DOI:
10.1039/C9NA00108E
(Review Article)
Nanoscale Adv., 2019,
1, 1640-1671
Review of electromagnetic interference shielding materials fabricated by iron ingredients
Received
21st February 2019
, Accepted 1st April 2019
First published on 1st April 2019
Abstract
Iron (Fe) and its counterparts, such as Fe2O3, Fe3O4, carbonyl iron and FeO, have attracted the attention of researchers during the past few years due to their bio-compatibility, bio-degradability and diverse applications in the field of medicines, electronics and energy; including water treatment, catalysis and electromagnetic wave interference shielding etc. In this review paper, we aimed to explore iron based materials for the prevention of electromagnetic interference (EMI) by means of both reflection and absorption processes, including the standard methods of synthesis of Fe-based materials along with the determination of EMI performance. It is customary that a proper combination of two dielectric-losses, i.e. electrical and magnetic losses, give excellent microwave absorption properties. Therefore, we focused on the different strategies of preparation of these iron based composites with dielectric carbon materials, polymers etc. Additionally, we explained their positive and negative aspects.
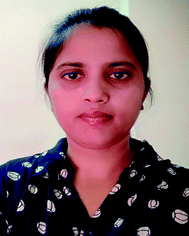 Vineeta Shukla | Vineeta Shukla was born in Uttar Pradesh, India, and received degrees from the University of C. S. J. M. Kanpur: Master of Philosophy in Physics (2012); Post-Graduation in Physics (2010); and Graduation in Physics and Mathematics (2007). She received two gold medals, the Sir C. V. Raman Commemoration Medal and the Kailashpat Singhania Medal for attaining the first position in the University. Currently, she is pursuing a PhD from the Indian Institute of Technology, Kharagpur, West Bengal, under the supervision of Dr Sanjeev Kumar Srivastava. Her research work includes the characterization and energy applications of carbon, polymer, metal and alloy materials. |
1 Introduction
1.1 Electromagnetic interference (EMI) pollution
In recent years, electromagnetic (EM) wave radiation in the gigahertz (GHz) range has been regarded as an alarming danger for commercial appliances, biological systems, high quality information technology and defense safety technologies, etc., because when these EM waves interfere with the input signal of the electronic devices, they create a noise that is known as electromagnetic interference (EMI) pollution. In general, EMI pollution could be considered as an undesirable outcome of modern engineering that has become grievous to human health, causing many diseases, e.g. headaches, sleeping disorders and trepidation. In communication devices (e.g. cell phones, computers, bluetooth devices, laptops), commercial appliances (i.e. microwave ovens, the design of microwave circuits) and the automotive industries (i.e. integrated electrical circuits), EMI pollution deteriorates the durability and proper functioning of electronic equipment. Therefore, this new kind of pollution has become a serious worldwide problem and its mitigation could be achieved only by use of EMI shielding materials.1 EMI shielding is defined in terms of the reflection and/or absorption of electromagnetic radiation by a material that acts as a barrier against the penetration of the radiation passing through the shielding materials. These materials prevent the transmission of EM radiation by reflection and/or absorption of the electromagnetic radiation or by suppressing the EM signals so that EM waves do not affect the functioning and durability of electronic equipment. In general, conductive materials like metals, owing to their high reflectivity, are widely used to isolate spaces or equipment from surrounding EM waves. This reflection shielding is based on the principle of the Faraday cage, in which inside the cage, space is completely impervious to external electric fields. On the other hand, absorption shielding is related to permeable materials i.e. magnetic materials. Accordingly, metallic conductors suffer a lack of flexibility, heaviness, and high costs. Meanwhile, ferromagnetic or ferrimagnetic materials have an intrinsic cut-off frequency, usually below the low GHz range, that hinders their use in EMI shielding over a broad GHz range. From this we concluded that, at present, we need to explore broadband shielding materials, those do not only work in the MHz range, but also neutralize EM waves in the GHz range. Most importantly, a lot of effort has been made in this direction; unfortunately to obtain simultaneously minimum reflection with a view to maximum absorption is still challenging task for practical applications.2–6
2 Scope of review
Up to date, iron (Fe) based composites have been extensively studied and are most desirable composites in various applications. Ten years of data on Fe-containing composites, collected by Scopus and shown in Fig. 1(a and b), show how the demand for Fe composites has increased year-by-year in several fields of research such as materials science, engineering and many others. It is expected that this review article will benefit ongoing research pertaining to iron nanostructures in the field of EMI shielding, since reviews play a crucial role in continuing interest on current aspects of research in every academic field. Therefore, this review mainly focuses on the development of high performance EMI shielding materials, considering iron as one of the important ingredients.
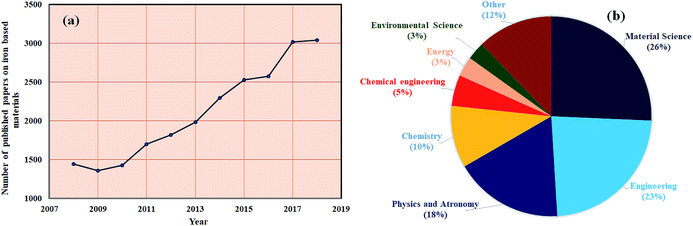 |
| Fig. 1 (a) and (b) Scopus database (09/12/2018) for iron based research articles. | |
2.1 Mechanisms of shielding
2.1.1 Shielding efficiency in terms of reflection/absorption.
Shielding efficiency (SET) could be defined as parameter that measures how well a material impedes the EM energy of a certain frequency when passing through it. Fig. 2a represents the possible interactions of EM waves with materials. When the EM waves fall on the front-face of the material then a certain part of the incident power (PI) is reflected (PR), while a certain part is absorbed and dissipated in form of energy, and the remaining part is transmitted (PT) through the shielding material. Therefore, three different processes namely reflection, absorption and multiple internal reflections contribute to the whole attenuation, corresponding to shielding effectiveness SER, SEA and SEM, respectively. |  | (1) |
Here P, E and H refer to power and electric and magnetic field intensities while subscripts I, R and T represent the incident, reflected and transmitted components, respectively. Thus, SER refers to net reflection and SEA represents shielding due to absorption. Note that contributions from secondary reflections (output interface) in Fig. 2a and b occur in finite-dimensional media but in thicker slabs SEM can be neglected (Fig. 2c). Then, the equation takes the form
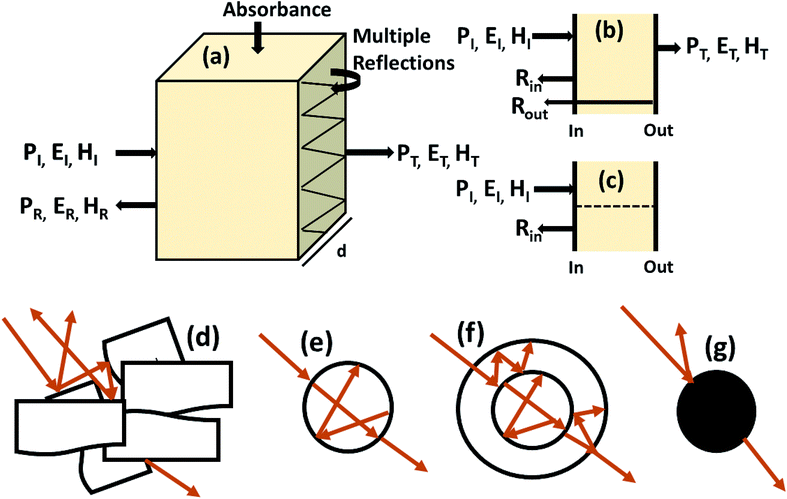 |
| Fig. 2 (a) Schematic diagram of incident, reflected and transmitted power and electro-magnetic field intensities when an EM wave is incident on a 3D material; (b and c) sources of reflection in a thin sample (input and output interfaces, Rin and Rout) and in a thick sample; (d–g) multiple reflections in the case of a porous structure, a hollow structure, a multiple shell structure and a solid sphere. | |
2.1.2 Reflection loss (SER).
The primary mechanism of EMI shielding is reflection. Reflection loss (SER) is related to the relative impedance mismatching between the surface of the shielding material and the EM waves. The magnitude of the reflection loss can be given by |  | (3) |
where σ is the total conductivity, f is the frequency, and μ is the relative permeability. It can be seen that SER is a function of the ratio of conductivity (σ) and permeability (μ) of the material i.e. SER ∝ (σ/μ). Thus, for a constant σ and μ, SER decreases with frequency. Therefore, materials must have mobile charge carriers (electrons or holes) for reflection of the EM radiation.
2.1.4 Multiple reflection (SEM).
For thinner materials, radiation is trapped between two boundaries due to multiple reflection, i.e. EM waves reflect from the second boundary, come back to first boundary and are re-reflected from the first to second boundary, and so on, as shown in Fig. 2a. | 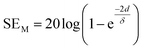 | (7) |
where δ is the skin depth, defined as the thickness below the outer surface at which the incident field is attenuated to 1/e of its initial value, given by
SEM depends on d and is closely related to absorption. Hence, multiple reflection plays an important role for porous structures and some definite geometries. For more visualization, Fig. 2d–g shows trapping/scattering of EM radiation by porous, hollow, multi-shell and solid structures. In this structure, a large surface area and a big vacant space excluding the solid structure gives more active sites for scattering and multiple reflection of electromagnetic waves. The hollow/porous structure shows unique properties, e.g., high surface area, disciplinable internal structures, low density and complimentary permeability that can fulfil the quest for improving EMI performance. These multiple reflections (SEM) can be neglected when the thickness of the shielding materials is greater than the penetration depth (δ) or when SEA is more than 10 dB because in thick shielding materials (high SEA) the EM wave hits at the second boundary with negligible amplitude so SEM can be neglected.
2.1.5 Perspective to minimize reflection.
It is clear that reflection, SER, depends solely on σ/μ, while SEA (dσμ) also depends on the sample thickness. Such dependency of SER/SEA on μ and σ indicates that in non-magnetic materials shielding is mainly governed by reflection, while in magnetic conducting metals shielding is dominated by absorption rather than reflection. This situation is quite different for composite materials in which heterogeneous micro structures show the great variations in the local fields due to these nano/micro extent which works as polarization sites. These sites create the lag of the displacement current relative to conduction current. Further, matrix and filler inclusions both have different electro-magnetic properties. In these conditions permittivity and permeability can be replaced by effective permittivity (ε = ε′ + iε′′) and permeability (μ = μ′ + jμ′′), respectively:where i is an imaginary number and ε are complex numbers. In the above equation, ε′ denotes the electric energy storage capacity, while ε′′ is related to dielectric losses. Similarly, permeability is given bywhere j is an imaginary number. In case of magnetic systems, μ′ denotes the magnetic energy storage while the imaginary part relates to ohmic losses similar to an electrical system. In complex permeability the μ′ and μ′′ of the materials are directly related to the energy density and magnetic loss power stored in the magnetic system. Therefore, these possess a complex dependency on the geometry, size, conductivity and volume fraction of each constituent. For several applications such as radar (to reduce the radar cross section) and military applications (e.g. hiding military devices), the essential requirement is to adjust the effective permittivity and permeability to certain values by which reflection can be minimized. Therefore, a prerequisite of conductive EMI shielding composites is to limit reflection and enhance absorption for effective EMI shielding materials. This is possible only when we minimize the mismatch of impedance between free space and shielding materials. According to the transmission line theory, intrinsic surface impedance in relation to complex permittivity and permeability for a given medium can be written as, | 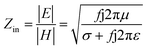 | (11) |
The microwave absorption properties of the materials in terms of reflection loss could be given by
| 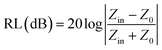 | (12) |
The maximum absorption of microwaves means that a minimum reflection loss (RLmin) occurs when the impedance of the composite and free space is matched. The ideal impedance matching conditions are when Zin = Z0 = 377 Ω. Here Z0 is the impedance of air, and Zin is the input impedance of the absorber. The above condition is fulfilled at a specific matching thickness (tm) and matching frequency (fm). An ideal EM absorption should make the effective width as broad as possible, which can be controlled by the 1/4 wavelength equation:5
| 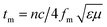 | (13) |
where
n is the refractive index and
c is the velocity of light. The RL value of −20 dB is considered to be 99% microwave absorption according to
eqn (8) and
(11), which is believed as an adequate level of absorption. In order to minimize the impedance mismatch, the best way is to increase the effective permeability or decrease the effective permittivity. Hence, high-performance microwave absorbing materials have been considered extensively to prevent incident EM wave radiation. These materials convert EM energy into thermal energy through dielectric loss and/or magnetic loss by the balance outcome of integralities between the relative permittivity and/or permeability. Moreover, technological fields desire not only efficient shielders, but also fulfil some necessitous criteria such as being lightweight, having a minimum thickness, corrosion- and chemical resistance, good flexibility, tunable morphology, ease of processing, and cheapness.
7
2.2 Factors affecting the EMI performance
2.2.1 Permittivity and permeability.
Ideal EMI shielders require impedance matching characteristics of composites which are influenced by permittivity and permeability according to following equation:8 |  | (14) |
Therefore, permittivity and permeability are crucial parameters to design an effective EMI shielding material, as explained in the previous section. For electrical shielding, conductivity and polarization loss are two key factors that are responsible for the dielectric loss (ε′′). Polarization loss could be based on electronic, ionic, dipole orientation (raised by bound charges) and interfacial polarization (due to trapping of space charge). Based on free electron theory, the dielectric loss is given by ε′′ = σ/2πε0f or ε′′ ∝ σ, where σ is the conductivity, which indicates that a high electric conductivity enhances ε′′. Ionic polarization and electronic polarization works only at the very high frequency region (above 1000 GHz) hence their effects can be neglected in the low microwave frequency region. Dipole polarization comes into the picture due to the presence of defects and residual groups in the material9,10 and mainly depends on the fabrication processes, chosen materials, annealing temperature etc. The interfacial polarization and respective relaxation appear to be due to trapped space charges at the interfaces. In this case the relaxation process can be investigated by a Cole–Cole semicircle obtained from the Debye dipolar relaxation process. The relationship between ε′ and ε′′ is | (ε′ − ε∞)2 + (ε′′)2 = (εs − ε∞)2 | (15) |
where εs and ε∞ are the static and relative dielectric permittivity at higher frequencies. If polarization relaxation takes place then an ε′′ versus ε′ plot will be a single semicircle. This plot is popular as the Cole–Cole semicircle plot. This type of polarization mostly appears in hierarchical and multi-interface composites.
On the other hand, magnetic loss comes from natural ferromagnetic resonance, exchange resonance and eddy current loss in the microwave frequency band. The natural resonance frequency fr correlates to an anisotropy field Ha which can be expressed by the natural-resonance equation: fr = γHa/2π, where γ/2π is the gyromagnetic ratio. The anisotropy field Ha is given by Ha = 2K/μ0Ms, where K is the anisotropy constant and Ms is the saturation magnetization. A high saturation magnetization (or a smaller anisotropy field) is ascribed to a red shift of the resonance frequency. In other words, a smaller anisotropy field improves the absorption bandwidth. For an excellent microwave-absorbing material, magnetic shielding requires conservation of its magnetic permeability over the GHz range, but it can be seen that at the cut-off frequency fr, permeability sharply decreases according to the Snoek’s limit, fr(μ − 1) ∝ Ms. Hence, a high Ms is required at high frequency fr. Magnetic metals and their alloys (Fe, FeNi, FeCo) possess high Ms and good permeability, although their high conductive behavior produces eddy current losses resulting in reduced permeability at lower frequencies (in the MHz range). Fortunately, ferrites are semiconducting in nature, but these ferrites possess a significantly lower Ms value and hence the fr occurs at the low GHz range. Therefore, the above-mentioned situations limit their use in the GHz range to the maximum bulk ferromagnetic materials. To overcome the above problem, researchers have focused on nano- or micro-sized materials because these low-dimension materials lower the eddy current loss.
2.2.3 Size, shape and morphology.
The dimensions of magnetic particles have a great impact on permeability. It is observed that below a critical small size, eddy current losses decreases due to the decrease in induced eddy voltage (Eeddy ∝ area). It is believed that anisotropy energy dominates at the small size of the nanostructures due to the breaking of some exchange bonds. The change in anisotropy energy modifies the spin relaxation time or frequency. Apart from the bulk magnet situation, permeability in nanomaterials is governed by relaxation mechanisms, in contrast to the intrinsic resonance which predicts a constant permeability until relaxation. In the superparamagnetic state, spin fluctuation remains very fast due to its small size, hence relaxation occurs at higher frequencies.7,14–18 Furthermore, some complicated structures consisting of high porosity and large surface area introduced multi-interfaces that accumulate to bound charges at the interfaces, causing the Maxwell–Wagner effect. In addition, several surfaces within complicated geometries possess unsaturated bonds that are responsible for dipole polarization. Therefore, multi-interfaces are beneficial for electromagnetic attenuation due to conductivity loss and interfacial/dipole orientation polarization. Many Fe and Fe-alloy based systems have been reported that confirm the effect of magnetic anisotropy and relaxation processes.
Bayat et al. have observed the effect of particle size and the thickness of material on the EMI performance of Fe3O4/CFs composites. When the particle size varies from 10–20 nm to 20–30 nm, then SETotal also varies from 47 dB to 68 dB. The above observation shows that larger size particles improve the electrical conductivity as they boost the graphitization of the carbon matrix. Thus, larger Fe3O4 NPs increase the magnetic permeability of the composite and hence improve the shielding efficiency of the composite. Similarly, thickness variation revealed that a 0.1 mm to 0.7 mm sample thickness enhances SET from 24 dB to 68 dB. This happened due to an increase in the conductive network, which enhances the SEA and total SETotal.
2.2.4 Temperature and time.
It is a well known fact that heat treatment increases disorder and creates defects in the form of vacancies, dangling bonds or substitutions in materials, as observed in the ferrite system in which reflection loss is reversed by the annealing temperature.19 These defects create an extra energy level around the Fermi level and hence enhance attenuation rather than reflection. Furthermore, reaction time and temperature also influence reflection loss, as reported for FeCo/ZnO composites20 because of structural changes that occur as the time and temperature increase.
2.2.5 Mass ratio.
Generally, the electrical properties of any of material depend on the percolation threshold value of conductivity:where σ is the electrical conductivity of the materials, σ0 is natural conductivity, V is the volume fraction of filler, Vc is the volume fraction at the percolation threshold and c is the critical exponent. At the percolation threshold, conductive networks form within matrices. The percolation threshold depends on certain factors like the shape, morphology, aspect ratio and conductivity of the filler. Moreover, it also depends on the distribution, concentration and compatibility of the filler with the host matrix.21 Above the percolation threshold, the properties of the composites start decreasing. For example, in elastomer composites a high volume fraction of filler (mostly metals) in the host matrices decreases the resilience of composites. For this region, a low volume fraction is most desirable. For example, Li and coworkers observed that, in nano Fe3O4 coated CNTs, reflection loss does not only depend on the Fe3O4 coating structure, but is also related to the CNT-to-Fe3+ mass ratio. This is because the mass ratio ultimately generates dielectric relaxation processes and also enhances the magnetic loss in the form of the eddy current effect.22
2.2.6 Thickness.
Minimal reflection, RLmin, of the microwave power occurs when the sample thickness, t, of the absorber approximates a quarter of the propagating wavelength multiplied by an odd number, that is |  | (24) |
where n = (1, 3, 5, 7, 9…), so that n = 1 corresponds to the first dip at low frequency. The propagating wavelength in the material (λm) is given by |  | (25) |
The matching condition results in the cancellation of the incident and reflected waves at the surface of the absorber material, e.g. the dips for t = 7 mm occurred at the sample thicknesses 1.0(λm/4), 3.0(λm/4) and so on. Hence, with increasing sample thickness, reflection peaks shift toward the lower frequencies. Apart from sample thickness, coating on the surface of the Fe component also changes the microwave absorption properties. This can be attributed to EM wave dimensional resonance, which increases with the increase of coating thickness. Du et al. have shown the influence of shell thickness on the absorption properties of Fe3O4@C composites. The thickness of the carbon shell in Fe3O4@C was controlled in the range of 20–70 nm.23 A critical thickness of carbon shells shows superior dielectric behavior.
3 Measurement techniques
Experimentally, network analyzer instruments are used to measure EMI shielding efficiency. There are two types of network analyzer: scalar network analyzers (SNA) and vector network analyzers (VNA). As its name indicates, the SNA measures signal amplitudes only, that is why it is not useful for measuring complex signals. On the other hand, the vector network analyzer (VNA) measures signal magnitude along with various phases. Therefore the VNA is a highly demanded and widely used instrument. In a VNA, its two ports (S1, S2) indicate the incident and transmitted waves in terms of complex scattering S parameters (Fig. 3), i.e. S11 or S22 and S21 or S12, respectively. These are known as the forward reflection coefficient (S11), the reverse reflection coefficient (S22), the forward transmission coefficient (S12) and the backward transmission coefficient (S21). Different conversion approaches such as the short circuit line (SCL), NIST iterative, delta-function method, new non-iterative, transmission line theory and Nicolson–Ross–Weir (NRW) technique have been adopted to obtain the characteristic parameters (i.e. ε, μ, RL and Z). The above conversion techniques also have some benefits and limitations. For instance, the short circuit line (SCL) method can estimate ε only, while the NIST iterative approach provides ε and μ but with the limitation μ = 1. Among them all, the NRW technique (presented by Nicolson and Ross in 1970 and by Weir in 1974) gives a direct calculation of complex permittivity and permeability from the input S-parameters. Therefore, the transmission line theory and the Nicolson and Ross and Weir algorithm are the more popular methods due to their ease of use.24 Parameters Z (Ω), RL (dB), SEA (dB), SET (dB) and SER (dB) can be obtained by using the following equations | 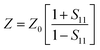 | (26) |
| RL = 20 log |S11| | (27) |
|  | (28) |
where T is the transmittance |  | (29) |
where R is the reflectance |  | (30) |
Summation of the reflectance (R), transmittance (T) and absorbance (A) is always equal to 1;
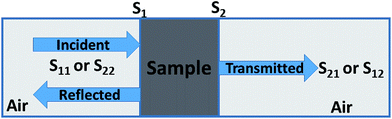 |
| Fig. 3 Reflected and transmitted EM wave in a filled transmission line. | |
Some researchers have also studied impedance matching by means of the delta-function method, in which the delta-function shows the impedance matching degree. The delta-function is given by following equation:9,25
| |Δ| = |sinh2(Kfd) − M| | (32) |
where
K and
M are
| 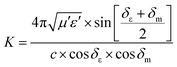 | (33) |
and
| 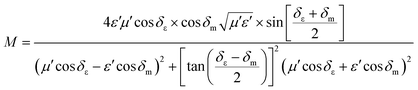 | (34) |
where
δε and
δm are the dielectric and magnetic dissipation factor and
c is the velocity of light. At a certain thickness, the maximum absorption occurs when RL approaches −∞. This leads to better impedance matching when the delta value tends to zero.
