DOI:
10.1039/D2RA05384E
(Review Article)
RSC Adv., 2022,
12, 32744-32755
Optical chemosensors for environmental monitoring of toxic metals related to Alzheimer's disease†
Received
27th August 2022
, Accepted 7th November 2022
First published on 15th November 2022
Abstract
Alzheimer's disease (AD) is the most common type of dementia and progresses from mild memory loss to severe decline in thinking, behavioral and social skills, which dramatically impairs a person's ability to function independently. Genetics, some health disorders and lifestyle have all been connected to AD. Also, environmental factors are reported as contributors to this illness. The presence of heavy metals in air, water, food, soil and commercial products has increased tremendously. Accumulation of heavy metals in the body leads to serious malfunctioning of bodily organs, specifically the brain. For AD, a wide range of heavy metals have been reported to contribute to its onset and progression and the manifestation of its hallmarks. In this review, we focus on detection of highly toxic heavy metals such as mercury, cadmium, lead and arsenic in water. The presence of heavy metals in water is very troubling and regular monitoring is warranted. Optical chemosensors were designed and fabricated for determination of ultra-trace quantities of heavy metals in water. They have shown advantages when compared to other sensors, such as selectivity, low-detection limit, fast response time, and wide-range determination under optimal sensing conditions. Therefore, implementing optical chemosensors for monitoring levels of toxic metals in water represents an important contribution in fighting AD.
1. Introduction
Dementia, a neurodegenerative disorder, is characterized by loss of memory, language and problem-solving along with other thinking abilities, which can interfere with a patient's daily life. Dementia can be manifested in different formats such as Alzheimer's Disease, vascular dementia, and Lewy body dementia.1 Alzheimer's disease (AD) is known to be the most common form of dementia where according to the WHO it represents 60–70% of dementia cases worldwide.2 Although AD is commonly diagnosed in aged societies that are older than 65 years old, some cases are considered “early-onset” where symptoms are witnessed as early as 30 years old. For diagnosis, AD is known to have very distinct hallmarks represented in the intracellular neurofibrillary tangles (NFTs) containing the protein tau in a hyper-phosphorylated state and the extracellular plaques containing amyloid beta (Aβ).3–6 Regardless the distinctive diagnostic markers for AD, unfortunately, the causes behind AD are not quite understood up till now. As such, studying the etiology of AD has been quite a challenging task where it resulted in declaring AD as a multifactorial disorder. Generally, the increase in the incidence of AD along with its progression has been attributed to genetics, environmental factors, and acquired factors such as cerebrovascular diseases, stress, anxiety, and sleep disorders. It can be quite understandable that some risk factors are hardly controllable, still, identifying the controllable ones has become paramount to reduce the AD progression.7,8 Latest observations have identified environmental risk factors as key causal players in the progression and onset of AD. Those factors including air pollution, poor diet, contaminated water, and infections can participate in developing AD by initiating oxidative stress and inflammation.9,10 Among the well-known environmental risk factors is the prolonged exposure to heavy metals especially those of industrial origin. Such pollutants can be released in the air as fine dust or in water as well as the soil. Those heavy metals have the capacity to initiate the formation of Aβ plagues and the phosphorylation of the tau protein; thereof, neuronal cell death is a definite outcome.11 In this review, we give a special attention to those heavy metals and how to detect them in water, in hope that these measures act as a protective shield against AD.
2. Heavy metals and Alzheimer's disease
Heavy metal poisoning is an anticipated consequence for the excessive exposure to heavy metals through many routes such as air, water, improperly coated food containers, and industrial exposure. While all those routes are critical, water contamination can be of the highest incidence as well as the largest impact. Accordingly, understanding the water contamination and the available techniques for its detection is essential. Although many heavy metals such as lead (Pb), mercury (Hg), cadmium (Cd), chromium (Cr), copper (Cu), zinc (Zn), iron (Fe), manganese (Mn), and arsenic (As) have shown some contribution to AD,12,13 we herein focus on the highly toxic ones i.e. Hg, Cd, Pb, As (Fig. 1). Upon accumulation in the body, these metals contribute to the AD progression as illustrated below. Various agencies and organizations such as the World Health Organization (WHO), European Union (EU), and the US Environmental Protection Agency (US EPA) have established drinking water guidelines for heavy metals in water (Table S1†).
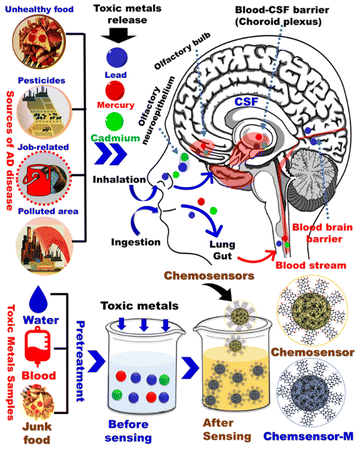 |
| Fig. 1 General schematic diagram of common environmental factors associated with Alzheimer's disease. | |
2.1. Mercury
Mercury (Hg) can exist in elemental state, ionic state, and organic form (methyl mercury). Mercury is introduced into the environment as a result of volcanic emissions or industrial activities. Since the industrial revolution, the amount of mercury in both air and water has increased by 3–5 folds.14–16 Hg is a potent neurotoxin that causes cognitive and motion disorders. It has been shown to affect AD onset and progression via multiple routes. Mercury was reported to (a) stimulate the formation of beta-amyloid protein causing senile plaques;17–19 (b) increase the phosphorylation of tau protein resulting in NFTs;20 (c) inhibit glutamate uptake and stimulate its release causing neurodegeneration in AD;21 and (d) interfere with some enzymes related to AD such as beta-amyloid cleaving enzyme BACE-1, monoamine oxidase, and acetylcholine transferase.22–24 Surprisingly, Hg was also linked to the genetic component of AD where it causes presenilin1 gene mutation which is linked to 30% of early-onset AD cases.25 Also, research on rat model has shown that neuronal beta-tubulin defects may be induced by inorganic mercury proposing that Hg may interfere with the assembly of microtubules from tubulin that eventually leads to plaques.26,27 In humans, Hg concentration in the plasma of AD patients was almost twice the values from age matched controls.28 Also, workers exposed to Hg vapors for long time, showed signs of cognitive deficits.29,30 Finally, studies on brain autopsies of AD patients showed Hg deposition in the hippocampus and amygdala, which are involved in memory and other cognitive functions.31,32
2.2. Cadmium
Cadmium (Cd) is a toxic water-soluble metal characterized by a long half-life in humans where it accumulates in the body, especially in the kidneys, liver, lung, and brain and can induce several toxic effects, depending on its concentration and exposure time. Cd is a carcinogen with a certain ability to cross the blood–brain barrier and has been linked to neurological diseases such as AD. Cd pollution of water, air, soil and food arises from cigarette smoking and several industries such as nickel–cadmium batteries, pigments, plastic stabilizers, and metal refineries.33–35 Several studies reported the interference of Cd with some key factors related to AD. Cd is able to interact with beta amyloid proteins inducing their aggregation which is a distinctive hallmark for the pathogenesis of AD.33,36,37 It also downregulates the expression of α-secretase (ADAM10) and neutral endopeptidase which play important roles in reducing Aβ levels in the brain.38 Cd may be involved in self-aggregation of tau in the AD brain and selectively blocks M1 receptors that help in the negative regulation of GSK-3β (an enzyme when overexpressed increase both total and phosphorylated tau protein).39,40 Additionally, Cd was reported to activate mitogen-activated protein kinase (MAPK) and NF-κB signaling pathways which elevate the levels of IL-6 and IL-8 that are associated with AD pathogenesis.41,42 Unfortunately, there has been difficulty in assessing the effects of Cd in vivo or in AD patients, since metal binding proteins, stress proteins and antioxidants may modulate the toxicity of the element. Still, in an in vivo study, APP/PS1 transgenic mice which consumed to drinking water contaminated with Cd exhibited an increase in the number and size of plaques in their hippocampus and cerebral cortex.43 Also, recent studies reported that blood cadmium levels were significantly associated with AD-related mortality among older adults.44,45
