Computational model of inductively coupled plasma sources in comparison to experimental data for different torch designs and plasma conditions. Part I: experimental study†
Received
19th May 2016
, Accepted 5th December 2016
First published on 5th December 2016
Abstract
Emission of argon, analyte atoms and ions, and OH lines in standard and low-argon-flow inductively coupled plasmas (ICPs) was studied spatially using a novel imaging acousto-optical spectrometer (AOS). Unique characteristics of the AOS (wavelength range: 250–800 nm, spectral resolution: 0.05–0.5 nm, time resolution: 5 ns, change of wavelength: 0.2 ms, spatial resolution: >125 μm) allowed the measurement of high quality images at 21 different wavelengths for each set of plasma conditions within approximately 20 min. Fundamental plasma properties were determined over a wide range of operational parameters. Rotational, excitation, and electron temperatures were ranging from 4580–5120 K, 4360–5000 K, and 8770–10
840 K in the analytical zone of the low-argon-flow ICP, and 3230–4250 K, 3000–5560 K, and 6130–10
200 K in the analytical zone of the standard ICP, respectively. The emission investigations were complemented by IR-measurements of the torch wall temperature and measurements of electrical properties of the ICP. RF voltages and RF currents were measured directly in the load coil and were used to calculate the plasma power. Depending on plasma conditions, the measured power differs from the indicated one (vendor software) by up to a factor of two.
1 Introduction
The combination of this article with Part II (ref. 1) presents an ICP model, which is well supported by experimental data with different torch geometries and plasma conditions. It is assumed that this will be used in the future for computational optimization of ICP torches. Part I of the study focuses on the experimental plasma diagnostics. Part II explains the computational model in detail.
The ICP is a well-established source for optical emission spectrometry (OES) and mass spectrometry.2 The ICP torch is important for the formation of the plasma and has not been modified significantly since decades. Today's commercially available ICP torch geometries still feature three concentric quartz tubes based on the initial designs by Greenfield and Fassel.3–5 Typically, the ICP is operated at a total Ar gas consumption of 12–20 L min−1 (depending on the manufacturer) with a radio frequency (RF) power consumption of 1–1.5 kW. In the past, several studies reported modifications of torch geometries with the goal of reducing the RF power consumption and/or the total Ar consumption. Torches with Fassel geometry but reduced size were developed, for example, in the research groups of Hieftje6–9 and Barnes.10,11 They are characterized by requiring approximately 50% less RF power and Ar compared to conventional torches. In the laboratory of de Galan, torches without the outer cooling gas flow were developed.12,13 The heat in these sources is dissipated to the surrounding air through a refractory boron nitride wall and a fused silica wall, respectively, with additional air-cooling from outside. These torches featured a reduced Ar consumption of approximately 1 L min−1. Two water-cooled ICP torches were developed by Kawaguchi et al.14 and in laboratory of de Galan.15 They reduce the Ar consumption to 5 L min−1 and 2 L min−1, respectively. Also, an enclosed ICP source was developed in the group of Barnes.16 Today, some ICP instrumentation manufacturers offer so-called “eco” torches, which allow a reduction of the Ar consumption of less than 50%. To the best knowledge of the authors, torches with lower Ar consumption have not been commercialized yet, leaving a lot of room for improvement. The ultimate goal would be a more efficient and environmentally friendly ICP at significantly reduced operating costs and with even better analytical performance for ultra trace analysis.
Another low-argon-flow torch variant, the SHIP torch, was developed by Buscher et al. Here, external cooling of the torch wall by compressed air together with a bulb-shaped torch geometry helped to reduce the total Ar consumption to only 0.6 L min−1 with detection limits, that are similar to the standard Fassel-type torch. Detailed information about the design, analytical and physical characteristics, as well as applications of the SHIP torch can be found in earlier publications.17–28
Interestingly, all these developments described above were mainly based on empirical studies. Further improvement over existing ICP torches would require numerical calculations for modeling and optimization including, for example, the cooling efficiency of the torch wall cooling device, plasma temperature, electron number density, and emission/ions profiles. A short review of the existing ICP models can be found in our complementary article.1
Currently, there is still a lack of experimental data for comparison with simulation results, especially for alternative torch geometries. Most of the published plasma diagnostics measurements, as well as simulations, were carried out for the standard ICP torch (see ref. 2 and references therein) or a few alternative torches17,20,22 at (mostly) optimal conditions. This article reports results of a variety of different plasma diagnostics in a wide range of ICP parameters to partly fill this gap. Two different ICP torches were used: a Fassel-type ICP torch and a low-argon-flow (SHIP) torch, both operated in the Spectro CIROSCCD ICP-OES instrument. The plasma diagnostics performed here include measurements of voltage and current directly in the load coil and subsequent calculation28 of plasma power Pmeas (in the cited article the plasma power calculated from the measured voltage and current of the load coil is called measured power Pmeas to emphasize difference of this power and the power indicated by the controlling software. Therefore here the term Pmeas is preserved), as well as measurements of the spatial distribution of intensities of Ar, OH, and analyte (Fe and Mg) emission lines. The spatial distribution of the rotational, excitation, and electron temperature were obtained from the measured spectral distributions of OH lines, Fe lines, and line/background intensity ratio, respectively. Additionally, the temperature of the torch wall, Twall, was measured. Most of the listed characteristics were already published in literature (for example, ref. 2 and references therein; refs. 17,20,22), but in a limited range of plasma conditions (mostly near the optimal one). In addition to the optimal parameters, this article focuses on the influence of a wide set of plasma parameters on the measured magnitudes. Also, it was the goal to characterize two different plasmas (SHIP and standard ICP) using identical instrumentation and to build an experimental dataset for direct comparison with the results obtained in the ICP simulations.1
Note that the used ICP instrument has an axially oriented optical interface equipped with a counter argon gas flow (Spectro CIROSCCD, Kleve, Germany), which is situated in the optical axis of the ICP. In principle, all results and conclusions presented here are valid only for this special instrumental setup (see Experimental setup for details). However, general trends may also be extrapolated (with care) to other commercial ICP excitation sources.
2 Theoretical background
The electronic excitation temperature (Texc) can be determined by means of the Boltzmann plot method.29 By measuring relative intensities of emission lines with different excitation energies, Texc can be calculated from the slope (−1/kTexc) of the straight line fitted to a plot of log(Ipqλpq/gpApq) as function of Ep, where k is the Boltzmann constant, Ipq is the emission intensity of the spectral transition, λpq is the wavelength, gp is the degree of degeneracy of level p, Apq is the transition probability for spontaneous emission, and Ep is the excitation energy of the level p with respect to the ground state. In our measurements atomic lines of Fe were used in the range 370–380 nm. A list of the monitored spectral lines and fundamental data is provided in the ESI, Table S-1.† Six iron emission lines were used to obtain the excitation temperature with the Boltzmann plot method. A significant curvature in the data was not observed but the range of excitation energies (around 3.3 eV and 4.2 eV) was also small.
The rotational temperature (Trot) is a good approximation of the plasma gas-kinetic temperature.29 Relative intensity distributions in the rotational fine structure of the A2Σ+ → X2Πi electronic band of the OH radical at 306.4 nm were measured and Trot was calculated from the slope of a Boltzmann plot of log(Iλ/A) versus the energy of the upper excited level E. Further details of the method are described by Ishii and Montaser for the Ar ICP.30 In Table S-2 of the ESI† a list of the monitored OH spectral lines and fundamental data is presented.
