DOI:
10.1039/D4TB00743C
(Review Article)
J. Mater. Chem. B, 2024,
12, 7463-7479
Magneto-responsive biocomposites in wound healing: from characteristics to functions
Received
5th April 2024
, Accepted 30th June 2024
First published on 4th July 2024
Abstract
The number of patients with non-healing wounds continuously increases, and has become a prominent societal issue that imposes a heavy burden on both patients and the entire healthcare system. Although traditional dressings play an important role in wound healing, the complexity and diversity of the healing process pose serious challenges in this field. Magneto-responsive biocomposites, with their excellent biocompatibility, remote spatiotemporal controllability, and unique convenience, demonstrate enticing advantages in the field of wound dressings. However, current research on magneto-responsive biocomposites as wound dressings lacks comprehensive and in-depth reviews, which to some extent, restricts the deeper understanding and further development of this field. Based on this, this paper reviews the latest advances in magnetic responsive wound dressings for wound healing. First, we review the process of skin wound healing and parameters for assessing repair progress. Then, we systematically discuss the preparation strategies and unique characteristics of magneto-responsive biocomposites, focusing on magneto-induced orientation, magneto-induced mechanical stimulation, and magnetocaloric effect. Subsequently, this review elaborates the multiple mechanisms of magneto-responsive biocomposites in promoting wound healing, including regulating cell behavior, enhancing electrical signal, controlling drug release, and accelerating tissue reconstruction. Finally, we further propose the development direction and future challenges of magnetic responsive biomaterials as wound dressings in clinical application.
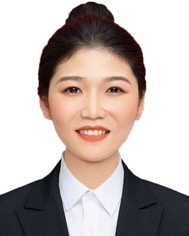
Lili Hao
| Dr Lili Hao, received her PhD from the University of Science and Technology of China in June 2021, and currently serves as an associate professor at Nanjing Tech University. She is primarily engaged in the development of magneto-responsive biomaterials and the mechanism investigation of magneto-promoted osteogenesis, and her current research focuses on the fabrication and application research of novel intelligent responsive hydrogels and engineered cells. She has published over 10 papers in high-impact academic journals such as Adv. Funct. Mater., and J. Mater. Sci. Technol. |
1. Introduction
Skin, as the largest organ of the human body, not only serves multiple core functions such as sensing external stimulation, regulating body temperature, and metabolism, but also acts as the primary defense line safeguarding human health and safety.1–4 However, because skin has direct contact with the external environment, it is also one of the tissues most susceptible to injury.5,6 Every year, hundreds of millions of people suffer from skin trauma caused by burns, abrasions, surgeries, and other factors.7,8 The healing process of wounds varies depending on their size, depth, and the extent of damage on the epidermis and dermis. Additionally, other factors such as systemic malnutrition, immune compromise, aging, chronic stress, and complications often lead to poor healing or prolonged healing time.9 The healing process experienced by skin trauma patients is often challenging, with significant physical pressure and pain, as well as potential psychological issues, social isolation, and decreased productivity, resulting in a heavy economic burden on society.7,10 Therefore, treatment and rehabilitation are crucial, requiring collective attention and support from individuals, healthcare professionals, and policymakers to provide comprehensive care and support for patients. This has profound significance for the entire healthcare system, economic development, and social stability.
In recent years, with the continuous advancement of medical technology, the research and application of wound dressings have gradually gained widespread attention.11–15 Wound dressings, as materials designed to cover wounds to protect them from external infections, can also serve as scaffolds to guide the reorganization of skin cells and facilitate the infiltration and integration of host tissues, thereby accelerating the wound healing process.16,17 To date, researchers have developed various forms of wound dressings, such as gauze, hydrogels, and foams, to meet diverse clinical needs.18–26 However, despite the wide variety of existing wound dressing types, there are still unmet needs in practical applications. For instance, most available dressings lack the ability to adaptively adjust their performance based on the progress of wound healing, which greatly limits their therapeutic efficacy. Additionally, there is a lack of wound dressings tailored for specific locations, such as joints and neck, which are subjected to high tensile stress. Furthermore, the pain issue experienced by patients during dressing changes has not received sufficient attention and resolution. With the deepening understanding of wound healing mechanisms, the demands for wound dressings are gradually increasing. Therefore, the development of smart-responsive, adaptive-regulating, and site-specific wound dressings has become particularly urgent.27
Smart wound dressings are advanced medical devices that utilize built-in sensors and/or smart materials to continuously monitor wound conditions or environmental changes and respond effectively and appropriately in real-time.28–31 Compared to traditional wound dressings, smart wound dressings offer higher flexibility and adaptability, and can meet the needs of different stages of wound healing better. Smart wound dressings not only interact with the wound environment and provide continuous wound monitoring but also adjust treatment strategies based on the changes in wound status, controlling wound infections, reducing complications, and promoting autonomous wound healing.32,33 Various types of smart wound dressings have been developed, including stimulus-responsive wound dressings, biomechanical wound dressings, self-repairing wound dressings, and self-monitoring wound dressings.34–38 In stimulus-responsive wound dressings, compared to pH, light, heat, and electricity stimulation, magnetic stimulation possesses convenient remote temporal control, non-invasiveness, strong tissue penetration, and excellent physicochemical properties, showing great potential in the field of skin wound healing.39,40 Researchers have confirmed that magneto-responsive biocomposites can effectively promote skin wound healing through mechanisms such as modulating cell behavior, magnetic hyperthermia sterilization, and controlled drug release.41–44 However, a comprehensive review of magneto-responsive biocomposites as wound dressings has yet to be reported.
This review comprehensively outlines the current status and progress of wound dressings made from magneto-responsive biocomposites in the field of skin wound healing. Firstly, the complex process of skin wound healing and the key parameters for assessing its progress are introduced. Secondly, the preparation strategies of magneto-responsive biocomposites are systematically classified. Their unique characteristics, particularly magneto-induced orientation, magneto-induced mechanical stimulation, and magnetocaloric effect, are thoroughly discussed. Subsequently, this review elucidates the multiple mechanisms by which magneto-responsive biocomposites promote wound healing, including conduction regulating cell behavior, enhancing electrical signal transmission, controlling drug release, and accelerating tissue reconstruction. Finally, challenges and future prospects faced by magneto-responsive wound dressings in the field of translational medicine are discussed, aiming to deepen understanding of this area and stimulate new research directions (Scheme 1).
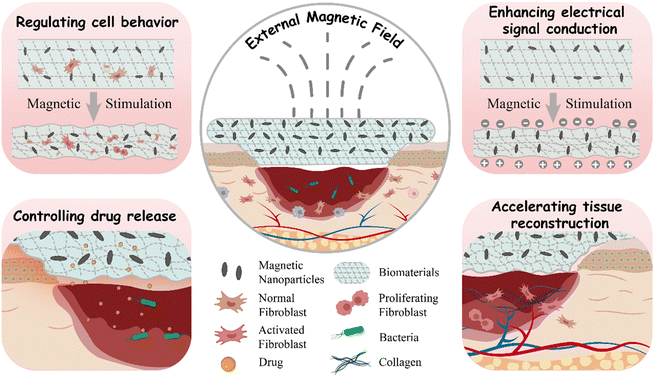 |
| Scheme 1 Schematic illustrations of the pathways through which magneto-responsive biocomposites promote wound healing. | |
2. Wound healing process and evaluation parameters
2.1 Wound healing process
Wound healing is a complex natural process involving tissue, cells, growth factors, and cytokines.45 Its core objective is to restore tissue integrity and stability.46,47 This repair process consists of four interconnected stages, as described below (Fig. 1).
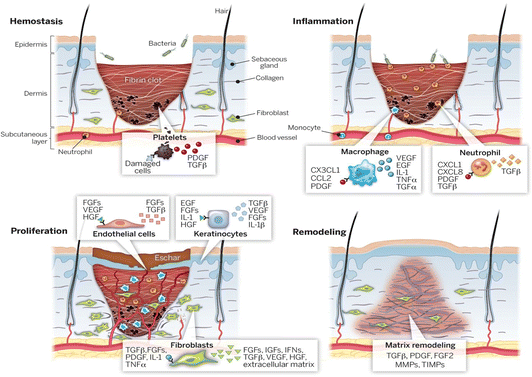 |
| Fig. 1 Four stages of wound healing: hemostasis, inflammation, proliferation, and remodeling.45 Copyright 2014, American Association for the Advancement of Science. | |
Hemostasis phase.
Hemostasis is a key initial step in wound healing. In this stage, injured blood vessels constrict, and platelets aggregate to form a platelet plug that stops bleeding. Simultaneously, activated clotting factors initiate the coagulation cascade, forming fibrin clots that stop bleeding and trigger inflammation. As coagulation progresses, fibrinogen converts into fibrin, creating a mesh that entraps platelets and cells to form a stable thrombus and initiate the inflammatory immune response.5,48
Inflammation phase.
The inflammatory stage is the second phase of wound healing, representing the body's defense response following vascular injury. During this stage, damaged tissues release inflammatory mediators that increase vasodilation and vascular permeability, allowing inflammatory cells to infiltrate the wound and promote cleansing. However, excessive inflammation may cause complications such as chronic wound stagnation, making it important to modulate the inflammatory response.31,49
Proliferation phase.
As inflammation subsides, cells begin to fill the wound through proliferation and migration. Epithelial cells cover the wound by proliferating and migrating, restoring skin integrity. Fibroblasts and myoblasts synthesize and deposit extracellular matrix (ECM) proteins, strengthening the wound structure. Notably, a balance between ECM deposition and degradation is essential to prevent the formation of abnormal scars.50,51
Remodeling phase.
The remodeling stage is the final phase of wound healing, marked by the disappearance of capillary, macrophages, and fibroblasts through apoptosis or other processes. Concurrently, collagen fiber content increases, with type I collagen replacing type III collagen. The damaged tissue is restored to its normal structure, with enhanced tensile strength.52
Overall, all stages of wound healing are exceedingly intricate, and inhibition at any stage can lead to chronic wounds. Utilizing bioactive materials with various properties can enhance the effectiveness of wound healing and skin regeneration.
2.2 Evaluation parameters
Due to the complexity of the wound healing process, there are diverse factors influencing its healing, and the parameters for evaluating its repair are also numerous.
Inflammation-related cytokines.
Moderate inflammation facilitates the recruitment of inflammatory cells to clear damaged tissue, eradicate pathogens, and initiate repair mechanisms.53 However, excessive inflammatory action can harm surrounding tissues and delay healing. Inflammatory factors such as TNF-α, TGF-β, IL-1, IL-6, and CD68 are crucial indicators for assessing the wound healing process.54–57
Granulation tissue.
