DOI:
10.1039/D3RA00184A
(Review Article)
RSC Adv., 2023,
13, 7798-7817
Success stories of natural product-derived compounds from plants as multidrug resistance modulators in microorganisms
Received
10th January 2023
, Accepted 1st March 2023
First published on 8th March 2023
Abstract
Microorganisms evolve resistance to antibiotics as a function of evolution. Antibiotics have accelerated bacterial resistance through mutations and acquired resistance through a combination of factors. In some cases, multiple antibiotic-resistant determinants are encoded in these genes, immediately making the recipient organism a “superbug”. Current antimicrobials are no longer effective against infections caused by pathogens that have developed antimicrobial resistance (AMR), and the problem has become a crisis. Microorganisms that acquire resistance to chemotherapy (multidrug resistance) are a major obstacle for successful treatments. Pharmaceutical industries should be highly interested in natural product-derived compounds, as they offer new sources of chemical entities for the development of new drugs. Phytochemical research and recent experimental advances are discussed in this review in relation to the antimicrobial efficacy of selected natural product-derived compounds as well as details of synergistic mechanisms and structures. The present review recognizesand amplifies the importance of compounds with natural origins, which can be used to create safer and more effective antimicrobial drugs by combating microorganisms that are resistant to multiple types of drugs.
1. Introduction
Multidrug-resistant microorganisms (MDRMOs) are progressively being regarded as a major global health challenge.1,2 As a result of conventional antibiotic pressure, bacterial efflux pumps were increased, reducing drug concentrations; enzymes were induced to modify and inactivate antibiotic compounds; or the drug target site was elevated, reducing antibiotic potency (Fig. 1).3,4
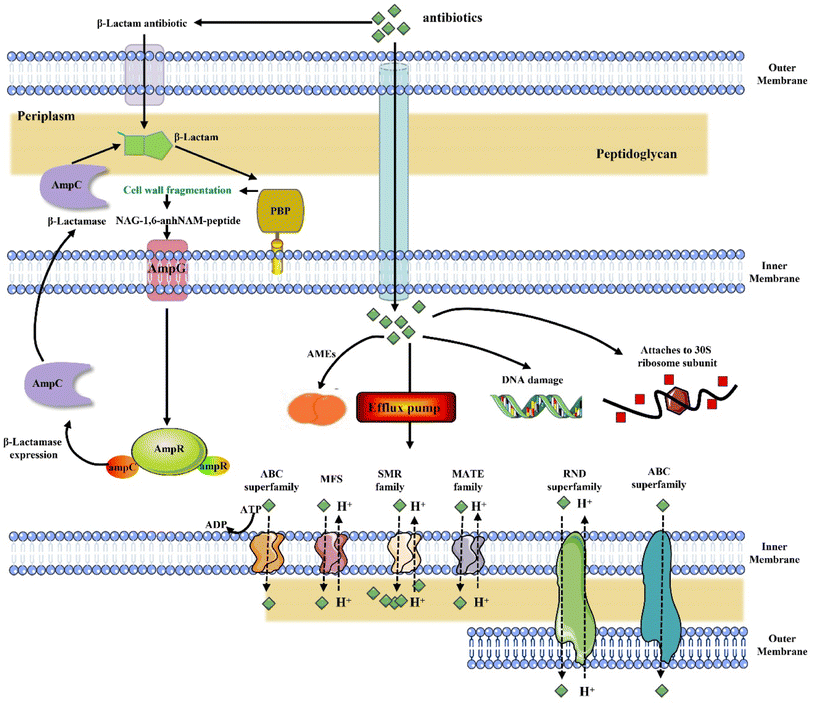 |
| Fig. 1 Mechanism of development of multidrug resistance. AMEs: modifying aminoglycoside modifying enzymes; ABC: ATP-binding cassette; MFS: major facilitator superfamily; MATE: multidrug and toxin extrusion; SMR: small multidrug resistance; RND: resistance-nodulation-cell division; PBP: penicillin-binding protein. | |
Infections by pathogenic bacteria, especially those that are multidrug resistant (MDR), are leading to a growing global health crisis. As a result, MDR bacteria can spread rapidly in hospitals and in the community, posing a staggering burden on health care systems both economically and epidemiologically.5 In addition, the inability of current clinical technologies to accurately diagnose infections in a timely manner further aggravates the resistance situation.6 By 2050, the number of antimicrobial resistance-related deaths could amount to 10 million, which is far more than the number of cancer deaths.2 As we approach the postantibiotic era, normal infections and minor injuries will no longer be treatable. However, in contrast to the rapidly spreading threat of antibiotic resistance, recent decades have seen a dramatic decline in new antibiotic development, with many pharmaceutical companies abandoning antibiotic discovery programs. Due to the subsiding development of antibiotics, alternative therapeutics are needed to treat MDR bacteria, and antimicrobial stewardship necessitates urgent action to stop the evolution of resistance and prevent the emergence of new resistance.
Drug development is aided by natural compounds, especially in regard to the discovery of antimicrobial drugs.7–9 Microorganisms have become increasingly resistant and the use of antibiotics is causing numerous side effects, making it essential to develop new and improved antibacterial agents using plant-based derivatives.10,11 The purpose of this review is to discuss compounds of natural products and derivatives derived from plants that have been investigated as modulators of MDR by inhibiting or otherwise stopping their activity.
2. Molecular antibiotic resistance mechanisms
By overusing antibiotics, microbes have evolved resistance to many conventional antimicrobials. There are several mechanisms that can lead to drug resistance in microbes, including inactivation or modification of antimicrobial drugs, alteration of drug target sites and expulsion of antimicrobial drugs across the cell membrane reduces drug accumulation. The combination of all of these factors contributes to the development of resistance to current antimicrobial therapy.
2.1. Alterers: inactivated antimicrobial drugs
2.1.1 β-Lactamases. The most widely used antimicrobial agents are β-lactams. Additionally, Gram-negative bacteria produce β-lactamases to resist β-lactams. To overcome β-lactamase-mediated resistance, a valuable alternative is to develop new β-lactams that are completely stable to the prevalent β-lactamases; however, this goal is likely impossible.12,13 To protect β-lactam antibiotics from the activity of β-lactamases, combinations of β-lactams with inhibitors of β-lactamases have been used. Through this strategy, three very successful combination drugs have been produced, namely, ampicillin-sulbactam, amoxicillin-clavulanate, and piperacillin-tazobactam. These drugs are still among the most commonly prescribed antibiotics in clinical settings after two decades of use.14,15 There are several advantages to using combinations of β-lactam and β-lactamase inhibitors compared to expanded-spectrum cephalosporins, and these drugs are less likely to result in resistance.Current therapeutic β-lactamase inhibitors are only effective against class A β-lactamases and do not protect against other β-lactamases. A further challenge for β-lactams and β-lactamase inhibitors is that some strains produce multiple enzymes. Despite reports that new β-lactamase inhibitors are appearing increasingly frequently in the medical literature, some of these inhibitors demonstrate good inhibition properties, as well as inhibiting a variety of β-lactamases simultaneously; other than AVE1330 and NXL104, none of these inhibitors have even reached phase I trials.16,17 Molecular modeling and resolution of new lactamase structures may help to overcome one of the most serious threats to human health.
2.1.2 Aminoglycoside modifying enzymes (AMEs). For decades, aminoglycosides have been an integral part of the arsenal of antibiotics used to treat life-threatening infections. Sadly, their effectiveness has been diminished by surges in resistance and dissemination. Occasionally, their level of resistance was so great that the aminoglycosides were virtually useless.18 The most common mechanism of resistance to aminoglycosides is enzymatic modification. These enzymes modify aminoglycosides at different –OH or –NH2 groups on the deoxystreptamine nucleus or on sugar moieties. The number of aminoglycoside-modifying enzymes identified to date, as well as the genetic environments in which the coding genes are found, are impressive.19,20 Moreover, aminoglycoside resistance can be supported by virtually any bacterium. Along with developing aminoglycosides resistant to as many modifying enzymes as possible, the following main strategies are being pursued for overcoming aminoglycoside modifying enzyme action: inhibiting enzyme action or expressing enzymes that modify enzyme action.
2.2. Blockers: modify the targets of antimicrobial agents
2.2.1 Penicillin binding proteins. Penicillin-binding proteins (PBPs), membrane-associated macromolecules that are involved in cell wall synthesis and are targets of β-lactam antibiotics, have been exploited. However, PBPs are inactivated once they are acylated by β-lactam antibiotics, they cannot catalyze the hydrolysis of covalent acyl enzymes, they cannot perform the transpeptidation of peptidoglycans, and the cell wall is weakened. Despite the strength of β-lactam antibiotics, drug-resistant strains are becoming an increasingly serious issue around the world. The periplasm of Gram-negative bacteria such as Pseudomonas aeruginosa and Escherichia coli evades the action of β-lactamases. Another mechanism preventing antibiotics from reaching their macromolecular targets is to force them out of the bacterium via antibiotic efflux pumps, such as MexA and B-OprM pumps in Pseudomonas strains.21 PBPs that are highly mutated and resistant to drugs are also produced by some Gram-positive bacteria, such as streptococci that do not produce β-lactamases. A new, highly resistant class B PBP (PBP2a) has been acquired by Staphylococcus aureus (S. aureus) strains following horizontal transfer of mecA from a yet unidentified species.22,23 While resistant bacteria incorporate these key mutations in PBP, they still divide and turn over peptidoglycan at similar rates; there is a lack of knowledge about the mechanisms underlying this sustained catalytic efficiency.
2.2.2 Processes of recycling peptidoglycan, the cell wall precursor. Bacterial cell walls contain large amounts of biopolymers that ensure the bacterial structural integrity to compensate for their inability to regulate osmotic pressure. In the cell wall, crosslinked peptidoglycan plays a major role, and an NAG-N-acetylmuramic acid (NAM) linear backbone is assembled. In this step, Lipid II is polymerized to yield a pentapeptide stem that is attached to NAM. In PBPs, typical peptide stems in G (−) bacteria are L-Ala-γ-D-Glu-m-DAP-D-Ala-D-Ala (in which DAP is diaminopimelate), which crosslink with other peptide stems on neighboring strands.24As a dynamic matrix, the cell wall is constantly being constructed and recycled at the same time as enzymes are involved in the process. With each generation, approximately half of a G (−) bacteria's cell wall is remodeled.25–27 A fragment of the cell wall can be biologically recycled for cell wall synthesis, and bacteria as well as eukaryotes use them as messengers for communication. PBP4, a penicillin-binding protein with DD-carboxypeptidase and 4,3-endopeptidase activity, has emerged as a key player in the detection and response to β-lactam antibiotics.28 Muropeptides are also very relevant as signaling molecules that are capable of provoking the multiresistance protein AmpC. When P. aeruginosa is resistant to a β-lactam antibiotic, signaling molecules have been identified in only two peptides over 20.29 Antibiotic resistance can be caused by many mechanisms, including the expression of AmpC, but new mechanisms linking cell-wall recycling to resistance have been described recently.30 There is a metabolic pathway in P. putida that links cell wall recycling to an intrinsic resistance to phosphomycin. As peptidoglycan plays a crucial role in bacterial survival and is remodeled by a large number of proteins, an emerging pathway could link recycling and antibiotic resistance in the near future.
2.2.3 Enzymes for DNA replication. An antimicrobial that inhibits bacterial DNA replication might be a promising strategy for combating antibiotic resistance because the replication of DNA involves multiple enzymes, and blocking any step in a single enzyme reaction can result in bacterial death, providing many potential targets for drug development; there is a conserved, distinct, or lack of expression of these enzymes in prokaryotic and eukaryotic cells, which confers selectivity to their corresponding inhibitors, preventing toxicity to humans.31,32 In clinics, only quinolones, which inhibit type II topoisomerases, are widely used,33 so any inhibitor of another DNA replication enzyme will provide a new antibacterial mechanism that is effective against a variety of antibiotic-resistant bacteria.
2.2.4 Ribosomes. A number of mechanisms have evolved that allow bacteria to resist antibiotics, and a large part of these mechanisms use ribosomes as their target. Throughout eukaryotic cells, an essential process of protein synthesis is carried out by ribosomes. Ribosomes are among the most complex and conserved macromolecular machines in nature. Although ribosomes are large, most of the clinically relevant antibiotics target relatively few regions that are functionally important, which has led to the overlapping of many binding sites.34,35 There is overlap between PTC-targeting antibiotics and A-site tRNA binding sites, or the binding sites span both A- and P-sites. During translation, the PTC transports the growing polypeptide chain to the macrolides and SB classes by binding to their binding sites.36–38 Most peptide bonds are not prevented by macrolides or SB members per se, but they do alter peptide chain elongation, which results in a drop-off in peptidyl-tRNA and abortive translation, causing an imbalance in protein synthesis. A variety of ribosome-targeting antibiotics have been identified as having antibiotic-resistance mechanisms.39–41 How antibiotics become resistant is fairly well understood, as well as their clinical significance. In some cases, ribosome target sites can be mutated or modified to reduce or eliminate the ability to bind antibiotics. A variety of enzymes can degrade, modify, or excrete antibiotics directly from the cell. Thus, the intracellular concentration is reduced from toxic to nontoxic.
