Strontium-90 pollution can be bioremediated with the green microalga Tetraselmis chui†
Received
3rd August 2023
, Accepted 5th February 2024
First published on 6th February 2024
Abstract
Strontium-90 (90Sr) is an artificial radioisotope produced by nuclear fission, with a relatively long half-life of 29 years. This radionuclide is released into the environment in the event of a nuclear incident, posing a serious risk to human and ecosystem health. There is a need to develop new efficient methods for the remediation of 90Sr, as current techniques for its removal have significant technical limitations and involve high energy and economic costs. Recently, several species of green microalgae within the class Chlorodendrophyceae have been found to form intracellular mineral inclusions of amorphous calcium carbonate (ACC), which can be highly enriched in natural (non-radiogenic) Sr. As bioremediation techniques are an attractive option to address radioactive pollution, we investigated the capacity of the unicellular alga Tetraselmis chui (class Chlorodendrophyceae) to sequester 90Sr. The 90Sr uptake capacity of T. chui cells was assessed in laboratory cultures by monitoring the time course of radioactivity in the culture medium using liquid scintillation counting (LSC). T. chui was shown to effectively sequester 90Sr, reducing the initial radioactivity of the culture medium by up to 50%. Thus, this study demonstrates the potential of the microalga T. chui to be used as a bioremediation agent against 90Sr pollution.
Environmental significance
This study addresses the urgent issue of strontium-90 pollution, a hazardous radioactive isotope with a relatively long half-life. Human activities, such as nuclear weapons testing and nuclear accidents, have released this radionuclide into the environment, posing significant risks to human and ecosystem health. Current disposal methods are limited and costly. Our research introduces a promising solution using the green microalga Tetraselmis chui as an effective bioremediation agent against strontium-90 pollution, offering a sustainable and potentially more efficient approach to mitigate its harmful impacts on the environment and safeguarding the well-being of our ecosystems.
|
Introduction
Radioactive contamination of ecosystems is a major environmental problem that poses a serious risk to wildlife and human health.1 The presence of radioisotopes in the environment can be of natural origin (e.g., weathering of U- or Th-rich minerals) or due to human activities such as mining, nuclear reprocessing activities, detonation of nuclear weapons, and nuclear accidents.2,3 The radionuclide strontium-90 (90Sr) is one of the most hazardous anthropogenic isotopes, with a relatively long half-life (T1/2) of 28.91 years (compared to the human lifespan and to other well-known harmful radionuclides such as iodine-131 (131I) (T1/2 = 8 days)).2 Strontium-90, through β− decay (Q− = 546 keV), is transformed into yttrium-90 (90Y) (T1/2 = 64.05 hours), which in turn decays by β− radiation (Q− = 2280 keV) into stable zirconium-90 (90Zr).
Produced by nuclear fission, 90Sr can be released into the environment and bioaccumulate as it easily moves up the food chain.4 Nuclear disasters such as Kyshtym (1957), Chernobyl (1986), and Fukushima (2011), as well as past nuclear weapons tests (1945–1980), represent the main source of 90Sr contamination.5 Due to its high mobility, hazardous concentrations of this radionuclide have been detected in soils, sediments, waterbodies, fauna, and flora.6,7 Human exposure to 90Sr occurs mainly through the ingestion of contaminated food and water.5 Due to its biochemical similarity to Ca, 90Sr accumulates in the bone marrow, leading to chronic suppression of the immune function, hematopoietic bone marrow destruction, leukemia, and cancer.8
Traditional physicochemical techniques to remove radionuclides include processes such as precipitation, coagulation, ion exchange with resins, solvent extraction, selective adsorption, nanofiltration, reverse osmosis, and electrokinetic treatment.5,9,10 However, these methods have technical limitations and high economic and energy costs, which makes the development of new effective bioremediation techniques very attractive.11–14
The microalgae Scenedesmus spinosus and Closterium moniliferum have been suggested as potential 90Sr bioremediation agents due to their high capacity to remove stable Sr from their living environment. S. spinosus selectively adsorbs Sr on the cell wall, while C. moniliferum sequesters Sr by intracellular precipitation of (Ba,Sr)SO4 crystals.15,16 Recently, many species of green microalgae of the class of Chlorodendrophyceae have been shown to form intracellular inclusions of amorphous calcium carbonate (ACC), called micropearls, that can contain high concentrations of natural Sr.17 This suggests their possible use for 90Sr bioremediation purposes. The use of these microalgae would be of particular interest because they live in very diverse aquatic environments and some species such as Tetraselmis suecica and Tetraselmis chui are already massively cultivated as aquaculture feed.18–21
A recent study with laboratory cultures showed a high Sr removal from the culture medium by T. chui cells.22 This high Sr uptake was related to the intracellular formation of micropearls, which supports the idea of considering micropearl-forming microalgae as potential bioremediation agents targeting 90Sr contamination. However, there is a lack of evidence proving the suitability of T. chui and other micropearl-forming species for the development of new bioremediation techniques for the removal of 90Sr. In particular, the ability of these microorganisms to bioaccumulate this radionuclide and to tolerate ionizing radiation has never been studied and is therefore the subject of this study.
The experiments presented here were conducted with laboratory cultures of T. chui amended with different 90Sr concentrations ranging from 3.1 × 10−12 to 2.7 × 10−10 M (=1.4 to 124.2 Bq mL−1). These values fell within the higher range of 90Sr concentrations measured in the past in aquatic environments after major nuclear incidents.6,23–26 The assessment of the 90Sr uptake capacity of T. chui cells was performed by following the time variation of the radioactivity of the culture medium by liquid scintillation counting (LSC).
Material and methods
Strains and culture conditions
The strains Tetraselmis chui (SAG 8-6) and Tetraselmis marina (202.80) were obtained from the Algal Culture Collection of the University of Göttingen, Germany. The algal growth medium27,28 was prepared with sterilized (autoclaved) seawater from the Adriatic Sea (Lido di Jesolo, northeastern Italy). Salinity was adjusted to 20‰ before adding the algal nutrient solution (1000× concentrated). The natural Sr and Ca concentrations measured by Inductively Coupled Plasma Mass Spectrometry (ICP-MS) in the culture medium were 9 × 10−5 and 1.1 × 10−2 M, respectively.