Granulation tissue provides nutrients and oxygen to the wound and helps clear pathogens and dead tissue.20,58 However, thicker granulation tissue can lead to scar formation. Assessing the extent of granulation tissue formation can provide crucial insights into the wound healing.
Re-epithelialization.
The complete regeneration of the epidermal layer helps prevent pathogen invasion and restores skin function at the wound area.59,60 As a pivotal indicator of wound healing, re-epithelialization can be assessed by detecting factors such as epidermal growth factor (EGF).61
Collagen deposition.
Collagen deposition can enhance the mechanical strength of repaired tissue. Studies have indicated that increased and organized collagen deposition improves wound healing efficiency.62–65 Consequently, assessing collagen deposition is of great concern for evaluating wound healing progress and predicting outcomes.
Angiogenesis.
Neovascularization supplies nutrients and oxygen to damaged tissues, promotes cell migration and proliferation, and modulates inflammatory. It also helps clear waste from the wound site, supporting rapid healing. Vascular endothelial growth factor (VEGF) and CD31 are closely associated with angiogenesis.66–68
Scarring.
Scarring directly impacts wound healing outcomes and patients’ quality of life. Highly active fibroblasts can promote healing, but they may also lead to abnormal collagen synthesis and scarring.69–72 Therefore, modulating the formation of extracellular matrix and collagen during healing is essential to prevent scar formation.
In addition, several other indicators can be used to assess wound healing quality. For instance, wound closure rate can visually assess healing speed, while hair follicle regeneration can effectively evaluate functional recovery of the wound site.63,73,74
3. Magneto-responsive biocomposites and their characteristics
3.1 Fabrication strategy of magneto-responsive biocomposites
Magneto-responsive biocomposites typically consist of biocompatible materials and magnetic components.75 These composites are widely designed for applications in medical fields such as tissue engineering, drug delivery, and medical imaging.76–78 The magnetic components in magneto-responsive biocomposites can be micron-sized or nano-sized magnetic particles (e.g., iron oxide, nickel, cobalt), while the matrix materials can be artificial synthesized biocompatible polymers (e.g., PLGA, PCL, PEG) or natural polymer materials (e.g., gelatin, hyaluronic acid, chitosan).79,80 The magnetic particles within the composites respond to external magnetic fields to manipulate the position, morphology, and functionality of the biocomposites. The performance of the magnetic biocomposites depends on the type and structure of the matrix material, as well as the size, distribution, orientation of magnetic particles, and the interaction between the matrix material and magnetic particles.76 When considering in vivo applications, the size of nanoparticles needs to be constrained within a certain range, typically between 10 nm and 100 nm, to ensure it can cross biological barriers to target specific tissue or be metabolized. Hence, it is commonly referred to as magnetic nanoparticles (MNPs).81–83 Various methods have been developed for the preparation of magneto-responsive biocomposites, including blending, in situ precipitation, and grafting-onto methods, as illustrated in Fig. 2.
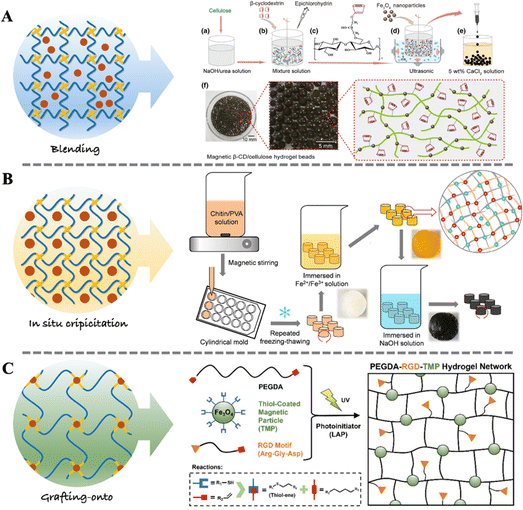 |
| Fig. 2 Fabrication strategy of magneto-responsive biocomposites. (A) Schematic steps for the synthesis of magnetic β-CD/cellulose hydrogel using the blending method.84 Copyright 2019, Springer Netherlands. (B) Schematic steps for the synthesis of chitin/PVA-based magnetic hydrogel using the in situ precipitation method.85 Copyright 2019, Elsevier. (C) Schematic steps for the synthesis of PEGDA-RGD-TMP hydrogel using the grafting-onto method.43 Copyright 2023, Wiley. | |
Blending method.
Blending is a common method for preparing composite materials. In this approach, MNPs and biopolymers are separately prepared. Subsequently, they are dissolved in suitable solvents, and then mixed together using physical blending, mechanical stirring or ultrasonic treatment to achieve uniform dispersion.86 This method is straightforward and applicable to the preparation of most composite materials. Lin et al. dissolved cellulose in NaOH/urea aqueous solution, then added beta-cyclodextrin followed by dropwise addition of epichlorohydrin as a crosslinker (Fig. 2A). After sufficient reaction, commercially purchased Fe3O4 nanoparticles were added and uniformly dispersed. Finally, gradual addition of CaCl2 solution resulted in the formation of spherical magnetic beads. These spherical magnetic beads exhibit rapid swelling–deswelling properties under an external magnetic field and enable remote control of gradual drug release.84 Liu et al. fabricated poly(N-isopropylarcylamide) (PNIPAAm)/Fe2O3 magnetic hydrogels using the blending method. The prepared ferrofluid was added to NIPAAm solution and hydrogels were formed using a suspension polymerization method, which were readily moved and collected through an external MF.87
The blending method offers advantages such as low cost since no special equipment and materials are required. Additionally, it is independent from complicated processes and can be easily extended to large-scale production. Furthermore, blending can be employed to fabricate composites with good biocompatibility. However, mere physical mixing may result in poor dispersion among materials, and the precise control of interface properties may be compromised. When there is disparity in the solubility properties between MNPs and polymers, the blending method becomes unsuitable.
In situ precipitation method.
The basic principle of the in situ precipitation method for preparing biocomposites involves utilizing biopolymers as chemical reactors, enabling the in situ precipitation of metal ions with precipitating agents within the polymer network to form nanoparticles.88 This method allows for control of the morphology, composition, and structure of the precipitate by adjusting reaction conditions such as temperature, pH, reaction time, etc., thereby enabling modulation of the properties of the composites. Liao et al. first mixed polyvinyl alcohol (PVA) solution with chitosan solution in equal proportions, followed by freezing at −20 °C for 8 h and then thawing at room temperature for 2 h (Fig. 2B). After three freeze–thaw cycles, chitosan/PVA hydrogel was obtained. The clean hydrogel was directly immersed in FeCl2/FeCl3 aqueous solution for 4 h, then transferred to NaOH solution for another 4 h, allowing the in situ generation of Fe3O4 NPs within the hydrogel. Subsequently, magnetic chitosan/PVA/Fe3O4 hydrogel was obtained.85 Similarly, Gao et al. immersed disk-shaped sodium 2-acrylamido-2-methyl-1-propanesulfonate hydrogel (PNaAMPS) with negative charges in an iron solution to adsorb Fe2+ and Fe3+. Subsequently, it was transferred to a NaOH solution and soaked for 12 h to obtain needle-shaped, rod-shaped, or octahedral Fe3O4 nanocrystals. The shape of the nanocrystals depends on the concentration of the NaOH solution.89
The advantages of the in situ precipitation method include its wide applicability. It can be used to prepare various types of biocomposites. Importantly, in situ precipitation can introduce a large number of nanoparticles into the polymer network while ensuring their good dispersion.90 However, the in situ precipitation method requires strict reaction conditions, as deviations may disrupt the established polymer network. Additionally, the reaction process may generate waste liquid, necessitating additional post-reaction processing.
Grafting method.
Both in situ precipitation and blending methods are unable to ensure the dispersion stability of MNPs within the polymer matrix due to the absence of strong interaction (chemical bonding or physical adsorption) between them.91 The charm of the grafting-onto method lies in its capability to combine nanoparticles with the polymer network via either covalent or non-covalent bonds. Furthermore, MNPs can even be introduced into the polymer network as crosslinking agents, thereby enhancing the mechanical properties of the composites and the dispersion stability of MNPs. The steps before crosslinking are akin to those of the blending method, where MNPs and polymer materials need to be prepared separately. Subsequently, functional groups are grafted onto the surface of MNPs, thereby establishing strong bonding interactions with the polymer. Grafting-onto is a versatile method for preparing biocomposites, offering considerable potential for applications. Shou et al. incorporated acrylic acid-functionalized RGD peptide sequences into poly(ethylene glycol) diacrylate (PEGDA) polymer chains via ene reaction to improve the biocompatibility of the hydrogel. Magnetic particles coated with thiol (TMP) were then dispersed into the hydrogel network (i.e., PEGDA-RGD-TMP) to impart magnetic responsiveness to the hydrogel. Under ultraviolet irradiation, TMP was grafted onto the PEGDA backbone via thiol–ene click reaction, and the precursor solution of PEGDA-RGD-TMP quickly transformed into a solid gel (Fig. 2C). Compared to physically encapsulated groups, the degree of TMP leakage from the hydrogel was much weaker. The PEGDA-RGD-TMP hydrogel network exhibited excellent mechanical properties and maintained good biostability in acidic and alkaline environments. The hydrogel was filled with an interconnected porous network, providing ample space for nutrient diffusion, cell growth, and migration, while ensuring oxygen levels within the hydrogel. This hydrogel can play a multifunctional role in the process of wound healing.43 Injectable polysaccharide-based magnetic hydrogels based on HA and Fe3O4 were synthesised using MNP suspensions mixed with a bisphosphonate (BP) solution containing hyaluronic acid (HA). The BP chelating ligand in the resulting HA-BP derivative was coordinated with the iron atoms to form the final magnetic hydrogel.92
The advantage of the grafting-onto method lies in its ability to ensure the dispersion stability of MNPs as well as the adjustability of the biodegradability and mechanical properties of the biocomposites. However, the preparation process of this method is relatively complex, requiring high technical and processing requirements. This may lead to prolonged preparation cycles and increased production costs.
3.2 Characteristics of magneto-responsive biocomposites
Magneto-responsive biocomposites are advanced materials characterized by excellent responsiveness to magnetic fields and superior biocompatibility. Under the influence of a specific external magnetic field, these materials can rapidly and precisely respond, exhibiting unique physical or chemical property changes, such as magneto-induced orientation, magneto-induced mechanical stimulation and the magnetocaloric effect.
Magneto-induced orientation.