2.3. Expellers: pump out antimicrobial agents
2.3.1 Efflux. One possible mechanism for multidrug resistance is the overexpression of efflux pumps. There are currently six family members of bacterial efflux pumps associated with efflux pathways. In one pump, ATP is directly used to drive transport via ATP-binding cassettes (ABCs). Five more types of active transporters utilize transmembrane gradients to capture electrochemical energy; these transporters are the major facilitator superfamily (MFS), the multidrug and toxin extrusion (MATE) family, the small multidrug resistance (SMR) family, the resistance-nodulation-cell division (RND) superfamily and the proteobacterial antimicrobial compound efflux (PACE) family.The association between efflux and drug resistance was first described over 40 years ago. For drug resistance to be effective, there must be an equal or greater amount of efflux than influx. In turn, drug entry is determined by the composition of the cell envelope and the presence of porins or other entry channels. There is no doubt that porin loss can contribute to resistance, and it has been observed to occur during treatment.42,43 Additionally, several cases of efflux-mediated resistance have been reported among clinical isolates, some of which involve the overexpression of pumps.
It is essential to have a clear understanding of efflux regulation because the overexpression of efflux proteins is caused by alterations in the regulatory system and transcriptional regulator mutations, including local and global mutations.44,45 There can be differences in efflux regulation between species, as well as within species, based on the physiological state of the cell. There are many pumps that are interconnected and regulated by complex circuits. Inhibiting or deleting one pump can lead to the expression of others.46–48 This complicates the interpretation of resistant phenotypes caused by efflux pump networks.
2.4. Decreased intracellular concentration
2.4.1 Decreased membrane permeability. Decreasing the degree of membrane permeability may increase the antibiotic resistance. By mediating the transport of molecules, the pores in the outer membrane play a critical role in the resistance and virulence of Acinetobacter baumannii (A. baumannii) strains. The decrease in membrane pore protein density of Acinetobacter A. baumannii was associated with increased carbapenem resistance.49,50 As another membrane porin involved in A. baumannii, OmpA has been reported to be resistant to aztreonam, chloramphenicol, and nalidixic acid. Studies have shown that OmpA increases virulence, lung infection, sepsis, and mortality.51–53
3. MDR modulators of natural product-derived compounds from plants
Even though bioactive molecules with plant origins are utilized for a variety of purposes, the use of these molecules for fighting MDR bacteria and restoring the effectiveness of antibiotics in clinics has largely been ignored. According to medicinal chemists, natural products are miscellaneous molecules that have evolved to interact with a wide variety of protein targets to accomplish specific goals.54–57 Hence, natural sources are considered to be an advantageous source for discovering new antimicrobial molecules. Over half of the drugs approved by the American Food and Drug Administration (FDA) are based on or inspired by natural products.
The necessary starting materials for drug discovery that are used by pharmaceutical companies have always been provided by nature, since these materials have always solved many complex clinical problems. Natural products have been studied extensively as modulators of MDRs. The MDR modulators of natural product-derived compounds from plants are summarized in Table 1 as three major categories: flavonoids, alkaloids and terpenoids.
Table 1 Natural product-derived compounds from plants as multidrug resistance modulators in microorganismsa
Classification |
Compound |
Source |
Antibacterial activity |
Mechanism |
Reference |
MRSA, methicillin-resistant Staphylococcus aureus; VER, vancomycin-resistant Enterococcus; MIC90, lowest drug concentration inhibiting 90% of bacterial or fungi growth; MIC, minimum inhibitory concentration; MDR, multidrug-resistant; UPEC, Uropathogenic Escherichia coli; CRKP, carbapenem-resistant Klebsiella pneumoniae; CRSM, carbapenem-resistant Serratia marcescens. |
Flavonoids |
(1) Isobavachalcone (IBC) |
Psoralen |
MRSA and VRE (MIC50: 0.5 μg mL; MIC90: 4–8 μg mL−1) |
Membrane homeostasis |
66 |
(2) α-Mangostin (AMG) |
Quercetin |
— |
P. aeruginosa and A. baumannii (MIC: 16–256 μg mL−1) |
Enzyme inhibition and efflux pump inhibition |
67 |
ATCC 25922 (MIC: 1 μg mL−1) |
E. coli clinical isolates (MIC: 64 μg mL−1) |
(1) 3,7-Diacylquercetin |
Quercetin |
Multidrug-resistant gram-positive (MIC: 0.13 to 128 μg mL−1) |
Enzymatic inhibition (DNA gyrase and topo IV) |
69 |
(2) Quercetin 6′′-acylgalactoside |
(3) Quercetin 2′′,6′′-diacylgalactoside analogues |
(1) Procyanidin B3-3-O-gallate |
W. uniflora |
MRSA |
Abnormal cell formation |
71 |
(2) Rhamnetin 3-O-(6′′-galloyl)-β-D-glucopyranoside |
(3) Rhamnetin 3-O-α-L-rhamnopyranoside |
(4) Quercetin 3-O-(6′′-galloyl)-β-D-glucopyranoside |
(1) Isobavachalcone; |
— |
E. coli AG100A and E. aerogenes EA298 isobavachalcone (MIC: 8 μg mL−1) |
Efflux pump components (AcrAB, TolC) |
72 |
(2) Diospyrone |
Diospyrone (MIC: 4 μg mL−1) |
Lupinifolin |
Albizia myriophylla |
MDR enterococcal clinical isolates (MIC: 0.5 and 2.0 μg mL−1) |
Membrane permeability and salt tolerance |
73 |
Phloretin |
Apple |
E. coli ZJ478 or Salmonella sp. Stain (MIC: 2 μg mL−1) |
Cell membranes |
74–76 |
Type A procyanidin (TAP |
Cinnamomum zeylanicum |
UPEC |
Biofilm formation |
77 |
Artonin I |
Morus mesozygia Stapf |
MDR bacterial (MIC: 4–8 mg L−1) |
Efflux pump and cell membrane |
78 |
AFPO |
Chalcones |
S. aureus 10 strain (MIC: 1024 μg mL−1) |
Internal resistance mechanism |
79 |
E. coli 06 strain (MIC: 256 μg mL−1) |
3′,4′,7-trihydroxyflavone |
Kernel |
P. stuartii ATCC299645 (MIC: 4 μg mL−1) |
Efflux pumps |
80 |
Epicatechin gallate (ECg) |
Green tea |
S. aureus BB568, EMRSA-15, EMRSA-16 (MIC: 128 mg L−1) |
Cell membrane |
89 |
Curcumin |
— |
A. baumannii (MIC >256 μg mL−1 |
— |
65 |
Epigallocatechin gallate |
Green tea |
A. baumannii (MIC >128–1024 μg mL−1) |
— |
65 |
Eriodictyol |
Citrus fruits |
S. aureus USA 300 (MIC >512 μg mL−1) |
Inhibitor of SrtA |
92 |
Rutin |
— |
K. pneumoniae ATCC700603 (MIC: 1024 μg mL−1) |
Downregulated luxS gene and wabG gene |
63 |
E. coli ATCC25922 (MIC: 512 μg mL−1) |
Baicalein |
— |
MRSA |
Cell wall and Tet K-mediated tetracycline efflux |
70 |
Ursolic acid (UA) |
Apple pomace |
CRKP (MIC: 0.8 mg mL−1) |
Cell membrane integrity |
93 |
5-Hydroxy-3,7,4′-trimethoxyflflavone |
V. gardneriana |
MDR bacterial strains S. aureus 358 and E. coli 27 (MIC≤512 μg mL−1) |
— |
94 |
Alkaloids |
Compound 1f (with a 7-phenyl group) |
7-Substituted cyclophosphamide (CBBR) |
MRSA and VRE (MIC: 1-8 μg mL−1) |
DNA Topo IV ParE subunit |
102 |
1,4-Naphthoquinones |
— |
MRSA (MIC: 0.0078 to 0.125 mg mL−1) |
— |
103 |
Chelerythrine (CHE) |
— |
CRSM (MIC: 125 mg mL−1) |
Integrity of the cell membrane |
105 |
Berberine hydrochloride (BBH) |
Berberine |
MDR A. baumannii (MIC: 256 mg L−1) |
AdeB and AdeB transporters |
106 |
Piperine |
White pepper |
MDR strains of P. aeruginosa, E. coli (in the presence of 125 mg mL−1) |
— |
107 |
Squalamine |
Dogfish shark squalus acanthias |
P. aeruginosa ATCC 27853 (MIC: 8 mg L−1) |
Cell membranes |
108 |
E. coli ATCC 25922 (MIC: 4 mg L−1) |
S. aureus ATCC 25923 (MIC: 2 mg L−1) |
N-tetradecyl derivative (BnI-14) |
Quaternary ammonium compounds (QACs) |
S. aureus ATCC 29213 (MIC: 0.03 μg mL−1) |
Membrane disruption and DNA |
110 |
S. aureus ATCC 29213 clinical/MRSA (MIC: 0.12 μg mL−1) |
E. coli clinical (MIC: 0.98 μg mL−1) |
Arborinine |
R. angustifolia |
Candida albicans arborinine (MIC: 250 μg mL−1) |
ICL1 gene |
111 |
Arborinine (MIC: 500 μg mL−1) |
Catharanthine |
Catharanthus roseous |
P. aeruginosa (MIC: 400 mg L−1) |
Efflux pump proteins MexA, MexB and OprM |
113 |
Chanoclavine |
I. muricata |
E. coli |
Efflux pumps and the cell envelope |
115 |
Terpenoids |
Limonene |
— |
E. coli (MIC: 16 μg mL−1) |
Cell membrane and DNA transcription and translation |
118 and 119 |
Carvacrol |
— |
S. aureus (MIC: 256 μg mL−1) |
Efflux pump inhibition |
120 and 122 |
Thymol |
Oleanolic acid |
— |
E. faecalis (MIC: 6.25 mg L−1) |
Efflux pump |
121 |
MRSA COLOXA (MIC: 1600 mg L−1) |
Clerodane diterpene 16α-hydroxycleroda-3,13(14)-Z-dien-15,16-olide (CD) |
Polyalthia longifolia |
MRSA-ST1745/ST2071/P4620 (MIC: 31.25 μg mL−1) |
EtBr efflux |
123 |
MRSA-P4627/P4423/ST3151 (MIC: 15.62 μg mL−1) |
Geraniol (Ger) |
Geranium oil |
C. albicans (MIC: 225 μg mL−1) |
Cell wall integrity and cell adhesion |
124 |
Oleanane triterpenoid aegicerin |
ClaVija procera |
MTB (MIC: 1.6-3.12 μg mL−1) |
— |
125 |
Artesunate (ART) |
Artemisia annua L |
MDR E. coli isolates |
mRNA expression of qnrB and qnrS |
126 |
Glycyrrhizin (GLY) |
Glycyrrhiza glabra |
MDR9 (MIC: 40 mg mL−1) |
— |
127 |
B1045 (MIC: 15 mg mL−1) |
Conjugates |
— |
S. aureus (MIC: 20 μg mL−1) |
— |
129 |
E. coli (MIC: 20 μg mL−1) |
1,8-Cineole |
Rosemary volatile oil |
E. coli |
Biofilm biomass disruption |
135 |
3.1. Flavonoids
Among the most important classes of phenolic compounds are flavonoids, which are plant secondary metabolites in nonglycosylated (aglycones) or glycosidic states. In addition to the 2-phenylchromane ring system, flavonoids also have different subclasses depending on the substitution at ring B and oxidation status of ring C.58,59 There has been considerable evidence that flavonoids possess a variety of pharmacological properties, including anticancer, anti-inflammatory, and antiviral properties.60–62 Additionally, flavonoids have been found to exhibit antibacterial properties against many pathogenic microorganisms, such as Staphylococcus aureus (S. aureus), Vibrio harveyi, Pseudomonas aeruginosa (P. aeruginosa), and Enterococcus faecalis (E. faecalis).63–65 There are over 8000 known flavonoids in plants, making them a potentially valuable resource for new antibiotic discoveries. We reviewed the potential antimicrobial activity of flavonoids against fungal and bacterial infections in Sections 3.1.1–3.1.13, and their structure was drown in Fig. 2.
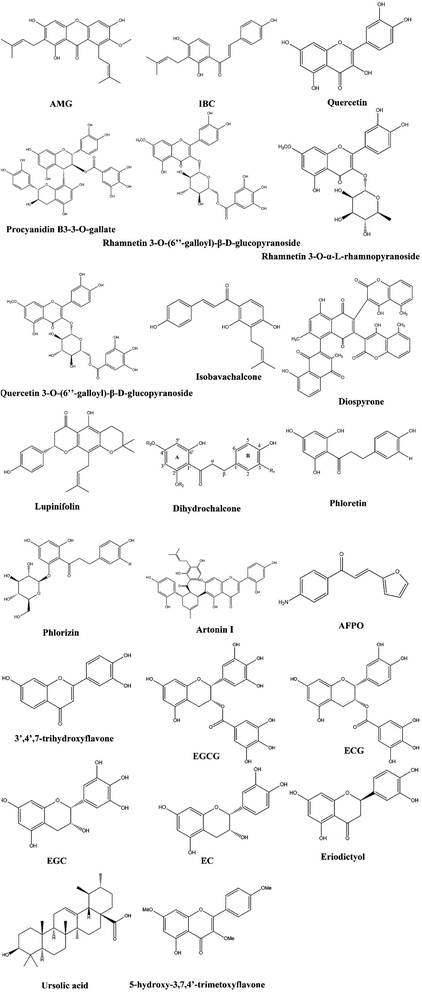 |
| Fig. 2 Structure of flavonoids having MDR modulatory activity. | |
3.1.1 Isobavachalcone (IBC) and α-mangostin (AMG). Analyses of structure-activity relationships show that prenylation modulates their activity in flavonoids and produces two compounds, isobavachalcone (IBC) and α-mangostin (AMG). Additionally, AMG and IBC are bactericidal against Gram-positive bacteria and restore the susceptibility of colistin against Gram-negative bacteria. AMG and IBC had MIC50 values of 0.5 μg mL−1 and MIC90 values of 4–8 μg mL−1 against methicillin-resistant Staphylococcus aureus (MRSA) and vancomycin-resistant enterococcus (VRE), respectively. AMG and IBC are capable of targeting almost all kinds of phospholipids in bacterial membranes and involve faster time-killing dynamics than that of nearly all antibiotics currently used in clinics, including vancomycin. Despite their ability to disrupt membranes, these agents are unlikely to develop resistance to antibiotics, since bacteria are unable to change their membranes without losing functions.66 In mice, AMG or IBC single-dose models were constructed for wound infection and intestinal colonization of S. aureus. The results showed that by using AMG and IBC, it may be possible to reduce the number of foodborne bacterial infections and poisonings.