4 Materials used for EMI shielding
4.1 Iron (Fe) ingredient
For the development of high performance microwave absorption materials, magnetic nanostructures have been of great interest in the last few years. Their low cost facile synthesis along with the high biodegradability and biocompatibility advantages of iron and other components have made them desirable materials relative to other transition-metals in terms of potential applications. In the earth’s crust, the transition metal iron is the fourth most ubiquitous material that forms the inner as well as the outer surface of the earth. Iron is one of the most promising candidates for several applications including catalysis, microwave absorption, water pollution treatment and magnetic materials and many others. Ion can exhibit from the +2 to the +7 oxidation state, nevertheless the +2 and +3 states are more common due to the ease of hopping of the charge carriers. Fe is well known as the highest room temperature ferromagnetic material with a high saturation magnetization of 218 A m2 kg−1 at 293 K, and a curie temperature, TC = 1043 K, above room temperature. Furthermore, iron is a very soft magnetic material compared to cobalt and possesses low magnetocrystalline anisotropy. For a few decades, design of Fe based nanostructures has increased greatly because nanostructured materials have many advantages such as a high aspect ratio, good porosity and the high magnetic moment (superparamagnetic behavior) of the nanomaterials compared to bulk materials.26 Pure Fe is found either in the body-centered cubic (bcc) structure or face-centered cubic (fcc) structures, but exhibits extreme sensitivity of the structure of iron to changes in air conditions (orthorhombic, spinel) and hence the properties (such as electrical, magnetic, optical) of the Fe material. The most common iron species are iron oxides, ferric oxide, magnetite, ferrous oxides (FeO) and iron hydroxide (FeOOH), as depicted in Fig. 4. Although the fabrication of a magnetic iron nanostructure is quite difficult, much effort has been made to prepare Fe nanostructures using ball milling, DC arc plasma and sputtering methods.27 Among these, Fe nanostructures such as nanoflakes, nanoparticles and core–shell (Fe as core coated with oxide shell) structures are evidently the more common structures, because oxide shells not only prevent Fe from oxidation in the presence of air, but also prevent the forefront reflections as previously observed in pure Fe sheets that show negligible microwave absorption, due to the good conductivity of Fe elements (σ ∼ 107 S cm−1) and the strong skin effect at GHz high frequency. This is the main reason that Fe structures have been part of rather few studies. Some other studied Fe-based microwave materials include nanoparticles (NPs) and dendrite-like micro-structures that crystallize in bcc structures prepared by ball milling and hydrothermal process, respectively. The reflection loss of Fe NPs was observed by pelleting in a paraffin matrix, so for Fe/paraffin = 4/1, RLmin = 11 dB at 13.6 GHz. A complete energy dissipation of the EM wave occurs means no reflection and satisfies the impedance matching condition Z = Z0, indicating the absence of an actual absorbing resonance. In case of dendrite-like micro-structures, Ms is found to be higher than Fe nanoparticles but less than the bulk and hence attains an RLmin = −25.0 dB (matching frequency 2.5 GHz, matching thickness 3 mm). The excellent microwave absorption properties of Fe dendritic microstructures could be result of their hierarchical morphology, providing surface defects and a large surface area.22,28–30 More importantly iron occurs in various shapes, size and dimensions, such as nanowires, nanoparticles, nanorods, nanotubes, hollow fibers, microspheres and dendrite-like microstructures31 which enhance the reflection loss, but can moderate the conductivity. To overcome the above problem, iron oxides such as ferric oxide, magnetite and ferrous oxides (FeO) have been preferred for the design of effective microwave absorption materials because they are semiconductors (highly resistive).32
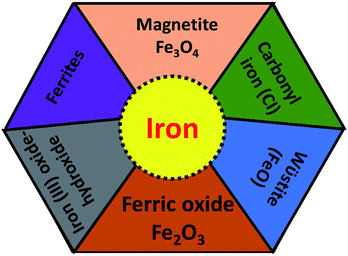 |
| Fig. 4 Different type of iron components. | |
4.1.1 Ferrites.
Ferrites have iron oxide as their main constituent, along with other metal oxides. These materials have been used from more than half a century due to their interesting magnetic properties. Compared to iron, ferrites possess high resistivity (0.1–10−5 Ω-m), high saturation magnetization and a tunable anisotropy field which make them a preferable choice in a wide range of applications such as bubble devices, the memory cores of computers and microwave devices, recording media, magnetic motors etc. Depending upon the crystal structure, ferrites can be classified into the following types:
4.1.1.1 Spinel ferrite.
Spinel ferrites are given by formula PFe2O4, where tetrahedral and octahedral interstitial sites are designated with P (divalent metal ions like Cu, Co, Mn, Ni, Zn) and Fe, respectively. Considering its applicability in the microwave region, the spinel ferrites can be utilized as microwave-absorbing materials, because these ferrites have large magnetic losses and moderate conductivity (semiconductor property). However, spinel ferrites in MW-absorbing applications are restricted because of their low natural magnetic resonance frequency.
4.1.1.2 Garnet.
This is described by Pe3Fe5O12 where Pe stand for a trivalent ion e.g. a rare earth element. These ferrites have a similar structure to spinel ferrites but with some extra sites (a dodecahedral c axis). Doping of cations in these sites may be helpful because lattice interaction with these sites may tune the physical properties of ferrites.33 Like spinel ferrites, garnet ferrites are soft ferromagnetic materials with high remanence, a large saturation magnetization and low coercivity. Moreover, the good chemical stability and EM compatibility of these ferrites show their potential for EMI suppression.
4.1.1.3 Ortho-ferrites.
The general formula for these ferrites is PeFeO3, where Pe is a large trivalent metal or rare earth ion such as Bi or Y. These ferrites exhibit a weak/canted antiferromagnetism with affluent magnetic properties. For instance, ortho-ferrite shows a phase transition from paramagnetic to antiferromagnetic at 620–750 K. Moreover, these kind of ferrites possess excellent multi-ferroelectricity and tunable magnetic properties in which the interaction between Fe3+ and Pe3+ ions decides the magnetic properties of the ferrites.34
4.1.1.4 Hexagonal ferrites.
Hexagonal ferrites have a high magnetocrystalline anisotropy field and a planar anisotropy that improves their natural resonance in the upper gigahertz range. This property of hexagonal ferrites increases their versatility in a variety of applications. These ferrites crystallize in a hexagonal structure. Apart from spinel ferrites, the magneto-plumbite structure of these ferrites enables theme to working in the entire GHz range due to their high intrinsic magnetocrystalline anisotropy.35 Hence, some of them have gained considerable technological importance in recent years. There are six type of hexagonal ferrites:
4.1.1.4.1 M-Type.
M-Type ferrites are given by the formula PFe12O19 where P = Ba, Sr, Mg, Pb etc. These ferrites are composed of the form SRS*R*, in which R and S indicate the three and two oxygen-ion layer blocks. The large magneto-crystalline anisotropy, inexpensive price, high Curie temperature and competent saturation magnetization properties of these kind of ferrites stand them as effective microwave materials.
4.1.1.4.2 Y-Type.
The Y-type ferrites are ferrimagnetic materials, generally given by the formula P2Q2Fe12O22 where P = Ba, Sr, Mg, Pb, and Q = Cu, Co, Zn etc. The magnetic properties of these type of ferrites are greatly susceptible to their crystalline structure, especially in presence of a magnetic environment. Thus, the addition of divalent, trivalent and tetravalent species in these hexaferrites controls their magnetic characteristics in order to obtain improved microwave absorption.36
4.1.1.4.3 W-Type.
W-Type ferrites are given by the formula P2Q2Fe16O27. The crystal structures of these ferrites are closely related to the M-type. The characteristics of these ferrites depend on their particle size or morphology, synthesis method and the distribution of the cations in the crystal structure. These hexagonal ferrites are made up of the structure SSRS*S*R* in which R is a three oxygen-ion layer block with a composition of PFe6O11, S is a two oxygen-ion layer block with the composition of Fe6O8, called the spinel block. In the above equation, an asterisk indicates the rotation of the block by 180° along the hexagonal axis. The W-type structure composed of spinel blocks is twice as thick with respect to the M-type hexagonal structure.37,38
4.1.1.4.4 X-Type.
X-Type ferrites are represented by the formula P2Q2Fe28O46. These are composed by the structre 3(SRS*S*R*). X-Type hexagonal ferrites can be considered as a mixture of M and W-type hexagonal ferrites. In comparison with M and W-type hexagonal ferrites, these ferrites possess a larger Curie temperature and saturation magnetization, and hence work as excellent microwave absorbing materials.
4.1.1.4.5 Z-Type.
The Z-type ferrites are given by the formula P3Q2Fe14O41. These hexagonal ferrites have much good permeability and a higher resonance frequency (fr) in comparison with spinel ferrites. That is why these ferrites are only used in microwave devices like antennas, inductors and absorbers etc.39
4.1.1.4.6 U-Type.
The U-type hexagonal ferrites are represented by the formula P4Q2Fe36O60. Among the hexagonal ferrites, the U-type ferrites possess better thermal stability, a large magnetic anisotropy (Ha) and a large saturation magnetization (Ms).35 Therefore these ferrites have been used in many studies on EMI applications.
4.1.2 Ferric oxide (Fe2O3).
Among the iron oxides, biocompatible Fe2O3 is the most common oxide of iron. Therefore, it is one of the most extensively used biomaterials in different applications like cell separation and drug delivery etc. Fe2O3 occurs in an amorphous form and consists of four polymorphs (alpha, beta, gamma and epsilon).31 The multitudinous polymorph structures α and γ named as hematite and maghemite, respectively. The α-Fe2O3 has a rhombohedral–hexagonal type structure, whereas γ-Fe2O3 shows a cubic spinel structure, as shown in Fig. 5a. On the other hand, the β-Fe2O3 and ε-Fe2O3 polymorphs have cubic bixbyite and orthorhombic structures. The α- and β-Fe2O3 are termed antiferromagnetic and paramagnetic materials, respectively. Hence these are extremely useful in photocatalysis, conversion of pigments, solar energy and water treatment. In contrast, γ and ε-Fe2O3 possess ferromagnetism40 so that these are particularly useful in bio-medicine. α-Fe2O3 and γ-Fe2O3 have been widely investigated in EMI shielding applications. Different composites comprising α-Fe2O3 in attractive morphologies such as popcorn-like α-Fe2O3, coin-like α-Fe2O3, watermelon-like α-Fe2O3 microspheres, α-Fe2O3 nanorods and hollow γ-Fe2O3 have been studied and have shown excellent microwave performance. In general, thermo-chemical, two step hydrothermal, solvothermal, chemical reduction and sol–gel approaches are some of the reported methods which have been employed to prepare Fe2O3 based composites.41–46
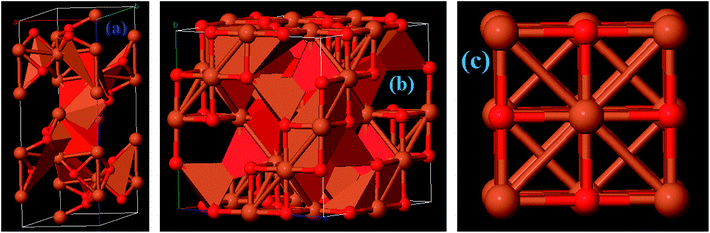 |
| Fig. 5 Crystal structure of (a) Fe2O3, (b) Fe3O4, and (c) FeO materials. | |
4.1.3 Magnetite (Fe3O4).
Among all the Fe oxides, Fe3O4 is the most comprehensively investigated magnetic nanostructure because of its ease of synthesis, high biocompatibility, superparamagnetic nature, high chemical stability, low toxicity etc. Several low cost preparation methods of Fe3O4 nanostructures can be found in the literature such as sol–gel, solvothermal, co-precipitation and magnetic separation methods etc. As a result, magnetite has versatile applications in fields of magnetic storage devices, food analysis, magnetic resonance imaging (MRI), segregation of biomolecules, hyperthermia, and EMI applications,47 particularly in the field of magnetism owing to its high magnetic moment. Moreover, Fe3O4 nanostructures possess a cubic inverse spinel structure with two Fe3+ and one Fe2+ valence state in which oxygen frames a fcc closed-pack structure, as depicted in Fig. 5b. It is an indispensable kind of half-metallic material in which electron hopping takes place between the Fe2+ and Fe3+.48,49 Consequently, the outstanding magnetic/dielectric properties of the Fe3O4 nanostructure make it a favorable candidate for magnetic/electric attenuation sources in the EMI shielding mechanism. Fe3O4 has an abundant number of morphologies e.g. it occurs in sandwich-like Fe3O4, dendritic forms, and as nanorods, nanoparticles, microspheres and nanospindles. Thus, Fe3O4 can be considered as a good choice for energy applications, including EMI.
4.1.4 Wüstite (FeO).
Iron(II) oxide (FeO) has a cubic (rock salt) structure in which iron and oxygen atoms are octahedrally coordinated to each other, as depicted in Fig. 5c. FeO is not stable at normal temperature and hence shows high temperature and pressure stability only above 560 °C (ref. 50) which results in high costs of preparation and limits its potential application. Therefore, FeO has rarely been studied. Zhu et al. prepared for the first time Fe@FeO dispersions in a polyurethane (PU) matrix.51 It was seen that Fe@FeO NPs became magnetically harder after being dispersed in the PU matrix. Fe@FeO/PU possess a significant eddy current effect hence RL is >20 dB even at larger absorber thicknesses. Nevertheless, a coating of SiO2 exhibits better performance than Fe@FeO and Fe@FeO/PU composites because the silica shell significantly reduces the eddy current loss and causes an upsurge in the anisotropy energy.
4.1.5 Iron oxy-hydroxide (FeOOH).
Iron(III) oxy-hydroxide occurs in following forms: goethite (α-FeOOH), akaganeite (β-FeOOH), lepidocrocite (γ-FeOOH) and feroxyhyte (δ-FeOOH). These are widely used in electrode materials and lithium batteries. Iron(III) oxy-hydroxide has poor magnetic as well as electrical properties, which are a primary requirement for EMI applications. Therefore iron(III) oxy-hydroxide materials are not very popular among material scientists.
4.1.6 Carbonyl iron (CI).
Finally, carbonyl iron (CI) is another captivating magnetic absorbing material that has attracted much attention due to its virtuous properties including superior saturation magnetization, a high Curie temperature, and a high magnetic loss with low permittivity. Interestingly, the magnetic properties of CI are tunable in accordance with its size, morphology and shape. In fact, planar anisotropy as observed in CI nanoflakes, effectively improves the Snoek’s limit which increases the permeability and resonance frequency at the same time. Besides, in the high frequency range, such flakes-type structures can ignore the skin effect.52
Although these Fe materials offer several advantages, their high density, heavy weight, processing difficulties, flexibility and narrow absorption bandwidth impede their further application. Fe materials suffer from the skin depth problem; on the other hand ferrites are restricted by Snoek’s limit. As we explained earlier, to design an excellent microwave absorbing material, one needs to optimize its permeability and permittivity, due to the magnetic/dielectric loss capabilities of EM energy. Hence, poor permittivity in comparison to permeability is the main drawback of these Fe oxides. Accordingly, scientists have mainly concentrated on materials which show the complementary relation between permittivity and permeability. In this direction, conducting polymers and carbon based materials have attracted the attention of researchers. Many strategies have been employed to develop effective shielding materials.
5 Anchoring of metal oxides
Anchoring of transition metal oxides such as ZnO, ZrO2, MnO2, SnO2, BaTiO3, TiO2, SiO2 with Fe ingredient enhance the permittivity of EMI preventing materials. Thus the combination of these oxides with Fe ingredients significantly improve the dielectric losses and magnetic losses in materials by mean of double attenuation mechanism which is accountable for superior microwave absorption performance. Their cheap, natural richness and environmentally friendly properties make them more accessible for EMI shielding. To date, numerous Fe and transition metal oxides with great EM properties have been explored. However, these semiconductor oxides are restricted at the high GHz range due to their lack of permittivity. Moreover, processing-related difficulties, agglomeration during synthesis and poor dispersion are major drawbacks in the use of Fe/metal oxides composites.
6 Conducting polymers (CPs)
In comparison to conventional metals and semiconductors, conducting polymers (CPs) possess exclusive properties such as a lower density (1–1.3 g cm−3) than iron (7–8 g cm−3), gentle processing and preparation conditions, structural flexibility, and most importantly tunable conductivity (0.1–10−10 S cm−1). Conductive polymers have various applications in sensing, metal corrosion protection, and specifically in energy storage like electromagnetic shielding and microwave absorption. The peculiarities of conducting polymers are believed to depend on their doping level, dopant ion size, water content and protonation level. Two well-known methods have been reported to prepare CPs: CPs are either prepared by electrochemical oxidative polymerization, or by the chemical oxidative polymerization method. Chemical oxidative (in situ) polymerization is the most frequently used method to prepare such polymer composites, and is also known as the chemical encapsulation technique. In this method, a filler such as Fe3O4 nanoparticles are first dispersed in a liquid monomer. The polymerization reaction is initiated by heat/radiation, the diffusion of the appropriate initiator takes place, then the organic initiator/catalyst is set on the surface of the nanoparticles under the required temperature, pressure and stimulation (stirring) conditions, as shown in Fig. 6. In fact, fabrication of polymer nanocomposites is a hybridization process between the organic/inorganic polymer matrix and the inorganic/organic nanofiller to achieve a single material which comprises integrated properties with respect to the matrix and filler only.53 This method also helps the modulation of shell thickness in the case of a core–shell structure just by controlling the weight ratio of the monomer and the Fe-based nanostructure, which influences the EM wave absorption properties effectively. According to dissipation mechanisms, microwave absorbing materials show dielectric loss and magnetic loss. In microwave absorbing materials, conducting polymers (CP) serve as dielectric loss materials which makes them the most attractive candidate.54 Among the various conducting polymers polyaniline (PANI), polypyrrole (PPy), poly(3,4-ethylenedioxythiophene) (PEDOT), polythiophene (PT), polyfuran (PF), poly(para-phenylene) (PPP) and poly(phenylenevinylene) (PPV) are of particular interest due to their easy availability, environmental sustainability, cost-effectiveness and versatile doping chemistry.
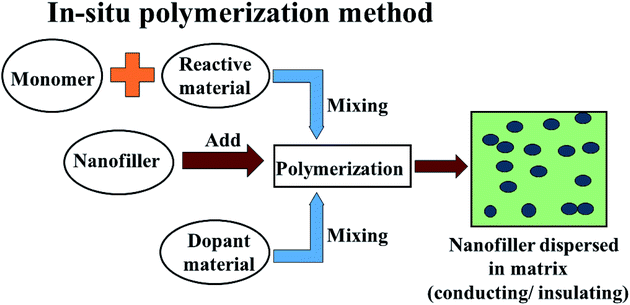 |
| Fig. 6 The in situ polymerization method of preparation of conductive and insulating polymers. | |
6.1 Polyaniline (PANI) polymer
Among the different conducting polymers, polyaniline (PANI) is one of the most commonly used polymers as a host material for micro/nano-sized nanofillers owing to its unique physico-chemical properties. These polymers show improved mechanical properties (tensile strength and elongation at break), thermal stability and particularly enhanced electrical conductivity and magnetic properties; these are the prerequisites for the design of effective EMI shielding materials. In comparison with other CPs, PANI is one of the oldest CPs and was first highlighted in 1862 due to the oxidation of an aniline monomer in sulphuric acid. The conductivity of PANI lies between 0.1 and 10−10 S cm−1. Moreover, PANI is a biocompatible and anti-corrosive polymer which has a controllable dielectric loss ability and is feasible for composition with micro/nano-sized magnetic metals.55 Over the last two decades, many efforts have been made to prepare composites comprising polymers and nanofillers. However, improvement of the electric and magnetic properties of the filler/polymer composites are insufficient to design effective EMI shielding materials; one important factor that is still required is how to combine influentially the permeability and permittivity of the these composites. To fulfil these conditions ferromagnetic materials possessing high permeability such as Fe, Fe3O4 and Fe2O3 and dielectric materials such as TiO2, SiO2, and ZnO are widely used in polymer composites. At broad GHz range, however, these dielectrics suffer from a lack of permittivity. For this purpose, carbonaceous materials such as graphene, MWCNT and RGO have also been used with these polymers.
6.2 Polypyrrole (PPy) polymer
After the PANI polymer, polypyrrole (PPy) is another most promising conductive polymer because of its tunable stability and ease of preparation, but suffers from poor mechanical strength and processability problems along with insolubility and infusiblity.56 These drawbacks hinder its commercial application. To conquer the above problems, magnetic/metal nano-fillers as inorganic filler can be used with PPy to integrate the electro-magnetic properties of polymer composites. These nanoscale fillers have received increased interest due to their intriguing properties arising from their large surface area and nanosize in the host matrix. When the proper combination of magnetic nanofillers along with dielectric materials are encapsulated within the PPy polymer matrix then these polymer composites provide a new perspective to tune the dielectric and permeability properties of magnetic and dielectric materials in a different way by the control of the polymer structure and functionalisation.
