Laser-induced reduced graphene oxide for high-performance electrochemical sensors of antipyretic drug in real samples†
Received
30th October 2023
, Accepted 22nd December 2023
First published on 25th January 2024
Abstract
Laser-induced graphene (LIG) has gained dominance recently as a very sought after material for fabrication, patterning graphitic structures, and electrodes for various applications in electronics. In this study, we increase the ability of RGO-graphitized nanosheets by carefully regulating the laser fluence. We used an advanced cutting-edge technique for direct writing with a pulse laser in an open atmosphere to reduce graphite oxide nanosheets in aqueous media. The nano-sized RGO was confirmed as being produced by converting the sp3 structure to sp2 reduced form. The laser-induced RGO-1-3 were characterized by field emission scanning electron microscopy (FESEM), transmission electron microscopy (TEM), Raman spectroscopy, Fourier transform infrared (FTIR), powder X-ray diffraction (XRD), and X-ray photoelectron spectroscopy (XPS). Non-agglomerated and different porous nanostructures of RGO-1–3 were successfully obtained during laser irradiation. Electrochemical impedance spectroscopy (EIS) was performed and high surface-active edge reactive regions were found after laser irradiation of the RGO nanostructures, which promoted excellent electrochemical detection performance with rapid electron transfer, in a low potential window. All LI-RGO nanostructures were fabricated on GCE to determine their capacity for the precise detection of acetaminophen using CV and DPV voltametric techniques. In particular, the RGO-3/GCE fabricated electrode exhibited the lowest level of 5.2 nanomolar detection of acetaminophen with an outstanding sensitivity of 2.7271 μA Mm−1 cm−2. The designed RGO-3/GCE electrode also exhibited remarkable reproducibility, selectivity, and stability. In addition, the RGO-3/GCE device was successfully investigated for the detection of an antipyretic pharmaceutical drug in river and human urine samples and showed excellent results. In the search for acetaminophen, the RGO-3/GCE fabricated device can be a low-cost and reliable RGO GCE electrode.
Environmental significance
One of the most commonly used medications in the world is acetaminophen (IUPAC name: N-(4-hydroxyphenyl)acetamide, known as paracetamol), a phenolic substance that has a negative influence on the quality of environmental resources. It is utilized for organic synthesis in industries including plastics and drugs. Due to the acute toxicity of its phenol moiety, it has negative impacts on humans, animals, plants, and aquatic life. The World Health Organization (WHO) has categorized acetaminophen into three classes of carcinogen. It also possesses a limited ability to biodegrade in ponds, lakes, domestic wastewater, streams, seas, and ecological environments, even in the presence of nanomole amounts on the surface of the environment. To prevent water contamination, a precise and quick method is essential for AP detection. In this way, AP could be transformed into less toxic AP electrochemically by sensors, which could provide new insights into handling functional pollution. Therefore, it is necessary to develop a quick, inexpensive, accurate, and practical method for sensing the presence of AP substances in water bodies.
|
1. Introduction
Graphene1 has attracted significant attention as a promising electrode material for versatile applications. Due to its excellent physical, chemical, surface morphology, and electronic properties,2 graphene has been widely considered as a potential material for an active electrode in many applications.3 The electronic properties of reduced graphene oxide (RGO) have made substantial progress.4 A number of methods, including chemical reaction,5 thermal treatment,6 photo-chemical reaction,7 and laser reduction procedures,8–10 have been utilized to remove the oxygen species prior to GO reduction. The reduction of graphene oxide mostly consists of decomposition, and the subsequent elimination of oxygen-containing groups (OCGs) confined in GO11,12 and comparisons of GO reduction have been made.13,14
In the past year, versatile methods have been developed, dealing with the preparation of high-performance nanomaterials for the fabrication of electrodes for sensing.15–17 Among these nanomaterials, graphene as a 2-dimensional (2D) network with an enhanced surface area18–31 and outstanding electrical conductivity has been employed in sensing electrodes32–35 both alone and in combination.36 Nevertheless, because of strong van der Waals interactions, an aggregation of graphene layers throughout the preparation poses a challenge in retaining the anticipated large surface area and electronic conductivity.37 An appropriate physical modification to pristine graphene and its derivatives, in terms of the construction of 3D-porous graphene, disturbed the dramatic change in surface area and provided the required “rapid” electron transport.38
The manufacture of laser-induced graphene is a one-step green synthesis unlike other methods. Besides, among many approaches, the laser-induced graphene (LIG) technique is eco-friendly, low cost, and effective, and this method does not involve the usage of corrosive and toxic chemicals, solvents, and a laborious method. Compared to conventional graphene/binder composites, LIG films possess a number of unique advantages, including excellent flexibility, foldability, robust electric/thermal conductivity, and a high surface area. LIG fabrication is quick and ecologically friendly because it does not need any chemical processing, which saves a lot of time and energy. LIG strategies have been confirmed to produce an excellent yield of reduced GO with improved properties.38,39 Under picosecond laser treatment, graphene oxide is reduced and exfoliated under ambient conditions, simultaneously producing an open 3D-network structure.40
The nanostructure can avoid an agglomeration of graphene nanosheets, which enhances electron transport in the fabricate electrode. Therefore, laser-induced graphene oxide can be used to generate RGO, which allows flexible device integration and patterning. The majority of reported approaches for laser-irradiated GO fall into one of two categories: (a) reduction of GO solution41–44 and (b) reduction followed by the design of a GO film with an appropriate solid-state substrate.45,46 There has been a lot of interest in the laser-induced reduction of free-standing GO sheets as a method for improving the physiochemical properties of RGO without being constrained by a temperature-sensitive substrate for mass-scale devices. Other advantages of graphene films can be obtained via mechanical exfoliation of an extremely well-ordered neat pyrolytic graphite design,47 epitaxial methods, and the development of d-block transition metal substrates through chemical vapor deposition (CVD).48
One of the most commonly used medications in the world, acetaminophen (IUPAC name: N-(4-hydroxyphenyl)acetamide) reduces temperature and relieves pain.49,50 At present, N-(4-hydroxyphenyl)acetamide is found in various water resources all over the world. Drinking water, river, industrial waste, and pond water samples51 have been contaminated due to routine usage of AP. Nearly 50% of drug-induced acute liver failure in patients is due to excess intake of acetaminophen (AP). The World Health Organization (WHO) has also categorized acetaminophen into three classes of carcinogen.52
Targeting the sensitive and quick detection of AP is crucial for the administration of pharmaceutical excellence, consumption, and water pollution. Most recently, different strategies of analytical performance have been applied like spectrophotometry, chromatography, electrophoresis, and electrochemical sensors for AP detection. In contrast, electrochemical sensors play crucial roles and have many advantages, such as instrumental simplicity, low cost, short time, excellent reproducibility, no pretreatment, and effortless portability. Electrochemical sensing is a highly recommended and promising type of investigative technique as a target to provide convenient solutions for challenging situations while being associated with conventional diagnostic tools.53–55 The incorporation of active and robust candidates on electrodes has been designed to utilize the selectivity and sensitivity in the conduct of electrochemical sensing of AP target analytes.
Numerous materials have been scrutinized to detect target molecules together with metal nanoparticles, such as hybrid sp3-C, sp2-C, 2D/3D-architectures,56–59 polymers, host–guest, core–shell, and porous carbon-based materials. At present, 3D meso-porous carbon nanomaterials60,61 have been recommended for showing characteristic structural features like 3D pore-structures with a high degree of graphitization, large surface area, and rich active sites.
It is anticipated that specific aspects need to be improved, such as conductivity, material stability, and mass transfer events of RGO. Furthermore, the RGO framework contains hollow carbon, 3D-pores, and great voids, which introduce other sites in the structures of reduced GO, resulting in enhanced electrocatalytic activity.62,63 Inspired by these observations, we intend to explore laser-induced reduced GO (RGO) for the advancement of a sensing platform for the detection of AP. The RGO was prepared by a laser-irradiated GO process. The concentration of KMnO4 was varied while that of sodium nitrate was fixed, while loading the amount of GO precursor. After irradiation of GO, ceramic slides were fabricated to yield uniform GO to produce different topologies. All synthesized RGO-1–3 were used as a rapid electron transfer moderator on a glassy carbon electrode (GCE) surface. The as-fabricated RGO sensor exhibited excellent electrocatalytic performance against target analytes, such as good selectivity, high sensitivity, stability, a wide linear range concentration for detection, and a low detection limit, toward the determination of AP. The real-time practical application of the as-designed sensor was successfully authenticated in real samples, such as human urine samples, river samples, and antipyretic tablets for the determination of AP.
2. Experimental section
2.1. Chemicals and instruments
The ESI† includes a description of the materials (S1†) and instruments (S2†).
2.2. Synthesis of precursors GO-1–3
Preparation of precursor graphene oxide (GO-1).
The modified Hummer's procedure64 was used to synthesize graphene oxide (GO). In short, 1 g of sodium nitrate, 1 g of graphite powder, and 45 mL of concentrated H2SO4 (98%) were combined with 1 g of KMnO4. The temperature of the reaction mixture was then carefully lowered to 0–5 °C in an ice bath followed by stirring for 3 hours. Then, after progressively raising the reaction mixture temperature to 95 °C, 100 mL of DD water was added while it was being continually stirred for 15 minutes. After that, 10 mL of H2O2 was carefully added, and the mixture was again agitated for 2 hours. After that, the mixture was centrifuged (5000 rpm for 25 min). The pH was then adjusted to 6.5–7.0 by washing the residual solid material five times with 80 mL of 37% HCl and 100 mL of double-distilled water. After each washing, the desired solution was centrifuged (at 5000 rpm for 20 minutes) and the leftover precipitate was collected. Finally, the resulting product was vacuum dried for 24 hours at 45 °C after being kept in a refrigerator for 1 hour and yielded graphene oxide (GO).
Preparation of precursor graphene oxide (GO-2).
Graphene oxide GO-2 was prepared in a similar manner to graphene oxide (GO-1) using 1 g of graphite powder, 2 g of KMnO4, 1 g of sodium nitrate, 45 mL of H2SO4 (98%), and 10 mL of H2O2. GO-2 purification was done in a similar manner to graphene oxide (GO-1).
Preparation of precursor graphene oxide (GO-3).
Graphene oxide (GO-3) was prepared in a similar manner to graphene oxide (GO-1) using 1 g of graphite powder, 3 g of KMnO4, 1 g of sodium nitrate, 45 mL of H2SO4 (98%), and 10 mL of H2O2. GO-3 purification was done in a manner similar to graphene oxide (GO-1).
