DOI:
10.1039/D4MH90029D
(Commentary)
Mater. Horiz., 2024,
11, 1832-1837
A reflection on ‘The synthesis, structure and electronic properties of a lead-free hybrid inorganic–organic double perovskite (MA)2KBiCl6 (MA = methylammonium)’
First published on 2nd April 2024
Abstract
Interest in the field of hybrid double perovskite halides has grown rapidly over the last few years, with important developments in 3D, 2D and 1D systems. Their chemical scope has also expanded from an initial focus on materials containing post-transition metals, which are of potential utility for photovoltaic applications, to recent work that covers transition metals and rare earths with very interesting magnetic and optical properties. This reflection article traces the development of this important area from our 2016 communication in Materials Horizons (F. Wei, Z. Deng, S. Sun, F. Xie, G. Kieslich, D. M. Evans, M. A. Carpenter, P. D Bristowe and A. K. Cheetham, Mater. Horiz., 2016, 3, 328–332, https://doi.org/10.1039/C6MH00053C).
Introduction
During the first three years of the publication of Materials Horizons, the Cheetham group at Cambridge contributed three articles to the journal. The first article (https://doi.org/10.1039/C3MH00087G) concerned the unique structures and electronic properties of reduced titanium dioxide nanoparticles.1 The work compared the topotactic reduction of single crystals versus nanoparticles of the rutile polymorph of TiO2. While the reduced single crystals formed TiO1.48 (i.e., Ti2O3) with the corundum structure, the reduced nanoparticles had the composition TiO1.83. Studies using X-ray total scattering showed that the reduced nanoparticles contained relatively crystalline Ti2O3 together with a second component of nanocrystalline Ti4O7 (a so-called Magnéli phase). Studies of their electronic structure using hard X-ray photoelectron spectroscopy and electron energy loss spectroscopy indicated that their conductivity was lower than the reduced TiO2 single crystals due to interparticle resistance. It was suggested that the unique electronic properties of reduced nanoparticles could result in unusual functionalities that are not found in bulk materials. Interestingly, the first author of the 2014 paper and an author of the present work, Satoshi Tominaka, later demonstrated that reduced TiOx nanoparticles could form unusual amorphous intermediates that showed very interesting catalytic reactivity for the oxygen reduction reaction.2
In our second Materials Horizons paper (https://doi.org/10.1039/C4MH00009A), also published in 2014, Paul Saines and other collaborators determined the magnetic structure of a new metal–organic framework (MOF), cobalt adipate, by using a combination of powder neutron diffraction, synchrotron X-ray powder diffraction, and magnetic susceptibility studies.3 Magnetic structure studies are very challenging with MOFs because the magnetic ions are diluted by the many atoms from the organic ligands, but we were able to establish the antiferromagnetic spin structure and demonstrate the presence of magnetoelastic coupling in a MOF framework for the first time. As a consequence of this coupling, cobalt adipate exhibits negative thermal expansion at low temperatures.
In spite of the interest around our first two papers in Materials Horizons, we have decided to focus this reflection article on our third article (https://doi.org/10.1039/C6MH00053C), which was published two years later in 2016.4 We are doing so because, with the knowledge of hindsight, it has had a much greater impact than we had expected when the article was originally written. At the time, there was growing interest in the search for lead-free alternatives to halide perovskite materials such as (MA)PbI3 (MA = methylammonium), which were displaying excellent performance in photovoltaic devices.5–8 The idea that was developed in our paper was that it should be possible to avoid the use of lead by creating hybrid double perovskites in which two PbII ions were replaced by equal numbers of MI and MIII cations according to the general formula (A)2MIMIIIX6 (A = amine cation). Our paper illustrated the principle of this approach by reporting the synthesis and characterization of (MA)2KBiCl6, which was prepared by Fengxia Wei.4 In the following sections we trace the subsequent impact of this paper on both our own work and that of others, not only in the area of hybrid double perovskites, but also in terms of developing new families of perovskite iodides, as well as low-dimensional analogues.
