DOI:
10.1039/C4RA15804K
(Review Article)
RSC Adv., 2015,
5, 17283-17295
Photon conversion in lanthanide-doped powder phosphors: concepts and applications
Received
4th December 2014
, Accepted 28th January 2015
First published on 28th January 2015
Abstract
The investigation of luminescent lanthanide doped nanostructures (LDNs) has attracted a great deal of interest in the last decades. This is a multidisciplinary research field in which scientific challenges as well as societal needs are addressed by chemists, physicists, and materials scientists. In this scenario, research groups involved in developing novel LDNs have concentrated their efforts on engineering structures with superior luminescence quantum efficiencies for photonics applications. In this article, we briefly present the strategy that we have been using to develop nanocrystalline lanthanide-doped powders with superior luminescence yield using the combustion synthesis technique. Furthermore, the use of these lanthanide-doped powders as up-conversion phosphors in temperature sensing, lighting and solar cell technologies is discussed.
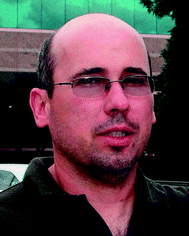 Glauco S. Maciel | Glauco S. Maciel received his D.Sc. degree in Physics from Universidade Federal de Pernambuco, Brazil, in 1999. He was a post-doc fellow in the Chemistry Department of the State University of New York at Buffalo, USA, in 1999–2000. He joined the faculty of the Physics Department of Universidade Federal de Pernambuco in 2002. In 2006 he moved to the Physics Institute of the Universidade Federal Fluminense, Brazil. His main research interest is focused on luminescent nanomaterials for photonics applications. |
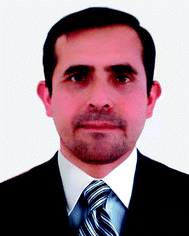 Nikifor Rakov | Nikifor Rakov received his D.Sc. degree in Physics from Universidade Federal de Pernambuco, Brazil, in 2001. He was a post-doc fellow in the Universidad Nacional Autónoma de Mexico in 2002–2004. He joined the faculty of the Mechanical Engineering Department in the Materials Sciences Division of Universidade Federal do Vale do São Francisco, Brazil, in 2004. His main research interests are in synthesis and optical spectroscopy of luminescent nanomaterials. |
1. Introduction
Photon conversion is generally produced by light–matter interactions in which modifications of the refractive index and nonlinear absorption processes are induced by high-power coherent light sources, i.e. short-pulse lasers, in crystalline bulky materials and glassy fibers.1 In recent years, nonlinear optical processes have regained interest, as we move toward miniaturization. There has been great effort in developing novel nanomaterials for photon conversion with the most popular being semiconductor quantum dots,2 organic molecules,3 and lanthanide doped nanostructures (LDNs).4 Compared to the other listed compounds, LDNs have a better photochemical stability, lower toxicity, narrower emission bands with larger anti-Stokes shifts (covering a broad spectral range: from the near-infrared to the ultraviolet), longer luminescence lifetime and the unique possibility to show multi-photon conversion when low-power diode lasers are used as excitation sources. However, LDNs generally have lower luminescence quantum efficiency (LQE) when compared to the other nanostructured systems mentioned above.
The most common oxidation state found for lanthanides ions in condensed matter is +3. Optically active electrons are found in 4f band with parity-forbidden 4f–4f radiative electronic transitions dominating the spectra at the visible and near-infrared regions. Because of the parity-forbidden nature of the 4f–4f transitions, the oscillator strength and as a consequence the luminescence yield generated by photon conversion is not high. It is accepted that these transitions occur because of the admixture of electronic wave functions of state 4f with states of opposite parity mediated by odd-parity crystal field components.5 Therefore the choice of the host material is of very importance to the strength of the 4f-intraband electronic transitions and as a consequence to the luminescence. Another important characteristic of the host material is that it must present low-energy phonons to guarantee small luminescence quench due to nonradiative loss.6 Luminescence can also be enhanced by manipulating the doping concentration of the lanthanide species but above critical values complex interaction mechanisms between the lanthanides, such as energy transfer, may quench the luminescence.7 On the other hand, energy transfer mechanisms may be helpful to enhance the luminescence when different lanthanide species playing the roles of sensitizer/energy donor and activator/energy acceptor are present in the host.8 Detrimental energy transfer mechanisms can be controlled by letting the doping concentration of the lanthanide ion fixed while changing the concentration of an impurity. Impurities may introduce lattice distortions around the lanthanide ions and dissociate lanthanide clusters by increasing the distance between them with the consequence of enhancing the luminescence.9
Different from semiconductor quantum dots, LDNs do not present quantum confinement effects on the electronic states of lanthanide ions but electron–phonon interactions are highly influenced by the particles size/morphology10 and surface impurities states.11 As the size of the particle decreases, the importance of the luminescence from lanthanide ions at the surface of the particles increases and the luminescence becomes highly sensitive to the host parameters and the synthesis route. Conventionally, solid–state reaction12 has been used to prepare lanthanide doped powders due to its simplicity. Unfortunately, this method produces powders with poor homogeneity and large particles (size of tens of micrometers). Solution-based also known as wet chemical methods have been received considerable attention since they offer the possibilities for controlling homogeneity, purity of phase, size distribution and surface area of the phosphors. The mostly popular wet chemical methods are hydrothermal,13 sol–gel,14 co-precipitation15 and combustion.16 Combustion synthesis has received attention because it is a low cost technique (it uses open furnaces or hot plates operating at relatively low temperatures) that produces several grams of nano and microcrystalline ceramic powders in the as-synthesized state. This is possible due to the high internal temperature generated during the exothermic combustion reaction.
Concerning photonics applications, bulky crystalline and glassy materials doped with lanthanide ions have been extensively investigated in the past for use in laser media,17 optical signal amplifiers,18 displays,19 optical refrigerators,20 optical storage of information21 and temperature sensing.22 LDNs as compact powders are highly light scattering media and the use of such systems in light propagating phenomena such as stimulated emission and amplification is undermined. However, LDNs have found potential use in environmentally friendly lighting industry,23 tissue and cell imaging,24 temperature25 and radiation26 sensing, and solar cell technology.27
In this review, we summarize our recent results on lanthanide doped powders prepared by combustion synthesis and their application in temperature sensing, lighting devices and solar cell technology using photon conversion mechanisms. Firstly, a discussion about photon conversion mechanisms is presented in the next section.
2. Photon conversion mechanisms involving lanthanide ions is solids
Photon conversion may be obtained by refractive or absorptive processes. For the particular case of lanthanide ions, photons are absorbed and subsequent emission of longer wavelength (down-conversion) or shorter wavelength (up-conversion) photons, a photoluminescence process, occurs. While down-conversion pathways are simple to describe, up-conversion processes may have origin in different mechanisms. Fig. 1 shows the most common down- and up-conversion processes.
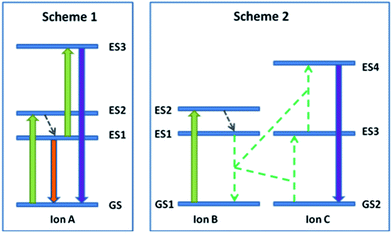 |
| Fig. 1 Photon conversion mechanisms in lanthanides ions involving single ions (Scheme 1) and pairs of different species (Scheme 2). | |
Scheme 1 shows both down- and up-conversion processes that may occur in individual ions. First, one photon with energy matching the band gap between the electronic ground state (GS) and the excited state (ES) 2 is absorbed by an ion at GS promoting it to ES2. Internal multi-phonon assisted nonradiative relaxation produced by electron–lattice interactions generates phonons (vibration modes whose energies match the electronic gap between the electronic states) and promotes electronic population jump from state ES2 to state ES1. After that, two events may occur: (a) photon emission originating from the electronic relaxation from ES1 to GS; (b) absorption of a second incoming photon promoting the ion to a higher lying electronic state ES3. The photon generated by event “a” has energy smaller than that of the absorbed photon and this process is known as down-conversion. The event “b” has many possible subsequent photon conversion processes: (a) a down-converted photon originating from the electronic relaxation from ES3 to ES2, (b) emission of a photon of energy equal to the incident ones corresponding to the electronic transition from ES3 to ES1, and (c) more energetic photons produced by electronic relaxation from ES3 to GS. The process involving production of photons more energetic than the incident ones is known as up-conversion. Photon up-conversion may also occur due to interactions between lanthanide ions in close proximity in the host lattice. These interactions between “neighbour” ions are called energy transfer mechanisms and they are strongly dependent on the relative distance between the ions in the lattice. Energy transfer mechanisms28 occur generally through multipolar interactions but exchange interactions dominates at very short distances (less than 5 Å). A simple representation of an energy transfer assisted up-conversion process is depicted in Scheme 2 of Fig. 1. In this case, initially both ions are at their electronic ground-state but only ion “B” can absorb photons from the excitation source. Ions “B” and “C” cannot produce photon up-conversion individually, under this specific excitation scheme, but when these ions are in close proximity in the lattice ion “B” may transfer its accumulated energy to ion “C”. This energy transfer process occurs under certain energy resonance conditions. For the specific case shown in Scheme 2, the energy gap ES1–GS1 must match the energy gaps GS2–ES3 and ES3–ES4. This is a two-step energy transfer process in which firstly the excited ion “B” undergoes an electronic relaxation from ES1 to GS1 while ion “C” is promoted from GS2 to ES3. Secondly, another excitation photon is absorbed by ion “B” with subsequent relaxation to donor level ES1. If the reload of population at level ES1 is fast enough, i.e. in a time interval shorter than the lifetime of intermediate state ES3, another energy transfer occurs between ions “B” and “C”. This second energy transfer promotes ion “C” to level ES4 while takes ion “B” back to GS1. Finally, radiative electronic relaxation from ES4 to GS2 may occur generating an up-converted photon. A variation of this scheme involves two excited ions “B” simultaneously transferring their accumulated energies from ES1 states to an ion “C” at GS2. In this case, ion “C” is directly promoted from GS2 to ES4. However, this energy transfer process involves three ions (two ions “B” and one ion “C”) and therefore it is less probable than the process that involves only two ions (one ion “B” and one ion “C”). Note that while the down-conversion process is a linear optical process (one emitted photon per absorbed photon), the up-conversion process is nonlinear (one emitted photon per two absorbed photons) and needs a larger amount of excitation photons. There are more complex up-conversion processes involving absorption of more than two excitation photons29 but they follow the same principles illustrated in Fig. 1.