6.3 Poly(3,4-ethylenedioxythiophene) (PEDOT) polymer
Among the conductive polymers, a polythiophene derivative poly(3,4-ethylenedioxythiophene) (PEDOT) possesses a moderate band gap, controllable electrical conductivity, attractive electrochemical activity. Interestingly, the light weight, easy synthesis processes, good environmental stability, and dielectric loss ability properties of PEDOT make it a promising microwave absorbing material. It is a well known fact that poor EM impedance matching is attributable to magnetic or dielectric loss only. For this purpose, to achieve excellent microwave absorption performance PEDOT has been used with magnetic γ-Fe2O3, Fe or Fe3O4 components.47
6.4 Polythiophene (PT) polymer
Similar to other conducting polymers, polythiophene (PT) is used in anti-corrosion devices, rechargeable batteries and chemical sensors. Similar to other CPs, the conductivity of the PT materials could be controlled from a conducting to an insulating nature by only changing the polymerization route. Nevertheless, its poor solubility restricted its commercial use in many applications. Besides, the inert sulphur atom in thiophene enhances the oxidation potential, which makes the fabrication of polythiophene more complicated.57 Therefore, these polymers have been the subject of few studies.
7 Nonconducting polymers nanocomposites
Though the conductive polymers have many advantages, they suffer from a lack of flexibility and processability during the large scale production of materials. Herein, insulating polymers like rubber and resin have been utilized as alternative substrates for conductive polymers. This is because non-conducting/extrinsic polymer synthesis processes are very cheap, easy, time sparing and environmentally stable. In addition, they can be prepared on large-scale quantities. To overcome the poor electrical, thermal and mechanical properties of these insulating polymers, metal, alloy and carbon nano fillers are often mixed into the polymer matrices to enhance the mechanical strength, conductivity and permeability, which improves reflection well as absorption, depending on the filler characteristics. Mostly facile solution mixing, melt mixing and in situ polymerization methods are used for the preparation of these polymer-containing composites. In the solution mixing method the polymer and filler are dissolved or dispersed in a common solvent and undergo a stirring and sonication process until the complete mixing/blending of matrix and filler occurs, followed by casting and drying of the as-prepared composites. In melt blending/mixing, the polymer is melted at high temperature. To avoid the use of a solvent, the mixing of filler and matrix (polymer) takes place at high temperature followed by cooling and drying, as shown Fig. 6 and 7. In situ polymerization processes have been generally used to synthesize nanocomposites having insoluble and thermally unsteady matrices (insulating polymers) that can not be developed by solution/melting methods. For more details, some of these extrinsic polymers are explained in later section.
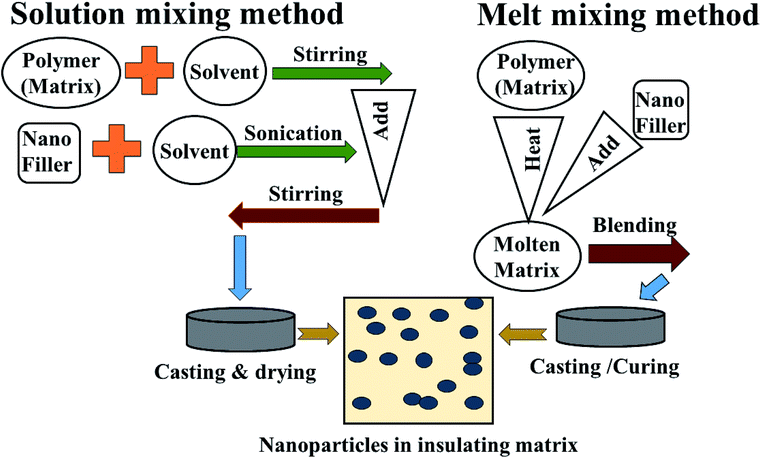 |
| Fig. 7 Method of preparation of extrinsic polymers: (1) solution mixing and (2) melt mixing methods. | |
7.1 Polyvinylidene fluoride (PVDF) polymer
The fantastic piezoelectric behavior, light weight, compact size, good flexibility, and excellent dielectric properties of the PVDF thermoplastic open up the door to wide applications in various fields. Polyvinylidene fluoride (PVDF) (transparent to light) is a semi-crystalline polymer having significant thermal stability and good chemical resistance among polymers. It occurs in five crystalline phases α, β, γ, δ and ε, each with different chain conformations. Hence, PVDF as a matrix in nanocomposites is one of the key parameters for a wide range of applications. Pure PVDF has poor EMI shielding properties,6 but the addition of Fe based nanoparticles within the PVDF matrix improves its conductivity and enhances its response by capitalizing on the nature and properties of the nanoscale filler. In this direction, Zheng and coworkers investigated the microwave properties of the PVDF polymer with the nanofiller MnFe2O4/RGO.58 They found that the composites had a minimum reflection loss of 29.0 dB at 9.2 GHz at a 5 wt% loading of filler. Moreover, a high dielectric loss and magnetic loss occur due to synergistic effect between RGO and MnFe2O4, RGO and PVDF, and PVDF and MnFe2O4. This analysis shows that PVDF takes a part in impedance mismatching and improved the performance.
7.2 Thermosetting polymers
Versatile thermosetting-resin-based composites offer good adhesion, resistance to corrosion, high strength and stability. These polymers commonly establish a good dispersion and interfacial adhesion between the filler and the polymers. Epoxy resins are one of the most important thermosetting resins, especially for industrial applications. In general, polyurethanes (PU) and polyethylene terephthalate (PET) polymers (after epoxy) are used to suppress EMI pollution. However, these polymers do not respond in presence of EM waves due to their insulating behavior. Therefore, they are widely used with conducting polymers and carbon materials along with Fe materials. Moreover, thermosetting polymers like epoxy compounds are also used as binders with Fe materials that prevent the aggregation of Fe nanostructures and serve as ideal dispersing materials.
7.3 Elastomeric polymers
Elastomers are polymers which exhibit visco-elasticity and are bounded with weak intermolecular forces. These polymers are insulating in nature and have poor physico-mechanical properties (e.g. low Young’s modulus). Ethylene-vinyl acetate (EVA), ethylene-propylene-diene monomer (EPDM) and nitrile rubber (NBR) are some examples of these synthetic rubbers. Apart from some weaknesses, rubber has excellent weathering resistance, resistance to aging, and chemical resistance along with good compatibility with many kinds of fillers.59 Therefore, these elastomers have been used with magnetic Fe ingredients and conducting polymers or carbon materials, which improve its conductivity and enhance the EMI performance.
7.4 Other polymers
Apart from the polymer matrices discussed earlier, other polymers such as polyvinylpyrrolidone (PVP), polyvinyl chloride (PVC), poly(p-phenylenevinylene) (PPV), polypropylene (PP), polyvinyl butyral (PVB), polyvinyl alcohol (PVA), polyethylenimine (PEI) and polycarbonate, along with blends (PC (polycarbonate)/SAN [poly(styrene-co-acrylonitrile)]) and polymer composites have also been studied. Akinay et al.60 synthesized polyvinyl butyral (PVB)/Fe2O4 and (PVB)/NiFe2O4 composites and observed that the composites exhibit good RLmin performances in the 1–14 GHz range. In NiFe2O4/PVB composites, percolation of NiFe2O4 particles within the PVB matrix resulted in good RLmin values. In contrast, the overall microwave absorption performance was better in Fe3O4/PVB in comparison with (PVB)/NiFe2O4. In similar way, Yao et al.61 reported better EMI performance of PVC/graphene/Fe3O4 composites. PVC composites have negligible EMI SET due to their insulating behavior. In comparison to pure PVC, the addition of 5 wt% graphene and 5 wt% Fe3O4 nanoparticles form sufficient conducting interconnected graphene–Fe3O4 networks in the insulating PVC matrix. Hence graphene/Fe3O4/PVC obtained an improved ST value compared to PVC/Fe3O4 and PVC/graphene composite.
8 Carbonaceous materials
Carbonaceous materials with unique characteristics such as low density, high permittivity, excellent conductivity, high chemical, thermal and mechanical stability are a current fields of growing interest scientifically as well as technically. These materials offer a great opportunity to fabricate a lot of varieties of new generic materials, with tunable optical, electrical, mechanical and magnetic properties. Most importantly, the high permittivity of carbonaceous materials establishes complementary behavior between the Fe ingredients and the carbon based materials that make it suitable for EMI applications. It is a well known fact that in the universe, after the evolution of hydrogen, helium, and oxygen, carbon (C) is the fourth most common chemical element. Pure carbon occurs in two main ordered lattice structures: diamond and graphite, shown in Fig. 8(a and b). Diamond has many industrial uses like cutting, and polishing of equipment, along with some scientific applications. Moreover, diamond is the hardest natural material, highly thermally conductive and electrically insulating (band gap ∼ 5.5 eV), as well as valuable and venerable; these properties cause it to be disfavoured in potential energy applications. On the other hand, graphite is soft, lubricating and electrically conductive. Furthermore, carbon possesses various allotropes, as depicted in Fig. 9(a–c), comprising 2D graphene, 0D buckminsterfullerene and 1D carbon nanotubes (single wall and multi wall). These lightweight carbonaceous materials and their derivatives with Fe ingredients serve as excellent candidates for the design of effective EM reflection/or absorption materials. A brief introduction to some carbon materials which are usually used in EMI shielding applications, along with their pro and cons, is given in a later subsection.
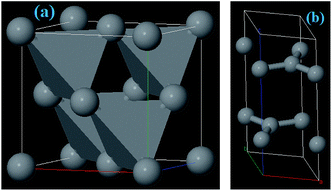 |
| Fig. 8 (a) Crystal structure of diamond and (b) graphite. | |
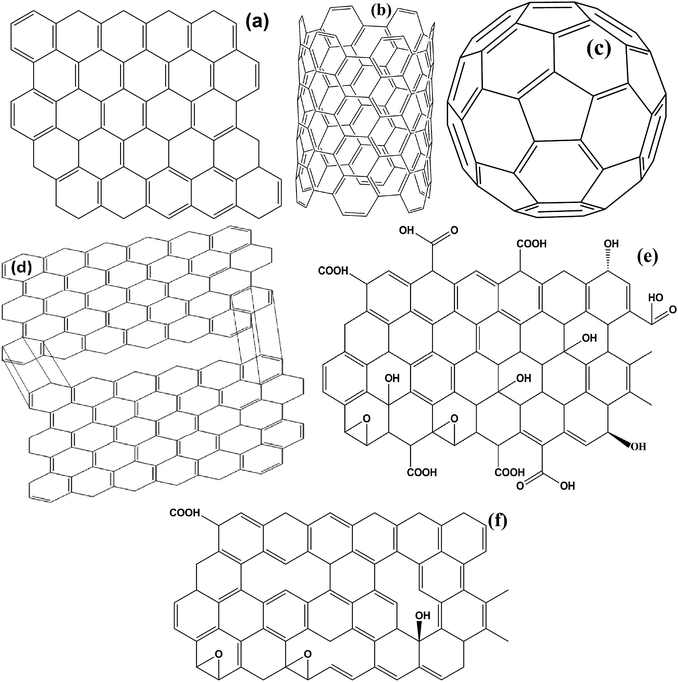 |
| Fig. 9 (a) 2D graphene, (b) carbon nanotube, (c) fullerene, (d) expanded graphite, (e) graphene oxide and (f) reduced graphene oxide. | |
8.1 Graphite/expanded graphite
Graphite is a traditional carbon material which has a layered lattice consisting of hexagonal rings of carbon atoms attached by weak van der Waals forces in different planes. Within a plane, the carbon atoms are joined together by covalent bonds. As a low cost, lightweight lubricant graphite possess good electrical conductivity, a high aspect ratio, and good mechanical and thermal stability that establish it as attractive filler in several potential applications in the fields of electronic, optical and energy devices. However, the major drawback of graphite is its poor dispersion in solvents. Therefore, functionalized graphite, produced by HCl and H2SO4 acid treatment, is mostly used to prepare the composites.62 Apart from conventional graphite, more and more interest is being extended to expanded graphite. Expanded graphite (EG), obtained by thermal treatment, has many advantages, e.g., EG is 2-dimensional, consisting of a small stack of graphite layers, low cost and has poor resistivity and high mechanical stability (Fig. 9d). The major problem of using these materials is their poor magnetic properties that restrict their practical application. Therefore, anchoring of Fe ingredients with graphite or expanded graphite integrates their magnetic properties due to the synergistic effect between iron and the graphite and thus enhancing the EMI performance in the microwave region.
8.2 Graphene
Graphene (G or GNS) is defined as a 2-dimensional (2D) allotrope of carbon atom formed by a single atomic layer of a honeycomb hexagonal lattice that hybridizes by sp2 bonding, as depicted in Fig. 9a. Graphene has an amazing mechanical strength with good elasticity, excellent electrical conductivity, superior thermal conductivity, extremely high surface area (∼2630 m2 g−1 theoretical value) and extraordinary electrical and thermal stability. Moreover, the theoretical dielectric loss of graphene is found to be superior than conventional oxide materials like ZnO, TiO2 or SnO2.5 Several preparation methods of graphene, including top-down or bottom-up approaches and chemical vapor deposition (CVD) have been reported till now. However, these physical methods do not offer the large scale production of graphene. Additionally, the lack of surface functionalities and the excessively high carrier mobility of graphene is also harmful for EM absorption, creating impedance mismatching between air and the material. Hence graphene’s derivatives such as graphene oxide (GO) and reduced graphene oxide (RGO) are more broadly used as alternative to graphene in practical applications.
8.2.1 Graphene oxide (GO).
It has been demonstrated that when graphite is oxidized with strong oxidizing agents, the resulting attached oxygen functionalities, carboxyl, carbonyl, hydroxyl and epoxy groups (i.e. COOH, O–H) expand the layer separation within graphite along the c axis and make it hydrophilic. This hydrophilicity enables us to extract graphene oxide after water sonication (Fig. 9e). The most appealing property of GO is easy dispersion in either kind of solvent (organic or inorganic), because organic groups pave the way for GO to be modified easily by other materials. Moreover, GO can be well dispersed in a polymer matrix because of the strong and specific interactions (e.g. hydrogen bond) among the organic groups on the GO surface and the polymers. As reported by Samadi et al.63 for Fe3O4–GO/PVDF composites show better electromagnetic microwave absorption than pure PVDF. GO in Fe3O4–GO/PVDF composites does not only affect the reflection loss and absorption bandwidth but also has a great impact on the α-to-β phase transformation of the PVDF crystals. To evaluate quantitatively the EMI performance by GO we shall discuss its electro-magnetic properties. The disruption of sp2 bonding in GO diminishes its electrical properties. Hence GO acts as an electrical insulator, directly this is not very useful. However, the Fe components improve its conductivity to a certain extent. Furthermore, to recover the honeycomb structure of GO, different methods like reflux, hydrothermal and sol–gel approaches have been employed.
8.2.2 Reduced graphene oxide (RGO).
Among all the carbon-derived materials, reduced graphene oxide (RGO) is a most promising material with diverse applications in several branches of science. In RGO, the oxygen functional group is removed using a reducing agent such as hydrazine hydrate, NaBH4 or NaOH etc. Reduced graphene oxide (RGO) is the most studied carbon derivative due to its cost effective preparation, good flexibility, superior electric/thermal conductivity and attractive barrier properties.64 Moreover, RGO comprises remanent functional groups and defects within the sheet which improve impedance mismatch, defect polarization relaxation and electronic dipole relaxation (Fig. 9f). All these groups and defects increase absorption rather than reflection, as can be seen in graphite and carbon nanotubes. For example, Wang et al.65 investigated the microwave absorption properties of chemically reduced graphene oxide. They observed that residual defects and organic groups within RGO not only improved the individual impedance matching but also produced energy transitions from the continuous states to the Fermi level. Furthermore, these peculiarities introduce relaxation polarization, defect polarization relaxation and electronic dipole relaxation which favor EM wave penetration and absorption. Compared with graphite and carbon nanotubes, reduced graphene oxide has a higher dielectric/magnetic loss by means of microwave absorption. Thus, due to the unique properties of RGO and Fe-based materials, as well as the synergistic effect between them, many reduced graphene oxide/Fe based composites for EMI shielding have been investigated. He and coworkers66 mixed reduced graphene oxide (RGO) nanosheets with the flaky carbonyl iron (FCI) as depicted in Fig. 10(a and b). They observed that FCI/RGO composites (−65.4 dB at 5.2 GHz at thickness 3.87 mm) lead to better microwave absorption properties compared with pure FCI (−13.8 dB at 13.7 GHz at thickness of 2.28 mm), as shown in Fig. 11(a and b). More interestingly, they used the delta-function method to see the contribution of typical dielectric dispersion behavior in FCI/RGO. It is anticipated that a smaller delta value gives better impedance matching. Since FCI/RGO possesses a larger area close to zero, which can directly explain the better matching of the characteristic impedance in FCI/RGO composites. Therefore, recent investigations have mainly concentrated on RGO and Fe-, Fe3O4- and Fe2O3-based composites due to their ease of preparation. In most of these cases the chemical reduction method is employed to fabricate the Fe- and RGO-based composites. In this method, Fe3O4 nanoparticles and GO are ultrasonicated/stirred followed by the addition of a reducing agent/surfactant and heat treatment by re-fluxing or hydro-thermal means, etc. which reduced the GO into RGO, as shown in Fig. 12.
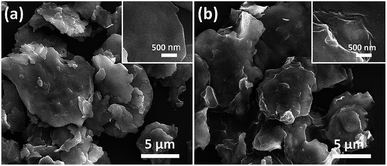 |
| Fig. 10 (a) SEM images of FCI and (b) RGO-coated FCI66 – reproduced by permission of the Royal Society of Chemistry. | |
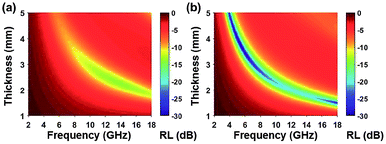 |
| Fig. 11 (a) Reflection loss mapping of FCI and (b) RGO-coated FCI with absorbers thickness from 1 mm to 5 mm in the frequency range of 2.0–18.0 GHz66 – reproduced by permission of The Royal Society of Chemistry. | |
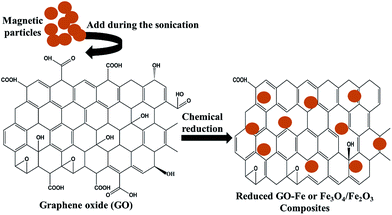 |
| Fig. 12 Chemical reduction method of preparation for Fe and RGO based composites. | |
8.3 Carbon nano tubes (CNTs)
Carbon nanotubes are unique one-dimensional (1D) nanostructures that can be understood hypothetically as a 1D quantum wire. These nanotubes belong to the fullerene family. Structurally, CNTs are a long, hollow structure with cylindrical walls framed by a honeycomb lattice (similar to graphene). Carbon nanotubes have received much recognition due to their intriguing electronic, mechanical (tensile strength is >60 GPa) and thermal properties.67,68 CNTs may be semiconductors or metallic depending on their structure and diameter. Furthermore, the high aspect ratio, low mass density (∼1.6 g cm−3), and wall integrity of CNTs enable them to serve them as superb nanofillers for improving the properties of composites.22,62 There are two main types of carbon nanotubes: single walled carbon nanotubes (SWCNTs) and multi-walled carbon nanotubes (MWCNTs).
8.3.1 Single walled carbon nanotubes (SWCNTs).
Single walled carbon nanotubes (SWCNTs) are an allotrope of sp2 hybridized carbon atom, similar to fullerenes. A single sheet of carbon comprises the wall thickness all around the circumference (diameter ∼ 1.4 nm). The structure of SWCNTs is a cylindrical tube including six-membered carbon rings similar to graphite. Single walled nanotubes are a crucial type of carbon nanotube owing to their good electric properties compared to MWCNTs. The electrical properties of SWCNTs are distinctly different from their larger diameter MWCNTs counterparts due to their smaller diameters and larger aspect ratios. Because of this, the EM-absorbing properties of MWCNTs and SWCNTs are expected to be altogether different.69,70 The main flaws of SWCNTs are the complicated synthesis procedure, extremely high conductivity and poor magnetic properties. These characteristics of SWCNTs inhibit their use as excellent microwave absorbing materials. Although the incorporation of Fe materials improves their magnetic and electrical properties, as studied by Kuchi et al. in Fe3O4/SWCNT composites,71 SWCNTs still have been the subject of rather few studies.
8.3.2 Multi walled carbon nanotubes (MWCNTs).
Multiwalled carbon nanotubes (MWCNTs) are one of the most preferable CNTs. Structurally, MWCNTs possess multiple layers of graphite superimposed and rolled in on themselves to make a tube shape. Moreover, these can be considered as a collection of concentric SWCNTs consisting of different diameters, lengths and natures. The distance between each layer is well known to be approximately 0.34 nm.72 MWCNTs are most promising 1D materials due to their attractive properties. Note that structural disorders, appearing in pristine MWCNTs during their development, are responsible for the unusual electrical and optical etc. properties of MWCNTs. These structural disorders might be Stone–Wales defects, atomic defects or in the form of vacancies and incomplete bonding defects etc. As the result of their high aspect ratio, large surface area and low percolation threshold, MWCNTs are favored as effective fillers rather than SWCNTs in terms of EMI shielding potential; despite this, their comparatively high cost limits their application to some extent.
The available literature on CNTs demonstrates that pure CNTs manifest low absorption but a significantly larger skin depth. However, the addition of Fe species to CNTs greatly improves their microwave absorption, as predicted by Che et al. and Qi et al. in the case of CNTs/CoFe2O4 and Fe/CNTs composites, respectively. The combination of Fe compounds with CNTs (e.g. Fe/CNTs or CNTs/CoFe2O4) presents better matching between the dielectric and magnetic losses. Moreover, observations have revealed that a fine dispersion of CoFe2O4 nanoparticles within the CNT matrix weakened the congregation of the CoFe2O4 particles, resulting in dipolar interaction and the resonance absorption effect, owing to the shape anisotropy.3,73
8.3.3 Carbon fibers (CFs).
Similar to other carbon materials, carbon fibers (CFs) also possess a high mechanical strength modulus and a low density but a poor thermal expansion coefficient. CFs composed of fibers between (50 to 10 μm in diameter) mainly consisted of carbon atoms. Nevertheless, their lower magnetism and high conductivity increases impedance mismatching in EMI due to increasing their skin depth, similar to CNTs. Hence, modification of CFs with Fe, Fe3O4, Fe2O3 or alloys could be a useful approach to handle the above problem. Still, the high cost of CFs limits their potential for extensive use as effective fillers.