3.1.2 Quercetin. In 2002, Denny et al. demonstrated for the first time that quercetin inhibited L1 metallo-β-lactamase.67 At high concentrations, quercetin has been found to inhibit Gram-positive and Gram-negative bacterial growth. By inhibiting blaNDM expression, quercetin could potentiate meropenem activity. The combination of quercetin-meropenem induced bacterial death by altering cell morphology and disrupting cell wall/membrane integrity, inhibiting carbapenem hydrolysis in periplasmic spaces and preventing bacterial efflux. Furthermore, quercetin inhibits carbapenemase and efflux pump activity in carbapenem-resistant E. coli, Klebsiella pneumoniae, P. aeruginosa and A. baumannii.68 Das et al. (2019) identified naphthoate synthase (1,4-dihydroxy-2-naphthoyl-CoA synthase, EC: 4.1.3.36; DHNS, EfDHNS) as an anti-E. faecalis target in novel antibacterial drugs. Moreover, quercetin inhibits EfDHNS catalytic activity, potentially allowing the use of quercetin for the treatment of MDR E. faecalis infections.69 According to structure-guided molecular design, quercetin diacylglycoside analogs were optimized, including 3,7-diacylquercetin, quercetin 6′′-acylgalactoside, and quercetin 2′′,6′′-diacylgalactoside analogs, which exhibited four times greater activity against Gram-positive bacteria than that of previous compounds. A possible mechanism of action of this drug is to inhibit bacterial DNA gyrase and topoIV enzymes simultaneously. More importantly, its acute toxicity in mice was very low, as well as realistic in situ intestinal absorption in rats.70 In another study, quercetin significantly enhanced the tetracycline sensitivity of E. coli. According to further studies, the combination therapy causes profound ultrastructural changes in the cells that result in an increase in permeability and a weakening of the cell envelope.71
3.1.3 The methanolic extract of W. Uniflora. Based on phytochemical analysis of the methanolic extract of W. uniflora leaves coupled with LC/MS analysis, the following flavonoid analogs (1–4) were isolated: procyanidin B3-3-O-gallate (1), rhamnetin 3-O-(6′′-galloyl)- β-D-glucopyranoside (2), rhamnetin 3-O-α-L-rhamnopyranoside (3), and quercetin 3-O-(6′′-galloyl)-β-D-glucopyranoside (4). In addition to inhibiting MRSA biofilm formation, these compounds also synergize with methicillin. Compounds achieved this synergistic effect by remodeling metabolism, and defects in central carbon metabolism and glutamine biosynthesis led to abnormal MRSA cell formation and a reduction in biofilm formation. Moreover, rhamnetin 3-O-(6′′-galloyl)-β-D-glucopyranoside (2) and quercetin 3-O-(6′′-galloyl)-β-D-glucopyranoside (4) showed no significant cytotoxicity.72
3.1.4 Isobavachalcone and diospyrone. Isobavachalcone and diospyrone were evaluated against multidrug-resistant (MDR) Gram-negative bacteria. The results showed that both compounds had intrinsic antibacterial properties against Gram-negative bacteria, and in the presence of an efflux pump inhibitor, their activity was significantly enhanced (MIC values decreased to below 10 μg mL−1). A significant increase in activity was also observed for diospyrone, and isobavachalcone was shown to be efficient against strains that have deletions of major efflux pump components (AcrAB, TolC). As a result of the study, it appears that isobavachalcone and diospyrone could be used as new antimicrobials against MDR bacteria, particularly by combining them with efflux pump inhibitors, which reinforces their activity.73
3.1.5 Lupinifolin. Lupinifolin, a prenylated flavonoid extracted from Albizia myriophylla Benth., was shown to exhibit a potent antimicrobial effect on enterococci. The bacterial membrane is damaged by lupinifolin, which inhibits S. aureus and Streptococcus mutans growth. Significantly reducing the salt tolerance of lupinifolin-disrupted bacterial cell membranes can also cause the membranes to lose their ability to osmoregulate effectively or to exclude toxic materials, eventually causing acterial cell death. As lupinifolin penetrated and passed through the cell wall of S. mutans, lupinifolin was observed in the cytoplasm of lupinifolin-treated cells. By doing so, lupinifolin disrupts the integrity of the cytoplasmic membrane, resulting in the death of the bacteria. Research on lupinifolin's antibacterial activity against a variety of MDR enterococci clinical isolates has furthered our understanding of its properties and mechanisms.74 In light of this, lupinifolin could play a critical role in fighting MDR enterococcal infections. There is potential for this compound to be developed into a new potent therapeutic agent.
3.1.6 Dihydrochalcone. Apples contain a specific group of flavonoids called dihydrochalcones, which possess a chemical structure of C6–C3–C6, and there is no direct link between the A-ring and B-ring; instead, a flexible C3 chain connects them. It was found that dihydrochalcones exhibited excellent antibacterial properties against both Gram-positive and Gram-negative bacteria. The glycosylation of the A-ring at the 2′-position, as well as the hydroxyl group at the 3-position of the B-ring, was important for the antibacterial activity of dihydrochalcones. A positive correlation might exist between dihydrochalcone hydrophobicity and its antibacterial properties. Dihydrochalcones act mainly by damaging cell membranes in antibacterial mechanisms.75 Despite this, strain type also influenced antibacterial mechanisms. The most abundant dihydrochalcone compound in apple is phlorizin, which accounts for 60–90% of the overall flavonoids. Gram-positive bacteria were inhibited by phloretin, which exhibited the highest levels of antimicrobial activity, especially S. aureus ATCC 6538, L. monocytogenes ATCC 13932, methicillin-resistant S. aureus clinical strains and S. typhimurium ATCC 13311. Considering that C20 contains a free hydroxyl group, it can be assumed that glycosyl molecules, or their absence, play an important role in interactions with other biological molecules, and C20, C30, and C50 glycosylated structures demonstrate dramatic decreases in antimicrobial activity. In addition, this compound's antimicrobial activity can be understood through the analysis of cytosolic enzymes from S. aureus. Essentially, phloretin inhibits S. aureus energetic metabolism by lowering lactate dehydrogenase (LDH) and isocitrate dehydrogenase (IDH) enzyme activity. It significantly inhibits catalase activity and determines the use of biological fuels (sugars, fatty acids, amino acids) by bacteria and its ability to deal with oxidative damage.76 Phloretin restored polymyxin E's sensitivity to E. coli ZJ478 or Salmonella sp. stain HYM2 from 64 μg mL−1 to 2 μg mL−1 for both bacteria. Phloretin and polymyxin E were used to treat a variety of strains, including those that were mCR-1 positive and negative, and the fractional inhibitory concentration (FIC) values were all determined to be under 0.5. Despite this, bacterial resistance was not triggered by the combination of phloretin and polymyxin E. Combined treatment led to an 80% survival rate of infected mice, as well as a significant decrease in cecal colony counts.77
3.1.7 Type A procyanidin. The antimicrobial activity of Type A procyanidin (TAP) from Cinnamomum zeylanicum against QSLUPEC7 (a multidrug-resistant strain that forms strong biofilms) was researched. In contrast to the growth of the MDR strain, TAP treatment affected the formation of biofilms (∼70%). Furthermore, different pH levels were used to study the synergy between TAP and nitrofurantoin (NIT).78 The results of the study indicate that depending on the pH level, TAP interacts synergistically with nitrofurantoin. At pH 5.8, the greatest growth inhibition was observed. Gene expression analysis indicated that TAP inhibits Uropathogenic Escherichia coli (UPEC) fimbriae adhesins alone or in combination with NIT. In conclusion, TAP inhibits the growth of multidrug-resistant strains of UPEC without affecting their antibiofilm activity.
3.1.8 Artonin I. Artonin I inhibits MDR S. aureus growth both on its own and when combined with different antibiotics. Artonin I also showed potential to reverse resistance in further mechanistic studies. Depolarization of bacterial cell membranes and inhibition of efflux mechanisms have been revealed by flow cytometric studies.79 Artonin I is responsible for the production of ROS, which causes cell death. Artonin I damages cell membranes and alters the pattern of cell division, resulting in cellular destruction.
3.1.9 AFPO. The chalcone (E)-1-(4-aminophenyl)-3-(furan-2-yl)-prop-2-en-1-one (C13H11NO2) AFPO has altered the effectiveness of norfloxacin, penicillin, ampicillin/sulbactam, and gentamicin against multiresistant strains of S. aureus, combining all antibiotics synergistically and reducing concentration requirements. AFPO also modified the activities of norfloxacin, gentamicin, and penicillin and lowered the MIC for the E. coli 06 strain.80 This means that AFPO in combination with antibiotics tested may reduce the effects of medications used to treat infections caused by S. aureus 10 and E. coli 06 in humans.
3.1.10 3′,4′,7-trihydroxyflavone. Kernel methanol extract (MFS) and one of its derived compounds, 3′,4′,7-trihydroxyflavone, exhibit important antibacterial activity against MDR strains. On the tested MDR, a crude MFS extract and 3′,4′,7-trihydroxyflavone both showed improved antibacterial activity in the presence of PAβN (EPI) on 11/15 (73,37%) and 7/7 (100%), respectively.81 Based on these results, we believe that crude MFS extracts and their active constituents may be substrates for efflux pumps and thus have an intracellular target. 3′,4′,7-Trihydroxyflavone enhanced antibiotic activity in most bacterial strains.
3.1.11 Tea polyphenols. In addition to having numerous biological activities, tea always attracts worldwide interest. Due to their ability to scavenge free radicals, tea polyphenols are well known for their medicinal value, and several studies have shown that they can improve the body's immune system and alleviate a number of symptoms.82,83 In addition, there is much evidence that tea catechins have antiviral and antimicrobial properties. In vitro anti-methicillin resistant Staphylococcus aureus (MRSA) activity can be enhanced by combining several β-lactams with Japanese green tea extracts. Additionally, green tea extract itself was shown to inhibit S. aureus ATCC 25923 as well as MRSA at 400 μg mL−1 MICs.84 As much as 30-40% of the water-soluble solids and 20–30% of the dry matter in green tea are flavan-3-ols (also called catechins). Catechins in green tea mainly include ester (−)-epigallocatechin-3-O-gallate (EGCG), epicatechin-3-O-gallate (ECG), (−)-epigallocatechin (EGC), (−)-epicatechin (EC) together with some (−)-gallocatechin-3-O gallate (GCG), and (−)-catechin-3-O-gallate (CG).85,86 In total, EGCG accounted for nearly 59%, followed by EGC at 19%, ECG at 13.6%, and EC at 6.4%. Studies have shown that green tea catechins, mainly EGC, EGCG, and ECG, have antibacterial properties.87,88 Natural and semisynthetic catechins, as well as their gallated derivatives, were tested for anti-MRSA activity, and it was found that they have MICs in the range of 64–256 mg L−1.89 Gallate esterification at the C-ring of catechins is also a method of enhancing oxacillin and lactam antimicrobial activity. The use of various 3-O-acyl chains instead of the gallate moiety of epicatechin gallate (ECg), could also enhance the antibacterial strength of catechins against MRSA.90A high percentage of therapeutic antimicrobials are no longer effective against MDR strains, and EGCG should be able to improve their treatment. Another study found that EGCG was effective in modifying resistance in Campylobacter spp. by inhibiting efflux pump activity in the wild-type strain NCTC 11168 as well as in the cmeB, cmeF, and cmeR mutants.91 EGCG prevents conjugative drug resistance by reducing the transfer of drug resistance plasmids in a dose-dependent manner. Tetracycline resistance is reversed by EGCG when it inhibits the Tet(K) efflux pump (EP) in staphylococci, resulting in antibiotic sensitivity in infected staphylococci.92
Curcumin (CCM) and EGCG were investigated and evaluated against multidrug-resistant strains of A. baumannii via checkerboard and time-kill assays. CCM had an MIC of >256 μg mL−1 against all strains of A. baumannii, whereas EGCG had an MIC of 128–1024 μg mL−1. Five of nine isolates showed synergy, and four isolates showed additive effects, according to checkerboard studies. CCM's MIC was reduced by 3- to 7-fold when EGCG was added, and a CCM MIC of 4 μg mL−1 was the strongest interaction. In comparison with the most effective polyphenol alone, a combination of CCM and EGCG (1
:
8 and 1
:
4) demonstrated 4- to 5-log reductions in viable counts after 24 hours. Although CCM alone exhibits little antibacterial activity, EGCG significantly enhances its activity against MDR A. baumannii. Combinations of these two substances may be useful for medicinally treating or preventing infections caused by A. baumannii.65
3.1.12 Eriodictyol. Eriodictyol, a reversible inhibitor of SrtA with an IC50 of 2.229 ± 0.014 g mL−1, can be used as an innovative tool for countering resistance as well as virulence. Eriodictyol inhibited bacterial adhesion to fibrinogen and decreased biofilm formation and staphylococcal protein A (SpA) anchoring to cell walls. It was found that eriodictyol and SrtA had a strong interaction during fluorescence quenching experiments. In subsequent mechanistic studies, eriodictyol was shown to interact with the R197 amino acid residue of SrtA. Moreover, eriodictyol inhibited adhesion-dependent invasion of A549 cells by S. aureus and was effective in treating mouse pneumonia.93
3.1.13 Other flavonoids. Rutin, a flavonoid, induces luxS and wabG gene expression to inhibit K. pneumoniae in biofilm cells. A positive correlation was observed between biofilm biomass and the type III fimbriae biosynthesis gene mrkA.63Through its direct binding to the peptidoglycan in the cell wall, baicalein can work synergistically with tetracycline to compromise the integrity of the cell wall. In addition to inhibiting Tet K-mediated tetracycline efflux, baicalein inhibits Tet M and other pumps that are responsible for methicillin-resistant S. aureus (MRSA) growth.71
An antimicrobial agent found in apple pomace, ursolic acid (UA), exhibits antimicrobial properties against some microorganisms. UA was found to be effective against Klebsiella pneumoniae (CRKP) at an MIC of 0.8 mg mL−1. The drug disrupted CRKP's cell membrane integrity, inhibited biofilm formation, and inactivated cells that were encased in biofilms.94
The compound 5-hydroxy-3,7,4′-trimetoxyflavone, which has a chemical formula of C18H16O6, was isolated from the leaves of V. gardneriana. In combination with norfloxacin and gentamicin, this flavone exhibits antimicrobial activity against the MDR bacteria S. aureus 358 and E. coli 27. Hence, this natural compound contributes to the control of resistant bacteria by increasing antibiotic activity.95
3.2. Alkaloids
Compared to synthetic antibacterial agents, alkaloids have fewer side effects, a higher efficacy and superior antibacterial properties against both resistance acquired pathogens and nonresistance acquired pathogens.96,97 In addition, alkaloids combined with antibiotics increased their efficacy, reduced side effects, and increased their concentration.98–100 In addition to inhibiting protein synthesis and efflux pumps, alkaloids inhibit the formation of biofilms, inhibit drug deactivating enzymes, and destroy cell membranes and cell walls.101,102 Using this natural gift to its full potential requires advanced studies. A variety of advanced methods, such as virtual screening, molecular modeling, and biological studies, can be used to alter the properties of these substances. This new era of antimicrobial agents may arise through the development of these alkaloids (Fig. 3).