The energy transfer process shown in Scheme 2 of Fig. 1 is called cooperative up-conversion as each ion of different species cannot separately generate photons with energy higher than the incident photons. However, when a high concentration of ions of the same species is present in the host material, energy transfer mechanisms can occur between ions of the same species and these interactions can be beneficial or detrimental to the up-conversion production. Let us take as an example another ion “A” with its energy level diagram shown in Fig. 2. Note that individually no up-conversion can be produced because the energy of the excitation photon does not match with any energy gap between excited states (the excitation photon is resonant only with the energy gap between GS and ES2). Note though that there are many matches between different excited state energy gaps. For example, the energy gap between ES4 and ES2 is equal to the energy gap between ES2 and ES1. In this case, if there are two ions populated at excited state ES2 in close proximity in the host lattice (neighbouring ions), they can exchange energy in such a way that one ion is promoted to ES4 state while the other ion suffers de-excitation to state ES1. Then, the ion populated at ES4 returns to GS after ejection of an up-converted photon. This photon conversion mechanism is called energy transfer assisted up-conversion (ETAU). The basic problem with such a pair system is that the up-conversion process may be quenched as well by other energy transfer mechanisms. Fig. 2 shows an example in which an excited ion at state ES4 is in close proximity with an unexcited ion. The excited ion may lose part of its electronic energy through an energy transfer channel in which it is de-excited from ES4 to ES3 while the other ion is promoted from GS to ES1. In the process, the production of up-converted photons originating from ES4 is quenched. This energy transfer mechanism is called cross-relaxation (CR). The rate in which energy is transferred between the donor and the acceptor ions can be calculated based on rate-equation models, as will be exemplified later in the text.
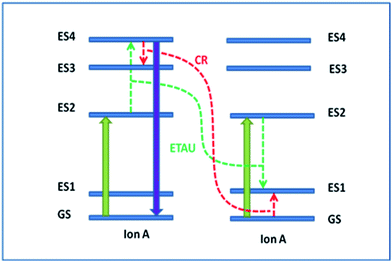 |
| Fig. 2 Up-conversion mechanism in pairs of lanthanides ions of the same species. | |
3. Sample preparation by combustion synthesis
The preparation of the samples has to take into consideration a balanced mixture between the amount of fuel (reducing element) and the amount of metal nitrates (oxidizers) to generate maximum energy release during the reaction. A stoichiometric composition means that the ratio between the metal nitrates and the fuel must produce a complete reaction without residual fuel or metal nitrates after the reaction takes place. The stoichiometry of metal nitrates–fuel mixture can be expressed in terms of the elemental stoichiometric coefficient (ϕe). The value of ϕe is equal to one for a stoichiometrically balanced composition. If ϕe < 1 the mixture is fuel rich and if ϕe > 1 the mixture is fuel lean.30
As an example, we will discuss the production of yttrium silicate powder samples synthesized by combustion method using urea and glycine as reducing agents in accordance with the following stoichiometrically balanced reaction equations:
|
2Y(NO3)3 + 1SiO2 + 5CH4N2O → 1Y2SiO5 + 10H2O + 8N2 + 5CO2
| (1) |
|
6Y(NO3)3 + 3SiO2 + 10C2H5NO2 → 3Y2SiO5 + 25H2O + 14N2 + 20CO2
| (2) |
The process involves the mixture of hydrated yttrium nitrate, fumed silica and the fuel in a proper molar ratio with a small amount of deionized water. The solution is kept under constant stirring for about 10 min and then it is placed in muffle furnace pre-heated at 500 °C. After a few minutes the following reaction steps occur: dehydration, decomposition and burn. Aqueous vapor and gases (N2 and CO2) are eliminated during the process. The burning process, which generates internal temperatures higher than 1800 °C, results in a foamy homogeneous powder. The powder sample prepared with glycine was darker (like ashes) than the powder sample prepared with urea. A heat-treatment process eliminates surface residues and increase crystallization as will be shown shortly in the text. Both powders were crushed and heat-treated at higher temperatures (typically 900–1200 °C) for a few hours with a heating rate of 200 °C h−1. The annealed powders were left to cool down naturally to room temperature. Both powder samples became white after heat-treatment. We found that the ideal temperature to obtain phase X1-Y2SiO5 was 1100 °C. For temperatures above 1100 °C, the formation of phase X2-Y2SiO5 dominates. Temperatures below 900 °C do not give full crystallization. The X-ray powder diffraction patterns of Y2SiO5 powders prepared using glycine and urea are shown in Fig. 3. The as-prepared samples show poor crystallization and the sample prepared with glycine presents less defined diffraction peaks indicating that the internal flame temperature generated during the exothermic combustion reaction must have been higher when urea was used as the fuel agent. After heat-treatment, both samples present high degree of crystallization and there is no major difference among samples heat-treated for 1 or 3 hours or among samples prepared with glycine or urea.
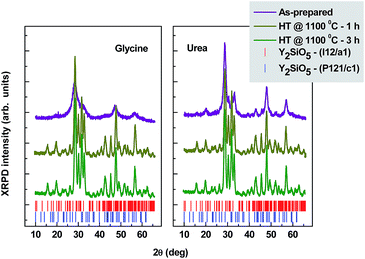 |
| Fig. 3 X-ray powder diffraction patterns of yttrium silicate powders prepared by combustion synthesis using two different fuels. HT = Heat-treatment temperature. | |
Morphological analysis obtained with a scanning electron microscope is shown in Fig. 4. Both samples present particles with large surface areas (micrometer-scale) and high porosity due to the drastic character of the combustion synthesis reaction. Sample prepared with glycine (left image) presents smoother surfaces while sample prepared with urea (right image) has a more irregular surface area with a large amount of smaller particles (sub-micrometer-scale) aggregated to the surface of the bigger particles.
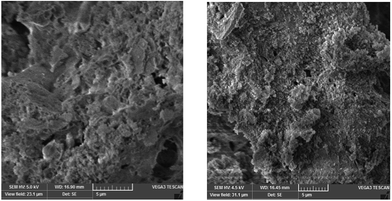 |
| Fig. 4 Scanning electron microscope images of yttrium silicate powders prepared by combustion synthesis using two different fuels, glycine (left) and urea (right). The full scale bar is 5 μm. | |
4. Luminescence quantum efficiency measurements in compact powders
The general definition of Stokes, or down-converted, LQE (η) involves the ratio between the number of emitted photons and the number of absorbed photons.31 Electronic relaxation can occur via radiative or nonradiative decay and therefore the quantum efficiency is expected to be the highest (η = 1) if the nonradiative decay channel is suppressed. This approach can be inaccurate in powders due to the large light scattering and an alternative way to estimate the LQE is by measuring the luminescence lifetime. In this case, η is expressed by the product of the radiative emission rate and the luminescence lifetime.32 For trivalent lanthanide ions in solids, the radiative emission rate may be associated mainly to 4f–4f forced electric dipole and magnetic dipole transitions:33 |
 | (3) |
where Δ is the energy band gap (in cm−1 units) between the αJ and α′J′ states (α and α′ represent all the quantum numbers except the total angular momentum associated with the electronic states involved in the transition) and n is the index of refraction. The contribution of the forced electric dipole and magnetic dipole to the transition between αJ and α′J′ states are given by DE and DM, respectively, with the forced electric dipole contribution being written as: |
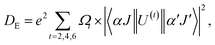 | (4) |
where Ωt are the Judd–Ofelt (JO) standard intensity parameters and U(t) are the reduced matrix elements of unit tensor operator of rank t calculated from intermediate coupling approximation.33 The JO standard intensity parameters are related to the static (nonrelativistic) radial integrals and odd parts of the crystal field potential. Therefore, the crystal field symmetry has a strong influence on the radiative emission rate and as a consequence the LQE. The reduced matrix elements contain the angular part of the wave functions for the αJ and α′J′ states and they can be calculated.33 These elements vary little with the host matrix and the tabulated values may be generally used.34 The JO standard intensity parameters can be estimated experimentally from the best-fit values of the line strengths in the absorption spectra32 but in powder samples the absorption data is not accurate due to strong light scattering. An alternative method to estimate the LQE using the JO standard intensity parameters in powder samples involves the use of the luminescence spectrum of trivalent europium (Eu3+). The JO standard intensity parameters can be calculated by analyzing the integrated emission intensities (areas below the luminescence bands) and the equation:35 |
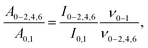 | (5) |
where I0–J′ is the integrated emission intensity and ν0–J′ is the transition frequency of transition 5D0 → 7FJ′ (J′ = 1,2,4,6). The magnetic dipole transition 5D0 → 7F1 is almost insensitive to the host symmetry properties and it has a radiative emission rate with an average value of ≈50 s−1.36 The radiative emission rates A0–2,4,6 are related to forced electric dipole transitions and they may be written as a function solely of the JO intensity parameters. The nonzero squared reduced matrix elements are solely U(2) for 5D0 → 7F2, U(4) for 5D0 → 7F4 and U(6) for 5D0 → 7F6.34 Thus, the values of Ω2, Ω4 and Ω6 can be found straightforwardly by use of (3) and (4) in (5). It is important to point out that the JO intensity parameters have information concerning chemical bonding and local crystalline structure. For example, the Ω2 intensity parameter generally reflects symmetry distortions and the degree of covalency between the lanthanide ions and the ligands anions. Our results in yttrium oxyfluoride powders showed that the values of Ω2 increased with the doping concentration of Eu3+ in rhombohedral YOF and orthorhombic Y6O5F8 powders prepared by combustion synthesis.37 This indicates that the inclusion of Eu3+ produces lattice distortions probably due to the difference in ionic radius between europium and yttrium. We also found that the values of Ω2 are larger for the rhombohedral YOF powders compared to the values found for the orthorhombic Y6O5F8 powders, comparing samples with the same Eu3+ doping concentration. This is a reasonable result as the rhombohedral phase is less symmetric than the orthorhombic phase. The JO parameters Ω4 and Ω6 are more complex but it is generally accepted that they are related to the rigidity of the network. Our results on rhombohedral YOF and orthorhombic Y6O5F8 powders suggested stronger rigidity for samples containing a high level of Eu3+ concentration.
