DOI:
10.1039/D1JA00328C
(Critical Review)
J. Anal. At. Spectrom., 2022,
37, 50-68
Microsampling of biological fluids for elemental and isotopic analysis by ICP-MS: strategies and applications for disease diagnosis
Received
22nd September 2021
, Accepted 4th November 2021
First published on 5th November 2021
Abstract
Human biological fluids are quite varied and complex. Some examples include blood, cerebrospinal fluid, synovial fluid, peritoneal fluid, intraocular fluids, and fluids that do not require an invasive sample uptake, such as lacrimal fluid, seminal fluid, sweat, urine and saliva. They are present in the body in very different volumes, from the low microliter level to the liter range. For those requiring invasive sampling, just a small percentage can be sampled in order not to disrupt the living body. Furthermore, quite often, the extracted samples must be divided into several aliquots required for different independent (bio)chemical assays. In addition, volumes of biofluids are much smaller in new-borns and infants. Therefore, just a microvolume of the biofluid is quite often available for analysis. Inductively coupled plasma-mass spectrometry (ICP-MS) is a suitable technique for elemental and isotopic determination of biological fluids at the trace and ultra-trace levels. Such determinations contributes to the interpretation of biochemical processes within our bodies and in many cases serve for the diagnosis of diseases. A research trend along the years has been to increase the capabilities of ICP-MS for the analysis of microsamples. This review focusses on strategies for microsample collection of fluids from the human body and their introduction into an ICP-MS. In addition, representative examples of applications in the clinical field are briefly described.
1. Introduction
It is well-known that metals such as Na, K, Mg, Ca, Fe, Mn, Co, Cu, Zn and Mo are essential for humans and our body must have appropriate amounts of them.1 Disruption of metal homeostasis caused by element deficiency, or imbalance, induces detrimental intracellular events, including oxidative stress, inflammation, DNA fragmentation, protein misfolding and activation of apoptosis, giving rise to pathogenesis and progression of many diseases.2 Moreover, the detection in body fluids of metals such as Ti, V, Cr, Al, Co, Ni, Zr and Mo leaching from prostheses and implants (e.g. made of titanium alloys, stainless steel, high-strength alloys, alumina or zirconia),3,4 as well as the unnoticed exposure to, or intake of, toxic elements (e.g. Cd, Pb, Hg and Tl)5 also requires highly sensitive analytical techniques.
Inductively coupled plasma – mass spectrometry (ICP-MS) is a suitable technique for elemental determination of biological fluids, as it enables reliable analysis at the trace and ultra-trace levels with a wide dynamic range.6 Many advances have been made in the last thirty years to improve analytical capabilities of ICP-MS instruments. For instance, sector field (SF)-ICP-MS, collision/reaction cell (CRC) quadrupole ICP-MS, and tandem ICP-MS allow for reduction of spectral interferences, while time of flight (TOF) ICP-MS permits quasi-simultaneous multielemental analysis. On the other hand, improvements in electronics for fast data acquisition, including shorter dwell and settling times, make ICP-MS ideal for time-resolved analysis applications such as single particle (sp) and single cell (sc) detection.7–10 All these developments contribute to improve the understanding of the role of trace and ultratrace elements in biological processes and under different medical conditions.
In addition, high precision isotopic analysis can be achieved with the multicollector (MC)-ICP-MS. The accurate measurement of isotopic ratios in biofluids may contribute to interpret biochemical processes within our bodies and to serve as potential diagnosis/prognosis markers of diseases, providing complementary information to the elemental analysis. It is well known that a low amount of sample and/or a low target isotope concentration can strongly compromise applications focused on high-precision isotopic analysis of biofluids by MC-ICP-MS.11 The use of aerosol desolvating systems, high-efficiency jet interfaces, high gain Faraday cup amplifiers (1013 Ω with respect to the 1011 Ω conventional ones) and ion counter devices has proved to enhance instrument sensitivity.12,13 Methods based on sample mixing of the analyte with a non-enriched in-house standard,11 the use of droplets of serum deposited on silicon,14 or preconcentration procedures,15 have been proposed to extend the applications of MC-ICP-MS within a clinical context.