Hybrid double perovskite halides
The existence of inorganic double perovskite halides has a long history, going back to the seminal 1970 work of Edelstein and co-workers, who reported a large family of Cs2NaMIIICl6 compounds based largely on the use of trivalent transition metals, rare earths and actinides.9 At about the same time as our publication on (MA)2KBiCl6, other groups began to revisit the inorganic systems. For example, Woodward et. al. reported Cs2AgBiX6 (X = Cl, Br) with a band gap of 2.77 eV for X = Cl and 2.19 eV for X = Br,10 while a paper by Karunadasa et. al. described the X = Br phase with a band gap of 1.95 eV and a long photoluminescence lifetime of ca. 660 ns.11 In contrast to these findings, the band gap of our (MA)2KBiCl6 was 3.04 eV, reflecting the more electropositive character of potassium versus silver, while all of these double perovskite halide compounds had the disadvantage of having indirect band gaps. Unlike the inorganic double perovskites, which are normally cubic, our (MA)2KBiCl6 was rhombohedral at room temperature. In more recent work, we have shown that it undergoes a transition to cubic symmetry on heating to 330 K, and a further transition to a disordered lattice on cooling to 230 K.12
In light of these important contributions from our own group and others, we redoubled our efforts to make further examples of double perovskite halides, noting that hybrid systems have some advantages over purely inorganic ones, including lower processing temperatures and the lower cost of MA versus caesium. In parallel with our ongoing synthetic efforts, we also performed extensive calculations using density functional theory (DFT). The treatment of amine disorder in DFT calculations on cubic hybrid perovskites can be challenging, but by a stroke of good fortune our first (MA)2KBiCl6 structure was rhombohedral with the MA cations sitting in an ordered position on the 3-fold axis. Jerry Deng, under the supervision of Paul Bristowe, performed DFT calculations in this ordered setting for a large family of hypothetical (MA)2MIMIIIX6 compounds with MI = K, Cu, Ag and Tl; MIII = Bi; and X = Cl, Br and I.13 BiIII was our preferred cation for the MIII site because it is both isoelectronic with PbII and is also non-toxic. From this family of 12 hypothetical compounds, our next synthetic success, performed again by Fengxia Wei, was with (MA)2TlBiBr6, so the computational results and the discovery of (MA)2TlBiBr6 were published together in our second paper on hybrid double perovskite halides.13
The successful synthesis of (MA)2TlBiBr6, which is cubic rather than rhombohedral, was very satisfying in one respect because the band gap was only 2.00 eV, which is approaching the range where it could be suitable for photovoltaic (PV) applications. In addition, the band gap was direct as a consequence of having two ions that are isoelectronic with PbII on the alternating TlI and BiIII sites. Nevertheless, it was not attractive to solve the toxicity problem with lead by replacing half of it with thallium, which is even more toxic! Fengxia therefore continued her efforts in the laboratory, inspired in part by the work of the Woodward and Karunadasa groups,10,11 and finally succeeded in making the non-toxic hybrid double perovskite bromide, (MA)2AgBiBr6.14 Its cubic structure has an indirect band gap of 2.20 eV, which is close to the values reported for the caesium analogue, and it is very stable in addition to being non-toxic. The band gap is considered to be narrow enough to exhibit semiconducting properties, but only ionic conductivity (4.8 × 108 Ω cm) was observed by single-crystal conductivity measurements performed by Satoshi Tominaka. Its electronic conductivity is probably far lower than the ionic conductivity due to the small number of carriers and/or the low mobility.
Our fourth and final synthetic success in this phase of our work on hybrid double perovskites was actually carried out by our computational specialist, Jerry Deng, who synthesized the first hybrid double perovskites containing rare earths. He succeeded in making two examples in this area: (MA)2KLnCl6, with Ln = Y and Gd.15 Like the bismuth analogue, these phases are rhombohedral at room temperature, but they also undergo a rhombohedral to cubic phase transition on heating to 435 K and ∼375 K, respectively. However, like the rare-earth halides themselves, these phases are very unstable to moisture so we were unable to make pure bulk samples for optical or magnetic studies. However, our density functional calculations on the rhombohedral phase indicated that both materials have large direct band gaps, are mechanically stable, and, in the case of (MA)2KGdCl6, could exhibit magnetic ordering at low temperatures.