The electron temperature (Te) gives a measure of the kinetic energy of the electrons and can be determined from the line-to-continuum intensity ratio as described by Bastiaans and Mangold:31
εI/Ic(λ) = C(Apqgp/Ui)(1/Te)exp((Ei − Ep − ΔEi)/kTe)(λ/Δλ)/(ξ(1 − α) + Gα), |
where
εI is the emission intensity of an atomic line,
Ic is the emission intensity of the adjacent continuum, the constant
C incorporates fundamental constants,
Ui is the partition function of the ion,
Ei is the ionization potential, Δ
Ei is lowering of the ionization potential of atoms in the presence of a field of ions, Δ
λ is the wavelength bandwidth of the measurement,
ξ is the free-bound continuum correction factor,
G is free–free Gaunt factor,
α = exp(−
hν/
kTe),
ν is the transition frequency,
h is the Plank constant. To find
Te, this equation needed to be solved numerically. Practically, this equation is often simplified
22,31 assuming
α = 0. In our conditions, this simplification introduces a deviation of the resulting
Te of about 25%. However, this general simplification is also used in this work, in a way that the results can be compared with results of other publications. Thus, the calculation of
Te is not as accurate as the calculation of
Trot or
Texc, where the accuracy can be estimated from the deviation of the Boltzmann plot from a straight line. The intensity of Ar I 430.010 nm line was computed for the
Te measurements, because the value of the transition probability is considered to be accurately reported.
31,32 Related values (
Qi,
Ai,
etc.) were taken from the earlier published article.
32
3 Experimental setup
A commercial ICP-OES instrument (model CIROSCCD, SPECTRO Analytical Instruments GmbH, Kleve, Germany) was used in this study. For routine analysis, radiation from the ICP torch is detected end-on (axial viewing) by the built-in spectrometer through an optical plasma interface (OPI). A counter gas flow of Ar towards the ICP torch is used to flush the optical path. This extends the spectral range of the instrument into the ultraviolet region. The ICP torch is positioned in a solid silver load coil with 2.5 turns for operation. The diameter of the wire of the coil is 5 mm, the diameter of the inner cylindrical envelope of the coil is approximately 2.5 cm. Two fundamentally different ICP torch geometries were used in this study: First, a standard ICP torch (CIROS EOP quartz torch with 17 mm i.d., 19 mm o.d., and 2.5 mm wide central channel tube). The second is a low-argon-flow SHIP torch. The latter is bulb-shaped, uses only two Ar gas flows, and features a slightly larger maximum outer diameter (22 mm i.d.) as compared to a standard torch. The total Ar consumption is 0.6 L min−1. This torch is cooled externally by compressed air. Detailed information about the design, analytical and physical characteristics of the SHIP torch can be found in earlier publications.17–28 The optimal conditions for the torches are presented in Table 1. The sample introduction system includes a cyclonic spray chamber (Tracey HF Resistant Spray Chamber, Thermo Fisher Scientific Inc., Bremen, Germany) and a pneumatic nebulizer. For the standard torch, a pneumatic nebulizer with a sample uptake rate of 2 mL min−1 (Meinhard TR-30-A3, Golden, Colorado, USA) was used. The SHIP torch requires a pneumatic micronebulizer with an uptake rate of 200 μL min−1 (Micro Mist AR27-04-FM02E, Glass Expansion, West Melbourne, Australia). Both nebulizers were used in self-aspirating mode. For Fe and Mg measurements, solutions containing 100 mg L−1 of Mg and Fe were prepared from the following standards: Fe CGFE1-125 mL, Inorganic Ventures, Christiansburg, Virginia, USA; Mg Certipur 1000 mg L−1, Merck KGaA, Darmstadt, Germany.
Table 1 Optimal conditions of ICP operation. The optimal conditions for the standard torch are recommended by the manufacturer of the CIROS instrument. The operational parameters of SHIP were optimized earlier19 to reach the best possible detection limits
|
SHIP torch |
Standard torch |
Indicated RF power (W) |
1100 |
1200 |
Central carrier gas flow (L min−1) |
0.4 |
1 |
Auxiliary plasma gas flow (L min−1) |
0.2 |
1 |
Outer plasma gas flow (L min−1) |
— |
12 |
External cooling air flow (L min−1) |
245 |
— |
Ar counterflow (L min−1) |
0 |
0.6 |
Distance from OPI (cm) |
1.0 |
1.0 |
Sample uptake rate (mL min−1) |
0.2 |
2 |
In this study, the spatial distribution of the radiation from the ICP torch is detected side-on (radial viewing) by an AOS.33 In our experimental setup, the AOS provided a spectral resolution of approximately 0.15 nm FWHM (full width at half maximum) and a spatial resolution of approximately 300 μm. These values were considered sufficient for the study of most of the spectral features, with the exception of the OH band. Here, the spectral resolution of the AOS was found to be not sufficient and an alternative monochromator-based approach was used (see below). However, the intense OH 309.0 nm line was detected with the AOS to demonstrate the benefits of imaging and to gain some insight into the spatial nature of the OH emission directly. The bandwidth of the measurement Δλ, which is used for Te calculation,31 was 0.20 nm. This was determined by measuring the Ar I 430.0 nm line shape and calculating the area of the corresponding peak. Data presented in this work was not subjected to an Abel inversion procedure. Abel inversion is usually applied to calculate radially resolved from lateral spatially resolved emission intensities in plasma sources.34–36 However, it was not applied here, because the low-argon-flow ICP exhibited a less cylindrical symmetry as the standard ICP.22 Abel inversion itself may also introduce errors, particularly near the center of the radial emission profiles.37,38 In the future, a detailed comparison of Abel-inverted vs. non-inverted data from low-argon-flow ICP would be helpful to investigate potential errors/benefits in more detail.
For Trot determination, a Czerny–Turner monochromator (Digikröm DK480, Spectral Products, Albuquerque, USA, photomultiplier tube R889, Hamamatsu, Hamamatsu City, Japan) with a spectral resolution of approximately 50 pm FWHM was used. Plasma radiation was collected with an optical fiber and collimating lens positioned at a distance of 5 cm to the torch and moved stepwise to probe different plasma regions. Thus the lateral resolution was limited here by the diameter of the lens (4 mm). The PMT signal was amplified with a current amplifier (SR570, Stanford Research Systems, Sunnyvale, USA) and then transferred to a computer by a digital multimeter (HAMEG HM 8112-2, Mainhausen, Germany).
Spectral lines measured for the calculation of Texc and Trot are situated in a narrow range of wavelengths (within 4 and 12 nm, as can be seen in Tables S-1 and S-2 in the ESI, respectively†). The sensitivity of the AOS and the additional Czerny–Turner monochromator varies very slightly in such narrow ranges. Therefore no additional calibration of the sensitivity of monochromator and AOS was carried out.
Electrical voltage and current in the load coil of the ICP were measured directly with probes that were recently developed by the authors.28 Based on the measured voltage and current values, the plasma power Pmeas was then calculated as described in detail earlier28 (in this reference the plasma power is denoted as P). The power value, which is displayed by the ICP instrument's operation software, can be different from Pmeas because of the indirect measurement of the power in the CIROS instrument.28 This is a typical feature also of many commercial ICP instruments.