Once the values of Ω2,4,6 are known, they can be used to estimate the radiative emission rates A0–2,4,6 of 5D0 state of Eu3+. The total radiative emission rate (Arad) is obtained by summing up all the A0–J parameters. The luminescence lifetime (τ) is estimated by fitting a theoretical curve to the decay of the down-converted luminescence signal that follows UV excitation produced by a short pulse laser.37,38 Once the luminescence lifetime and the radiative emission rate are estimated, the LQE can be found by use of η = τ × Arad. The values found for powder samples prepared by combustion synthesis are shown in Table 1.
Table 1 LQE for Eu3+ (1 wt%) doped in various hosts
Host |
Al2O3 |
Y2O3 |
Y2SiO5 |
Y6O5F8 |
Lu2SiO5 |
η (%) |
19 |
40 |
49 |
53 |
55 |
It has been proposed that the up-conversion LQE could be defined as the number of emitted photons at the visible divided by the number of absorbed photons at the near-infrared. In this case, the up-conversion LQE may be written as a function of the down-converted LQE, η, as:39
|
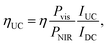 | (6) |
where
Pvis and
PNIR are the absorbed powers for direct and nonlinear light excitation, respectively. The luminescence intensities under direct and nonlinear excitation are represented by
IUC and
IDC, respectively. As in the case of down-converted luminescence, light absorption and emission measurements are difficult to estimate with accuracy in highly scattering media such as powder samples. An interesting alternative approach that has been used to measure the LQE in both down- and up-converted luminescence from lanthanide doped powders involves the use of commercially available fluorimeters and integrating spheres.
40 In this case, it is necessary to calibrate the responses of the monochromators, photodetectors and integrating sphere before the measurements. The use of a reference sample (undoped powder) has to be used for calibration as well.
5. Strategies to increase the up-conversion luminescence quantum efficiency by co-doping with other lanthanides
There are different strategies to improve the up-conversion luminescence yield of lanthanide doped materials. The most commonly used approaches involve surface plasmon resonance with the inclusion of metallic nanoparticles to increase luminescent yield through near field effects,41 the engineering of core–shell structures to suppress low luminescence associated to nonradiative losses at surface states caused by impurities and discontinuity in lattice periodicity,42 and co-doping with other metals to (a) introduce lattice distortions around the lanthanide ions and (b) control the energy transfer by manipulation of the spatial separation in the lattice.43 However, the most studied mechanism to improve LQE of lanthanide doped materials is by co-doping with different species of lanthanide ions. Many lanthanide ions present weak up-conversion emission and in this scenario their application as efficient up-conversion phosphors is limited. As discussed in Section 2, another lanthanide ion may be used to improve the up-conversion efficiency by acting as a sensitizer. Its role is to (a) improve light absorption from the excitation source and (b) enhance population at the up-conversion activator ion by efficiently donating its accumulated energy. A well-known near-infrared sensitizer is ytterbium (Yb3+) because it has the largest absorption cross-section among the trivalent lanthanide ions at the near-infrared around 1 μm. One trivalent lanthanide ion that benefits from efficient energy transfer from Yb3+ is praseodymium (Pr3+) as it is shown in Fig. 5 for aluminum oxide powder samples.
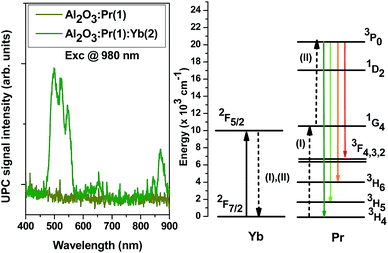 |
| Fig. 5 (Left) up-conversion emission spectra of aluminium oxide powders prepared by combustion synthesis. The numbers between parentheses are wt% doping concentrations of the trivalent lanthanide ions. (Right) scheme of up-conversion involving praseodymium and ytterbium ions. | |
A tremendous enhancement of the up-conversion signal, corresponding to Pr3+ 4f–4f transitions 3P0 → 3H4 (497 nm), 3P0 → 3H5 (525 and 550 nm), 3P0 → 3H6 (620 nm) and 3P0 → 3F2 (650 nm), is clearly observed. The emission band at the near-infrared (800–900 nm) is associated to defect states.44 In this case, up-conversion is produced by a two-step energy transfer process. First, an excited Yb3+ ion transfer its energy to a neighboring Pr3+ ion at the ground-state (dotted line “I” in Fig. 5). Then, a second near-infrared photon is absorbed by the Yb3+ ion and a second Yb → Pr energy transfer occurs promoting the Pr3+ ion to state 3P0 (dotted line “II” in Fig. 5). A second possibility involves simultaneous energy transfer from two excited Yb3+ ions to a neighboring Pr3+ ion at the ground-state. This second possibility has a higher probability to occur as the Yb3+ doping concentration increases as there will be more sensitizers close to the activator ion in the lattice. The two possibilities can be discriminated by the analysis of the dynamics of the up-conversion signal intensity. Sequential energy transfer will generate a slower build-up of the up-conversion signal intensity compared to the simultaneous energy transfer process.
While other lanthanide ions such as erbium (Er3+), holmium (Ho3+), and thulium (Tm3+) have benefited from co-doping with Yb3+,45 special attention has been given to europium (Eu3+) and terbium (Tb3+) because they conventionally generate bright green and red light, respectively, under UV excitation.46 Up-conversion, on the other hand, is very difficult to achieve in these elements using excitation sources of moderate power, such as near-infrared diode lasers, as there are no intermediate 4f levels to absorb photons in the wavelength range between ∼2 μm and ∼600 nm. In this case, it is necessary to co-dope the material with another lanthanide ion (the sensitizer). In most of the cases, Yb3+ is the ion of choice and in this case, Tb3+ and Eu3+ emit their characteristic luminescence by cooperative energy transfer from pairs of excited Yb3+ ions.47 Fig. 6 shows the down-conversion and cooperative up-conversion luminescence spectra of Eu3+ and Tb3+ co-doped with Yb3+ in yttrium silicate powders under, respectively, UV lamp (255 nm) and near-infrared (975 nm) diode laser excitation.
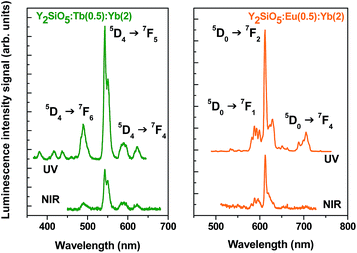 |
| Fig. 6 Characteristic down- and up-conversion spectra of Tb3+ and Eu3+ doped in yttrium silicate powders. The excitation wavelengths were 255 nm (UV) and 975 nm (NIR). The numbers between parentheses are wt% doping concentrations of the trivalent lanthanide ions. | |
Note that the cooperative up-conversion luminescence spectra are similar to the down-conversion luminescence spectra but they are weaker in intensity due to the nonlinear nature of the up-conversion process. Luminescence produced by cooperative up-conversion is depicted in Fig. 7 for both Tb3+–Yb3+ and Eu3+–Yb3+ co-doped systems.