2.3. General procedure for the preparation of laser-induced RGO-1–3: sample preparation, fiber laser process, and reduction mechanism parameters
Initially, 50 mg of graphene oxide (GO-1–3) was dispersed in 5 mL of DI water and sonicated for 24 h. The GO solution was effectively dispersed to prevent any particles settling at the bottom of the flask. Fig. 1A–D show the laser-induced technique for the fabrication of graphene oxide as well as a simplified illustration of the electrochemical detection of AP. GO solution was coated on alumina ceramic (Al2O3) substrates with dimensions of (L × W) 7.5 cm × 5.5 cm through a drop coating technique to form a wet film. Then, dried GOF was achieved with a hot-air oven at 60 °C for 2 h. In this study, a 1064 nm laser was attached with fiber optics and transformed into a laser via a telecentric lens (specification: f = 125 mm, λ = 1064 nm), The laser passed over an excitation filter and was reflected by a shrinking mirror into a mirror spot and focused by an objective lens to excite GO bound on the ceramic substrate. Detailed specifications of the laser patterning apparatus are given in Table S1.†
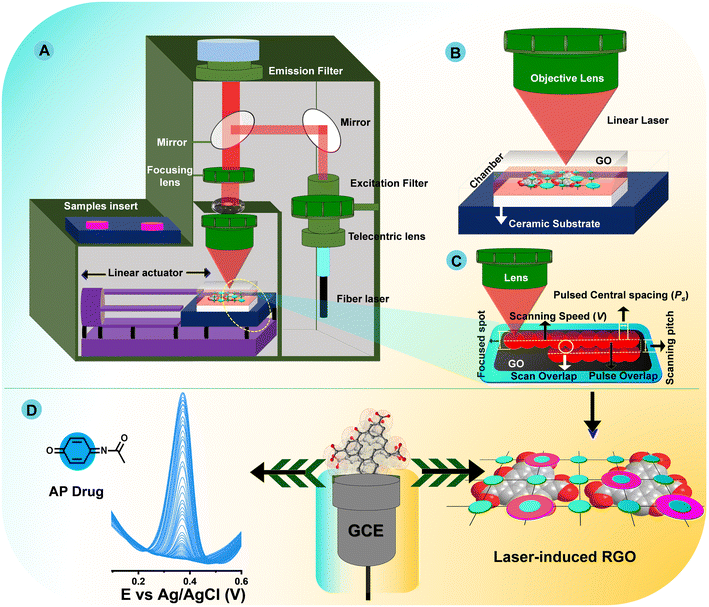 |
| Fig. 1 Schematic setup of the fiber laser system (A), zoomed-in schematic showing the scanning of the laser (B), laser scanning path (C), and electrochemical detection of AP (D). | |
The ceramic plate was placed on a sample holder and sat on a stage fixed with a linear actuator (Fig. 1A and B). The GO-bounded ceramic substrate was scanned by moving the stage in the xy-direction (Fig. 1C). Fig. 1D show typical π–π interactions with AP for electrochemical detection. The simultaneous action of rapid cooling and ultrafast heating produced by a nanosecond laser results in an intriguing zone of improved GO reduction in an open atmosphere. Given this open temperature, oxidation mostly occurs along the GO surface and captures oxygen in the atmosphere, and groups that include oxygen from the surface of GO form into molecules of CO, CO2, and H2O. At the same time, the GO bulk domains undergo fast reduction and annealing without exhibiting any sign of oxidation, since surface edge carbon atoms are usually much more chemically active than those involved in π-conjugation in the graphene skeleton. Therefore, edge carbon atoms may also account for a large portion of laser-induced chemical reduction processes in this study. Later, GO changes into RGO with high local order and appropriate mass loss of oxygen-containing moieties, mostly from its surface edges. A plausible mechanism for laser-induced RGO and the results can be summarized as follows:
• At high temperatures, oxygen-containing groups detach from the GO surface and form radicals, predominantly –OH and –O, when exposed to laser light.
• The oxidation process is initiated at the strongly defective parts and grain boundaries of GO by oxygen-containing radicals and atmospheric oxygen. In turn, highly defective zones are mostly eliminated and oxygen is captured as CO2.
• The fast kinetics of covalent bond rearrangements at high temperature during laser treatment is greatly accelerated by annealing of point defects in GO. This increases the structural order of the final product even further.
3. Results and discussion
3.1. Choice of materials and method
Although all experimental work has focused on using the same GO precursors, the ideas as well as the innovations beyond the work presented here are completely unique and sustainable, and these aspects were chosen due to the fact that they may also be innovative in comparison to previously published work. To eliminate the oxygen species of GO reduction, a number of strategies have been used, including chemical reaction, thermal treatment, and photo-chemical reaction. However, edge surface oxygen reduction of GO is very limited. To address this, appropriate methods need to be found. The laser-induced reduction technique is a very promising approach to reduce oxygen species at the edge surface of GO. For a clear comparison of their general features for GO reduction, the most commonly utilized techniques8,41,65–80 have been summarized in Chart 1.
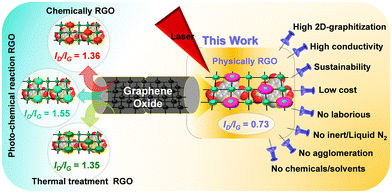 |
| Chart 1 Different strategies for developing an RGO and the advantages of a laser-induced technique. | |
Reduced graphene oxide (RGO), which allows for flexible device integration and patterning must be produced via laser-reduced graphene oxide. Numerous GO laser-irradiated techniques have been stated and most of them fall into one of two categories: the first is the reduction of GO solution and the second is the reduction and patterning of GO film with a solid-state substrate. However, our aim in this research is an innovative approach for the reduction of freestanding GO sheets by one-stop utilization of a picosecond pulse laser. In contrast, the advantages of LIG procedures are a high degree of graphitization, a large surface area, low cost, eco-friendliness, no agglomeration, no chemicals utilized, no inert atmosphere needed, and excellent scale-up, as depicted in Chart 1.
3.2. XRD, Raman, and FT-IR analysis of RGO-1–3
The characteristic XRD peaks of as-prepared precursors GO-1–3 and laser-induced RGO-1–3 were investigated using the XRD technique. Characteristic structural peaks, interlayer d-spacing, and the crystallinity size (D) of laser-induced RGO-1–3 were scrutinized and are presented in Table S2.† Fig. S1A† illustrates the powder XRD spectra of pristine graphene oxide (GO). The characteristic diffraction peak pattern of GO-1–3 confirmed a sharp line at 2θ = 11° that corresponds to the (002) diffraction plane, along with a small graphitic characteristic peak located at around 2θ = 26°, which is attributed to the effective oxidation of parent graphite.81,82 An intensification in d-spacing from graphite to GO-1–3 proved the extension of the graphitic stack attributable to the occurrence of oxygen functional groups on graphene sheets.
The effective reduction of carboxyl at the surface edge and periphery position and of epoxide and hydroxide groups on GO was obtained by laser-induced technique. Fig. 2A shows strong evidence with the vanishing of the basic characteristic of graphene oxide at 2θ = 11°, and the occurrence of a new broadened diffraction peak (002) at about 2θ = 26.05° after the laser treatment of RGO-1, RGO-2, and RGO-3. The slight broadening of the peak at about 26° (between 20° and 30°), is a strong confirmation of the disordered nature of the RGO layers. The interlayer d-spacing and crystallinity size (D) show the excellent crystallinity of laser-induced RGO-3, compared with RGO-1–2, which is also a further confirmation that RGO-3 could be an effective electrocatalyst (Table S2†). Raman spectroscopic analysis was the final non-destructive method used to illustrate the structure of RGO. The quality of the graphene nanomaterials, defects, and the well-ordered and disordered nanolayer structure of laser-induced RGO-1–3 were confirmed. The calculated ID/IG ratio and as-recorded Raman spectra of RGO-1–3 are shown in Table S2† and Fig. 2B, respectively. In contrast, we also examined the Raman analysis for precursors GO-1–3, and the spectra are presented in the ESI† (Fig. S1B).
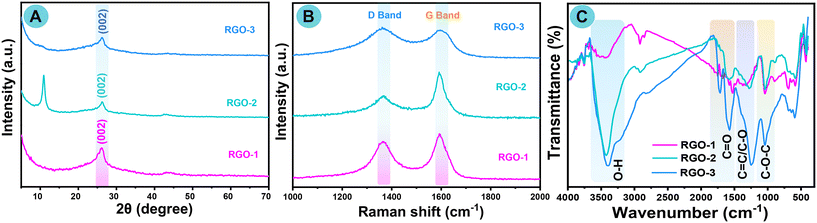 |
| Fig. 2 XRD pattern, (A) Raman spectra (B), and FTIR (C) spectra of RGO-1–3. | |
Raman spectra of GO-1–3 showed a couple of distinguishable peaks at around 1350 and 1604 cm−1, which are consistent with the D and G bands. The appearance of the G band is due to the E2g-vibration behavior of sp2 hybridized carbon and the domains can elucidate the degree of graphitization, while the D band is related to structural flaws and the partly disordered skeleton structure of the sp2 domains. After the laser treatment of GO, the as-prepared RGO-1–3 samples revealed a G band at around 1581 cm−1 and a D band at 1351 cm−1.83 An improved ID/IG ratio of all RGO after reduction has commonly been reported in the literature. As shown in Table S2,† the ID/IG values of as-prepared RGO-1–3 reveal a significant increase compared with earlier reported RGO.84
Not only was the ID/IG ratio of RGO-3 improved but also it was higher than RGO-1 or RGO-2. We resolved that laser-induced RGO-1–3 obtained from GO-1–3 by reduction with a laser had a healing effect in the presence of the resultant sp2 domains. Since the stretching vibration of the oxygenated groups is infrared active, FTIR spectra can provide qualitative information about the deoxygenating functional group. Fig. 2C and S1C† show the characteristic FTIR spectra of RGO1–3 and GO-1–3, respectively. The oxygen-bound groups were efficiently diminished after laser treatment of GO. The FTIR spectra of GO show a broad peak at about 3400 cm−1 due to a hydroxyl moiety on graphene, and the characteristic sharp peak positioned around 1720 cm−1 was attributed to carboxyl functional groups of the GO nanosheet. Moreover, the peak located at around 1620 cm−1 was attributable to the aromatic stretching vibration of C
C bonds. Furthermore, the main peaks oscillating from 1050 cm−1 to 1380 cm−1 were attributable to the vibration frequency of C–O and C–O–C, respectively.85
The characteristic peak intensities of all RGO-1–3 around 1720 cm−1, 1220 cm−1, and 1050 cm−1 have moderately decreased. In particular, laser-induced RGO-3 peaks are dramatically altered, and the broad peak around 3400 cm−1 is weakened, which clearly confirms that the surface edge oxygen-bearing functional groups had vanished from the GO sheets. Simultaneously, a new peak appeared around 1560 cm−1 after laser irradiation of RGO, confirming its rebuilding as a highly π-conjugated nanostructure of RGO, and those characteristic peak data agreed extremely well with those of chemically modified RGO.86
3.3. XPS analysis of LI-RGO
To gain an in-depth understanding of the elements present in the RGO nanolayered structure, XPS surveys were carried out in terms of binding energy. The deconvoluted and Gaussian-fitted XPS spectra of RGO-1–3 are shown in Fig. 3A–L. The core-level binding energy (eV) values are listed in Tables S3 and S4.†Fig. 3A, D, G and J show the wide-scan XPS survey spectra of laser-induced RGO-1–3, and GO-3, respectively. The unique peaks of C1s and O1s further confirm the occurrence of carbon and oxygen elements. The core-level C1s XPS spectra of all laser-induced RGO-1–3 exhibited five types of functionalized carbon atoms with appropriate binding energies (Table S3†), and the corresponding Gaussian-fitted curves are shown in Fig. 3B, E, H and K. The deconvoluted C1s core-level spectra of all RGO-1–3 and GO-3 are shown with four curves. The major curve is revealed at ∼283.5 eV attributable to the C
C bond of sp2 carbon and is effectively accounted by the RGO skeleton framework. The second Gaussian-fitted peak is at ∼284.9 due to the C–O/C–OH (epoxy/hydroxy) binding signal. In addition, other oxygen-bearing groups, such as C–O and C–O, occurred at ∼287.7 eV and ∼289.8 eV, respectively, corresponding to the carbonyl group (C
O/COOH). Fig. 3C, F, I and L show the high-resolution O1s XPS spectra of RGO-1–3/GO-3, exhibiting three types of oxygenated carbon that correspond to several C–O bonding moieties.
