-crystal Ln-MOF thin film for the recognition and detection of pharmaceuticals, Sens. Actuators, B, 2018, 257, 931 CrossRef CAS.
- H. Zhao, J. Ni, J. Zhang, S. Liu, Y. Sun, H. Zhou, Y. Li and C. Duan, A trichromatic MOF composite for multidimensional ratiometric luminescent sensing, Chem. Sci., 2018, 9, 2918 RSC.
- J. Peng, C. L. Teoh, X. Zeng, A. Samanta, L. Wang, W. Xu, D. Su, L. Yuan, X. Liu and Y. Chang, Development of a highly selective, sensitive, and fast response upconversion luminescent platform for hydrogen sulfide detection, Adv. Funct. Mater., 2016, 26, 191 CrossRef CAS.
- C. Wang, L. Li, J. G. Bell, X. Lv, S. Tang, X. Zhao and K. M. Thomas, Hysteretic gas and vapor sorption in flexible interpenetrated lanthanide-based metal-organic frameworks with coordinated molecular gating via reversible single-crystal-to-single-crystal transformation for enhanced selectivity, Chem. Mater., 2015, 27, 1502 CrossRef CAS.
- J. Hao and B. Yan, A dual-emitting 4d-4f nanocrystalline metal-organic framework as a self-calibrating luminescent sensor for indoor formaldehyde pollution, Nanoscale, 2016, 8, 12047 RSC.
- X. Z. Song, S. Y. Song, S. N. Zhao, Z. M. Hao, M. Zhu, X. Meng, L. L. Wu and H. J. Zhang, Single-crystal-to-single-crystal transformation of a europium(III) metal-organic framework producing a multi-responsive luminescent sensor, Adv. Funct. Mater., 2014, 24, 4034 CrossRef CAS.
- W. Xie, S. R. Zhang, D. Y. Du, J. S. Qin, S. J. Bao, J. Li, Z. M. Su, W. W. He, Q. Fu and Y. Q. Lan, Stable luminescent metal-organic frameworks as dual-functional materials to encapsulate Ln3+ ions for white-light emission and to detect nitroaromatic explosives, Inorg. Chem., 2015, 54, 3290 CrossRef CAS.
- B. Wang, X. L. Lv, D. W. Feng, L. H. Xie, J. Zhang, M. Li, Y. B. Xie, J. R. Li and H. C. Zhou, Highly stable Zr(IV)-based metal-organic frameworks for the detection and removal of antibiotics and organic explosives in water, J. Am. Chem. Soc., 2016, 138, 6204 CrossRef CAS.
- J. Hao and B. Yan, Determination of urinary 1-hydroxypyrene for biomonitoring of human exposure to polycyclic aromatic hydrocarbons carcinogens by a lanthanide-functionalized metal-organic framework sensor, Adv. Funct. Mater., 2017, 27, 1603856 CrossRef.
- T. Xia, Y. Wan, Y. Li and J. Zhang, Highly Stable Lanthanide Metal−Organic Framework as an Internal Calibrated Luminescent Sensor for Glutamic Acid, a Neuropathy Biomarker, Inorg. Chem., 2020, 59, 8809 CrossRef CAS.
- A. J. Cross, L. M. Ferrucci, A. Risch, B. I. Graubard, M. H. Ward, Y. Park, A. R. Hollenbeck, A. Schatzkin and R. Sinha, A Large Prospective Study of Meat Consumption and Colorectal Cancer Risk: An Investigation of Potential Mechanisms Underlying this Association, Cancer Res., 2010, 70, 2406 CrossRef CAS.
- L. B. Maia and J. J. G. Moura, How Biology Handles Nitrite, Chem. Rev., 2014, 114, 5273 CrossRef CAS.
-
World Health Organization, Guidelines for Drinking Water Quality, WHO Press, Geneva, Switzerland, 3rd edn, 2008 Search PubMed.
- T. L. Mako, A. M. Levenson and M. Levine, Ultrasensitive Detection of Nitrite through Implementation of N-(1-Naphthyl)ethylenediamine-Grafted Cellulose into a Paper-Based Device, ACS Sens., 2020, 5, 1207 CrossRef CAS.
- C. Lopez-Moreno, I. V. Perez and A. M. Urbano, Development and validation of an ionic chromatography method for the determination of nitrate, nitrite and chloride in meat, Food Chem., 2016, 194, 687 CrossRef CAS.
- H. Chen, T. Yang, F. Liu and W. Li, Electrodeposition of gold nanoparticles on Cu-based metal-organic framework for the electrochemical detection of nitrite, Sens. Actuators, B, 2019, 286, 401 CrossRef CAS.
- Y. Zhan, Y. Zeng, L. Li, F. Luo, B. Qiu, Z. Lin and L. Guo, Ratiometric Fluorescent Hydrogel Test Kit for On-Spot Visual Detection of Nitrite, ACS Sens., 2019, 4, 1252 CrossRef CAS.
- Su Zhu, L. Zhao and B. Yan, A novel spectroscopic probe for detecting food preservative NO2−: Citric acid functionalized metal-organic framework and luminescence sensing, Microchem. J., 2020, 155, 104768 CrossRef CAS.
- J. Wu and B. Yan, Luminescent Hybrid Tb3+ Functionalized Metal−Organic Frameworks Act as Food Preservative Sensor and Water Scavenger for NO2−, Ind. Eng. Chem. Res., 2018, 57, 7105 CrossRef CAS.
- Z. Hu, B. J. Deibert and J. Li, Luminescent metal–organic frameworks for chemical sensing and explosive detection, Chem. Soc. Rev., 2014, 43, 5815 RSC.