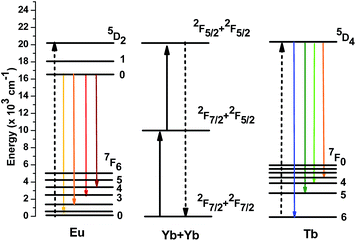 |
| Fig. 7 Scheme showing the cooperative up-conversion emission in europium and terbium ions due to energy transfer (dotted lines) from pairs of excited ytterbium ions. The most relevant emission lines from europium and terbium are shown as downward solid arrows and the excitation at the near-infrared region is shown as upward solid lines. | |
In both cases, it is necessary a pair of excited Yb3+ ions to transfer, simultaneously, their energy to a nearby Tb3+, or Eu3+ in order to generate luminescence at the visible.
The energy transfer rate from pairs of Yb3+ to Eu3+, or Tb3+, can be estimated by analyzing the dynamics of the cooperative up-conversion luminescence and the time evolution of the electronic populations involved in the process:48
|
 | (7) |
|
 | (8) |
|
 | (9) |
|
 | (10) |
where
Ng,
N0,
Ne and
N1 are the most relevant levels involved in the cooperative up-conversion process. They are assigned to the ground states of Yb
3+ and Eu
3+(Tb
3+), excited state
2F
5/2 of Yb
3+ and excited state
5D
0(
5D
4) of Eu
3+(Tb
3+), respectively. The pump rate
R is given by
σI/
hν where
σ is the Yb
3+ absorption cross-section (
2F
7/2 →
2F
5/2) given by 2.3 × 10
−20 cm
2,
48 I is the pump intensity at the sample surface and
hν is the pump photon energy. The
2F
5/2 and
5D
0(
5D
4) lifetimes,
τe and
τ1 respectively, are taken from the Stokes luminescence generated by direct excitation of the emitting level or by excitation of some energetically close higher lying state, as is the case of
5D
1(
5D
3) of Eu
3+(Tb
3+) using pulsed (typically a few ns duration) lasers.
Fig. 8 shows the fit of
(10) with the time evolution of the cooperative up-conversion luminescence signal at 611 nm for a sample containing 1.0
![[thin space (1/6-em)]](https://www.rsc.org/images/entities/char_2009.gif)
:
![[thin space (1/6-em)]](https://www.rsc.org/images/entities/char_2009.gif)
1.0 (wt%) of Yb
3+![[thin space (1/6-em)]](https://www.rsc.org/images/entities/char_2009.gif)
:
![[thin space (1/6-em)]](https://www.rsc.org/images/entities/char_2009.gif)
Eu
3+ and an excitation intensity
I = 2.6 × 10
6 W cm
−2 at 975 nm. The excited state lifetimes
τe and
τ1 were found as 0.9 and 2.1 ms, respectively. Therefore, the only fitting parameter is the energy transfer rate and the best-fit value found for this particular sample is shown in the figure. We found that the value of
k increased from 700 to 850 s
−1 when the concentration of Yb
3+ was doubled while keeping the concentration of Eu
3+ fixed indicating that the distance between the donor and acceptor ions decreased.
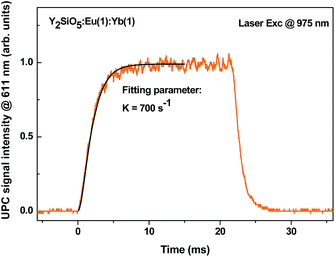 |
| Fig. 8 Up-conversion luminescence dynamics and fitting curve using the rate equation for the time evolution of the electronic population of level 5D0 of europium in yttrium silicate powder. The numbers between parentheses are wt% doping concentrations of the trivalent lanthanide ions. | |
The use of Yb3+ or other sensitizers may also cause additional effects in the up-conversion luminescence signal of the activator lanthanide ion, as will be discussed in Section 6.1.
6. Photonics applications for lanthanide doped powders
6.1 Color tuning and white light production
Trivalent erbium ion (Er3+) is a very convenient up-conversion activator due to its regular spacing between 4f electronic states. Therefore, it is possible to achieve UV and visible luminescence with a single-wavelength excitation source in the near-infrared.49 Another fine characteristic of Er3+ is that its 4f excited electronic state 4I11/9 has the same energy of the 4f excited state 2F5/2 of Yb3+ favoring energy transfer from Yb3+, which has a large absorption cross-section, to Er3+. The consequence of that is a strong enhancement of the Er3+ up-conversion intensity but besides that a perceived color change is observed (Fig. 9). The two major emission bands are associated to 4f–4f Er3+ transitions 2H11/2, 4S3/2 → 4I15/2 (520–575 nm) and 4F9/2 → 4I15/2 (650–690 nm). Digital photographs taken from the samples in the dark room show that the addition of Yb3+ produces a perceived color change of Er3+ up-conversion luminescence from green to yellow. Indeed, a large gamut of colors can be achieved by addition of other lanthanide ions. For example, thulium (Tm3+) doped powders can emit bright up-conversion blue light when co-doped with sensitizers. Tri-doping the host crystalline material with Tm3+, Er3+ and Yb3+ has produced white light when a careful balance between the doping concentrations is obtained.50
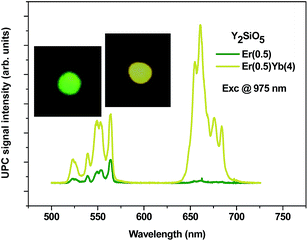 |
| Fig. 9 Up-conversion luminescence spectra of two samples of yttrium silicate powder excited with 975 nm diode laser. The numbers between parentheses are wt% doping concentrations of the trivalent lanthanide ions. The inset shows digital photographs of the samples (to facilitate identification, the emission spectra are shown in the same color of the photographs). | |
White light has been produced by down-conversion mechanisms in Eu3+, Tb3+ co-doped nanocrystalline powders using UV light as the excitation source of choice51 but white light generated by up-conversion has a greater appeal when one deals with unwanted human exposure to UV light. White light has also been reported in Eu3+ singly doped powder samples using UV light and low doping concentrations of Eu3+ (ref. 52) and near-infrared diode laser excitation in vacuum atmosphere.53
Applications of luminescent lanthanide doped powders ranging from environmentally friendly lighting to solar cells and displays generally involve deposition of the lanthanide doped powder in a transparent layer. When the luminescence is transmitted through the layer, particular attention has to be taken to perceived color modifications due to reabsorption of luminescence, a phenomenon known as inner filter effect. We observed this phenomenon in the up-conversion emission spectra of Er3+ highly doped yttrium oxide (Y2O3) close-packed powders prepared by combustion synthesis.54 The inner filter effect was suppressed by the inclusion of free-standing undoped Y2O3 nanoparticles. We also observed that the differences among the reflected and transmitted green emission band had not been completely eliminated with the inclusion of the spacers. We believe that this effect may be due to Rayleigh scattering that is stronger at smaller wavelengths (scaling with λ−4). One proposed solution to eliminate the scattering problem may be the elimination of the refractive index mismatch produced by the air gap between the particles by the injection of an index-matching nonabsorbing polymer solution into the voids of the compacted powder.
The control over the color emitted by the up-conversion phosphor may be obtained by other parameters besides co-doping. The choice of the host material can also produce changes in the perceived emitted color. Fig. 10 shows the up-conversion emission spectra of Er3+ in samples containing Tm3+, which usually acts as a sensitizer of radiation at 800 nm, in two different hosts. Note that the emission profile is strikingly different in spite of the same lanthanides doping concentrations for both samples. As a consequence, the perceived color is also different being bright yellow for Y2O3 host and orange-reddish for YOF host.