T. chui was inoculated at a cell density of 2 × 105 cells per mL−1 in 250 mL Erlenmeyer flasks containing 100 mL of algal medium amended with different concentrations of 90SrCl2: 3.1 × 10−12, 6.2 × 10−11, and 2.7 × 10−10 M (1.4, 28.2, and 124.2 Bq mL−1, respectively). These culture conditions were selected on the basis of the highest 90Sr concentrations measured in the past in various aquatic environments contaminated with this radionuclide (Table 1). Cultures were established in triplicate and placed for 14-15 days at room temperature on an orbital shaker at 110 rpm and continuous light at 1500 lux intensity. Three types of controls were included in the experimental design based on a similar study performed with cyanobacteria.29 For all 90Sr concentrations tested, non-inoculated culture media were used as abiotic controls. As an inactivated control, T. chui cells pre-killed by autoclaving were resuspended at a cell concentration of 2 × 105 cells per mL in culture media containing 3.1 × 10−12 and 2.7 × 10−10 M 90Sr. Finally, T. marina (SAG 202.80) cultures with an initial cell concentration of 1 × 105 cells per mL, amended with 3.1 × 10−12 M 90Sr, were used as biotic controls as this species does not form micropearls and, therefore, is not supposed to bioaccumulate high concentrations of Sr. The controls were placed in the culture conditions cited above for T. chui cultures (i.e., placed for 14–15 days at room temperature on an orbital shaker at 110 rpm and continuous light at 1500 lux intensity).
Table 1 Strontium-90 concentrations measured in aquatic environments after major nuclear incidents
Event |
Year of the event |
Measured 90Sr |
Contaminated body of water or groundwater |
Year of measurement |
Reference |
(M) |
(Bq mL−1) |
Nuclear waste discharge in Techa River (Russia) |
1950–1958 |
6 × 10−11 |
27 |
Techa River |
1951 |
23
|
Nuclear waste discharge Lake Karachay and Kishthym disaster (Russia) |
1950–1958 |
2 × 10−11 |
8.8 |
Aquifer in the vicinity of Lake Karachay |
1994 |
6
|
1.6 × 10−8 |
7 × 104 |
Lake Karachay |
1993 |
6
|
Chernobyl disaster (Ukraine) |
1986 |
6.7 × 10−15 to 2.2 × 10−13 |
3 × 10−3 to 0.1 |
Borschi wetlands |
2000 |
24
|
Fukushima Daiichi disaster (Japan) |
2011 |
2.2 × 10−16 to 2.2 × 10−12 |
1 × 10−4 to 1 |
Pacific Ocean near Fukushima Power Plant |
2011–2012 |
25
|
26
|
Sampling
Two-mL samples were collected from the cultures and controls every two to four days. One mL was used to follow the growth of the algal cultures, while the remaining volume (1 mL) was used to measure the radioactivity of the culture media and, in some cases, for Scanning Electron Microscopy (SEM) observations.
Monitoring of algal growth
The cell concentration of the cultures over time was estimated by measuring their optical density at 750 nm (OD750) (Thermo Scientific Genesys 30 spectrophotometer). Prior to the measurements, a correlation between OD750 and cell density of the T. chui cultures was established by performing cell counting in a Neubauer chamber (ESI Fig. S1†).
The cell concentration (cells per mL) was calculated as:
| Cell concentration = OD750 × 3.21 × 106 | (1) |
The biomass dry weight (DW) was estimated from OD750 after obtaining the corresponding correlation. DW (g L−1) was calculated as:
Monitoring of strontium-90 uptake by T. chui
Strontium-90 uptake by T. chui was followed by measuring the radioactivity of the culture medium over time in T. chui cultures using liquid scintillation counting (LSC).
Sample preparation.
One-mL samples of T. chui cultures were centrifuged in 1.5 mL Eppendorf tubes at 5000 rpm for 5 min. This centrifugation procedure was effective in obtaining well-defined cell pellets and clear supernatants, indicating a successful separation of the cells from the culture medium. Five hundred μL of the supernatants were accurately weighed using a high precision scale (AX504, Mettler Toledo) and mixed with 15 mL of scintillating solution (Ultima Gold AB, PerkinElmer) in 20 mL liquid scintillation vials. The same procedure was followed for the control cultures.
Samples from cultures amended with 3.1 × 10−12 M 90Sr (1.4 Bq mL−1) were measured for two hours using LSC (Packard 2900TR), while cultures amended with 6.2 × 10−11 and 2.7 × 10−10 M 90Sr (28.2, and 124.2 Bq mL−1, respectively) were measured for one hour. Controls were measured in the same way.
Strontium-90 and yttrium-90 detection efficiencies calibration.
The efficiency of 3H detection using LSC as a function of the spectral index of external standard (tSIE) was already known. However, the detection efficiencies of 90Sr and 90Y as a function of tSIE had to be calculated. For this purpose, the freeware program CN2003 (ref. 30) was used to simulate the detection efficiencies of 90Sr and 90Y as a function of 3H efficiency. These results, together with the tSIE as a function of 3H efficiency, were then used to calculate the LSC detection efficiencies of 90Sr and 90Y as a function of tSIE and to apply a fourth-degree polynomial fit for each element.
Estimation of strontium-90 and yttrium-90 activities in the growth medium.
As mentioned above, 90Sr decays by β− radiation into 90Y, which in turn undergoes β− decay into stable 90Zr. Thus, the total activity in the culture media was the result of both 90Sr and 90Y activities. The total photon count obtained from the culture media using LSC was therefore the sum of the 90Sr and 90Y activities multiplied by their respective detection efficiencies as expressed in eqn (3). |  | (3) |
where cpstot(t) is the total number of photon counts per second obtained by liquid scintillation counting at time t,
and
(Bq mL−1) are the activities at time t of 90Sr and 90Y, respectively, and
and
their respective detection efficiencies.
Based on calculations using the nuclear science portal NUCLEONICA,31 we considered that the secular equilibrium between 90Sr and 90Y was reached at the start of the experiments (t = 0) in the cultures, as more than 17 days elapsed between the production of 90Sr and the start of the experiments (ESI Fig. S2†). Under this equilibrium condition:
|  | (4) |
In turn, the activity of a radio element is known to be expressed as
eqn (5).
| 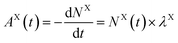 | (5) |
where
AX(
t) (Bq mL
−1) is the activity of the radioisotope X at time
t,
NX(
t) the number of atoms (atom per mL) of this radionuclide at time
t (s), and
λX (s
−1) its decay constant. Therefore, combination of
eqn (3)–(5) allowed to obtain the initial number of
90Sr atoms (

).