Apart from these fillers, graphitic carbon, carbon black and carbon coils have also been investigated for EMI applications. Although the large surface area of these fillers improves many properties, the major impedance to using these materials as fillers is the requirement for a high weight % ratio, which deteriorates the mechanical properties in case of these polymers.
9 Strategies for the preparation of effective EMI shielding materials
As we discussed in earlier sections, Fe-related materials offer significant improvements of the complex permeability μ which lead to a larger impedance matching value. Subsequently, these magnetic composites shows strong interface polarization (in case of multi-interface materials) which offer advantages with respect to conversion of the incidence EM thermal energy into thermal energy. Keeping this in mind, different strategies for EMI shielding materials and magnetic absorbers have been proposed by scientists, as shown in Fig. 13, and explained in later subsections.
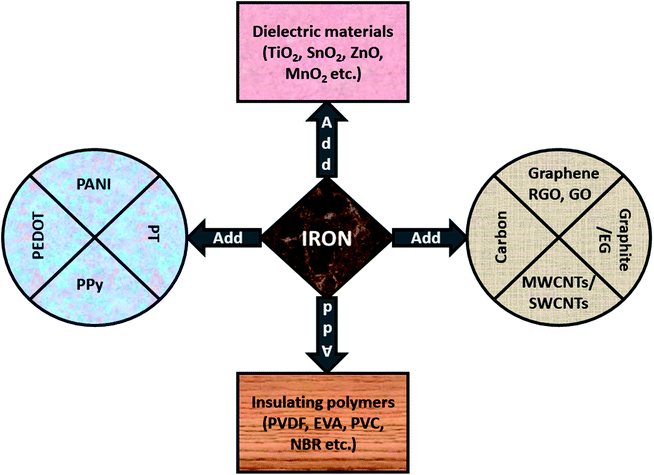 |
| Fig. 13 Iron can be used with carbonaceous materials, conducting polymers, dielectric materials or insulating polymers. | |
9.1 Hierarchical/porous structure or divalent/trivalent ion substitution in ferrites
The first strategy is to make a hierarchical structure of Fe-based materials that improve the performance. It is well established that there are two factors that mainly affect microwave absorption: dielectric loss and magnetic loss. Permittivity and permeability result from electronic polarization, ion polarization, dipole polarization, natural resonance, exchange resonance, hysteresis and eddy losses, in which size, distinct geometrical morphology and crystal structure may have an essential impact. Therefore, several scientists have merely paid attention to complicated morphologies of Fe materials. To my knowledge, Fe-based structures including flakes of α-Fe2O3 and Fe,41,74 octahedral Fe3O4,75 Fe nanowires, urchin-like structures of Fe3O4, α-Fe2O3,76 nanocapsules such as α-Fe/ZnO, α-Fe/SnO and Fe/Fe3B/Y2O3,77 dendritic structures of Fe, Fe3O4 and α-Fe2O3,40 and loose nano-Fe3O4 (ref. 78) have until now been exposed as efficient EMI shielding materials. Shang et al.75 have shown that, in the case of octahedral Fe3O4 nanoparticles, their anisotropic structure has excellent magnetic characteristics on account of its shape anisotropy. Moreover, the octahedral structure is also advantageous for reflection scattering from multi edges. Zhang et al.79 made a comparative study of sphere-shaped particles and flake-shaped carbonyl iron particles. They found an optimal reflection loss (12.2 dB at 4.4 GHz at 1 mm thickness) in flake carbonyl iron particles, which was better than that in sphere-shaped particles. Similarly, porous Fe materials have also been widely studied due to their large specific surface area, pore volume and attractive magnetic properties. In addition, the porous structure endows multiple reflection properties to the material which contribute to its total effectiveness. Li et al.80 noticed improved absorption in MnxFe3−xO4 hollow/porous spherical chains. These findings in MnxFe3−xO4 occurred due to the porous and hollow structures, the oriented arrangement of the nanocrystals and Mn2+ substitution, because Mn2+ replacement induced a dual-frequency absorption in MnxFe3−xO4 in the 2–18 GHz range. Since substitution has great impact on the EMI properties, divalent and trivalent impurities such as Bi, Al, Nb, Cu, Ni, Zn are therefore widely substituted in several ferrites, as listed in Table 1 where
is the total shielding effectiveness and RL is the reflection loss. Moreover, the line width of magnetic resonance is directly related to the magnetocrystalline anisotropy of the ferrite system. To achieve broad-band and strong magnetic loss in ferrites, a high anisotropy field (Ha) is needed. In this context, doping of divalent or trivalent elements in ferrites enhances their EMI performance. For instance, Song et al.81 studied the influence of Al-substitution on the microwave absorbing properties of Ba1.5Sr0.5CoZnA1−xFe12−xO22 (for x = 0–1) hexaferrites in which domain wall resonance and natural resonance were found to responsible for effective microwave absorption. Zhang and coworkers82 observed the effect of NdCo substitution of SrFe12O19 ferrites, i.e. Sr1−xNdxFe12−xCoxO19 (x = 0–0.4). The complex permittivity of these ferrites resulted from the significant contributions of Nd3+ and Co2+ ions. Further dielectric properties of SrFe12O19 ferrites arise mainly because of the interfacial polarization and intrinsic electric dipole polarization which occurs as a result of electron hopping between ions of different valence states. Thus, Nd3+ ions preferentially substitute for Sr3+ ions, and Co2+ ions preferentially substitute for Fe3+ ions, which enhances the electron hopping. In the meantime, magnetic loss in ferrites usually originates from natural ferromagnetic resonance and domain wall resonance, but domain wall resonance was found in the low-frequency region (<2 GHz). On the other hand, resonance due to the spin rotational component occurs at high frequency regions. Thus, the magnetic loss in the M-type strontium hexaferrite/paraffin was found to be due to natural resonance. Li et al.83 have studied Fe3O4/NiFe2O4/Ni heterostructured porous rods in which they observed that controlling the NiFe2O4 interface layers and Ni content can improve impedance matching and dielectric losses, thereby leading to lighter weight, a stronger absorption, and a broader absorption band of Fe2O4/NiFe2O4/Ni compared to Fe2O4.
Table 1 Fe based microwave absorber
Fe based components |
Materials |
Methods |
Thickness/mm |
 /dB |
Frequency/GHz |
Ref. |
Porous Fe particles |
Corrosion technique |
1.8 |
−42.2 |
13.2 |
84
|
Porous CI flake |
Two-step approach |
3.5 |
−41.8 |
4 |
85
|
α-Fe2O3 nanoflake |
Hydrothermal |
5 |
−41.67 |
2.8 |
41
|
Loose nano-Fe3O4 |
Hydrothermal |
5.5 |
−30.33 |
13.54 |
78
|
Hexagonal Fe flakes |
Hydrothermal |
1.1 |
−15.3 |
12.2–16.6 |
74
|
Fe nanoparticles |
Ball milling |
1.4 |
−23.67 |
15.24 |
75
|
Urchin like α-Fe2O3 |
Two step process |
1–5 |
−9.2 |
3.76–8.15 |
76
|
Urchin like Fe3O4 |
Two step process |
3–4 |
−29.96 |
3.76–8.15 |
76
|
Octahedral Fe3O4 |
In situ molten salt |
1.4 |
−23.67 |
15.24 |
75
|
γ-Fe2O3 dendritic |
Hydrothermal |
4 |
−50 |
2–13 |
40
|
Fe3O4 dendritic |
Hydrothermal |
4 |
−53 |
2.2 |
40
|
Fe dendritic |
Hydrothermal |
3 |
−25 |
2.5 |
40
|
Fe3O4 nanosphere |
Hydrothermal, calcination |
3 |
−12 |
12.7 |
86
|
Porous flower like Fe3O4 |
Reflux |
2 |
−28.31 |
13.2 |
87
|
Coin-like Fe |
Reduction |
1.4 |
−53.2 |
16 |
88
|
SiC–Fe3O4 nanowires |
Polyol approach |
— |
−51 |
8.6 |
89
|
γ-Fe2O3 nanosphere |
Hydrothermal, calcination |
3 |
−18 |
14.8 |
86
|
Fe nanowire/epoxy |
Chemical vapour deposition |
2 |
−47 |
9.4 |
90
|
Porous α-Fe2O3 nanosphere |
Hydrothermal, calcination |
3.5 |
−25 |
13 |
86
|
MnxFe3−xO4 spherical chains (x = 0.74) |
Solvothermal |
2.6 |
−52.8 |
10 |
80
|
Coinlike α-Fe2O3@CoFe2O4 |
Solvothermal |
2 |
−60 |
16.6 |
45
|
Co/CI nanoparticles |
Electroless plating process |
1.8 |
−27.8 |
10.4 |
91
|
CoxFe3−xO4 (x = 0.9) |
Solvothermal |
2 |
−41.09 |
12.08 |
92
|
Fe/ZnFe3O4/CI |
Ball milling, in situ |
1.5 |
−47 |
6.2 |
93
|
Ni.5Zn0.5/Fe2O4/Co flake |
Co-precipitation |
1.5 |
−33.8 |
11.5 |
94
|
Ni1−xZnx/Fe2O4 (x = 0.5) |
Sol–gel |
5 |
−29.6 |
6.5 |
95
|
Ni1−xCox/Fe2O4 (x = 0.3) |
Co-precipitation |
2 |
−18 |
2.45 |
96
|
ZnxCu1−x/Fe2O4 (x = 0.8) |
Sol–gel, auto-combustion |
3 |
4–9 |
8.2–12.4 |
97
|
CuxNi0.4−xZn0.6/Fe2O4 (x = 0.2) |
Ceramic method |
1 |
−60 |
0.01 |
98
|
α Fe/Fe3B/Y2O3 |
Melt-spun technique |
4 |
−33 |
4.5 |
99
|
Ba1−2xLaxNaxFe10Co0.5TiMn0.5O19 (x = 0.1) |
Solid state reaction |
1.3 |
−45.94 |
8.33 |
100
|
Ba1−xCexFe12O19 (x = 0.21) |
Sol–gel |
10 |
−20.47 |
16.22 |
101
|
Ag3PO4/SrFe12O19 |
Hydrothermal |
5.1 |
−63.18 |
4.72 |
102
|
Sr1−xNdxFe12−xCoxO19 (x = 0.4) |
Sol–gel, autocombustion |
1.9 |
−22 |
16.2 |
82
|
BaFe12−xTixO19/CI (x = 0.2) |
Sol–gel, physical blending |
4.5 |
−30.7 |
5.67 |
103
|
Sr1−xBa3−xCo2Fe24O41 (x = 1.5) |
Sol–gel |
5 |
−48 |
17.6 |
39
|
Ba3Co2Fe24O41 |
Sol–gel |
5 |
−50 |
4.5 |
104
|
(CuZn)xCo2(1−x)Fe24O41 (x = 0.3) |
Sol–gel |
25 |
−29 |
11.4 |
105
|
Ba2−xDyxZn2Fe28−yMnyO46 |
Sol–gel |
— |
−55 |
11.62 |
106
|
BaSrCo2−xNixFe12O22 (x = 0.5) |
Solid state reaction |
1.2 |
>−45 |
12 |
107
|
BaSr0.5CoZnFe12−xAlxO22 (x = 0.3) |
Sol–gel |
3 |
−19 |
11.5 |
36
|
Sr(MnTi)xCoZnFe12−2xO19 (x = 1) |
Aqueous combustion |
2 |
−22.7 |
11.5 |
108
|
SrZnCoFe16O17 |
Combustion synthesis |
2.6 |
−33.6 |
10.4 |
109
|
Sr0.9Nd0.1ZnCoFe16O17 |
Ceramic |
4.6 |
−21 |
9.6 |
37
|
SrZn2−xCox/2Nix/2Fe16O17 (x = 0.4) |
Co-precipitation |
1.8 |
−29.11 |
14.57 |
38
|
Ba0.5Sr0.5CoxWxFe12−xO19 (x = 0.2) |
Ceramic |
2.8 |
−15.2 |
11.22 |
110
|
9.2 Alloying of Fe with other metals
The second strategy is to make alloys of Fe with other materials. In this case the other material may be either magnetic, such as Co or Ni, or nonmagnetic like Zn, Cu etc. It is well established that ferrites have a low Curie temperature TC, moderate saturation magnetization (Ms) value and a negative thermal coefficient of resistivity, while pure Fe suffers a stability problem in air along with forefront reflection due to its high conductivity. Nevertheless, a material with high saturation magnetization Ms is required for better microwave absorption/EMI performance. Therefore, Fe based alloys can be used as an ideal candidate because their combined properties not only improve Ms and the Curie temperature (TC) but also provide environmental stability to Fe. In this regard, intermingling of the properties of metals could be achieved by alloying two (binary alloy), three (ternary alloy) or more metallic elements. In EMI applications, alloying provides strong bonding between the alloy filler and the matrix and also increases the interfacial polarization, saturation magnetization and permittivity etc., which cannot be obtained in pure metals. For example, a 20% Fe content in an FeNi alloy shows interesting physical properties in comparison with pure Fe or Ni, and also the FeNi alloy is cost-effective compared to magnetic materials such as Ni, Co and Fe3O4. Extensive research has been conducted on Fe alloying with Co, Ni, Cu, Si and Al elements within dielectric materials like ZnO, carbon (graphite, CNTs etc.), polymers such as PANI (conducting) and PVDF, and EPDM (non-conducting) matrices, respectively.111–115 These matrices enhance the permittivity of the alloy filler and make it low in weight and flexible. For example, Feng et al. have shown dual dielectric relaxation in the case of FeNi@C nanocomposites which occurs due to a cooperative consequence of the FeNi–C interfaces and dielectric carbon.116 Therefore, the synergy of dielectric and magnetic losses in FeNi@C provides excellent microwave absorption performance. Meanwhile, enhanced EMI shielding effectiveness (ST) was obtained by Choi et al. in FeCo hollow fibers mixed with ethylene propylene diene monomer (EPDM).117 In the FeCo/EPDM composites an increased number of conducting paths were formed because of the high aspect ratio of the fibers, which enhanced the reflection loss by impedance mismatching. Some of the studied alloy systems are listed in Table 2.
Table 2 Fe alloying based microwave absorber
Materials |
Method |
Thickness/mm |
 /dB |
Frequency/GHz |
Ref. |
Fe3O4 coated Fe0.65Co0.35 flakes |
Solvothermal |
5.89 |
−41.4 |
2.02 |
118
|
Ni–Fe–P/PET |
Electrodeposition |
— |
69.2–80.3* |
1.5 |
119
|
FeCo/EPDM |
Electroless plating process |
— |
30* |
12.5 |
117
|
Fe–Si–Al/PP |
Two roller mixer |
— |
−9.3 |
0.8 |
120
|
Ni–Fe–P/TS |
Electroless plating process |
— |
60* |
1.5 |
121
|
FeCo/ZnO |
Hydrothermal |
1.5 |
−31 |
5.5 |
122
|
FeNi/CFs |
Two-step electrodeposition |
2 |
42* |
0.03–0.1 |
111
|
FeNi@C |
Hydrothermal |
2 |
−47.6 |
3.17 |
116
|
FeNi3@C@RGO |
Three step reaction |
2.6 |
−47.6 |
10.2 |
114
|
FeCo@SiO2@MWCNT |
Two step reaction |
3 |
−35 |
18 |
123
|
FeCo@SnO2@graphene@PANI |
Three step reaction |
3 |
−39.8 |
6.4 |
124
|
Co3Fe7@C yolk–shell |
Hydrothermal, heat treatment |
2 |
−35.3 |
9.1 |
125
|
FeCoNi-EG |
Electroless plating process |
3 |
−28.8 |
13.5 |
126
|
FeCo@SiO2@MWCNTs |
Multi step processes |
3 |
−35* |
18 |
127
|
Carbon–FeCu@CNBs |
Sol–gel, reduction |
7.5 |
−21.02 |
12.21 |
128
|
FeCo13@C |
Three steps |
1.5 |
−6.7 |
11.1 |
129
|
FeCo/graphite nanoflakes |
Jet milling, acid treatment |
2 |
−30.6 |
7.4 |
130
|
FexNi1−x@PVDF@MWCNT |
Acid treatment, melt mixing |
2.5 |
−58* |
8–12 |
115
|
CI/Fe91Si9 |
Blending technique |
3 |
−45 |
5.2 |
131
|
9.3 Core@shell structures
The third strategy for achieving high-performing EMI materials is the fabrication of a core–shell structure of Fe-related materials, which is indicated by the term core@shell (core/shell or core–shell). Core@shell structures have emerged as a type of important nanostructure for various applications in different branches of sciences such as electronics, chemistry, biomedicine, energy, optics etc. Core@shell nanostructures could be attributed as a new kind of important functional material consisting of different functional compositions on the nanometer scale. The core and the shell can be made by two different materials such as organic/inorganic and vice versa, or by the same material, i.e. organic/organic or inorganic/inorganic, with distinct structures. Core and shell properties either arising from the core or the shell. These materials can be modified by varying the building materials or the core or shell thickness ratio. The core and shell occur in different forms, as shown in Fig. 14. The core may be a single sphere wrapped by single shell (Fig. 14a), or a multi-shell (Fig. 14b). Moreover, the shell might be hollow, in which a small sphere as the core is trapped in to shell. This type of core@shell is known as a yolk–shell structure (Fig. 14c). On other hand, a yolk–shell also can be multi-shell structure (Fig. 14d). In particular, the core@shell structure might take the form of rod-, tube- or hexagonal-flake-type morphologies (Fig. 14e–g). Furthermore, the core can be an accumulation of small spheres (Fig. 14h) within the shell. The core@shell structure might take the form of smaller spheres attached on the surface of the shell (Fig. 14i); instead of a continuous layer shell, it might also take the form of smaller spheres attached to a core sphere (Fig. 14j). The challenge in the preparation of core@shell nanoparticles (NPs) is to find a simple, cost-effective and less time-consuming strategy with minimum environmental impact. Du et al.23 prepared a 14a-type core@shell Fe3O4@C structure with 500 nm Fe3O4 microspheres. Observation revealed that carbon coating on the Fe3O4 microspheres increased the complex permittivity, and improved impedance matching occurred due to multiple relaxation processes. On the specific thickness of shells, Fe3O4@C showed an unusual dielectric behavior that favored a strong reflection loss, even at high frequencies. On other hand, Guo et al.132 formed a mesoporous α-iron oxide@nSiO2@mSiO2 multi-layer 14b-type core–shell structure. The α-iron oxide@nSiO2@mSiO2 composites showed improved electromagnetic interference (EMI) shielding effectiveness (SE) compared to the pure hematite materials. Yu et al. produced a yolk–shell Fe3O4@ZrO2 core@shell structure of the 14c type.133 They analyzed the effect of temperature on reflection loss and observed that even at 500 °C, Fe3O4@ZrO2 sustained over 90% of its reflection loss (RL) value with respect to its room temperature properties. Liu and co-workers present134 a 14d-type yolk core–shell. The unique morphology, well-defined shells, favorable magnetization, large specific surface area, and high porosity of these double-shelled Fe3O4@SnO2 yolk–shell microspheres significantly enhanced their microwave absorption characteristics. The exceptional microwave absorption properties of these Fe3O4@SnO2 yolk–shell microspheres may be ascribed to the distinctive double-shelled yolk–shell structure and the synergistic effect between the magnetic Fe3O4 cores and the dielectric SnO2 shells. Chen and coworkers generated Fe3O4@carbon nanorod (14e-type) structure via a three-step process.135 The outstanding EM wave absorption properties of these porous Fe3O4@carbon core/shell nanorods were ascribed to complementary behaviour between the dielectric loss and the magnetic loss as well as the unique structure of the porous Fe3O4@carbon structure. Furthermore, the Fe3O4@TiO2 core/shell nanotubes type of core@shell (14f-type) morphology was predicted by Zhu and coworkers,136 in which the eddy current effect decreased effectively and an improvement in anisotropy energy was observed due to the presence of the TiO2 shells. The maximum reflection loss was obtained −20.6 dB at 17.28 GHz with a 5 mm thickness in the tube like core@shell structure. Liu et al. have shown137 a 14g-type core@shell structure of Fe@SiO2 microflakes which demonstrated excellent microwave absorption performance of compared to Fe microflakes. In this case, effective balancing between the dielectric loss and magnetic loss improved the impedance matching. The structure of the Fe@MoS2 composite shows a 14h type core@shell structure, as explained by138 Pan et al., in which 2 dimensional MoS2 nanosheets were distributed on porous coin-like Fe micro-sheets. The addition of MoS2 with Fe controls the permittivity and improves the impedance matching. Therefore, an optimal reflection loss was obtained around −37.02 dB at a coating thickness of 2.0 mm. In Fe@MoS2 composites, the magnetic loss is dominant over the dielectric loss. Among all magnetic losses, such as hysteresis loss, domain wall resonance loss, natural ferromagnetic resonance loss and eddy current loss, natural ferromagnetic resonance loss and the eddy current effect normally play a vital role in Fe@MoS2. Therefore, efficient microwave absorption can be attributed to improved impedance matching and the synergistic effect between the magnetic loss and the dielectric loss. Liu et al. presented excellent microwave properties with 14i-type iron oxide cores and hierarchical copper silicate shells.139 A maximum reflection loss value of these Fe3O4@Cu-silicate microspheres of 23.5 dB was achieved at 7 GHz with a thickness of 2 mm. The high porosity, large specific surface area and synergistic effect of both the magnetic Fe3O4 cores and the hierarchical copper silicate shells and their unique morphology plays an important role for impedance matching in microwave absorption applications. Zhu et al.140 produced the 14j-type structure in a Fe3O4 polyelectrolyte (PE)@PANI/paraffin composite. The Fe3O4-PE@PANI/paraffin composite exhibits an RLmin of −6.5 dB at 14.3 GHz. In the above nanocomposites, the peculiar structural interfaces produce interfacial relaxation between the Fe3O4 nanoparticles and PANI hollow spheres, which is also beneficial for microwave absorption.