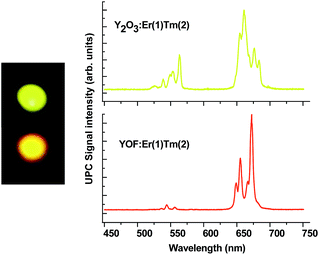 |
| Fig. 10 Up-conversion luminescence spectra of two samples: yttrium oxide and yttrium oxyfluoride doped with erbium and thulium ions. The powders were excited with 975 nm diode laser. The numbers between parentheses are wt% doping concentrations of the trivalent lanthanide ions. The digital photographs on the left show the apparent color of the samples illuminated in the dark. | |
6.2 Optical temperature sensing
The luminescence from lanthanide doped materials have been used as an interesting solution to collect information about environment temperature in hostile sites (e.g. containing high magnetic fields) and small dimensions (microelectronic devices and cellular ambient).55 The most widespread method used for optical temperature sensing is based on the measurement of the ratio between the luminescence intensities from two closely spaced 4f electronic states which are thermally coupled and are assumed to be in a thermodynamical quasi-equilibrium.56 Various lanthanides have been used as luminescent probes of environment temperature57 but the most studied lanthanide element for this specific application is Er3+, which has generally been coupled with Yb3+ to enhance the near-infrared to visible up-conversion efficiency, as discussed earlier in the text. Concerning its temperature sensing characteristics, green up-conversion emission from 2H11/2 and 4S3/2 of Er3+ is the most studied case. In the last years, advances in materials engineering and microscopy techniques have made possible advances towards temperature monitoring at a nanoscale. In this scenario, lanthanide doped nanocrystalline powders have been extensively investigated. The sensitivity of the sensor, defined as the ratio in which the luminescence intensity ratio changes with the temperature, has been identified as the standard figure-of-merit and it has been optimized by a careful choice of the host material.58 Fig. 11(a) shows the up-conversion spectra of an Er3+, Yb3+ co-doped Y2SiO5 powder recorded at room temperature and in contact to a heated plate set at 350 °C. The characteristic band-shape change expected from thermally coupled electronic multiplets is clearly observed. Fig. 11(b) shows the monolog plot of the luminescence intensity ratio (LIR) versus the inverse absolute temperature. The luminescence intensities of the two transitions were obtained by integration over a specified bandwidth centered at the peak positions to simulate luminescence detection through band-pass optical filters in a two-channel signal division electronic system. The data follows a straight line, as expected from a luminescent system that contains two states with a Boltzmann distribution of populations:55–59 |
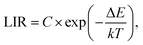 | (11) |
where k is the Boltzmann constant, T is the absolute temperature, and C is a constant that contains information about the luminescence collection efficiency, the emission frequency, level degeneracy, and the spontaneous emission rate of the two thermally coupled states. The linear best-fit provides a value for the barycenter of the energy band gap (ΔE) between the two thermally coupled states and this value may be used to estimate the sensitivity of the system, defined as:59 |
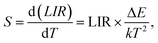 | (12) |
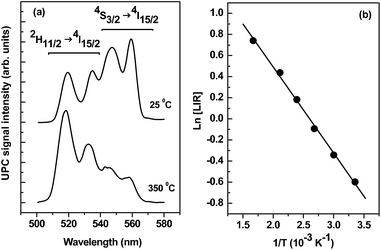 |
| Fig. 11 (a) Up-conversion luminescence spectra of Er3+, Yb3+ co-doped Y2SiO5 powder taken at two different environment temperatures. (b) Monolog plot of the luminescence intensity ratio as a function of the inverse absolute temperature. The solid line represents the linear best-fit function. | |
However, it has been shown recently that the internal heat generated by the strong absorption of the laser light associated to poor heat dissipation may give misleading readings of the sensor sensitivity in Er3+-doped compact powders.60 Fig. 12 shows the results for yttrium silicate powders doped with Er3+ and co-doped with Tm3+ under near-infrared 800 nm diode laser excitation. The environment temperature during the experiment was 25 °C. Note that when the sample doped with Er3+ (bottom of the figure) is exposed to a higher excitation power level its up-conversion spectral profile changes in a way similar to the one shown in Fig. 11 obtained when the sample was externally heated up. In Fig. 12, the heating was generated internally by strong laser absorption and poor thermal dissipation. Note that when combined with an appropriate doping concentration of Tm3+, the heating effect disappears (top of the figure). The phenomenon may be explained in terms of Er3+ → Tm3+ energy transfer mechanisms that reduce the number of phonons locally activated by nonradiative excited state relaxation in Er3+ sites.61
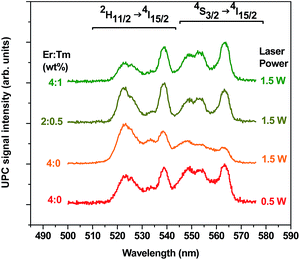 |
| Fig. 12 Up-conversion luminescence spectra of Er3+ doped and Er3+, Tm3+ co-doped Y2SiO5 powders subjected to near-infrared (800 nm) laser excitation. The spectra were obtained at room temperature. | |
6.3 Photon conversion for solar cells
Lanthanide doped nanocrystalline materials have been studied as energy converters for use in crystalline silicon (c-Si) based solar cells.62 A common problem regarding energy solar harvesting in c-Si based solar cells is associated to lattice thermal losses and transparency losses. These losses can be mitigated by adding layers containing light converters. One phenomenon that has been extensively studied for this particular application is quantum cutting (QC).63 QC is a process in which high energy photons are absorbed by the lanthanide ion and two photons are emitted in the longer wavelength spectral region. This process may achieve an efficiency that can be much higher than 100%, as the number of photons emitted has a theoretical limit of twice the number of absorbed photons. The QC mechanism may involve one, two, or more ions. In all cases, the choice of the host material with small phonon energy and the manipulation of the lanthanide doping concentrations are factors of paramount importance. Strümpel and co-workers64 discussed that the visible to near-infrared photon conversion is the most appropriate QC process for solar cells based on c-Si (band gap of 1.12 eV) and in this case samples containing Yb3+ and other lanthanide ions seem to be the best choice. With its single electronic excited state in the 4f-shell and relatively large energy gap between the excited state and the ground state, around 1.25 eV, Yb3+ has the largest near-infrared luminescence quantum efficiency. However, individual Yb3+ ions do not produce QC as photons with twice the energy of its near-infrared energy gap cannot be absorbed. The most common solution that has been employed involves the use of a sensitizer for absorption of green/blue photons. Generally, cerium trivalent (Ce3+) and europium divalent (Eu2+) are the best sensitizers of choice as both ions have a broad absorption band at the green/blue region due to 4f–5d electronic transitions. These electronic transitions are parity allowed and therefore they have higher oscillator strength than the parity-forbidden 4f–4f transitions. The QC process is obtained after efficient energy transfer between the sensitizer and Yb3+ ions. The proposed energy transfer scheme is shown in Fig. 13. This cooperative energy transfer scheme is similar to the one described in Section 5 among Yb3+ pairs and Eu3+/Tb3+ but in this case Yb3+ acts as energy acceptors. It is important to point out that the energy transfer efficiency from Ce3+ to Yb3+ pairs depends strongly on the location of the 5d band.
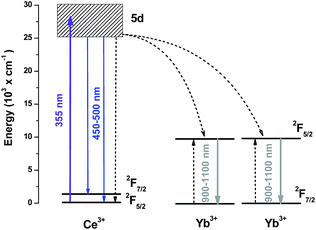 |
| Fig. 13 Quantum cutting via energy transfer (dotted lines) from Ce3+ ions to pairs of Yb3+ ions. Upward and downward solid arrows represent absorption and radiative emission, respectively. | |
The energy transfer mechanism is confirmed by the analysis of the spectral and temporal profiles of the luminescence. Fig. 14 shows the luminescence spectra spanning the visible and near-infrared region for yttrium silicate powders prepared by combustion synthesis. As expected, the presence of Yb3+ produces a quench of the blue-green luminescence originating from Ce3+. Note also that the sample doped only with Yb3+ does not show any luminescence for excitation at 355 nm and that the near-infrared luminescence of Yb3+ increases with its concentration. As expected from an energy transfer process, the luminescence lifetime of the donor ion is shortened in the presence of the acceptor ion (inset of Fig. 14).
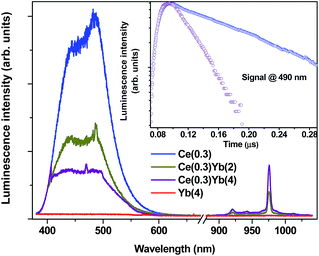 |
| Fig. 14 Luminescence spectra of yttrium silicate powders doped with Ce3+ and Yb3+ irradiated with UV (355 nm) light. The numbers between parentheses represent wt% doping concentrations of the trivalent lanthanide ions. Inset: Ce3+ luminescence dynamics recorded at 490 nm for samples without and with Yb3+ ions. The excitation source was a pulsed (5 ns) laser at 355 nm. | |
The Ce–Yb QC efficiency can be estimated by use of the following equation:65
|
 | (13) |
where the intensity of the Ce
3+ luminescence peaked at 490 nm measured for samples without Yb
3+ and with the highest Yb
3+ concentration before saturation of the near-infrared luminescence are represented by
ICe(
t) and
ICe–Yb(
t), respectively.
Eqn (13) is valid when it is assumed that all excited acceptor and residual excited donor ions decay radiatively.
66 This is reasonable as the large energy gap between 5d band and
2F
J states of Ce
3+ and between
2F
5/2 and
2F
7/2 states of Yb
3+ prohibits nonradiative (multiphonon) relaxation to occur. The QC efficiency found for our sample containing 0.3 wt% of Ce and 4 wt% of Yb in Y
2SiO
5 was 160%, which is around the same value recently reported in multi component oxide glass.
67
7. Summary and outlook
In this review we summarized our investigation on luminescent lanthanide-doped powders prepared by combustion synthesis for photonics applications. We presented the strategies that we have been using to optimize the luminescence quantum efficiency and control the emitted color through co-doping and manipulation of the host constituents. The applicability of the lanthanide-doped powders in all-optical devices such as optical temperature sensors, illumination panels (lamps and displays) and c-Si based solar cells using photon conversion mechanisms was also presented.
Lanthanide-doped nanocrystalline powders have been extensively studied in the last decades from the fundamental view point of understanding atomic interactions and photon conversion mechanisms to the technological challenges of modern photonic devices. This is an exciting field of research that has attracted attention of materials scientists, physicists, chemists, engineers, and biologists with exciting possibilities to be explored in the years, or decades, to come.
Acknowledgements
We would like to thank Prof. Renato Guimarães (Universidade Federal Fluminense) and Prof. Isabel Carvalho (PUC-Rio) for their valuable contribution in some of our work published on the subject in the last years. The work presented here was partially supported by the Brazilian agencies Conselho Nacional de Desenvolvimento Científico e Tecnológico (CNPq) and Fundação de Amparo à Pesquisa do Estado da Bahia (FAPESB).