The Bateman equations account for the number of atoms of a parent and daughter (in this case, 90Sr and 90Y) as defined in:
| 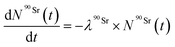 | (6) |
|  | (7) |
Here, we assumed that (i) only
90Sr is taken up by the microalgae and (ii)
90Y produced inside the cells is not released into the culture medium (to date, no transmembrane transport of Y has been documented in the green microalgae class Chlorodendrophyceae). Hence, the decrease in radioactivity observed in the culture medium is mainly due to
90Sr removal by the microalgae, which occurs at a much higher rate than the natural decay rate of this radioelement. Therefore,
eqn (6) was adapted to calculate the number of
90Sr atoms (

) as a function of time as follows:
| 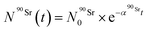 | (8) |
where
α (s
−1) is the reduction coefficient of
90Sr, mainly due to the microalgal uptake of this radioisotope and, to a lesser extent, to the natural decay of
90Sr.
By solving the differential eqn (7), the number of 90Y atoms (
) was calculated as a function of time as:
|  | (9) |
After obtaining
and
values, activities for each element were calculated by applying eqn (5).
Data correction due to evaporation.
The effect of evaporation on the total activity of the culture medium was calculated by measuring the total activity of non-inoculated controls over time. For all 90Sr concentrations tested, the increase in radioactivity due to evaporation of the culture medium was calculated and subtracted from the data set.
Strontium-90 uptake rate estimation.
Strontium-90 uptake rate (UR) was calculated using a formula applied in previous studies:22,32 | 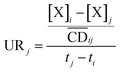 | (10) |
where t is time (h), i and j are two successive measuring times,
is the average cell density from time i to j, and [X] the concentration of the element X (fmol mL−1).
Scanning electron microscopy (SEM) observation and energy-dispersive X-ray spectroscopy (EDXS)
Samples for SEM observation were prepared by gently filtering 20 μL of T. chui cultures with polycarbonate membranes (Whatman® Nuclepore™) of 1 μm pore size. The membranes were dried at room temperature and placed on aluminum (Al) stubs using a double-sided conductive carbon tape. They were then coated with a 10 nm gold (Au) layer by sputter coating under low vacuum. Imaging and EDXS measurements were performed with a JEOL JSM 7001F Scanning Electron Microscope equipped with an EDXS detector (EX-943000S4L1Q; JEOL). Images were obtained using backscattered electrons. EDXS measurements of micropearls were performed with an accelerating voltage of 15 kV, a beam current of 7 nA, and an acquisition time of 30 s.
Results and discussion
Strontium-90 removal and its effect on T. chui cell growth
To study the 90Sr uptake by T. chui cells, we measured the radioactivity over time of the growth medium of T. chui cultures and controls amended with 3.1 × 10−12, 6.2 × 10−11, and 2.7 × 10−10 M 90Sr (1.4, 28.2, and 124.2 Bq mL−1, respectively) (Fig. 1A–C). We also followed the growth of the cultures to determine the influence of cell density on 90Sr removal and, reciprocally, to understand the impact of radioactivity on cultures growth (Fig. 1D–F).
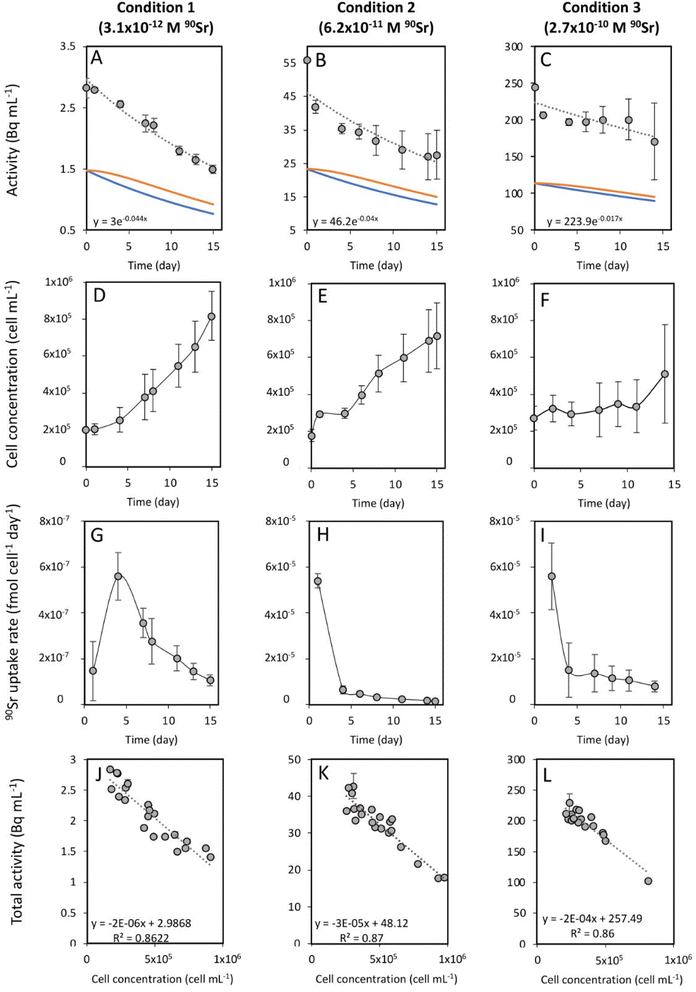 |
| Fig. 1 (A–C) Time evolution of the activity measured in the growth medium of T. chui cultures amended with (A) 3.1 × 10−12, (B) 6.2 × 10−11, and (C) 2.7 × 10−10 M 90Sr. Grey circles correspond to the total activity of the culture medium. The dashed line represents the calculated exponential reduction fit of the total activity. The corresponding equation appears at the bottom left of each graph. The orange line corresponds to the estimated 90Y activity and the blue line to the estimated 90Sr activity. (D–F) Time evolution of the cell concentration of T. chui cultures amended with (D) 3.1 × 10−12, (E) 6.2 × 10−11, and (F) 2.7 × 10−10 M 90Sr. (G–I) Variation over time of the 90Sr uptake rate by T. chui in cultures amended with (G) 3.1 × 10−12, (H) 6.2 × 10−11, and (I) 2.7 × 10−10 M 90Sr. (J–L) Total activities measured in the growth medium of T. chui cultures as a function of their cell concentration (values of the three culture replicates are represented). Cultures were amended with (J) 3.1 × 10−12, (K) 6.2 × 10−11, and (L) 2.7 × 10−10 M 90Sr. The dashed line corresponds to the estimated linear fit with its respective equation represented in the bottom left area of each graph. The error bars represent the standard deviations (n = 3). | |
As mentioned above, the effect of evaporation (i.e., the increase in radioactivity over time) was corrected for non-inoculated controls. While radioactivity decreased over time in T. chui cultures in all three growing conditions (Fig. 1A–C), it remained stable in T. marina and T. chui dead cell cultures (Fig. 2A, 3 and ESI Fig. S4†).