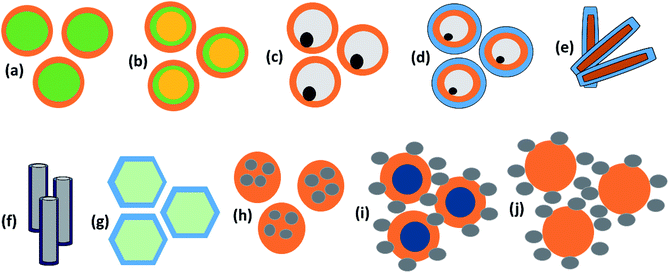 |
| Fig. 14 Schematic representation of the different types of core@shell structures. | |
In brief, Fe-based core–shell composites are of great interest due to their potential applications, because the core–shell structure have several advantages such as the confinement effect, interfacial polarization, complementary behavior, and core-corrosion protection. Therefore, a variety of core@shell composites with satisfactory EM wave absorption have been studied till now. These structures enhance microwave absorption due to their specific structure, e.g. a hollow multi-shell favors multiple reflections; meanwhile their porous nature and multiple interfaces increase interfacial polarization. Accordingly, the core structure might be binary (containing two different elements), ternary (containing two different elements) or quaternary core@shell (containing four different elements).
9.3.1 Binary composites.
Different strategies have been employed to fabricate binary core@shell structures for microwave applications. The binary core@shell can be prepared in the following ways:
9.3.1.1 Magnetic core@magnetic shell.
In this case, the core and shell are both prepared by magnetic materials. The core can be any magnetic element, alloy, ferrite or ceramic. Wang et al.141 studied the same type of Fe3O4/Co core@shell type structure. In this work, they showed that Co nanoshells form surface layers over the Fe3O4 core which greatly enhance the conductivity and permittivity. Moreover, free electrons in a metallic Co nanoshell can move freely within it. These charge carriers accumulate at the Fe3O4/Co interface, and form a structure similar to a boundary-layer capacitor and generate interfacial electric dipolar polarization. The permeability could be explained by hysteresis loss, domain-wall resonance, the eddy current effect and natural resonance. They excluded hysteresis loss because the applied microwave field was weak. Domain-wall resonance usually takes place at a frequency lower than the gigahertz range. Furthermore, they observed a high skin depth with respect to the diameter of the materials’ grain size, hence the eddy current can be precluded. For these reasons, natural resonance is deemed to be the main magnetic loss mechanism for the Fe3O4/Co sample. Despite this, the magnetic core@magnetic shell shows superior EMI performance, but suffers from a dual magnetic loss which decreases the dielectric loss and support to impedance mismatching.
9.3.1.2 Magnetic core@dielectric shell.
In this case the core is selected as magnetic and the shell is dielectric. The core may be any magnetic element, alloy, ferrite or ceramic, similar to those detailed in magnetic core@magnetic shell section. As a dielectric source, several dielectric materials such as Al2O3, TiO2, ZnO, ZrO2, SnO2, carbon materials and polymers have been investigated so far, as shown in Table 3. Among all core@shell structures, the magnetic core@dielectric shell is considered to be a highly desirable core–shell structure, because protective encapsulation on the surface of core prevents oxidation and agglomeration of the Fe metal nanoparticles. Additionally, the above type of core@shell material works as bridge between dielectric and magnetic losses. Hitherto, a lot of studies have been performed on Fe-based core@shell nanostructures. For example, Fe3O4@ZnO core–shell,142 ZnO-coated iron nanocapsules,143 SnO-coated-Fe(Sn) nanocapsules,77 Fe@Al2O3,144 Fe3O4@SnO2 double shells,134 Fe@SiO2 nanoflakes,145 spinel Fe3O4@ TiO2,146 Fe nanoparticles with amorphous Al2O3/FeOx composites shells,147 and yolk–shell Fe3O4@ZrO2 (ref. 133) have been investigated as effective microwave absorbing materials. Liu et al.148 reported the synergistic effect of magnetic loss and dielectric loss in the Fe/SnO2 nanocapsules which enhanced its microwave absorption properties. They found two typical dielectric resonances at 3.8 and 16 GHz arising due to a synergistic effect of the Fe nanoparticles cores and SnO2 shells. An additional peak at 14 GHz could be explained as spin wave excitation. In the intervening time, they observed that the μ values indicate a peak at 3.2 GHz due to natural resonance within the Fe/SnO2 nanocapsules. They explained that the natural resonance might be the result of a surface/small size effect and spin wave excitations of the Fe/SnO2 nanocapsules.149 Similarly, Qiang et al.150 prepared Fe/C nanocubes obtained from Prussian blue (PB) nanocubes at varying pyrolysis temperatures (ranging from 600–700 °C) for their EMI properties. Fig. 15(a–d) shows SEM images of the Prussian blue (PB) nanocubes and Fe/C at different pyrolysis temperatures of 600 °C, 650 °C, and 700 °C. Fig. 15a shows the PB nanocubes with smooth surface and an edge length of around 600 nm. At high temperature pyrolysis, the PB nanocubes are in situ converted into Fe/C composites (Fig. 15b and c) but at 700 °C a little shrinkage in the cubic skeletal structure was observed. Fig. 16(a–c and e–g) shows the complex permittivity and permeability of Fe/C nanocubes in the frequency range 2–18 GHz. It is observed that the three samples exhibit different complex permittivity and permeability at different pyrolysis temperatures. Generally, the values of ε′/μ′ and ε′′/μ′′ are related to the electrical conductivity and magnetic losses of the EM absorbers. A high electrical conductivity is advantageous for a high complex permittivity while magnetic loss mainly comes from hysteresis, domain wall resonance, natural ferromagnetic resonance, and the eddy current effect. It is well established that both the carbon material and iron component are electrically conductive; the electrical conductivity of the carbon materials is more sensitive to the pyrolysis temperature with respect to the metal iron. Thus the improved graphitization degree of carbon components in Fe/C nanocubes increase the complex permittivity while the natural ferromagnetic resonance is the primary reason for the magnetic loss. Fig. 16(d and h) represent the dielectric/magnetic loss tangents, which indicate that dielectric loss ability is regularly improved with increasing pyrolysis temperature, whereas magnetic loss tangents varies in the limited range. It is clear from Fig. 16(f–l) that different Fe/C nanocubes display different EM absorption responses. The optimum RL was obtained at 650 °C, due to its promising dielectric loss and magnetic loss, as well as improved matched characteristic impedance.
Table 3 Binary Fe based microwave absorber
Materials |
Methods |
Thickness/mm |
 /dB |
Frequency/GHz |
Ref. |
Binary composites
|
Fe3O4@TiO2 nanotubes |
Three-step process |
5 |
20.6 |
17.28 |
136
|
Fe3O4@C yolk–shell |
Chemical reduction |
3 |
−45.8 |
10.6 |
152
|
Fe3O4 sphere@C |
In situ polymerization |
2 |
−20.6 |
13.4 |
23
|
Fe@NiFe2O4 |
Ball milling |
1.5 |
−27 |
11.2–18 |
153
|
Fe@ZnFe2O4 |
Ball milling |
1.5 |
−39 |
11.2–18 |
153
|
Fe3O4@ZrO2 |
Sol–gel |
2 |
22 |
6 |
133
|
Fe3O4@MnO2 |
Hydrothermal |
3 |
−43.6 |
9.2 |
154
|
Fe3O4 nanocrystal@ZnO |
Heterogeneous nucleation |
3.5 |
−22.69 |
10.08–15.97 |
155
|
Porous Fe3O4/carbon nanorods |
Hydrothermal |
2 |
−27.9 |
14.96 |
135
|
Fe3O4@SnO2 double shell |
Hydrothermal |
2 |
27.8 |
7 |
146
|
Fe@Al2O3 |
Mechanosynthesis |
1.4 |
21.4 |
13.3 |
144
|
Spinel Fe3O4@TiO2 microsphere |
Solvothermal, calcination |
2 |
23.3 |
7 |
146
|
3D array Fe3O4@mesoporous C |
Template assisted |
2 |
−57 |
8 |
156
|
Fe@Al2O3/FeOx |
Arc discharge |
2.3 |
38.46 |
6.2 |
147
|
Fe nanoparticles@SnO2 |
Arc discharge |
2 |
−39.2 |
16.8 |
148
|
Sr0.8La0.2Fe11.8Co0.2O19@Fe |
Chemical vapor deposition |
2.8 |
−30 |
8 |
157
|
Fe3O4@Cu-silicate |
Sol–gel |
2 |
−23 |
7 |
147
|
Fe3O4@PEDOT |
Two step |
2 |
−30 |
9.5 |
47
|
Fe3O4@PPy |
Three step |
2 |
−41.9 |
13.3 |
158
|
CeO2@Fe3O4 |
Two step solvothermal |
— |
−28.9 |
15.3 |
151
|
Durian like Fe3O4@TiO2 |
Solvothermal |
2 |
−15.71 |
6.5 |
159
|
Fe nanoflake@SiO2 |
Ball milling |
2.2–3.6 |
−20 |
3.8–7.3 |
145
|
![[thin space (1/6-em)]](https://www.rsc.org/images/entities/char_2009.gif) |
Ternary composites
|
ZnFe2O4@graphene/TiO2 |
Hydrothermal |
2.5 |
55.6 |
3.8 |
160
|
ZnFe2O4@SiO2/RGO |
Three step process |
3.7 |
45.8 |
7.6 |
161
|
Fe3O4@SnO2/RGO |
Three step process |
4.5 |
45.5 |
6.4 |
162
|
HCNT/Fe@Fe2O3 |
Two step process |
1.5 |
−45.8 |
8–9 |
163
|
Fe3O4/BaTiO3/RGO |
Two step solvothermal |
4 |
38.2 |
5 |
164
|
HGS@Fe3O4@RGO |
Ferrite plating method |
2.5 |
15.8 |
11 |
8
|
C@Fe@Fe3O4 |
Template, pyrolysis |
1.5 |
40 |
5.2 |
165
|
Ag@Fe3O4/RGO |
Solvothermal |
2 |
40.05 |
11.9 |
154
|
G/Fe3O4@Fe |
Multi steps process |
2 |
−35.2 |
17–18 |
166
|
Fe/Fe3O4@C |
IP |
3.9 |
29.3 |
12.6 |
167
|
GN–pFe3O4@ZnO |
Three steps process |
5 |
−40 |
11.4 |
142
|
Fe@SiO2/PU |
Surface initiated polymerization |
1.8 |
−21.2 |
11.3 |
51
|
Fe@FeO/PU |
Surface initiated polymerization |
3 |
<−20 |
3.4 |
51
|
CIP@SiO2@Mn0.6Zn0.4Fe2O4 |
Co-precipitation |
2 |
−44.24 |
11.57 |
168
|
Fe3O4@Fe/G |
Two step process |
4.6 |
−58 |
5.2 |
169
|
ZnFe2O4@RGO@CuS |
Two step hydrothermal |
2.2 |
−55.4 |
14.6 |
170
|
![[thin space (1/6-em)]](https://www.rsc.org/images/entities/char_2009.gif) |
Quaternary composites
|
Graphene@Fe3O4@WO3@PANI |
Hydrothermal, chemical oxidation polymerization |
4 |
46.7 |
9.4 |
171
|
G/Fe3O4@Fe/RGO |
Multi steps process |
2.5 |
−32.5 |
14–15 |
166
|
GNP/Fe3O4@BaTiO3/MWCNTs/VMQ |
Solvothermal, solution blending |
2.6 |
−26.7–33.3 |
1–20 |
172
|
G/Fe3O4@C@MnO2 |
Hydrothermal, thermal treatment |
1.8 |
−38.8 |
15 |
173
|
ZnO/Fe/Fe3C/C |
Thermal decomposition, heat treatment |
1.5 |
−30.4 |
14.5 |
174
|
Graphene@Fe3O4@SiO2@PANI |
Three steps process |
2.5 |
−40.7 |
12.5 |
121
|
Dextran/Fe3O4@Fe/RGO |
Solvothermal, hydrothermal |
4 |
−20.26 |
4.72 |
175
|
Graphene@Fe3O4@PANI@TiO2 |
Hydrothermal, IP |
1.6 |
−41 |
14.4 |
176
|
Epoxy–PPy/Fe3O4–ZnO |
Co-precipitation, solution mixing |
2 |
−32.53 |
9.96 |
177
|
Graphene@Fe3O4@PANI@TiO2 |
Hydrothermal, IP |
1.6 |
−41 |
14.4 |
176
|
Polycarbonate/MWCNTs/Fe3O4@C |
In situ hydrothermal |
1 |
−41.3 |
17.7 |
178
|
Graphene@Fe3O4@SiO2@NiO |
Hydrothermal, sol–gel |
1.8 |
−51.5 |
14.6 |
179
|
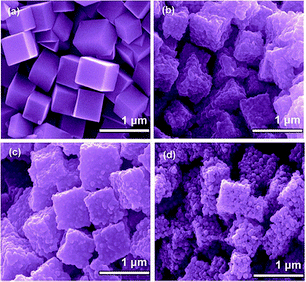 |
| Fig. 15 SEM images of the as-prepared PB nanocubes (a) and Fe/C nanocubes obtained at different pyrolysis temperatures: (b) 600 °C, (c) 650 °C and (d) 700 °C 150 – reproduced by permission of the Royal Society of Chemistry. | |
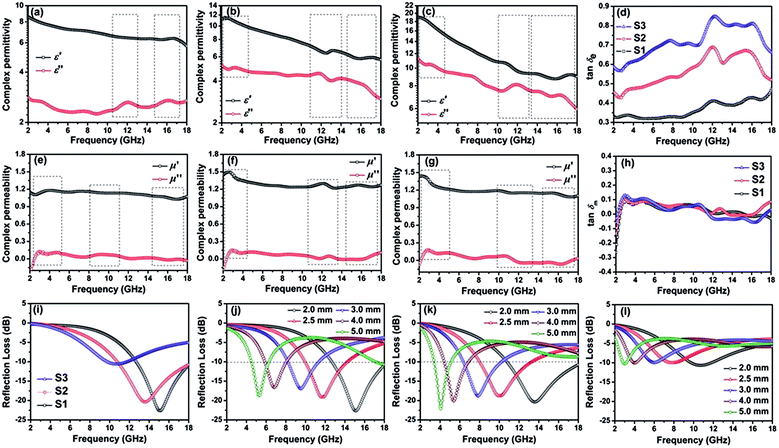 |
| Fig. 16 Complex permittivity of S1 (a), S2 (b), S3 (c), and their dielectric loss tangents (d); complex permeability of S1 (e), S2 (f), S3 (g), and their magnetic loss tangents (h); microwave reflection losses (absorber thickness = 2 mm) of Fe/C nanocubes (i), and reflection losses of S1 (j), S2 (k), S3 (l) with variable absorber thicknesses here S1, S2, and S3 indicate that their final temperature was set to 600 °C, 650 °C, and 700 °C, respectively150 – reproduced by permission of the Royal Society of Chemistry. | |
9.3.1.3 Dielectric core@magnetic shell.
Another way of making a core@shell structure is to take dielectric materials as the core and magnetic materials as the shell. These materials can be chosen as we suggested in previous section. Wang et al.151 produced for the first time such a kind of core@shell structure using CeO2 (core) and Fe3O4 (shell). Their observations indicate that, compared to the magnetic@dielectric core–shell structure (Fe3O4@CeO2), the dielectric shell@magnetic core CeO2/Fe3O4 nanocapsules show improved dielectric properties owing to the increased O-vacancy concentration in the CeO2 cores of the larger grains as well as the O-vacancy-induced enhancement in interfacial polarization between the CeO2 cores and the Fe3O4 shells, respectively.
9.3.2 Ternary composites.
As we have seen in the binary composites section, the proliferation of microwave absorption performance is mainly an outcome of improved impedance matching, but we have neglected the important thing i.e. introduction of magnetic materials decreases the dielectric loss. This is a serious problem which influences the microwave absorption performance. Now, if we desire to preserve high dielectric loss even after inserting further magnetic materials, another high dielectric loss material must be introduced into the absorbing materials. For this purpose, carbonaceous materials such as graphene, CNTs, CFs or polymers like PANI, PPy etc. are preferred due to their attractive properties. In this case, the mixing of a magnetic material with a binary dielectric material not only enhances its attenuation ability, but also conserves the degree of impedance matching. In this direction, several composites such as Ag@Fe3O4/RGO,154 SiC@SiO2@Fe3O4,180 hollow carbon@Fe@Fe3O4 nanospheres165 and many others have been studied so for. Ternary composites can be made either by a core@shell@shell structure, by a combination of three materials or by dispersion of the core@shell structure into a matrix like graphene, PPy or PVDF etc. For example, Chen and co-workers produced a core@shell@shell-type structure from carbonyl iron powder (CIP)@SiO2@Mn0.6Zn0.4Fe2O4 ferrite. The as-prepared (CIP)@SiO2@Mn0.6Zn0.4Fe2O4 composites displayed better dielectric and magnetic loss characteristics at high frequency compared to pure CIP, CIP@SiO2 and CIP@Mn0.6Zn0.4Fe2O4.168 Sun and coworkers181 prepared mesoporous Fe3O4@ZnO sphere decorated graphene (GN-pFe3O4@ZnO) composites with sufficient porosity, uniform size, high magnetization and excellent EM wave absorption properties. They adopted a three-step method to synthesise these composites. The as-prepared pFe3O4 nanoparticles have a mean diameter of 200 nm (Fig. 17a and b), but coating the ZnO layer increases the diameter of the pFe3O4@ZnO spheres (Fig. 17c and d). Fig. 17e and f represent the TEM image of pFe3O4@ZnO sphere decorated by graphene (GN). It is evident that each GN sheet is well anchored by pFe3O4@ZnO spheres and no individual pFe3O4@ZnO sphere can be observed outside of the GN sheet. The EM wave absorption properties of the GN-pFe3O4@ZnO composites were studied in the thickness range of 1–5 mm. A stronger RL peak is found at high-frequency and also at the normal RL peak. With increasing thickness, both peaks shift from the high frequency to the low-frequency side and show a decreasing minimal RL value (Fig. 18). The minimal RL of the GN-pF3O4@ZnO composite was almost −40 dB, with an absorption bandwidth corresponding to RL < −10 dB at 11.4 GHz frequency.
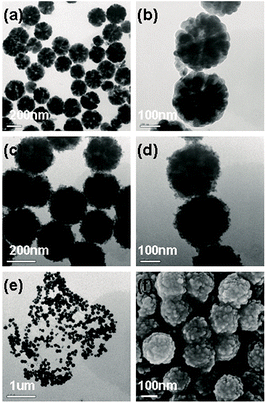 |
| Fig. 17 TEM images of the products at different stages (a and b) pFe3O4, (c and d) pFe3O4@ZnO, (e) GNpFe3O4@ZnO, and (f) corresponding FE-SEM image181 – reproduced by permission of the Royal Society of Chemistry. | |
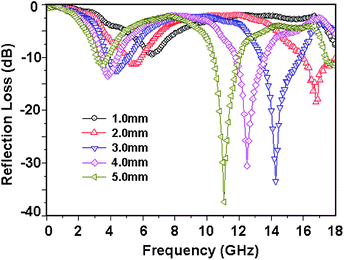 |
| Fig. 18 Reflection loss of GNpFe3O4@ZnO composites with thickness 1–5 mm 181 – reproduced by permission of the Royal Society of Chemistry. | |
9.3.3 Quaternary composites.
Nowadays researchers have focused on the synthesis of quaternary composites. The benefit of quaternary composites over ternary composites is the presence of multiple interfaces, as we know that interfacial polarization plays a crucial role in EMI-preventing materials. Therefore, multiple interfaces in heterogeneous quaternary composites not only enhance the dielectric loss due to interfacial and space polarization but also promote multiple reflection owing to their complicated morphologies. These can be synthesized either in core@shell@shell hetero nanostructures distributed on surface of substrates like graphite, graphene, CNTs, PANI etc. or by combinations of different materials. Wang and coworkers121 fabricated core@shell@shell/substrate type Fe3O4@SiO2@polyaniline hetero nanostructures wrapped with a graphene substrate. According to them, the presence of triple-interfaces and junctions in the Fe3O4@SiO2@polyaniline/graphene composites increases the interfacial polarization. Meanwhile, active sites present in PANI and the void space between Fe3O4 and PANI led to a somewhat large specific surface area which increases the reflection of EM waves. The above-moentioned void spaces are effective in terms of restricting the spreading of EM waves and produce heating because of the impendence dissimilarity, boosting the microwave absorption properties. Although quaternary composites nevertheless have several benefits, the synthesis of quaternary core–shell is quite complicated and hence they have been the subject of few studies.
9.4 Fe species with 1D, 2D and 3D carbon materials
The third strategy is to assemble magnetic Fe-related nanomaterials onto one-dimensional 1D and 2D carbon materials like CNTs, CNFs, 2D graphene or 3D graphene sheets, or graphene capsules to form a hybrid structure. Carbonaceous materials are a high-dielectric material. However, these materials cannot be used alone due to their limitations in terms of impedance matching with the absorber matrix. Thus the combination with magnetic materials is beneficial to improve the microwave absorption, due to their adjustable dielectric and magnetic properties. He et al. observed superior performance in flaky carbonyl iron (FCI) coated with RGO nanosheets. In fact, FCI/RGO is a typical example of the dielectric dispersion behaviour of complex permittivity in which the contribution of RGO as a dielectric lossy material confers FCI with advanced dielectric loss and magnetic loss abilities.66 Hu et al.182 have shown the remarkable microwave absorption properties of 3D reduced graphene oxide and single-crystalline Fe3O4. In 3D graphene/Fe3O4 composites, graphene confers a big contact surface for the homogeneous distribution of Fe3O4 particles because 3D graphene has a large surface area compared to 2D graphene. Therefore, 3D graphene acts as an ideal substrate for the absorption of microwaves. This improves both the dielectric loss and magnetic loss, hence the improved absorption characteristics for the 3D graphene/Fe3O4 composites in comparison to those of the 3D graphene and Fe3O4. On other hand, to explore the probable attenuation process in trilayer MnO2@Fe-graphene composites, an antenna mechanism of the rod-like structure was proposed by Lv and coworkers. According to them, EM energy transfers in the form of a microcurrent in the rod-like structures. Furthermore, addition of Fe with MnO2 increases impedance matching so that MnO2@Fe composite converts more-and-more EM energy into the microcurrent. This microwave current is now expected to transmit from one rod to another. In these condition, the graphene works as an electrically conductive network path, since electrical energy is attenuated due to the resistance of the graphene. Meanwhile, dual interfaces e.g. Fe–C and MnO2–Fe also lead to electron polarization, carrying through the attenuation EM wave.5 Many examples of ternary (e.g. PANI/graphene@Fe3O4), quaternary (e.g. graphene@Fe3O4@WO3@PANI) and even quinary composites (e.g. methyl vinyl silicone rubber (VMQ)-graphene nanoplate/Fe3O4@BaTiO3/MWCNTs) can be seen on graphene, which is one of the most important substituents.171,172 To illustrate this, we shall take the example of the quaternary composite Fe3O4@BaTiO3/MWCNTs. In this composite, firstly the presence of multi-interfaces causes the enhancement of interfacial polarization. Secondly, the layering of PANI on the graphene sheet is attributed to electron tunnelling and the development of electronic clouds which are responsible for converting EM energy into heat energy. It is a well-known fact that EM wave absorption properties depend significantly on the microstructure of the absorbers. Once the EM wave strikes the absorbers, the sandwich multilayer structure can efficiently enhance multiple reflection and offer a maximum absorption of the EM wave and a minimum reflection. In addition, the upturn in absorption also causes better impedance matching and a synergistic effect. This accounts for the superior absorption properties of graphene@Fe3O4@WO3@PANI composites. Some popular examples of multi-functional carbon materials with Fe components are listed in Table 4.