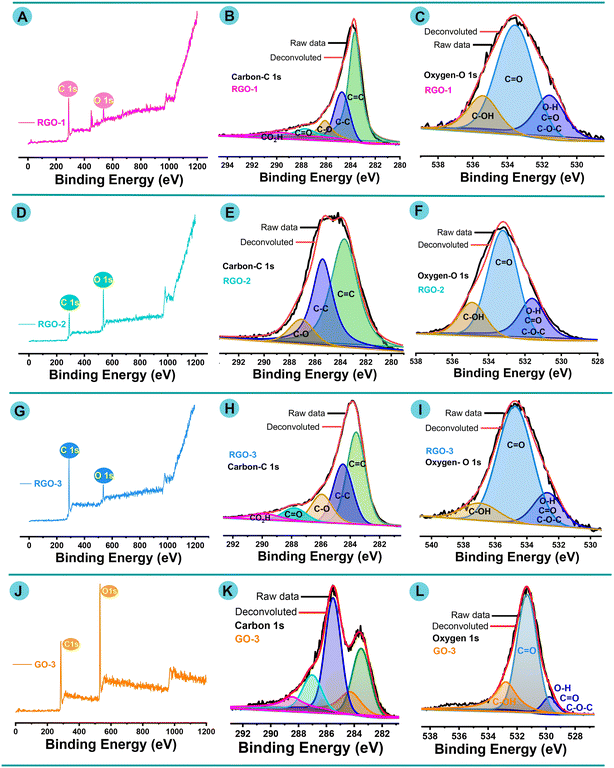 |
| Fig. 3 (A, D, G and J) XPS survey spectra of RGO-1–3 and GO-3. (B and C) Gaussian-fitted spectra of C 1s and O 1s for RGO-1. (E and F) Gaussian-fitted spectra of C 1s and O 1s for RGO-2. (H and I) Gaussian-fitted spectra of C 1s and O 1s for RGO-3. (K and L) Gaussian-fitted spectra of C 1s and O 1s for GO-3. | |
Fig. 3C, F, I and L show that the O 1s peak consists of three different components with binding energies of ∼532.9, ∼534.8, and ∼536.41 eV. The dominant deconvoluted peaks at ∼532.9 eV and ∼534.8 eV correspond to C–O and C–O, respectively. A small deconvoluted signal between 534.4 and 536.9 eV is due to the C–O–H group. In addition, the intensities of the oxygen-bearing functional group peaks were altered in all RGO nanostructures after laser treatment, showing that delocalized π-conjugation was restored in the RGO samples. XPS binding energy spectra of core C1s and O1s were matched with previously published reports.85,87–91
3.4. FESEM and TEM analysis of LI-RGO
All laser-induced RGO and their parent GO morphologies were thoroughly inspected by the FESEM technique. Parent GO-1–3 FESEM morphologies, EDX, and elemental mapping spectra are depicted in Fig. S2A–I.† The low-magnification (1 μm) view of RGO-1–3 shown in Fig. 4A, F and K confirms a flat morphology made up of tiny, wrinkled, and “stacked-paper-like” nanolayered structures. However, the edge surface morphology of RGO-3 was altered significantly after laser irritation of GO-3, as seen in Fig. 4B, G and L at the same magnification (1 μm). Moreover, as shown in Fig. 4K and L, the surface morphology of RGO-3 changed from a closed stacked-paper-like morphology to the cracks-wrinkled morphology. SEM morphologies confirm the stacked-paper-like nanostructure through clear edges between each nanolayer. Further, the outcome is consistent with that found in earlier reports from Raman scrutiny of high-surface-area RGO.92
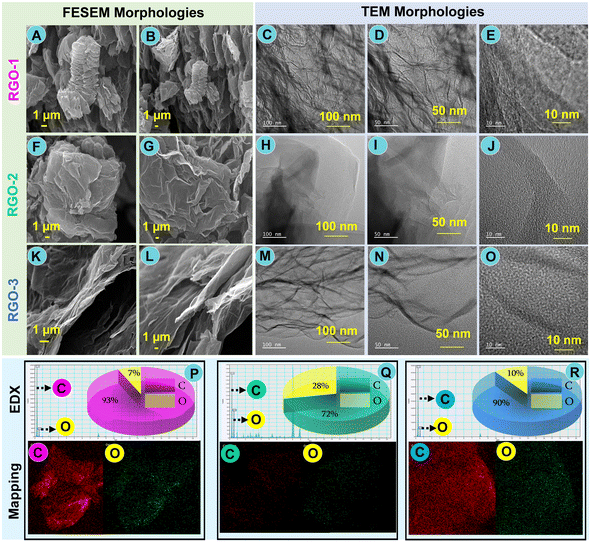 |
| Fig. 4 FESEM and TEM images at different magnifications: (A and B) SEM images, (C–E) TEM images, and (P) EDX/pie chart/elemental mapping of RGO-1; (F and G) SEM images, (H–J) TEM images, and (Q) EDX/pie chart/elemental mapping of RGO-2; and (K and L) SEM images, (M–O) TEM images, and (R) EDX/pie chart/elemental mapping of RGO-3. | |
To determine the surface morphology of the soft edges of all laser-induced RGO-1–3, TEM analysis at different magnifications (100, 50, and 10 nm) was undertaken, and the captured morphologies are shown in Fig. 4 (excluding SEM). TEM images confirmed the nano-morphology of various RGOs (2D-carbon) and the correspondingly distinct morphological transformations occurring within the RGO sample. A closer inspection of RGO-1 shows that crumpled as well as wavy silk-veil nanosheets were unevenly arranged, as shown in Fig. 4C–E. It is confirmed that RGO-2 has transparent and wrinkled morphologies, whereas the TEM images of RGO-3 show a well-defined graphitic lattice-wrinkled morphology after laser irradiation of the appropriate parent GO, as illustrated in Fig. 4H–J and M–O, respectively.
The morphologies of our laser-induced RGO are much better or fairly similar to those reported earlier.93 The chemical composition, elemental percentage, EDX, and elemental mapping for laser-induced RGO-1–3 were scrutinized. The EDX survey spectra and C/O (%) values of all RGO are shown in Fig. 4P–R. The insets to Fig. 4P–R show the elemental mapping and pie charts of carbon (C) and oxygen (O) for LI-RGO-1–3. The EDX spectra show the presence of core elements such as “C” and “O” of the parent GO-1–3 nanosheet and their laser-induced RGO-1–3, respectively. The finding that the combined elemental quantity of carbon and oxygen is reduced in the RGO-3 periodic structure, according to XRD, XPS, and other supporting evidence, is interesting.
4. Electrochemical analysis
4.1. GCE electrode fabrication and electrochemical impedance spectroscopy (EIS) characterization
To examine the GCE electrode characterization and sensing capabilities for AP detection, GCE fabrication was undertaken. The GCE was first thoroughly washed using alumina slurry, DI water, and H2O/ethanol rinsing. After the cleaning procedure, the GCE electrode was drop-cast with an appropriate amount of RGO-1–3, which was about 6 μL, and left to dry at 45 °C for 13 min in a hot-air oven. The fabricated GCE was then investigated for resistance (Rct) and AP detection with appropriate electrolyte media. The same procedure was continued and executed freshly each time for all other parameter analyses, and it was also followed for all other samples to be studied.
An electrochemical impedance spectroscopy (EIS) experiment was conducted in 5 mM of ferro/ferricyanide solution [Fe(CN)6]3/4− and 0.1 M of potassium chloride (KCl) as the supporting electrolyte media using a three-electrode setup to acquire the interfacial characteristic properties of all samples. Fig. 5A shows a typical Nyquist plot (EIS), in particular, Z′ vs. Z′′ in relation to the fabricated GCE electrodes, which are bare GCE, RGO-1/GCE, RGO-2/GCE, and RGO-3/GCE. The histogram in Fig. 5B shows the resistance (Rct) values for appropriate film-modified GCE electrodes. Semicircle expressions are found in two distinct zones, high-frequency and low-frequency regions, on the Nyquist plots. These semicircles correspond to the charge-transfer resistance, Rct, and electron transfer phenomena. RGO-3/GCE EIS data have been fitted using the Randles circuit, as shown in Fig. 5B (inset), where charge transfer resistance (Rct), Warburg constant (W), solution resistance (RS), and constant phase element (CPE) are shown. In the following sequence, a decrease in charge transfer resistance was observed: RGO-1/GCE > bare GCE > RGO-2/GCE > RGO-3/GCE. Because of its highly conductive nature brought about by its active surface area, RGO-3/GCE is confirmed to show the lowest charge transfer resistance.
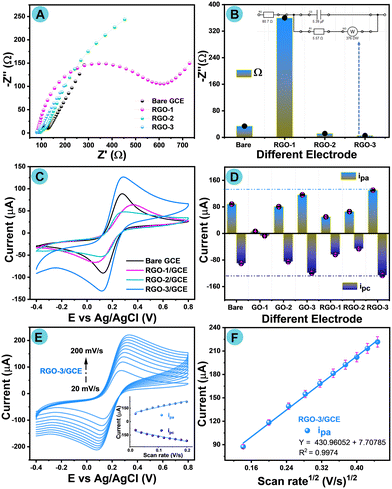 |
| Fig. 5 (A) Nyquist plot (EIS) analysis of different modified GCE in the supporting electrolyte solution. (B) Histogram showing corresponding resistance for the different modified electrode (inset: Randles circuit). (C) CV curves of RGO-1–3/GCE. (D) Histogram showing the corresponding peak current of different modified electrodes in the supporting electrolyte with a scan rate of 50 mV s−1. (E) CV curves of RGO-3/GCE in the supporting electrolyte with scan rates from 20 to 200 mV s−1. (F) Square root of scan rate (anodic peak) of RGO-3/GCE. | |
4.2. Electrochemical analysis of RGO-1–3 modified electrodes
Cyclic voltammetry (CV) is a modest technique to characterize the oxidation–reduction properties of modified electrodes. Electrochemical investigations of the bare GCE, parent GO-1–3, and their corresponding laser-induced RGO-1–3 were systematically conducted in the presence of 5 mM of [Fe(CN)6]3−/4− and 0.1 M of KCl as a supporting electrolyte under ambient conditions at a constant scan rate 50 mV s−1, as shown in Fig. S3A† and 5C, respectively. Studies at different scan rates (20 to 200 mV s−1) of bare GCE, GO-1–3, and RGO-1–2 were also performed and are shown in the ESI† (Fig. S4–S9(A–C)). Fig. 5C and D shows the film response of laser-induced RGO-1–3, and the corresponding histogram represents the oxidation–reduction events of all fabricated electrodes. For bare GCE, there was a reversible redox event in ferro/ferricyanide solution [Fe(CN)6]3−/4− with an anodic peak current (Ipa) of 88.49 μA and cathodic peak current (Ipc) of 90.29 μA. The Ipa/Ipc for other modified electrodes differed after fabrication with RGO-1–3/GCE.