Notes and references
- R. Boyd, Nonlinear Optics, Academic Press, USA, 1998 Search PubMed; Handbook of Nonlinear Optical Crystals, ed. V. G. Dmitriev, G. G. Gurzadyan and D. N. Nikogosyan, Springer, Germany, 1999 Search PubMed; G. Agrawal, Nonlinear Fiber Optics, Academic Press, USA, 2006 Search PubMed.
- L. M. Maestro, E. M. Rodríguez, F. S. Rodríguez, M. C. Iglesias-de la Cruz, A. Juarranz, R. Naccache, F. Vetrone, D. Jaque, J. A. Capobianco and J. G. Solé, Nano Lett., 2010, 10, 5109 CrossRef CAS PubMed; J. Tang and R. A. Marcus, J. Chem. Phys., 2005, 123, 054704 CrossRef PubMed; A. D. Yoffe, Adv. Phys., 2001, 50, 1 CrossRef; M. Bruchez Jr, M. Morronne, P. Gin, S. Weiss and A. P. Alivisatos, Science, 1998, 281, 2013 CrossRef; L. E. Brus, J. Chem. Phys., 1984, 98, 3575 CrossRef.
- M. Pawlicki, H. A. Collins, R. G. Denning and H. L. Anderson, Angew. Chem., Int. Ed., 2009, 48, 3244 CrossRef CAS PubMed; G. S. He, P. P. Markowicz, T. C. Lin and P. N. Prasad, Nature, 2002, 415, 767 CrossRef PubMed; B. R. Cho, K. H. Son, S. H. Lee, Y. S. Song, Y. K. Lee, S. J. Jeon, J. H. Choi, H. Lee and M. Cho, J. Am. Chem. Soc., 2001, 123, 10039 CrossRef PubMed; B. A. Reinhardt, L. L. Brott, S. J. Clarson, A. G. Dillard, J. C. Bhatt, R. Kannan, L. X. Yuan, G. S. He and P. N. Prasad, Chem. Mater., 1998, 10, 1863 CrossRef.
- X. C. Ye, J. E. Collins, Y. J. Kang, J. Chen, D. T. N. Chen, A. G. Yodh and C. B. Murray, Proc. Natl. Acad. Sci. U. S. A., 2010, 107, 22430 CrossRef CAS PubMed; G. S. Yi, H. C. Lu, S. Y. Zhao, G. Yue, W. J. Yang, D. P. Chen and L. H. Guo, Nano Lett., 2004, 4, 2191 CrossRef.
- Optical Spectra of Transparent Rare Earth Compounds, ed. S. Hufner, Academic Press, USA, 1978 Search PubMed.
- Mid-Infrared Coherent Sources and Applications, ed. M. Ebrahim-Zadeh and I. T. Sorokina, Springer, Netherlands, 2008 Search PubMed.
- A. Lakshmanan, Luminescence and Display Phosphors: Phenomena and Applications, Nova Science Pub Inc., USA, 2007 Search PubMed.
- F. Auzel, Chem. Rev., 2004, 104, 139 CrossRef CAS PubMed.
- S. Yuan, H. Zeng, X. Wu, Z. Liu, J. Ren, G. Chen, Z. Wang and L. Sun, RSC Adv., 2014, 4, 16710 RSC; G. D. Webler, M. J. M. Zapata, G. S. Maciel, A. Patra, J. M. Hickmann and M. A. R. C. Alencar, J. Nanomater., 2014, 708719 Search PubMed; H. Yu and J. C. Yu, J. Lumin., 2013, 137, 274 CrossRef CAS PubMed; R. Okram and N. R. Singh, Prog. Nanotechnol. Nanomater., 2013, 2, 26 Search PubMed.
- X. Bai, H. Song, L. Yu, L. Yang, Z. Liu, G. Pan, S. Lu, X. Ren, Y. Lei and L. Fan, J. Phys. Chem. B, 2005, 109, 15236 CrossRef CAS PubMed; H. S. Yang, K. S. Hong, S. P. Feofilov, B. M Tissue, R. S. Meltzer and W. M. Dennis, J. Lumin., 1999, 83–84, 139 CrossRef.
- F. Wang, J. Wang and X. Liu, Angew. Chem., Int. Ed., 2010, 49, 7456 CrossRef CAS PubMed; H. X. Mai, Y. W. Zhang, L. D. Sun and C. H. Yan, J. Phys. Chem. C, 2007, 111, 13721 Search PubMed.
- L. Li, S. H. Zhou and S. Y. Zhang, Chem. Phys. Lett., 2008, 453, 283 CrossRef CAS PubMed; Y. S. Tang, S. F. Hu, C. C. Lin, N. C. Bagkar and R. S. Liu, Appl. Phys. Lett., 2007, 90, 151108 CrossRef PubMed; S. Neeraj, N. Kijima and A. K. Cheetham, Solid State Commun., 2004, 131, 65 CrossRef PubMed.
- L. S. Liu, H. H. Chen, B. Q. Liu, H. Zhang, B. Tang, X. J. Feng, Z. J. Sun and J. T. Zhao, J. Rare Earths, 2014, 32, 686 CrossRef CAS; X. Li, J. Yang, P. P. Yang, H. Z. Lian and J. Lin, Chem. Mater., 2008, 20, 4317 CrossRef; M. Yoshimura and S. C. Somiya, Mater. Chem. Phys., 1999, 61, 1 CrossRef.
- V. Venkatramu, S. F. León-Luis, U. R. Rodríguez-Mendoza, V. Monteseguro, F. J. Manjón, A. D. Lozano-Gorrín, R. Valiente, D. Navarro-Urrios, C. K. Jayasankar, A. Muñozd and V. Lavín, J. Mater. Chem., 2012, 22, 13788 RSC; K. K. Rao, T. Banu, M. Vithal, G. Y. S. K. Swamy and K. R. Kumar, Mater. Lett., 2002, 54, 205 CrossRef CAS.
- G. Q. Chai, G. P. Dong, J. R. Qiu, Q. Y. Zhang and Z. M. Yang, Sci. Rep., 2013, 3, 1598 Search PubMed; X. R. Hou, S. M Zhou, Y. K. Li and W. J. Li, J. Alloys Compd., 2010, 494, 382 CrossRef CAS PubMed; A. Mech, M. Karbowiak, L. Kepinski, A. Bednarkiewicz and W. Strek, J. Alloys Compd., 2004, 380, 315 CrossRef PubMed.
- S. Mukherjee, V. Sudarsan, R. K. Vatsa and A. K. Tyagi, J. Lumin., 2009, 129, 69 CrossRef CAS PubMed; R. V. Mangalaraja, J. Mouzon, P. Hedström, I. Kero, K. V. S. Ramam, C. P. Camurri and M. Odén, J. Mater. Process. Technol., 2008, 208, 415 CrossRef PubMed; N. Rakov, F. E. Ramos, G. Hirata and M. Xiao, Appl. Phys. Lett., 2003, 83, 272 CrossRef PubMed; J. A. Capobianco, F. Vetrone, T. D'Alesio, G. Tessari, A. Speghini and M. Bettinelli, Phys. Chem. Chem. Phys., 2000, 2, 3203 RSC.
- R. Scheps, Prog. Quantum Electron., 1996, 20, 271 CrossRef CAS; P. Xie and S. C. Rand, Appl. Phys. Lett., 1993, 63, 3125 CrossRef PubMed.
- A. J. Kenyon, Prog. Quantum Electron., 2002, 4–5, 225 CrossRef CAS; R. Paschotta, J. Nilsson, A. C. Tropper and D. C. Hanna, IEEE J. Quantum Electron., 1997, 33, 1049 CrossRef; W. J. Miniscalco, J. Lightwave Technol., 1991, 9, 234 CrossRef.
- S. Q. Xu, H. P. Ma, D. W. Fang, Z. X. Zhang and Z. H. Jiang, Mater. Lett., 2005, 59, 3066 CrossRef CAS PubMed; G. S. Maciel, A. Biswas, R. Kapoor and P. N. Prasad, Appl. Phys. Lett., 2000, 76, 1978 CrossRef PubMed; J. Ballato, J. S. Lewis and P. Holloway, Mater. Res. Soc. Symp. Proc., 1999, 24, 51 Search PubMed; E. Downing, L. Hesselink, J. Ralston and R. Macfarlane, Science, 1996, 273, 1185 Search PubMed.
- M. P. Hehlen, M. Sheik-Bahae, R. I. Epstein, S. D. Melgaard and D. V. Seletskiy, J. Mater. Chem. C, 2013, 1, 7471 RSC; M. P. Hehlen, R. I. Epstein and H. Inoue, Phys. Rev. B: Condens. Matter Mater. Phys., 2007, 75, 144302 CrossRef CAS; R. I. Epstein, M. I. Buchwald, B. C. Edwards, T. R. Gosnell and C. E. Mungan, Nature, 1995, 377, 500 CrossRef.
- W. Tittel, M. Afzelius, T. Chanelière, R. L. Cone, S. Kroll, S. A. Moiseev and M. Sellars, Laser Photonics Rev., 2010, 4, 244 CrossRef CAS; B. S. Ham, M. S. Shahriar, M. K. Kim and P. R. Hemmer, Opt. Lett., 1997, 22, 1849 CrossRef; M. Mitsunaga, R. Yano and N. Uesugi, Opt. Lett., 1991, 16, 1890 CrossRef.