2.3. Lead
Humans are exposed to lead (Pb), a significant toxic metal, through ingestion of contaminated food and water or inhalation of polluted air. Pb pollution can be caused by lead batteries, paints, electronic waste and smelting operations.46 The presence of Pb in the blood affects many organs but the CNS is considered the most vulnerable. Pb can compete with the binding site of metals such as calcium leading to biometal dyshomeostasis, cross the blood–brain barrier rapidly and modify neural differentiation and synaptogenesis leading to severe damage. Furthermore, Pb exposure was found to contribute to the hallmarks of AD, including Aβ accumulation, expression of tau protein, and neuroinflammation.47 In vitro studies on SH-SY5Y neuroblastoma cells revealed that Pb exposure led to Aβ accumulation, APP expression and decreased mRNA and protein levels of neprilysin endopeptidase, a Aβ degrading enzyme.48,49 Additionally, Pb exposure in young rats increased the expression of APP and BACE1 which subsequently induced AD-like pathology by inducing Aβ accumulation and plaque formation in the hippocampus and cortex.50 In another in vivo study on rats drinking water containing 200 ppm Pb at the age of 1–20 days, a delayed overexpression of APP and elevation of its amyloidogenic Aβ product in old age was observed.51 Also, elevations in the oxidative DNA marker 8-hydroxy-2′-deoxyguanosine (8-oxo-dG) were reported in older rat brains that had been developmentally exposed to Pb.52 The first to perform primates' studies were Wu et al. who reported that exposure of monkeys to Pb at birth to 400 days upregulates the expression of APP and BACE1 in old age.53
2.4. Arsenic
Arsenic is a naturally occurring environmental toxicant in which contaminates the soil and drinking water and represents a global health threat. Arsenic is generally used in manufacturing lead alloys and semiconductor electronic devices. Arsenic exposure causes several health problems such as cancer, liver damage, and nervous system disturbances such as polyneuropathy, hallucinations, disorientation, and agitation.54–56 Arsenic has been found to be the most toxic metalloid responsible for the neurotoxicity associated with AD development in the brain, impairing cognitive functions.57 Such observation was supported by evidence that prolonged arsenic exposure causes an increase in tau phosphorylation and insoluble tau aggregates in SH-SY5Y cells.58 Another in vitro study showed that incubation of cholinergic SN56.B5.G4 cells with organic dimethylarsinic acid led to an increase in Aβ levels59 Finally, an in vivo study using 3xTgAD AD mice model suggested that exposure to inorganic arsenic can lead to behavioral impairments, presence of amyloid plaques and neurofibrillary tangles, thus exacerbating AD pathophysiological progression.60 Selected studies investigating the effects of mercury, lead, cadmium, and arsenic exposure on AD pathology are summarized in Table 1.
Table 1 Summary of studies on heavy metals effect on AD pathology
Metal |
Study model |
Outcome |
References |
Mercury |
Rats |
Mercury induces neuronal beta-tubulin defects |
26 and 27 |
AD patients |
Mercury concentration in plasma is doubled than control group |
28 |
Workers with high exposure to mercury |
Symptoms of cognitive deficit are witnessed |
29 and 30 |
Autopsied brain of AD patient |
Mercury deposition in hippocampus and amygdala |
31 |
Cadmium |
Transgenic mice (APP/PS1 mice) |
Cd increases Aβ-42 production and enlarges size of senile plaque |
43 |
AD patients |
Blood cadmium levels is associated with AD morbidity rate |
44 and 45 |
Lead |
SH-SY5Y neuroblastoma cells |
Pb exposure leads to Aβ secretion, APP expression and decrease in NEP |
48 and 49 |
Rats |
Increased the expression of APP and BACE1, induce Aβ accumulation and plaque formation in the hippocampus and cortex |
50 |
Rats |
Pb exposure at birth causes overexpression of APP and elevation of its amyloidogenic Aβ product in old age |
51 |
Rats |
Elevations of 8-oxo-dG as a result of Pb exposure |
52 |
Monkeys |
Pb exposure at birth lead to expression of APP and BACE1 in old age |
53 |
Arsenic |
SH-SY5Y cells |
As exposure causes an increase in tau phosphorylation and insoluble tau aggregates |
58 |
Cholinergic SN56.B5.G4 cells |
Organic dimethylarsinic acid (DMA) led to an increase in Aβ levels |
59 |
3xTgAD AD mice model |
Inorganic arsenic can lead to behavioral impairments, presence of amyloid plaques and neurofibrillary tangles |
60 |
3. Chemosensors for sensing metal ions
Recently, optical chemosensors have shown a great potential for monitoring the toxic heavy metals present in the environment.61 Optical chemosensors generate visual signals for qualitative detection of toxic metals as ref. 62. Other advantages of optical chemosensors include sensitivity, selectivity, low cost, fast response time, and low detection limits. Furthermore, they allow for simple and quantitative simultaneous detection of multiple toxic metals if used in conjunction with simple colorimeters which reduces the errors caused by sample storage and transportation for analyses by central sophisticated laboratory instruments such as ICP-MS. Optical chemosensors change color upon binding of their immobilized indicators (chromophores) to their target analytes.
3.1. Chemosensor design
Selecting the right carriers is critical for designing chemosensors. In general, many carriers have been used such as zeolites, metal oxides, polymers, metallosupramolecular network, and carbon-based materials.63 Mesoporous silica or metal organic frameworks are generally used as carriers in the preparation of optical chemosensors (Fig. 2).64,65 Although, some heavy metals are directly detected via ocular property such as luminescence or absorption, there are some targets has no absorption or luminescence property, therefore, optical chemosensors with selective and sensitive optical probe were designed. The designed chemosensor may take various forms such as powder, gels, thin films or nanoparticles. The sensor changes its optical properties in presence of toxic metals.66 The detection of common cations in water, cosmetic, or blood is based on complexation between the chromophore and its specific target cation. Therefore, highly selective chromophores with electron donating atoms were prepared and immobilized on carriers via covalent, physical, or electrostatic interactions.67 Proper selection of mesoporous or MOFs nanomaterials is crucial as it affects mechanical stability of the sensor, dye immobilization, and access of the toxic metals.68
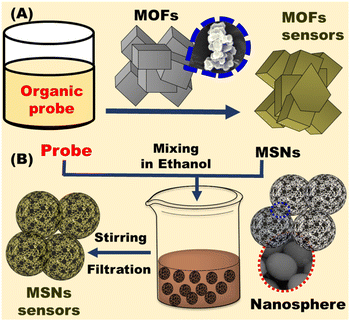 |
| Fig. 2 Fabrication of optical chemosensors using (A) metal organic frameworks (MOFs) and (B) mesoporous silica (MSNs). | |
3.1.1. Nanoporous carriers. Common porous materials include porous metal oxides, mesoporous silicates, porous carbons, and porous polymers.63 According to their pore size, porous substance can be categorized into macroporous, mesoporous and microporous materials.69 Mesoporous and microporous materials act as adsorbents70,71 or scaffolds for loading chromophores when utilized in metal ion detection. This improves recognition of the target toxic metals and enhance the generated signal. The large surface area of these materials provides more active sites for metal adsorption. The powerful and rapid response toward metal ions can be achieved by adjusting their surface area, and the pore diameter, allowing various metal ions monitoring and a low detection limit. Mesoporous silica and metal–organic frameworks have been used to design optical chemosensors.72,73
3.1.1.1. Mesoporous silica. The large surface area and stability of mesoporous silica nanomaterials enabled their utilization as carriers in the fields of sensing74 and catalysis.75 The soft and hard template methods were used to fabricate different structures and formation of mesoporous cavity. In the hard template approach, the rattle-type of silica nanoparticles was prepared with controllable morphology and structure. The calcination process is used for preparation of mesoporous silica as shown in Fig. 3. Therefore, the synthesis of MSN is conducted under relatively mild conditions (Fig. 3). The mesoporous nanomaterials are employed in optical chemosensors as carriers of chromophores for enhanced heavy metal recognition. To detect the selected heavy metal ions, the outer surface of MSN nanomaterials is decorated with the organic chromophore via physical or chemical interactions.