The electrochemical redox behavior of GO-1–2 shows less current response than GO-3, which is due to the effective oxidation by KMnO4 followed by the impact of oxygen content. Conversely, the higher Ipa of 130.31 μA and Ipc of −126.3 μA for RGO-3/GCE could be credited to ferro/ferricyanide solution (Fig. 5D), which may also further facilitate the electron transference phenomenon. RGO-3/GCE exhibited slight shifting of the peak potential with lower current response, which undertakes the oxidation–reduction events in the supporting electrolyte with [Fe(CN)6]3−/4− acquisition of the electrons. Thus, the electron transfer kinetics of RGO-3/GCE are shown to be higher than for other modified electrodes. A study of different scan rates was conducted from 20 to 200 mV s−1 for all fabricated electrodes, such as bare GCE, parent GO-1–3/GCE, and RGO-1–3/GCE, under the same experimental conditions. Fig. 5E shows a cyclic voltammogram with different scan rates for RGO-3/GCE. Weak oxidation–reduction events were noted with bare GCE, which may suggest sluggish electron transfer over the bare GCE, whereas RGO-3/GCE exhibited sharp anodic and cathodic events compared with other RGO-modified electrodes.
The modification of GCE with RGO-3 raises the oxidation–reduction current peaks and minimizes the difference in potential (V) due to its high EASA. Therefore, the surface area (EASA) was calculated with the Randles–Ševčík equation94 in accordance with the square root of scan rates (Ipa). The calculated EASA values are presented in Table S5.† These EASA values obviously confirm the rapid electron transport. Fig. 5E shows cyclic voltammograms of RGO-3/GCE with different scan rates from 20 to 200 mV s−1, in which an anodic peak current was linearly plotted with the square root of the scan rate (Fig. 5F), and the corresponding anodic–cathodic peaks are shown in Fig. 5E (inset).
Fig. 5F illustrates the linearity plot for the square root of the different scan rates for RGO-3/GCE, which proves the linear regression equation with its correlation co-efficient to be
Ipa = 430.960 mV s−1 + 7.707, R2 = 0.9974. |
4.3. Electrochemical sensing analysis of acetaminophen with GO-1–3/RGO-1–3 modified electrodes
To inspect the electrochemical sensing of acetaminophen, CV experiments with different fabricated electrodes, such as GO-1–3/GCE and laser-induced RGO-1–3/GCE, were conducted in 0.1 M of PBS as a supporting electrolyte solution in the presence of AP (100 μM) at neutral pH under inert conditions with a fixed scan rate of 50 mV s−1, as seen in Fig. S3B† and 6A, respectively. For the sake of comparison, the CV of bare GCE was also investigated with the same experimental parameters and is shown in Fig. 6A. The histogram in Fig. 6B shows redox peak responses with different fabricated electrodes.
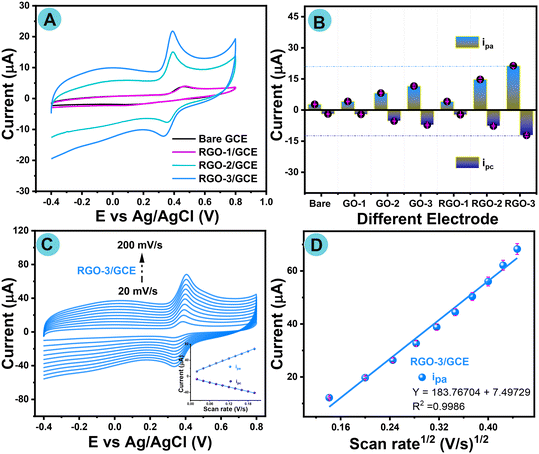 |
| Fig. 6 (A) Cyclic voltammograms of RGO-1–3/GCE in 0.1 M PB solution in the presence of AP (100 μM) under an N2 atmosphere with a scan rate of 50 mV s−1. (B) Histogram showing the current response for different modified electrodes. (C) Cyclic voltammograms of RGO-3/GCE in 0.1 M PBS in the presence of AP (100 μM) under an N2 atmosphere with different scan rates (20 to 200 mV s−1), inset: scan rate vs. current. (D) Square root of scan rates of the anodic peak for RGO-3/GCE. | |
The cyclic voltammogram of bare GCE exhibited poor sensitivity against the AP redox reaction, whereas the sensitivity to AP was progressively boosted in accordance with GCE electrode modification with RGO-1/GCE, RGO-2/GCE, and RGO-3/GCE, especially the cyclic voltammogram results of AP electrochemical sensing at RGO-3/GCE, as a result of the significantly improved active electrode surface areas. Furthermore, the histogram comparison plot confirms that a higher current response was noted for RGO-3/GCE compared with different modified GCEs. RGO-3/GCE shows a high current response of Ipa = 21.34 and Ipc = −12.2, which confirms a fast electron transfer phenomenon. There is clear evidence of an enhanced current response compared to those observed for GO/GCE and RGO/GCE electrodes.
Ultimately, the results show that the designed RGO-3 nanomaterial used for fabrication on GCE shows an extraordinary electrocatalytic capability for AP sensing. Although the RGO-3 potential (0.38 V) is close to that of GO-3 (0.42 V), the redox current response of RGO-3 is remarkably higher than that of parent GO-3, as shown in Fig. 6B. This is due to the impact of oxygen content by reduction (LIG). To further understand the kinetic reaction phenomenon of RGO-3/GCE, cyclic voltammetry with different scan rates (20 to 200 mV s−1) was also investigated under same experimental conditions. As-recorded cyclic voltammograms are displayed in Fig. 6C and S10A–F.† Cyclic voltammogram redox events did not shift towards either the negative or positive potentials. The inset to Fig. 6C shows oxidation (Ipa) and reduction (Ipc) peak currents with a good linear relationship with the varying scan rate. Fig. 6D shows good linear regression in the anodic peak (Ipa) current and with the square root of the scan rates. Following the least squares process, the linear regression equations were resolved as follows:
Ipa (μA) = 183.7670 + 7.4972V1/2 (mV1/2 s1/2); (R2 = 0.9986) |
These findings show that the electro-oxidation reaction of AP should be a diffusion-controlled electron transfer phenomenon at the RGO-3/GCE electrode, and the obtained results are better than for other reported chemically modified electrodes.
4.4. pH studies for the electrochemical detection of acetaminophen (AP) on RGO-3/GCE
The electrochemical redox performance of AP was investigated under different pH conditions ranging from a strong acid to a basic medium (pH 3.0 to 11.0), and the maximum current response was noted at neutral pH 7.0 in the presence of the same AP concentration of 100 μM (0.001 M) under a nitrogen atmosphere with a scan rate at 50 mV s−1. Fig. 7A–D show a more detailed peak potential and current response, and a plausible electrooxidation mechanism of acetaminophen at the RGO-3/GCE fabricated electrode. Fig. 7A shows cyclic voltammograms with increased current response to AP by varying the pH from 3.0 to 11.0 in PBS and it reached a maximum at pH 7.0, whereas the redox response then decreased with respect to varying basic pH up to 11.0.
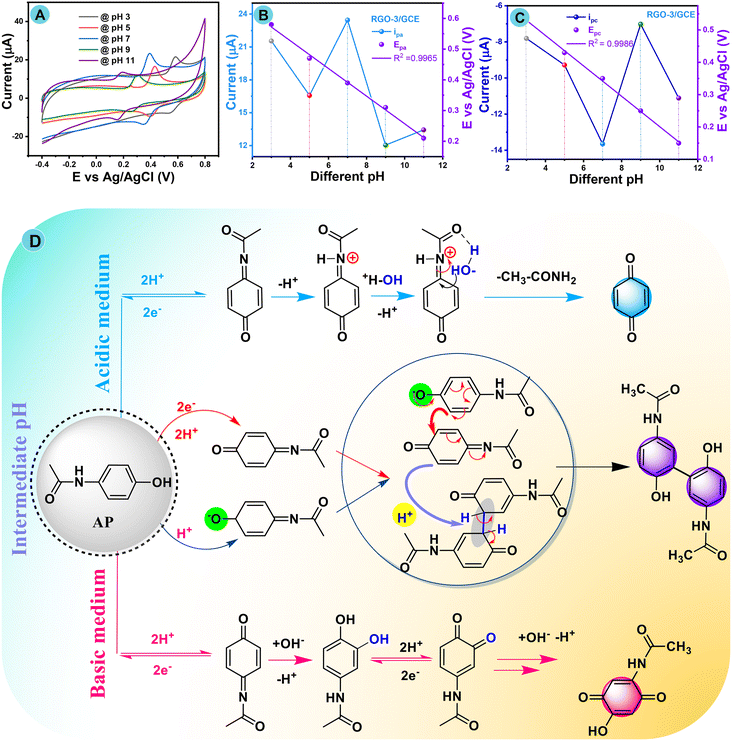 |
| Fig. 7 (A) Cyclic voltammograms of RGO-3/GCE in different pH media (0.1 M PBS) from 3.0 to 11.0 in the presence of AP 100 μM (0.001 M) under inert conditions at a scan rate of 50 mV s−1. (B) and (C) curves obtained from various pH (3–11) vs. AP peak current and peak potentials over RGO-3/GCE. (D) Plausible electrochemical redox mechanism of AP. | |
Fig. 7B and C show excellent evidence that together the anodic and cathodic peak potentials assigned to the acetaminophen electrode redox reaction have been influenced by different pH media and show a potential shift in the direction of the more negative or more positive side. This was also further confirmed from the plotting of Ipa, and EPavs. pH, as revealed in Fig. 7B and C. The change in dEp/dpH slope values closely matched the theoretical Nernstian value of 59 mV s−1, which again proves that the whole mechanism includes part transfer of the equivalent number of leaving protons and gaining electrons, in accordance with the linear relationship.95,96Fig. 7D shows a complete plausible electrochemical oxidation mechanism for AP at the RGO-3/GCE modified electrode. Briefly, N-acetyl-p-benzoquinone imine (NAPQI) is less stable in more basic (pH more than 9.0) and more acidic (pH less than 4.0) electrolytes in accordance with this pH study. In fact, many protons assist with NAPQI in acidic conditions (pH less than 4.0), which causes the synthesis of benzoquinone (Fig. 7D). However, in basic conditions (pH greater than 9.0), the presence of additional hydroxide ions causes NAPQI to become unstable. However, N-acetyl-p-benzoquinone imine has a stable form in the pH range of 5.0 to 9.0; intrinsic instability occurred in this pH range, possibly due to NAPQI dimerization.