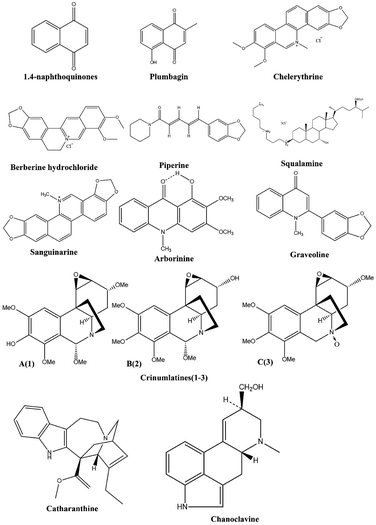 |
| Fig. 3 Structure of alkaloids having MDR modulatory activity. | |
3.2.1 Cycloberberine (CBBR). A novel family of MRSA agents has been identified using cycloberberine (CBBR) scaffolds that were constructed from Chinese natural alkaline berberine (BBR). Based on the structure-activity relationship (SAR), this compound series was significantly more potent against both reference strains and MDR clinical isolates by substituting at C8 or C13 (Fig. 4A). CBBR 7-monosubstitution was the focus of the initial SAR study. After attaching aliphatic chains or rings to the C7 position, compounds 1a–e (Fig. 4B) (with MIC values between 2 and 64 μg mL−1) display moderate antimicrobial activity against all tested strains, which is better than CBBR. Furthermore, measurements were performed on compounds 1f–j (Fig. 4B) with different substituted benzene groups, and compound 1f showed promising activity against both MRSA and vancomycin-resistant Enterococcus (VRE) strains. In contrast to vancomycin, compound 1f with a 7-phenyl group shows higher activity against MRSA and VRE, with MIC values of 1–8 μg mL−1. Based on its rapid bactericidal action against MRSA, 1f might target the DNA ParE subunits of Topo IV bacteria, suggesting that antibacterial drugs currently used have a different mode of action. Compared with SAR results at the C8 or C13 positions, a phenyl ring at the C7 position may enhance the antimicrobial spectrum. Further studies indicated that antimicrobial activity was dependent on the methylenedioxy ring.103
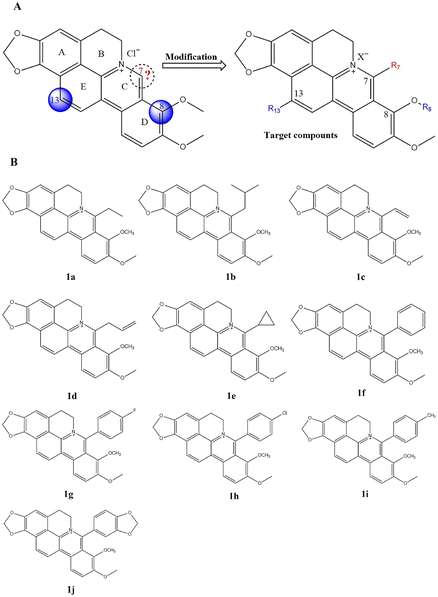 |
| Fig. 4 (A) The chemical structure of CBBR and the structure modification strategy. (B.) the structure of the target compounds 1a–j. | |
3.2.2 1,4-Naphthoquinones. It was demonstrated that 1,4-naphthoquinones inhibit bacterial growth. In both Gram-positive and Gram-negative bacteria, bacteriostatic and bactericidal effects are observed with 1,4-naphthoquinone and plumbagin. In clinical strains of methicillin-sensitive S. aureus and MRSA, 1,4-naphthoquinone showed synergistic interactions with imipenem, cefuroxime, and cefotaxime. In MRSA cultured with ATCC, 1,4-naphthoquinone and cefotaxime have synergism. Combining 1,4-naphthoquinone with imipenem produced only an additive effect, while 1,4-naphthoquinone and cefuroxime had an antagonistic effect. While 1,4-naphthoquinone on its own is not as effective as common antibiotics, it synergizes with imipenem, cefuroxime, and cefotaxime in the treatment of MRSA, and it may be used as an adjunctive antibiotic to treat multidrug resistant bacteria.104
3.2.3 Chelerythrine (CHE). The natural product chelerythrine (CHE) is a benzophenanthridine alkaloid with antimicrobial potential. In the case of methicillin-resistant Staphylococcus aureus and E. coli producing extended-spectrum β-lactamases, CHE greatly improved the antibacterial efficacy of the antibiotics.105 CHE had an MIC of 125 mg mL−1 against CRSM, inhibited growth and destroyed the integrity of the cell membrane, and clearly altered the morphology of the cell. Biofilm components are produced by sub-MIC CHEs, which showed robust inhibitory effects against CRSM biofilm formation. In addition, according to CLSM- and FESEM-mediated evaluation of biofilm damage and biofilm persistence, biofilms can be compromised by CHE at high concentrations and remove previously formed biofilms.106
3.2.4 Berberine hydrochloride (BBH). Berberine hydrochloride (BBH) is the most common form of berberine. An in vitro study was conducted to determine the synergistic effects of BBH with antibiotics against MDR A. baumannii. There was weak antimicrobial activity for BBH alone against MDR A. baumannii (MIC of 256 mg L−1). A dramatic increase in susceptibility to antimicrobials when fractional inhibitory concentration index values <0.5 was achieved, even reversing the resistance of MDR strains. According to an in vivo study, BBH with sulbactam had greater antimicrobial effectiveness than that of monotherapy in neutropenic murine thigh infections. According to the antibiotic-sensitizing mechanism, boosting the expression of AdeB and binding to AdeB transporters resulted in a lower uptake of BBH, resulting in fewer antibiotics being extruded by the AdeABC pump. As a result of knockout of the adeB gene, BBH was more readily taken up by MDR strains, and resistance to antibiotics as well as synergistic effects between antibiotics and BBH were lessened. As a result, BBH effectively resensitizes this MDR pathogen to antibiotics that have become almost ineffective due to antibiotic resistance.107 Due to this, BBH may work synergistically with antibiotics and have antibiotic-sensitizing activity against MDR A. baumannii.
3.2.5 Piperine. The growth of V. cholerae, an active ingredient of white pepper, with 200 and 300 μg mL−1 piperine was inhibited regardless of biotypes or serogroups. Piperine (in the presence of 200 μg mL−1) also showed growth inhibitory activity against MDR Gram-negative bacteria, including toxigenic Vibrio cholerae, Pseudomonas aeruginosa and enterohemorrhagic/enteroaggregative E. coli O104 German outbreak strains.108
3.2.6 Squalamine. Squalamine, a natural aminosterol, has been demonstrated to possess antibacterial properties against several drug-resistant bacteria and molds. A squalamine's positively charged amino group interacts with a Gram-negative bacteria's lipopolysaccharide's negatively charged phosphate groups. As a result of its depolarizing effect, it causes cell death in Gram-positive bacteria, similar to colistin (polymyxin drug). Colistin displaces the divalent cations (Ca2+ and Mg2+) after forming a bond with LPS's phosphate groups.109
3.2.7 Other alkaloids. Chelerythrine, an isoquinoline alkaloid, inhibits cellular division and nucleic acid synthesis. A protein called FtsZ, which contributes to Z ring formation during cell division, is tampered with by isoquinoline, inhibiting cellular division.110 The mechanism of action of quaternary ammonium compounds (QACs) targets the bacterial membrane, making them effective antimicrobial agents. For N-benzylimidazole, the synthesis of QACs based on imidazole derivatives, the activity varied in the range of the lowest inhibitory concentration (MICs) to 7 ng mL−1. A bacterial biofilm-fighting compound, n-tetradecyl derivative (BnI-14), was also found to bind to DNA, implying interference with bacterial growth.111 In Gram-positive and Gram-negative bacteria, sanguinarine inhibits cytokinesis by disrupting Z-ring assembly through binding to FtsZ and affecting the bundling of protofilamints.Arborinine and graveoline exhibit inhibitory activity against C. albicans at 250 and 500 lg/ml, respectively. MIC/MFC indicated that these alkaloids played a bactericidal role. Analysis of gene and protein expression revealed effective effects for both compounds on ICL1 gene andprotein expression. Hence, arborinine and graveoline appear to be promising inhibitors of ICL1 in C. albicans glyoxylate cycles.112 A phytochemical investigation of the 90% ethanol aqueous extract of the bulbs of Crinum latifolium led to the isolation of three new crinane-type alkaloids, designated crinumlatines A-C (1–3). Compounds 1–3 exhibited some antimicrobial activity against the tested Gram-negative bacteria with minimum inhibitory concentration values less than 50 lg mL−1.113
Catharanthine was deduced first through in silico inhibition of efflux pump proteins MexA, MexB and OprM. Catharanthine-induced efflux pump inhibition may be useful for lowering antibiotic doses, reducing drug resistance, and increasing the efficacy of old antibiotics against multidrug-resistant bacteria.114
Plants selected for anti-mastitis protection contain higher concentrations of phytochemicals, with alkaloids of A. sativum and B. persicum showing significant antibacterial activity compared to that of the other selections. Additionally, ATCC strains show greater inhibition of phytochemicals of tested plant species compared to that of MDR bacterial strains.115
We can see that chanoclavine may make it easier to repurpose antibiotics that have become nonfunctional because of acquired bacterial resistance. By inhibiting efflux pumps, weakening the cell envelope, and increasing cell growth, the molecule synergizes with conventional antibiotics to target bacterial cell membranes or cell walls.116
3.3. Terpenoids
Terpenoids, which are abundant in nature and produced in response to microbial attack, have huge potential as antimicrobial agents through a variety of mechanisms, such as disruption of membranes, anti-quorum sensing, inhibition of protein synthesis and ATP.92,117,118 Combinations of terpenoids and antimicrobials, such as combination therapy, have increased the effectiveness of treatment against multidrug resistant microorganisms by showing synergy (Fig. 5).
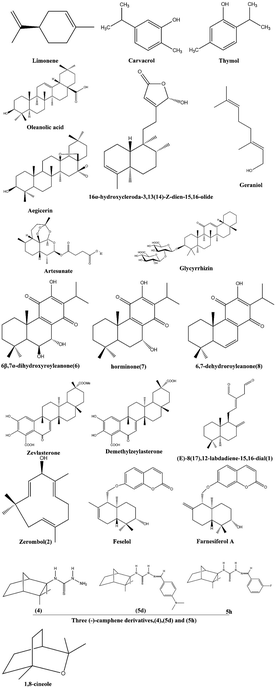 |
| Fig. 5 Structure of terpenoids having MDR modulatory activity. | |
3.3.1 Limonene. The antibacterial activity of limonene-containing essential oils has been demonstrated against resistant strains of S. aureus, Salmonella enterica, and Listeria monocytogenes. The mechanism may be due to the lipophilic properties of limonene. Limonene may cross the cell wall and alter the permeability of bacterial cell membranes.119 When E. coli cells were treated with limonene, the cell membrane was disrupted, cellular leakage occurred, and cell death occurred. Protein leakage, lipid leakage, and nucleic acid leakage confirmed that membrane damage and disruption of the permeability barrier of the cell occurred. In addition, the release of intracellular ATP suggested disruption of membrane barriers occurred. The interaction of limonene with DNA revealed its ability to unwind plasmids, which could eventually inhibit DNA transcription and translation.120 Various proteins and enzymes involved in transport, respiration, metabolism, chemotaxis, and protein synthesis were expressed differently, confirming the mechanistic role that limonene plays in these processes.