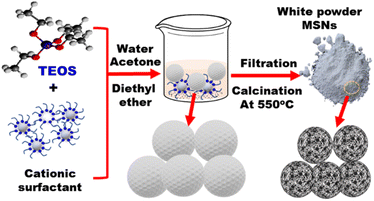 |
| Fig. 3 Preparation of mesoporous silica as nanoporous carrier. Briefly, TEOS (tetraethoxysilane) is reacted with a cationic surfactant (e.g., CTAB) in water/acetone/diethyl ether solvent to form mesoporous silica nanospheres (MSNs). Filtration is then carried out followed by calcination at 550 °C. | |
3.1.1.2. Metal–organic frameworks. Metal organic frameworks (MOFs) are promising hybrid porous materials, prepared by joining metal–oxygen clusters with organic linkers. MOFs consist of metal nodes linked with organic ligands via coordinate bond, and the ligands play a vital role in designing high specific surface areas and porous structure.76 Generally, the metal nodes and linkers play a significant role in the structure of MOFs (Fig. 4). The organic linkers are secondary building units (as rods) whereas the metal centers act as junctions of MOF constructions. To build up MOF structures, changing the metal centers and selecting a suitable linker are considered the main factors in designing the framework topology. The superior porosity with significant large pores over volume properties are tailored by broadened MOF's topology (Fig. 5). The substantial MOF pore volume is dependent on the organic linker size and bulky metal clusters' network. Moreover, MOFs are used for immobilizing catalysts on conductive scaffolds with high surface area and pore size. Owing to their crystallinity and porosity, the MSNs or MOFs were used as the carrier in optical chemical sensors for detection of common cations.77,78 MOFs materials were used for other applications such as catalysis79 and gas adsorption/storage.80
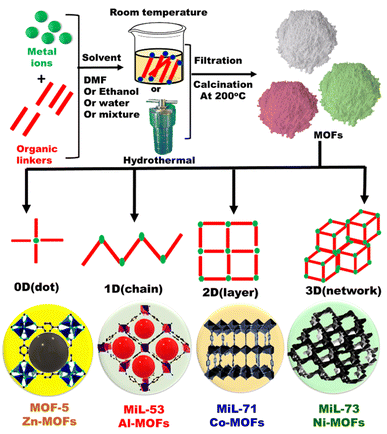 |
| Fig. 4 General schematic representation for fabrication of metal organic frameworks (MOFs) with different dimensions. | |
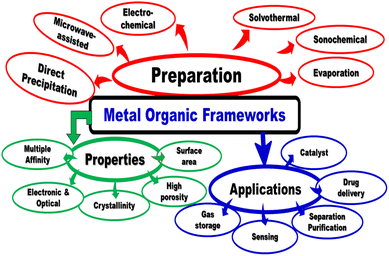 |
| Fig. 5 Preparation, properties and applications of metal organic frameworks. | |
3.1.1.3. Other nanoporous carriers. Several nanomaterials have been utilized as porous carriers because of their unique characteristics.81 Nanocrystalline TiO2 films were employed for colorimetric naked-eye detection of mercury in aqueous solution.82,83 Chey et al. investigated indirect determination of Hg2+ using ZnO nanorods.84 Furthermore, polymeric carriers play an important role in protecting photochromic characteristics from environmental degradation. The flat sheet cellulose acetate membrane was used as a substrate for loading dithizone chromophore for naked eye detection of Hg2+.85 Paper-based chemosensors (PBCs) were recently developed for monitoring Co2+ and Cd2+ in cosmetic products by adsorbing the chromophore onto filter papers coated with mesoporous silica.64
3.1.2. Probe immobilization. The immobilization of an organic probe onto a suitable porous material or polymer matrix, has a significant effect on the sensor monitoring features. The common approaches for immobilization of optical chemosensors include impregnation techniques, covalent bonding, and doping approach (Fig. 6).
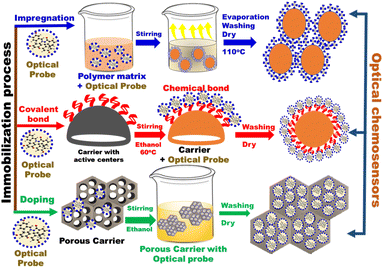 |
| Fig. 6 General schematic diagram of different immobilization approaches of organic probes on different carriers. | |
3.1.2.1. Impregnation of existing matrices. Impregnation is a classical method for loading a chromophore on a porous carrier. In this approach, a thin support film of the carrier is dipped into a saturated solution of chromophore and the solvent is left to evaporate.86,87 The organic chromophore is loaded into the polymer matrix via chemisorption or electrostatic interactions. Although the impregnation technique is inexpensive, leaching of the chromophore (due to weak bonds with the carrier) hinders the large-scale utilization of this approach.
3.1.2.2. Covalent bonding to existing matrices. In this approach, the optical chromophore is chemically bonded to the surface of mesoporous or microporous materials via its functional groups. Alternatively, the chromophore may interact chemically with certain monomers to form a copolymer.88,89 Chemosensors prepared by covalent immobilization are highly stable and can be used multiple times. Disadvantages of chemosenors prepared by this method include low sensitivity and extended response time.
3.1.2.3. Doping. This is the most commonly used approach for immobilization of optical probes onto nanomaterials. Significantly, the selected chromophore is introduced to the porous nanomaterial solution during the preparation process.90–93 An optical sensor prepared by the doping method has high stability as compared to that prepared using the impregnation technique. Moreover, the response time is better than that observed with chemosensors prepared using the covalent bonding approach.
4. Detection of AD related heavy metals
Optical chemosensors can be divided according to the mechanism scheme into three main categories: carrier-based ion sensing, intrinsic metal ion sensors, and indicator-mediated ion sensing.78
4.1. Mercury (Hg)
Kongasseri and co-workers reported the fabrication of sensor for the quantification of toxic Hg2+ ions through sol–gel process using two different block-polymer surfactants (PEO and F108) via doping method. The low limit of detection for probe anchored F108-MSM in sensing of mercury ions was 0.61 and 2.05 ppb; respectively (Fig. 7A). The sensor can be reused over 12 times for six months of storage.94 Radwan et al., used optical chemosensors via impergnation method of bis(4-(dimethylamino)phenyl) methanethione into Al-MOFs for sensing Hg2+ in water samples and skin whitening products.95 The sensitivity and adsorption capacity of optical chemosensors for Hg2+ were 0.8 ppb and 1110 mg g−1; respectively (Fig. 7B). The Hg2+ sensor was used after multiple regeneration/reuse cycles (>9 cycles). Simple optical sensor for monitoring and removal of Hg2+ from aqueous media using amino-functionalized MOF with ninhydrin was designed by Shahat and co-workers using covalent bonding approach.96 They detected Hg2+ with a low limit of detection of ∼0.494 ppb in water and the sensor can be for (≥6) cycles (Fig. 7C).