Furthermore, the redox peak at ∼0.36 V represents the loss of the first electron, which forms a carboxylate group on the aryl. This is followed by electron rearrangement to form a dimer between the intermediate molecules with aromatization of an NAPQI dimer. The reaction then continues through a proton rearrangement reaction to generate an aryl dimer, which involves another electron loss. Finally, hydration takes place to yield the final product. Therefore, AP oxidizes to the final derivative from a strong acid to a basic medium, with the participation of a pair of electrons and protons, respectively, in accordance with earlier literature.97,98 The electrochemical pH assessment study strongly proved that pH 7.0 in a PB buffer solution is a suitable condition.
4.5. Different concentrations of acetaminophen (AP) by CV and DPV techniques
To further understand the anodic and cathodic potential shifts, a study of the conductivity of the proposed RGO-3/GCE modified electrode with different concentrations (20 to 400 μM) of AP was conducted again by CV in PBS as a supporting electrolyte medium at neutral pH in an inert atmosphere with a fixed scan rate of 50 mV s−1, and the as-recorded voltammograms are shown in Fig. 8A. The AP oxidation current peaks are located at around ∼0.38 V and AP reduction peaks are also positioned at ∼0.33 V, and no potential shifted peak is observed in the negative region, as shown in Fig. 8A. Cyclic voltammograms show that there is a linear increase in the redox peaks with increasing AP concentration. Fig. 8B shows a linear relationship between the increased acetaminophen concentration and redox peak current with linear regression. The linear regression was plotted for the anodic peak, with the equation y = 0.08016 ± 0.00286 with R2 = 0.9778, while the linear regression equation for the cathodic peak was y = −0.004425 ± 0.00107 with R2 = 0.9898.
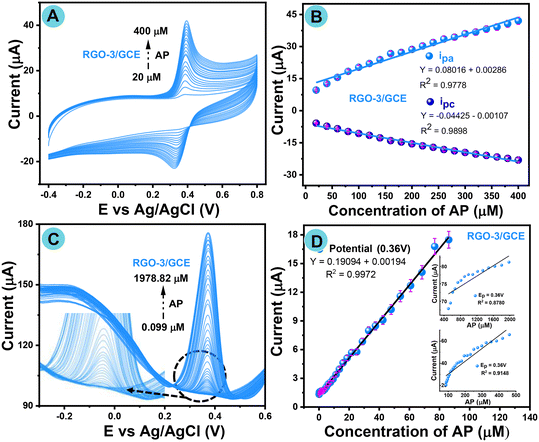 |
| Fig. 8 (A) Cyclic voltammograms of RGO-3/GCE for the consecutive injection of AP from 20 to 400 μM in PBS with N2-purging at 50 mV s−1. (B) Linearity plot for redox current vs. AP concentration. (C) DP voltammograms of RGO-3/GCE in 0.1 M of PBS for different additions of AP from 0.099 to 1978.82 μM (0.0001 M) under N2-purged conditions (inset: expanded view of lower μM). (D) Corresponding calibration curve for AP vs. current plot. | |
Fig. 8C shows the differential pulse voltammogram responses of the RGO-3/GCE modified electrode, with the consecutive addition of AP (0.099–1978.82 μM) in a supporting electrolyte at neutral pH 7.0 in a potential window ranging from −0.1 to 0.8 V at a fixed scan rate of 50 mV s−1 under an inert atmosphere. Fig. 8D shows the reliable AP current response plot with a correlation coefficient of 0.9972, with the same potential window of 0.36 V up to a high concentration of 1978.82 μM. The fabricated electrochemical sensor then showed excellent LOD (5.2 nM) and sensitivity (2.7271 μA Mm−1 cm−2) despite the comparison with earlier reported analytical performance of AP sensors (Table S6†). The proposed RGO-3/GCE proves tremendously comparable with prior analytical performance, and is thus a sensible platform for AP detection.
The reasons behind the efficient DPV response are related to the rapid electron transfer phenomenon, leading to excellent intermolecular communications between the fabricated electrode and AP in terms of the active surface area of RGO-3/GCE. The obtained results confirm the better reliability and practicability of the RGO-3/GCE fabricated electrode.
4.6. Interference study of RGO-3/GCE by the differential pulsed voltammetry technique
To gain additional information about the anti-interference properties of the RGO-3/GCE-modified electrode, DPV electrochemical studies were conducted with different interference species. Certain low interferents, such as ion species, organic substances, nicotinamide, 2-NP, 2-NA, DPA, glucose, dopamine, uric acid, and potassium ions (K+) were selected while encountering AP. Fig. S11A and B† show DP voltammograms of AP with interfering compounds (1
:
10 fold) at neutral pH 7.0 in N2-purged PB solution. The AP potential shown at 0.37 V is identical, but their currents differ slightly, bringing out the impact of the interferents. A relative bar histogram of anti-interference is shown in Fig. S11B† with different interference compounds versus a slight current response at 0.37 V. The anti-interference study has verified that there was an outstanding sensor response to AP.
4.7. Other sensing parameter studies of the RGO-3 fabricated GCE electrode
To gain a deeper understanding of the RGO-3 fabricated GCE electrode, electrochemical investigations, such as repeatability, reproducibility, cyclic stability, and long-term storage stability were conducted. Fig. S12A–D† shows cyclic and DP voltammograms recorded in the presence of AP in 0.1 M PBS as a supporting electrolyte under a nitrogen atmosphere with an appropriate scan rate. Repeatability analysis of the cyclic voltammograms determines the redox peak for five separate studies, and sensing of AP at RGO-3/GCE was consistent, as seen in Fig. S12A.† Reproducibility studies using three different GCE electrodes in the same experimental setup were evaluated. The as-acquired cyclic voltammograms are shown in Fig. S12B.† When detecting AP, the potential and oxidation peak performed in a similar manner, but there were slight differences in the anodic current, corresponding to the various GCEs that were used as working electrodes (GCE-1–3).
The RGO-3/GCE-fabricated electrode must be stable with the same potential and anodic peak current for use in sensors and real-sample analysis while resisting the effect of AP sensing on its own stability. Therefore, a stability investigation was also carried out for a long-term cycle of around 100 segments at RGO-3/GCE fabricated in the presence of AP, and it was shown that these segments exhibited outstanding electro-stability under the experimental conditions (Fig. S12C†). The long-term storage stability was further scrutinized by measuring current responses over 15 days using the same RGO-3/GCE, as presented in Fig. S12D.† As seen, the DP voltammogram response of all electrodes for AP sensing was within 5% deviation for all measurements. This slight deviation may be due to human or machine error, suggesting that the proposed sensor was highly stable. RGO-3/GCE may be considered a viable fabricated electrode for electrochemical sensors in real-time monitoring.
4.8. Real-sample analysis by DPV
The fabricated sensor was exploited in real-time use to verify its practicability and feasibility. The preparation of real sample solutions is described in ESI† S3(A–D). The designed electrode device was applied in the detection of AP in real samples by DPV, to estimate the μm concentration of AP in river, urine, and pharmaceutical (antipyretic) tablet samples. As collected, the real river and urine samples did not exhibit any kind of oxidation current response in 0.1 M buffer solution in the potential window from 0.2 to 0.8 V. During the standard addition of AP from low 25 to 125 μM concentration into the same PB solution, an oxidation peak matching AP was exhibited at ∼0.37 V, which confirmed that use of the fabricated sensor was extremely feasible for the detection of AP in urine, tablet, and river samples.
Fig. 9A–C show that during the consecutive standard addition of different micromolar concentrations of AP into the same PBS, the oxidation current was improved by reducing its potential, which also confirms the stability of the fabricated electrode in easy hands-on applications. The RGO-3 fabricated GCE electrode can also be well exploited for the sensing of AP in pharmaceutical tablets (Fig. 9B). It was revealed that the oxidation current peak around +0.40 V in drugs dissolved in PB solution may be attributable to the oxidation of AP. A known concentration of AP was further added into the same analyte solution, and it was found to increase the oxidation peak current, which should confirm the detected peaks in the paracetamol drug.
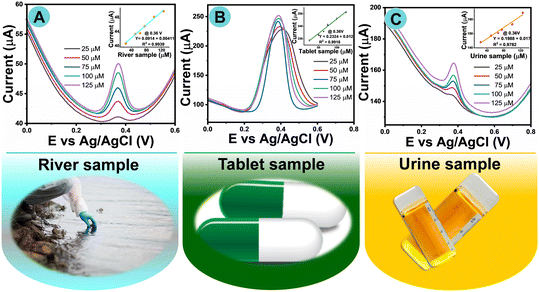 |
| Fig. 9 DP voltammograms for scrutiny of real samples: (A) river sample, (B) tablet sample, and (C) urine sample by standard addition of AP (inset: linear plots current vs. real samples). | |
Relative standard linear plots of AP recovery are shown in the insets to Fig. 9A–C. The recovery concentration percentages were calculated based on the corresponding calibration plots, which are provided in Table 1. The designed electrochemical sensor has the potential to be a viable platform for the detection of AP in biological and environmental samples, as proved by the good recovery values obtained from the experiment (Table 1). The real-time monitoring results authenticate the feasibility of the present RGO fabricated sensor with outstanding reproducibility as well as repeatability. It is feasible that the present RGO fabricated sensor could have a positive influence on society in the detection of AP in the future.
Table 1 Assessment of AP in spiked samples using RGO-3/GCE
Real samples |
Addeda (μM) |
Founda (μM) |
Recoverya (%) (n = 3) |
Average. (Standard addition (each 25 μM)).
|
River sample |
25–125 |
74.44 |
99.12 |
Tablet sample |
25–125 |
74.65 |
99.44 |
Urine sample |
25–125 |
74.57 |
99.27 |
5. Conclusions
We have proposed one-step direct nano-structuration of reduced graphene oxide from a bulky GO nanosheet. An experiment proved the feasibility of RGO fabricated by a 1065 nm-pulse laser in an open atmosphere. Combined with rigorous control over the operational laser variable, this change produced enhanced conductive LIG structures with very low sheet resistance, which corresponded to apparent conductivities. Additionally, the Raman spectra of LIG reveal highly attractive crystallinity and a low defect density was achieved. Characterization exhibited the laser-induced desorption of oxygen functional groups with improved well-ordered construction, and minimal defects because of an ID/IG ratio of 1.06 and a 2D-band at 2710 cm−1 for RGO-3. We undertook a thorough comparison of the AP sensing performance of RGO with that of several chemically modified RGO and physically modified RGO devices, which proved the advantages of this physically modified RGO owing to its higher effective surface area and rapid electron transfer ability. The physically modified RGO sensor was checked for the first time for the detection of AP from real samples including human urine. Under the optimized parameter settings, we found a linear concentration of AP 0.099–1987 μM with the lowest detection limit of 5.2 nM (S/N = 3) at the RGO-3 modified electrode. The established sensor revealed a superior sensitivity of 2.7271 μA Mm−1 cm−2 in DPV performance. The fabricated sensor was exploited to confirm its practicability by defining the concentration from a marketable AP drug in pharmaceutical formulations. Our strategy an ultimate route for an ideal GO reduction as RGO nanosheets that is crucial for low-cost, environmentally friendly, and large-scale manufacture of excellent-performance RGO.