Table 4 Fe component/carbon based microwave absorber
Carbonaceous based Fe composites |
Materials |
Method |
Thickness/mm |
RLmin/dB |
Frequency/GHz |
Ref. |
CNT/Fe3O4/RGO |
Solvothermal, ultrasonic method |
2.5 |
−50.0 |
8.7 |
30
|
RGO/Fe3O4 |
Hummer, solvothermal |
2 |
−27 |
5.4 |
183
|
Bowl like Fe3O4/RGO |
Solvothermal |
2 |
−24 |
12.9 |
184
|
Fe3O4/RGO composites |
One-pot co-precipitation |
3.9 |
−44.6 |
6.6 |
185
|
NiFe2O4 nanorod/graphene |
One-step hydrothermal |
2 |
−29.2 |
16.1 |
186
|
Popcorn likeα-Fe2O3/3D G |
Template assisted, co-precipitation |
1.4 |
−55.7 |
17.3 |
42
|
α-Fe2O3 nanorod/graphene |
Chemical reduction |
2 |
−45 |
12.8 |
187
|
Flaky CI/RGO |
Modified Hummer, reflux |
3.87 |
−64.4 |
5.2 |
66
|
RGO/α-Fe2O3 hydrogel |
Two-step hydrothermal |
3 |
−33.5 |
0.712 |
44
|
RGO/spherical CI |
Wet chemical method |
3 |
−52.46 |
7.79–11.98 |
188
|
RGO/NiFe2O4 |
One-step hydrothermal |
3.0 |
−39.7 |
9.2 |
189
|
RGO/CoFe2O4ZnS |
Hydrothermal, co-precipitation |
1.8 |
−43.2 |
10.2–15.7 |
190
|
RGO/CoFe2O4SnO2 |
Two steps hydrothermal |
1.6 |
−54.4 |
16.5 |
191
|
Fe3O4//graphene |
Two steps |
1.5 |
−29 |
8–12 |
81
|
α-Fe2O3/γ-Fe2O3/RGO |
Thermochemical reactions |
4.0 |
−13.6 |
3.76 |
43
|
ZnFe2O4@graphene@TiO2 |
Hydrothermal |
2.5 |
−55.6 |
3.8 |
160
|
TiO2/RGO/Fe2O3 |
Hydrothermal |
2.0 |
−44.0 |
14.8 |
192
|
RGO/SiO2/Fe3O4 |
Two steps reaction |
4.5 |
−56.4 |
8.1 |
193
|
RGO/ZnFe2O4 |
Solvothermal |
2.5 |
−41.1 |
9.4 |
194
|
Co0.5Ni0.5Fe3O4/RGO |
Chemical reduction |
2.5 |
−13.1 |
14.8 |
195
|
Ni0.8Zn0.2Ce0.06Fe1.94O4/GNS |
Sol–gel deoxidation technique |
— |
−37.4 |
12.3 |
196
|
RGO/BaFe12O19/Fe3O4 |
Two step hydrothermal |
1.8 |
−46.04 |
15.6 |
197
|
RGO@Fe3O4 |
Two step process |
2.0 |
−56.25 |
12.62 |
198
|
Porous Fe3O4/C |
Hydrothermal process |
3 |
−31.75 |
7.76–12.88 |
199
|
Fe–C nanocapsules |
Arc-discharge method |
3.1 |
−43.1 |
9.6 |
200
|
Fe3O4C yolk–shell |
In situ reduction process |
3 |
−45.8 |
10.6 |
152
|
Fe3O4 microsphere@C |
In situ polymerization |
2 |
−20.6 |
13.4 |
23
|
CI/C |
Hydrothermal |
1.3 |
−46.69 |
11.5 |
201
|
Fe3O4 graphite |
Molten salt route, temperature reduction |
4.8 |
−51 |
4.3 |
202
|
EG/Fe3O4 |
Solvothermal, sintering route |
2.6 |
−24.8 |
6.8 |
203
|
EG/Fe/Fe3O4 |
Chemical vapor deposition |
1.9 |
−42.4 |
9.36 |
204
|
Epoxy graphitized nanosheet@Fe3O4–MnO2 |
Hydrothermal |
4.5 |
−31.7 |
5.85 |
205
|
Flower like Fe3O4/MWCNTs |
Acid treatment, hydrothermal |
0.9 |
−64* |
18 |
206
|
CNTs/Fe3O4/RGO |
Solvothermal, ultrasonic method |
2.5 |
−50.0 |
8.7 |
30
|
CNTs/CoFe2O4 |
Chemical vapor deposition |
— |
18 |
9 |
3
|
MWCNTs/Fe2O4 |
In situ growth method |
1.5 |
−30 |
5.7 |
207
|
MWCNT/NiO–Fe2O4 |
Electroless plating |
2 |
−55 |
3.5–18 |
208
|
Fe2O3/Fe3O4/MWCNTs |
Hydrothermal |
2.5 |
44.1 |
10.4 |
209
|
Fe-MWCNT |
Chemical method |
4.27 |
−39 |
2.7 |
210
|
Fe3O4/CF |
Electrophoretic deposition |
1.7 |
−11 |
10.37–11.4 |
211
|
9.5 Fe ingredients with polymers
In general, different methods have been adopted to coat metal films onto a substrate (another material) for shielding purposes. Nonetheless the poor scratch resistance and enrobing weaken their application to a certain extent. In this aspect, an electrically conducting polymer matrix either with a magnetic filler or without a filler can be a good alternative to metals. It is important that the crucial loss mechanisms in non-magnetic materials (like carbon materials and conductive polymers) are the dielectric (dipolar) and conduction losses. Conduction losses typically dominate in high conductivity materials and dipolar losses dominate in poor conductivity materials. It was seen that pure conducting polymers weakly absorb the EM wave, as Zhang et al. observed for pure PANI, in which RLmin reached only −18 dB at 13.8 GHz with a thickness of 2 mm, which shows PANI weakly absorb EM waves; whereas Sui et al. have reported a similar result obtained for PPy, which possesses an RLmax of 16.7 dB at 17.6 GHz. Another conducting polymer, pure PEDOT, also has weak attenuation towards EM waves, and has an RLmax of only −14.5 dB at 7 GHz, as investigated by Zhang et al.260–263 On other hand, magnetic materials show magnetic losses such as hysteresis, electron spin resonance and domain wall resonance etc. Additionally, magnetic materials with relatively higher electrical conductivity exhibit great conduction losses. Hence, effective EMI shielding can be achieved when materials exhibit both magnetic and dielectric loss processes together. In this context, ferrites and iron oxides are extensively used for the improvement of the electrical as well magnetic properties of conductive polymers. Liu and coworkers219 made barium hexa-ferrite (BaFe12O19)@PANI core@shell nano-composites. Addition of BaFe12O19 benefits the conductance loss and magnetic resonance loss as well interfacial loss. Moreover, tuning the shell thickness provides us with optimal impedance matching. As can be seen in BaFe12O19@PANI composites, the maximum absorption loss was 28 dB at 12.8 GHz with an absorption bandwidth of 3.8 GHz and a thickness of 2.0 mm (30–40 nm thick shell). Moreover, tuning the mole ratio of the doped acid to the monomer may give a non-magnetic state (NM). This NM state weakens the dielectric loss and magnetic loss simultaneously, but raises the impedance matching and establishes the complementary behaviour between the dielectric loss and the magnetic loss, and strengthens the absorbing properties of the composites. Fan and coworkers investigated the effect of different acid [p-toluenesulfonic acid]/[aniline] ratios ([p-TSA]/[ANI] = 0.005/1, 0.05/1, 0.2/1) on the microwave properties of PANI/CIP composites.264 Among all the ratios, 0.2/1 shows the best reflection loss (>−20 dB) due to the appearance of a nonmagnetic state owing to the CIP size (microspheres). On other hand, the core–shell structure of CIP@PANI composites suppresses saturation magnetization, weakening the magnetic loss and dielectric loss, but enhancing impedance matching. Nowadays, carbon materials are also being used with conducting polymer and iron materials because the carbon material not only serves as superior substrate but also improves the mechanical and thermal characteristic of these composites. Besides, carbon materials facilitate the hopping of charge carriers, enhance multiple reflection and increase the interfacial polarization. Considering all these points, Wang et al. for the first time fabricated FeCo@SnO2@graphene@PANI quaternary composites with three SnO2, graphene and PANI dielectric loss absorbers, while the FeCo particles serve as magnetic loss absorbers. A maximum reflection loss was reached of −39.8 dB at 6.4 GHz with a thickness of 3 mm due to the high specific surface area and the presence of residual defects and organic groups of RGO that act as polarized centers, increasing the polarization relaxation process and multiple reflections. Moreover, hopping charge carriers enhance the eddy current loss between PANI and graphene which converts electrical energy into heat energy. This was despite the fact that multi-interfaces between FeCo, SnO2, PANI and graphene work as polarization centers and create dipole and interfacial polarization of the composites owing to the synergistic effects of different types of material.124 Some of the studied polymer composites are listed in Table 5. Therefore, Fe materials are widely used with these polymers. But then again, these polymers suffer limitations because of their poor processibility and mechanical properties. Therefore, rubber polymer composites containing Fe-based fillers have also been examined as effective EMI shielding materials because of their unique combination of polymeric flexibility, electrical conductivity and magnetic properties, as reported by Nasouri and coworkers.258 This group used Fe3O4 as nanofiller in a polyvinylpyrrolidone (PVP) matrix. It was found that with increasing Fe3O4 nanoparticle concentration EMI shielding efficiency increases up to 22 dB (4% of Fe3O4 filler) in which absorption was the major shielding mechanism. Similarly, Al-Ghamdi et al. used Fe3O4 as filler in an NBR matrix257 and obtained an 80–90 dB SET at 40% of Fe3O4 filler. For moderate conductivity Fe materials, such as Fe3O4, a large quantity of nanofiller is required to reach the threshold value to connect the conducting path in insulating matrices. Thus, to enhance the further electrical conductivity, the concept of double percolation can be adopted by means of using RGO, CNTs, black carbon etc. as reported by Pawar et al.259 in MWCNT/Fe3O4 within PC (polycarbonate)/SAN [poly(styrene-co-acrylonitrile)] blend composites, in which an SET of around −32.5* dB at 18 GHz was observed for 3 wt%-MWCNT and 3 vol%-Fe3O4 in 60/40 PC/SAN blends. In this case, absorption occurs due to synergy between the MWCNTs and Fe3O4 nanoparticles in which the MWCNTs absorb the electrical field while the dopamine-anchored Fe3O4 absorbs the magnetic field of the EM radiation, resulting in improved EMI shielding.
Table 5 Polymer and Fe materials based microwave absorber
Polymer based composites |
Materials |
Methods |
Thickness/mm |
 /dB |
Frequency/GHz |
Ref. |
PANI@Fe3O4 hybrid |
Solvothermal, IP |
4.5 |
29.3 |
7 |
212
|
PANI/Fe3O4 |
In situ polymerization (IP) |
0.5 |
−54 |
33.72 |
213
|
Hollow PANI/Fe3O4 |
Three steps process |
2 |
−10 |
9–10 |
214
|
Fe3O4@PANI NPs |
Oxidation reduction, IP |
1.7 |
35.1 |
16.7 |
215
|
Fe3O4@PANI MS |
IP |
2 |
−37.4 |
15.4 |
216
|
HPAP/Fe3O4 |
Co-precipitation, in situ EP |
2 |
−3 |
9–10 |
217
|
PANI/BaFe12O19 |
IP |
2 |
−12 |
32.3 |
218
|
BaFe12O19@PANI |
Sol–gel, auto-combustion, IP |
2 |
−28 |
12.8 |
219
|
PANI/MWCNTs/Fe3O4 |
Co-precipitation, IP |
4 |
16 |
8–15 |
220
|
PANI@nano-Fe3O4@CFs |
Three steps process |
1.5 |
−11.11 |
8.1–18 |
221
|
PANI@graphene@Fe3O4 |
Hydrothermal, IP |
3 |
−43.7 |
10.7 |
222
|
PANI/Zn0.6Cu0.4Cr0.5Fe1.46Sm0.04O4 |
Rheological phase reaction, IP |
2 |
−22.46 |
2–18 |
223
|
PANI/CIP/Fe3O4 |
Co-precipitation, in situ EP |
1.76 |
48.3 |
9.6 |
224
|
PANI/CuI/Fe3O4 |
Mechanical mixing |
2.8 |
35.3 |
13.28 |
225
|
PANI/PPy/Fe3O4 |
Three steps |
2 |
−47.3 |
13.45 |
226
|
PANI/Ag/SrFe12O19 |
Three steps |
3 |
−14.86 |
9.98 |
227
|
PANI/MnFe3O4 |
Three steps |
1.4 |
−15.3 |
10.4 |
228
|
Graphene@Fe3O4@SiO2@PANI |
Stöber method, IP |
2.5 |
−40.7 |
12.5 |
121
|
PANI–BF0.25 |
Surfactant assisted solvothermal, IP |
2.5 |
−45.2 |
11.2 |
229
|
CuS/RGO/PANI/Fe3O4 |
Hydrothermal, IP |
2.5 |
−69.2 |
10.2 |
222
|
Fe3O4@PPy microspheres |
Chemical oxidative polymerization |
2.5 |
−31.5 |
15.5 |
230
|
PPy/Fe3O4/PVDF |
Chemical vapor deposition, IP |
2.5 |
−21.5 |
16.8 |
54
|
PPy/Zn0.6Cu0.4Cr0.5Fe1.46Sm0.04O4 |
Rheological phase reaction, IP |
2 |
−20.9 |
14.05 |
223
|
PPy–γ-Fe2O3 fly ash |
In situ emulsion polymerization (EP) |
2 |
25.5* |
12.4–18 |
223
|
Fe3O4@SiO2@PPy |
Microemulsion polymerization |
5 |
40.9 |
6 |
231
|
γ-Fe2O3/(SiO2)xSO3H/polypyrrole |
Solgel, IP |
4 |
43.1 |
15.1 |
46
|
γ-Fe2O3–SiO2–PEDOT |
Two step reaction |
2 |
−27.5 |
13.8 |
232
|
Hollow γ-Fe2O3@SiO2@PEDOT |
Two step reaction |
2 |
−21.3 |
14.1 |
233
|
Fe3O4/PEDOT |
Mechanical mixing |
4 |
−15.8 |
3.2 |
234
|
BaFe12O19/PEDOT |
In situ EP |
— |
24.5* |
15 |
235
|
γ-Fe2O3@PEDOT |
Two step reaction |
2 |
−44.7 |
12.9 |
233
|
Fe3O4–RGO/PIL–PEDOT |
Poly(ionic liquid) mediated hybridization |
0.01 |
22* |
0.02–1 |
236
|
Fe3O4–PEDOT nanospindles |
Oxidative molecular layer deposition |
1.4 |
−55 |
16.2 |
237
|
PEDOT:PSS/Fe3O4 |
Reflux mixing |
— |
40* |
8–12.5 |
238
|
Fe3O4/C/PVDF |
Wet chemical method, heat treatment |
2.1 |
−38.8 |
11–12 |
239
|
PVDF/Fe3O4/CNT |
Twin screw compounding method |
0.7 |
28.8 |
5.6 |
240
|
PVDF/Fe3O4–PANI/SWCNH |
IP, solution blending |
2 |
−29.7* |
14.5–20 |
241
|
Fe3O4/PVDF/PPy |
Chemical vapour deposition, IP |
2.5 |
−21.5 |
16.8 |
54
|
PVDF/PS/HDPE/MWCNT/Fe3O4 |
Melt blending |
— |
25* |
9.5 |
242
|
Flake shaped CI/RGO/PVP |
Chemical reduction |
1.5 |
−130.3 |
16.88 |
52
|
Fe3O4/PEI |
Co-precipitation |
2.4 |
−30.69 |
7.24 |
243
|
PS/graphene/Fe3O4 |
Solution blending |
— |
30* |
9.8–12 |
244
|
PVA–GAPC–Fe3O4 |
Solution casting method |
0.3 |
15* |
8.3–12.4 |
245
|
FePc–Fe3O4–basalt fiber |
Solvothermal |
5 |
−31.1 |
5.9 |
246
|
Flake carbonyl-Fe/MAA/PS |
Dispersion polymerization |
2.5 |
−39 |
3.3 |
247
|
Ni0.5Zn0.5–Fe2O4/PU |
Mixing method |
3 |
−2.8 |
15–16 |
248
|
CoFe2O4/paraffin |
Surfactant-assisted hydrothermal |
2 |
−40 |
10.7 |
249
|
CoFe2O4/epoxy |
Surfactant-assisted hydrothermal |
2 |
−59.8 |
11.86 |
249
|
EVA/polycrystalline iron fibers |
Mechanical method |
2.0 |
23.7 |
7.2 |
250
|
CI/polyurethane |
Mechanical mixing |
3 |
−25.2 |
6.64 |
251
|
CI/epoxy-silicone |
Mechanical mixing |
2.5 |
−40 |
6.2 |
252
|
Flaked shape CI/RGO-epoxy |
Ball milling |
2 |
−32.3 |
11 |
253
|
CI/Ethylene propylene diene monomer rubber (EPDM) |
Thermal decomposition, two roller mixer |
3 |
−21.7 |
3.5 |
59
|
Epoxy/silicon/GNS/flake CI |
Multi-step process |
1.2 |
−8 |
6.6–18 |
254
|
Fe/silicone rubber |
Mixing process |
1 |
−5 |
1–2 |
255
|
Polydimethylsiloxane (PDMS)/CNFs/Fe3O4 |
Solution-casting |
5.5 |
44 |
15.75 |
256
|
Nitrile butadiene rubber (NBR)/Fe3O4 |
Hydrothermal, two roller mixing |
2 |
80–90* |
1–12 |
257
|
Polyvinyl pyrrolidone (PVP)/Fe3O4 |
Two step process |
1 |
22* |
8.2–12.4 |
258
|
Polyvinyl chloride (PVC) graphene/Fe3O4 |
Co-precipitation, heating |
1.8 |
13* |
8–12 |
61
|
MWCNT/g-Fe3O4 polycarbonate/poly (styrene-co-acrylonitrile) |
Three step process |
— |
32* |
18 |
259
|
10 Conclusions
In brief, iron (Fe) and its oxide materials are very useful from an applications points of view in the fields of energy, medical, research and many others. In this review paper, we explored composites comprising carbonaceous, polymer and dielectric materials with iron components as important constituents for the prevention of electromagnetic interference (EMI) by reflection as well as by absorption. Two losses, dielectric and magnetic, are responsible for high microwave absorption and the total shielding performance. In this context, iron and its components can be versatile choice for EMI shielding applications in combination with conductive polymers and carbon materials etc. which integrate with its EMI efficiency.
Conflicts of interest
There are no conflicts to declare.
Acknowledgements
V. Shukla acknowledges the Indian Institute of Technology Kharagpur and MHRD, India for providing the financial assistance to carry out this research work. V. Shukla is also thankful to her supervisor Dr S. K. Srivastava for providing a conducive environment to write this manuscript.
References
- X. Jian, B. Wu, Y. Wei, S. X. Dou, X. Wang, W. He and N. Mahmood, ACS Appl. Mater. Interfaces, 2016, 8, 6101–6109 CrossRef CAS PubMed
.
- S. R. Dhakate, K. M. Subhedar and B. P. Singh, RSC Adv., 2015, 5, 43036–43057 RSC
.
- R. Che, C. Zhi, C. Liang and X. Zhou, Appl. Phys. Lett., 2006, 88, 033105 CrossRef
.
- P. R. Agarwal, R. Kumar, S. Kumari and S. R. Dhakate, RSC Adv., 2016, 6, 100713–100722 RSC
.
- H. Lv, G. Ji, X. Liang, H. Zhang and Y. Du, J. Mater. Chem. C, 2015, 3, 5056–5064 RSC
.
- A. Kumar, A. P. Singh, S. Kumari, A. Srivastava, S. Bathula, S. Dhawan, P. Dutta and A. Dhar, J. Mater. Chem. A, 2015, 3, 13986–13993 RSC
.
-
M. C. Ribadeneyra, J. P. de Diego and M. G. González, PhD thesis, Universidad Carlos III de Madrid, 2014
.
- J. Wang, J. Wang, B. Zhang, Y. Sun, W. Chen and T. Wang, J. Magn. Magn. Mater., 2016, 401, 209–216 CrossRef CAS
.
- F. Meng, H. Wang, F. Huang, Y. Guo, Z. Wang, D. Hui and Z. Zhou, Composites, Part B, 2018, 137, 260–277 CrossRef CAS
.
- J. Liu, W.-Q. Cao, H.-B. Jin, J. Yuan, D.-Q. Zhang and M.-S. Cao, J. Mater. Chem. C, 2015, 3, 4670–4677 RSC
.
- G. G. Bush, J. Appl. Phys., 1988, 63, 3765–3767 CrossRef CAS
.
- T. Nakamura, J. Appl. Phys., 2000, 88, 348–353 CrossRef CAS
.