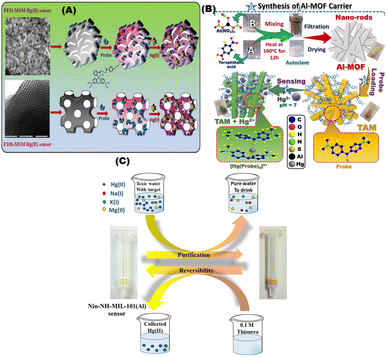 |
| Fig. 7 (A) Schematic diagram for sensing Hg2+ ions using bis(diethylamino)-3oxospiro[isoindoline-1,9′-xanthen]-2ylcarbamothioyl)-4-butylbenzamide (BOICB) probe.94 (B) Schematic representation of fabrication of nanorod TAM optical chemosensors and interactions with Hg2+ ions under optimum conditions.95 (C) Representative design of the Nin-NH-MIL-101(Al) sensor applied for purification of water polluted with Hg(II) ions and the reversible process by using 0.1 M thiourea solution (which can be repeated several times).96 Reproduced with permission from ref. 94–96. | |
4.2. Cadmium (Cd)
Aluminosilica-based network platforms were used as carriers in designing optical sensors via direct impregnation of TMPyP moieties without any surface modification. The optical sensor was used for detection and removal of Cd2+ ions at low concentrations (10−10 mol dm−3) for different analytical applications (Fig. 8A).69 Shenashen et al., used aluminosilica carrier to immobilize chromophore for Cd2+ ions detection using impregnation approach (Fig. 8B). This optical chemosensor was utilized for removal and visualization of some toxic metals such as Cd2+ at (∼10−11 mol dm−3) in water and a suspension of red blood cells (RBCs) and could be regenerated/reused for 6 cycles.97 Shahat and co-workers developed an optical sensor based on Zr-MOFs (UiO-66) for detection, and removal of Cd2+ ions. The UiO-66 was used for impregnation of dithizone (Fig. 8C) without any coupling agent. The reported detection limit was 10−10 mol dm−3 and could be reused for 6 times.98 Radwan et al., designed optical chemosensors via using impregnation technique of the 1-(2-pyridylazo)-2-naphthol with the mesoporous cavities of nanospheres silica for visual detection of Cd2+ in water samples. Digital image analysis was applied to determine the Cd2+ concentration in well water samples with a low detection limit of 10−9 mol L−1. The sensor could be stored for more than 8 months and reused for (i.e. ≥9) cycles.99
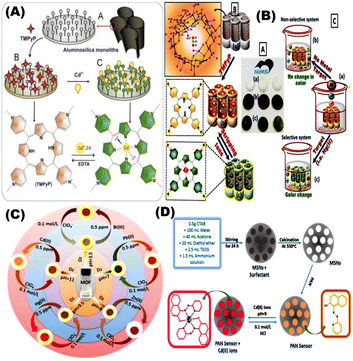 |
| Fig. 8 (A) General schematic presentation of optical chemosensors based on aluminosilica network platforms via direct functionalization with δ-tetrakis(1-methylpyridinium-4-yl)porphine ρ-toluenesulfonate for Cd(II) ions detection.76 (B) Chelating ligand immobilized mesostructures Ia 3d aluminosilica for visualization and removal of Cd(II).97 (C) The Zr-based metal–organic frameworks (UiO-66) with its micropore geometry for the visual detection, determination and removal of ultra-trace of some toxic metal ions such as Cd2+.98 (D) General steps of fabricating Cd2+ ion optical chemosensors built on mesoporous nanosphere silica for naked-eye determination of ultra-traces of Cd2+ ions.99 Reproduced with permission from ref. 69,97–99. | |
4.3. Lead (Pb)
Xuanxuan and coworkers prepared fluorescent probe based on amino-functionalized MOFs (MOF-5-NH2) using covalent bonding approach for sensing of Pb2+ using a single step synthesis (Fig. 9A). The Pb2+ coordinated with the amino groups on the surface of the MOF-5-NH2 thus allowing for fluorescence quenching with a low limit of detection of 0.25 μmol L−1.100 Kuiyu Yi and Lei Zhang designed fluorescence probe by encapsulating thioglycolic acid modified CdTe quantum dots (QDs) and carbon dots (CDs) into porous (MOFs) for detection of Pb2+ using doping method in biological samples (Fig. 9B).101 Shifen Xua and coworkers developed Pb2+ phosphorescent chemosensors using MOF-5. The Pb2+ chemosensors showed a low detection limit of 2 nmol L−1 and linear range of 0.01–10 μmol L−1 (Fig. 9C). They prepared phosphorescent chemosensors in a polyethylene glycol film using impregnation method, which exhibit color change at 1.0 μmol /L−1 Pb2+ under 365 nm UV light.102
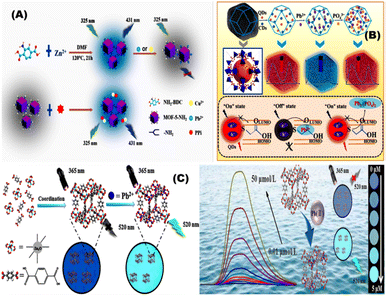 |
| Fig. 9 (A) General representation of fluorescent probe prepared based on amino-functionalized metal–organic frameworks (MOF-5-NH2) for the detection of Pb2+.100 (B) Ratiometric fluorescent (RF) probe CDs/QDs@ZIF-8 by encapsulating carbon dots (CDs) and thioglycolic acid modified CdTe quantum dots (QDs) into porous metal–organic frameworks (MOFs) for detection of Pb2+.101 (C) Phosphorescent sensor to monitor Pb2+ using metal–organic framework-5.102 Reproduced with permission from ref. 100–102. | |
4.4. Arsenic
Biswajit et al. reported a carboxylate-rich MOF for As(III) detection where a linear increase in the fluorescence intensity was observed for arsenic concentration in the range of 3.67–332 ng L−1.103 In another work, a MOF functionalized nanoconjugate material was prepared by Shahat et al. for selective sensing of As(V) and phosphate in the aqueous media. The detection limits of As(V) and phosphate were 0.15 μg L−1 and 0.13 μg L−1, respectively. Detection was observed via change of the MOF color to blue, which was analyzed by UV-vis spectroscopic techniques.104 Different chemosensors were utilized for detection of mercury, lead, cadmium, and arsenic were listed in Table 2.
Table 2 Chemosensors used for detection of mercury, cadmium, lead, and arsenic ions
Metal |
Chemosensors |
Substrate |
Probe mechanism |
Detection limit (μM) |
Ref. |
Hg |
Probe anchored F108-MSM |
MSNs |
Absorbance |
0.003 |
94 |
Bis(4(dimethylamino)phenyl)methanethione into Al-mofs |
MOFs |
Absorbance |
0.0039 |
95 |
Amino-functionalized MOF with ninhydrin |
MOFs |
Absorbance |
0.0024 |
96 |
Cd |
Aluminosilica-based network platforms |
Aluminosilica |
Absorbance |
0.03 |
69 |
A porphyrinic chelating ligand |
Aluminosilica |
Absorbance |
0.03 |
97 |
Optical sensor based on Zr-mofs (uio-66) |
MOFs |
Absorbance |
0.18 |
98 |
1-(2-Pyridylazo)-2-naphthol with the mesoporous silica |
MSNs |
Absorbance |
0.034 |
99 |
Pb |
Amino-functionalized MOFs (MOF-5-NH2) |
MOFs |
Fluorescence |
0.25 |
100 |
CdTe quantum dots (QDs) and carbon dots (CDs) into porous (MOFs) |
MOFs |
Fluorescence |
0.0235 |
101 |
Phosphorescent chemosensors using MOF-5 |
MOFs |
Phosphorescence |
0.02 |
102 |
As |
Carboxylate-rich MOF |
MOFs |
Fluorescence |
0.049 |
103 |
MOF functionalized nanoconjugate material |
MOFs |
Absorbance |
0.15 |
104 |
5. Challenges in the field of optical sensors
Although qualitative results generated by optical chemosensors are useful to identify contaminated water sources, quantitative results are needed to guide actions based on guideline cut off values. Since the basic idea behind the development of chemosensors is the specificity of the signal obtained, it is important to implement selective recognition moieties (probes) for the target metal. Adjusting the detection conditions (such as temperature, pH, sensor concentration, and contact time) is critical for optimal detection. The ideal optical chemosensor should have high selectivity, sensitivity, low detection limit, and a short response time. It should also be reusable, inexpensive, and simple to use. Optical chemosensors have been used for sensing and monitoring of toxic metals and radioactive isotopes and bioactive species.