The most intuitive response of magnetic biocomposites to a magnetic field is the orientation of MNPs. This is not limited to the rotation of anisotropic magnetic nanoparticles (such as rod-shaped or plate-shaped particles) induced by an external magnetic field. For isotropic magnetic nanospheres, the orientation of induced magnetic dipoles by the external magnetic field leads to attractive forces between the nanospheres.93–97 And the attraction further results in the development of pearl-chain-like structures of MNPs aligned along the direction of the magnetic field. Magneto-induction orientation can be utilized in the preparation of biocomposites with highly anisotropic structures, exhibiting multiple direction-dependent characteristics such as anisotropic elastic modulus and swelling.98
In our previous review, we systematically described the preparation methods, properties, and biomedical applications of magnetic anisotropic biomaterials.40 A commonly used preparation method is inducing the alignment of magnetic nanoparticles, nanorods, or nanoplates in a specific direction using an external static magnetic field before the matrix material is shaped. Subsequently, this ordered structure is preserved during the solidification process of the matrix material (Fig. 3A). The magneto-induced anisotropic structure not only imparts anisotropic mechanical strength to biocomposites but also exhibits directional dependence in their magnetic heating effects. Additionally, the anisotropic structure can increase the conductivity or transparency of the biomaterial in a specific direction. Importantly, the ordered structure also provides directional cues for cell growth, facilitating the directional growth of neurons or muscle cells, thereby accelerating tissue repair or promoting tissue function recovery.
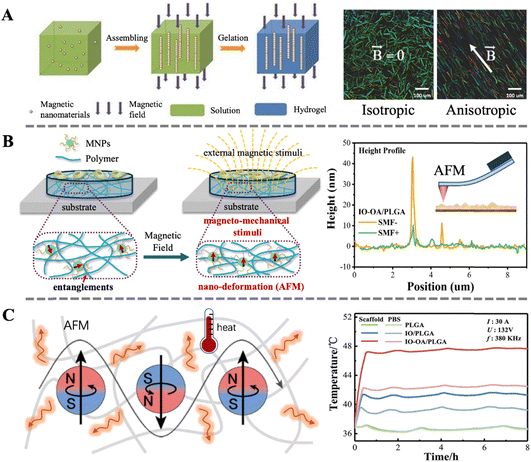 |
| Fig. 3 (A) Schematic illustration of the fabrication process of magnetically anisotropic hydrogels; depth color-coded images of 3D fibrin hydrogels containing PLGA-based magnetic fibers.40 Copyright 2023, Royal Society of Chemistry. (B) Schematic illustration of magneto-mechanical stimulation induced by the nano-deformation of magnetic biocomposites under a SMF; a height profile map from 5IO-OA/PLGA obtained via in situ AFM scanning.99 Copyright 2019, Royal Society of Chemistry. (C) Schematic illustration of the thermal effect of magnetic biocomposites under AMF; temperature changes of the scaffolds and PBS media under AMF.100 Copyright 2021, Wiley. | |
Magneto-induced mechanical stimulation.
It is well recognized that mechanical stimulation plays a pivotal role in regulating various physiological processes, ranging from embryonic development to tissue regeneration. Mechanical stimulation can activate intracellular signaling pathways, thereby influencing gene expression and cellular functions. The effects of mechanical stimulation on stem cells have also emerged as a focal point in the field of tissue engineering. Furthermore, mechanical stimulation can modulate tissue morphology and structure.101–103 However, directly applying appropriate mechanical stimulation to damaged tissues is often challenging, especially when the depth of tissue damage is significant. Controlling the intensity, frequency, and spatiotemporal characteristics of mechanical stimulation is also difficult. In this context, magnetic biocomposites undergo subtle deformations under the influence of external magnetic stimulation, thereby transmitting mechanical cues to cells or tissues.99,104 Magnetic-induced mechanical stimulation, compared to direct physical stimulation, offers a more sophisticated approach and allows precise control of mechanical stimulation by modulating the external magnetic field. Designing the matrix material of magnetic biocomposites as hydrogels and incorporating magnetic nanoparticles as crosslinkers can further enhance the deformation magnitude of the material under magnetic field exposure, thereby augmenting magnetic-driven mechanical stimulation.105,106
Using in situ scanning with atomic force microscopy, we observed for the first time the nanoscale deformation of magnetic bio-composite materials under a static magnetic field. Combined with the activation of mechanosensitive channel proteins (Pizeo1) in cells, we demonstrated the regulatory function of magneto-induced mechanical stimulation on cellular behavior (Fig. 3A).99 Subsequently, molecular dynamics simulations and in vitro experiments were used to investigate the effect of nanoparticle surface modification on the intensity of magneto-induced mechanical stimulation.104 Recent research indicates that magneto-induced mechanical stimulation can also promote skin wound healing through mechanisms such as promoting angiogenesis and tissue reconstruction.43 These findings lay a solid foundation for the widespread application of magneto-induced mechanical stimulation in the field of biomedicine.
Magnetocaloric effect.
When the external magnetic field is not static but alternative in high-frequency, MNPs will undergo Néel Brownian relaxation and generate heat, known as the magnetocaloric effect.107,108 This effect has been widely studied over the past decade, with one of the most extensively researched areas being magnetic hyperthermia therapy.109–111 A shock response in tumor cells can be induced by the heat released by MNPs, effectively treating cancer. Compared to other physical stimulation (such as light or sound), magnetic heating has no depth penetration limits and is not attenuated by tissues. Recently, the magnetocaloric effect has been more broadly applied in other biomedical fields, such as activation of heat-sensitive receptors, cellular signal transduction pathways involving gene expression and protein production, and magnetic heat-triggered substance delivery.110
The magnetocaloric effect can not only directly affect cells and tissues but also impact biomaterials. For instance, in our previous work, we utilized the magnetocaloric effect of MNPs to control the real-time temperature of PLGA scaffolds, thereby achieving controlled degradation of the scaffolds. The interaction between the grafted MNPs and the polymeric matrix is stronger than bare MNPs, leading to a more pronounced heat transfer effect and faster degradation rate (Fig. 3C).100 This result was further semi-quantitatively demonstrated through molecular dynamics simulations. Recent studies also indicate that the magnetocaloric effect can be utilized for deep sterilization or drug release in skin wound healing.44
4. Magneto-responsive biocomposites for enhancing wound healing
4.1 Magneto-responsive biocomposites for regulating cell behavior
Precise control of cellular behavior plays a central role in the process of skin wound healing. Biological processes such as cell proliferation, migration, differentiation, and apoptosis intertwine to form the complex process of skin regeneration and repair. Precise control of cellular behavior is crucial to ensuring rapid and complete repair of skin wounds.112–114 In recent years, with the rapid development of molecular biology and cell biology, researchers have gained a deeper understanding in regulating cellular behavior. By intervening in the function of membrane proteins and downstream signaling pathways, cell proliferation, migration, and differentiation can be effectively regulated, thereby accelerating the wound healing process.115,116 Among these, magnetic biocomposites, as a novel type of smart responsive biomaterial, demonstrate unique responsiveness under the influence of an external magnetic field due to the embedded magnetic particles, thereby achieving precise control of cellular behavior. They can not only alter the cellular microenvironment through physical stimulation but also can influence the interaction between cells and materials, guiding the directed cell growth and wound repair.117–119 This characteristic makes magneto-responsive biocomposites show vast potential in the field of skin wound healing, offering new possibilities for future medical treatments.
Zhu et al. utilized MNPs as a non-contact actuator, combined with hydrogel as the matrix material, to develop a novel dynamic adjustment and repair system, which they termed the magneto-responsive massage membrane (MMM). In this composite material, they designed reinforced fibers as a negative Poisson's ratio structure to enhance the mechanical strength of the hydrogel, while a high concentration of MNPs was designed in an umbrella-like structure to achieve a more uniform stress–strain distribution (Fig. 4A). The results indicate that the magnetic hydrogel can be activated and deformed to different amplitudes periodically using a dynamic magnetic system. During this process, the mechanical transduction signals of fibroblasts are activated. Subsequently, the expression of α-smooth muscle actin (α-SMA) increases, indicating the differentiation of fibroblasts towards myofibroblast under the stimulation. The expression of the typical epithelial marker Keratin 14 (K14) also increases simultaneously. However, prolonged (over 6 h) magnetic-mechanical stimulation instead leads to cell apoptosis. This study fully demonstrates the potential application of appropriate magneto-mechanical stimulation in regulating cellular behavior and promoting wound healing.41 Moreover, Ganguly et al. utilized the magnetic response of cellulose nanocrystals (CNCs) embedded in alginate-silk fibroin (ASF) matrix under low-intensity magnetic fields to enable the fabrication of magnetically aligned, anisotropic three-dimensional wound healing scaffolds (Fig. 4B). The anisotropic structure significantly enhanced the mechanical strength of the scaffolds and promoted the proliferation of skin fibroblasts, keratinocytes, and endothelial cells, along with the alignment of their cytoskeletons. Concurrently, the expression of keratins (KRT1, KRT5, KRT10, and KRT14) in HaCaT cells was notably affected, while the expression of platelet endothelial cell adhesion molecule (PECAM), vascular endothelial growth factor (VEGF), and vascular endothelial cadherin (VE-CAD) in human umbilical vein endothelial cells (HUVEC) increased significantly. Further in vivo experiments demonstrated that the anisotropic biomimetic scaffold structure effectively accelerated wound closure. These studies highlight the unique advantages of magneto-responsive biocomposites in modulating cellular biology and promoting wound healing.120
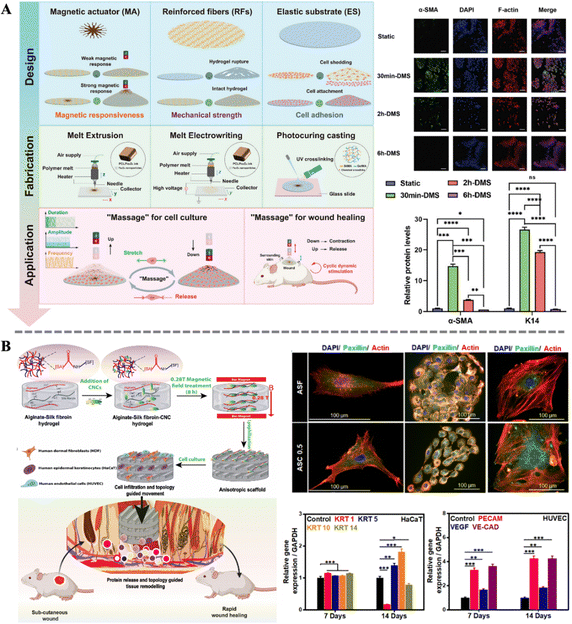 |
| Fig. 4 (A) Design and fabrication of MMM and graphic illustration of the massage concept; effect of stimulus duration on the expression of wound healing-related proteins α-SMA and K14.41 Copyright 2024, Wiley. (B) Schematic of the preparation of the anisotropic scaffold and the topology guided wound healing; immunofluorescence staining of HDF (left), HaCaT (middle), and HUVEC (right); qRT-PCR analysis of marker genes in the HaCaT and HUVEC.120 Copyright 2022, Elsevier. | |
4.2 Magneto-responsive biocomposites for enhancing electrical signal conduction
The skin healing process involves a series of complex biological reactions, in which electrical signals play a crucial role in cell proliferation and differentiation, as well as tissue maturation and maintenance. Electrical signal transduction, as one of the important means of intercellular information transmission, converts external stimulation into intracellular electrical signals through ion channels or receptors on the cell membrane, thereby triggering corresponding biological effects.121–124 During the skin wound healing process, electrical signal transduction can rapidly respond to injury signals, activate intracellular signaling pathways, promote cell proliferation, migration, and differentiation, thereby accelerating wound healing. Therefore, the reconstruction of endogenous bioelectric signals in damaged tissue has become a research hotspot. Traditional electrical stimulation methods rely on invasive exogenous devices, making it difficult to accurately adjust the dose and spatial range of the physical stimulation. And electrodes are prone to induce inflammation and infection, hindering wound healing and prolonging treatment time.125 To overcome these drawbacks, the development of smart biocomposites for bioelectric stimulation has become a feasible strategy.