- O. Acher and S. Dubourg, Phys. Rev. B: Condens. Matter Mater. Phys., 2008, 77, 104440 CrossRef
.
- D. L. Leslie-Pelecky and R. D. Rieke, Chem. Mater., 1996, 8, 1770–1783 CrossRef CAS
.
- X. Zhang, P. Guan and X. Dong, Appl. Phys. Lett., 2010, 97, 033107 CrossRef
.
- R. Dosoudil, M. Usakova, J. Franek, J. Slama and A. Gruskova, IEEE Trans. Magn., 2010, 46, 436–439 CAS
.
- A. Habib, S. Xu, E. Walker, M. Ondeck, R. Swaminathan and M. McHenry, J. Appl. Phys., 2012, 111, 07B305 CrossRef
.
- R. Kodama, J. Magn. Magn. Mater., 1999, 200, 359–372 CrossRef CAS
.
- Y. Liu, S. Wei, B. Xu, Y. Wang, H. Tian and H. Tong, J. Magn. Magn. Mater., 2014, 349, 57–62 CrossRef CAS
.
- H. Lv, G. Ji, M. Wang, C. Shang, H. Zhang and Y. Du, RSC Adv., 2014, 4, 57529–57533 RSC
.
-
P. Saini and M. Arora, in New Polymers for Special Applications, InTech, 2012 Search PubMed
.
- Y. Li, M. Yu, P. Yang and J. Fu, Ind. Eng. Chem. Res., 2017, 56, 8872–8879 CrossRef CAS
.
- Y. Du, W. Liu, R. Qiang, Y. Wang, X. Han, J. Ma and P. Xu, ACS Appl. Mater. Interfaces, 2014, 6, 12997–13006 CrossRef CAS PubMed
.
- A. F. Ahmad, Z. Abbas, S. J. Obaiys, N. Ibrahim, M. Hashim and H. Khaleel, PLoS One, 2015, 10, e0140505 CrossRef PubMed
.
-
Z. Ma, C.-T. Cao, Q.-F. Liu and J.-B. Wang, arXiv preprint arXiv:1110.3184, 2011.
- D. L. Huber, Small, 2005, 1, 482–501 CrossRef CAS PubMed
.
- H. Ping, K. Lu, Y. Jun, Y. Fan, W. Kuaishe, D. Jinjing, Y. Zhanlin, C. Weicheng and L. Dongxin, Rare Met. Mater. Eng., 2016, 45, 3112–3114 CrossRef
.
- X. T. Chu, B. N. Ta, L. T. Ngo, M. H. Do, P. X. Nguyen and D. N. Nam, J. Electron. Mater., 2016, 45, 2311–2315 CrossRef CAS
.
-
G. Zeng, H. Zhang, Y. Chen, et al., Electromagnetic Compatibility (APEMC), 2012 Asia-Pacific Symposium, 2012, pp. 533–536 Search PubMed
.
- R. C. Che, L.-M. Peng, X. F. Duan, Q. Chen and X. Liang, Adv. Mater., 2004, 16, 401–405 CrossRef CAS
.
- M. Chirita, I. Grozescu, L. Taubert, H. Radulescu, E. Princz, É. Stefanovits-Bányai, C. Caramalau, L. Bulgariu, M. Macoveanu and C. Muntean, Chem. Bull., 2009, 54, 1–8 Search PubMed
.
- H. Bae, T. Ahmad, I. Rhee, Y. Chang, S.-U. Jin and S. Hong, Nanoscale Res. Lett., 2012, 7, 44 CrossRef PubMed
.
- M. N. Akhtar, M. Saleem and M. A. Khan, J. Phys. Chem. Solids, 2018, 123, 260–265 CrossRef CAS
.
- A. Kumar, J. Shen, H. Zhao, Q. Zhengjian and Q. Li, J. Cryst. Growth, 2018, 490, 1–5 CrossRef CAS
.
- S. Kumar, D. P. Dubey, S. Shannigrahi and R. Chatterjee, J. Alloys Compd., 2019, 774, 52–60 CrossRef CAS
.
- Y. Song, J. Zheng, M. Sun and S. Zhao, J. Mater. Sci.: Mater. Electron., 2016, 27, 4131–4138 CrossRef CAS
.
- A. Farhadizadeh, S. S. Ebrahimi and S. Masoudpanah, J. Supercond. Novel Magn., 2016, 29, 1273–1278 CrossRef CAS
.
- G. R. Gordani, M. Mohseni, A. Ghasemi and S. R. Hosseini, Mater. Res. Bull., 2016, 76, 187–194 CrossRef CAS
.
- D. Guo, W. Kong, J. Feng, X. Li and X. Fan, J. Alloys Compd., 2018, 751, 80–85 CrossRef CAS
.
- G. Sun, B. Dong, M. Cao, B. Wei and C. Hu, Chem. Mater., 2011, 23, 1587–1593 CrossRef CAS
.
- Z. Yang, M. Li, Y. Zhang, X. Lyu and D. Hu, J. Alloys Compd., 2019, 781, 321–329 CrossRef CAS
.
- S. Yang, D.-W. Xu, P. Chen, H.-F. Qiu and X. Guo, J. Mater. Sci.: Mater. Electron., 2018, 29, 19443–19453 CrossRef CAS
.
- L. Zhang, X. Yu, H. Hu, Y. Li, M. Wu, Z. Wang, G. Li, Z. Sun and C. Chen, Sci. Rep., 2015, 5, 9298 CrossRef CAS PubMed
.
- H. Zhang, A. Xie, C. Wang, H. Wang, Y. Shen and X. Tian, J. Mater. Chem. A, 2013, 1, 8547–8552 RSC
.
- H. Lv, X. Liang, Y. Cheng, H. Zhang, D. Tang, B. Zhang, G. Ji and Y. Du, ACS Appl. Mater. Interfaces, 2015, 7, 4744–4750 CrossRef CAS PubMed
.
- C. Li, S. Ji, X. Jiang, G. I. Waterhouse, Z. Zhang and L. Yu, J. Mater. Sci., 2018, 1–15 Search PubMed
.
- W. Zhou, X. Hu, X. Bai, S. Zhou, C. Sun, J. Yan and P. Chen, ACS Appl. Mater. Interfaces, 2011, 3, 3839–3845 CrossRef CAS PubMed
.
- H. El Ghandoor, H. Zidan, M. M. Khalil and M. Ismail, Int. J. Electrochem. Sci., 2012, 7, 5734–5745 CAS
.
- A. K. Singh, O. Srivastava and K. Singh, Nanoscale Res. Lett., 2017, 12, 298 CrossRef PubMed
.
- P. Liu, W. Cai and H. Zeng, J. Phys. Chem. C, 2008, 112, 3261–3266 CrossRef CAS
.
- J. Zhu, S. Wei, N. Haldolaarachchige, D. P. Young and Z. Guo, J. Phys. Chem. C, 2011, 115, 15304–15310 CrossRef CAS
.
- X. Weng, B. Li, Y. Zhang, X. Lv and G. Gu, J. Alloys Compd., 2017, 695, 508–519 CrossRef CAS
.
- M. Shahadat, M. Z. Khan, P. F. Rupani, A. Embrandiri, S. Sultana, S. Z. Ahammad, S. W. Ali and T. Sreekrishnan, Adv. Colloid Interface Sci., 2017, 249, 2–16 CrossRef CAS PubMed
.
- Y. Li, Y. Zhao, X. Lu, Y. Zhu and L. Jiang, Nano Res., 2016, 9, 2034–2045 CrossRef CAS
.
- R. Kumar, S. K. Ha, K. Verma and S. K. Tiwari, J. Sci.: Adv. Mater. Devices, 2018, 3, 263–288 Search PubMed
.
- S. Varshney, A. Ohlan, V. K. Jain, V. P. Dutta and S. K. Dhawan, Ind. Eng. Chem. Res., 2014, 53, 14282–14290 CrossRef CAS
.
- S. Kamat, S. Tamboli, V. Puri, R. Puri, J. Yadav and O. S. Joo, J. Optoelectron. Adv. Mater., 2010, 12, 2301–2305 CAS
.
- J. Zheng, H. Lv, X. Lin, G. Ji, X. Li and Y. Du, J. Alloys Compd., 2014, 589, 174–181 CrossRef CAS
.
- Y.-B. Feng, T. Qiu, C.-Y. Shen and X.-Y. Li, IEEE Trans. Magn., 2006, 42, 363–368 CAS
.
- Y. Akinay, F. Hayat, M. Çakir and E. Akin, Polym. Compos., 2018, 39, 3418–3423 CrossRef CAS
.
- K. Yao, J. Gong, N. Tian, Y. Lin, X. Wen, Z. Jiang, H. Na and T. Tang, RSC Adv., 2015, 5, 31910–31919 RSC
.
- S. S. Chauhan, M. Abraham and V. Choudhary, RSC Adv., 2016, 6, 113781–113790 RSC
.
- A. Samadi, S. M. Hosseini and M. Mohseni, Org. Electron., 2018, 59, 149–155 CrossRef CAS
.
- A. Ahmad, S. Ab Aziz, Z. Abbas, S. Obaiys, A. Khamis, I. Hussain and M. Zaid, Polymers, 2018, 10, 1180 CrossRef PubMed
.
- C. Wang, X. Han, P. Xu, X. Zhang, Y. Du, S. Hu, J. Wang and X. Wang, Appl. Phys. Lett., 2011, 98, 072906 CrossRef
.
- L. He, Y. Zhao, L. Xing, P. Liu, Z. Wang, Y. Zhang, Y. Wang and Y. Du, RSC Adv., 2018, 8, 2971–2977 RSC
.
-
M. S. Dresselhaus and P. Avouris, in Carbon Nanotubes, Springer, 2001, pp. 1–9 Search PubMed
.
-
G. Rius, in Carbon for Sensing Devices, Springer, 2015, pp. 15–42 Search PubMed
.
- Z. Liu, G. Bai, Y. Huang, F. Li, Y. Ma, T. Guo, X. He, X. Lin, H. Gao and Y. Chen, J. Phys. Chem. C, 2007, 111, 13696–13700 CrossRef CAS
.
- B. Singh, D. Saket, A. Singh, S. Pati, T. Gupta, V. Singh, S. Dhakate, S. Dhawan, R. Kotnala and R. Mathur, J. Mater. Chem. A, 2015, 3, 13203–13209 RSC
.
- R. Kuchi, H. M. Nguyen, V. Dongquoc, P. C. Van, S. Surabhi, S.-G. Yoon, D. Kim and J.-R. Jeong, Phys. Status Solidi A, 2018, 215, 1700989 CrossRef
.
-
K. V. Mantena, Electrical and mechanical properties of MWCNT filled conductive adhesives on lead free surface finished PCB's, Master's Thesis, University of Kentucky, 2009
.
- X. Qi, J. Xu, Q. Hu, W. Zhong and Y. Du, Mater. Sci. Eng., B, 2015, 198, 108–112 CrossRef CAS
.
- L.-S. Fu, J.-T. Jiang, C.-Y. Xu and L. Zhen, CrystEngComm, 2012, 14, 6827–6832 RSC
.
- C. Shang, G. Ji, W. Liu, X. Zhang, H. Lv and Y. Du, RSC Adv., 2015, 5, 80450–80456 RSC
.
- G. Tong, W. Wu, J. Guan, H. Qian, J. Yuan and W. Li, J. Alloys Compd., 2011, 509, 4320–4326 CrossRef CAS
.
- Z. Wang, X. He, X. Wang, Z. Han, D. Geng, Y. Zhu and Z. Zhang, J. Phys. D: Appl. Phys., 2010, 43, 495404 CrossRef
.
- K. Jia, R. Zhao, J. Zhong and X. Liu, J. Magn. Magn. Mater., 2010, 322, 2167–2171 CrossRef CAS
.
- B. Zhang, Y. Feng, J. Xiong, Y. Yang and H. Lu, IEEE Trans. Magn., 2006, 42, 1778–1781 CAS
.
- Y. Li, T. Wu, K. Jiang, G. Tong, K. Jin, N. Qian, L. Zhao and T. Lv, J. Mater. Chem. C, 2016, 4, 7119–7129 RSC
.
- W.-L. Song, X.-T. Guan, L.-Z. Fan, W.-Q. Cao, Q.-L. Zhao, C.-Y. Wang and M.-S. Cao, Mater. Res. Bull., 2015, 72, 316–323 CrossRef CAS
.
- Z. Zhang, X. Liu, X. Wang, Y. Wu and R. Li, J. Alloys Compd., 2012, 525, 114–119 CrossRef CAS
.
- Y. Li, T. Wu, K. Jin, Y. Qian, N. Qian, K. Jiang, W. Wu and G. Tong, Appl. Surf. Sci., 2016, 387, 190–201 CrossRef CAS
.
- G.-X. Tong, W.-H. Wu, Q. Hu, J.-H. Yuan, R. Qiao and H.-S. Qian, Mater. Chem. Phys., 2012, 132, 563–569 CrossRef CAS
.
- A. Wang, W. Wang, C. Long, W. Li, J. Guan, H. Gu and G. Xu, J. Mater. Chem. C, 2014, 2, 3769–3776 RSC
.
- H. Wu, G. Wu and L. Wang, Powder Technol., 2015, 269, 443–451 CrossRef CAS
.
- X. Li, B. Zhang, C. Ju, X. Han, Y. Du and P. Xu, J. Phys. Chem. C, 2011, 115, 12350–12357 CrossRef CAS
.
- H. Lv, X. Liang, Y. Cheng, G. Ji, D. Tang, B. Zhang, H. Zhang and Y. Du, RSC Adv., 2015, 5, 25936–25941 RSC
.
- C. Liang, C. Liu, H. Wang, L. Wu, Z. Jiang, Y. Xu, B. Shen and Z. Wang, J. Mater. Chem. A, 2014, 2, 16397–16402 RSC
.
- J.-r. Liu, M. Itoh, M. Terada, T. Horikawa and K.-i. Machida, Appl. Phys. Lett., 2007, 91, 093101 CrossRef
.
- H. Wang, D. Zhu, W. Zhou and F. Luo, J. Magn. Magn. Mater., 2015, 393, 445–451 CrossRef CAS
.
- R. Ji, C. Cao, Z. Chen, H. Zhai and J. Bai, J. Mater. Chem. C, 2014, 2, 5944–5953 RSC
.
- G. Wu, H. Zhang, X. Luo, L. Yang and H. Lv, J. Colloid Interface Sci., 2019, 536, 548–555 CrossRef CAS
.
- X. Yan, D. Gao, G. Chai and D. Xue, J. Magn. Magn. Mater., 2012, 324, 1902–1906 CrossRef CAS
.
- M. Zhang, Q. Liu, Z. Zi, Y. Dai, X. Zhu, Y. Sun and J. Dai, Sci. China: Technol. Sci., 2013, 56, 13–19 CAS
.
- A. Maqsood and K. Khan, J. Alloys Compd., 2011, 509, 3393–3397 CrossRef CAS
.
- H. Kavas, A. Baykal, A. Demir, M. S. Toprak and B. Aktaş, J. Inorg. Organomet. Polym. Mater., 2014, 24, 963–970 CrossRef CAS
.
- M. S. Ruiz, P. G. Bercoff and S. E. Jacobo, Ceram. Int., 2013, 39, 4777–4782 CrossRef CAS
.
- J. R. Liu, M. Itoh and K.-i. Machida, Appl. Phys. Lett., 2003, 83, 4017–4019 CrossRef CAS
.
- S. B. Narang and A. Arora, J. Magn. Magn. Mater., 2019, 473, 272–277 CrossRef CAS
.
- Z. Mosleh, P. Kameli, A. Poorbaferani, M. Ranjbar and H. Salamati, J. Magn. Magn. Mater., 2016, 397, 101–107 CrossRef CAS
.
- J. Yu, J. Yu, T. Ying, C. Cui, Y. Sun and X. Liu, J. Alloys Compd., 2019, 775, 225–232 CrossRef CAS
.
- W. Feng, Y. Cao, J. Gang and W. Su, Integr. Ferroelectr., 2018, 190, 63–70 CrossRef CAS
.
- D. Guo, W. Kong, J. Feng, X. Li and X. Fan, Mater. Sci. Eng., B, 2018, 228, 213–217 CrossRef CAS
.
- K. Zhou, J. Cheng, L. Deng, S. Huang, L. Zhou, L. Tang and L. Yang, J. Cent. South Univ. (Sci. Technol.), 2015, 5, 007 Search PubMed
.
- I. Sadiq, S. Naseem, S. Riaz, S. S. Hussain, H. M. Khan, M. N. Ashiq and M. Rana, J. Alloys Compd., 2017, 715, 284–290 CrossRef CAS
.
- C. A. Stergiou and G. Litsardakis, J. Magn. Magn. Mater., 2016, 405, 54–61 CrossRef CAS
.
- C. Yuan and Y. Tuo, J. Magn. Magn. Mater., 2013, 342, 47–53 CrossRef CAS
.
- P. Azizi, S. Masoudpanah and S. Alamolhoda, J. Alloys Compd., 2018, 739, 211–217 CrossRef CAS
.
- R. Joshi, C. Singh, D. Kaur, S. B. Narang, R. Jotania and J. Singh, J. Mater. Sci.: Mater. Electron., 2017, 28, 228–235 CrossRef CAS
.
- F. He, J. Li, L. Chen, L. Chen and Y. Huang, Surf. Rev. Lett., 2015, 22, 1550028 CrossRef CAS
.
- X. Liu, Z. Ou, D. Geng, Z. Han, J. Jiang, W. Liu and Z. Zhang, Carbon, 2010, 48, 891–897 CrossRef CAS
.
- a. Han, D. Li, H. Wang, X. Liu, J. Li, D. Geng and Z. Zhang, Appl. Phys. Lett., 2009, 95, 023114 CrossRef
.
- X. Ding, Y. Huang and J. Wang, RSC Adv., 2015, 5, 64878–64885 RSC
.
- V. Bhingardive, S. Suwas and S. Bose, RSC Adv., 2015, 5, 79463–79472 RSC
.
- C. Feng, X. Liu, Y. Sun, C. Jin and Y. Lv, RSC Adv., 2014, 4, 22710–22715 RSC
.
- J. R. Choi, B. M. Jung, U. H. Choi, S. C. Cho, K. H. Park, W.-j. Kim, S.-K. Lee and S. B. Lee, Composites Research, 2015, 28, 333–339 CrossRef
.
- H. Luo, R. Gong, X. Wang, Y. Nie, Y. Chen and V. G. Harris, J. Alloys Compd., 2015, 646, 345–350 CrossRef CAS
.
-
K. Yao, X. Wu and Z. An, AIP Conference Proceedings, 2017, p. 020023 Search PubMed
.
- K. Oh, S. M. Hong and Y. Seo, Polym. Adv. Technol., 2014, 25, 1366–1370 CrossRef CAS
.
- L. Wang, J. Zhu, H. Yang, F. Wang, Y. Qin, T. Zhao and P. Zhang, J. Alloys Compd., 2015, 634, 232–238 CrossRef CAS
.
- H. Lv, G. Ji, M. Wang, C. Shang, H. Zhang and Y. Du, RSC Adv., 2014, 4, 57529–57533 RSC
.
- I. Arief, S. Biswas and S. Bose, ACS Appl. Nano Mater., 2018, 1, 2261–2271 CrossRef
.
- Y. Wang, W. Zhang, C. Luo, X. Wu and G. Yan, Ceram. Int., 2016, 42, 12496–12502 CrossRef CAS
.
- H. Li, S. Bao, Y. Li, Y. Huang, J. Chen, H. Zhao, Z. Jiang, Q. Kuang and Z. Xie, ACS Appl. Mater. Interfaces, 2018, 10, 28839–28849 CrossRef CAS PubMed
.
- Y. Jia, H. Cui, Z. Y. Yu, H. S. Zhao and X. J. Wang, Adv. Mater. Res., 2012, 482–484, 2322–2329 Search PubMed
.
- I. Arief, S. Biswas and S. Bose, ACS Appl. Nano Mater., 2018, 1, 2261–2271 CrossRef CAS
.
- X. Qi, Y. Yang, W. Zhong, C. Qin, Y. Deng, C. Au and Y. Du, Carbon, 2010, 48, 3512–3522 CrossRef CAS
.
- M. Xu, W. Zhong, Z. Wang, C. Au and Y. Du, Phys. E, 2013, 52, 14–20 CrossRef CAS
.
- C. Wang, R. Lv, Z. Huang, F. Kang and J. Gu, J. Alloys Compd., 2011, 509, 494–498 CrossRef CAS
.
- J. Guo, Y. Duan, L. Liu, L. Chen and S. Liu, J. Electromagn. Anal. Appl., 2011, 3, 140 CAS
.
- X. Guo, Y. Deng, D. Gu, R. Che and D. Zhao, J. Mater. Chem., 2009, 19, 6706–6712 RSC
.
- M. Yu, C. Liang, M. Liu, X. Liu, K. Yuan, H. Cao and R. Che, J. Mater. Chem. C, 2014, 2, 7275–7283 RSC
.
- J. Liu, J. Cheng, R. Che, J. Xu, M. Liu and Z. Liu, J. Phys. Chem. C, 2013, 117, 489–495 CrossRef CAS
.
- Y.-J. Chen, G. Xiao, T.-S. Wang, Q.-Y. Ouyang, L.-H. Qi, Y. Ma, P. Gao, C.-L. Zhu, M.-S. Cao and H.-B. Jin, J. Phys. Chem. C, 2011, 115, 13603–13608 CrossRef CAS
.
- C.-L. Zhu, M.-L. Zhang, Y.-J. Qiao, G. Xiao, F. Zhang and Y.-J. Chen, J. Phys. Chem. C, 2010, 114, 16229–16235 CrossRef CAS
.
- G. Liu, L. Wang, Z. Yang and R. Wu, J. Alloys Compd., 2017, 718, 46–52 CrossRef CAS
.
- J. Pan, X. Sun, T. Wang, Z. Zhu, Y. He, W. Xia and J. He, Appl. Surf. Sci., 2018, 457, 271–279 CrossRef CAS
.
- J. Liu, J. Cheng, R. Che, J. Xu, M. Liu and Z. Liu, ACS Appl. Mater. Interfaces, 2013, 5, 2503–2509 CrossRef CAS PubMed
.