6. Conclusions and future perspectives
Exposure to heavy metals such as Hg, Cd, Pb and As poses a serious threat to the nervous system and may contribute to the onset and progression of AD. Therefore, monitoring environmental exposure to heavy metals is critical. Monitoring protocols based on nanoporous metal–organic framework and mesoporous silica carriers have been developed for detection of heavy metals. Rapid improvements in the nanoporous materials presented significant opportunities to fabricate novel sensors for different monitoring applications. These sensors can be used in different environments where the hazardous materials are produced or accumulate. The fabrication of optical chemosensors with exceptional structures and morphologies led to the progress of various detection techniques of ultra-trace concentrations of heavy metals. In conclusion, optical chemosensors, including those with hieratical nanochannels, could play a major role in large scale monitoring of exposure to water contaminated with AD-related heavy metals.
Conflicts of interest
Prof. Hassan Azzazy is an inventor on a granted patent on development of chemosensors for detection of toxic metals. Other authors declare no conflict of interest.
Acknowledgements
This work has been funded by an award from the French Chamber of Commerce in Cairo to Prof. Hassan Azzazy.
References
- T. Dening and M. B. Sandilyan, Dementia: definitions and types, Nursing Standard, 2015, 29(37), 37–42 CrossRef.
- World Health Organization (WHO), Factsheet Dementia, WHO, Geneva, Switzerland, 2020, available online: https://www.who.int/news-room/fact-sheets/detail/dementia accessed on 31 March 2022 Search PubMed.
- P. Tiraboschi, L. A. Hansen, L. J. Thal and J. Corey-Bloom, The importance of neuritic plaques and tangles to the development and evolution of AD, Neurology, 2004, 62, 1984–1989 CrossRef PubMed.
- R. D. Terry, E. Masliah, D. P. Salmon, N. Butters, R. DeTeresa, R. Hill, L. A. Hansen and R. Katzman, Physical basis of cognitive alterations in Alzheimer's disease: synapse loss is the major correlate of cognitive impairment, Ann. Neurol., 1991, 30, 572–580 CrossRef PubMed.
- K. Iqbal, F. Liu and C. X. Gong, Tau and neurodegenerative disease: the story so far, Nat. Rev. Neurol., 2016, 12, 15–27 CrossRef PubMed.
- S. Itagaki, P. L. McGeer, H. Akiyama, S. Zhu and D. Selkoe, Relationship of microglia and astrocytes to amyloid deposits of Alzheimer disease, J. Neuroimmunol., 1989, 24, 173–182 CrossRef PubMed.
- M. V. F. Silva, C. D. M. G. Loures and L. C. V. Alves, Alzheimer's disease: risk factors and potentially protective measures, J. Biomed. Sci., 2019, 26, 33 CrossRef PubMed.
- E. Becker, C. Orellana Rios, C. Lahmann, G. Rücker, J. Bauer and M. Boeker, Anxiety as a risk factor of Alzheimer's disease and vascular dementia, Br. J. Psychiatry, 2018, 213(5), 654–660 CrossRef PubMed.
- M. N. Wainaina, Z. Chen and C. Zhong, Environmental factors in the development and progression of late-onsetAlzheimer’s disease, Neurosci. Bull., 2014, 30, 253–270 CrossRef CAS PubMed.
- W. B. Grant, A. Campbell, R. F. Itzhaki and J. Savory, The significance of environmental factors in the etiology ofAlzheimer's disease, J. Alzheimer's Dis., 2002, 4, 179–189 Search PubMed.
- Z. Zhang, M. Miah, M. Culbreth and M. Aschner, Autophagy in Neurodegenerative Diseases and Metal Neurotoxicity, Neurochem. Res., 2016, 41, 409–422 CrossRef CAS PubMed.
- T. J. Huat, J. Camats-Perna, E. A. Newcombe, N. Valmas, M. Kitazawa and R. Medeiros, Metal Toxicity Links to Alzheimer's Disease and Neuroinflammation, J. Mol. Biol., 2019, 431(9), 1843–1868 CrossRef CAS.
- A. Odobašić, I. Šestan, and S. Begić, Biosensors for Determination of Heavy Metals in Waters, in Biosensors for Environmental Monitoring, London, United Kingdom, IntechOpen, 2019, Online, available: https://www.intechopen.com/chapters/66031, DOI:10.5772/intechopen.84139.
- P. E. Drevnick, C. H. Lamborg and M. J. Horgan, Increase in mercury in Pacific yellow fin tuna, Environ. Toxicol. Chem., 2015, 34, 931–934 CrossRef CAS PubMed.
- A. Renzoni, F. Zino and E. Franchi, Mercury levels along the food chain and risk for exposed populations, Environ. Res., 1998, 77(2), 68–72 CrossRef CAS PubMed.
- A. Franzblau, H. d'Arcy, M. B. Ishak, R. A. Werner, B. W. Gillespie, J. W. Albers, C. Hamann, S. E. Gruninger, H. N. Chou and D. M. Meyer, Low-level mercury exposure and peripheral nerve function, Neurotoxicology, 2012, 33(3), 299–306 CrossRef CAS PubMed.
- F. Monnet-Tshudi, M. G. Zurich, C. Boschat, A. Corbaz and P. Honegger, Involvement of environmental mercury and lead in the etiology of neurodegenerative diseases, Rev. Environ. Health, 2006, 21, 105–118 Search PubMed.
- K. Yano, N. Hirosawa, Y. Sakamoto, H. Katayama, T. Moriguchi, K. E Young, Y. Y. Sheen and K. Asaoke, Aggregations of amyloid beta-proteins in the presence of metal ions, Toxicol. Lett., 2002, 144, 134 CrossRef.
- N. H. Zawai, M. D. Basha and W. Wei, The influence of lead and mercury on beta-amyloid aggregation and cytotoxicology, Soc. Neurosci. Abstr., 2002, 688, 10 Search PubMed.
- M. Fujimura, F. Usuki, M. Sawada, O. Rostene, D. Godefroy and A. Takashima, Methyl mercury induces neuropathological changes with tau hyperphosphorylation mainly through activation of the c-jun-n terminal kinase pathway in the cerebral cortex, but not in the hippocampus of the mouse brain, Neurotoxicology, 2009, 30, 1000–1007 CrossRef CAS.
- J. Albrecht and E. Matja, Glutamate: A potential mediator of inorganic mercury neurotoxicity, Metab. Brain Dis., 1996, 11, 175–184 CrossRef CAS.
- J. W. Song and B. S. Choi, Mercury induced the accumulation of amyloid Beta (AB) in PC 12 cells: The Role of production and degradation of AB, Toxicol. Res., 2013, 29, 235–240 CrossRef CAS PubMed.