- C. R. Li, B. Dong, S. F. Li and C. H. Song, Chem. Phys. Lett., 2007, 443, 426 CrossRef CAS PubMed; P. V. dos Santos, M. T. de Araujo, A. S. Gouveia-Neto, J. A. Medeiros Neto and A. S. B. Sombra, Appl. Phys. Lett., 1998, 73, 578 CrossRef PubMed; H. Kusama, O. J. Sovers and T. Yoshioka, Jpn. J. Appl. Phys., 1976, 15, 2349 CrossRef.
- C. H. Huang and T. M. Chen, J. Mater. Chem. C, 2011, 115, 2349 Search PubMed; S. Ye, F. Xiao, Y. X. Pan, Y. Y. Ma and Q. Y. Zhang, Mater. Sci. Eng., R, 2010, 71, 1 CrossRef CAS PubMed; S. Neeraj, N. Kijima and A. K. Cheetham, Chem. Phys. Lett., 2004, 387, 2 CrossRef PubMed.
- Z. Liu, F. Pu, S. Huang, Q. H. Yuan, J. S. Ren and X. G. Qu, Biomaterials, 2013, 34, 1712 CrossRef CAS PubMed; J. Zhou, M. X. Yu, Y. Sun, X. Z. Zhang, X. J. Zhu, Z. H. Wu, D. M. Wu and L. Y. Li, Biomaterials, 2011, 32, 1148 CrossRef PubMed; X. X. He, K. Wang and Z. Cheng, Wiley Interdiscip. Rev.: Nanomed. Nanobiotechnol., 2010, 2, 349 CrossRef PubMed; F. Vetrone, R. Naccache, A. J. de la Fuente, F. Sanz-Rodríguez, A. Blazquez-Castro, E. M. Rodriguez, D. Jaque, J. G. Solé and J. A. Capobianco, Nanoscale, 2010, 2, 495 RSC.
- C. D. S. Brites, P. P. Lima, N. J. O. Silva, A. Millán, V. S. Amaral, F. Palacio and L. D. Carlos, J. Lumin., 2013, 133, 230 CrossRef CAS PubMed; B. Dong, B. S. Cao, Y. Y. He, Z. Liu, Z. P. Li and Z. Q. Feng, Adv. Mater., 2012, 15, 1987 CrossRef PubMed; L. H. Fischer, G. S. Harms and O. S. Wolfbeis, Angew. Chem., Int. Ed., 2011, 50, 4546 CrossRef PubMed; M. A. R. C. Alencar, G. S. Maciel, C. B. de Araújo and A. Patra, Appl. Phys. Lett., 2004, 84, 4753 CrossRef PubMed.
- K. S. Shah, J. Glodo, M. Klugerman, W. W. Moses, S. E. Derenzo and A. J. Weber, IEEE Trans. Nucl. Sci., 2003, 50, 2410 CrossRef CAS; E. V. D. van Loef, P. Dorenbos, C. W. E. van Eijk, K. Krämer and H. U. Güdel, Appl. Phys. Lett., 2001, 79, 1573 CrossRef PubMed; D. Cavouras, I. Kandarakis, G. S. Panayiotakis, E. K. Evangelou and C. D. Nomicos, Med. Phys., 1996, 23, 1965 CrossRef.
- G. Chen, J. Seo, C. Yang and P. N. Prasad, Chem. Soc. Rev., 2013, 42, 8304 RSC; H. Q. Wang, M. Batentschuk, A. Osvet, L. Pinna and C. J. Brabec, Adv. Mater., 2011, 23, 2675 CrossRef CAS; Q. Y. Zhang and X. Y. Huang, Prog. Mater. Sci., 2010, 55, 353 CrossRef PubMed; B. M. van der Ende, L. Aartsa and A. Meijerink, Phys. Chem. Chem. Phys., 2009, 11, 11081 RSC.
- Advances in Energy Transfer Processes, ed. B. Di Bartolo and X. Chen, World Scientific Pub. Co. Inc., England, 2011 Search PubMed.
- J. Zheng, X. F. Wang, W. Y. He, Y. Y. Bu and X. H. Yan, Appl. Phys. B, 2014, 115, 443 CrossRef CAS; X. Wang, C. Liu and X. Yan, RSC Adv., 2014, 4, 24170 RSC; N. Rakov, W. B. Lozano, E. L. Falcão-filho, R. B. Guimarães, G. S. Maciel and C. B. de Araújo, Opt. Mater. Express, 2013, 3, 1803 CrossRef; G. J. De, W. P. Qin, J. S. Zhang, J. S. Zhang, Y. Wang, C. Y. Cao and Y. N. Cui, J. Lumin., 2007, 122, 128 CrossRef PubMed.
- Inorganic Phosphors: Compositions, Preparation and Optical Properties, ed. W. M. Yen and M. J. Weber, CRC Press, USA, 2006 CrossRef CAS; S. R. Jain, K. C. Adiga and V. R. P. Verneker, Combust. Flame, 1981, 40, 71 CrossRef CAS.
- J. R. Lakowicz, Principles of Fluorescence Spectroscopy, Springer, USA, 2006 Search PubMed.
- An Introduction to the Optical Spectroscopy of Inorganic Solids, ed. J. Solé, L. Bausá and D. Jaque, John Wiley & Sons Inc., England, 2005 Search PubMed.
- M. P. Hehlen, M. G. Brik and K. W. Krämer, J. Lumin., 2013, 136, 221 CrossRef CAS PubMed.
- W. T. Carnall, H. M. Crosswhite, and H. Crosswhite,Energy level structure and transition probabilities in the spectra of the trivalent lanthanides in LaF3, Spec. Rep., Chemistry Division, Argonne National Laboratory, USA, 1977 Search PubMed.
- C. Kodaira, J. Lumin., 2003, 101, 11 CrossRef CAS.
- G. de Sá, C. M. Donegá, O. L. Malta, A. M. Simas, R. L. Longo, P. A. Santa-Cruz and E. F. da Silva, Coord. Chem. Rev., 2000, 196, 165 CrossRef.
- N. Rakov, R. B. Guimarães, W. B. Lozano and G. S. Maciel, J. Appl. Phys., 2013, 114, 043517 CrossRef PubMed.
- T. Grzyb and S. Lis, Inorg. Chem., 2011, 50, 811 CrossRef PubMed.
- F. Vetrone, J. C. Boyer, J. A. Capobianco, A. Speghini and M. Bettinelli, Appl. Phys. Lett., 2002, 80, 1752 CrossRef CAS PubMed.
- M. Pokhrel, A. Gangadharan and D. K. Sardar, Mater. Lett., 2013, 99, 86 CrossRef CAS PubMed; J. C. Boyer and F. C. J. M. van Veggel, Nanoscale, 2010, 2, 1417 RSC.
- M. Saboktakin, X. Ye, S. J. Oh, S. H. Hong, A. T. Fafarman, U. K. Chettiar, N. Engheta, C. B. Murray and C. R. Kagan, ACS Nano, 2012, 6, 8758 CrossRef CAS PubMed; H. Mertens and A. Polman, Appl. Phys. Lett., 2006, 89, 211107 CrossRef PubMed; L. P. Naranjo, C. B. de Araújo, O. L. Malta, P. A. S. Cruz and L. R. P. Kassab, Appl. Phys. Lett., 2005, 87, 241914 CrossRef PubMed.
- W. Shao, G. Y. Chen, J. Damasco, X. L. Wang, A. Kachynski, T. Y. Ohulchanskyy, C. H. Yang, H. Ågren and P. N. Prasad, Opt. Lett., 2014, 39, 1386 CrossRef CAS PubMed; F. Zhang, R. C. Che, X. M. Li, C. Yao, J. P. Yang, D. K. Shen, P. Hu, W. Li and D. Y. Zhao, Nano Lett., 2012, 12, 2852 CrossRef PubMed; Y. Wang, L. P. Tu, J. W. Zhao, Y. J. Sun, X. G. Kong and H. Zhang, J. Phys. Chem. C, 2009, 113, 7164 Search PubMed; P. Ghosh, J. Oliva, E. De la Rosa, K. K. Haldar, D. Solis and A. Patra, J. Phys. Chem. C, 2008, 112, 9650 Search PubMed.
- H. Yu, Q. M. Huang, E. Ma, X. Q. Zhang and J. C. Yu, J. Alloys Compd., 2014, 613, 253 CrossRef CAS PubMed; N. Rakov, R. B. Guimarães, D. F. Franceschini and G. S. Maciel, Mater. Chem. Phys., 2012, 135, 317 CrossRef PubMed; G. Chen, H. Liu, H. Liang, G. Somesfalean and Z. Zhang, J. Phys. Chem. C, 2008, 112, 12030 Search PubMed.
- G. S. Maciel, R. B. Guimarães, P. G. Barreto, I. C. S. Carvalho and N. Rakov, Opt. Mater., 2009, 31, 1735 CrossRef CAS PubMed.