- Y.-F. Zhu, Q.-Q. Ni, Y.-Q. Fu and T. Natsuki, J. Nanopart. Res., 2013, 15, 1988 CrossRef PubMed
.
- G. Wang, Y. Chang, L. Wang and C. Liu, Adv. Powder Technol., 2012, 23, 861–865 CrossRef CAS
.
- X. Sun, G. Ma, X. Lv, M. Sui, H. Li, F. Wu and J. Wang, Materials, 2018, 11, 780 CrossRef PubMed
.
- X. Liu, D. Geng, H. Meng, P. Shang and Z. Zhang, Appl. Phys. Lett., 2008, 92, 173117 CrossRef
.
- Z.-d. Zhang, Z.-c. Shi, R.-h. Fan, M. Gao, J.-y. Guo, X.-g. Qi and K.-n. Sun, Mater. Chem. Phys., 2011, 130, 615–618 CrossRef CAS
.
- L. Yan, J. Wang, X. Han, Y. Ren, Q. Liu and F. Li, Nanotechnology, 2010, 21, 095708 CrossRef PubMed
.
- J. Liu, R. Che, H. Chen, F. Zhang, F. Xia, Q. Wu and M. Wang, Small, 2012, 8, 1214–1221 CrossRef CAS PubMed
.
- X. Liu, Y. Sun, C. Feng, C. Jin and W. Li, Appl. Surf. Sci., 2013, 280, 132–137 CrossRef CAS
.
- X. Liu, G. Zhou, S. W. Or and Y. Sun, RSC Adv., 2014, 4, 51389–51394 RSC
.
- R. Pang, X. Hu, S. Zhou, C. Sun, J. Yan, X. Sun, S. Xiao and P. Chen, Chem. Commun., 2014, 50, 12493–12496 RSC
.
- R. Qiang, Y. Du, H. Zhao, Y. Wang, C. Tian, Z. Li, X. Han and P. Xu, J. Mater. Chem. A, 2015, 3, 13426–13434 RSC
.
- J. Wang, P. Zhu, J. Wang, S. W. Or, S. Ho and J. Tan, AIP Adv., 2017, 7, 055811 CrossRef
.
- K. Wang, G. Wan, G. Wang, Z. He, S. Shi, L. Wu and G. Wang, J. Colloid Interface Sci., 2018, 511, 307–317 CrossRef CAS PubMed
.
- H. Lv, H. Zhang, G. Ji and Z. J. Xu, ACS Appl. Mater. Interfaces, 2016, 8, 6529–6538 CrossRef CAS PubMed
.
- G. Liu, W. Jiang, Y. Wang, S. Zhong, D. Sun, J. Liu and F. Li, Ceram. Int., 2015, 41, 4982–4988 CrossRef CAS
.
- Z. Wang, L. Wu, J. Zhou, B. Shen and Z. Jiang, RSC Adv., 2013, 3, 3309–3315 RSC
.
- K. Yuan, R. Che, Q. Cao, Z. Sun, Q. Yue and Y. Deng, ACS Appl. Mater. Interfaces, 2015, 7, 5312–5319 CrossRef CAS PubMed
.
- Z. Zhang, X. Liu, X. Wang, Y. Wu and Y. Liu, J. Magn. Magn. Mater., 2012, 324, 2177–2182 CrossRef CAS
.
- Z. Wu, D. Tan, K. Tian, W. Hu, J. Wang, M. Su and L. Li, J. Phys. Chem. C, 2017, 121, 15784–15792 CrossRef CAS
.
-
C. Wang, Fuzzy Systems and Knowledge Discovery (FSKD), 2012 9th International Conference, 2012, pp. 2473–2476 Search PubMed
.
- Y. Wang, H. Zhu, Y. Chen, X. Wu, W. Zhang, C. Luo and J. Li, Mater. Chem. Phys., 2017, 202, 184–189 CrossRef CAS
.
- N. Zhang, Y. Huang, M. Zong, X. Ding, S. Li and M. Wang, Ceram. Int., 2016, 42, 18879–18886 CrossRef CAS
.
- Y. Wang, Z. Peng and W. Jiang, Ceram. Int., 2016, 42, 10682–10689 CrossRef CAS
.
- C. Chen, S. Bao, B. Zhang, Y. Chen, W. Chen and C. Wang, Appl. Surf. Sci., 2019, 467–468, 836–843 CrossRef CAS
.
- Z. Peng, W. Jiang, Y. Wang and S. Zhong, J. Mater. Sci.: Mater. Electron., 2016, 27, 1304–1313 CrossRef CAS
.
- H. Lv, G. Ji, W. Liu, H. Zhang and Y. Du, J. Mater. Chem. C, 2015, 3, 10232–10241 RSC
.
- Y.-L. Ren, H.-Y. Wu, M.-M. Lu, Y.-J. Chen, C.-L. Zhu, P. Gao, M.-S. Cao, C.-Y. Li and Q.-Y. Ouyang, ACS Appl. Mater. Interfaces, 2012, 4, 6436–6442 CrossRef CAS PubMed
.
- A. Feng, Z. Jia, Y. Zhao and H. Lv, J. Alloys Compd., 2018, 745, 547–554 CrossRef CAS
.
- Q. Chen, L. Li, Z. Wang, Y. Ge, C. Zhou and J. Yi, J. Alloys Compd., 2019, 779, 720–727 CrossRef CAS
.
- B. Qu, C. Zhu, C. Li, X. Zhang and Y. Chen, ACS Appl. Mater. Interfaces, 2016, 8, 3730–3735 CrossRef CAS PubMed
.
- Y. Wang, X. Gao, X. Wu, W. Zhang, Q. Wang and C. Luo, Ceram. Int., 2018, 44(8), 9816–9822 CrossRef CAS
.
- Y. Wang, X. Wu, W. Zhang, C. Luo, J. Li and Q. Wang, Synth. Met., 2017, 231, 7–14 CrossRef CAS
.
- L. Jin, X. Zhao, J. Xu, Y. Luo, D. Chen and G. Chen, RSC Adv., 2018, 8, 2065–2071 RSC
.
- L. Wang, Y. Huang, C. Li, J. Chen and X. Sun, Compos. Sci. Technol., 2015, 108, 1–8 CrossRef CAS
.
- W. Liu, L. Liu, G. Ji, D. Li, Y. Zhang, J. Ma and Y. Du, ACS Sustainable Chem. Eng., 2017, 5, 7961–7971 CrossRef CAS
.
- Y. Wang, Z. Luo and R. Hong, Mater. Lett., 2011, 65, 3241–3244 CrossRef CAS
.
- P. Liu, Y. Huang, Y. Yang, J. Yan and X. Zhang, J. Alloys Compd., 2016, 662, 63–68 CrossRef CAS
.
- A. Olad and S. Shakoori, J. Magn. Magn. Mater., 2018, 458, 335–345 CrossRef CAS
.
- S. P. Pawar, M. Gandi, C. Saraf and S. Bose, ChemistrySelect, 2016, 1, 3829–3838 CrossRef CAS
.
- Y. Wang, Y. Huang and J. Ding, Mater. Sci. Semicond. Process., 2014, 26, 632–641 CrossRef CAS
.
- P. Zhou, J.-H. Chen, M. Liu, P. Jiang, B. Li and X.-M. Hou, Int. J. Miner., Metall. Mater., 2017, 24, 804–813 CrossRef CAS
.
- D. Sun, Q. Zou, Y. Wang, Y. Wang, W. Jiang and F. Li, Nanoscale, 2014, 6, 6557–6562 RSC
.
- C. Hu, Z. Mou, G. Lu, N. Chen, Z. Dong, M. Hu and L. Qu, Phys. Chem. Chem. Phys., 2013, 15, 13038–13043 RSC
.
- X. Sun, J. He, G. Li, J. Tang, T. Wang, Y. Guo and H. Xue, J. Mater. Chem. C, 2013, 1, 765–777 RSC
.
- H.-L. Xu, H. Bi and R.-B. Yang, J. Appl. Phys., 2012, 111, 07A522 CrossRef
.
- M. Zong, Y. Huang, Y. Zhao, X. Sun, C. Qu, D. Luo and J. Zheng, RSC Adv., 2013, 3, 23638–23648 RSC
.
- M. Fu, Q. Jiao and Y. Zhao, J. Mater. Chem. A, 2013, 1, 5577–5586 RSC
.
- L. Wang, X. Bai and M. Wang, J. Mater. Sci.: Mater. Electron., 2018, 29, 3381–3390 CrossRef CAS
.
- Z. Zhu, X. Sun, H. Xue, H. Guo, X. Fan, X. Pan and J. He, J. Mater. Chem. C, 2014, 2, 6582–6591 RSC
.
- M. Zong, Y. Huang, X. Ding, N. Zhang, C. Qu and Y. Wang, Ceram. Int., 2014, 40, 6821–6828 CrossRef CAS
.
- N. Zhang, Y. Huang, M. Zong, X. Ding, S. Li and M. Wang, Chem. Eng. J., 2017, 308, 214–221 CrossRef CAS
.
- N. Zhang, Y. Huang, M. Zong, X. Ding, S. Li and M. Wang, Ceram. Int., 2016, 42, 15701–15708 CrossRef CAS
.
- B. Quan, G. Xu, D. Li, W. Liu, G. Ji and Y. Du, J. Colloid Interface Sci., 2017, 498, 161–169 CrossRef CAS PubMed
.
- Y. Xu, Q. Wang, Y. Cao, X. Wei and B. Huang, RSC Adv., 2017, 7, 18172–18177 RSC
.
- R. Shu, G. Zhang, J. Zhang, X. Wang, M. Wang, Y. Gan, J. Shi and J. He, Mater. Lett., 2018, 215, 229–232 CrossRef CAS
.
- Y. Li, D. Li, J. Yang, H. Luo, F. Chen, X. Wang and R. Gong, Materials, 2018, 11, 508 CrossRef PubMed
.
- Y. Wang, Y. Huang, Q. Wang and M. Zong, Powder Technol., 2013, 249, 304–308 CrossRef CAS
.
- S. Jiao, M. Wu, X. Yu, H. Hu, Z. Bai, P. Dai, T. Jiang, H. Bi and G. Li, Mater. Res. Bull., 2018, 108, 89–95 CAS
.
- Q. Zhang, Z. Du, X. Huang, Z. Zhao, T. Guo, G. Zeng and Y. Yu, Appl. Surf. Sci., 2019, 473, 706–714 CrossRef CAS
.
- C. Li, Y. Ge, X. Jiang, G. I. Waterhouse, Z. Zhang and L. Yu, Ceram. Int., 2018, 44, 19171–19183 CrossRef CAS
.
- X. Zhang, X. Dong, H. Huang, B. Lv, J. Lei and C. Choi, J. Phys. D: Appl. Phys., 2007, 40, 5383 CAS
.
- H. Pourabdollahi and A. R. Zarei, Results Phys., 2017, 7, 1978–1986 CrossRef
.
- F. Peng, F. Meng, Y. Guo, H. Wang, F. Huang and Z. Zhou, ACS Sustainable Chem. Eng., 2018, 6, 16744–16753 CrossRef CAS
.
- Y. Zhao, L. Liu, K. Jiang, M. Fan, C. Jin, J. Han, W. Wu and G. Tong, RSC Adv., 2017, 7, 11561–11567 RSC
.
- L. Liu, Z. He, Y. Zhao, J. Sun and G. Tong, J. Alloys Compd., 2018, 765, 1218–1227 CrossRef CAS
.
- X. Su, J. Wang, B. Zhang, W. Chen, Q. Wu, W. Dai and Y. Zou, Mater. Res. Express, 2018, 5, 056305 CrossRef
.
- S. Biswas, S. S. Panja and S. Bose, J. Phys. Chem. C, 2017, 121, 13998–14009 CrossRef CAS
.
- Y.-c. Sun, W.-y. Cui, J.-l. Li and J.-z. Wu, J. Alloys Compd., 2019, 770, 67–75 CrossRef CAS
.
- L. Yu, X. Lan, C. Wei, X. Li, X. Qi, T. Xu, C. Li, C. Li and Z. Wang, J. Alloys Compd., 2018, 748, 111–116 CAS
.
-
L. Huang, X. Liu and R. Yu, Progress in Natural Science: Materials International, 2018 Search PubMed
.
- F. Wen, F. Zhang and Z. Liu, J. Phys. Chem. C, 2011, 115, 14025–14030 CAS
.
- K. Osouli-Bostanabad, H. Aghajani, E. Hosseinzade, H. Maleki-Ghaleh and M. Shakeri, Mater. Manuf. Processes, 2016, 31, 1351–1356 CAS
.
- X. Zhang, Int. J. Polym. Sci., 2018, 2018, 3417060 Search PubMed
.
- F. Xu, L. Ma, Q. Huo, M. Gan and J. Tang, J. Magn. Magn. Mater., 2015, 374, 311–316 CrossRef CAS
.
- C. Yang, H. Li, D. Xiong and Z. Cao, React. Funct. Polym., 2009, 69, 137–144 CrossRef CAS
.
- Y. Sun, F. Xiao, X. Liu, C. Feng and C. Jin, RSC Adv., 2013, 3, 22554–22559 RSC
.
- B. Zhang, Y. Du, P. Zhang, H. Zhao, L. Kang, X. Han and P. Xu, J. Appl. Polym. Sci., 2013, 130, 1909–1916 CrossRef CAS
.
- Y.-F. Zhu, L. Zhang, T. Natsuki, Y.-Q. Fu and Q.-Q. Ni, Synth. Met., 2012, 162, 337–343 CrossRef CAS
.
- T.-H. Ting and K.-H. Wu, J. Magn. Magn. Mater., 2010, 322, 2160–2166 CrossRef CAS
.
- J. Liu, J. Zhang, Y. Li and M. Zhang, Mater. Chem. Phys., 2015, 163, 470–477 CrossRef CAS
.
- M.-S. Cao, J. Yang, W.-L. Song, D.-Q. Zhang, B. Wen, H.-B. Jin, Z.-L. Hou and J. Yuan, ACS Appl. Mater. Interfaces, 2012, 4, 6949–6956 CrossRef CAS PubMed
.
- F. Movassagh-Alanagh, A. Bordbar-Khiabani and A. Ahangari-Asl, Compos. Sci. Technol., 2017, 150, 65–78 CrossRef CAS
.
- Y. Wang, X. Wu, W. Zhang, C. Luo, J. Li, Q. Wang and Q. Wang, Mater. Chem. Phys., 2018, 209, 23–30 CrossRef CAS
.
- L. Li, C. Xiang, X. Liang and B. Hao, Synth. Met., 2010, 160, 28–34 CrossRef CAS
.
- L. Liu, H. Zhang, J. Li, Y. Shen, C. Wang, L. Qiu, Y. Yuan and A. Xie, Mater. Res. Bull., 2014, 53, 58–64 CrossRef CAS
.
- Z. He, Y. Fang, X. Wang and H. Pang, Synth. Met., 2011, 161, 420–425 CrossRef CAS
.
- B. Li, X. Weng, G. Wu, Y. Zhang, X. Lv and G. Gu, J. Saudi Chem. Soc., 2017, 21, 466–472 CrossRef CAS
.
- Z. He, S. Qi, X. Zhong, H. Ma, P. Wang and H. Qiu, J. Alloys Compd., 2015, 621, 194–200 CrossRef CAS
.
- S. H. Hosseini, S. Mohseni, A. Asadnia and H. Kerdari, J. Alloys Compd., 2011, 509, 4682–4687 CrossRef CAS
.
- L. Du, Y. Du, Y. Li, J. Wang, C. Wang, X. Wang, P. Xu and X. Han, J. Phys. Chem. C, 2010, 114, 19600–19606 CrossRef CAS
.
- M. Qiao, X. Lei, Y. Ma, L. Tian, K. Su and Q. Zhang, Ind. Eng. Chem. Res., 2016, 55, 6263–6275 CrossRef CAS
.
- T. Liu, N. Liu, S. Zhai, S. Gao, Z. Xiao, Q. An and D. Yang, J. Alloys Compd., 2019, 779, 831–843 CrossRef CAS
.
- S. Ji, Z. Zhang, X. Jiang and L. Yu, J. Mater. Sci., 2017, 52, 12358–12369 CrossRef CAS
.
- S. Ji, C. Li, Z. Zhang, X. Jiang and L. Yu, Synth. Met., 2018, 239, 59–65 CrossRef CAS
.
- W. Zhou, X. Hu, C. Sun, J. Yan, S. Zhou and P. Chen, Polym. Adv. Technol., 2014, 25, 83–88 CrossRef CAS
.
- A. Ohlan, K. Singh, A. Chandra and S. K. Dhawan, ACS Appl. Mater. Interfaces, 2010, 2, 927–933 CrossRef CAS PubMed
.
- T. T. Tung, J.-F. Feller, T. Kim, H. Kim, W. S. Yang and K. S. Suh, J. Polym. Sci., Part A: Polym. Chem., 2012, 50, 927–935 CrossRef CAS
.
- L. Yan, X. Wang, S. Zhao, Y. Li, Z. Gao, B. Zhang, M. Cao and Y. Qin, ACS Appl. Mater. Interfaces, 2017, 9, 11116–11125 CrossRef CAS PubMed
.
- Y. Xia, J. Fang, P. Li, B. Zhang, H. Yao, J. Chen, J. Ding and J. Ouyang, ACS Appl. Mater. Interfaces, 2017, 9, 19001–19010 CrossRef CAS PubMed
.
- X. Liu, X. Cui, Y. Chen, X.-J. Zhang, R. Yu, G.-S. Wang and H. Ma, Carbon, 2015, 95, 870–878 CrossRef CAS
.
- C. Tsonos, N. Soin, G. Tomara, B. Yang, G. C. Psarras, A. Kanapitsas and E. Siores, RSC Adv., 2016, 6, 1919–1924 RSC
.
- R. Bera, A. K. Das, A. Maitra, S. Paria, S. K. Karan and B. B. Khatua, Composites, Part B, 2017, 129, 210–220 CrossRef CAS
.
- L.-y. Li, S.-l. Li, Y. Shao, R. Dou, B. Yin and M.-b. Yang, Curr. Appl. Phys., 2018, 18, 388–396 CrossRef
.
- A. Zhang, M. Tang, X. Cao, Z. Lu and Y. Shen, J. Mater. Sci., 2014, 49, 4629–4635 CrossRef CAS
.
- Y. Chen, Y. Wang, H.-B. Zhang, X. Li, C.-X. Gui and Z.-Z. Yu, Carbon, 2015, 82, 67–76 CrossRef CAS
.
- B. B. Rao, P. Yadav, R. Aepuru, H. Panda, S. Ogale and S. Kale, Phys. Chem. Chem. Phys., 2015, 17, 18353–18363 RSC
.
- H. Guo, Y. Zhan, Z. Chen, F. Meng, J. Wei and X. Liu, J. Mater. Chem. A, 2013, 1, 2286–2296 RSC
.
- Z. Song, L. Deng, J. Xie, P. Zhou, H. Lu and X. Wang, Surf. Interface Anal., 2014, 46, 77–82 CrossRef CAS
.
-
K. Tripathi, S. Abbas, R. Sharma, P. Alegaonker, M. Verma and S. Kumar, Electromagnetic and Microwave Absorption Properties of Ni0.5Zn0.5Fe2O4 Nano Ferrite/PU Based Nano-Composite, 2015 Search PubMed
.
- O. Yalçın, H. Bayrakdar and S. Özüm, J. Magn. Magn. Mater., 2013, 343, 157–162 CrossRef
.
- Z. Guo, H. Huang, D. Xie and H. Xia, Sci. Rep., 2017, 7, 11331 CrossRef PubMed
.
- R.-B. Yang, P. M. Reddy, C.-J. Chang, P.-A. Chen, J.-K. Chen and C.-C. Chang, Chem. Eng. J., 2016, 285, 497–507 CrossRef CAS
.
- Y. Qing, W. Zhou, F. Luo and D. Zhu, Carbon, 2010, 48, 4074–4080 CrossRef CAS
.
- C.-C. Chen, W.-F. Liang, Y.-H. Nien, H.-K. Liu and R.-B. Yang, Mater. Res. Bull., 2017, 96, 81–85 CrossRef CAS
.
- Y. Qing, D. Min, Y. Zhou, F. Luo and W. Zhou, Carbon, 2015, 86, 98–107 CrossRef CAS
.
- S.-S. Kim, S.-T. Kim, Y.-C. Yoon and K.-S. Lee, J. Appl. Phys., 2005, 97, 10F905 CrossRef
.
- B. Mordina, R. Kumar, R. K. Tiwari, D. K. Setua and A. Sharma, J. Phys. Chem. C, 2017, 121, 7810–7820 CrossRef
.
- A. Al-Ghamdi, O. A. Al-Hartomy, F. Al-Salamy, A. A. Al-Ghamdi, E. El-Mossalamy, A. Abdel Daiem and F. El-Tantawy, J. Appl. Polym. Sci., 2012, 125, 2604–2613 CrossRef CAS
.
- K. Nasouri and A. M. Shoushtari, J. Thermoplast. Compos. Mater., 2018, 31, 431–446 CrossRef CAS
.
- S. P. Pawar, D. A. Marathe, K. Pattabhi and S. Bose, J. Mater. Chem. A, 2015, 3, 656–669 RSC
.
- Y. Zhang, J. Liu, Y. Zhang, J. Liu and Y. Duan, RSC Adv., 2017, 7, 54031–54038 RSC
.
- M. Sui, X. Lü, A. Xie, W. Xu, X. Rong and G. Wu, Synth. Met., 2015, 210, 156–164 CrossRef CAS
.
- J. Jin, Y. Liu, M. G. Drew and Y. Liu, J. Mater. Sci.: Mater. Electron., 2017, 28, 11325–11331 CrossRef CAS
.
- X. Zhang, Y. Huang and P. Liu, Nano-Micro Lett., 2016, 8, 131–136 CrossRef PubMed
.
- M. Fan, Z. He and H. Pang, Synth. Met., 2013, 166, 1–6 CrossRef CAS
.
|
This journal is © The Royal Society of Chemistry 2019 |
Click here to see how this site uses Cookies. View our privacy policy here.