3.3.2 Carvacrol and thymol. Both carvacrol and thymol are moderately effective against multiresistant S. aureus strains with the NorA, SA-1199 (wild type), and SA-1199B (overexpressed NorA) efflux pumps. Additionally, carvacrol and thymol potentiated the MIC of norfoxacin and ethidium bromide, increasing the effectiveness of these compounds against strains that carry the NorA efflux proteins SA-1199 and SA-1199B.121 Based on the docking results, the NorA binding pocket is involved in the interaction between carvacrol and thymol. It is confirmed that these terpenes compete with Norfoxacino and ethidium bromide for NorA binding sites.
3.3.3 Oleanolic acid. Martins et al. (2011) isolated and tested six compounds, namely, uvaol, β-amyrin, oleanolic acid, catechin, epicatechin and monogalactosyldiacylglycerol. In studies involving a wide range of bacterial strains, oleanolic acid showed strong antimicrobial activity. In comparison to all of these compounds, uvaol reduced MRSA COLOXA's resistance to the antibiotic oxacillin most effectively. Overexpressed efflux pump systems are responsible for the MDR phenotype of MRSA COLOXA. Uvaol inhibits EtBr accumulation and efflux by MRSA COLOXA strains that are dependent on glucose levels, suggesting that the effect of uvaol on the efflux pump system of this organism appears to be the mechanism that contributes to its ability to reduce oxacillin resistance.122 Uvaol's effect on the efflux pump system of other MDR strains also contributes to reversing their resistance to given antibiotics.
3.3.4 Thymol. Oil from T. ammi contains mostly thymol, which is among a class of natural phenolic monoterpenes known as biocides. Whether used alone or in conjunction with other biocides, such as carvacrol, thymol has a strong antimicrobial effect.123 Previous studies on Gram-positive test strains have found that compounded substances influence many physiological characteristics, including cytoplasmic membrane permeability, coagulase activity, and salt tolerance.
3.3.5 16α-hydroxycleroda-3,13(14)-Z-dien-15,16-olide (CD). CD from leaves of Polyalthia longifolia (Sonn.) were examined. By using Thwaites (Annonaceae) as RAM, current staphylococcal infection treatment strategies can be improved. CD significantly reduced the MIC of fluoroquinolones up to 16-fold (FICI 0.315-0.500). Combining CD with norfloxacin significantly (p < 0.01, p < 0.001) reduced the systemic microbial burden in blood, liver, kidney, lung, and spleen tissues of Swiss albino mice infected with S. aureus, norfloxacin alone or untreated control.124 Flow cytometry analysis clearly demonstrated that CD significantly inhibited EtBr efflux and extended its postantibiotic effects.
3.3.6 Geraniol (Ger). In aromatherapy, Ger is traditionally used to treat vaginal candidiasis since it is a monoterpene alcohol that comprises approximately 20% of geranium oil. Through transmission electron micrographs, we were able to observe depleted ergosterol levels and altered plasma membrane ATPase activity as signs of membrane tampering. The effect of Ger on cell walls has also been documented by spot assays with agents that perturb the cell wall and scanning electron micrographs.125 There seems to be an essential role for the calcineurin pathway in the antifungal effect of Ger because the calcineurin signaling mutant was highly resistant to Ger, whereas the strains overexpressing calcineurin remained resistant. Ger also causes mitochondrial dysfunction, iron homeostasis disturbances, and genotoxicity. Moreover, Ger inhibits both hyphal morphogenesis and biofilm formation, two of its leading virulence attributes.
3.3.7 Aegicerin. Based on a colorimetric bioassay-guided fractionation protocol against Mycobacterium tuberculosis (MTB), the active constituent of an extract of the Peruvian plant ClaVija procera was identified as oleanane triterpenoid aegicerin126. Its MIC values ranged between 1.6 and 3.12 μg mL−1 against 37 different sensitive and resistant MTB strains (1H37Rv, 21 susceptible clinical isolates, 2 INH-resistant clinical isolates, and 13 MDR clinical isolates).
3.3.8 Artesunate (ART). According to recent studies, ART is effective at inhibiting efflux pump genes and may increase the effectiveness of various β-lactam antibotics in the treatment of MDR E. coli.127 Another study revealed that antimicrobial activity was not demonstrated by ART. However, a dramatic synergistic effect of ART and FQs was observed with (∑FIC) = 0.12–0.33. As a result of ART, MDR E. coli isolates accumulated DNR and expressed acrAB-tolC mRNA less than control isolates, but compared to the controls, qnrB and qnrS exhibited a greater expression.
3.3.9 Glycyrrhizin (GLY). GLY is a glycoconjugated triterpene derived from licorice root (Glycyrrhiza glabra), and the antimicrobial and anti-inflammatory effects of GLY can cause severe and difficult-to-treat keratitis in vivo in isolates of MDR organisms. Various mechanisms appear to be involved, including the MIC of ciprofloxacin being lowered, bacteria being permeabilized, swelling occurring, and clumps forming.128 Through the reduction of efflux pump activity and the increase in bacterial killing, ciprofloxacin has been shown to improve clinical outcomes.
3.3.10 Ryleanones. The royleanones, which contain a p-benzoquinone C ring, i.e., 6β,7a-dihydroxyroyleanone (6), horminone (7), and 6,7-dehydroroyleanone (8), showed mild activities against the MDR strain (MIC values 12.5 mg mL−1), and, distinctively, the MIC value of 7a-acetoxy-6b-hydroxyroyleanone (5) was 3.12 mg mL−1, which was the most potent antimycobacterial agent against the MDR strain and against the H37Rv strain with an MIC value of 25 mg ml−1.129 Based on these results, the presence of the 7a-AcO group in the B ring may be essential for increasing MDR-MTB activity.
3.3.11 Conjugates. By computing docking scores for outlandish conjugates of SMZ and monoterpenes, effective agents against DHPs of five bacterial species can be identified. The docking scores for the monoterpenes or SMZ conjugates against five bacterial DHPs were also higher than those for monoterpenes or SMZ alone. In addition, conjugates have been shown to be safer antibacterial agents when both toxicity and LD50 values are taken into consideration. Conjugate 5 (SMZ + thymol) with docking scores greater than −10.0 kcal mol−1 was the most effective and safest antibacterial agent, while SMZ had docking scores of −8.46 and a high LD50 value of 3500 mg kg−1.130
3.3.12 Farnesol. By inhibiting biofilm resistance, farnesol, a QSM secreted by C. albicans, affects the formation of biofilms. C. albicans has been shown to be inhibited not only on its ERG genes but also on its MDR1 gene, an important multidrug resistance gene.131 In addition to its many other effects on eukaryotic and prokaryotic cells, FAR also alters ABC efflux transporters, resulting in changes in resistance to azoles in C. albicans isolates.132 However, this effect is dependent on FAR concentrations.
3.3.13 Zeylasterone and demethylzeylasterone (6-oxophenolic triterpenoids). Zeylasterone and demethylzeylasterone (6-oxophenolic triterpenoids) obtained from the roots of Maytenus blepharodes showed potent activity against Staphylococcus aureus. Compared to demethylzeylasterone, zeylasterone showed superior activity against microbial cytoplasmic membranes, causing them to rupture. Ethyl acetate extracts from Croton macrostachys were tested for their antibacterial properties against S. aureus and antifungal properties against Candida.133 Five compounds were obtained, including two were lupane triterpenoids and three clerodane diterpenoids, with betulin and 12-oxo-hardwickic acid showing the greatest activity. It was found that lupeol isolated from the aerial parts of brillantaisia lamium and trichodesma amplexicaule inhibited bacterial and fungal growth significantly by causing membrane damage as a possible mechanism of action.
3.3.14 Other terpenoids. Bioassay-directed isolation of secondary metabolites from the rhizomes of Zingiber montanum (E)-8(17),12-labdadiene-15,16-dial (1), zerombol (2) demonstrated antibacterial activity (MIC values 32–128 g mL−1) against a series of clinical isolates of MDR and MRSA.134Feselol and farnesiferol A are sesquiterpene coumarins that are obtained by extracting aerial parts of Ferula vesceritensis (Apiaceae) in dichloromethane, which is associated with a model of the recombinant nucleotide binding site of Cryptosporidium parvum's enteropathogenic efflux pump.135
For the first time, the antibiofilm activity of 1,8-cineole against E. coli producing MDR ESBLs was demonstrated.136 There is no doubt that the compound is capable of causing substantial bacterial death in biofilm-attached as well as biofilm-released cells. The (−)-camphene derivatives tested in the present study, particularly (4) (5d) and (5 h), were shown to be promising anti-TB molecule scaffolds due to the low MIC values in acidic pH against the reference strain H37Rv and MDR M. tuberculosis clinical isolates, their low cytotoxicity and the synergism observed with PZA.137
All bacteria tested were inhibited by the highest polar fraction of Cistus ladaniferus essential oil, which contained mono- and sesquiterpene alcohols with MIC values ranging from 0.05 to 0.8 mg mL−1.138 There was high activity of this fraction against the MDR strsain Enterobacter aerogenes EA289, and its mechanism involves a distortion of the cell wall with detachment of the outer cytoplasmic membrane.
In vitro antibacterial activity of the essential oil of E. camaldulensis against multidrug resistant A. baumannii wound isolates, demonstrating the plant's traditional use for wounds. As constituents of the E. camaldulensis oil, polar terpene compounds and spathulenol may contribute to the observed antibacterial activity.139 Additionally, synergistic interactions of the antibiotics ciprofloxacin, gentamicin and polymyxin B with E. camaldulsensis essential oils were detected in this study for the first time against MDR A. baumannii isolates.
4. Conclusion and perspectives
In contemporary society, antibiotics have become an important part of everyday life; the wonders of antibiotics reveal how useful nature can be. Multidrug resistance (MDR) as well as cross-resistance to other drugs have become global concerns due to inappropriate use and overprescription of medications. In response to this emerging problem, we are entering an era of responsibility. Novel solutions are urgently needed to address this significant issue. Natural products are currently being investigated for their antimicrobial properties. Based on the current literature, it appears that there is an urgent need to coordinate efforts for meaningful research and for finding novel alternatives that utilize natural products.
The present study presents a wealth of information regarding the phytocomplexes and mode of action of purified antimicrobials that are isolated and purified from potential medicinal plants. Additionally, these components can be used to design new nutraceuticals or other drugs that are effective. New therapeutics will, however, be discovered by exploring the additional bioactivity of the corresponding species and genus.
Conflicts of interest
There are no conflicts to declare.
References
- N. S. Isles, A. Mu, J. C. Kwong, B. P. Howden and T. P. Stinear, Gut microbiome signatures and host colonization with multidrug-resistant bacteria, Trends Microbiol., 2022, 30, 853–865 CrossRef CAS PubMed.
- X. Pang, D. Li, J. Zhu, J. Cheng and G. Liu, Beyond Antibiotics: Photo/Sonodynamic Approaches for Bacterial Theranostics, Nanomicro Lett., 2020, 12, 144 CAS.
- F. Baquero, J. L. Martinez, V. F. Lanza, J. Rodriguez-Beltran, J. C. Galan, A. San Millan, R. Canton and T. M. Coque, Evolutionary Pathways and Trajectories in Antibiotic Resistance, Clin. Microbiol. Rev., 2021, 34, e0005019 CrossRef CAS PubMed.
- M. F. Chellat, L. Raguz and R. Riedl, Targeting Antibiotic Resistance, Angew. Chem., Int. Ed. Engl., 2016, 55, 6600–6626 CrossRef CAS PubMed.
- S. H. Jung, C. M. Ryu and J. S. Kim, Bacterial persistence: Fundamentals and clinical importance, J. Microbiol., 2019, 57, 829–835 CrossRef PubMed.
- A. J. Trotter, A. Aydin, M. J. Strinden and J. O'Grady, Recent and emerging technologies for the rapid diagnosis of infection and antimicrobial resistance, Curr. Opin. Microbiol., 2019, 51, 39–45 CrossRef CAS PubMed.
- M. S. Butler, Natural products to drugs: natural product-derived compounds in clinical trials, Nat. Prod. Rep., 2008, 25, 475–516 RSC.
- E. K. Davison and M. A. Brimble, Natural product derived privileged scaffolds in drug discovery, Curr. Opin. Chem. Biol., 2019, 52, 1–8 CrossRef CAS PubMed.
- T. Rodrigues, D. Reker, P. Schneider and G. Schneider, Counting on natural products for drug design, Nat. Chem., 2016, 8, 531–541 CrossRef CAS PubMed.
- K. H. Kyung, Antimicrobial properties of allium species, Curr. Opin. Biotechnol., 2012, 23, 142–147 CrossRef CAS PubMed.
- M. Daglia, Polyphenols as antimicrobial agents, Curr. Opin. Biotechnol., 2012, 23, 174–181 CrossRef CAS PubMed.
- K. Bush and P. A. Bradford, beta-Lactams and beta-Lactamase Inhibitors: An Overview, Cold Spring Harbor Perspect. Med., 2016, 6, a025247 CrossRef PubMed.
- K. M. Papp-Wallace, A. Endimiani, M. A. Taracila and B. R. A. Carbapenems, past, present, and future, Antimicrob. Agents Chemother., 2011, 55, 4943–4960 CrossRef CAS PubMed.
- V. Miguel-Ruano and J. A. Hermoso, Teaching an old dog new tricks: repurposing beta-lactams, Trends Pharmacol. Sci., 2021, 42, 617–619 CrossRef CAS PubMed.