With the continuing search for lower-band-gap materials, another inorganic double perovskite, Cs2AgSbBr6, was synthesized by our group in 2019;16 it showed an indirect band gap of only 1.64 eV. Replacing the BiIII 6s6p orbitals with the SbIII 5s5p orbitals can lower the conduction band minimum, thus resulting in a smaller band gap, just as it does when PbII is replaced by SnII. SbIII–SbV charge transfer also contributed to the dark colour in the as-synthesized crystals. However, as with the hybrid double perovskites of the rare earths, it was challenging to make phase-pure Cs2AgSbBr6 in bulk form.
The impact of our 2016 Materials Horizons paper on subsequent work on hybrid double perovskites was very rewarding, but there was nevertheless some disappointment on our part that we did not succeed in extending this approach to the synthesis of double perovskite iodides. These were highly sought after because, on the basis of both intuition and our DFT calculations,13 their band gaps are expected to be much lower than those of the corresponding chlorides and bromides, which would make them more attractive for PV applications. However, neither we nor others succeeded in making hybrid double perovskite iodides in the late 2010s, though there were two claims concerning the synthesis of (MA)2AgSbI6
17 and (MA)2AgBiI6
18 that were not substantiated with proper structural characterization and were never reproduced. Attempts to synthesise inorganic analogues such as Cs2MIMIIII6 were also unsuccessful, though there was a credible report that it was possible to make nanocrystals of Cs2AgBiI6 by anion exchange of its bromide analogue with trimethylsilyl iodide.19 This claim was recently substantiated by full Rietveld refinement of the structure of Cs2AgBiI6 nanoparticles.20
In light of the above challenges with the synthesis of bulk double perovskite iodides, we have recently explored the origins of this problem and proposed a solution. This strand of work, which we undertook when one of us (AKC) returned to UC Santa Barbara in 2018, is discussed in the following section.
Why are double perovskite iodides so rare?
The prediction of whether an inorganic perovskite is likely to exist has traditionally relied on calculations of the Goldschmidt tolerance factor (TF),21 which depends upon the radii of the A and B cations and the X anions22 in a classical AMX3 perovskite: | 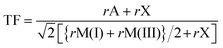 | (1) |
where rA, rM, and rX are the ionic radii of the A, M and X ions, respectively. Perovskite formation is considered to be viable if the TF lies between 0.80 and 1.00, and cubic structures are expected when the value is between 0.90 and 1.00. The use of the TF descriptor has served the community well for nearly 100 years, and it was successfully extended to hybrid systems about a decade ago.23,24 However, with the knowledge of hindsight, it has worked fortuitously well because it has been overwhelmingly applied to perovskite oxides, fluorides, chlorides and bromides. In these cases, there is no doubt that most M cations are able to adopt an octahedral coordination, but when the anion is exceptionally large, as in the case of iodides, then we also need to consider the radius ratio (or octahedral factor, μ) for the M cations to determine whether the cation is large enough to sustain octahedral coordination: |  | (2) |
In the case of double perovskites, the MIII cation is usually the smallest one (noting that it is normal to use an average of the MI and MIII radii when estimating their TFs), so we need to consider whether the MIII cation meets the radius ratio criterion for octahedral coordination, which is μ > 0.414. In fact, the use of this second descriptor results in a minimum radius of 0.90 Å for MIII when X = I−, and only the largest trivalent cations meet this second criterion. These are principally the rare earths and actinides. Note that while SbIII is clearly too small, BiIII does meet the radius ratio criterion, but generally prefers to form layered A3Bi2I9 structures, which are presumably stabilized by van der Waals interactions between the iodide layers. In a 2021 article, which is eponymous with the title of this section, we showed with Pratap Vishnoi and Ram Seshadri that the use of the two descriptors should apply to both inorganic and hybrid double perovskites and proposed a large number of candidate double perovskite iodides that should be amenable to synthesis.25
A new family of inorganic double perovskite iodides