- S. A. Shaffi, Sublethal effect of mercury and lead on monoamine oxidase in different regions of the brain in three fresh water teleosts, Rev. Esp. Fisiol., 1995, 51, 125–128 CAS.
- D. Chandradhar, R. Raghunathan and B. Joshi, Effect of mercury compounds on choline acetyltransferase, Res. Commun. Chem. Pathol. Pharmacol., 1980, 30, 381–384 Search PubMed.
- R. Siblerud, J. Mutter, E. Moore, J. Naumann and H. Walach, A Hypothesis and Evidence That Mercury May be an Etiological Factor in Alzheimer's Disease, Int. J. Environ. Res. Public Health, 2019, 16, 5152 CrossRef CAS.
- E. F. Duhr, J. C. Pendergrass, J. T. Slevin and B. E. Haley, HgEDTA complex inhibits GTP interactions with the E-site of brain beta-tubulin, Toxicol. Appl. Pharmacol., 1993, 122(2), 273–280 CrossRef CAS PubMed.
- J. C. Pendergrass, B. E. Haley, M. J. Vimy, S. A. Winfield and F. L. Lorscheider, Mercury vapor inhalation inhibits binding of GTP to tubulin in rat brain: similarity to a molecular lesion in Alzheimer diseased brain, Neurotoxicology, 1997, 18(2), 315–324 CAS.
- C. Hock, G. Drasch, S. Golombowski, F. Müller-Spahn, B. Willershausen-Zönnchen, P. Schwarz and R. M. Nitsch, Increased blood mercury levels in patients with Alzheimer's disease, J. Neural Transm., 1998, 105(1), 59–68 CrossRef CAS PubMed.
- H. Frumkin, R. Letz, P. L. Williams, F. Gerr, M. Pierce, A. Sanders, L. Elon, C. C. Manning, J. S. Woods, V. S. Hertzberg, P. Mueller and B. B. Taylor, Health effects of long-term mercury exposure among chloralkali plant workers, Am. J. Ind. Med., 2001, 39, 1–18 CrossRef CAS.
- T. Mathiesen, D. G. Ellingsen and H. Kjuus, Neuropsychological effects associated with exposure to mercury vapor among former chloralkali workers, Scand. J. Work, Environ. Health, 1999, 25, 342–350 CrossRef CAS PubMed.
- D. Wenstrup, W. D. Ehmann and W. R. Markesbery, Trace element imbalances in isolated subcellular fractions of Alzheimer's disease brains, Brain Res., 1990, 533, 125–131 CrossRef CAS PubMed.
- P. D. Pigatto, A. Costa and G. Guzzi, Are mercury and Alzheimer's disease linked?, Sci. Total Environ., 2018, 613–614, 1579–1580 CrossRef CAS.
- G. Notarachille, F. Arnesano, V. Calò and D. Meleleo, Heavy metals toxicity: effect of cadmium ions on amyloid beta protein 1–42. Possible implications for Alzheimer's disease, BioMetals, 2014, 27, 371–388 CrossRef CAS.
- S. Qadir, S. Jamshieed, S. Rasool, M. Ashraf, N. A. Akram and P. Ahmad, Modulation of plant growth and metabolism in cadmium-enriched environments, Rev. Environ. Contam. Toxicol., 2014, 229, 51–88 CAS.
- B. Wang and Y. Du, Cadmium and its neurotoxic effects, Oxid. Med. Cell. Longevity, 2013, 2013, 898034 Search PubMed.
- C. D. Syme and J. H. Viles, Solution 1H NMR investi-gation of Zn2+ and Cd2+ binding to amyloid-beta pep-tide(Abeta) of Alzheimer's disease, Biochim. Biophys. Acta, 2006, 1764, 246–256 CrossRef CAS.
- K. Yano, N. Hirosawa, Y. Sakamoto, H. Katayama and T. Moriguchi, 500 Aggregations of amyloid beta-proteins in the presence of metal ions, Toxicol. Lett., 2003, 144, 134 CrossRef.
- K. Endres and F. Fahrenholz, The role of the anti-amyloidogenic secretase adam10 in shedding the app-like proteins, Curr. Alzheimer Res., 2012, 9, 157–164 CrossRef CAS PubMed.
- J. Del Pino, G. Zeballos, M. J. Anadon, P. Moyano, M. J. Diaz, J. M. Garcia and M. T. Frejo, Cadmium-induced cell death of basal forebrain cholinergic neurons mediated by muscarinic m1 receptor blockade, increase in gsk-3beta enzyme, beta-amyloid and tau protein levels, Arch. Toxicol., 2016, 90, 1081–1092 CrossRef CAS PubMed.
- L. F. Jiang, T. M. Yao, Z. L. Zhu, C. Wang and L. N. Ji, Impacts of cd(ii) on the conformation and self-aggregation of alzheimer's tau fragment corresponding to the third repeat of microtubule-binding domain, Biochim. Biophys. Acta, 2007, 1774, 1414–1421 CrossRef CAS.
- S. Phuagkhaopong, D. Ospondpant, T. Kasemsuk, N. Sibmooh, S. Soodvilai, C. Power and P. Vivithanaporn, Cadmium-induced il-6 and il-8 expression and release from astrocytes are mediated by mapk and nf-kappab pathways, Neurotoxicology, 2017, 60, 82–91 CrossRef CAS.
- C. Liu, G. Cui, M. Zhu, X. Kang and H. Guo, Neuroinflammation in alzheimer's disease: Chemokines produced by astrocytes and chemokine receptors, Int. J. Clin. Exp. Pathol., 2014, 7, 8342–8355 Search PubMed.
- X. Li, Y. Lv, S. Yu, H. Zhao and L. Yao, The effect of cadmium on Abeta levels in APP/PS1 transgenic mice, Exp. Ther. Med., 2012, 4, 125–130 CrossRef CAS.
- J. Y. Min and K. B. Min, Blood cadmium levels and alzheimer's disease mortality risk in older us adults, Environ. Health, 2016, 15, 69 CrossRef.
- Q. Peng, K. M. Bakulski, B. Nan and S. K. Park, Cadmium chloride and, Alzheimer's disease mortality in U.S. adults: updated evidence with a urinary biomarker and extended follow-up time, Environ. Res., 2017, 157, 44–51 CrossRef CAS PubMed.
- H. Needleman, Low level lead exposure: History and discovery, Ann. Epidemiol., 2009, 19, 235–238 CrossRef.
- T. Sanders, Y. Liu and V. P. B. Buchner Tchounwou, Neurotoxic effects and biomarkers of lead exposure: A review, Rev. Environ. Health, 2009, 24, 15–45 CAS.
- A. Reuben, Childhood lead exposure and adult neurodegenerative disease, J. Alzheimer's Dis., 2018, 64, 17–42 Search PubMed.
- S. W. Bihaqi, Early life exposure to lead (Pb) and changes in DNA methylation: relevance to Alzheimer's disease, Rev. Environ. Health, 2019, 34, 187–195 CrossRef CAS PubMed.
- C. C. Zhou, Z. Y. Gao, J. Wang, M. Q. Wu, S. Hu, F. Chen, J. X. Liu, H. Pan and C. H. Yan, Lead exposure induces Alzheimers's disease (ad)-like pathology and disturbs cholesterol metabolism in the young rat brain, Toxicol. Lett., 2018, 296, 173–183 CrossRef CAS PubMed.
- M. R. Basha, W. Wei, S. A. Bakheet, N. Benitez, H. K. Siddiqi, Y. W. Ge, D. K. Lahiri and N. H. Zawia, The fetal basis of amyloidogenesis: exposure to lead and latent overexpression of amyloid precursor protein and beta-amyloid in the aging brain, J. Neurosci., 2005, 25, 823–829 CrossRef CAS.