- R. B. Hamed, J. R. Gomez-Castellanos, L. Henry, C. Ducho, M. A. McDonough and C. J. Schofield, The enzymes of beta-lactam biosynthesis, Nat. Prod. Rep., 2013, 30, 21–107 RSC.
- K. Bush and P. A. Bradford, Interplay between beta-lactamases and new beta-lactamase inhibitors, Nat. Rev. Microbiol., 2019, 17, 295–306 CrossRef CAS PubMed.
- D. Yahav, C. G. Giske, A. Gramatniece, H. Abodakpi, V. H. Tam and L. Leibovici, New beta-Lactam-beta-Lactamase Inhibitor Combinations, Clin. Microbiol. Rev., 2020, 34, e00115–e00120 CrossRef PubMed.
- M. S. Ramirez and M. E. Tolmasky, Aminoglycoside modifying enzymes, Drug Resistance Updates, 2010, 13, 151–171 CrossRef CAS PubMed.
- B. O. Costa, M. H. Cardoso and O. L. Franco, Development of Peptides that Inhibit Aminoglycoside-Modifying Enzymes and beta-Lactamases for Control of Resistant Bacteria, Curr. Protein Pept. Sci., 2020, 21, 1011–1026 CrossRef CAS PubMed.
- G. C. Sati, V. A. Sarpe, T. Furukawa, S. Mondal, M. Mantovani, S. N. Hobbie, A. Vasella, E. C. Bottger and D. Crich, Modification at the 2'-Position of the 4,5-Series of 2-Deoxystreptamine Aminoglycoside Antibiotics To Resist Aminoglycoside Modifying Enzymes and Increase Ribosomal Target Selectivity, ACS Infect. Dis., 2019, 5, 1718–1730 CrossRef CAS PubMed.
- P. Macheboeuf, C. Contreras-Martel, V. Job, O. Dideberg and A. Dessen, Penicillin binding proteins: key players in bacterial cell cycle and drug resistance processes, FEMS Microbiol. Rev., 2006, 30, 673–691 CrossRef CAS PubMed.
- M. W. Shalaby, E. M. E. Dokla, R. A. T. Serya and K. A. M. Abouzid, Penicillin binding protein 2a: An overview and a medicinal chemistry perspective, Eur. J. Med. Chem., 2020, 199, 112312 CrossRef CAS PubMed.
- M. Goel, R. Kalra, P. Ponnan, J. Jayaweera and W. W. Kumbukgolla, Inhibition of penicillin-binding protein 2a (PBP2a) in methicillin resistant Staphylococcus aureus (MRSA) by combination of oxacillin and a bioactive compound from Ramalinaroesleri, Microb. Pathog., 2021, 150, 104676 CrossRef CAS PubMed.
- T. Dominguez-Gil, R. Molina, M. Alcorlo and J. A. Hermoso, Renew or die: The molecular mechanisms of peptidoglycan recycling and antibiotic resistance in Gram-negative pathogens, Drug Resistance Updates, 2016, 28, 91–104 CrossRef PubMed.
- M. A. Boudreau, J. F. Fisher and S. Mobashery, Messenger functions of the bacterial cell wall-derived muropeptides, Biochemistry, 2012, 51, 2974–2990 CrossRef CAS PubMed.
- S. Cho, Q. Wang, C. P. Swaminathan, D. Hesek, M. Lee, G. J. Boons, S. M. Mobashery, S. Mobashery and R. A. Mariuzza, Structural insights into the bactericidal mechanism of human peptidoglycan recognition proteins, Proc. Natl. Acad. Sci. U. S. A., 2007, 104, 8761–8766 CrossRef CAS PubMed.
- K. L. Woodhams, J. M. Chan, J. D. Lenz, K. T. Hackett and J. P. Dillard, Peptidoglycan fragment release from Neisseria meningitidis, Infect. Immun., 2013, 81, 3490–3498 CrossRef CAS PubMed.
- M. Lee, D. Hesek, B. Blazquez, E. Lastochkin, B. Boggess, J. F. Fisher and S. Mobashery, Catalytic spectrum of the penicillin-binding protein 4 of Pseudomonas aeruginosa, a nexus for the induction of beta-lactam antibiotic resistance, J. Am. Chem. Soc., 2015, 137, 190–200 CrossRef CAS PubMed.
- M. Lee, S. Dhar, S. De Benedetti, D. Hesek, B. Boggess, B. Blazquez, K. Mathee and S. Mobashery, Muropeptides in Pseudomonas aeruginosa and their Role as Elicitors of beta-Lactam-Antibiotic Resistance, Angew. Chem., Int. Ed. Engl., 2016, 55, 6882–6886 CrossRef CAS PubMed.
- J. Gisin, A. Schneider, B. Nagele, M. Borisova and C. Mayer, A cell wall recycling shortcut that bypasses peptidoglycan de novo biosynthesis, Nat. Chem. Biol., 2013, 9, 491–493 CrossRef CAS PubMed.
- L. Yi and X. Lu, New Strategy on Antimicrobial-resistance: Inhibitors of DNA Replication Enzymes, Curr. Med. Chem., 2019, 26, 1761–1787 CrossRef CAS PubMed.
- A. Seddek, T. Annamalai and Y. C. Tse-Dinh, Type IA Topoisomerases as Targets for Infectious Disease Treatments, Microorganisms, 2021, 9, 86 CrossRef CAS PubMed.
- D. C. Hooper, Mechanisms of action of antimicrobials: focus on fluoroquinolones, Clin. Infect. Dis., 2001, 15(Suppl 1), S9–S15 CrossRef PubMed.
- S. Rebuffat, Ribosomally synthesized peptides, foreground players in microbial interactions: recent developments and unanswered questions, Nat. Prod. Rep., 2022, 39, 273–310 RSC.
- D. N. Wilson, Ribosome-targeting antibiotics and mechanisms of bacterial resistance, Nat. Rev. Microbiol., 2014, 12, 35–48 CrossRef CAS PubMed.
- J. L. M. Liljeruhm, L. Bao, T. Truu, M. Calvo-Noriega, N. S. Freyer, A. Liiv, J. Wang, R. C. Blanco, R. Ero, J. Remme and A. C. Forster, Plasticity and conditional essentiality of modification enzymes for domain V of Escherichia coli 23S ribosomal RNA, RNA, 2022, 28, 796–807 CrossRef CAS PubMed.
- V. Chandrasekaran, N. Desai, N. O. Burton, H. Yang, J. Price, E. A. Miska and V. Ramakrishnan, Visualizing formation of the active site in the mitochondrial ribosome, Elife, 2021, 10, e68806 CrossRef CAS PubMed.
- F. Prosdocimi, G. S. Zamudio, M. Palacios-Perez and S. M. V. J. Torres de Farias, The Ancient History of Peptidyl Transferase Center Formation as Told by Conservation and Information Analyses, Life, 2020, 10, 134 CrossRef CAS PubMed.
- Z. D. Aron, A. Mehrani, E. D. Hoffer, K. L. Connolly, P. Srinivas, M. C. Torhan, J. N. Alumasa, M. Cabrera, D. Hosangadi, J. S. Barbor, S. C. Cardinale, S. M. Kwasny, L. R. Morin, M. M. Butler, T. J. Opperman, T. L. Bowlin, A. Jerse, S. M. Stagg, C. M. Dunham and K. C. Keiler, trans-Translation inhibitors bind to a novel site on the ribosome and clear Neisseria gonorrhoeae in vivo, Nat. Commun., 2021, 12, 1799 CrossRef CAS PubMed.
- E. Breiner-Goldstein, Z. Eyal, D. Matzov, Y. Halfon, G. Cimicata, M. Baum, A. Rokney, A. V. Ezernitchi, A. N. Lowell, J. J. Schmidt, H. Rozenberg, E. Zimmerman, A. Bashan, L. Valinsky, Y. Anzai, D. H. Sherman and A. Yonath, Ribosome-binding and anti-microbial studies of the mycinamicins, 16-membered macrolide antibiotics from Micromonospora griseorubida, Nucleic Acids Res., 2021, 49, 9560–9573 CrossRef CAS PubMed.
- M. L. Pinel-Marie, R. Brielle, C. Riffaud, N. Germain-Amiot, N. Polacek and B. Felden, RNA antitoxin SprF1 binds ribosomes to attenuate translation and promote persister cell formation in Staphylococcus aureus, Nat. Microbiol., 2021, 6, 209–220 CrossRef CAS PubMed.
- S. Kumar, M. Lekshmi, A. Parvathi, M. Ojha, N. Wenzel and M. F. Varela, Functional and Structural Roles of the Major Facilitator Superfamily Bacterial Multidrug Efflux Pumps, Microorganisms, 2020, 8, 266 CrossRef CAS PubMed.
- L. Vachova, V. Stovicek, O. Hlavacek, O. Chernyavskiy, L. Stepanek, L. Kubinova and Z. Palkova, Flo11p, drug efflux pumps, and the extracellular matrix cooperate to form biofilm yeast colonies, J. Cell Biol., 2011, 194, 679–687 CrossRef CAS PubMed.
- P. Blanco, S. Hernando-Amado, J. A. Reales-Calderon, F. Corona, F. Lira, M. Alcalde-Rico, A. Bernardini, M. B. Sanchez and J. L. Martinez, Bacterial Multidrug Efflux Pumps: Much More Than Antibiotic Resistance Determinants, Microorganisms, 2016, 4, 14 CrossRef PubMed.
- I. T. Paulsen, Multidrug efflux pumps and resistance: regulation and evolution, Curr. Opin. Microbiol., 2003, 6, 446–451 CrossRef CAS PubMed.
- D. Du, X. Wang-Kan, A. Neuberger, H. W. van Veen, K. M. Pos, L. J. V. Piddock and B. F. Luisi, Multidrug efflux pumps: structure, function and regulation, Nat. Rev. Microbiol., 2018, 16, 523–539 CrossRef CAS PubMed.
- J. A. Delmar, C. C. Su and E. W. Yu, Bacterial multidrug efflux transporters, Annu. Rev. Biophys., 2014, 43, 93–117 CrossRef CAS PubMed.
- D. Du, H. W. van Veen and B. F. Luisi, Assembly and operation of bacterial tripartite multidrug efflux pumps, Trends Microbiol., 2015, 23, 311–319 CrossRef CAS PubMed.
- C. R. Lee, J. H. Lee, M. Park, K. S. Park, I. K. Bae, Y. B. Kim, C. J. Cha, B. C. Jeong and S. H. Lee, Biology of Acinetobacter baumannii: Pathogenesis, Antibiotic Resistance Mechanisms, and Prospective Treatment Options, Front. Cell. Infect. Microbiol., 2017, 7, 55 Search PubMed.
- C. O. Vrancianu, I. Gheorghe, I. B. Czobor and M. C. Chifiriuc, Antibiotic Resistance Profiles, Molecular Mechanisms and Innovative Treatment Strategies of Acinetobacter baumannii, Microorganisms, 2020, 8, 935 CrossRef CAS PubMed.
- Y. Smani, A. Fabrega, I. Roca, V. Sanchez-Encinales, J. Vila and J. Pachon, Role of OmpA in the multidrug resistance phenotype of Acinetobacter baumannii, Antimicrob. Agents Chemother., 2014, 58, 1806–1808 CrossRef PubMed.
- P. Espinal, A. Pantel, D. Rolo, S. Marti, R. Lopez-Rojas, Y. Smani, J. Pachon, J. Vila and J. P. Lavigne, Relationship Between Different Resistance Mechanisms and Virulence in Acinetobacter baumannii, Microb. Drug Resist., 2019, 25, 752–760 CrossRef CAS PubMed.
- Y. Sato, Y. Unno, S. Kawakami, T. Ubagai and Y. Ono, Virulence characteristics of Acinetobacter baumannii clinical isolates vary with the expression levels of omps, J. Med. Microbiol., 2017, 66, 203–212 CrossRef PubMed.
- A. L. Harvey, R. Edrada-Ebel and R. J. Quinn, The re-emergence of natural products for drug discovery in the genomics era, Nat. Rev. Drug Discov., 2015, 14, 111–129 CrossRef CAS PubMed.
- W.-H. Jesse and J. C. V. Li, Drug discovery and natural products: end of an era or an endless frontier?, Science, 2009, 325, 161–165 CrossRef PubMed.
- Z. Guo, Y. Tang, W. Tang and Y. Chen, Heptose-containing bacterial natural products: structures, bioactivities, and biosyntheses, Nat. Prod. Rep., 2021, 38, 1887–1909 RSC.
- L. Zhang, J. Song, L. Kong, T. Yuan, W. Li, W. Zhang, B. Hou, Y. Lu and G. Du, The strategies and techniques of drug discovery from natural products, Pharmacol. Ther., 2020, 216, 107686 CrossRef CAS PubMed.
- N. F. Shamsudin, Q. U. Ahmed, S. Mahmood, S. A. Ali Shah, A. Khatib, S. Mukhtar, M. A. Alsharif, H. Parveen and Z. A. Zakaria, Antibacterial Effects of Flavonoids and Their Structure-Activity Relationship Study: A Comparative Interpretation, Molecules, 2022, 27, 1149 CrossRef CAS PubMed.
- S. Lin, J. D. Wade and S. Liu, De Novo Design of Flavonoid-Based Mimetics of Cationic Antimicrobial Peptides: Discovery, Development, and Applications, Acc. Chem. Res., 2020, 54, 104–119 CrossRef PubMed.