Combining magneto-mechanical stimulation with piezoelectric materials is a clever design. Zhang et al. designed an aligned magnetoelectric nanofibrous membrane system (containing poly-L-lactic acid (PLLA) and cobalt ferrite (CFO)) with magneto-mechano-electric cascade stimulation (Fig. 5A). Excellent magnetic properties, enhanced crystallinity, and resulting piezoelectric responsiveness were achieved by simply adjusting the loading of magnetic nanoparticles. Specifically, under the influence of an external magnetic field, the magnetic response in PLLA/CFO is amplified, leading to significant deformation and subsequent generation of both mechanical and electrical signals. Importantly, this performance can be finely tuned to match the actual wound healing process, facilitating accelerated repair of full-thickness skin defects in rats. This work presents a novel strategy for reconstructing complex biophysical microenvironments remotely.42 Apart from the magneto-mechano-electric effect, the increase in conductivity due to magnetic anisotropy can also be utilized to enhance intercellular electrical signal transmission. Inspired by the highly ordered hierarchical structure of natural soft tissues, Li et al. introduced ordered magneto-electric nanosheets (MPG) into gelatin-oxidized dextran (Gel-ODex) hydrogels, imparting anisotropy to the hydrogel (Fig. 5B). The directed arrangement of MPG within the hydrogel formed an ordered conductive pathway. Then, the Gel-ODex-MPG hydrogel exhibited anisotropic mechanical and electrical properties under near-infrared light (NIR, 808 nm). By optimizing the content of MPG, the electrical conductivity of the hydrogel material can be maximized. Moreover, anisotropic Gel-ODex-MPG conductive hydrogels can accelerate wound healing and sensitively detect human movement, aiding in monitoring wound status and rehabilitation training during the wound recovery process. This provides insights into the development of multifunctional anisotropic hydrogels integrated for wound healing and personal health monitoring.126
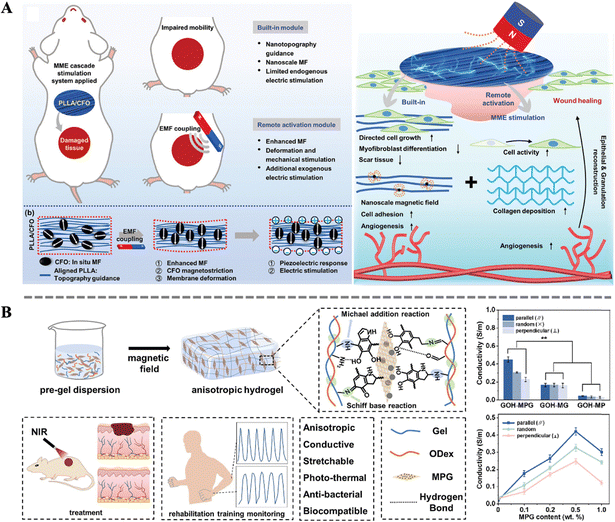 |
| Fig. 5 (A) Schematic illustration of the design of an MME cascade stimulation system constructed by the coupled PLLA/CFO nanofibrous membrane and the mechanism of the system for promoting wound healing.42 Copyright 2023, Wiley. (B) Schematics of the preparation of the anisotropic GOH-MPG conductive hydrogel and its medical application; effect of rGO, PDA and MPG on the conductive performance of the hydrogels.126 Copyright 2023, Elsevier. | |
4.3 Magneto-responsive biocomposites for controlling drug release
In the wound healing process, any infection can potentially delay or disrupt the repair process. Therefore, sterilization is a crucial aspect in ensuring smooth wound healing. Effectively eliminating pathogens around the wound can reduce the risk of infection, protect the wound from further inflammation, and provide a clean healing environment. Additionally, sterilization helps alleviate symptoms such as swollen and pain, and accelerates the regeneration of wound tissues.127–129 Researchers have developed various novel drug molecules and antibacterial materials that not only enhance bactericidal efficacy but also enable non-invasive real-time monitoring of the treatment process, effectively avoiding irritation and damage to normal tissues.130 The controlled release of these drug molecules poses the next challenge for researchers. Among various strategies, magnetically induced drug delivery stands out for its remote and spatiotemporal controllability. Specifically, MNPs embedded in biocomposites can rapidly respond to external magnetic stimulation and generate the magnetocaloric effect. This property enables MNPs to achieve an “on–off” switching function.98 Combined with the modulation of the magnetic field, drug release can be precisely controlled. Magnetic hyperthermia-triggered drug delivery systems not only integrate multiple thermo-responsive interactions, such as molecular contraction, dissociation, and cleavage, but also demonstrate significant advantages in the precision and efficiency of drug release. This innovative drug delivery method not only provides a safer and more efficient treatment for skin wound healing but also offers new solutions to drug delivery issues in other medical fields.
Yang et al. designed a 2D MXene-based hydrogel system consisting of MXene encapsulated MNPs and poly(N-isopropylacrylamide)-alginate double crosslinking hydrogels (Fig. 6A). By further loading Ag NPs a novel smart drug carrier was constructed. Under near-infrared light irradiation and alternating magnetic field, the system's temperature could rapidly increase, triggering the thermosensitive properties of the hydrogel, thereby releasing Ag NPs in a controlled manner. The system's multifaceted responsiveness and controllable drug delivery capability can reduce drug side effects and promote wound healing processes. Its practicality was demonstrated through its application in treating full-thickness skin wounds and subcutaneous infected wounds in a rat model.131 Coincidentally, Chung et al. also conducted research on magnetically controlled drug release. By combining nitric oxide (NO) carrier ([Fe(μ-S-thioglycerol(NO)2)]2) with porous Fe3O4@C derived from a metal–organic framework (MOF), further encapsulating them in thermo-responsive PLGA microspheres, assembly of MagNORM was achieved. Continuous application of alternating magnetic fields to the material enabled ON/OFF control of NO release, thereby triggering bactericidal effects against Gram-positive Staphylococcus aureus (S. aureus) and Gram-negative Escherichia coli (E. coli). Moreover, after the withdrawal of the alternating magnetic field, stable release of NO from MagNORM promoted subsequent collagen formation and mouse wound healing. These results indicate the promising performance of magnetically controlled drug release in deep chronic wound healing and related biomedical fields.132
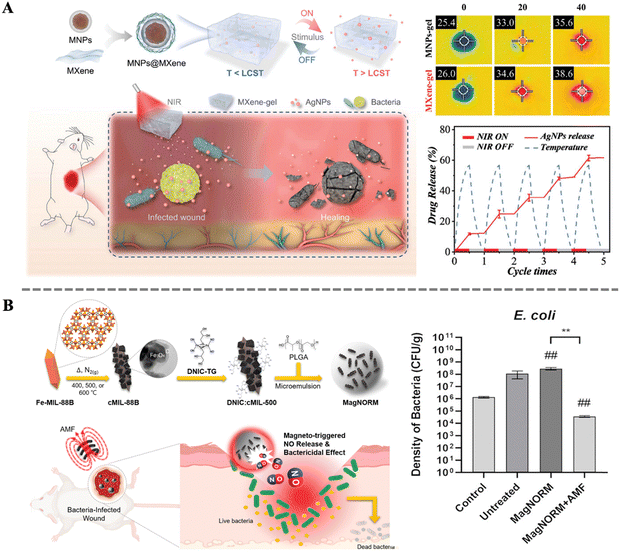 |
| Fig. 6 (A) Schematic illustration of the preparation and application of a stimulation-responsive MXene-based hydrogel system; thermal images of the hydrogel before and after NIR irradiation; controllable drug release abilities of the MXene-based hydrogel loaded with AgNPs under NIR.131 Copyright 2022, Wiley. (B) Schematic illustration for the preparation of MagNORM and for magneto-triggered bactericidal effect and treatment of a bacteria-infected cutaneous wound; density of bacteria on the cutaneous wounds treated with different samples.132 Copyright 2022, American Chemical Society. | |
4.4 Magneto-responsive biocomposites for accelerating tissue reconstruction
The importance of tissue reconstruction in the wound healing process is self-evident. Through a series of intricate and complex mechanisms, tissue reconstruction comprehensively assists wound repair.133 Firstly, tissue reconstruction can readjust the structure of damaged tissues, making them more orderly and stable, thereby laying a solid foundation for wound healing. Secondly, this process promotes the deposition of collagen, enhancing the stability of the wound edges and improving the resilience and elasticity of the skin. Furthermore, tissue reconstruction accelerates the process of re-epithelialization, allowing epithelial cells to quickly cover the wound surface and reduce the risk of infection. Simultaneously, neovascularization is also crucial for wound healing, as it not only provides ample blood supply to the wound area but also accelerates the delivery of nutrients and clearance of waste products.134 Finally, tissue reconstruction improves the local microenvironment, reducing the secretion of pro-inflammatory factors and infiltration of inflammatory cells, further promoting epidermal regeneration and wound closure.135–137 Failure in tissue reconstruction may lead to delayed repair processes, difficulty in wound closure, and even scarring, causing long-term distress and pain to patients. Therefore, in-depth research and understanding of the process and mechanisms of tissue reconstruction are of great significance in improving the quality of wound healing and reducing complications.