- S. Wu, H. Min, W. Shi and P. Cheng, Multicenter Metal-Organic Framework-Based Ratiometric Fluorescent Sensors, Adv. Mater., 2020, 32, 1805871 CrossRef CAS.
- Y. Lin, X. Zhang, W. Chen, W. Shi and P. Cheng, Three Cadmium Coordination Polymers with Carboxylate and Pyridine Mixed Ligands: Luminescent Sensors for FeIII and CrVI Ions in an Aqueous Medium, Inorg. Chem., 2017, 56, 11768 CrossRef CAS.
- J. Zhou, H. Li, H. Zhang, H. Li, W. Shi and P. Cheng, A bimetallic lanthanide metal-organic material as a selfcalibrating color-gradient luminescent sensor, Adv. Mater., 2015, 27, 7072 CrossRef CAS.
- Z. Han, K. Wang, Y. Guo, W. Chen, J. Zhang, X. Zhang, G. Siligardi, S. Yang, Z. Zhou, P. Sun, W. Shi and P. Cheng, Cation-induced chirality in a bifunctional metalorganic framework for quantitative enantioselective recognition, Nat. Commun., 2019, 10, 5117 CrossRef.
- S. Zhang, W. Shi, P. Cheng and M. J. Zaworotko, A Mixed-Crystal Lanthanide Zeolite-like Metal−Organic Framework as a Fluorescent Indicator for Lysophosphatidic Acid,
a Cancer Biomarker, J. Am. Chem. Soc., 2015, 137, 12203 CrossRef CAS.
- H. Ma, L. Wang, J. Chen, X. Zhang, L. Wang, N. Xu, G. Yang and P. Cheng, A multi-responsive luminescent sensor for organic small-molecule pollutants and metal ions based on a 4d–4f metal–organic framework, Dalton Trans., 2017, 46, 3526 RSC.
- H. Zhang, D. M. Chen, H. L. Ma and P. Cheng, Real-time detection of traces of benzaldehyde in benzyl alcohol as a solvent by a flexible lanthanide microporous metal-organic framework, Chem. – Eur. J., 2015, 21, 15854 CrossRef CAS.
- Q. Xu, Z. Chen, H. Min, F. Song, Y. Wang, W. Shi and P. Cheng, Water Stable Heterometallic Zn-Tb Coordination Polymer for Rapid Detection of the Ultraviolet Filter Benzophenone, Inorg. Chem., 2020, 59, 6729 CrossRef CAS.
- S. Wu, Y. Lin, J. Liu, W. Shi, G. Yang and P. Cheng, Rapid Detection of the Biomarkers for Carcinoid Tumors by a Water Stable Luminescent Lanthanide Metal-Organic Framework Sensor, Adv. Funct. Mater., 2018, 28, 1707169 CrossRef.
- L. Wang, G. Fan, X. Xu, D. Chen, L. Wang, W. Shi and P. Cheng, Detection of polychlorinated benzenes (persistent organic pollutants) by a luminescent sensor based on a lanthanide metal-organic framework, J. Mater. Chem. A, 2017, 5, 5541 RSC.
- G. M. Sheldrick, A short history of SHELX, Acta Crystallogr., Sect. A: Found. Crystallogr., 2008, 64, 112 CrossRef CAS.
- G. M. Sheldrick, Crystal structure refinement with SHELXL, Acta Crystallogr., Sect. C: Struct. Chem., 2015, 71, 3 Search PubMed.
- S. P. Cook and G. H. Dieke, 6P state of the Gd+++ ion, J. Chem. Phys., 1957, 27, 1213 CrossRef CAS.
- G. H. Dielre and L. Leopold, Absorption, fluorescence, and magnetic properties of gadolinium chloride (GdCl3·6H2O), J. Opt. Soc. Am., 1957, 47, 944 CrossRef.
- M. Latva, H. Takalo, V. M. Mukkala, C. Matachescu, J. C. Rodríguez-Ubis and J. Kankare, Correlation between the lowest triplet state energy level of the ligand and lanthanide(III) luminescence quantum yield, J. Lumin., 1997, 75, 149 CrossRef CAS.
- J. R. Lakowicz and G. Weber, Quenching of fluorescence by oxygen. Probe for structural fluctuations in macromolecules, Biochemistry, 1973, 12, 4161 CrossRef CAS.
- N. Chadborn, J. Bryant, A. J. Bain and P. O'Shea, Ligand-Dependent Conformational Equilibria of Serum Albumin Revealed by Tryptophan Fluorescence Quenching, Biophys. J., 1999, 76, 2198 CrossRef CAS.
- F. Kokal and T. Azuml, Excitation Energy Dependence of the Phosphorescence to Fluorescence Quantum Yield Ratio of NaNO2 Crystal. An Interplay between the Energy-Dependent Intersystem Crossing and Vibrational Relaxation, J. Phys. Chem., 1982, 86, 177 CrossRef.
- R. M. Hochstrasser and A. P. Marchetti, Electronic, Vibrational, and Zeeman Spectra of Triplet NO2−, J. Chem. Phys., 1969, 50, 1727 CrossRef CAS.
- S. Hinojosa, M. A. Meneses-Nava, O. Barbosa-Garcıa, L. A. Dıaz-Torres, M. A. Santoyo and J. F. Mosino, Energy back transfer, migration and energy transfer (Yb-to-Er and Er-to-Yb) processes in Yb, Er:YAG, J. Lumin., 2003, 102103, 694 CrossRef.
Footnote |
† Electronic supplementary information (ESI) available: Crystallographic data of Tb-MOF, PXRD, TGA and other figures and tables. CCDC 2011050. For ESI and crystallographic data in CIF or other electronic format see DOI: 10.1039/d0qi00780c |
|
This journal is © the Partner Organisations 2020 |