Ethical approval
A declaration that no human tissue was used in this experiment. However, we have included Dr. Hwa's Institutional Review Board (IRB), certification number: IRB202010EM1003. Real sample analysis and everything has been done in accordance with the guidelines of the Institutional Review Board (IRB).
Author contributions
RKM – methodology, electrochemical analysis, visualization, validation, editing, manuscript-writing-original draft, review & editing; SFT – LIT analysis, visualization, formal analysis, AS – methodology, data curation, visualization, LIT analysis, formal analysis; JYL – LIT analysis; KYH – conceptualization, validation, project administration, funding acquisition, resource, revised manuscript.
Conflicts of interest
All authors declare no conflict of interest.
Acknowledgements
The authors express their gratitude to the National Taipei University of Technology for the support. The authors would also like to acknowledge the Ministry of Science and Technology (MOST) of Taiwan for the funding this project (MOST-111-2221-E-027-098-MY2). Further, the authors express their gratitude to the Precision Analysis and Material Research Center, National Taipei University of Technology, for the characterization support.
References
- M. J. Allen, V. C. Tung and R. B. Kaner, Honeycomb Carbon: A Review of Graphene, Chem. Rev., 2010, 110, 132–145 CrossRef CAS PubMed.
- X. J. Lee, B. Y. Z. Hiew, K. C. Lai, L. Y. Lee, S. Gan, S. Thangalazhy-Gopakumar and S. Rigby, Review on Graphene and Its Derivatives: Synthesis Methods and Potential Industrial Implementation, J. Taiwan Inst. Chem. Eng., 2019, 98, 163–180 CrossRef CAS.
- A. Ambrosi, C. K. Chua, A. Bonanni and M. Pumera, Electrochemistry of Graphene and Related Materials, Chem. Rev., 2014, 114, 7150–7188 CrossRef CAS PubMed.
- F. Farjadian, S. Abbaspour, M. A. A. Sadatlu, S. Mirkiani, A. Ghasemi, M. Hoseini-Ghahfarokhi, N. Mozaffari, M. Karimi and M. R. Hamblin, Recent Developments in Graphene and Graphene Oxide: Properties, Synthesis, and Modifications: A Review, ChemistrySelect, 2020, 5, 10200–10219 CrossRef CAS.
- K. P. Loh, Q. Bao, G. Eda and M. Chhowalla, Graphene Oxide as a Chemically Tunable Platform for Optical Applications, Nat. Chem., 2010, 2, 1015–1024 CrossRef CAS PubMed.
- H. Saleem, M. Haneef and H. Y. Abbasi, Synthesis Route of Reduced Graphene Oxide via Thermal Reduction of Chemically Exfoliated Graphene Oxide, Mater. Chem. Phys., 2018, 204, 1–7 CrossRef CAS.
- J. A. Quezada Renteria, C. Ruiz-Garcia, T. Sauvage, L. F. Chazaro-Ruiz, J. R. Rangel-Mendez and C. O. Ania, Photochemical and Electrochemical Reduction of Graphene Oxide Thin Films: Tuning the Nature of Surface Defects, Phys. Chem. Chem. Phys., 2020, 22, 20732–20743 RSC.
- Y. C. Guan, Y. W. Fang, G. C. Lim, H. Y. Zheng and M. H. Hong, Fabrication of Laser-Reduced Graphene Oxide in Liquid Nitrogen Environment, Sci. Rep., 2016, 6, 1–7 CrossRef PubMed.
- T. X. Tran, H. Choi, C. H. Che, J. H. Sul, I. G. Kim, S. M. Lee, J. H. Kim and J. Bin In, Laser-Induced Reduction of Graphene Oxide by Intensity-Modulated Line Beam for Supercapacitor Applications, ACS Appl. Mater. Interfaces, 2018, 10, 39777–39784 CrossRef CAS PubMed.
- R. Li, J. Song, S. Zhi, X. Wang, L. Wang, K. Jiang and D. Wu, Laser-Induced 3D Porous Flower-like Fe2O3/Reduced Graphene Oxide Modified Nickel Foam Electrode for Enhanced Capacitive Deionization, Desalination, 2023, 548, 116286 CrossRef CAS.
- V. Strauss, M. Muni, A. Borenstein, B. Badamdorj, T. Heil, M. D. Kowal and R. Kaner, Patching Laser-Reduced Graphene Oxide with Carbon Nanodots, Nanoscale, 2019, 11, 12712–12719 RSC.
- J. K. Wassei and R. B. Kaner, the Places You'll Go with Graphene, Acc. Chem. Res., 2013, 46, 2244–2253 CrossRef CAS PubMed.
- I. Naseri, M. Ziaee, Z. N. Nilsson, D. R. Lustig and M. Yourdkhani, Electrothermal Performance of Heaters Based on Laser-Induced Graphene on Aramid Fabric, ACS Omega, 2022, 7, 3746–3757 CrossRef CAS PubMed.
- M. Burke, C. Larrigy, E. Vaughan, G. Paterakis, L. Sygellou, A. J. Quinn, G. Herzog, C. Galiotis and D. Iacopino, Fabrication and Electrochemical Properties of Three-Dimensional (3D) Porous Graphitic and Graphenelike Electrodes Obtained by Low-Cost Direct Laser Writing Methods, ACS Omega, 2020, 5, 1540–1548 CrossRef CAS PubMed.
- C. Cheng, C. Zhang, X. Gao, Z. Zhuang, C. Du and W. Chen, 3D Network and 2D Paper of Reduced Graphene Oxide/Cu2O Composite for Electrochemical Sensing of Hydrogen Peroxide, Anal. Chem., 2018, 90, 1983–1991 CrossRef CAS PubMed.
- F. Sun, H. Jiang, H. Wang, Y. Zhong, Y. Xu, Y. Xing, M. Yu, L. W. Feng, Z. Tang, J. Liu, H. Sun, H. Wang, G. Wang and M. Zhu, Soft Fiber Electronics Based on Semiconducting Polymer, Chem. Rev., 2023, 123, 4693–4763 CrossRef CAS PubMed.
- J. Min, J. Tu, C. Xu, H. Lukas, S. Shin, Y. Yang, S. A. Solomon, D. Mukasa and W. Gao, Skin-Interfaced Wearable Sweat Sensors for Precision Medicine, Chem. Rev., 2023, 123, 5049–5138 CrossRef CAS PubMed.
- Q. Li, Y. Xia, X. Wan, S. Yang, Z. Cai, Y. Ye and G. Li, Morphology-Dependent MnO2/Nitrogen-Doped Graphene Nanocomposites for Simultaneous Detection of Trace Dopamine and Uric Acid, Mater. Sci. Eng., C, 2020, 109, 110615 CrossRef CAS PubMed.
- J. W. Zhang and X. Zhang, Electrode Material Fabricated by Loading Cerium Oxide Nanoparticles on Reduced Graphene Oxide and Its Application in Electrochemical Sensor for Tryptophan, J. Alloys Compd., 2020, 842, 155934 CrossRef CAS.
- G. Li, X. Qi, J. Wu, X. Wan, T. Wang, Y. Liu, Y. Chen and Y. Xia, Highly Stable Electrochemical Sensing Platform for the Selective Determination of Pefloxacin in Food Samples Based on a Molecularly Imprinted-Polymer-Coated Gold Nanoparticle/Black Phosphorus Nanocomposite, Food Chem., 2024, 436, 137753 CrossRef CAS PubMed.
- X. Zhang, J. W. Zhang, P. H. Xiang and J. Qiao, Fabrication of Graphene-Fullerene Hybrid by Self-Assembly and Its Application as Support Material for Methanol Electrocatalytic Oxidation Reaction, Appl. Surf. Sci., 2018, 440, 477–483 CrossRef CAS.
- M. Maria Stanley, A. Sherlin V, S.-F. Wang, J. N. Baby, B. Sriram and M. George, Deep Eutectic Solvent Assisted Synthesis of Molybdenum Nitride Entrapped Graphene Aerogel Heterostructure with Enhanced Electrochemical Behavior for Ronidazole Drug Detection, J. Mol. Liq., 2023, 375, 121308 CrossRef CAS.
- J. W. Zhang, K. P. Wang and X. Zhang, Fabrication of SnO2 Decorated Graphene Composite Material and Its Application in Electrochemical Detection of Caffeic Acid in Red Wine, Mater. Res. Bull., 2020, 126, 110820 CrossRef CAS.
- Y. Xia, G. Li, Y. Zhu, Q. He and C. Hu, Facile Preparation of Metal-Free Graphitic-like Carbon Nitride/Graphene Oxide Composite for Simultaneous Determination of Uric Acid and Dopamine, Microchem. J., 2023, 190, 108726 CrossRef CAS.
- X. Wan, H. Du, D. Tuo, X. Qi, T. Wang, J. Wu and G. Li, UiO-66/Carboxylated Multiwalled Carbon Nanotube Composites for Highly Efficient and Stable Voltammetric Sensors for Gatifloxacin, ACS Appl. Nano Mater., 2023, 6(20), 19403–19413 CrossRef CAS.
- B. Sriram, J. N. Baby, Y. F. Hsu, S. F. Wang and M. George, Surfactant-Assisted Synthesis of Praseodymium Orthovanadate Nanofiber-Supported NiFe-Layered Double Hydroxide Bifunctional Catalyst: The Electrochemical Detection and Degradation of Diphenylamine, Inorg. Chem., 2022, 61, 5824–5835 CrossRef CAS PubMed.
- B. Sriram, J. N. Baby, S. F. Wang, M. George, X. B. Joseph and J. T. Tsai, Surface Engineering of Three-Dimensional-like Hybrid AB2O4(AB = Zn, Co, and Mn) Wrapped on Sulfur-Doped Reduced Graphene Oxide: Investigation of the Role of an Electrocatalyst for Clioquinol Detection, ACS Appl. Electron. Mater., 2021, 3, 362–372 CrossRef CAS.
- G. Li, X. Wan, Y. Xia, D. Tuo, X. Qi, T. Wang, M. Mehmandoust, N. Erk, Q. He and Q. Li, Lamellar α-Zirconium Phosphate Nanoparticles Supported on N-Doped Graphene Nanosheets as Electrocatalysts for the Detection of Levofloxacin, ACS Appl. Nano Mater., 2023, 6(18), 17040–17052 CrossRef CAS.
- G. Li, Y. Xia, Y. Tian, Y. Wu, J. Liu, Q. He and D. Chen, Review—Recent Developments on Graphene-Based Electrochemical Sensors toward Nitrite, J. Electrochem. Soc., 2019, 166, B881–B895 CrossRef CAS.
- G. Li, J. Wu, X. Qi, X. Wan, Y. Liu, Y. Chen and L. Xu, Molecularly Imprinted Polypyrrole Film-Coated Poly(3,4-Ethylenedioxythiophene):Polystyrene Sulfonate-Functionalized Black Phosphorene for the Selective and Robust Detection of Norfloxacin, Mater. Today Chem., 2022, 26, 101043 CrossRef CAS.