- C. M. Bolin, R. Basha, D. Cox, N. H. Zawia, B. Maloney, D. K. Lahiri and F. Cardozo-Pelaez, Exposure to lead and the developmental origin of oxidative DNA damage in the aging brain, FASEB J., 2006, 20, 788–790 CrossRef CAS PubMed.
- J. Wu, M. R. Basha, B. Brock, D. P. Cox, F. Cardozo-Pelaez, C. A. McPherson, J. Harry, D. C. Rice, B. Maloney and D. Chen, et al, Alzheimer's disease (AD)-like pathology in aged monkeys after infantile exposure to environmental metal lead (Pb): evidence for a developmental origin and environmental link for AD, J. Neurosci., 2008, 28, 3–9 CrossRef CAS.
- H. Garelick, H. Jones, A. Dybowska and E. Valsami-Jones, Arsenic pollution sources, Rev. Environ. Contam. Toxicol., 2008, 197, 17–60 CAS.
- M. Valko, H. Morris and M. T. D. Cronin, Metals, toxicity and oxidative stress, Curr. Med. Chem., 2005, 12, 1161–1208 CrossRef CAS.
- A. H. Smith, P. A. Lopipero and M. N. Bates, et al., Arsenic epidemiology and drinking water standards, Science, 2002, 296, 2145–2146 CrossRef CAS PubMed.
- C. R. Tyler and A. M. Allan, The Effects of Arsenic Exposure on Neurological and Cognitive Dysfunction in Human and Rodent Studies: A Review.Curr, Environ. Health Rep., 2014, 1, 132–147 Search PubMed.
- C. Wisessaowapak, D. Visitnonthachai, P. Watcharasit and J. Satayavivad, Prolonged arsenic exposure increases tau phosphorylationin differentiated SH-SY5Y cells: The contribution of GSK3 and ERK1/2, Environ. Toxicol. Pharmacol., 2021, 84, 103626 CrossRef CAS PubMed.
- S. Zarazua, S. Bürger, J. M. Delgado, M. E. Jiménez-Capdeville and R. Schliebs, Arsenic affects expression and processing of amyloidprecursor protein (APP) in primary neuronal cells overexpressing the Swedish mutation of human, Int. J. Dev. Neurosci., 2011, 29, 389–396 CrossRef CAS PubMed.
- S. A. Nino, A. Morales-Martínez, E. Chi-Ahumada, L. Carrizales, R. Salgado-Delgado, F. Pérez-Severiano, S. Díaz-Cintra, M. E. Jiménez-Capdeville and S. Zarazúa, Arsenic Exposure Contributes to the Bioenergetic Damage in an Alzheimer's Disease Model, ACS Chem. Neurosci., 2019, 10, 323–336 CrossRef CAS PubMed.
- X. D. Wang and O. S. Wolfbeis, Fiber-optic chemical sensors and biosensors, Anal. Chem., 2012, 85, 487–508 CrossRef.
- H. Ezoji and M. Rahimnejad, Nanoparticles-based Electrochemical Sensors and Biosensors. Nanoparticle Design and Characterization for Catalytic Applications in Sustainable Chemistry, 2019,38,p. 329 Search PubMed.
- X. S. Zhao, F. Su, Q. Yan, W. Guo, X. Y. Bao, L. Lv and Z. Zhou, Templating methods for preparation of porous structures, J. Mater. Chem., 2006, 16(7), 637–648 RSC.
- A. Radwan, I. M. El-Sewify and H. M. E. S. Azzazy, Monitoring of Cobalt and Cadmium in Daily Cosmetics Using Powder and Paper Optical Chemosensors, ACS Omega, 2022, 7(18), 15739–15750 CrossRef CAS PubMed.
- M. M. H. Khalil, A. Shahat, A. Radwan and M.F. El-Shahat, Colorimetric determination of Cu(II) ions in biological samples using metal-organic framework as scaffold, Sens. Actuators, B, 2016, 233, 272–280 CrossRef CAS.
- A. Lobnik, M. Turel, Š. K. Urek and A. Košak, Nanostructured materials use in sensors: their benefits and drawbacks, Carbon and Oxide Nanostructures, Springer Berlin Heidelberg, Springer, 2010, pp. 307–354 Search PubMed.
- M. Braim, Investigating the binding capabilities of triazole-calix [4] arene functionalized microcantilever sensors toward heavy metals in aqueous solution, Diss. Memorial University of Newfoundland, 2017 Search PubMed.
- S. Afsaneh and M. Bagheri, A novel optical sensor for uranium determination, Anal. Chim. Acta, 2005, 530, 55–60 CrossRef.
- S. A. El-Safty, M. A. Shenashen and M. Khairy, Optical detection/collection of toxic Cd (II) ions using cubic Ia3d aluminosilica mesocage sensors, Talanta, 2012, 98, 69–78 CrossRef CAS.
- A. E. Soliman, M. A. Shenashen, I. M. El-Sewify, G. M. Taha, M. A. El-Taher, H. Yamaguchi, A. S. Alamoudi, M. M. Selim and S. A. El-Safty, Mesoporous organic–inorganic core–shell necklace cages for potentially capturing Cd2+ ions from water sources, ChemistrySelect, 2017, 2(21), 6135–6142 CrossRef CAS.
- A. M. Azzam, M. A. Shenashen, M. M. Selim, H. Yamaguchi, I. M. El-Sewify, S. Kawada, A. A. Alhamid and S. A. El-Safty, Nanospherical inorganic α-Fe core-organic shell necklaces for the removal of arsenic (V) and chromium (VI) from aqueous solution, J. Phys. Chem. Solids, 2017, 109, 78–88 CrossRef CAS.
- S. A. El-Safty, M. Khairy and M. Ismael, Visual detection and revisable supermicrostructure sensor systems of Cu(II) analytes, Sens. Actuators, B, 2012, 166, 253–263 CrossRef.
- S. A. El-Safty, S. Abdellatef, M. Ismael and A. Shahat, Optical nanosphere sensor based on shell-by-shell fabrication for removal of toxic metals from human blood, Adv. Healthcare Mater., 2013, 2, 854–862 CrossRef CAS.
- S. Jafari, H. Derakhshankhah, L. Alaei, A. Fattahi, B. S. Varnamkhasti and A. A. Saboury, Mesoporous silica nanoparticles for therapeutic/diagnostic applications, Biomed. Pharmacother., 2019, 109, 1100–1111 CrossRef CAS.
- B. Singh, J. Na, M. Konarova, T. Wakihara, Y. Yamauchi, C. Salomon and M. B. Gawande, Functional mesoporous silica nanomaterials for catalysis and environmental applications, Bull. Chem. Soc. Jpn., 2020, 93(12), 1459–1496 CrossRef CAS.
- I. M. El-Sewify, M. A. Shenashen, A. Shahat, M. M. Selim, M. M. Khalil and S. A. El-Safty, Sensitive and selective fluorometric determination and monitoring of Zn2+ ions using supermicroporous Zr-MOFs chemosensors, Microchem. J., 2018, 139, 24–33 CrossRef CAS.
- A. Thomas, R. Ahamed and M. Prakash, Effect of functional group in the zeolitic imidazolate framework for selective CH4/CO and CO/N2 separation: A theoretical study, Mater. Lett., 2021, 303, 130575 CrossRef CAS.
- T. W. Clarkson, L. Magos and G. J. Myers, The toxicology of mercury−current exposures and clinical manifestations, N. Engl. J. Med., 2003, 349, 1731–1737 CrossRef CAS.