- A. T. Rufino, V. M. Costa, F. Carvalho and E. Fernandes, Flavonoids as antiobesity agents: A review, Med. Res. Rev., 2021, 41, 556–585 CrossRef CAS PubMed.
- V. Vukics and A. Guttman, Structural characterization of flavonoid glycosides by multi-stage mass spectrometry, Mass Spectrom. Rev., 2010, 29, 1–16 CrossRef CAS PubMed.
- L. Pourcel, J. Routaboul, V. Cheynier, L. Lepiniec and I. Debeaujon, Flavonoid oxidation in plants: from biochemical properties to physiological functions, Trends Plant Sci., 2007, 12, 29–36 CrossRef CAS PubMed.
- Z. Wang, Z. Ding, Z. Li, Y. Ding, F. Jiang and J. Liu, Antioxidant and antibacterial study of 10 flavonoids revealed rutin as a potential antibiofilm agent in Klebsiella pneumoniae strains isolated from hospitalized patients, Microb. Pathog., 2021, 159, 105121 CrossRef CAS PubMed.
- C. J. Raorane, J.-H. Lee, Y.-G. Kim, S. K. Rajasekharan, R. García-Contreras and J. Lee, Antibiofilm and Antivirulence Efficacies of Flavonoids and Curcumin Against Acinetobacter baumannii, Front. Microbiol., 2019, 10, 990 CrossRef PubMed.
- W. B. Jonathan and D. W. W., In vitro activity of curcumin in combination with epigallocatechin gallate (EGCG) versus multidrug-resistant Acinetobacter baumannii, BMC Microbiol., 2014, 14, 172 CrossRef PubMed.
- M. Song, Y. Liu, T. Li, X. Liu, Z. Hao, S. Ding, P. Panichayupakaranant, K. Zhu and J. Shen, Plant Natural Flavonoids Against Multidrug Resistant Pathogens, Adv. Sci., 2021, 8, e2100749 CrossRef PubMed.
- B. J. Denny, P. A. Lambert and P. W. West, The flavonoid galangin inhibits the L1 metallo-beta-lactamase from Stenotrophomonas maltophilia, FEMS Microbiol.
Lett., 2002, 208, 21–24 CAS.
- A. Pal and A. Tripathi, Quercetin inhibits carbapenemase and efflux pump activities among carbapenem-resistant Gram-negative bacteria, APMIS, 2020, 128, 251–259 CrossRef CAS PubMed.
- S. Das, S. Batra, P. P. Gupta, M. Kumar, V. K. Srivastava, A. Jyoti, N. Singh and S. Kaushik, Identification and evaluation of quercetin as a potential inhibitor of naphthoate synthase fromEnterococcus faecalis, J. Mol. Recognit., 2019, 32, e2802 CAS.
- A. M. Hossion, Y. Zamami, R. K. Kandahary, T. Tsuchiya, W. Ogawa, A. Iwado and K. Sasaki, Quercetin diacylglycoside analogues showing dual inhibition of DNA gyrase and topoisomerase IV as novel antibacterial agents, J. Med. Chem., 2011, 54, 3686–3703 CrossRef CAS PubMed.
- S. Qu, C. Dai, Z. Shen, Q. Tang, H. Wang, B. Zhai, L. Zhao and Z. Hao, Mechanism of Synergy Between Tetracycline and Quercetin Against Antibiotic Resistant Escherichia coli, Front. Microbiol., 2019, 10, 2536 CrossRef PubMed.
- J. S. Yu, J. H. Kim, L. Rashan, I. Kim, W. Lee and K. H. Kim, Potential Antimicrobial Activity of Galloyl-Flavonoid Glycosides From Woodfordia uniflora Against Methicillin-Resistant Staphylococcus aureus, Front. Microbiol., 2021, 12, 784504 CrossRef PubMed.
- V. Kuete, B. Ngameni, J. G. Tangmouo, J. M. Bolla, S. Alibert-Franco, B. T. Ngadjui and J. M. Pages, Efflux pumps are involved in the defense of Gram-negative bacteria against the natural products isobavachalcone and diospyrone, Antimicrob. Agents Chemother., 2010, 54, 1749–1752 CrossRef CAS PubMed.
- W. Sianglum, K. Muangngam, N. Joycharat and S. P. Voravuthikunchai, Mechanism of Action and Biofilm Inhibitory Activity of Lupinifolin Against Multidrug-Resistant Enterococcal Clinical Isolates, Microb. Drug Resist., 2019, 25, 1391–1400 CrossRef CAS PubMed.
- J. Wang, R. Yang, Z. Xiao, Q. Xu, P. Li and F. Ma, Dihydrochalcones in Malus inhibit bacterial growth by reducing cell membrane integrity, Food Funct., 2020, 11, 6517–6527 RSC.
- D. Barreca, E. Bellocco, G. Lagana, G. Ginestra and C. Bisignano, Biochemical and antimicrobial activity of phloretin and its glycosilated derivatives present in apple and kumquat, Food Chem., 2014, 160, 292–297 CrossRef CAS PubMed.
- R. Du, Q. Lv, W. Hu, X. Hou, Y. Zhou, X. Deng, L. Sun, L. Li, Y. Deng and J. Wang, Phloretin potentiates polymyxin E activity against gram-negative bacteria, Life Sci., 2021, 287, 120085 CrossRef CAS PubMed.
- S. Vasudevan, G. Thamil Selvan, S. Bhaskaran, N. Hari and A. P. Solomon, Reciprocal Cooperation of Type A Procyanidin and Nitrofurantoin Against Multi-Drug Resistant (MDR) UPEC: A pH-Dependent Study, Front. Cell. Infect. Microbiol., 2020, 10, 421 CrossRef CAS PubMed.
- S. Farooq, A. T. Wahab, C. D. Fozing, A. U. Rahman and M. I. Choudhary, Artonin I inhibits multidrug resistance in Staphylococcus aureus and potentiates the action of inactive antibiotics in vitro, J. Appl. Microbiol., 2014, 117, 996–1011 CrossRef CAS PubMed.
- C. A. N. Ferraz, S. R. Tintino, A. M. R. Teixeira, P. N. Bandeira, H. S. Santos, B. G. Cruz, C. E. S. Nogueira, T. F. Moura, R. L. S. Pereira, D. M. Sena, Jr., T. S. Freitas, J. E. Rocha and H. D. M. Coutinho, Potentiation of antibiotic activity by chalcone (E)-1-(4'-aminophenyl)-3-(furan-2-yl)-prop-2-en-1-one against gram-positive and gram-negative MDR strains, Microb. Pathog., 2020, 148, 104453 CrossRef CAS PubMed.
- J. K. Dzotam, I. K. Simo, G. Bitchagno, I. Celik, L. P. Sandjo, P. Tane and V. Kuete, In vitro antibacterial
and antibiotic modifying activity of crude extract, fractions and 3',4',7-trihydroxyflavone from Myristica fragrans Houtt against MDR Gram-negative enteric bacteria, BMC Complementary Altern. Med., 2018, 18, 15 CrossRef PubMed.
- F. Taleghani, G. Rezvani, M. Birjandi and M. Valizadeh, Impact of green tea intake on clinical improvement in chronic periodontitis: A randomized clinical trial, J. Stomatol., Oral Maxillofac. Surg., 2018, 119, 365–368 CrossRef CAS PubMed.
- C. Astill, M. R. Birch, C. Dacombe, P. G. Humphrey and P. T. Martin, Factors affecting the caffeine and polyphenol contents of black and green tea infusions, J. Agric. Food Chem., 2001, 49, 5340–5347 CrossRef CAS PubMed.
- M. Radji, R. A. Agustama, B. Elya and C. R. Tjampakasari, Antimicrobial activity of green tea extract against isolates of methicillin–resistant Staphylococcus aureus and multi–drug resistant Pseudomonas aeruginosa, Asian Pac. J. Trop. Biomed., 2013, 3, 663–667 CrossRef CAS PubMed.
- E. D. Pauli, I. S. Scarminio and R. Tauler, Analytical investigation of secondary metabolites extracted from Camellia sinensis L. leaves using a HPLC-DAD-ESI/MS data fusion strategy and chemometric methods, J. Chemom., 2016, 30, 75–85 CrossRef CAS.
- L. H. Yao, Y. M. Jiang, N. Caffin, B. D’Arcy, N. Datta, X. Liu, R. Singanusong and Y. Xu, Phenolic compounds in tea from Australian supermarkets, Food Chem., 2006, 96, 614–620 CrossRef CAS.
- Y. S. Cho, N. L. Schiller and K. H. Oh, Antibacterial effects of green tea polyphenols on clinical isolates of methicillin-resistant Staphylococcus aureus, Curr. Microbiol., 2008, 57, 542–546 CrossRef CAS PubMed.
- T. S. S. S. Yam and J. M. Hamilton-Miller, Microbiological activity of whole and fractionated crude extracts of tea (Camellia sinensis), and of tea components, FEMS Microbiol. Lett., 1997, 152, 169–174 CrossRef CAS PubMed.
- P. D. Stapleton, S. Shah, J. C. Anderson, Y. Hara, J. M. Hamilton-Miller and P. W. Taylor, Modulation of beta-lactam resistance in Staphylococcus aureus by catechins and gallates, Int. J. Antimicrob. Agents, 2004, 23, 462–467 CrossRef CAS PubMed.
- P. D. Stapleton, S. Shah, J. M. Hamilton-Miller, Y. Hara, Y. Nagaoka, A. Kumagai, S. Uesato and P. W. Taylor, Anti-Staphylococcus aureus activity and oxacillin resistance modulating capacity of 3-O-acyl-catechins, Int. J. Antimicrob. Agents, 2004, 24, 374–380 CrossRef CAS PubMed.
- M. Kurincic, A. Klancnik and S. Smole Mozina, Effects of efflux pump inhibitors on erythromycin, ciprofloxacin, and tetracycline resistance in Campylobacter spp. isolates, Microb. Drug Resist., 2012, 18, 492–501 CrossRef CAS PubMed.
- R. Gaur and G. H. Bao, Chemistry and Pharmacology of Natural Catechins from Camellia sinensis as Anti-MRSA Agents, Curr. Top. Med. Chem., 2021, 21, 1519–1537 CrossRef CAS PubMed.
- L. Wang, Q. Li, J. Li, S. Jing, Y. Jin, L. Yang, H. Yu, D. Wang, T. Wang and L. Wang, Eriodictyol as a Potential Candidate Inhibitor of Sortase A Protects Mice From Methicillin-Resistant Staphylococcus aureus-Induced Pneumonia, Front. Microbiol., 2021, 12, 635710 CrossRef PubMed.
- W. Qian, W. Wang, J. Zhang, T. Wang, M. Liu, M. Yang, Z. Sun, X. Li and Y. Li, Antimicrobial and antibiofilm activities of ursolic acid against carbapenem-resistant Klebsiella pneumoniae, J. Antibiot., 2020, 73, 382–391 CrossRef CAS PubMed.
- I. Macedo, J. H. da Silva, P. T. da Silva, B. G. Cruz, J. P. C. do Vale, H. S. Dos Santos, P. N. Bandeira, E. B. de Souza, M. R. Xavier, H. D. M. Coutinho, R. Braz-Filho and A. M. R. Teixeira, Structural and Microbiological Characterization of 5-Hydroxy-3,7,4'-Trimethoxyflavone: A Flavonoid Isolated from Vitex gardneriana Schauer Leaves, Microb. Drug Resist., 2019, 25, 434–438 CrossRef CAS PubMed.
- Z. Cao, P. Yang and Q. Zhou, Multiple biological functions and pharmacological effects
of lycorine, Sci. China: Chem., 2013, 56, 1382–1391 CrossRef CAS PubMed.
- N. T. Son and A. I. Elshamy, Flavonoids and other Non-alkaloidal Constituents of Genus Erythrina: Phytochemical Review, Comb. Chem. High Throughput Screening, 2021, 24, 20–58 CrossRef CAS PubMed.
- S. Lopez-Molina, C. Galiana-Rosello, C. Galiana, A. Gil-Martinez, S. Bandeira and J. Gonzalez-Garcia, Alkaloids as Photosensitisers for the Inactivation of Bacteria, Antibiotics, 2021, 10, 1505 CrossRef CAS PubMed.
- W. T. Beaulieu, D. G. Panaccione, Q. N. Quach, K. L. Smoot and K. Clay, Diversification of ergot alkaloids and heritable fungal symbionts in morning glories, Commun. Biol., 2021, 4, 1362 CrossRef PubMed.
- J. T. Wibowo, P. Ahmadi, S. I. Rahmawati, A. Bayu, M. Y. Putra and A. Kijjoa, Marine-Derived Indole Alkaloids and Their Biological and Pharmacological Activities, Mar. Drugs, 2021, 20, 3 CrossRef PubMed.
- F. Barbosa, E. Pinto, A. Kijjoa, M. Pinto and E. Sousa, Targeting antimicrobial drug resistance with marine natural products, Int. J. Antimicrob. Agents, 2020, 56, 106005 CrossRef CAS PubMed.
- K. I.-N. Karen Kubo, S. Ohnuki, Y. Yashiroda, S. C. Li, H. Kimura, Y. Kawamura, Y. Shimamoto, K. Tominaga, D. Yamanaka, Y. Adachi, S. Takashima, Y. Noda, C. Boone and Y. Ohya, Jerveratrum-Type Steroidal Alkaloids Inhibit b-1,6-Glucan Biosynthesis in Fungal Cell Walls, Microbiol. Spectrum, 2022, 10, e0087321 CrossRef PubMed.