The existence of inorganic double perovskites containing rare earths, such as Cs2LiLnI6 (Ln = Sc, Lu, Tm, Ho) and Cs2NaLnI6 (Ln = Sc, Lu, Tm, Er), was reported by Meyer in 1980, but their structures were not characterized.26 Similarly, Cs2NaLaI6 has been investigated, but again without structural characterization.27 However, the existence of such phases has recently been definitively proven in our study at UCSB with Gregg Kent, Ram Seshadri and others. Samples were prepared by solid-state reaction between CsI, NaI and LnI3 at 675 °C, and single-crystal X-ray structures of Cs2NaLnI6 (Ln = Ce, Nd, Gd, Tb and Dy) were reported,28 along with their phase transitions and UV-visible, photoluminescence and magnetic properties. There are two reasons why such phases have been difficult to obtain hitherto. One is that they are extremely sensitive to hydrolysis and require synthetic and manipulation skills that are not often found in today's materials chemistry laboratories. The second is that their structures are typically monoclinic and heavily twinned at room temperature, so we were only able to fully resolve them by collecting SCXRD data at higher temperatures above the transitions to the cubic Fm
m structure. Double perovskite iodides of the rare earths are not suitable for PV applications because they are not semiconducting. Our effort was worthwhile, however, as we were rewarded by some extremely interesting optical and magnetic properties. The next challenge in the double perovskites area is to synthesize hybrid versions, such as (MA)2NaLnI6. This will clearly require a lower-temperature synthetic approach that may not yield single crystals for structure determination.
Low-dimensional double perovskite halides
It is also interesting to note that our work on 3D double perovskite halides has stimulated interest in low-dimensional hybrid double perovskites. The first hybrid layered double perovskite was obtained in 2018 by Karunadasa and co-workers by reacting butylammonium (BA) salts with the 3D Cs2AgBiBr6 system.29 Two new structures, (BA)4AgBiBr8 and (BA)2CsAgBiBr7, were obtained by using this innovative approach. Since then, a wide range of other examples, including some layered iodides, have been synthesized directly rather than by ion exchange of 3D compounds,30–32 including several by Lingling Mao at UC Santa Barbara.32 It is important to note that the chemical diversity of layered systems is far more extensive than their 3D analogues because the structures can accommodate a wide range of much larger organic cations between the layers. In addition, the concept can be extended beyond the p-block elements to transition metals.33,34 We have written a recent review of both inorganic and hybrid layered double perovskites.35
In addition to work on layered materials, there has also been important progress in 1D hybrid double perovskites, which, like their 3D analogues, have the general formula (A)2MIMIIIX6. The first example was reported in 2018 and described the structure of (MA)2AgInBr6.36 In this structure-type, infinite chains of face-sharing MIX6 and MIIIX6 octahedra are separated by the amine cations. This structure is normally found when the MIII cation is not large enough to adopt octahedral symmetry in a 3D system,25 and in our own laboratory we recently discovered six ruthenium(III) systems of this type, (MA)2MIRuIIIX6 (MI = Na, Ag, K with X = Cl and Br).37
New hybrid single perovskite iodides
The need to use two geometrical descriptors to predict the existence of double perovskite iodides suggests that this approach should equally apply to the prediction of single perovskite iodides of the general formula AMIII3. A small number of inorganic examples are known, including CsSrI3, CsEuI3 and CsPbI3, but until recently no hybrid compounds had been reported aside from the widely studied methylammonium and formamidinium compounds with the group IV metals, Ge, Sn and Pb (e.g. (MA)PbI3). However, a hybrid layered iodide of EuII, (C4H9NH3)2EuI4, was synthesized in 1997 by Mitzi and Liang using a solid-state reaction between butylammonium iodide and EuI2.38 Inspired by this synthetic approach, we have recently succeeded in making five new hybrid perovskite iodides of the general formula AMIII3 (A = methylammonium with MII = Sr, Sm, Eu, and A = formamidinium with MII = Sr, Eu).39 Their structures were obtained from X-ray powder diffraction data by Rietveld refinement and their calorimetric, optical, photoluminescence, and magnetic properties were also reported.