- Y. Dwivedi, A. Bahadur and S. B. Rai, J. Appl. Phys., 2011, 110, 043103 CrossRef CAS PubMed; G. Y. Chen, T. Y. Ohulchanskyy, R. Kumar, H. Ågren and P. N. Prasad, ACS Nano, 2010, 4, 3163 CrossRef PubMed; A. S. S. de Camargo, L. A. O. Nunes, J. F. Silva, A. C. F. M. Costa, B. S. Barros, J. E. C. Silva, G. F. de Sá and S. Alves Jr, J. Phys.: Condens. Matter, 2007, 19, 256209 CrossRef.
- C. M. Liu, D. Hou, J. Yan, L. Zhou, X. J. Kuang, H. B. Liang, Y. Huang, B. B. Zhang and Y. Tao, J. Phys. Chem. C, 2014, 118, 3220 Search PubMed; Z. Xia and R. S. Liu, J. Phys. Chem. C, 2012, 116, 15604 Search PubMed; W. R. Liu, C. C. Lin, Y. C. Chiu, Y. T. Yeh, S. M. Jang and R. S. Liu, Opt. Express, 2010, 18, 2946 CrossRef CAS PubMed; T. W. Kuo and T. M. Chen, J. Electrochem. Soc., 2010, 157, J216 CrossRef PubMed; C. F. Guo, F. Gao, Y. Xu, L. F. Liang, F. G. Shi and B. H. Yan, J. Phys. D: Appl. Phys., 2009, 42, 095407 CrossRef; N. Rakov and G. S. Maciel, Chem. Phys. Lett., 2004, 400, 553 CrossRef PubMed.
- G. S. Maciel, A. Biswas and P. N. Prasad, Opt. Commun., 2000, 178, 65 CrossRef CAS; F. W. Ostermayer Jr and L. G. Van Uitert, Phys. Rev. B: Condens. Matter Mater. Phys., 1970, 1, 4208 CrossRef.
- N. Rakov, D. F. Amaral, R. B. Guimarães and G. S. Maciel, J. Appl. Phys., 2010, 108, 073501 CrossRef PubMed.
- A. Oladeji, P. L. Arnold, M. I. Ali, S. Sujecki, A. Phillips, I. V. Sazanovich and J.
A. Weinstein, J. Mater. Chem. C, 2013, 1, 8075 RSC; J. Méndez-Ramosa, V. K. Tikhomirova, V. D. Rodrígueza and D. Furniss, J. Alloys Compd., 2007, 440, 328 CrossRef CAS PubMed; K. W. Kramer, H. U. Güdel and R. N. Schwartz, J. Alloys Compd., 1998, 275, 191 CrossRef; G. S. Maciel, C. B. de Araújo, Y. Messaddeq and M. A. Aegerter, Phys. Rev. B: Condens. Matter Mater. Phys., 1997, 55, 6335 CrossRef.
- Z. Wang, J. Feng, M. Pang, S. H. Panab and H. J. Zhang, Dalton Trans., 2013, 42, 12101 RSC; C. M. Zhang, P. A. Ma, C. X. Li, G. G. Li, S. S. Huang, D. M. Yang, M. M. Shang, X. J. Kang and J. Lin, J. Mater. Chem., 2011, 21, 717 RSC; G. Y. Chen, Y. Liu, Y. G. Zhang, G. Somesfalean, Z. G. Zhang, Q. Sun and F. P. Wang, Appl. Phys. Lett., 2007, 91, 133103 CrossRef CAS PubMed; D. Matsuura, Appl. Phys. Lett., 2002, 81, 4526 CrossRef PubMed.
- S. Ghosh, K. Das, G. Sinha, J. Lahtinen and S. K. De, J. Mater. Chem. C, 2013, 1, 5557 RSC; D. L. Geng, G. G. Li, M. M. Shang, C. Peng, Y. Zhang, Z. Cheng and J. Lin, Dalton Trans., 2012, 41, 3078 RSC; M. V. Nazarov, D. Y. Jeon, J. H. Kang, E. J. Popovici, L. E. Muresan, M. V. Zamoryanskaya and B. S. Tsukerblat, J. Solid State Commun., 2004, 131, 307 CrossRef CAS PubMed.
- X. M. Liu, C. K. Lin and J. Lin, Appl. Phys. Lett., 2007, 90, 081904 CrossRef PubMed.
- J. Wang and P. A. Tanner, J. Am. Chem. Soc., 2010, 132, 947 CrossRef CAS PubMed.
- N. Rakov and G. S. Maciel, Opt. Commun., 2012, 285, 5242 CrossRef CAS PubMed.
- F. Vetrone, R. Naccache, A. Zamarrón, A. J. de la Fuente, F. Sanz-Rodríguez, L. M. Maestro, E. M. Rodriguez, D. Jaque, J. G. Solé and J. A. Capobianco, ACS Nano, 2010, 4, 3254 CrossRef CAS PubMed; E. Saïdi, B. Samson, L. Aigouy, S. Volz, P. Löw, C. Bergaud and M. Mortier, Nanotechnology, 2009, 20, 115703 CrossRef PubMed.
- S. A. Wade, S. F. Collins and W. G. Baxter, J. Appl. Phys., 2003, 94, 4743 CrossRef CAS PubMed; H. Berthou and C. K. Jorgensen, Opt. Lett., 1990, 15, 1100 CrossRef.
- N. Rakov and G. S. Maciel, Opt. Lett., 2014, 39, 3787 CrossRef CAS PubMed; L. L. Xing, Y. L. Xu, R. Wang, W. Xu and Z. G. Zhang, Opt. Lett., 2014, 39, 454 CrossRef PubMed; W. Xu, H. Zhao, Y. X. Li, L. J. Zheng, Z. G. Zhang and W. W. Cao, Sens. Actuators, B, 2013, 188, 1096 CrossRef PubMed.
- W. Xu, X. Y. Gao, L. J. Zheng, P. Wang, Z. G. Zhang and W. W. Cao, Appl. Phys. Express, 2012, 5, 072201 CrossRef.
- C. Zaldo and C. Cascales, Phys. Chem. Chem. Phys., 2014, 16, 23274 RSC; N. Rakov, G. S. Maciel and M. Xiao, Electron. Mater. Lett., 2014, 10, 985 CrossRef CAS PubMed; X. Wang, X. G. Kong, Y. Yu, Y. J. Sun and H. Zhang, J. Phys. Chem. C, 2007, 111, 15119 Search PubMed; D. N. Messias, M. V. D. Vermelho, A. S. Gouveia-Neto and J. S. Aitchison, Rev. Sci. Instrum., 2002, 73, 476 CrossRef PubMed; E. Maurice, G. Monnom, B. Dussardier, A. Saissy, D. B. Ostrowsky and G. W. Baxter, Appl. Opt., 1995, 34, 8019 CrossRef PubMed.
- N. Rakov and G. S. Maciel, Sens. Actuators, B, 2012, 164, 96 CrossRef CAS PubMed; M. Quintanilla, E. Cantelar, F. Cussó, M. Villegas and A. C. Caballero, Appl. Phys. Express, 2011, 4, 022601 CrossRef.
- N. Rakov and G. S. Maciel, Dalton Trans., 2014, 43, 16025 RSC.
- B. S. Richards, Sol. Energy Mater. Sol. Cells, 2006, 90, 1189 CrossRef CAS PubMed; A. Shalav, B. S. Richards, T. Trupke, K. W. Krämer and H. U. Güdel, Appl. Phys. Lett., 2005, 86, 013505 CrossRef PubMed.
- C. Lorbeer and A. V. Mudring, Chem. Commun., 2014, 50, 13282 RSC; D. Q. Chen, Y. S. Wang, Y. L. Yu, P. Huang and F. Y. Weng, J. Appl. Phys., 2008, 104, 116105 CrossRef CAS PubMed; P. Vergeer, T. J. H. Vlugt, M. H. F. Kox, M. I. den Hertog, J. P. J. M. van der Eerden and A. Meijerink, Phys. Rev. B: Condens. Matter Mater. Phys., 2005, 71, 014119 CrossRef; R. T. Wegh, H. Donker, K. D. Oskam and A. Meijerink, Science, 1999, 283, 663 CrossRef.
- C. Strümpel, M. McCann, G. Beaucarne, V. Arkhipov, A. Slaoui, V. Švrček, C. del Cañizo and I. Tobias, Sol. Energy Mater. Sol. Cells, 2007, 91, 238 CrossRef PubMed.
- N. Rakov and G. S. Maciel, J. Appl. Phys., 2011, 110, 083519 CrossRef PubMed.
- X. Y. Huang, S. Y. Han, W. Huang and X. G. Liu, Chem. Soc. Rev., 2013, 42, 173 RSC; J. D. Chen, H. Zhang, F. Li and H. Guo, Mat. Chem. Phys., 2011, 128, 191 CrossRef CAS PubMed; H. Lin, S. Zhou, H. Teng, Y. Li, W. Li, X. Hou and T. Jia, J. Appl. Phys., 2010, 107, 043107 CrossRef PubMed.
- Z. J. Liu, J. Y. Li, L. Y. Yang, Q. Q. Chen, Y. B. Chu and N. L. Dai, Sol. Energy Mater. Sol. Cells, 2014, 122, 46 CrossRef CAS PubMed.
|
This journal is © The Royal Society of Chemistry 2015 |