Infrared radiation from the walls of the ICP torches was detected by an infrared camera (ThermaCAM B20 HSV, Flir Systems, Melrose, USA). This camera detects IR images in a wavelength range between 10 and 11 μm and transforms them to temperature maps. The camera requires known emissivity values of the sample for reliable determination of true temperature. Here, an emissivity value of 0.97 was used, which is typical for quartz glass objects in this wavelength range. To confirm the reliability of the measurements, the quartz glass SHIP torch was placed in a thermostatic oven with an opened window. The temperature of the torch was varied from 400 to 1000 °C before the images were measured. The difference between measured temperature and set oven/torch temperature did not exceed 3%. This indicates also that the transmissivity of quartz is negligible between 10 and 11 μm. Also, plasma radiation could contribute to background emission, but no significant plasma radiation between 10–11 μm was found. To estimate this potential influence, a movie was recorded with the IR camera, when the ICP is in operation and then switched off. No rapid change of the temperature map was detected in the switch-off moment, indicating that no significant influence of plasma radiation on the measured temperature occurred. According to technical specifications of the IR camera, error of the temperature measurements doesn't exceed 2%.
4 Standard ICP torch at optimal conditions
The spatial distribution of selected emission line intensities in the standard ICP torch at optimal plasma conditions is presented in Fig. 1. All the figures measured by AOS are also available in ESI.† In addition, axial emission line profiles across the plasma (data extracted from Fig. 1a–e at the axis of the torch), a photograph of the ICP and the wall temperature distribution measured by IR thermography are shown. Note the presence of the ICP load coil in the images, which leads to interruptions and artifacts in measurement data (including temperature calculations, see below). These positions are visible in the figures and labeled in Fig. 1f, e.g., in the axial line profiles at z ≈ −18 to −14.7 mm, −11.5 to −8.2 mm, and −4.3 to −0.6 mm above the load coil (ALC). In addition, note that the specific torch geometry (flared end of the torch, see label in Fig. 1b) produces a measurement artifact (reduction/enhancement of detected radiation due to scattering/light guide effect, for example in Fig. 1g) at z ≈ 8 mm ALC. This leads to a corresponding error in the calculated temperatures around 8 mm ALC (see below for details).
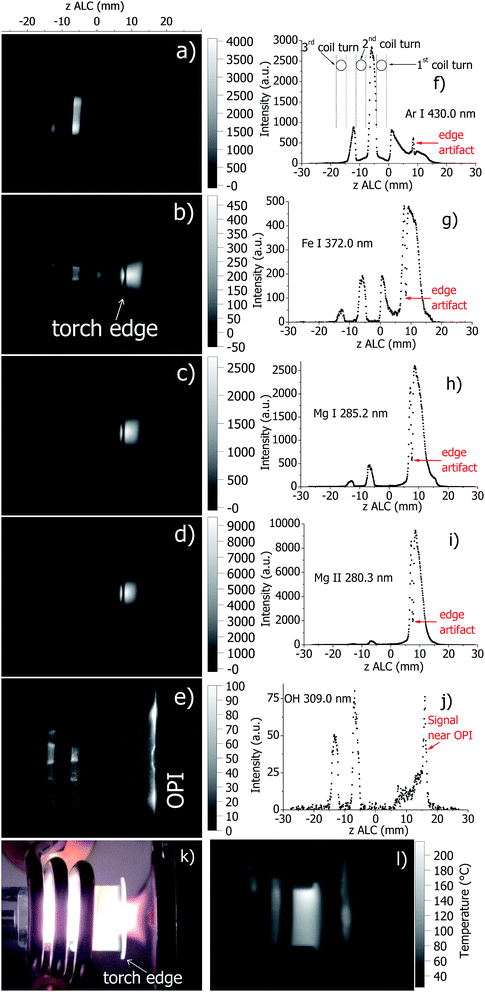 |
| Fig. 1 Spatially resolved images of a standard ICP at selected emission lines detected directly by the AOS ((a): Ar I 430.0 nm, (b): Fe I 372.0 nm, (c): Mg I 285.2 nm, (d): Mg II 280.3 nm, and (e): OH 309.0 nm) and corresponding emission profiles along the axis of the ICP (f–j); a photo of the ICP (k); and a temperature map of the torch wall during plasma operation measured by the IR camera (l). Images (a–e), k and l have similar scale. A standard ICP torch was used at the plasma conditions stated in Table 1. | |
The AOS was successfully used to image the standard ICP. Imaging was completed in approximately 20 min. As expected, atomic emission features in the ICP is maximal in the analytical zone of the ICP, which is positioned downstream at z = 8–15 mm ALC. The onset of emitted radiation from analyte atomic lines (Fe I 372.0 nm, Mg I 285.2 nm) starts inside the torch (−15 mm ALC) and is followed further downstream, i.e. later in time, by the onset of ionic line emission (Mg II 280.3 nm). Ar emission (Ar I 430.0 nm) originates mostly from inside the torch body and is broader in radial direction compared to the analyte emission in the center. This emission pattern (Fig. 1a) is in agreement with the well-known toroidal structure of the ICP. In contrast, molecular emission of OH (309 nm, Fig. 1e) is detected over a wider area inside the torch. This is in agreement with results of ICP simulation,39 where the nebulizer gas flow containing the aerosol recirculates to the entire volume of the ICP. Specifically, there is a distinct central part with enhanced OH emission, which is localized in the region where the Ar injector flow pinches the plasma gas flow. Molecular emission is also detected close to the torch walls. Interestingly, a relatively intense molecular emission between the plasma and the optical interface of the CIROS instrument (cone features become visible) is observed. This is probably due a secondary discharge between the boundary of the ICP and the optical or mass-analyzer interface.40 In general, these findings are in good agreement with the published literature on plasma imaging.
The wall of the standard torch has its maximal temperature near the front edge, which is in agreement with previous measurements.20 Note, however, that in this study the torch temperature at optimal conditions is somewhat lower (≈500 K) than in the previous measurements,20 where the temperature reaches approximately 800 K.
In Fig. 2, axial profiles of plasma temperatures in the standard ICP source (calculated from the measured spectral data) are presented. The standard errors of the Trot and Texc measurements correspond to the deviation of the Boltzmann plots from a straight line and were found to be approximately 7.5% for Trot (coefficient of correlation R2 ≈ 0.967). For Texc the standard error is typically about 6.5% (R2 ≈ 0.986) and reaches 14% in worst case (R2 ≈ 0.885) in areas with low intensity of Fe emission. In general, the magnitudes of Trot, Texc and Te are in agreement with values of Trot, Texc and Te reported elsewhere (see ref. 2 and references therein). However, as discussed above, the method that is used here to calculate Te is considered to be less accurate than the one for the determination of Trot and Texc. Note that there are only two data points for Trot in Fig. 2 (at z = −13 and z = −6 mm ALC). They correspond to the maxima of OH emission in Fig. 1. Unfortunately, it was not possible to calculate Trot in the analytical zone due to the low emission intensity.