Conclusions
This short reflection article describes the way in which the discovery of the first hybrid double perovskite, (MA)2KBiCl6, which was reported in Materials Horizons in 2016,4 had a profound impact on the area of double perovskite halides more broadly (Fig. 1). In addition to leading to many other examples of 3D double perovskite halides, including some important iodides, it also pointed the way towards making their low-dimensional 1D and 2D analogues. The geometrical understanding that has developed around this area25 has also enabled the recent discovery of a new family of 3D hybrid single perovskite iodides, (MA)MIII3 and (FA)MIII3, extending the 3D systems for the first time beyond the widely studied examples with MII = Ge, Sn and Pb. It is reasonable to expect that the next development in this latter area will involve the discovery of new families of 1D and 2D hybrid materials, such as A2MIII4, as foreshadowed by the earlier work of Mitzi in 1997.38 The timeline shown in Fig. 1 underlines the remarkable progress that has been made in both the inorganic and hybrid perovskite halide areas in only 8 years since the key breakthroughs in 2016.
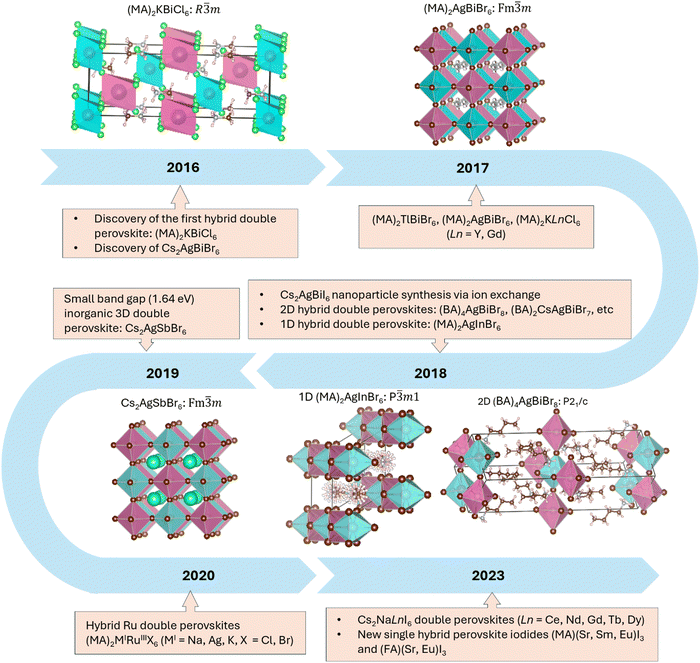 |
| Fig. 1 Key developments in lead-free halide perovskites since 2016. | |
In closing, it is interesting to reflect on why our 2016 Materials Horizons paper on hybrid double perovskite halides4 has had such a high impact in materials chemistry over the last few years. In part, it can be ascribed to the timeliness of the 2016 paper due to the high level of momentum at that time within the halide perovskite field. In addition, attention was also drawn to the paper by our immediate follow-up publications on other examples of hybrid double perovskite halides in 201613 and 2017,14,15 two of which have been highly cited. There seems to be some advantage in having a cluster of publications in an emerging field in order that the work will be noticed by more readers. The other option that we considered at the time was to wait until we had several examples and then try to publish them together in one of the Nature journals, but we were concerned about the delay that this would have caused and the possibility that we might be scooped. The decision to publish quickly in Materials Horizons (received 3rd March 2016, accepted 15th April 2016!) still seems to have been the best choice with the knowledge of hindsight.
Conflicts of interest
There are no conflicts to declare.
References
- S. Tominaka, H. Yoshikawa, Y. Matsushita, A. Yamamoto and A. K. Cheetham, Mater. Horiz., 2014, 1, 106–110 RSC
.
- S. Tominaka, A. Ishihara, T. Nagai and K.-I. Ota, ACS Omega, 2017, 2, 5209–5214 CrossRef CAS PubMed
.
- P. J. Saines, P. T. Barton, M. Jura, K. S. Knight and A. K. Cheetham, Mater. Horiz., 2014, 1, 332–337 RSC
.