Of course, physiology and pathophysiology depend not only on elemental and isotopic concentrations, but also on the oxidation/reduction states and on the variety of compounds to which they can be bound to, such as proteins with different functions (e.g. storage and transport metalloproteins, metalloenzymes, or signal-transduction metalloproteins), or inorganic ligands such as metal chelators. The most powerful analytical strategies for metal speciation require the initial separation of the sought species by chromatographic or electrophoretic methods and then the detection of the metal content of the isolated species.16 Sample volumes introduced in such separation systems are small and, in those cases where the separation technique is on-line connected to the detector, analytical signals follow a transient pattern.
Fig. 1 shows examples of human biological fluids which have been analysed by ICP-MS (the figure has been particularized for women; so it should be taken into account that some of those fluids are not present in men, while men have other exclusive fluids, such as seminal fluid). Also, typical volumes present in the body of an adult have been indicated in Fig. 1. Evidently, for many biofluids (particularly for those cases requiring invasive sampling), just a small percentage can be sampled in order not to disrupt the living body. Furthermore, it must be noted that, quite often, the extracted samples must be divided into several aliquots required for different independent (bio)chemical assays. In addition, volumes of fluids are much smaller in new-borns and infants. Moreover, although the focus of this review is human research, it must be kept in mind that before human research, animal experimentation is often necessary, and these animals often have even lower fluid volumes available. Therefore, the sample amount available for ICP-MS analysis can be minute in many applications. This will particularly affect the analysis of elements at very low native concentrations because the dilution step to get enough volume for conventional solution nebulisation is not feasible.
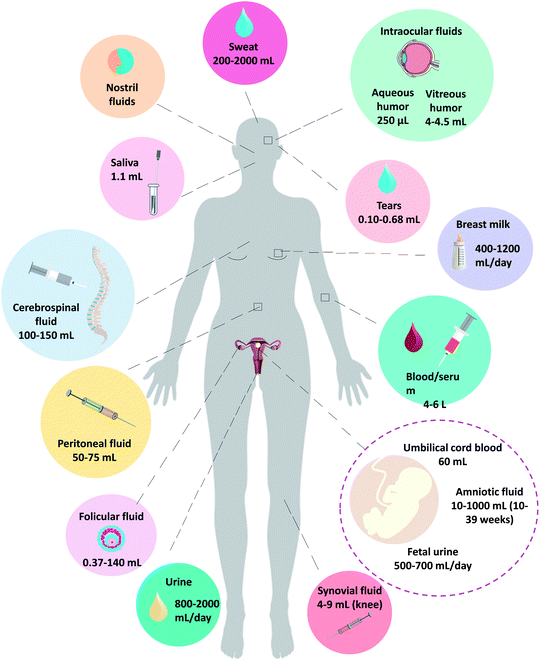 |
| Fig. 1 Selection of body fluids whose elemental concentrations have been analyzed by ICP-MS (in the figure it has been particularized for women). Typical volumes present in the body of an adult have been indicated between parentheses. | |
The number of publications addressing the elemental and/or isotopic analysis of human biological fluids by ICP-MS is immense. Development of approaches for sampling and analysis of minimum amounts of biofluids is a current research trend. This review is focused on the strategies for microsample collection of fluids from the human body and their introduction into an ICP-MS. Besides, representative examples of applications either to establish reference concentrations or for diagnosis of diseases will be briefly described.
2. Overview of microsample introduction systems into the ICP
Micro-flow nebulizers combined with small spray chambers, allowing sample delivery rates below 0.2 mL min−1, offer a great potential for elemental and isotopic determination of small volumes of biological fluids by ICP-MS.17–19 In addition, they can serve as an interface for on-line coupling of very low-flow continuous separation methods (e.g. capillary chromatography20 and capillary electrophoresis21) to ICP-MS for speciation purposes. The ICP-MS signals are proportional to the amount of analyte entering into the plasma per time unit, so, an efficient introduction system is required for sample delivery rates much lower than the typical 1–2 mL min−1 of conventional nebulizers.