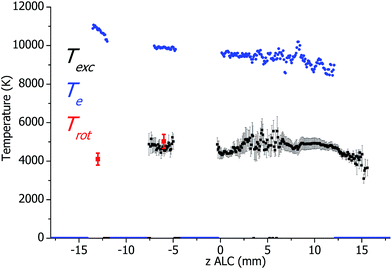 |
| Fig. 2 Axial profiles of plasma temperatures measured for the standard ICP torch at optimal plasma conditions. The spectral lines of OH for determination of Trot were measured by an additional Czerny–Turner monochromator. | |
5 SHIP torch at optimal conditions
In Fig. 3, images and corresponding axial profiles of atomic and molecular emission in the SHIP torch at optimal plasma conditions are presented together with a photograph of the SHIP torch and the wall temperature distribution measured by IR thermography. As mentioned above, the load coil is obstructing parts of the plasma and produces data interruptions in the axial profiles (Fig. 3f). Similarly to the standard torch, molecular emission from OH is observed from a relatively large area inside the torch with a significantly enhanced emission close to the Ar sample carrier gas flow injector. Ar emission (Ar I 430.0 nm) originates from an area between the 1st and the 2nd turn of the load coil (Fig. 3a), which is wide in radial direction and relatively narrow along the axis. The intensity of the Ar line features a local minimum in the center (in radial direction). This finding confirms the previously reported toroidal structure of the plasma in the SHIP torch.19,22 Emission of atomic and ionic analyte species (Fe and Mg) are maximal in the central area between the 1st and the 2nd turn of the load coil. Thus, it can be concluded that the region of most intense analyte emission (analytical zone) in the SHIP torch is situated inside the torch (in contrast to the standard ICP torch) at approximately −6 mm ALC. This is in agreement with the findings reported earlier.22 Also, as reported also earlier,20 the maximal temperature of the SHIP torch wall (≈1200 K) in the current setup is only slightly lower than the melting temperature of quartz (≈1400 K). This also explains the shorter torch lifetime compared to the standard torch. Clearly, these results are very useful as input parameters for optimization of the device and the cooling efficiency by computer modeling of the SHIP torch.
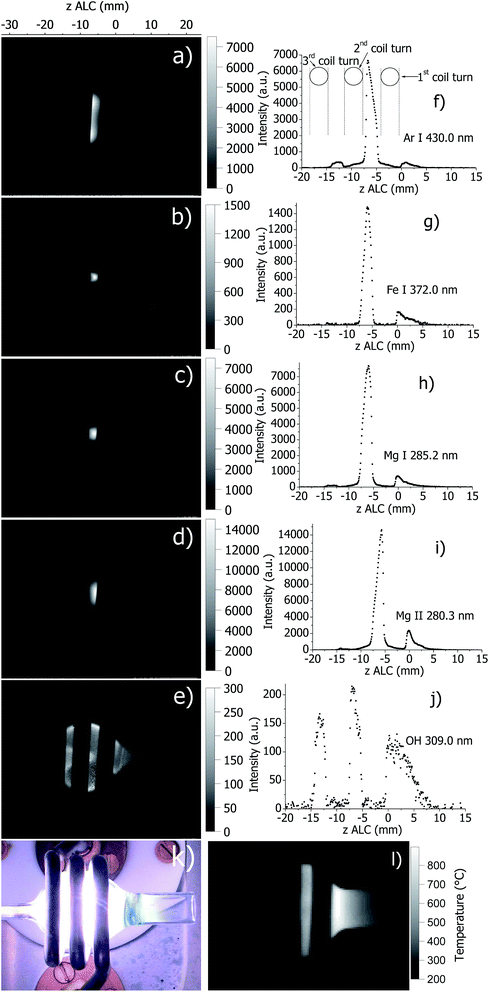 |
| Fig. 3 Spatially resolved images of plasma with SHIP torch at selected emission lines detected directly by the AOS ((a): Ar I 430.0 nm, (b): Fe I 372.0 nm, (c): Mg I 285.2 nm, (d): Mg II 280.3 nm, and (e): OH 309.0 nm) and corresponding emission profiles along the axis of the ICP (f–j); a photo of the ICP (k); and a temperature map of the torch wall during plasma operation measured by the IR camera (l). Images (a–e), k and l have similar scale. The SHIP torch was used at the plasma conditions stated in Table 1. | |
Axial profiles of the plasma temperatures in the SHIP source (calculated from the measured spectral data in Fig. 3) are presented in Fig. 4. Here, Trot is successfully determined over a wide area along the central axis. Again, the standard errors of the Trot and Texc measurements correspond to the deviation of the Boltzmann plots from a straight line and were found to be up to 8% for Trot (coefficient of correlation R2 ≈ 0.962). For Texc the standard error is typically about 6.5% (R2 ≈ 0.982) and sometimes 14% (R2 ≈ 0.885) in plasma zones with low intensity of Fe emission. Both, Trot and Texc ranged from 4000 K to 5000 K, which is comparable to the temperatures in a standard ICP torch. Te in the SHIP torch is approximately 10
500 K, which is similar to Te in the standard torch (about 9500–11
000 K). However, there is a difference between the presented results and results of plasma diagnostics of the SHIP torch published earlier,22 where the following approximate temperatures indicated in the analytical zone: Texc ≈ 9000 K, Trot ≈ 3500 K, Te ≈ 9500 K.
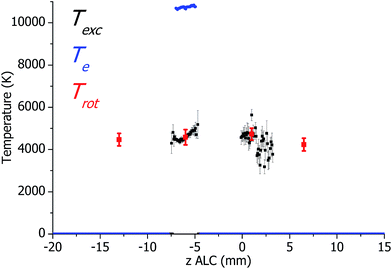 |
| Fig. 4 Axial profiles of plasma temperatures measured from the spectral data. SHIP torch at optimal plasma conditions. Note that spectral lines of OH for determination of Trot were measured by an additional Czerny–Turner monochromator. | |
6 Influence of different plasma parameters on the measured plasma properties
When describing the influence of a parameter, all other parameters were kept constant at optimal conditions according to Table 1, including the set (software indicated) plasma power. However, Pmeas can be influenced by changing parameters and conditions. Therefore, Pmeas is indicated in all figures together with the value of the parameter, which was varied. Because of the large amount of measured data, only those measured properties are presented in the following figures and described in the text, which are significantly different from the values at optimal conditions, which are presented in Fig. 1–4.
Plasma in SHIP and standard torches without sample introduction (dry plasma conditions)
Firstly, dry plasma conditions were investigated without sample introduction. This was achieved in two different ways: (a) the flexible capillary, through which the sample is pumped into the spray chamber, was closed off, and therefore only a dry Ar nebulizer gas flow was passing through the nebulizer; (b) the Ar nebulizer gas flow was set to zero. The corresponding Ar I 430.0 nm line intensity maps, calculated Te and Twall are presented in Fig. 5 and 6 for the SHIP and the standard torch, respectively. In both experiments, the toroidal plasma structure is obtained with a dry Ar nebulizer flow. Conditions change completely (as expected), when nebulizer gas flow was zero and only plasma gas flow was used; the toroidal feature was gone.