- F. Wei, Z. Deng, S. Sun, F. Xie, G. Kieslich, D. M. Evans, M. A. Carpenter, P. D. Bristowe and A. K. Cheetham, Mater. Horiz., 2016, 3, 328–332 RSC
.
- A. Kojima, K. Teshima, Y. Shirai and T. Miyasaka, J. Am. Chem. Soc., 2009, 131, 6050–6051 CrossRef CAS PubMed
.
- H. S. Kim, C. R. Lee, J. H. Im, T. Moehl, A. Marchioro, S. J. Moon, R. Humphry-Baker, J. H. Yum, J. E. Moser, M. Grätzel and N. G. Park, Sci. Rep., 2012, 2, 591 CrossRef PubMed
.
- M. M. Lee, J. Teuscher, T. Miyasaka, T. N. Murakami and H. J. Snaith, Science, 2012, 338, 643–647 CrossRef CAS PubMed
.
- M. Liu, M. B. Johnston and H. J. Snaith, Nature, 2013, 501, 395–398 CrossRef CAS PubMed
.
- L. R. Morss, M. Siegal, L. Stenger and N. Edelstein, Inorg. Chem., 1970, 9, 1771–1775 CrossRef CAS
.
- E. T. McClure, M. R. Ball, W. Windl and P. M. Woodward, Chem. Mater., 2016, 28, 1348–1354 CrossRef CAS
.
- A. H. Slavney, T. Hu, A. M. Lindenberg and H. I. Karunadasa, J. Am. Chem. Soc., 2016, 138, 2138–2141 CrossRef CAS PubMed
.
- F. Wei, Y. Wu, S. Sun, Z. Deng, L. T. Chew, B. Cheng, C. C. Tan, T. J. White and A. K. Cheetham, Molecules, 2023, 28, 174 CrossRef CAS PubMed
.
- Z. Deng, F. X. Wei, S. Sun, G. Kieslich, A. K. Cheetham and P. D. Bristowe, J. Mater. Chem. A, 2016, 4, 12025–12029 RSC
.
- F. Wei, Z. Deng, S. Sun, F. Zhang, D. M. Evans, G. Kieslich, S. Tominaka, M. A. Carpenter, P. D. Bristowe and A. K. Cheetham, Chem. Mater., 2017, 29, 1089–1094 CrossRef CAS
.
- Z. Deng, F. Wei, F. Brivio, Y. Wu, S. Sun, P. D. Bristowe and A. K. Cheetham, J. Phys. Chem. Lett., 2017, 8, 5015–5020 CrossRef CAS PubMed
.
- F. Wei, Z. Deng, S. Sun, N. T. P. Hartono, H. L. Seng, T. Buonassisi and A. K. Cheetham, Chem. Commun., 2019, 55, 3721–3724 RSC
.
- Y. J. Li, T. Wu, L. Sun, R. X. Yang, L. Jiang, P. F. Cheng, Q. Q. Hao, T.-J. Wang, R. F. Lu and W. Q. Deng, RSC Adv., 2017, 7, 35175–35180 RSC
.
- P. Cheng, T. Wu, Y. Li, L. Jiang, W. Deng and K. Han, New J. Chem., 2017, 41, 9598–9601 RSC
.
- S. E. Creutz, E. N. Crites, M. C. De Siena and D. R. Gamelin, Nano Lett., 2018, 18, 1118–1123 CrossRef CAS PubMed
.
- K. T. Kluherz, S. T. Mergelsberg, J. J. De Yoreo and D. R. Gamelin, Chem. Mater., 2023, 35, 5699–5708 CrossRef CAS
.
- V. M. Goldschmidt, Naturwissenschaften, 1926, 14, 477–485 CrossRef CAS
.
- R. D. Shannon, Acta Crystallogr., Sect. A: Cryst. Phys., Diffr., Theor. Gen. Crystallogr., 1976, 32, 751–767 CrossRef
.
- G. Kieslich, S. Sun and A. K. Cheetham, Chem. Sci., 2014, 5, 4712–4715 RSC
.