Zhang et al. designed a gel-based multifunctional dressing composed of two types of microfibers. One microfiber contains an Fe3O4@SiO2 interpenetrating polymer network, while the other microfiber carries human umbilical cord mesenchymal stem cells (Fig. 7A). The dressing significantly promotes cell proliferation and tissue reconstruction, improving re-epithelialization, granulation tissue formation, and collagen deposition at the wound site. Additionally, its unique woven structure provides additional capabilities such as absorbing exudate, providing a moist environment, and retaining therapeutic cells. In vivo experiments demonstrate that the gel-based multifunctional dressing, under the influence of a magnetic field, significantly accelerates the repair process of full-thickness skin injuries. Concurrently, hair growth is also significantly enhanced, indicating higher quality of wound repair.138 Moreover, Wang et al. utilized tannic acid to bridge magneto-deformable cobalt ferrite nanoparticles (CFO NPs) with a polyvinyl alcohol (PVA) matrix, resulting in a nanocomposite hydrogel with enhanced mechanical properties and magnetic responsiveness (Fig. 7B). Under a simple static magnetic field, the tannic acid bridge effectively transfers the magnetic responsiveness from the CFO NPs to PVA, leading to significant changes in the material surface morphology. These morphological changes further promote cell adhesion and proliferation. The synergistic action of the magneto-responsive bionanocomposites with the magnetic field also significantly accelerates early vascular formation at the wound site, thereby markedly expediting the tissue reconstruction and wound healing. These results reveal the potential of multifunctional dressings in accelerating tissue reconstruction.139
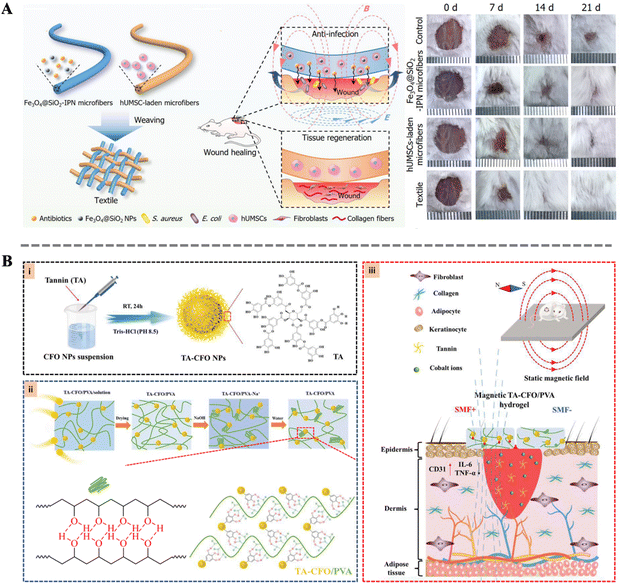 |
| Fig. 7 (A) Schematic illustration of the fabrication of the Fe3O4@SiO2-IPN microfibers and the wound healing process; wound images at different time after treatment.138 Copyright 2020, Wiley. (B) Schematic representation of the synthetic procedure of TA-CFO/PVA hydrogels and their application for wound repair.139 Copyright 2022, Royal Society of Chemistry. | |
4.5 Multifunctionality of magneto-responsive biocomposites
Wound healing is an extremely complex and dynamic biological process, akin to a meticulously orchestrated symphony, requiring coordinated efforts from multiple aspects. From the initial hemostasis and sterilization, to subsequent cell proliferation, migration, and differentiation, and finally tissue remodeling and angiogenesis, each step is crucial. Any deficiency or dysfunction in a particular step may trigger a cascade of reactions, leading to the failure of the entire repair process.5 For instance, inadequate sterilization may result in infection, thereby compromising the newly formed tissue and delaying the healing process; aberrant cell behavior may lead to excessive cell proliferation or overproduction of collagen peptide, resulting in scarring or other complications. Therefore, in the process of wound healing, comprehensive consideration and meticulous regulation of each step are imperative to ensure their coordination and mutual cooperation. To promote wound healing more effectively, the concerted action of multiple repair functions is typically required. In this regard, magnetic materials demonstrate immense potential.
He et al. designed magneto-thermal responsive bilayer microneedles (Fe-Se-HA-MNs) composed of functionalized HA, Fe3O4, and micelle-protected selenium nanoparticles (SeNPs@LAS). A self-designed disk-shaped electromagnetic field device (Disk-ZVS) was employed to apply magnetic stimulation to the microneedles (Fig. 8A). The electric field intensity and electromagnetic loss were mainly concentrated at the tips of the Fe-Se-HA-MNs. The microneedles can penetrate tough scabs, pierce bacterial biofilms, and perform effective magneto-thermal conversion for deep thermal therapy disinfection. As the microneedle structure gradually degrades, the sustained release of Se NPs can stimulate endothelial cell proliferation, migration, and the expression of genes related to angiogenesis. Additionally, Se NPs promote angiogenesis, facilitating blood vessel formation. In summary, Fe-Se-HA-MNs can achieve multiple functions such as magneto-thermal disinfection, deep non-invasive tissue penetration, anti-inflammatory effects, and promotion of angiogenesis.44 Fu et al. constructed a novel living electrospun short fibrous sponge by modifying bionic short fibers with engineered nanofat, which can load stem cells and release vascular endothelial growth factor, thereby promoting the tubulogenesis of human umbilical vein endothelial cells. This sponge can effectively and continuously act on wounds in vivo, facilitating the continuous healing of diabetic wounds.140 Furthermore, Shou et al. developed a powerful platform technology combining magnetic-responsive hydrogels, cells, and magneto-mechanical stimulation (Fig. 8B). The cells encapsulated in the hydrogel were FDA-approved fibroblasts and keratinocytes. These cells achieved a threefold increase in wound closure rate in diabetic mice. Additionally, magneto-mechanical stimulation activated fibroblasts, significantly enhancing their proliferation and collagen deposition. Furthermore, the platform improved the secretion profile of keratinocytes through the Ras/MEK/ERK pathway to promote angiogenesis. Importantly, the magnetic-responsive properties also facilitated on-demand insulin release for spatiotemporal glucose regulation by increasing network deformation and interstitial flow. This integrated system addresses most pathological factors associated with diabetic wounds on a single platform, demonstrating the multifunctionality of magneto-responsive bio-composites in the wound healing process.43
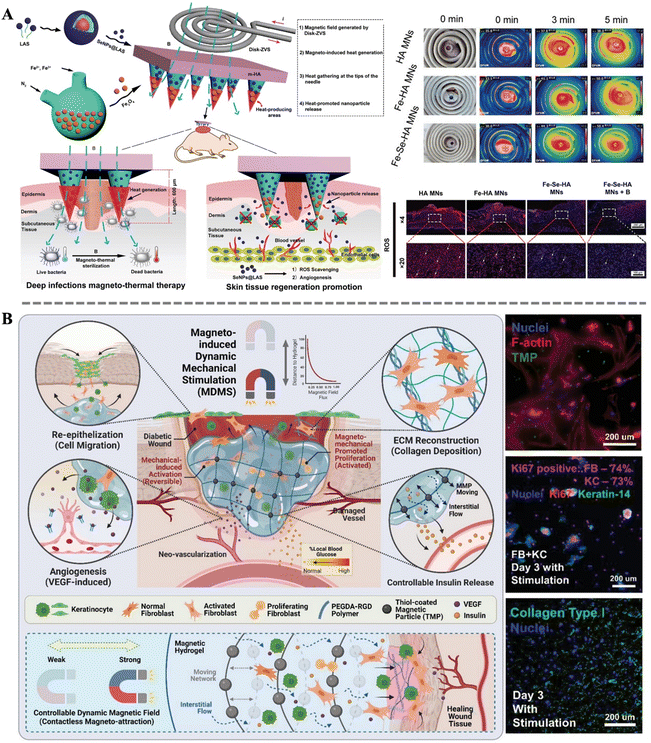 |
| Fig. 8 (A) Schematic diagram of the construction of Fe-Se-HA MNs bilayer microneedles and MN-based magneto-thermal therapy for infected diabetic wounds; optical images of the vortex coil and infrared thermography images of the magneto-thermal therapy; frozen section staining of reactive oxygen species (ROS) in the wound tissue.44 Copyright 2023, Wiley. (B) Schematic illustration of PEGDA-RGD-TMP magnetic hydrogel treated with magneto-induced dynamic mechanical stimulation for diabetic wound healing; fluorescent images of fibroblasts, Ki67 expression, and collagen type I.43 Copyright 2023, Wiley. | |
5. Conclusion and future perspective
Wound dressings have a developmental history spanning hundreds of years. With the increasingly profound understanding of wound healing mechanisms, various wound dressings have emerged to meet the needs in different stages of wound healing. In recent years, “magneto-responsive wound dressings” have emerged as a novel type of smart wound dressing. By responding to external magnetic stimulation and interacting with the wounds, they sense and react to changes in wound conditions or environments, greatly enhancing the speed and quality of wound repair. This review aims to comprehensively summarize the enhancement functions of magneto-responsive biocomposites as wound dressings reported in the literature. These magneto-responsive biocomposites can regulate cell behavior by directly affecting the interaction between the material and cells, such as promoting cell adhesion and proliferation, and accurately controlling their differentiation. And the MNPs can achieve directional arrangement under the influence of an external magnetic field, providing the composites with excellent mechanical anisotropy and effectively guiding the orientation growth of cells and the directional arrangement of collagen fibers. Moreover, under external magnetic stimulation, magneto-responsive biocomposites undergo nanoscale deformation, providing localized mechanical stimulation to the damaged area, thereby promoting tissue remodeling and regeneration. Remarkably, the magnetocaloric effect of the magneto-responsive biocomposites not only possesses profound antimicrobial capability but also allows for precise modulation and control of drug release in a spatiotemporal regime. By programmatically sterilizing, it effectively suppresses inflammation and accelerates wound repair. Based on the multifaceted mechanisms, magneto-responsive biocomposites demonstrate tremendous potential applications in the field of wound healing dressings. However, before the widespread clinical application of magnetic responsive wound dressings, a series of challenges still need to be addressed to ensure their safety and efficiency toward patients.
The cytotoxicity of MNPs has been a subject of controversy. While most studies indicate low cytotoxicity of MNPs, their toxicity can vary depending on factors such as size, surface coating, dosage, exposure time, and cell line.141,142In vivo studies can provide a more realistic reflection of the overall impact of MNPs on the body. They have not shown significant acute toxicity but have revealed potential risks of genetic, neurological, immunological, and reproductive toxicity. Therefore, it is necessary to extensively conduct both in vitro and in vivo studies to thoroughly investigate the cytotoxicity and long-term effects of MNPs. Furthermore, there are challenges in effectively and safely integrating MNPs with wound dressings. To optimize materials and determine their reliability in wound repair applications, a deeper understanding of the response of magneto-responsive wound dressings to different external magnetic fields. Particle distribution, surface chemistry, coatings, and their effects on wound healing are also needed. Establishing standardized research methods to explore the biological effects of MNPs with different physical properties is crucial. Before clinical application or preclinical trials, it is essential to conduct in-depth laboratory research on the biomedical applications of current and future magnetic nanoparticles to ensure their safety and efficacy.