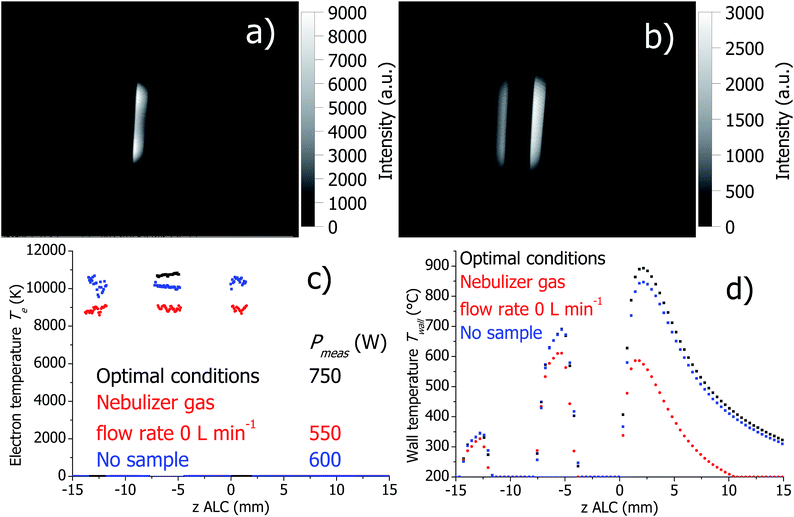 |
| Fig. 5 Distribution of emission intensities of the Ar I 430.0 nm line in the SHIP torch with closed sample tube (a), with no nebulizer gas flow (b), axial profile of Te (c) and Twall (d) at optimal conditions and without sample introduction. | |
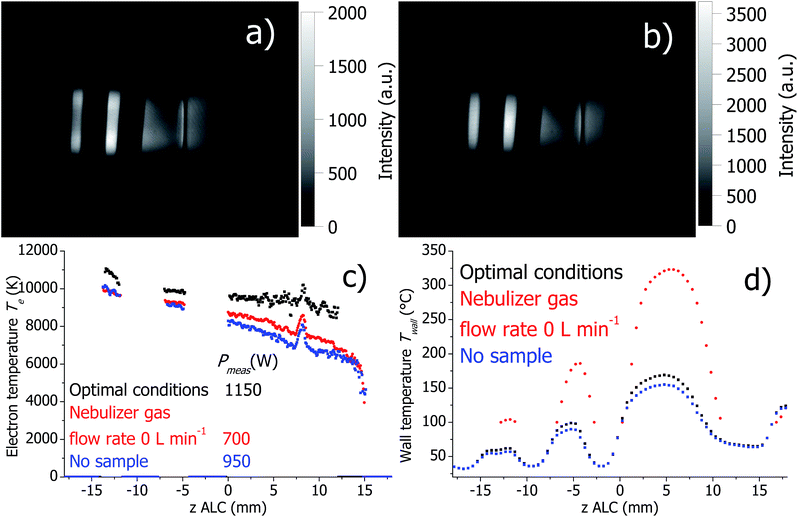 |
| Fig. 6 Distribution of emission intensities of the Ar I 430.0 nm line in standard ICP torch with closed sample tube (a), with no nebulizer gas flow (b), axial profiles of Te (c) and Twall (d) at optimal conditions and without sample introduction. The temperature presented by the red curve in (d) could not be measured below 100 °C due to the used range of the IR camera. | |
In both SHIP and standard torches and no sample introduction, Pmeas is significantly lower compared to conditions with wet aerosol introduction (for the conditions studied here). Independent of the presence of an Ar nebulizer flow, Te decreases in the absence of wet aerosol sample introduction in comparison to optimal conditions. This can be attributed to the decreased Pmeas.
At optimal conditions and with closed sample tube, Twall is similar for both SHIP and standard torches. However, setting the nebulizer gas flow to zero changes Twall significantly. In the SHIP torch, the heat power dissipated into the plasma is removed mostly through the quartz wall. Therefore, the decrease of Twall by about 300 K can be attributed directly to the decrease of Pmeas. In contrast, in the standard torch Twall increases by approximately 150 K when the nebulizer gas flow is off, despite the decrease of Pmeas. Presumably, cooling of the torch walls in the standard torch by the Ar cooling gas flow is less effective without the nebulizer gas flow (note that without the nebulizer flow the total Ar gas flow is reduced by 1 L min−1 of totally 12 L min−1).
Effect of Ar nebulizer flow for the standard torch
The plasma properties at optimal conditions and increased nebulizer gas flow rate are presented in Fig. 7. If the nebulizer gas flow is increased, it is expected that the solvent vapor loading of the plasma is increased, too. However, the intensities of the analyte emission lines decrease significantly in the analytical zone. This is clarified by the fact that the measured Texc decreases significantly if the Ar nebulizer flow rate is increased, probably due to the higher vapor loading. There is also a reduction of the Ar emission. In contrast to the analyte and Ar emission lines, the OH 309.0 nm line intensity increases in the analytical zone significantly.
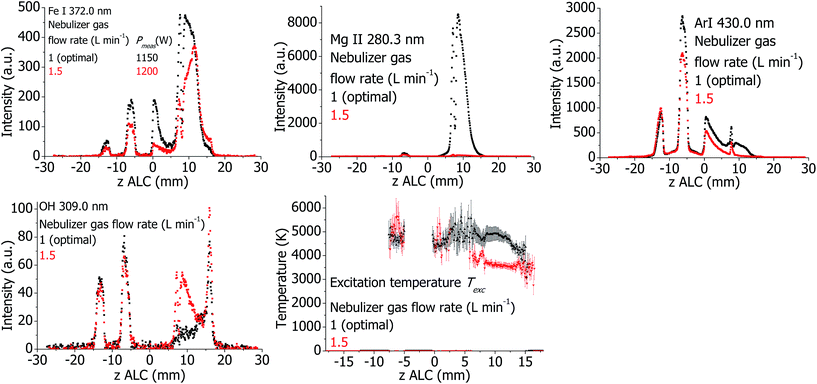 |
| Fig. 7 Plasma properties in the standard torch at different Ar nebulizer flows. | |
Effect of Ar nebulizer flow for the SHIP torch
The plasma properties are presented in Fig. 8. Similarly to the standard torch, the analyte and argon emission intensities decrease if the Ar nebulizer flow rate is increased. The OH emission intensity is increased in the analytical zone. However, no significant change of Texc was detected. At increased Ar nebulizer flow, Twall decreases in the analytical zone and increases at the downstream area. Thus, distribution of the temperature of the wall shifts downstream if the Ar nebulizer flow is increased.
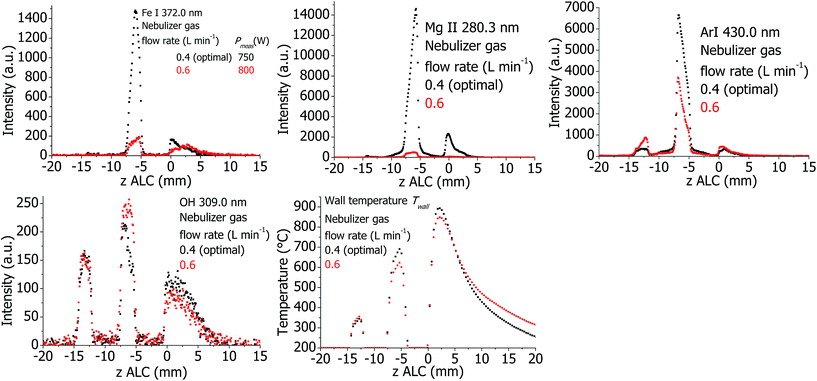 |
| Fig. 8 Plasma properties in the SHIP torch at different Ar nebulizer flows. | |
Effect of Ar auxiliary flow for the standard torch
The plasma properties at different auxiliary gas flows are presented in Fig. 9. Independent of Pmeas, the emission intensities of the Ar I 430.0 nm and the Fe I 372.0 nm lines increase as the auxiliary gas flow is increased; in contrast, Twall decreases by approximately 70 K.