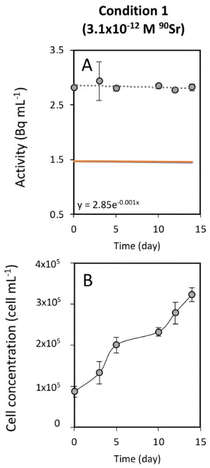 |
| Fig. 2 (A) Time evolution of the activity measured in the growth medium of T. marina cultures amended with 3.1 × 10−12 M 90Sr. Grey circles correspond to the total activity of the culture medium. The dashed line represents the calculated exponential reduction fit of the total activity. Its associated equation is expressed on the bottom left area of the graph. The orange line corresponds to the estimated 90Y activity and the blue line to the estimated 90Sr activity. In this case, both lines are superposed. (B) Time evolution of the cell concentration of T. marina cultures amended with 3.1 × 10−12 M 90Sr. The error bars represent the standard deviations (n = 3). | |
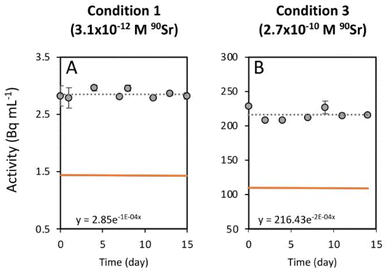 |
| Fig. 3 Time evolution of the activity measured in a growth medium where dead T. chui cells were resuspended. The growth medium was amended with (A) 3.1 × 10−12 M and (B) 2.7 × 10−10 M 90Sr. Grey circles correspond to the total activity of the culture medium. The dashed line represents the calculated exponential reduction fit of the total activity. The corresponding equation is shown on the bottom left area of each graph. The orange line corresponds to the estimated 90Y activity and the blue line to the estimated 90Sr activity. In both cases lines are superposed. The error bars represent the standard deviations (n = 3). | |
The total activity measured in T. chui cultures amended with 3.1 × 10−12 M 90Sr (1.4 Bq mL−1) decreased by half after 15 days of growth (from 2.8 to 1.5 ± 0.7 Bq mL−1), reaching a final estimated 90Sr concentration of 1.7 × 10−12 M 90Sr (0.77 Bq mL−1) (Fig. 1A). Cell density was 8.2 × 105 ± 1.3 × 105 cell per mL after 15 days of growth (Fig. 1D) and variation between the three replicates was minimal, showing comparable 90Sr removal and culture growth dynamics (ESI Fig. S3†). The 90Sr removal rate was estimated to be maximal the fourth day of growth, with a calculated value of 5.6 × 10−7 ± 1 × 10−7 fmol per cell per day (Fig. 1G). Thereafter, the rate of 90Sr removal progressively decreased on the following days. The average concentration of 90Sr in the dry biomass was 5.5 × 10−2 ± 3.3 × 10−3 ng g−1.
The total radioactivity of T. chui cultures with an initial 90Sr concentration of 6.2 × 10−11 M (28.2 Bq mL−1) also decreased by 50% after 15 days of growth (from 56 to 27.6 ± 7.3 Bq mL−1), showing a final estimated 90Sr concentration of 2.8 × 10−11 M (12.8 Bq L−1) (Fig. 1B). Although the cell concentration after 15 days of growth (7.2 × 105 ± 1.8 × 105 cells per mL) was not significantly lower than in the previous case (Fig. 1E), the variation between replicates was higher than in the previously tested condition. Both cell growth and 90Sr removal were significantly higher in replicate 3 compared to replicates 1 and 2 (ESI Fig. S5†), but 90Sr removal rates were comparable between replicates. The highest 90Sr removal rate was estimated at 5.4 × 10−5 ± 6.5 × 10−6 fmol per cell per day after one day of growth, and also decreased with time (Fig. 1H). The mean concentration of 90Sr in T. chui cells was 1.8 ± 0.3 ng per (g dry biomass).
Finally, the total activity measured in T. chui cultures amended with 2.7 × 10−10 M 90Sr (124.2 Bq mL−1) decreased by 30% after 14 days of growth, from 244.8 to 170.8 ± 51.2 Bq mL−1 (Fig. 1C). The final 90Sr concentration was estimated at 1.95 × 10−10 M (89.6 Bq mL−1). In this case, the variation between replicates was the highest observed, especially from the eighth day of growth onwards. After 14 days of growth, the cell density was 5.1 × 105 ± 2.7 × 105 cell per mL (Fig. 1F). Although culture growth was lower than that observed in the two previous cases, this difference was not significant due to the large variation between replicates. In fact, replicate 3 had a much higher growth and 90Sr removal rate than replicates 1 and 2 (ESI Fig. S6†). The highest calculated 90Sr removal rate was 5.6 × 10−5 ± 1.5 × 10−5 fmol per cell per day and was observed during the first two days of growth (Fig. 1I). The average concentration of 90Sr in the dry biomass was 6 ± 1.5 ng g−1.
For all 90Sr concentrations tested, the Pearson correlation coefficient showed a strong negative correlation (p < 0.0001) between T. chui cell density and total radioactivity of the cultures, confirming that 90Sr removal is directly dependent on T. chui cell concentration in the cultures (Fig. 1J–L). T. chui cultures amended with 2.7 × 10−10 M 90Sr showed the most pronounced decrease in radioactivity as a function of cell density. In fact, the results show that the overall coefficients of radioactivity reduction are proportional to the initial concentration of 90Sr in the cultures (ESI Fig. S7†). Therefore, higher concentrations of 90Sr in the culture medium result in higher absorption rates by T. chui cells and higher estimated concentrations of 90Sr in their dry biomass. Further research is needed to determine the maximum 90Sr uptake capacity of T. chui.