- T. K. Pal, D. De and P. K. Bharadwaj, Engineering the Confined Space of MOFs for Heterogeneous Catalysis of Organic Transformations, Chemical Reactivity in Confined Systems: Theory, Modelling and Applications, 2021, pp. 293–333 Search PubMed.
- I. M. El-Sewify, A. Radwan, A. Shahat, M. F. El-Shahat and M. M. Khalil, Superior adsorption and removal of aquaculture and bio-staining dye from industrial wastewater using microporous nanocubic Zn-MOFs, Microporous Mesoporous Mater., 2022, 329, 111506 CrossRef CAS.
- C. Zhu, G. Yang, H. Li, D. Du and Y. Lin, Electrochemical sensors and biosensorsbased on nanomaterials and nanostructures, Anal. Chem., 2015, 87, 230–249 CrossRef CAS PubMed.
- M. K. Nazeeruddin, D. Di Censo, R. Humphry Baker and M. Grätzel, Highly selective and reversible optical, colorimetric, and electrochemical detection of mercury (II) by amphiphilic ruthenium complexes anchored onto mesoporous oxide Films, Adv. Funct. Mater., 2006, 16, 189–194 CrossRef CAS.
- E. Palomares, R. Vilar and J. R. Durrant, Heterogeneous colorimetric sensor for mercuric salts, Chem. Commun., 2004, 4, 362–363 RSC.
- S. K. Sharma, N. Kaur, J. Singh, A. Singh, P. Raj, S. Sankar, D. Y. Kim, N. Singh, N. Kaur and H. Singh, Salen decorated nanostructured ZnO chemosensor for the detection of mercuric ions (Hg2+), Sens. Actuators, B, 2016, 232, 712–721 CrossRef CAS.
- N. A. Azmi, S. H. Ahmad and S. C. Low, Detection of mercury ions in water using a membrane-based colorimetric sensor, RSC Advances, 2018, 8(1), 251–261 RSC.
- S. Yu, W. Li, Y. Fujii, T. Omura and H. Minami, Fluorescent spherical sponge cellulose sensors for highly selective and semiquantitative visual analysis: Detection of Hg2+ and Cu2+ ions, ACS Sustainable Chem. Eng., 2019, 7(23), 19157–19166 CrossRef CAS.
- S. Boonruang, P. Naksen, W. Anutrasakda, K. Chansaenpak, V. Promarak, R. Saenmuangchin, C. Phechkrajang and P. Jarujamrus, Use of nitrogen-doped amorphous carbon nanodots (N-CNDs) as a fluorometric paper-based sensor: a new approach for sensitive determination of lead (ii) at a trace level in highly ionic matrices, Anal. Methods, 2021, 13(32), 3551–3560 RSC.
- J. Wu, B. Kwon, W. Liu, E. V. Anslyn, P. Wang and J. S. Kim, Chromogenic/fluorogenic ensemble chemosensing systems, Chem. Rev., 2015, 115, 7893–7943 CrossRef CAS.
- M. H. Lee, J. S. Kim and J. L. Sessler, Small molecule-based ratiometric fluorescence probes for cations, anions, and biomolecules, Chem. Soc. Rev., 2015, 44, 4185–4191 RSC.
- C. Zong, K. Ai, G. Zhang, H. Li and L. Lu, Dual-Emission Fluorescent Silica Nanoparticle-Based Probe for Ultrasensitive Detection of Cu2+, Anal. Chem., 2011, 83, 3126–3132 CrossRef CAS PubMed.
- I. M. El-Sewify, M. A. Shenashen, A. Shahat, H. Yamaguchi, M. M. Selim, M. M. Khalil and S. A. El-Safty, Dual colorimetric and fluorometric monitoring of Bi3+ ions in water using supermicroporous Zr-MOFs chemosensors, J. Lumin., 2018, 198, 438–448 CrossRef CAS.
- I. M. El-Sewify, M. A. Shenashen, A. Shahat, H. Yamaguchi, M. M. Selim, M. M. Khalil and S. A. El-Safty, Ratiometric Fluorescent Chemosensor for Zn2+ Ions in Environmental Samples Using Supermicroporous Organic-Inorganic Structures as Potential Platforms, ChemistrySelect, 2017, 2(34), 11083–11090 CrossRef CAS.
- I. M. El-Sewify and M. M. Khalil, Mesoporous nanosensors for sensitive monitoring and removal of copper ions in wastewater samples, New J. Chem., 2021, 45(5), 2573–2581 RSC.
- A. Kongasseri, N. K. Sompalli, B. C. V. S. Rao, S. Nagarajan, A. M. Mohan and P. Deivasigamani, Solid-state optical sensing of ultra-trace Hg2+ ions using chromoionophoric probe anchored silica monolithic architectures, Sens. Actuators, B, 2020, 321, 128558 CrossRef CAS.
- A. Radwan, I. M. El-Sewify, A. Shahat, H. ME Azzazy, M. MH Khalil and M. F. El-Shahat, Multiuse Al-MOF Chemosensors for Visual Detection and Removal of Mercury Ions in Water and Skin-Whitening Cosmetics, ACS Sustainable Chem. Eng., 2020, 8(40), 15097 CrossRef CAS.
- A. Shahat and S. Trupp, Sensitive, selective, and rapid method for optical recognition of ultra-traces level of Hg(II), Ag(I), Au(III), and Pd(II) in electronic wastes, Sens. Actuators, B, 2017, 245, 789–802 CrossRef CAS.
- M. A. Shenashen, E. A. Elshehy, S. A. El-Safty and M. Khairy, Visual monitoring and removal of divalent copper, cadmium, and mercury ions from water by using mesoporous cubic Ia3d aluminosilica sensors, Sep. Purif. Technol., 2013, 116, 73–86 CrossRef CAS.
- A. Shahat, H. M. Hassan and H. M. Azzazy, Optical metal-organic framework sensor for selective discrimination of some toxic metal ions in water, Anal. Chim. Acta, 2013, 793, 90–98 CrossRef CAS PubMed.
- A. Radwan, I. M. El-Sewify, A. Shahat, M. F. El-Shahat and M. M. Khalil, Decorated nanosphere mesoporous silica chemosensors for rapid screening and removal of toxic cadmium ions in well water samples, Microchem. J., 2020, 156, 104806 CrossRef CAS.
- A. Xuanxuan, Q. Tan, S. Pan, H. Liu and X. Hu, A turn-on luminescence probe based on amino-functionalized metal-organic frameworks for the selective detections of Cu2+, Pb2+ and pyrophosphate, Spectrochim. Acta, Part A, 2021, 247, 119073 CrossRef PubMed.
- Y. Kuiyu and L. Zhang, Embedding dual fluoroprobe in metal-organic frameworks for continuous visual recognition of Pb2+ and PO43− via fluorescence'turn-off-on'response: Agar test paper and fingerprint, J. Hazard. Mater., 2020, 389, 122141 CrossRef PubMed.
- X. Shifen, L. Zhan, C. Hong, X. Chen, X. Chen and M. Oyama, Metal–organic framework-5 as a novel phosphorescent probe for the highly selective and sensitive detection of Pb (II) in mussels, Sens. Actuators, B, 2020, 308, 127733 CrossRef.
- B. Dey, R. Saha and P. Mukherjee, A luminescent-water soluble inorganic co-crystal for a selective pico-molar range arsenic(iii) sensor in water medium, ChemComm, 2013, 49, 7064–7066 RSC.
- A. Shahat, M. A. Hassan, H. M. E. Azzazy, M. Hosni and Md. R. Awual, Novel nano-conjugate materials for effective arsenic(V) and phosphate capturing in aqueous media, Chem. Eng. J., 2018, 331, 54–63 CrossRef CAS.
|
This journal is © The Royal Society of Chemistry 2022 |