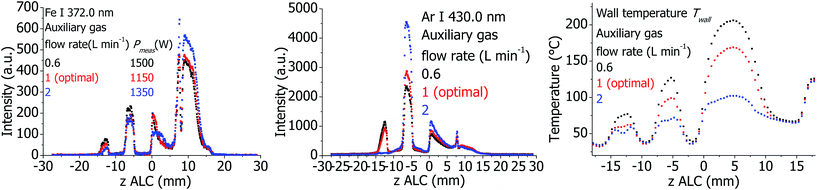 |
| Fig. 9 Plasma properties in the standard torch at different Ar auxiliary flows. | |
Effect of Ar auxiliary flow for SHIP torch
The plasma properties are presented in Fig. 10. Emission intensities of analytes, argon, and to a certain degree molecular emission (OH) decrease if the auxiliary flow is higher than the optimal value. In comparison to optimal conditions, Twall is increased in the analytical zone and decreased in the downstream area if the auxiliary gas flow is increased, shifting in this way the temperature distribution upstream. This behavior seems opposite to what would be expected. So far, the reason for this finding is not clear and should be studied in detail in the future.
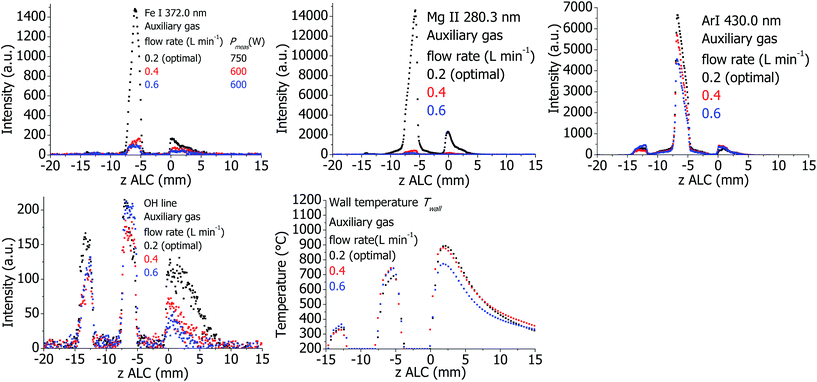 |
| Fig. 10 Plasma properties in the SHIP torch at different Ar auxiliary gas flow rate. | |
Effect of Ar cooling flow for the standard torch
The plasma properties are presented in Fig. 11. If the cooling Ar flow is increased from 12 L min−1 (optimal condition) to 20 L min−1, Texc decreases significantly in the analytical zone. Accordingly, the intensities of analyte and Ar emission lines decrease. High total gas flow rates lead to a better cooling of the torch, which is visible in the Twall values. In contrast, the emission from molecular species (OH line) in the analytical zone increases.
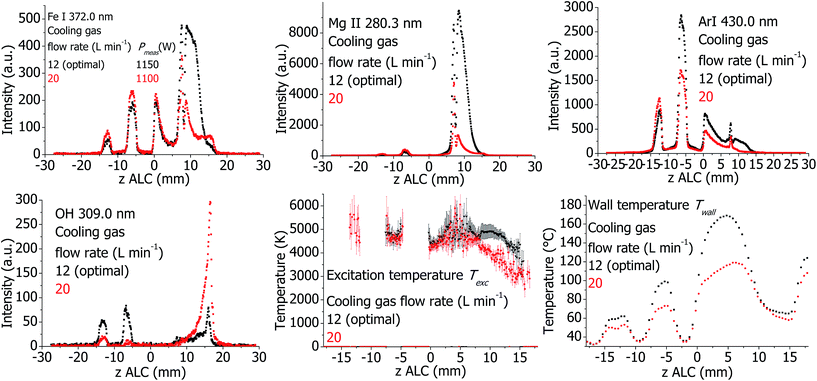 |
| Fig. 11 Plasma properties in the standard torch at different Ar cooling gas flow rate. | |
Effect of external air cooling flow for the SHIP torch
The plasma properties are presented in Fig. 12. The influence of air cooling in the SHIP torch is less significant than that of Ar cooling in the standard one: only the intensity of the Mg II 280.3 nm line changes moderately in the analytical zone. Intensity of the OH line in the downstream area is maximal at optimal plasma conditions and decreases at both more and less intensive air cooling. There is also a distinct decrease of Twall at higher air cooling flows.
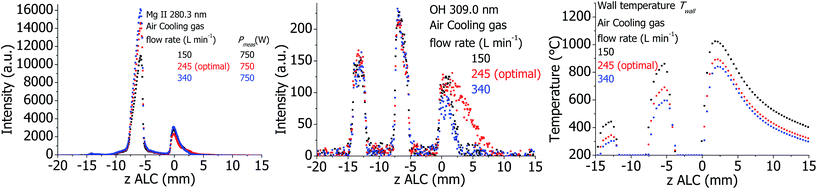 |
| Fig. 12 Plasma properties in the SHIP torch at different external air cooling gas flow rate. | |
Effect of RF power for the standard and SHIP torches
The plasma properties in the standard and SHIP torches are presented in Fig. 13 and 14, respectively. As expected, all investigated emission line intensities and Twall values increase towards higher RF power. This cannot be confirmed for the measured plasma temperatures. Only changes for Texc in the standard torch and Trot in the SHIP torch are visible. In the SHIP torch, both Texc and Te do not change significantly, probably because the Pmeas was varied little for this torch.