Additionally, the practical application of magneto-responsive wound dressings typically requires coordination with specific external magnetic fields to leverage their magnetic responsive properties. Although researchers have indicated the potential of magnetic fields in promoting wound healing, there is still a lack of sufficient evidence to support these positive effects in clinical trials. Various factors such as the form, intensity, and direction of the magnetic field significantly impact wound healing efficiency, making the application of magnetic fields more complex and nuanced. In particular, dynamic magnetic fields possess diverse parameters such as field intensity, frequency, pulse width, duration, and exposure frequency, complicating the mechanisms involved in wound healing and making them difficult to elucidate. Furthermore, the mode of magnetic field exposure is equally important. In practical applications, magnetic field exposure can be divided into local exposure and whole-body exposure. Static magnetic fields generated by permanent magnets can be conveniently placed near the wound or applied to the entire body. However, it still lacks the clear research conclusions on whether the beneficial effects of dynamic magnetic fields on wound healing are contributed by the direct infliction on the wound site or the regulation on the entire body.143 Wound healing is a complex and prolonged biological process involving interactions among various cells and molecules. The effects of magnetic field exposure may vary during different stages of wound healing. For dynamic magnetic field exposure, tissues undergo a heating process. Short-term exposure to dynamic magnetic fields can protect biological tissues from temperature elevation while exerting their positive effects. However, prolonged exposure to dynamic magnetic fields may have adverse effects on normal tissues, leading to ongoing debates about their safety, necessitating further research and assessment.
Furthermore, the specific mechanism of magneto-responsive biocomposites in promoting wound healing remains not fully elucidated. Although existing research studies mention some positive effects such as re-epithelialization, vascular regeneration, and collagen deposition, these studies often focus on intuitionistic observations, lacking in-depth, systematic exploration of mechanisms. To comprehensively understand how magneto-responsive biocomposites affect the wound healing process, further research is needed into their specific roles in cellular signal transduction, regulation of gene expression, and molecular interactions. The commercialization process of magneto-responsive wound dressings also faces numerous challenges. Due to the novel design and materials used in magneto-responsive wound dressings, coupled with high requirements for magnetic equipment, transitioning from research and development to commercial application entails a lengthy and complex process. This includes material safety assessment, efficacy validation, optimization of production processes, and cost control, etc. Additionally, large-scale production of magneto-responsive wound dressings poses a technological challenge, as most products are still in the experimental stage, lacking uniform, efficient production processes, and standards.
To promote the clinical application and commercialization process of magneto-responsive wound dressings, it is essential to enhance interdisciplinary collaboration. This involves integrating resources and technical expertise from fields such as materials science, biomedical engineering, pharmaceuticals, and clinical medicine. Through in-depth research, technological innovation, and process improvement, we can continuously enhance the therapeutic efficacy and production efficiency of magneto-responsive wound dressings while reducing production costs. Ultimately, this will lead to their widespread application and commercial success in the market. However, there is still a long road ahead to achieve perfect skin repair.
Data availability
No primary research results, software or code have been included and no new data were generated or analysed as part of this review.
Conflicts of interest
The authors declare no conflicts of interest.
Acknowledgements
This work was financially supported by the National Natural Science Foundation of China (32301246, 32271467), the Natural Science Foundation of Jiangsu Province (Grants No BK20230315), and the Priority Academic Program Development of Jiangsu Higher Education Institutions (PAPD).
References
- A. L. Byrd, Y. Belkaid and J. A. Segre, Nat. Rev. Microbiol., 2018, 16, 143–155 CrossRef CAS PubMed.
- A. Chortos, J. Liu and Z. A. Bao, Nat. Mater., 2016, 15, 937–950 CrossRef CAS PubMed.
- T. A. Harris-Tryon and E. A. Grice, Science, 2022, 376, 940–945 CrossRef CAS.
- M. Wang, Y. F. Luo, T. Wang, C. J. Wan, L. Pan, S. W. Pan, K. He, A. Neo and X. D. Chen, Adv. Mater., 2021, 33, 2003014 CrossRef CAS PubMed.
- G. C. Gurtner, S. Werner, Y. Barrandon and M. T. Longaker, Nature, 2008, 453, 314–321 CrossRef CAS PubMed.
- Y. Liang, J. He and B. Guo, ACS Nano, 2021, 15, 12687–12722 CrossRef CAS.
- M. Farahani and A. Shafiee, Adv. Healthcare Mater., 2021, 10, 2100477 CrossRef CAS PubMed.
- Q. Zeng, X. Qi, G. Shi, M. Zhang and H. Haick, ACS Nano, 2022, 16, 1708–1733 CrossRef CAS.
- S. Dhivya, V. V. Padma and E. Santhini, Biomedicine, 2015, 5, 22 CrossRef.
- K. Jarbrink, G. Ni, H. Sonnergren, A. Schmidtchen, C. Pang, R. Bajpai and J. Car, Syst. Rev., 2017, 6, 15 CrossRef.
- A. Bigham, N. Islami, A. Khosravi, A. Zarepour, S. Iravani and A. Zarrabi, Small, 2024, 2311903 CrossRef PubMed.
- M. Farokhi, F. Mottaghitalab, Y. Fatahi, A. Khademhosseini and D. L. Kaplan, Trends Biotechnol., 2018, 36, 907–922 CrossRef CAS.
- V. Jones, J. E. Grey and K. G. Harding, Br. Med. J., 2006, 332, 777–780 CrossRef.
- L. I. F. Moura, A. M. A. Dias, E. Carvalho and H. C. de Sousa, Acta Biomater., 2013, 9, 7093–7114 CrossRef CAS.
- J. Y. Shan, J. Y. Che, C. H. Song and Y. J. Zhao, Smart Med., 2023, 2, e20220025 CrossRef CAS.
- H. Derakhshandeh, S. S. Kashaf, F. Aghabaglou, I. O. Ghanavati and A. Tamayol, Trends Biotechnol., 2018, 36, 1259–1274 CrossRef CAS.
- D. Simoes, S. P. Miguel, M. P. Ribeiro, P. Coutinho, A. G. Mendonca and I. J. Correia, Eur. J. Pharm. Biopharm., 2018, 127, 130–141 CrossRef CAS PubMed.
- G. Chen, Y. Yu, X. Wu, G. Wang, J. Ren and Y. Zhao, Adv. Funct. Mater., 2018, 28, 1801386 CrossRef.
- J. Y. Chen, J. H. He, Y. T. Yang, L. P. Qiao, J. Hu, J. Zhang and B. L. Guo, Acta Biomater., 2022, 146, 119–130 CrossRef CAS PubMed.
- Y. Liang, X. Zhao, T. Hu, B. Chen, Z. Yin, P. X. Ma and B. Guo, Small, 2019, 15, 1900046 CrossRef PubMed.
- R. Z. Luo, Y. Liang, J. R. Yang, H. Q. Feng, Y. Chen, X. P. Jiang, Z. Zhang, J. Liu, Y. Bai, J. T. Xue, S. Y. Chao, Y. Xi, X. Q. Liu, E. G. Wang, D. Luo, Z. Li and J. P. Zhang, Adv. Mater., 2023, 35, 2208395 CrossRef CAS.
- A. S. Montaser, M. Rehan, W. M. El-Senousy and S. Zaghloul, Carbohydr. Polym., 2020, 244, 116479 CrossRef CAS PubMed.
- J. Xiang, R. Zhu, S. Lang, H. Yan, G. Liu and B. Peng, Chem. Eng. J., 2021, 409, 128291 CrossRef CAS.
- F. Yergoz, N. Hastar, C. E. Cimenci, A. D. Ozkan, T. Tekinay, M. O. Guler and A. B. Tekinay, Biomaterials, 2017, 134, 117–127 CrossRef CAS PubMed.
- X. Zhao, B. Guo, H. Wu, Y. Liang and P. X. Ma, Nat. Commun., 2018, 9, 2784 CrossRef.
- S. Yang, F. Wang, H. Han, H. A. Santos, Y. Zhang, H. Zhang, J. Wei and Z. Cai, Biomed. Technol., 2023, 2, 31–48 CrossRef CAS.
- R. Dong and B. Guo, Nano Today, 2021, 41, 101290 CrossRef CAS.
- H. F. Dong, L. Y. Wang, L. Du, X. Wang, Q. Li, X. Y. Wang, J. Zhang, J. Nie and G. P. Ma, Small, 2022, 18, 2201620 CrossRef CAS.
- R. N. Dong and B. L. Guo, Nano Today, 2021, 41, 101290 CrossRef CAS.
- M. Monavarian, S. Kader, S. Moeinzadeh and E. Jabbari, Tissue Eng., Part B, 2019, 25, 294–311 CrossRef PubMed.
- M. Rodrigues, N. Kosaric, C. A. Bonham and G. C. Gurtner, Physiol. Rev., 2019, 99, 665–706 CrossRef CAS.
- X. Lin, L. Cai, X. Cao and Y. Zhao, Smart Med., 2023, 2, e20220019 CrossRef CAS.
- B. Mirani, Z. Hadisi, E. Pagan, S. M. H. Dabiri, A. van Rijt, L. Almutairi, I. Noshadi, D. G. Armstrong and M. Akbari, Adv. Healthcare Mater., 2023, 12, 2203233 CrossRef CAS PubMed.
- S. O. Blacklow, J. Li, B. R. Freedman, M. Zeidi, C. Chen and D. J. Mooney, Sci. Adv., 2019, 5, eaaw3963 CrossRef CAS PubMed.
- S. Li, L. Wang, W. Zheng, G. Yang and X. Jiang, Adv. Funct. Mater., 2020, 30, 2002370 CrossRef CAS.
- J. Wang, X. Chen, Y. Zhao, Y. Yang, W. Wang, C. Wu, B. Yang, Z. Zhang, L. Zhang, Y. Liu, X. Du, W. Li, L. Qiu, P. Jiang, X. Mou and Y. Li, ACS Nano, 2019, 13, 11686–11697 CrossRef CAS.
- H. Zhao, J. Huang, Y. Li, X. Lv, H. Zhou, H. Wang, Y. Xu, C. Wang, J. Wang and Z. Liu, Biomaterials, 2020, 258, 120286 CrossRef CAS PubMed.
- Y. Zhu, J. Zhang, J. Song, J. Yang, Z. Du, W. Zhao, H. Guo, C. Wen, Q. Li, X. Sui and L. Zhang, Adv. Funct. Mater., 2020, 30, 1905493 CrossRef CAS.
- W. Chen, Z. B. Zhang and P. H. J. Kouwer, Small, 2022, 18, 2203033 CrossRef CAS.
- L. Hao and H. Mao, Biomater. Sci., 2023, 11, 6384–6402 RSC.
- M. Zhu, Z. Hu, N. Liu, K. Yao, G. Hong, Y. Li, Y. Chen, H. He, W. Wu, Y. Zhou, J. Shi and Y. He, Small, 2024, 2400644 CrossRef CAS.