- Y. S. Yang, X. Lu, Q. X. Zeng, J. Pang, T. Y. Fan, X. F. You, S. Tang, Y. X. Wang and D. Q. Song, Synthesis and biological evaluation of 7-substituted cycloberberine derivatives as potent antibacterial agents against MRSA, Eur. J. Med. Chem., 2019, 168, 283–292 CrossRef CAS PubMed.
- J. K. Y. Yap, S. Y. Y. Tan, S. Q. Tang, V. K. Thien and E. W. L. Chan, Synergistic Antibacterial Activity Between 1,4-Naphthoquinone and beta-Lactam Antibiotics Against Methicillin-Resistant Staphylococcus aureus, Microb. Drug Resist., 2021, 27, 234–240 CrossRef CAS PubMed.
- M. Wang, B. Ma, Y. Ni, X. Xue, M. Li, J. Meng, X. Luo, C. Fang and Z. Hou, Restoration of the Antibiotic Susceptibility of Methicillin-Resistant Staphylococcus aureus and Extended-Spectrum beta-Lactamases Escherichia coli Through Combination with Chelerythrine, Microb. Drug Resist., 2021, 27, 337–341 CrossRef CAS PubMed.
- W.-D. Qian, J. Huang, J.-N. Zhang, X.-C. Li, Y. Kong, T. Wang and Y.-D. Li, Antimicrobial and Antibiofilm Activities and Mechanism of Action of Chelerythrine Against Carbapenem-Resistant Serratia marcescens In Vitro, Microb. Drug Resist., 2021, 27, 1105–1116 CrossRef CAS PubMed.
- X. Li, Y. Song, L. Wang, G. Kang, P. Wang, H. Yin and H. Huang, A Potential Combination Therapy of Berberine Hydrochloride With Antibiotics Against Multidrug-Resistant Acinetobacter baumannii, Front. Cell. Infect. Microbiol., 2021, 11, 660431 CrossRef CAS PubMed.
- G. B. Manjunath, S. P. Awasthi, M. S. H. Zahid, N. Hatanaka, A. Hinenoya, E. Iwaoka, S. Aoki, T. Ramamurthy and S. Yamasaki, Piperine, an active ingredient of white pepper, suppresses the growth of multidrug-resistant toxigenic Vibrio cholerae and other pathogenic bacteria, Lett. Appl. Microbiol., 2022, 74, 472–481 CrossRef CAS PubMed.
- K. Alhanout, S. Malesinki, N. Vidal, V. Peyrot, J. M. Rolain and J. M. Brunel, New insights into the antibacterial mechanism of action of squalamine, J. Antimicrob. Chemother., 2010, 65, 1688–1693 CrossRef CAS PubMed.
- K. Tushar, M. K. S. Beuria and P. Dulal, Sanguinarine blocks cytokinesis in bacteria
by inhibiting FtsZ assembly and bundling, Biochemistry, 2005, 44, 16584–16593 CrossRef PubMed.
- D. Crncevic, L. Krce, L. Mastelic, A. Maravic, B. Soldo, I. Aviani, I. Primozic, R. Odzak and M. Sprung, The mode of antibacterial action of quaternary N-benzylimidazole salts against emerging opportunistic pathogens, Bioorg. Chem., 2021, 112, 104938 CrossRef CAS PubMed.
- L. Z. M. Kamal, M. A. A. Adam, S. N. M. Shahpudin, A. N. Shuib, R. Sandai, N. M. Hassan, Y. Tabana, D. F. Basri, L. T. L. Than and D. Sandai, Identification of Alkaloid Compounds Arborinine and Graveoline from Ruta angustifolia (L.) Pers for their Antifungal Potential against Isocitrate lyase (ICL1) gene of Candida albicans, Mycopathologia, 2021, 186, 221–236 CrossRef CAS PubMed.
- H. Tian, Q.-J. Liu, J.-T. Wang and L. Zhang, Antimicrobial crinane-type alkaloids from the bulbs of Crinum latifolium, J. Asian Nat. Prod. Res., 2020, 23, 1023–1029 CrossRef PubMed.
- G. R. Dwivedi, R. Tyagi, Sanchita, S. Tripathi, S. Pati, S. K. Srivastava, M. P. Darokar and A. Sharma, Antibiotics potentiating potential of catharanthine against superbug Pseudomonas aeruginosa, J. Biomol. Struct. Dyn., 2018, 36, 4270–4284 CrossRef CAS PubMed.
- R. Amber, M. Adnan, A. Tariq, S. N. Khan, S. Mussarat, A. Hashem, A. A. Al-Huqail, A. F. Al-Arjani and E. F. Abd Allah, Antibacterial activity of selected medicinal plants of northwest Pakistan traditionally used against mastitis in livestock, Saudi J. Biol. Sci., 2018, 25, 154–161 CrossRef PubMed.
- G. R. Dwivedi, A. Maurya, D. K. Yadav, V. Singh, F. Khan, M. K. Gupta, M. Singh, M. P. Darokar and S. K. Srivastava, Synergy of clavine alkaloid 'chanoclavine' with tetracycline against multi-drug-resistant E. coli, J. Biomol. Struct. Dyn., 2019, 37, 1307–1325 CrossRef CAS PubMed.
- M. M. Rahman, M. S. Rahaman, M. R. Islam, M. E. Hossain, F. Mannan Mithi, M. Ahmed, M. Saldias, E. K. Akkol and E. Sobarzo-Sanchez, Multifunctional Therapeutic Potential of Phytocomplexes and Natural Extracts for Antimicrobial Properties, Antibiotics (Basel), 2021, 10, 1076 CrossRef CAS PubMed.
- N. Jubair, M. Rajagopal, S. Chinnappan, N. B. Abdullah, A. Fatima and A. Zarrelli, Review on the Antibacterial Mechanism of Plant-Derived Compounds against Multidrug-Resistant Bacteria (MDR), J. Evidence-Based Complementary Altern. Med., 2021, 2021, 1–30 CrossRef PubMed.
- A. C. Justino de Araujo, P. R. Freitas, C. Rodrigues Dos Santos Barbosa, D. F. Muniz, J. E. Rocha, A. C. Albuquerque da Silva, C. Datiane de Morais Oliveira-Tintino, J. Ribeiro-Filho, L. Everson da Silva, C. Confortin, W. D. Amaral, C. Deschamps, J. M. Barbosa-Filho, N. T. Ramos de Lima, S. R. Tintino and H. D. Melo Coutinho, GC-MS-FID characterization and antibacterial activity of the Mikania cordifolia essential oil and limonene against MDR strains, Food Chem. Toxicol., 2020, 136, 111023 CrossRef CAS PubMed.
- A. Gupta, E. Jeyakumar and R. Lawrence, Strategic approach of multifaceted antibacterial mechanism of limonene traced in Escherichia coli, Sci. Rep., 2021, 11, 13816 CrossRef CAS PubMed.
- C. R. Dos Santos Barbosa, J. R. Scherf, T. S. de Freitas, I. R. A. de Menezes, R. L. S. Pereira, J. F. S. Dos Santos, S. S. P. de Jesus, T. P. Lopes, Z. de Sousa Silveira, C. D. de Morais Oliveira-Tintino, J. P. S. Junior, H. D. M. Coutinho, S. R. Tintino and F. A. B. da Cunha, Effect of Carvacrol and Thymol on NorA efflux pump inhibition in multidrug-resistant (MDR) Staphylococcus aureus strains, J. Bioenerg. Biomembr., 2021, 53, 489–498 CrossRef CAS PubMed.
- A. Martins, A. Vasas, M. Viveiros, J. Molnar, J. Hohmann and L. Amaral, Antibacterial properties of compounds isolated from Carpobrotus edulis, Int. J. Antimicrob. Agents, 2011, 37, 438–444 CrossRef CAS PubMed.
- F. Hosseinkhani, F. Jabalameli, M. Banar, N. Abdellahi, M. Taherikalani, W. B. Leeuwen and M. Emaneini, Monoterpene isolated from the essential oil of Trachyspermum ammi is cytotoxic to multidrug-resistant Pseudomonas aeruginosa and Staphylococcus aureus strains, Rev Soc Bras Med Trop, 2016, 49, 172–176 CrossRef PubMed.
- V. K. Gupta, N. Tiwari, P. Gupta, S. Verma, A. Pal, S. K. Srivastava and M. P. Darokar, A clerodane diterpene from Polyalthia longifolia as a modifying agent of the resistance of methicillin resistant Staphylococcus aureus, Phytomedicine, 2016, 23, 654–661 CrossRef CAS PubMed.
- S. Singh, Z. Fatima and S. Hameed, Insights into the mode of action of anticandidal herbal monoterpenoid geraniol reveal disruption of multiple MDR mechanisms and virulence attributes in Candida albicans, Arch. Microbiol., 2016, 198, 459–472 CrossRef CAS PubMed.
- L. C. Rosario Rojas, C. A. José, J. V. Abraham, H. L. Walter, G. Lamas., C. Sarasara., H. G. Robert and B. H. Gerald, Aegicerin, the first oleanane triterpene with wide-ranging antimycobacterial activity, isolated from Clavija procera, J. Nat. Prod., 2006, 69, 845–846 CrossRef PubMed.
- S. Wei, Y. Yang, W. Tian, M. Liu, S. Yin and J. Li, Synergistic Activity of Fluoroquinolones Combining with Artesunate Against Multidrug-Resistant Escherichia coli, Microb. Drug Resist., 2020, 26, 81–88 CrossRef CAS PubMed.
- L. D. Hazlett, S. A. Ekanayaka, S. A. McClellan and R. Francis, Glycyrrhizin Use for Multi-Drug Resistant Pseudomonas aeruginosa: In Vitro and In Vivo Studies, Investig. Ophthalmol. Vis. Sci., 2019, 60, 2978–2989 CrossRef CAS PubMed.
- M. F. S. Patrícia Rijo, A. P. Francisco., R. Rojas., H. G. Robert, J. V. Abraham, B. Rodríguez. and C. Moiteiro., Antimycobacterial metabolites from Plectranthus: royleanone derivatives against Mycobacterium tuberculosis strains, Chem. Biodiversity, 2010, 7, 922–932 CrossRef PubMed.
- S. S. Swain, S. K. Paidesetty and R. N. Padhy, Development of antibacterial conjugates using sulfamethoxazole with monocyclic terpenes: A systematic medicinal chemistry based computational approach, Comput. Methods Programs Biomed., 2017, 140, 185–194 CrossRef PubMed.
- L. H. Yu, X. Wei, M. Ma, X. J. Chen and S. B. Xu, Possible inhibitory molecular mechanism of farnesol on the development of fluconazole resistance in Candida albicans biofilm, Antimicrob. Agents Chemother., 2012, 56, 770–775 CrossRef CAS PubMed.
- L. Cernakova, S. Dizova, D. Gaskova, I. Jancikova and H. Bujdakova, Impact of Farnesol as a Modulator of Efflux Pumps in a Fluconazole-Resistant Strain of Candida albicans, Microb. Drug Resist., 2019, 25, 805–812 CrossRef CAS PubMed.
- A. Sharma, A. Biharee, A. Kumar and V. Jaitak, Antimicrobial Terpenoids as a Potential Substitute in Overcoming Antimicrobial Resistance, Curr. Drug Targets, 2020, 21, 1476–1494 CrossRef CAS PubMed.
- H. Siddique, B. Pendry and M. M. Rahman, Terpenes from Zingiber montanum and Their Screening against Multi-Drug Resistant and Methicillin Resistant Staphylococcus aureus, Molecules, 2019, 24, 385 CrossRef PubMed.
- K. Oughlissi-Dehak, P. Lawton, S. Michalet, C. Bayet, N. Darbour, M. Hadj-Mahammed, Y. A. Badjah-Hadj-Ahmed, M. G. Dijoux-Franca and D. Guilet, Sesquiterpenes from aerial parts of Ferula vesceritensis, Phytochemistry, 2008, 69, 1933–1938 CrossRef CAS PubMed.
- N. M. Vazquez, F. Mariani, P. S. Torres, S. Moreno and E. M. Galvan, Cell death and biomass reduction in biofilms of multidrug resistant extended spectrum beta-lactamase-producing uropathogenic Escherichia coli isolates by 1,8-cineole, PLoS One, 2020, 15, e0241978 CrossRef CAS PubMed.
- H. C. de Carvalho, A. L. Ieque, T. L. Valverde, V. P. Baldin, J. E. Meneguello, P. A. Z. Campanerut-Sa, F. Vandresen, L. D. Ghiraldi Lopes, M. R. Passos Souza, N. C. S. Santos, V. L. Dias Siqueira, K. R. Caleffi-Ferracioli, R. B. Lima Scodro and R. F. Cardoso, Activity of (-)-Camphene Derivatives Against Mycobacterium tuberculosis in Acidic pH, Med. Chem., 2021, 17, 485–492 CrossRef CAS PubMed.
- V. L. Elodie Guinoiseau, L. Anne, T. Félix, C. Joseph and L. Berti., Susceptibility of the multi-drug resistant strain of Enterobacter aerogenes EA289 to the terpene alcohols from Cistus ladaniferus essential oil, Nat. Prod. Commun., 2011, 6, 1159–1162 Search PubMed.
- P. Knezevic, V. Aleksic, N. Simin, E. Svircev, A. Petrovic and N. Mimica-Dukic, Antimicrobial activity of Eucalyptus camaldulensis essential oils and their interactions with conventional antimicrobial agents against multi-drug resistant Acinetobacter baumannii, J. Ethnopharmacol., 2016, 178, 125–136 CrossRef CAS PubMed.
Footnote |
† These authors contributed equally to this work. |
|
This journal is © The Royal Society of Chemistry 2023 |