- G. Kieslich, S. Sun and A. K. Cheetham, Chem. Sci., 2015, 6, 3430–3433 RSC
.
- P. Vishnoi, R. Seshadri and A. K. Cheetham, J. Phys. Chem. C, 2021, 125, 11756–11764 CrossRef CAS
.
- G. Meyer, Z. Naturforsch., B: J. Chem. Sci., 1980, 35, 394–396 CrossRef
.
- G. Gundiah, K. Brennan, Z. Yan, E. C. Samulon, G. Wu, G. A. Bizarri, S. E. Derenzo and E. D. Bourret-Courchesne, J. Lumin., 2014, 149, 374–384 CrossRef CAS
.
- G. T. Kent, E. E. Morgan, K. R. Albanese, A. Kallistova, L. Kautzsch, G. Wu, P. Vishnoi, R. Seshadri and A. K. Cheetham, Angew. Chem., Int. Ed., 2023, 62, e202306000 CrossRef CAS PubMed
.
- B. A. Connor, L. Leppert, M. D. Smith, J. B. Neaton and H. I. Karunadasa, J. Am. Chem. Soc., 2018, 140, 5235–5240 CrossRef CAS PubMed
.
- M. K. Jana, S. M. Janke, D. J. Dirkes, S. Dovletgeldi, C. Liu, X. Qin, K. Gundogdu, W. You, V. Blum and D. B. Mitzi, J. Am. Chem. Soc., 2019, 141, 7955–7964 CrossRef CAS PubMed
.
- L.-Y. Bi, Y.-Q. Hu, M.-Q. Li, T.-L. Hu, H.-L. Zhang, X.-T. Yin, W.-X. Que, M. S. Lassoued and Y.-Z. Zheng, J. Mater. Chem. A, 2019, 7, 19662–19667 RSC
.
- L. L. Mao, S. Teicher, C. C. Stoumpos, R. M. Kennard, R. A. DeCrescent, G. Wu, J. A. Schuller, M. L. Chabinyc, A. K. Cheetham and R. Seshadri, J. Am. Chem. Soc., 2019, 141, 19099–19109 CrossRef CAS PubMed
.
- P. Vishnoi, J. L. Zuo, X. T. Li, D. C. Binwal, K. E. Wyckoff, L. L. Mao, L. Kautzsch, G. Wu, S. D. Wilson, M. G. Kanatzidis, R. Seshadri and A. K. Cheetham, J. Am. Chem. Soc., 2022, 144, 6661–6666 CrossRef CAS PubMed
.
- J. Xue, Z. Wang, A. Comstock, Z. Wang, H. Y. Sung, I. D. Williams, D. Sun, J. Liu and H. Lu, Chem. Mater., 2022, 34, 2813–2823 CrossRef CAS
.
- H. A. Evans, L. L. Mao, R. Seshadri and A. K. Cheetham, Annu. Rev. Mater. Res., 2021, 51, 351–380 CrossRef CAS
.
- T. T. Tran, M. A. Quintero, K. E. Arpino, Z. A. Kelly, J. R. Panella, X. Wang and T. M. McQueen, CrystEngComm, 2018, 20, 5929–5934 RSC
.
- P. Vishnoi, J. L. Zuo, T. A. Strom, G. Wu, S. D. Wilson, R. Seshadri and A. K. Cheetham, Angew. Chem., Int. Ed., 2020, 59, 8974–8981 CrossRef CAS PubMed
.
- D. B. Mitzi and K. Liang, Chem. Mater., 1997, 9, 2990–2995 CrossRef CAS
.
- G. T. Kent, J. Zhuang, K. R. Albanese, A. Zohar, E. E. Morgan, A. Kallistova, L. Kautzsch, A. A. Mikhailovsky, P. Vishnoi, R. Seshadri and A. K. Cheetham, J. Am. Chem. Soc., 2023, 145, 27850–27856 CrossRef CAS PubMed
.
|
This journal is © The Royal Society of Chemistry 2024 |
Click here to see how this site uses Cookies. View our privacy policy here.