Strontium-90 accumulation mechanism in T. chui
Previous studies showed that the uptake of stable Sr by T. chui was related to the formation of micropearls, incorporating this alkaline earth metal into these mineral inclusions.22 In this study, we show that T. chui also sequesters radioactive 90Sr from a seawater culture medium. Furthermore, we observe that, unlike living T. chui cells, T. marina (a non-micropearl forming species) and dead T. chui cells do not sequester 90Sr. This finding indicates that, as expected, the removal of 90Sr by T. chui must be due to its active uptake for micropearl formation. This suggests that other micropearl-forming species within the class Chlorodendrophyceae may also sequester 90Sr.
Effect of radioactivity in T. chui cell growth variability
The greater variation in growth observed between culture replicates with higher 90Sr concentrations, and especially with the highest concentration tested 90Sr (2.7 × 10−10 M = 124.2 Bq mL−1), could indicate that, at these concentrations, β− radiation can alter the viability of microalgal cells. It is known that 90Sr and 90Y can affect plants, triggering growth abnormalities, as well as decreasing grain yield and seed viability33,34 but, unfortunately, the effect of radiation on microalgae has hardly been studied in the past. A study in which the green microalga Chlorella vulgaris was cultured with 200 and 2000 Bq mL−1 90Sr showed that its growth was not altered at these concentrations.35 However, in that study, most of the 90Sr did not penetrate the cells but was deposited on the cell wall, forming carbonates. In a study following the uptake of 90Sr by the cyanobacterium Gloeomargarita lithophora (which forms similar Sr-rich intracellular ACC inclusions), a possible toxic effect on the growth of cultures with 8.8 × 10−11 M (39.7 Bq mL−1) 90Sr was alluded to.29 It is conceivable that intracellular accumulation of 90Sr in micropearls could cause cell damage due to the delivery of higher doses of β− radiation into the cells. It is important to note, however, that the lowest concentrations of 90Sr tested in this study are those that best correspond to actual contamination levels in aquatic environments (except in cases of extreme contamination such as that observed in Lake Karachay, Russia) (Table 1). Overall, the fact that some replicate cultures of T. chui grew even with high concentrations of 90Sr in the medium is a promising result, as it demonstrates that this species can accommodate high levels of ionising radiation.
Finally, the greater variability between replicates observed in this study compared to a previous study with non-radioactive Sr (reference) may not be entirely due to radioactivity but to suboptimal culture conditions. Indeed, the use of a radioactive isotope made it necessary to work in a laboratory adapted to the handling of radioactive materials but where conditions were not optimal for microalgae cultivation.
Effect of radioactivity in T. chui cell morphology and Sr accumulation
To prevent instrument contamination, we only analysed cell samples obtained from cultures with the lowest 90Sr concentration tested in this study (i.e., 3.1 × 10−12 M 90Sr). T. chui cells grown for four days in cultures amended with this 90Sr concentration were observed using SEM in order to detect any possible abnormality in cells morphology and in the process of micropearl formation due to β− radiation (Fig. 4). At this 90Sr concentration, the cells did not show any alteration and micropearls were present. In fact, even several cells were observed in the dividing stage. The size and shape of the micropearls corresponded to that observed in previous studies.22 By performing EDXS analysis on the micropearls, we obtained their Sr/Ca mol% ratios (Fig. 4 and ESI Fig. S8†). The mean value was 0.32 ± 0.09 (n = 18, from 14 different cells). This value is comparable with the one obtained from T. chui cultures amended with stable Sr (0.42 ± 0.1),22 knowing that a strict comparison is precluded because of slightly different initial concentration in the culture media (9 × 10−5 M (stable) Sr and 1.1 × 10−2 M Ca here, and 1 × 10−4 M (stable) Sr, 2.5 × 10−3 M Ca in the previous study).
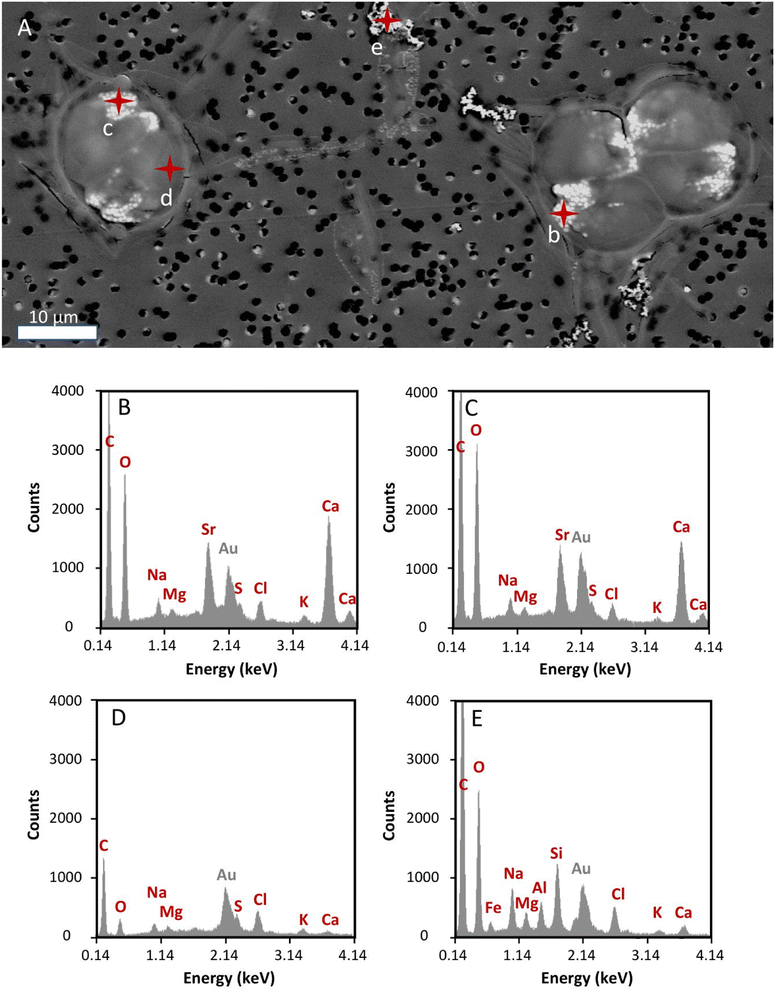 |
| Fig. 4 (A) SEM image of T. chui cells grown for four days in a culture medium amended with 3.1 × 10−12 M 90Sr. Three dividing cells are observed. Micropearls appear as bright white inclusions (<1 μm) in the anterior part of each daughter cell. Black dots correspond to the pores of the membrane used to prepare the sample. Red marks correspond to the EDXS analysis points. (B and C) EDXS spectra of T. chui micropearls (analysis points b and c, respectively). (D) EDXS spectrum of a cell area free of micropearls (analysis point d). (E) EDXS spectrum of a sea-salt particle present in the culture medium (analysis point e). | |
Is T. chui a plausible strontium-90 bioremediation agent?