- F. Li, B. Ni, Y. Zheng, Y. Huang and G. Li, A Simple and Efficient Voltammetric Sensor for Dopamine Determination Based on ZnO Nanorods/Electro-Reduced Graphene Oxide, Surf. Interfaces, 2021, 26, 101375 CrossRef CAS.
- C. Karthikeyan, K. Ramachandran, S. Sheet, D. J. Yoo, Y. S. Lee, Y. Satish Kumar, A. R. Kim and G. Gnana Kumar, Pigeon-Excreta-Mediated Synthesis of Reduced Graphene Oxide (RGO)/CuFe2O4 Nanocomposite and Its Catalytic Activity toward Sensitive and Selective Hydrogen Peroxide Detection, ACS Sustainable Chem. Eng., 2017, 5, 4897–4905 CrossRef CAS.
- J. C. Gabunada, M. Vinothkannan, D. H. Kim, A. R. Kim and D. J. Yoo, Magnetite Nanorods Stabilized by Polyaniline/Reduced Graphene Oxide as a Sensing Platform for Selective and Sensitive Non-Enzymatic Hydrogen Peroxide Detection, Electroanalysis, 2019, 31, 1524–1533 CrossRef CAS.
- G. Amala, J. Saravanan, D. Jin Yoo, A. R. Kim and G. Gnana Kumar, An Environmentally Benign One Pot Green Synthesis of Reduced Graphene Oxide Based Composites for the Enzyme Free Electrochemical Detection of Hydrogen Peroxide, New J. Chem., 2017, 41, 4022–4030 RSC.
- R. Santhosh Kumar, K. Govindan, S. Ramakrishnan, A. R. Kim, J. S. Kim and D. J. Yoo, Fe3O4 Nanorods Decorated on Polypyrrole/Reduced Graphene Oxide for Electrochemical Detection of Dopamine and Photocatalytic Degradation of Acetaminophen, Appl. Surf. Sci., 2021, 556, 149765 CrossRef CAS.
- D. J. Johnson and N. Hilal, Can Graphene and Graphene Oxide Materials Revolutionise Desalination Processes?, Desalination, 2021, 500, 114852 CrossRef CAS.
- Y. Rao, M. Yuan, F. Luo, Z. Wang, H. Li, J. Yu and X. Chen, One-Step Laser Fabrication of Phosphorus-Doped Porous Graphene Electrodes for High-Performance Flexible Microsupercapacitor, Carbon, 2021, 180, 56–66 CrossRef CAS.
- Z. Wan, E. W. Streed, M. Lobino, S. Wang, R. T. Sang, I. S. Cole, D. V. Thiel and Q. Li, Laser-Reduced Graphene: Synthesis, Properties, and Applications, Adv. Mater. Technol., 2018, 3, 1–19 Search PubMed.
- R. Ye, Y. Chyan, J. Zhang, Y. Li, X. Han, C. Kittrell and J. M. Tour, Laser-Induced Graphene Formation on Wood, Adv. Mater., 2017, 29, 1–7 Search PubMed.
-
R. Arul, R. N. Oosterbeek, B. P. P. Mallett and M. C. Simpson, Laser Direct-Writing Graphene Oxide to Graphene-Mechanisms to Applications, Handb. Graphene, 2019, vol. 8, pp. 237–287 Search PubMed.
- R. Trusovas, K. Ratautas, G. Račiukaitis, J. Barkauskas, I. Stankevičiene, G. Niaura and R. Mažeikiene, Reduction of Graphite Oxide to Graphene with Laser Irradiation, Carbon, 2013, 52, 574–582 CrossRef CAS.
- Y. L. Zhang, L. Guo, H. Xia, Q. D. Chen, J. Feng and H. B. Sun, Photoreduction of Graphene Oxides: Methods, Properties, and Applications, Adv. Opt. Mater., 2014, 2, 10–28 CrossRef.
- V. Abdelsayed, S. Moussa, H. M. Hassan, H. S. Aluri, M. M. Collinson and M. S. El-Shall, Photothermal Deoxygenation of Graphite Oxide with Laser Excitation in Solution and Graphene-Aided Increase in Water Temperature, J. Phys. Chem. Lett., 2010, 1, 2804–2809 CrossRef CAS.
- E. E. Ghadim, N. Rashidi, S. Kimiagar, O. Akhavan, F. Manouchehri and E. Ghaderi, Pulsed Laser Irradiation for Environment Friendly Reduction of Graphene Oxide Suspensions, Appl. Surf. Sci., 2014, 301, 183–188 CrossRef CAS.
- L. V. Thekkekara, B. Jia, Y. Zhang, L. Qiu, D. Li and M. Gu, On-Chip Energy Storage Integrated with Solar Cells Using a Laser Scribed Graphene Oxide Film, Appl. Phys. Lett., 2015, 107, 031105 CrossRef.
- Q. An, A. N. Rider and E. T. Thostenson, Electrophoretic Deposition of Carbon Nanotubes onto Carbon-Fiber Fabric for Production of Carbon/Epoxy Composites with Improved Mechanical Properties, Carbon, 2012, 50, 4130–4143 CrossRef CAS.
- J. Ortiz Balbuena, P. Tutor De Ureta, E. Rivera Ruiz and S. Mellor Pita, Enfermedad de Vogt-Koyanagi-Harad, Med. Clin., 2016, 146, 93–94 CrossRef PubMed.
- Y. Zhang, L. Zhang and C. Zhou, Review of Chemical Vapor Deposition of Graphene and Related Applications, Acc. Chem. Res., 2013, 46, 2329–2339 CrossRef CAS PubMed.
- I. V. Pylypchuk, V. G. Kessler and G. A. Seisenbaeva, Simultaneous Removal of Acetaminophen, Diclofenac, and Cd(II) by Trametes Versicolor Laccase Immobilized on Fe3O4/SiO2-DTPA Hybrid Nanocomposites, ACS Sustainable Chem. Eng., 2018, 6, 9979–9989 CrossRef CAS.
- K. Wang, K. Huang and G. Jiang, Enhanced Removal of Aqueous Acetaminophen by a Laccase-Catalyzed Oxidative Coupling Reaction under a Dual-PH Optimization Strategy, Sci. Total Environ., 2018, 616–617, 1270–1278 CrossRef CAS PubMed.
- A. Cernat, M. Tertiş, R. Săndulescu, F. Bedioui, A. Cristea and C. Cristea, Electrochemical Sensors Based on Carbon Nanomaterials for Acetaminophen Detection: A Review, Anal. Chim. Acta, 2015, 886, 16–28 CrossRef CAS PubMed.
- P. Zhao, M. Ni, C. Chen, Z. Zhou, X. Li, C. Li, Y. Xie and J. Fei, Stimuli-Enabled Switch-like Paracetamol Electrochemical Sensor Based on Thermosensitive Polymer and MWCNTs-GQDs Composite Nanomaterial, Nanoscale, 2019, 11, 7394–7403 RSC.
- T. Y. Huang, C. W. Kung, H. Y. Wei, K. M. Boopathi, C. W. Chu and K. C. Ho, A High Performance Electrochemical Sensor for Acetaminophen Based on a RGO-PEDOT Nanotube Composite Modified Electrode, J. Mater. Chem. A, 2014, 2, 7229–7237 RSC.
- N. Rohaizad, C. C. Mayorga-Martinez, Z. Sofer and M. Pumera, 1T-Phase Transition Metal Dichalcogenides (MoS2, MoSe2, WS2, and WSe2) with Fast Heterogeneous Electron Transfer: Application on Second-Generation Enzyme-Based Biosensor, ACS Appl. Mater. Interfaces, 2017, 9, 40697–40706 CrossRef CAS PubMed.
- G. Maduraiveeran, M. Sasidharan and V. Ganesan, Electrochemical Sensor and Biosensor Platforms Based on Advanced Nanomaterials for Biological and Biomedical Applications, Biosens. Bioelectron., 2018, 103, 113–129 CrossRef CAS PubMed.
- K. Y. Hwa, A. Santhan, A. Ganguly and T. S. Kanna Sharma, Two Dimensional Architectures of Graphitic Carbon Nitride with the Substitution of Heteroatoms for Bifunctional Electrochemical Detection of Nilutamide, Chemosphere, 2023, 320, 138068 CrossRef CAS PubMed.
- K. Y. Hwa, A. Santhan, A. Ganguly and T. S. K. Sharma, Point of Need Simultaneous Biosensing of Pharmaceutical Micropollutants with Binder Free Conjugation of Manganese Stannate Micro-Rods on Reduced Graphene Oxide in Real-Time Analysis, J. Taiwan Inst. Chem. Eng., 2022, 131, 104135 CrossRef CAS.
- K. Y. Hwa, A. Ganguly, A. Santhan and T. S. K. Sharma, Construction of Three-Dimensional/One-Dimensional Heterostructure of Flower-like Sr Nanoflowers on Se Microrods Decorated on Reduced Graphene Oxide: An Efficient Electrocatalyst for Oxidation of Promethazine Hydrochloride, Mater. Today Chem., 2022, 23, 100654 CrossRef CAS.
- J. Kim, J. H. Eum, J. Kang, O. Kwon, H. Kim and D. W. Kim, Tuning the Hierarchical Pore Structure of Graphene Oxide through Dual Thermal Activation for High-Performance Supercapacitor, Sci. Rep., 2021, 11, 1–10 CrossRef PubMed.
- C. Liu, J. Wang, J. Li, R. Luo, J. Shen, X. Sun, W. Han and L. Wang, Controllable Synthesis of Functional Hollow Carbon Nanostructures with Dopamine As Precursor for Supercapacitors, ACS Appl. Mater. Interfaces, 2015, 7, 18609–18617 CrossRef CAS PubMed.
- P. Zhang, L. Chen, L. Ge, P. Song, R. Xie, B. Wang, Y. Fu, S. Jia, T. Liao and Y. Xiong, A 3D RGO-Supported NiFe2O4 Heterostructure from Sacrificial Polymer-Assisted Exfoliation of NiFe-LDH for Efficient Oxygen Evolution Reaction, Carbon, 2022, 200, 422–429 CrossRef CAS.
- Y. Ma, J. Guo, Y. Chen, Y. Yi and G. Zhu, Electrochemical Sensing of Phenolics Based on Copper/Cobalt/Nitrogen Co-Doped Hollow Nanocarbon Spheres, J. Electroanal. Chem., 2021, 892, 115263 CrossRef CAS.
- T. Liu, L. Zhang, B. Cheng and J. Yu, Hollow Carbon Spheres and Their Hybrid Nanomaterials in Electrochemical Energy Storage, Adv. Energy Mater., 2019, 9, 1–55 Search PubMed.
- D. C. Marcano, D. V. Kosynkin, J. M. Berlin, A. Sinitskii, Z. Sun, A. Slesarev, L. B. Alemany, W. Lu and J. M. Tour, Improved Synthesis of Graphene Oxide, ACS Nano, 2010, 4, 4806–4814 CrossRef CAS PubMed.