- Q. Zhang, J. Zhu, H. Liu, X. Fei and M. Zhu, Adv. Funct. Mater., 2023, 34, 2309968 CrossRef.
- Y. Shou, Z. Le, H. S. Cheng, Q. Liu, Y. Z. Ng, D. L. Becker, X. Li, L. Liu, C. Xue, N. J. Y. Yeo, R. Tan, J. Low, A. R. K. Kumar, K. Z. Wu, H. Li, C. Cheung, C. T. Lim, N. S. Tan, Y. Chen, Z. Liu and A. Tay, Adv. Mater., 2023, 35, 2304638 CrossRef CAS PubMed.
- D. He, X. Liu, J. Jia, B. Peng, N. Xu, Q. Zhang, S. Wang, L. Li, M. Liu, Y. Huang, X. Zhang, Y. Yu and G. Luo, Adv. Funct. Mater., 2023, 34, 2306357 CrossRef CAS.
- B. K. Sun, Z. Siprashvili and P. A. Khavari, Science, 2014, 346, 941–945 CrossRef CAS PubMed.
- A. N. Dehkordi, F. M. Babaheydari, M. Chehelgerdi and S. R. Dehkordi, Stem Cell Res. Ther., 2019, 10, 111 CrossRef CAS.
- G. Broughton, J. E. Janis and C. E. Attinger, Plast. Reconstr. Surg., 2006, 117, 12S–34S CrossRef CAS.
- S. Fahimirad and F. Ajalloueian, Int. J. Pharm., 2019, 566, 307–328 CrossRef CAS.
- B. Dalisson and J. Barralet, Adv. Healthcare Mater., 2019, 8, 1900764 CrossRef PubMed.
- J. Cabral, A. E. Ryan, M. D. Griffin and T. Ritter, Adv. Drug Delivery Rev., 2018, 129, 394–406 CrossRef CAS PubMed.
- G. G. Gauglitz, H. C. Korting, T. Pavicic, T. Ruzicka and M. G. Jeschke, Mol. Med., 2011, 17, 113–125 CAS.
- M. Rahmati, J. J. Blaker, S. P. Lyngstadaas, J. F. Mano and H. J. Haugen, Mater. Today Adv., 2020, 5, 100051 CrossRef.
- S. Zhang, Y. Liu, X. Zhang, D. Zhu, X. Qi, X. Cao, Y. Fang, Y. Che, Z.-C. Han, Z.-X. He, Z. Han and Z. Li, Theranostics, 2018, 8, 5348–5361 CrossRef CAS PubMed.
- C. Chen, Y. Liu, H. Wang, G. Chen, X. Wu, J. Ren, H. Zhang and Y. Zhao, ACS Nano, 2018, 12, 10493–10500 CrossRef CAS.
- J. Qu, X. Zhao, Y. Liang, Y. Xu, P. X. Ma and B. Guo, Chem. Eng. J., 2019, 362, 548–560 CrossRef CAS.
- B. Saleh, H. K. Dhaliwal, R. Portillo-Lara, E. Shirzaei Sani, R. Abdi, M. M. Amiji and N. Annabi, Small, 2019, 15, 1902232 CrossRef PubMed.
- X. Zhao, Y. Liang, Y. Huang, J. He, Y. Han and B. Guo, Adv. Funct. Mater., 2020, 30, 1910748 CrossRef CAS.
- X. Zhao, H. Wu, B. Guo, R. Dong, Y. Qiu and P. X. Ma, Biomaterials, 2017, 122, 34–47 CrossRef CAS PubMed.
- D. Gan, T. Xu, W. Xing, X. Ge, L. Fang, K. Wang, F. Ren and X. Lu, Adv. Funct. Mater., 2019, 29, 1805964 CrossRef.
- J. Li, F. Yu, G. Chen, J. Liu, X.-L. Li, B. Cheng, X.-M. Mo, C. Chen and J.-F. Pan, ACS Appl. Mater. Interfaces, 2020, 12, 2023–2038 CrossRef CAS.
- Y. Luo, H. Diao, S. Xia, L. Dong, J. Chen and J. Zhang, J. Biomed. Mater. Res., Part A, 2010, 94A, 193–204 CrossRef CAS.
- M. Chen, J. Tian, Y. Liu, H. Cao, R. Li, J. Wang, J. Wu and Q. Zhang, Chem. Eng. J., 2019, 373, 413–424 CrossRef CAS.
- T. Phuong Le, Y. Lee, T. Dieu Linh, T. Thai Thanh Hoang, J. I. Kang, K. M. Park and K. D. Park, Acta Biomater., 2020, 103, 142–152 CrossRef PubMed.
- S. Y. Wang, H. Kim, G. Kwak, H. Y. Yoon, S. D. Jo, J. E. Lee, D. Cho, I. C. Kwon and S. H. Kim, Adv. Sci., 2018, 5, 1800852 CrossRef PubMed.
- M. Furtado, L. Chen, Z. Chen, A. Chen and W. Cui, Eng. Regener., 2022, 3, 217–231 Search PubMed.
- Y. Gao, H. Du, Z. Xie, M. Li, J. Zhu, J. Xu, L. Zhang, J. Tao and J. Zhu, J. Mater. Chem. B, 2019, 7, 3644–3651 RSC.
- H. Ma, Q. Zhou, J. Chang and C. Wu, ACS Nano, 2019, 13, 4302–4311 CrossRef CAS.
- C.-H. Su, W.-P. Li, L.-C. Tsao, L.-C. Wang, Y.-P. Hsu, W.-J. Wang, M.-C. Liao, C.-L. Lee and C.-S. Yeh, ACS Nano, 2019, 13, 4290–4301 CrossRef CAS PubMed.
- Y. Shen, G. Xu, H. Huang, K. Wang, H. Wang, M. Lang, H. Gao and S. Zhao, ACS Nano, 2021, 15, 6352–6368 CrossRef CAS.
- E. M. Tottoli, R. Dorati, I. Genta, E. Chiesa, S. Pisani and B. Conti, Pharmaceutics, 2020, 12, 735 CrossRef CAS.
- J. Zhang, Y. Zheng, J. Lee, J. Hua, S. Li, A. Panchamukhi, J. Yue, X. Gou, Z. Xia, L. Zhu and X. Wu, Nat. Commun., 2021, 12, 1670 CrossRef CAS PubMed.
- X. Zheng, Z. Ding, W. Cheng, Q. Lu, X. Kong, X. Zhou, G. Lu and D. L. Kaplan, Adv. Healthcare Mater., 2020, 9, 2000041 CrossRef CAS.
- L. Hao, X. Tao, M. Feng, K. Zhou, Y. He, J. Yang, H. Mao and Z. Gu, ACS Appl. Mater. Interfaces, 2023, 15, 24034–24046 CrossRef CAS.
- L. Hao, S. Zhao, S. Hao, Y. He, M. Feng, K. Zhou, Y. He, J. Yang, H. Mao and Z. Gu, Int. J. Biol. Macromol., 2023, 240, 124364 CrossRef CAS.
- Y. Li, G. Huang, X. Zhang, B. Li, Y. Chen, T. Lu, T. J. Lu and F. Xu, Adv. Funct. Mater., 2013, 23, 660–672 CrossRef CAS.
- V. F. Cardoso, A. Francesko, C. Ribeiro, M. Bañobre-López, P. Martins and S. Lanceros-Mendez, Adv. Healthcare Mater., 2018, 7, 1700845 CrossRef.
- Y. Kim and X. H. Zhao, Chem. Rev., 2022, 122, 5317–5364 CrossRef CAS PubMed.
- Y. H. Li, G. Y. Huang, X. H. Zhang, B. Q. Li, Y. M. Chen, T. L. Lu, T. J. Lu and F. Xu, Adv. Funct. Mater., 2013, 23, 660–672 CrossRef CAS.
- Z. G. Li, Y. Z. Li, C. Chen and Y. Cheng, J. Controlled Release, 2021, 335, 541–556 CrossRef CAS PubMed.
- A. Pardo, M. Gómez-Florit, S. Barbosa, P. Taboada, R. M. A. Domingues and M. E. Gomes, ACS Nano, 2021, 15, 175–209 CrossRef CAS.
- M. Colombo, S. Carregal-Romero, M. F. Casula, L. Gutiérrez, M. P. Morales, I. B. Böhm, J. T. Heverhagen, D. Prosperi and W. J. Parak, Chem. Soc. Rev., 2012, 41, 4306–4334 RSC.
- E. H. Fragal, V. H. Fragal, E. P. Silva, A. T. Paulino, E. C. da Silva, M. R. Mauricio, R. Silva, A. F. Rubira and E. C. Muniz, Carbohydr. Polym., 2022, 292, 119665 CrossRef CAS PubMed.
- M. T. Klem, M. Young and T. Douglas, Mater. Today, 2005, 8, 28–37 CrossRef CAS.
- F. Lin, J. Zheng, W. Guo, Z. Zhu, Z. Wang, B. Dong, C. Lin, B. Huang and B. Lu, Cellulose, 2019, 26, 6861–6877 CrossRef CAS.
- J. Liao and H. Huang, Int. J. Biol. Macromol., 2019, 138, 462–472 CrossRef CAS PubMed.
- P. S. Castro, M. Bertotti, A. F. Naves, L. H. Catalani, D. R. Cornejo, G. D. Bloisi and D. F. S. Petri, Colloids Surf., B, 2017, 156, 388–396 CrossRef CAS PubMed.
- H. Liu, C. Wang, Q. Gao, X. Liu and Z. Tong, Acta Biomater., 2010, 6, 275–281 CrossRef CAS PubMed.
- W. Haas, M. Zrinyi, H. G. Kilian and B. Heise, Colloid Polym. Sci., 1993, 271, 1024–1034 CrossRef CAS.
- Y. Gao, C. Hu, W. J. Zheng, S. Yang, F. Li, S. D. Sun, M. Zrinyi, Y. Osada, Z. M. Yang and Y. M. Chen, ChemPhysChem, 2016, 17, 1999–2007 CrossRef CAS.
- Z. Y. Liu, J. H. Liu, X. Cui, X. Wang, L. H. Zhang and P. F. Tang, Front. Chem., 2020, 8, 124 CrossRef CAS.
- N. A. D. Burke, H. D. H. Stöver and F. P. Dawson, Chem. Mater., 2002, 14, 4752–4761 CrossRef CAS.
- L. Shi, Y. Zeng, Y. Zhao, B. Yang, D. Ossipov, C. W. Tai, J. Dai and C. Xu, ACS Appl. Mater. Interfaces, 2019, 11, 46233–46240 CrossRef CAS PubMed.
- M. Antman-Passig and O. Shefi, Nano Lett., 2016, 16, 2567–2573 CrossRef CAS PubMed.