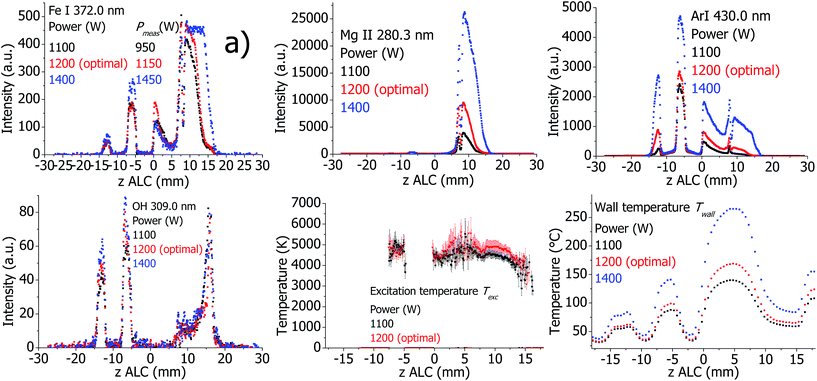 |
| Fig. 13 Plasma properties in the standard torch at different applied power values. At an indicated power of 1400 W some Fe spectral lines were detected with saturation of the detector in the analytical zone (see (a)). Therefore Texc is not presented for 1400 W. | |
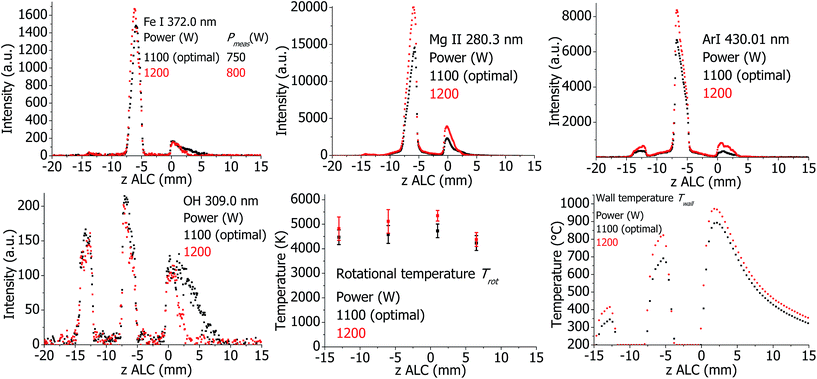 |
| Fig. 14 Plasma properties in SHIP torch at different applied power values. | |
Effect of the optical interface (OPI) for the standard torch
The OPI provides a cooled boundary for the plasma and additionally introduces a cold Ar counterflow into the plasma. To investigate the effect of the OPI, the Ar counterflow was varied when the OPI is at optimal position at 20 mm ALC. Additionally, the distance between torch and OPI was maximized to 45 mm ALC with switched off counterflow to achieve a plasma with possibly minimal influence of the OPI. The plasma properties are presented in Fig. 15. Not surprisingly, the analyte line intensities and Texc vary at z = 10.20 mm ALC, which is the edge of the analytical zone. With the minimal possible influence of the OPI (the torch is far from the OPI without the counterflow), the plasma is hottest and brightest in this area. The line emission is extended until approximately 20 mm ALC, which is 25 mm far from the OPI, indicating no influence of the OPI on the plasma in this case. If the OPI is at optimal distance, but the counterflow is still switched off, then Texc and analyte lines emission decrease at z above approximately 14 mm ALC. Thus, the presence of OPI without the counterflow influences the plasma properties at the edge of the analytical zone. If the Ar counterflow is further increased, Texc decreases additionally. The cooling of the plasma is accompanied by a corresponding reduction of the analyte line intensities at z = 10.15 mm ALC. Thus, the presence of the OPI and, in more degree, the presence of the counterflow reduce the length of the analytical zone. This is in agreement with experimental work of Gamez et al.,41 where a decrease of the electron and gas kinetic temperatures in presence of a mass-spectral interface was detected.
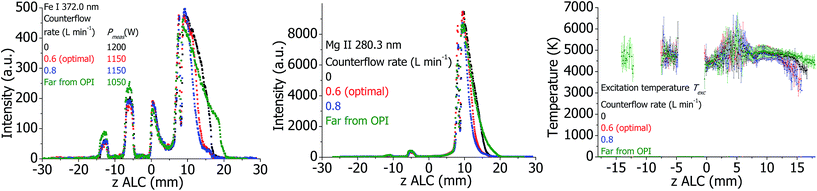 |
| Fig. 15 Plasma properties in the standard torch at different values of Ar counterflow, and with the torch positioned far from the optical interface. | |
Survey observations
In the standard torch, changes of the Ar nebulizer flow (Fig. 7), cooling flow (Fig. 11), plasma power (Fig. 13) and Ar counterflow (Fig. 15) lead to a significant change of both absolute intensities of Fe lines and their relation, which lead also to a visible change of Texc. If the Ar auxiliary flow in the standard torch is varied (see Fig. 9) only a moderate change of Fe line intensities is detected without a change of Texc. Therefore, there is a correlation of Texc with the analyte line intensities in the standard torch, and influence of the operational conditions on line intensities is more than the influence on Texc. This is expected if the plasma is in LTE conditions. In the SHIP torch, a significant change of the line intensities is not accompanied by a change of Trot or Texc, see Fig. 8 (change of Ar nebulizer flow), Fig. 10 (change of auxiliary gas flow) and Fig. 12 (change of external air cooling flow). This indicates that the plasma in the SHIP torch deviates more from LTE.
In both plasma sources, Te changes if the sample introduction is removed (see Fig. 5 and 6). In contrast, Te changes not significantly, if any other parameter is varied. Hence, Te is not depicted in the other figures presented. Therefore, the electrons are not in LTE with the other plasma species, which is also confirmed by the fact that Trot and Texc are significantly lower than Te. Also, Pmeas differs significantly with and without sample introduction. From these measurements it can be concluded that the plasma properties with and without sample introduction are quite different.
If a plasma parameter is changed, the emission intensity of the OH line in the analytical zone often changes in the opposite direction as the emission intensity of the analyte and Ar lines (see Fig. 7, 8 and 11), or does almost not change (see Fig. 10, 12 and 14). A possible reason for this is an increased decay of OH radicals, which can be expected, if the plasma power increases, providing in this way enhanced emission of the analyte lines.
7 Conclusions
In the first part of this study, the fundamental properties of two different ICP sources (a commonly used Fassel-type torch operated at 12–20 L min−1 and a low-argon-flow torch operated at 0.6 L min−1) were studied: emission of Ar, OH and analyte lines (Fe and Mg); rotational temperature, excitation temperature, and electron temperature; temperature of the torch walls; voltage and current in the load coil as well as plasma power. All the optical measurements were carried out with lateral resolution. For most of them, a novel acousto-optical imaging spectrometer33 was used, which has unique parameters (wavelength range: 250–800 nm, spectral resolution: 0.05–0.5 nm, time resolution: 5 ns, change of wavelength within 0.2 ms, spatial resolution: >125 μm) and is suitable for a wide range of fundamental and applied investigations.
The influence of different operational parameters on the measured plasma properties was studied. It was observed that almost every parameter changed the analyte and plasma emission: introduction of sample; Ar nebulizer, auxiliary, cooling flows (in case of the Fassel-type ICP torch), external air cooling flow (SHIP torch), plasma power, presence of an optical interface and the Ar counter flow (in standard ICP torch), respectively. A large experimental data set was obtained for the two plasma sources operated in the same instrument, which can now be used for a comparison with results from numerical plasma models (see second part of this study). Although only 2–3 measurements were carried out to vary each individual parameter, it is enough to reveal the existing tendencies.
It was confirmed that the toroidal structure of the plasma, which is well known in the standard ICP torch, exists also in the SHIP torch.19 Further, it was observed that the plasma is not toroidal in the absence of the nebulizer Ar flow: the central channel is not formed without the nebulizer gas flow. In both torches, Te is significantly higher than the other plasma temperatures, confirming that the electrons are not in LTE with other plasma species. Thus, the plasma in both torches deviates from LTE conditions. However, based on the data reported here, it is assumed that deviations from LTE are greater in the SHIP torch as compared to the standard torch.
Acknowledgements
Financial support from the German Research Foundation, grant No. BU 1140/5-1, HO 2062/9-1 is gratefully acknowledged.
References
- M. Voronov, V. Hoffmann, W. Buscher and C. Engelhard, J. Anal. At. Spectrom., 2017 10.1039/c6ja00192k.