- A. K. Das, M. Srivastav, R. K. Layek, M. E. Uddin, D. Jung, N. H. Kim and J. H. Lee, Iodide-Mediated Room Temperature Reduction of Graphene Oxide: A Rapid Chemical Route for the Synthesis of a Bifunctional Electrocatalyst, J. Mater. Chem. A, 2014, 2, 1332–1340 RSC.
- B. S. de Lima, M. I. B. Bernardi and V. R. Mastelaro, Wavelength Effect of Ns-Pulsed Radiation on the Reduction of Graphene Oxide, Appl. Surf. Sci., 2020, 506, 144808 CrossRef CAS.
- S. Park, S. G. Ji, Y. Yoon, S. K. Kim, W. Song, S. Myung, J. Lim, H. K. Jung, S. S. Lee and K. S. An, Fabrication of Fanlike L-Shaped Graphene Nanostructures with Enhanced Thermal/Electrochemical Properties via Laser Irradiation, Carbon, 2021, 182, 691–699 CrossRef CAS.
- L. J. Cote, R. Cruz-Silva and J. Huang, Flash Reduction and Patterning of Graphite Oxide and Its Polymer Composite, J. Am. Chem. Soc., 2009, 131, 11027–11032 CrossRef CAS PubMed.
- C. Xu, J. Gao, H. Xiu, X. Li, J. Zhang, F. Luo, Q. Zhang, F. Chen and Q. Fu, Can in Situ Thermal Reduction Be a Green and Efficient Way in the Fabrication of Electrically Conductive Polymer/Reduced Graphene Oxide Nanocomposites?, Composites, Part A, 2013, 53, 24–33 CrossRef CAS.
- O. Akhavan, M. Abdolahad, A. Esfandiar and M. Mohatashamifar, Photodegradation of Graphene Oxide Sheets by TiO2 Nanoparticles after a Photocatalytic Reduction, J. Phys. Chem. C, 2010, 114, 12955–12959 CrossRef CAS.
- C. K. Chua and M. Pumera, Chemical Reduction of Graphene Oxide: A Synthetic Chemistry Viewpoint, Chem. Soc. Rev., 2014, 43, 291–312 RSC.
- R. Larciprete, S. Fabris, T. Sun, P. Lacovig, A. Baraldi and S. Lizzit, Dual Path Mechanism in the Thermal Reduction of Graphene Oxide, J. Am. Chem. Soc., 2011, 133, 17315–17321 CrossRef CAS PubMed.
- R. Trusovas, G. Račiukaitis, G. Niaura, J. Barkauskas, G. Valušis and R. Pauliukaite, Recent Advances in Laser Utilization in the Chemical Modification of Graphene Oxide and Its Applications, Adv. Opt. Mater., 2016, 4, 37–65 CrossRef CAS.
- R. D. Rodriguez, G. V. Murastov, A. Lipovka, M. I. Fatkullin, O. Nozdrina, S. K. Pavlov, P. S. Postnikov, M. M. Chehimi, J. J. Chen and E. Sheremet, High-Power Laser-Patterning Graphene Oxide: A New Approach to Making Arbitrarily-Shaped Self-Aligned Electrodes, Carbon, 2019, 151, 148–155 CrossRef CAS.
- H. H. Shi, S. Jang and H. E. Naguib, Freestanding Laser-Assisted Reduced Graphene Oxide Microribbon Textile Electrode Fabricated on a Liquid Surface for Supercapacitors and Breath Sensors, ACS Appl. Mater. Interfaces, 2019, 11, 27183–27191 CrossRef CAS PubMed.
- N. D. Orekhov, J. V. Bondareva, D. O. Potapov, P. V. Dyakonov, O. N. Dubinin, M. A. Tarkhov, G. D. Diudbin, K. I. Maslakov, M. A. Logunov, D. G. Kvashnin and S. A. Evlashin, Mechanism of Graphene Oxide Laser Reduction at Ambient Conditions: Experimental and ReaxFF Study, Carbon, 2022, 191, 546–554 CrossRef CAS.
- S. W. Kim, J. Y. Cho, K. Bin Min, S. R. Lee, J. T. Han and Y. K. Kim, Exploring the Chemical Structures and Phototochemical Properties of Graphene Oxide Derivatives by Laser Desorption/Ionization Time-of-Flight Mass Spectrometry to Develop an Efficient Platform for Mass Spectrometric Analysis, ACS Omega, 2022, 7, 43092–43101 CrossRef CAS PubMed.
- X. Zheng, B. Jia, X. Chen and M. Gu, In Situ Third-Order Non-Linear Responses during Laser Reduction of Graphene Oxide Thin Films towards on-Chip Non-Linear Photonic Devices, Adv. Mater., 2014, 26, 2699–2703 CrossRef CAS PubMed.
- M. J. Low, H. Lee, C. H. J. Lim, C. S. Suchand Sandeep, V. M. Murukeshan, S. W. Kim and Y. J. Kim, Laser-Induced Reduced-Graphene-Oxide Micro-Optics Patterned by Femtosecond Laser Direct Writing, Appl. Surf. Sci., 2020, 526, 146647 CrossRef CAS.
- A. Scroccarello, R. Álvarez-Diduk, F. Della Pelle, C. de Carvalho Castro e Silva, A. Idili, C. Parolo, D. Compagnone and A. Merkoçi, One-Step Laser Nanostructuration of Reduced Graphene Oxide Films Embedding Metal Nanoparticles for Sensing Applications, ACS Sens., 2023, 8, 598–609 CrossRef CAS PubMed.
- L. Wang, J. Ding, Y. Chai, Q. Liu, J. Ren, X. Liu and W. L. Dai, CeO2 Nanorod/g-C3N4/N-RGO Composite: Enhanced Visible-Light-Driven Photocatalytic Performance and the Role of N-RGO as Electronic Transfer Media, Dalton Trans., 2015, 44, 11223–11234 RSC.
- Z. J. Fan, W. Kai, J. Yan, T. Wei, L. J. Zhi, J. Feng, Y. M. Ren, L. P. Song and F. Wei, Facile Synthesis of Graphene Nanosheets via Fe Reduction of Exfoliated Graphite Oxide, ACS Nano, 2011, 5, 191–198 CrossRef CAS PubMed.
- B. Gupta, N. Kumar, K. Panda, V. Kanan, S. Joshi and I. Visoly-Fisher, Role of Oxygen Functional Groups in Reduced Graphene Oxide for Lubrication, Sci. Rep., 2017, 7, 1–14 CrossRef CAS PubMed.
- K. T. Paula, M. V. Santos, M. H. M. Facure, M. B. Andrade, F. L. Araújo, D. S. Correa, S. J. L. Ribeiro and C. R. Mendonça, Laser Patterning and Induced Reduction of Graphene Oxide Functionalized Silk Fibroin, Opt. Mater., 2020, 99, 109540 CrossRef CAS.
- S. Park, J. An, R. D. Piner, I. Jung, D. Yang, A. Velamakanni, S. B. T. Nguyen and R. S. Ruoff, Aqueous Suspension and Characterization of Chemically Modified Graphene Sheets, Chem. Mater., 2008, 20, 6592–6594 CrossRef CAS.
- H. Wang and Y. H. Hu, Effect of Oxygen Content on Structures of Graphite Oxides, Ind. Eng. Chem. Res., 2011, 50, 6132–6137 CrossRef CAS.
- J. Zhang, Y. Xu, Z. Liu, W. Yang and J. Liu, A Highly Conductive Porous Graphene Electrode Prepared via in Situ Reduction of Graphene Oxide Using Cu Nanoparticles for the Fabrication of High Performance Supercapacitors, RSC Adv., 2015, 5, 54275–54282 RSC.
- F. T. Johra and W. G. Jung, Hydrothermally Reduced Graphene Oxide as a Supercapacitor, Appl. Surf. Sci., 2015, 357, 1911–1914 CrossRef CAS.
- A. Ganguly, K. Y. Hwa, A. Santhan and T. S. Kanna Sharma, Strategic Orchestration of MoSe2 Microspheres on β-Cd Functionalized RGO: A Sustainable Electrocatalyst for Detection of Rifampicin in Real Samples, Chemosphere, 2022, 307, 135373 CrossRef CAS PubMed.
- V. R. Moreira, Y. A. R. Lebron, M. M. da Silva, L. V. de Souza Santos, R. S. Jacob, C. K. B. de Vasconcelos and M. M. Viana, Graphene Oxide in the Remediation of Norfloxacin from Aqueous Matrix: Simultaneous Adsorption and Degradation Process, Environ. Sci. Pollut. Res., 2020, 27, 34513–34528 CrossRef CAS PubMed.
- B. Gupta, N. Kumar, K. Panda, V. Kanan, S. Joshi and I. Visoly-Fisher, Role of Oxygen Functional Groups in Reduced Graphene Oxide for Lubrication, Sci. Rep., 2017, 7, 1–14 CrossRef CAS PubMed.
- H. C. Youn, S. M. Bak, M. S. Kim, C. Jaye, D. A. Fischer, C. W. Lee, X. Q. Yang, K. C. Roh and K. B. Kim, High-Surface-Area Nitrogen-Doped Reduced Graphene Oxide for Electric Double-Layer Capacitors, ChemSusChem, 2015, 8, 1875–1884 CrossRef CAS PubMed.
- P. W. Albers, V. Leich, A. J. Ramirez-Cuesta, Y. Cheng, J. Hönig and S. F. Parker, The Characterisation of Commercial 2D Carbons: Graphene, Graphene Oxide and Reduced Graphene Oxide, Mater. Adv., 2022, 3, 2810–2826 RSC.
- N. Elgrishi, K. J. Rountree, B. D. McCarthy, E. S. Rountree, T. T. Eisenhart and J. L. Dempsey, A Practical Beginner's Guide to Cyclic Voltammetry, J. Chem. Educ., 2018, 95, 197–206 CrossRef CAS.
- T. Łuczak, Highly Selective Voltammetric Sensing of Paracetamol on Nanogold Modified Electrode in the Presence of Interfering Compounds, New J. Chem., 2017, 41, 5713–5722 RSC.
- R. Nehru, Y. F. Hsu, S. F. Wang, C. W. Chen and C. Di Dong, Selective Electrochemical Sensing Platform Based on the Synergy between Carbon Black and Single-Crystalline Bismuth Sulfide for Rapid Analysis of Antipyretic Drugs, ACS Appl. Bio Mater., 2021, 4, 7497–7508 CrossRef CAS PubMed.
- T. W. Tseng, T. W. Chen, S. M. Chen, T. Kokulnathan, F. Ahmed, P. M. Z. Hasan, A. L. Bilgrami and S. Kumar, Construction of Strontium Phosphate/Graphitic-Carbon Nitride: A Flexible and Disposable Strip for Acetaminophen Detection, J. Hazard. Mater., 2021, 410, 124542 CrossRef CAS PubMed.
- N. Karikalan, R. Karthik, S. M. Chen, M. Velmurugan and C. Karuppiah, Electrochemical Properties of the Acetaminophen on the Screen Printed Carbon Electrode towards the High Performance Practical Sensor Applications, J. Colloid Interface Sci., 2016, 483, 109–117 CrossRef CAS PubMed.
|
This journal is © The Royal Society of Chemistry 2024 |