At all 90Sr concentrations tested in this study, T. chui cultures reaching a cell density of approximately 1.5 × 106 cells per mL would be sufficient to remove more than 95% of the initially dissolved 90Sr (Fig. 1J and K). Since the present study demonstrates that 90Sr removal is directly related to the concentration of T. chui cells in the cultures and it has been shown that T. chui cell density can reach values above 3.2 × 106 cells per mL when culture conditions are optimized,36 one can expect to obtain higher 90Sr removal in the cultures if cell growth is improved.
The use of species within the class Chlorodendrophyceae for the development of new 90Sr decontamination techniques has many clear advantages compared to the use of other organisms suggested for this purpose in the past, such as plants,37,38 cyanobacteria,29,39 and bacteria,40,41 namely:
• The possibility of using organisms adapted to very diverse aquatic environments: Sr uptake by species of Chlorodendrophyceae is associated with their ability to form micropearls,22 this ability being shared by species living in very different habitats, including hypersaline water, seawater, brackish water and freshwater.17,20,42
• T. chui and other species such as Tetraselmis suecica also have high growth performances over a wide range of salinities and light intensities, which further increases their field of application.21,36,43,44
• These species can be highly resistant to bacteria and cyanobacteria contamination, which is an essential requirement for these organisms to be used for the treatment of non-sterile water.45–47
• An advantage of using microalgae over other types of organisms is their rapid growth and the low energy cost associated with their production, as natural sunlight can be used as the main source of energy. Microalgae of the genus Tetraselmis are already largely produced in mass culture systems for aquaculture purposes, as well as for biodiesel production, CO2 mitigation, and wastewater treatment.48–51 Currently, several outdoor infrastructures (photobioreactors) for large-scale production of Tetraselmis (including T. chui) already exist and could serve as a basis for the future development of ex-situ 90Sr decontamination systems -based on Tetraselmis.51,52
Conclusions
This study shows for the first time the ability of Tetraselmis chui to sequester radioactive 90Sr from a culture medium mainly composed of seawater, this capacity being related to the intracellular formation of micropearls. Strontium-90 removal depends on T. chui cell density in the cultures, as well as on the initial 90Sr concentrations in the medium. Although culture growth is not affected at 90Sr concentrations of 3.1 × 10−12 M (1.4 Bq L−1), we see a heterogeneous effect on T. chui cultures containing higher 90Sr concentrations that may be related to high levels of ionizing radiation.
Overall, our results clearly support the possibility of achieving complete 90Sr removal if T. chui growth is optimized. These results are highly relevant as T. chui is a robust species, easy to grow on a large scale and, therefore, particularly attractive for the development of new bioremediation techniques. Moreover, since 90Sr uptake is related to micropearl formation, it is likely that other micropearl-forming species could be used for the same purpose.
Author contributions
I. Segovia-Campos (conceptualization, data curation, formal analysis, investigation, methodology, resources, visualization, validation, writing – original draft, writing – review and editing); A. Kanellakopolulos (conceptualization, data curation, formal analysis, investigation, methodology, resources, visualization, validation); I. J. Barrozo (data curation, formal analysis, investigation, methodology, validation); E. Fock-Chin-Ming (data curation, formal analysis, investigation); M. Filella (conceptualization, funding acquisition, methodology, supervision, validation, writing – original draft, writing – review and editing); A. Baxarias Fontaine (conceptualization, data curation, formal analysis, investigation, methodology, resources); S. Pallada (methodology, resources, supervision), G. Triscone (methodology, resources, supervision), K. Perron (methodology, resources, supervision); D. Ariztegui (conceptualization, funding acquisition, supervision, validation, writing – original draft, writing – review and editing).
Conflicts of interest
There are no conflicts to declare.
Acknowledgements
This work has been entirely funded by the Gerbert Rüf Stiftung (Basel, Switzerland), Project Microbials GRS-071/17.
References
-
B. E. Sample and C. Irvine, in Environmental Contaminants in Biota, CRC Press, 2011, pp. 703–732 Search PubMed.
-
D. A. Atwood, Radionuclides in the Environment, John Wiley & Sons, 2013 Search PubMed.
- Q.-H. Hu, J.-Q. Weng and J.-S. Wang, Sources of anthropogenic radionuclides in the environment: a review, J. Environ. Radioact., 2010, 101, 426–437 CrossRef CAS PubMed.
- F. Hoffman, U. Bergström, C. Gyllander and A. Wilkens, Comparison of predictions from internationally recognized assessment models for the transfer of selected radionuclides through terrestrial food chains, Nucl. Saf., 1984, 25, 533–546 CAS.
-
P. Pathak and D. K. Gupta, Strontium Contamination in the Environment, Springer, Switzerland, 2020 Search PubMed.
- W. J. Standring, M. Dowdall and P. Strand, Overview of dose assessment developments and the health of riverside residents close to the “Mayak” PA facilities, Russia, Int. J. Environ. Res. Public Health, 2009, 6, 174–199 CrossRef CAS PubMed.
- A. V. Yablokov, V. B. Nesterenko and A. V. Nesterenko, Chapter III. Consequences of the Chernobyl catastrophe for the environment, Ann. N. Y. Acad. Sci., 2009, 1181, 221–286 CrossRef PubMed.
-
ATSDR, Toxicological Profile for Strontium, U.S. Department of Health Human Services, 2004 Search PubMed.
- K.-H. Kim, S.-O. Kim, C.-W. Lee, M.-H. Lee and K.-W. Kim, Electrokinetic processing for the removal of radionuclides in soils, Sep. Sci. Technol., 2003, 38, 2137–2163 CrossRef CAS.
-
ONR, Remediation Techniques For Radioactive Contaminated Land on Nuclear Licensed Sites. Office for Nuclear Regulation, 2020 Search PubMed.
- J. R. Lloyd and J. C. Renshaw, Bioremediation of radioactive waste: radionuclide–microbe interactions in laboratory and field-scale studies, Curr. Opin. Biotechnol., 2005, 16, 254–260 CrossRef CAS PubMed.