-
Inductively Coupled Plasma in Analytical Atomic Spectrometry, ed. A. Montaser and D. W. Golightly, VCH Publishers, New York, 2nd edn, 1992 Search PubMed.
- S. Greenfield, I. L. Jones and C. T. Berry, Analyst, 1964, 89, 713–730 RSC.
- R. H. Wendt and V. A. Fassel, Anal. Chem., 1965, 37, 920–922 CrossRef CAS.
-
S. Greenfield and A. Montaser, Common RF Generators, Torches, and Sample Introduction Systems, in Inductively Coupled Plasmas in Analytical Atomic Spectrometry, ed. A. Montaser and D. W. Golightly, VCH Publishers, Inc., New York, 1992, pp. 187–247 Search PubMed.
- R. N. Savage and G. M. Hieftje, Anal. Chem., 1979, 51(3), 408–413 CrossRef CAS.
- A. D. Weiss, R. N. Savage and G. M. Hieftje, Anal. Chim. Acta, 1981, 124, 245–258 CrossRef CAS.
- R. Rezaaiyaan, G. M. Hieftje, H. Anderson, H. Kaiser and B. Meddings, Appl. Spectrosc., 1982, 36(6), 627–631 CrossRef CAS.
- R. Rezaaiyaan and G. M. Hieftje, Anal. Chim. Acta, 1985, 173, 63–75 CrossRef CAS.
- C. D. Allemand and R. M. Barnes, Appl. Spectrosc., 1977, 31(5), 434–443 CrossRef CAS.
- C. D. Allemand, R. M. Barnes and C. L. Wohlers, Anal. Chem., 1979, 51(14), 2392–2394 CrossRef CAS.
- P. S. C. van der Plas and L. de Galan, Spectrochim. Acta, Part B, 1984, 39, 1161–1169 CrossRef.
- P. S. C. van der Plas, A. C. de Waaij and L. de Galan, Spectrochim. Acta, Part B, 1985, 40, 1457–1466 CrossRef.
- H. Kawaguchi, T. Ito, S. Rubi and A. Mizuike, Anal. Chem., 1980, 52, 2440–2442 CrossRef CAS.
- G. R. Kornblum, W. Van der Waa and L. de Galan, Anal. Chem., 1979, 51(14), 2378–2381 CrossRef CAS.
- A. A. Bol'shakov and R. M. Barnes, Spectrochim. Acta, Part B, 1997, 52, 2127–2150 CrossRef.
- A. Klostermeier, C. Engelhard, S. Evers, M. Sperling and W. Buscher, J. Anal. At. Spectrom., 2005, 20, 308–314 RSC.
- A. Scheffer, R. Brandt, C. Engelhard, S. Evers, N. Jakubowski and W. Buscher, J. Anal. At. Spectrom., 2006, 21, 197–200 RSC.
- C. Engelhard, A. Scheffer, S. Nowak, T. Vielhaber and W. Buscher, Anal. Chim. Acta, 2007, 583, 319–325 CrossRef CAS PubMed.
- C. Engelhard, A. Scheffer, T. Maue, G. M. Hieftje and W. Buscher, Spectrochim. Acta, Part B, 2007, 62, 1161–1168 CrossRef.
- A. Scheffer, C. Engelhard, M. Sperling and W. Buscher, Anal. Bioanal. Chem., 2007, 388, 1605–1613 CrossRef CAS PubMed.
- C. Engelhard, G. C.-Y. Chan, G. Gamez, W. Buscher and G. M. Hieftje, Spectrochim. Acta, Part B, 2008, 63, 619–629 CrossRef.
- C. Engelhard, T. Vielhaber, A. Scheffer, M. Brocksieper, W. Buscher and U. Karst, J. Anal. At. Spectrom., 2008, 23, 407–411 RSC.
- T. Pfeifer, R. Janzen, T. Steingrobe, M. Sperling, B. Franze, C. Engelhard and W. Buscher, Spectrochim. Acta, Part B, 2012, 76, 48–55 CrossRef CAS.
- S. Nowak, M. Gesell, M. Holtkamp, A. Scheffer, M. Sperling, U. Karst and W. Buscher, Talanta, 2014, 129, 575–578 CrossRef CAS PubMed.
- T. Steingrobe, A.-C. Niehoff, B. Franze, D. Lenhard, H. Pietsch, C. Engelhard, U. Karst and W. Buscher, J. Anal. At. Spectrom., 2015, 30, 2120–2124 RSC.
- S. Nowak, J. Künnemeyer, L. Terborg, S. Trümpler, A. Günsel, G. A. Wiesmüller, U. Karst and W. Buscher, Anal. Bioanal. Chem., 2015, 407, 1023–1026 CrossRef CAS PubMed.
- M. Voronov, V. Hoffmann, D. Birus, C. Engelhard and W. Buscher, J. Anal. At. Spectrom., 2015, 30, 2089–2098 RSC.
-
T. Hasegawa, M. Umemoto, H. Haraguchi, C. Hsiech and A. Montaser, Fundamental properties of inductively coupled plasma, ed. Inductively Coupled Plasmas in Analytical Atomic Spectrometry, in A. Montaser and D. W. Golightly, VCH Publishers, Inc., New York, 1992, pp. 373–449 Search PubMed.
- I. Ishii and A. Montaser, Spectrochim. Acta, Part B, 1991, 46, 1197–1206 CrossRef.
- G. J. Bastiaans and R. A. Mangold, Spectrochim. Acta, Part B, 1985, 40, 885–892 CrossRef.
- A. Batal, J. Jarosz and J. M. Mermet, Spectrochim. Acta, Part B, 1981, 36, 983–992 CrossRef.
- M. Voronov, V. Hoffmann, T. Wallendorf, S. Marke, J. Mönch, C. Engelhard, W. Buscher, S. J. Ray and G. M. Hieftje, J. Anal. At. Spectrom., 2012, 27, 419–425 RSC.
- R. Álvarez, A. Rodero and M. C. Quintero, Spectrochim. Acta, Part B, 2002, 57, 1665–1680 CrossRef.
- G. C. Y. Chan and G. M. Hieftje, Spectrochim. Acta, Part B, 2005, 60, 1486–1501 CrossRef.
- G. C. Y. Chan and G. M. Hieftje, Spectrochim. Acta, Part B, 2006, 61, 31–41 CrossRef.
- N. Furuta and G. Horlick, Spectrochim. Acta, Part B, 1982, 37, 53–64 CrossRef.
- M. W. Blades and G. Horlick, Appl. Spectrosc., 1980, 34, 696–699 CrossRef CAS.
- M. Aghaei, L. Flamigni, H. Lindner, D. Günther and A. Bogaerts, J. Anal. At. Spectrom., 2014, 29, 249–261 RSC.
- H. Niu and R. S. Houk, Spectrochim. Acta, Part B, 1996, 51, 779–815 CrossRef.
- G. Gamez, S. A. Lehn, M. Huang and G. M. Hieftje, Spectrochim. Acta, Part B, 2007, 62, 357–369 CrossRef.
Footnote |
† Electronic supplementary information (ESI) available. See DOI: 10.1039/c6ja00191b |
|
This journal is © The Royal Society of Chemistry 2017 |
Click here to see how this site uses Cookies. View our privacy policy here.