- S. Araujo-Custodio, M. Gomez-Florit, A. R. Tomas, B. B. Mendes, P. S. Babo, S. M. Mithieux, A. Weiss, R. M. A. Domingues, R. L. Reis and M. E. Gomes, ACS Biomater. Sci. Eng., 2019, 5, 1392–1404 CrossRef CAS PubMed.
- H. Chen, X. Zhang, L. Shang and Z. Su, Adv. Sci., 2022, 9, 2202173 CrossRef CAS.
- K. Hu, J. Sun, Z. Guo, P. Wang, Q. Chen, M. Ma and N. Gu, Adv. Mater., 2015, 27, 2507–2514 CrossRef CAS PubMed.
- C. D. L. Johnson, D. Ganguly, J. M. Zuidema, T. J. Cardina, A. M. Ziemba, K. R. Kearns, S. M. McCarthy, D. M. Thompson, G. Ramanath, D. A. Borca-Tasciuc, S. Dutz and R. J. Gilbert, ACS Appl. Mater. Interfaces, 2019, 11, 356–372 CrossRef.
- Y. He, J. Tang, Y. Hu, S. Yang, F. Xu, M. Zrínyi and Y. Mei Chen, Chem. Eng. J., 2023, 462, 142193 CrossRef CAS.
- L. Hao, L. Li, P. Wang, Z. Wang, X. Shi, M. Guo and P. Zhang, Nanoscale, 2019, 11, 23423–23437 RSC.
- L. L. Hao, J. X. Li, P. Wang, Z. L. Wang, Z. X. Wu, Y. Wang, Z. X. Jiao, M. Guo, T. F. Shi, Q. G. Wang, Y. Ito, Y. Wei and P. B. Zhang, Adv. Funct. Mater., 2021, 31, 2009661 CrossRef CAS.
- A. E. Goodship, Ann. Rheum. Dis., 1992, 51, 4–6 CrossRef CAS PubMed.
- P. A. Janmey and C. A. McCulloch, Annu. Rev. Biomed. Eng., 2007, 9, 1–34 CrossRef CAS.
- M. Wright, P. Jobanputra, C. Bavington, D. M. Salter and G. Nuki, Clin. Sci., 1996, 90, 61–71 CrossRef CAS PubMed.
- L. L. Hao, J. X. Li, P. Wang, Z. L. Wang, Y. Wang, Y. Z. Zhu, M. Guo and P. B. Zhang, Nanoscale, 2023, 15, 4123–4136 RSC.
- A. A. Adedoyin and A. K. Ekenseair, Nano Res., 2018, 11, 5049–5064 CrossRef CAS.
- S. Gil and J. F. Mano, Biomater. Sci., 2014, 2, 812–818 RSC.
- N. A. de Oliveira and P. J. von Ranke, Phys. Rep., 2010, 489, 89–159 CrossRef CAS.
- V. K. Pecharsky and K. A. Gschneidner, J. Magn. Magn. Mater., 1999, 200, 44–56 CrossRef CAS.
- X. L. Liu, Y. F. Zhang, Y. Y. Wang, W. J. Zhu, G. L. Li, X. W. Ma, Y. H. Zhang, S. Z. Chen, S. Tiwari, K. J. Shi, S. W. Zhang, H. M. Fan, Y. X. Zhao and X. J. Liang, Theranostics, 2020, 10, 3793–3815 CrossRef CAS.
- S.-h Noh, S. H. Moon, T.-H. Shin, Y. Lim and J. Cheon, Nano Today, 2017, 13, 61–76 CrossRef CAS.
- S. V. Spirou, M. Basini, A. Lascialfari, C. Sangregorio and C. Innocenti, Nanomaterials, 2018, 8, 401 CrossRef PubMed.
- R. T. Li, K. Liu, X. Huang, D. Li, J. X. Ding, B. Liu and X. S. Chen, Adv. Sci., 2022, 9, 2105152 CrossRef CAS.
- H. N. Wilkinson and M. J. Hardman, Open Biol., 2020, 10, 200223 CrossRef CAS PubMed.
- H. K. Zheng, X. W. Cheng, L. Jin, S. Z. Shan, J. Yang and J. Zhou, Biomed. Pharmacother., 2023, 165, 115199 CrossRef CAS.
- X. Jin, Z. L. Ou, X. Huang, L. Shi, R. Shi, J. Wang, J. M. Yang, K. J. Fang, J. Yang, D. L. Lv, C. Wang, Z. X. Yuan, W. H. Li, W. G. Liu, G. X. Luo, J. Deng and W. Wang, Nano Today, 2023, 51, 101898 CrossRef CAS.
- R. Shi, H. S. Li, X. Jin, X. Huang, Z. L. Ou, X. F. Zhang, G. X. Luo and J. Deng, Acta Biomater., 2022, 152, 425–439 CrossRef CAS.
- M. Carmen Echave, R. M. A. Domingues, M. Gomez-Florit, J. Luis Pedraz, R. L. Reis, G. Orive and M. E. Gomes, ACS Appl. Mater. Interfaces, 2019, 11, 47771–47784 CrossRef.
- R. Goodrich, Y. Tai, Z. Ye, Y. Yin and J. Nam, Adv. Funct. Mater., 2023, 33, 2211288 CrossRef CAS.
- R. Tognato, A. R. Armiento, V. Bonfrate, R. Levato, J. Malda, M. Alini, D. Eglin, G. Giancane and T. Serra, Adv. Funct. Mater., 2019, 29, 1804647 CrossRef.
- K. Ganguly, H. Jin, S. D. Dutta, D. K. Patel, T. V. Patil and K. T. Lim, Carbohydr. Polym., 2022, 287, 119321 CrossRef CAS PubMed.
- T. Greig, R. Torah and K. Yang, IEEE Rev. Biomed. Eng., 2024, 17, 264–279 Search PubMed.
- B. Reid and M. Zhao, Adv. Wound Care, 2014, 3, 184–201 CrossRef.
- J. Fromm and S. Lautner, Plant, Cell Environ., 2007, 30, 249–257 CrossRef CAS.
- M. R. Love, S. Palee, S. C. Chattipakorn and N. Chattipakorn, J. Cell. Physiol., 2018, 233, 1860–1876 CrossRef CAS.
- Z. Deng, R. Yu and B. Guo, Mater. Chem. Front., 2021, 5, 2092–2123 RSC.
- X. Li, Z. Tan, B. Guo, C. Yu, M. Yao, L. Liang, X. Wu, Z. Zhao, F. Yao, H. Zhang, S. Lyu, C. Yuan and J. Li, Chem. Eng. J., 2023, 463, 142387 CrossRef CAS.
- A. Gupta, P. Avci, T. Dai, Y.-Y. Huang and M. R. Hamblin, Adv. Wound Care, 2013, 2, 422–437 CrossRef PubMed.
- F. Y. Mo, M. J. Zhang, X. W. Duan, C. Y. Lin, D. P. Sun and T. H. You, Int. J. Nanomed., 2022, 17, 5947–5990 CrossRef.
- Y. L. Xu, H. L. Chen, Y. F. Fang and J. Wu, Adv. Healthcare Mater., 2022, 11, 2200494 CrossRef CAS.
- X. L. Li, Y. Liu, X. W. Qi, S. L. Xiao, Z. S. Xu, Z. X. Yuan, Q. Liu, H. S. Li, S. Y. Ma, T. F. Liu, Y. Huang, X. R. Zhang, X. Zhang, Z. W. Mao, G. X. Luo and J. Deng, Adv. Mater., 2022, 34, 2109004 CrossRef CAS.
- X. Yang, C. Zhang, D. Deng, Y. Gu, H. Wang and Q. Zhong, Small, 2022, 18, 2104368 CrossRef CAS PubMed.
- C. W. Chung, B. W. Liao, S. W. Huang, S. J. Chiou, C. H. Chang, S. J. Lin, B. H. Chen, W. L. Liu, S. H. Hu, Y. C. Chuang, C. H. Lin, I. J. Hsu, C. M. Cheng, C. C. Huang and T. T. Lu, ACS Appl. Mater. Interfaces, 2022, 14, 6343–6357 CrossRef CAS.
- M. Wang, C. G. Wang, M. Chen, Y. W. Xi, W. Cheng, C. Mao, T. Z. Xu, X. X. Zhang, C. Lin, W. Y. Gao, Y. Guo and B. Lei, ACS Nano, 2019, 13, 10279–10293 CrossRef CAS PubMed.
- Y. F. Lu, H. S. Li, J. Wang, M. Y. Yao, Y. Peng, T. F. Liu, Z. Li, G. X. Luo and J. Deng, Adv. Funct. Mater., 2021, 31, 2105749 CrossRef CAS.
- Y. Yuan, S. H. Shen and D. D. Fan, Biomaterials, 2021, 276, 120838 CrossRef CAS PubMed.
- Q. K. Zeng, Y. N. Qian, Y. J. Huang, F. Ding, X. L. Qi and J. L. Shen, Bioact. Mater., 2021, 6, 2647–2657 CAS.
- Y. Peng, D. F. He, X. Ge, Y. F. Lu, Y. H. Chai, Y. X. Zhang, Z. W. Mao, G. X. Luo, J. Deng and Y. Zhang, Bioact. Mater., 2021, 6, 3109–3124 CAS.
- X. Zhang, C. Tian, Z. Chen and G. Zhao, Adv. Ther., 2020, 3, 2000001 CrossRef CAS.
- P. Wang, C. Lv, X. Zhou, Z. Wu, Z. Wang, Y. Wang, L. Wang, Y. Zhu, M. Guo and P. Zhang, J. Mater. Chem. B, 2022, 10, 7808–7826 RSC.
- X. H. Fu, J. Wang, D. J. Qian, Z. W. Chen, L. Chen, W. G. Cui and Y. Wang, Adv. Fiber Mater., 2023, 5, 979–993 CrossRef CAS.
- Y. Q. Meng, Y. N. Shi, Y. P. Zhu, Y. Q. Liu, L. W. Gu, D. D. Liu, A. Ma, F. Xia, Q. Y. Guo, C. C. Xu, J. Z. Zhang, C. Qiu and J. G. Wang, J. Nanobiotechnol., 2024, 22, 24 CrossRef.
- K. A. Tran, Y. Jin, J. Bouyer, B. J. DeOre, L. Suprewicz, A. Figel, H. Walens, I. Fischer and P. A. Galie, Biomater. Sci., 2022, 10, 2237–2247 RSC.
- H. Lv, J. Liu, C. Zhen, Y. Wang, Y. Wei, W. Ren and P. Shang, Cell Proliferation, 2021, 54, e12982 CrossRef.
|
This journal is © The Royal Society of Chemistry 2024 |
Click here to see how this site uses Cookies. View our privacy policy here.