- G. M. Gadd, Metals, minerals and microbes: geomicrobiology and bioremediation, Microbiology, 2010, 156, 609–643 CrossRef CAS PubMed.
- C. Roh, C. Kang and J. R. Lloyd, Microbial bioremediation processes for radioactive waste, Korean J. Chem. Eng., 2015, 32, 1720–1726 CrossRef CAS.
- S.-y. Fukuda, K. Iwamoto, M. Atsumi, A. Yokoyama, T. Nakayama, K.-i. Ishida, I. Inouye and Y. Shiraiwa, Global searches for microalgae and aquatic plants that can eliminate radioactive cesium, iodine and strontium from the radio-polluted aquatic environment: a bioremediation strategy, J. Plant Res., 2014, 127, 79–89 CrossRef CAS PubMed.
- M. R. Krejci, L. Finney, S. Vogt and D. Joester, Selective Sequestration of Strontium in Desmid Green Algae by Biogenic Co-precipitation with Barite, ChemSusChem, 2011, 4, 470–473 CrossRef CAS PubMed.
- M. Liu, F. Dong, W. Kang, S. Sun, H. Wei, W. Zhang, X. Nie, Y. Guo, T. Huang and Y. Liu, Biosorption of strontium from simulated nuclear wastewater by Scenedesmus spinosus under culture conditions: adsorption and bioaccumulation processes and models, Int. J. Environ. Res. Public Health, 2014, 11, 6099–6118 CrossRef CAS PubMed.
- A. Martignier, M. Filella, K. Pollok, M. Melkonian, M. Bensimon, F. Barja, F. Langenhorst, J. M. Jaquet and D. Ariztegui, Marine and freshwater micropearls: Biomineralization producing strontium-rich amorphous calcium carbonate inclusions is widespread in the genus Tetraselmis (Chlorophyta), Biogeosci. Discuss., 2018, 2018, 1–22 Search PubMed.
- R. M. Gladue and J. E. Maxey, Microalgal feeds for aquaculture, J. Appl. Phycol., 1994, 6, 131–141 CrossRef.
- R. Robert, G. Parisi, L. Rodolfi, B. M. Poli and M. R. Tredici, Use of fresh and preserved Tetraselmis suecica for feeding Crassostrea gigas larvae, Aquaculture, 2001, 192, 333–346 CrossRef.
- A. Martignier, S. De Respinis, M. Filella, I. Segovia-Campos, B. Marin, G. Günther, F. Barja, M. Tonolla, J.-M. Jaquet, M. Melkonian and D. Ariztegui, Biomineralization Capacities of Chlorodendrophyceae: Correlation Between Chloroplast Morphology and the Distribution of Micropearls in the Cell, Protist, 2020, 125760 CrossRef CAS PubMed.
- S. L. Meseck, J. H. Alix and G. H. Wikfors, Photoperiod and light intensity effects on growth and utilization of nutrients by the aquaculture feed microalga, Tetraselmis chui (PLY429), Aquaculture, 2005, 246, 393–404 CrossRef.
- I. Segovia-Campos, M. Filella, K. Perron and D. Ariztegui, High calcium and strontium uptake by the green microalga Tetraselmis chui is related to micropearl formation and cell growth, Environ. Microbiol. Rep., 2023, 15, 38–50 CrossRef CAS PubMed.
- I. Kryshev, G. Romanov, V. Chumichev, T. Sazykina, L. Isaeva and M. Ivanitskaya, Radioecological consequences of radioactive discharges into the Techa River on the Southern Urals, J. Environ. Radioact., 1998, 38, 195–209 CrossRef CAS.
- R. Freed, L. Smith and D. Bugai, The effective source area of 90Sr for a stream near Chernobyl, Ukraine, J. Contam. Hydrol., 2004, 71, 1–26 CrossRef CAS PubMed.
- J. A. Kenyon, K. O. Buesseler, N. Casacuberta, M. Castrillejo, S. Otosaka, P. Masqué, J. A. Drysdale, S. M. Pike and V. Sanial, Distribution and Evolution of Fukushima Dai-ichi derived 137Cs, 90Sr, and 129I in Surface Seawater off the Coast of Japan, Environ. Sci. Technol., 2020, 54, 15066–15075 CrossRef CAS PubMed.
- M. Castrillejo, N. Casacuberta, C. F. Breier, S. M. Pike, P. Masqué and K. O. Buesseler, Reassessment of 90Sr, 137Cs, and 134Cs in the coast off Japan derived from the Fukushima Dai-ichi nuclear accident, Environ. Sci. Technol., 2016, 50, 173–180 CrossRef CAS PubMed.
- J. Fabregas, J. Abalde, C. Herrero, B. Cabezas and M. Veiga, Growth of the marine microalga Tetraselmis suecica in batch cultures with different salinities and nutrient concentrations, Aquaculture, 1984, 42, 207–215 CrossRef.
- H. Pereira, K. N. Gangadhar, P. S. Schulze, T. Santos, C. B. de Sousa, L. M. Schueler, L. Custódio, F. X. Malcata, L. Gouveia and J. Varela, Isolation of a euryhaline microalgal strain, Tetraselmis sp. CTP4, as a robust feedstock for biodiesel production, Sci. Rep., 2016, 6, 1–11 CrossRef PubMed.
- N. Mehta, K. Benzerara, B. D. Kocar and V. Chapon, Sequestration of radionuclides radium-226 and strontium-90 by cyanobacteria forming intracellular calcium carbonates, Environ. Sci. Technol., 2019, 53, 12639–12647 CrossRef CAS PubMed.
-
E. Gunther, Program CN2003: A Program to Calculate the LC Efficiency of a Nuclide vs. Efficiency the Tracer H-3, PTB, Germany, 2003 Search PubMed.
- J. Magill and R. Dreher, The NUCLEONICA nuclear science portal, AIP Conf. Proc., 2009, 1164, 100–106 CrossRef.
- N. Cam, K. Benzerara, T. Georgelin, M. Jaber, J.-F. Lambert, M. Poinsot, F. Skouri-Panet and L. Cordier, Selective uptake of alkaline earth metals by cyanobacteria forming intracellular carbonates, Environ. Sci. Technol., 2016, 50, 11654–11662 CrossRef CAS PubMed.
- R. Schulz and N. Baldar, Effects of beta radiation on wheat, peas, and lettuce exposed by foliar contamination with water-soluble yttrium-90 (1), Radiat. Bot., 1972, 12, 77–85 CrossRef CAS.
- A. Van Hoeck, N. Horemans, M. Van Hees, R. Nauts, D. Knapen, H. Vandenhove and R. Blust, β-Radiation stress responses on growth and antioxidative defense system in plants: a study with strontium-90 in Lemna minor, Int. J. Mol. Sci., 2015, 16, 15309–15327 CrossRef CAS PubMed.
- S. Y. Lee, K.-H. Jung, J. E. Lee, K. A. Lee, S.-H. Lee, J. Y. Lee, J. K. Lee, J. T. Jeong and S.-Y. Lee, Photosynthetic biomineralization of radioactive
Sr via microalgal CO2 absorption, Bioresour. Technol., 2014, 172, 449–452 CrossRef CAS PubMed.
- M. Mohammadi, N. Kazeroni and M. J. Baboli, Fatty acid composition of the marine micro alga Tetraselmis chuii Butcher in response to culture conditions, J. Algal Biomass Utln., 2015, 6, 49–55 Search PubMed.
- S. Eapen, S. Singh, V. Thorat, C. Kaushik, K. Raj and S. D'Souza, Phytoremediation of radiostrontium (90Sr) and radiocesium (137Cs) using giant milky weed (Calotropis gigantea R. Br.) plants, Chemosphere, 2006, 65, 2071–2073 CrossRef CAS PubMed.
- S. Singh, S. Eapen, V. Thorat, C. Kaushik, K. Raj and S. D’souza, Phytoremediation of 137cesium and 90strontium from solutions and low-level nuclear waste by Vetiveria zizanoides, Ecotoxicol. Environ. Saf., 2008, 69, 306–311 CrossRef CAS PubMed.
- P. Pohl and W. Schimmack, Adsorption of radionuclides (134Cs, 85Sr, 226Ra, 241Am) by extracted biomasses of cyanobacteria (Nostoc Carneum, N. Insulare, Oscillatoria Geminata and Spirulina Laxis-Sima) and phaeophyceae (Laminaria Digitata and L. Japonica; waste products from alginate production) at different pH, J. Appl. Phycol., 2006, 18, 135–143 CrossRef CAS.
- V. Achal, X. Pan and D. Zhang, Bioremediation of strontium (Sr) contaminated aquifer quartz sand based on carbonate precipitation induced by Sr resistant Halomonas sp, Chemosphere, 2012, 89, 764–768 CrossRef CAS PubMed.
- C.-H. Kang, J.-H. Choi, J. Noh, D. Y. Kwak, S.-H. Han and J.-S. So, Microbially induced calcite precipitation-based sequestration of strontium by Sporosarcina pasteurii WJ-2, Appl. Biochem. Biotechnol., 2014, 174, 2482–2491 CrossRef CAS PubMed.
- I. Segovia-Campos, A. Martignier, M. Filella, J. M. Jaquet and D. Ariztegui, Micropearls and other intracellular inclusions of amorphous calcium carbonate: an unsuspected biomineralization capacity shared by diverse microorganisms, Environ. Microbiol., 2022, 24, 537–550 CrossRef CAS PubMed.
- T. Ishika, P. A. Bahri, D. W. Laird and N. R. Moheimani, The effect of gradual increase in salinity on the biomass productivity and biochemical composition of several marine, halotolerant, and halophilic microalgae, J. Appl. Phycol., 2018, 30, 1453–1464 CrossRef CAS.
- M. Trovão, H. Pereira, J. Silva, J. Páramo, P. Quelhas, T. Santos, J. T. Silva, A. Machado, L. Gouveia and L. Barreira, Growth performance, biochemical composition and sedimentation velocity of Tetraselmis sp. CTP4 under different salinities using low-cost lab-and pilot-scale systems, Heliyon, 2019, 5, e01553 CrossRef PubMed.
- S. L. Meseck, Controlling the growth of a cyanobacterial contaminant, Synechoccus sp., in a culture of Tetraselmis chui (PLY429) by varying pH: Implications for outdoor aquaculture production, Aquaculture, 2007, 273, 566–572 CrossRef CAS.
- N. Biondi, G. Cheloni, E. Tatti, F. Decorosi, L. Rodolfi, L. Giovannetti, C. Viti and M. R. Tredici, The bacterial community associated with Tetraselmis suecica outdoor mass cultures, J. Appl. Phycol., 2017, 29, 67–78 CrossRef CAS.
- P. S. Schulze, C. F. Carvalho, H. Pereira, K. N. Gangadhar, L. M. Schüler, T. F. Santos, J. C. Varela and L. Barreira, Urban wastewater treatment by Tetraselmis sp. CTP4 (Chlorophyta), Bioresour. Technol., 2017, 223, 175–183 CrossRef CAS PubMed.
- M. Pérez-Rama, J. A. Alonso, C. H. López and E. T. Vaamonde, Cadmium removal by living cells of the marine microalga Tetraselmis suecica, Bioresour. Technol., 2002, 84, 265–270 CrossRef PubMed.
- P. Bondioli, L. Della Bella, G. Rivolta, G. C. Zittelli, N. Bassi, L. Rodolfi, D. Casini, M. Prussi, D. Chiaramonti and M. R. Tredici, Oil production by the marine microalgae Nannochloropsis sp. F&M-M24 and Tetraselmis suecica F&M-M33, Bioresour. Technol., 2012, 114, 567–572 CrossRef CAS PubMed.
- N. R. Moheimani,
Tetraselmis suecica culture for CO2 bioremediation of
untreated flue gas from coal-fired power station, J. Appl. Phycol., 2016, 28(4), 2139–2146 CrossRef CAS.
- H. Pereira, J. Páramo, J. Silva, A. Marques, A. Barros, D. Maurício, T. Santos, P. Schulze, R. Barros and L. Gouveia, Scale-up and large-scale production of Tetraselmis sp. CTP4 (Chlorophyta) for CO2 mitigation: From an agar plate to 100-m3 industrial photobioreactors, Sci. Rep., 2018, 8, 1–11 CAS.
- W.-K. Lee, Y.-K. Ryu, W.-Y. Choi, T. Kim, A. Park, Y.-J. Lee, Y. Jeong, C.-G. Lee and D.-H. Kang, Year-Round Cultivation of Tetraselmis sp. for Essential Lipid Production in a Semi-Open Raceway System, Mar. Drugs, 2021, 19, 314 CrossRef CAS PubMed.
|
This journal is © The Royal Society of Chemistry 2024 |