Micronebulizers usually have similar arrangements to conventional ones, but their critical dimensions are reduced, providing finer aerosols. This fact, along with the decrease in water coalescence inside small chambers, leads to an increase of the analyte transport efficiency. Micronebulizers and spray chambers of different materials such as glass, quartz, PFA, PEEK, Teflon®, etc. are available, being selected as the material based on the application (e.g. to permit the introduction of organic solvents or hydrofluoric acid, or to avoid contamination in ultra-trace analysis). Different designs of pneumatic micronebulizers have been investigated, such as those based on microconcentric flow,22 cross-flow,23 parallel path24 and flow burring.25Fig. 2 shows the schematics of some of such devices. The most popular ones are the microconcentric configurations. Also, parallel path designs are rather common.
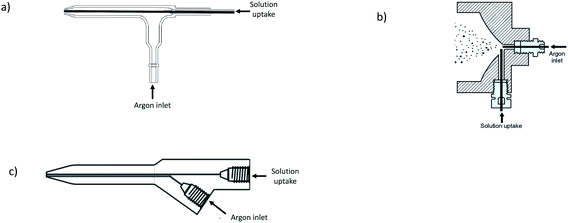 |
| Fig. 2 Schematics of three examples of micronebulizers employed in ICP-MS: (a) microconcentric flow, (b) cross-flow (reprinted with permission from ref. 23. Copyright 2021 American Chemical Society), and (c) parallel-path. | |
The suction in concentric nebulizers allows for self-aspiration, thus eliminating the need for peristaltic pump tubing (a potential source of contamination) and consequently reducing the signal noise. However, changes in the solution viscosity must be controlled in the self-aspiration mode, because viscosity affects the aspiration flow rate. Low flow rates could be introduced in conventional concentric nebulizers, but aerosol generation becomes erratic if the delivery rates are considerably lower than those obtained by natural aspiration. To overcome such problems, microconcentric nebulizers have been developed with reduced i.d. of the liquid capillary and a smaller gas exit cross-sectional area as compared to the conventional counterparts. The capillary i.d. and its position with respect to the nebulizer nozzle are important variables. A vast number of slight modifications of microconcentric nebulizers have been evaluated and several are commercially available, such as the so-called high efficiency nebulizer (HEN) made of borosilicate glass or quartz,26 without capillary tip recess. It is designed to operate at a sample flow lower than 0.3 mL min−1 and has a small gas cross-section in order to obtain a higher nebulizing gas velocity and small droplets. The HEN design can be especially useful for very clean samples. On the other hand, the MicroMist (made of glass, VitriCone® technology) and the OpalMist (made of PFA)27 nebulizers have the liquid capillary recessed with respect to the nebulizer tip, thus allowing for a relatively high content of solids in the sample. There are commercially available MicroMist models with natural sample uptake rates of 0.05; 0.1; 0.2; 0.4 and 0.6 mL min−1, and OpalMist models with natural uptake rates of 0.01; 0.05; 0.1; 0.2; 0.4; 0.6; 1 and 2 mL min−1. Another example is the so-called PFA Micro-Flow nebulizer28 whose sample capillary is more recessed than in the case of the MicroMist design (6 mm versus about 1 mm, respectively). Therefore, in that case the liquid and gas interaction occurs inside the nebulizer, promoting the production of fine droplets. Models commercially available include PFA-20, PFA-50, PFA-75, PFA-100, PFA-200 and PFA-400; the number is related to the free aspiration flow rate.
Parallel path nebulizers (see Fig. 2c) do not require a critical alignment of the gas and the liquid streams as in the case of the concentric ones, the liquid being introduced into the gas stream by the low pressure around it. Any liquid caught in the gas flow is pushed away with the gas, and broken into small droplets. Due to the configuration of these nebulizers, the liquid channel can be thicker than in the microconcentric designs, thus preventing tiny particles in the liquid plugging the sample path. Therefore, parallel path nebulizers offer advantages when samples contain undissolved particulates. Different models of nano- and micro-low flow parallel path nebulizers are commercially available,29 such as the Enya Mist model with a sample capillary of 60 μm i.d. and tolerating flow rates of 0.002–0.050 mL min−1 (it allows direct connection to micro HPLC), the Ari Mist HP (sample capillary of 150 μm i.d. and flow rates of 0.005–1 mL min−1), and the PFA 250 and PFA 260 (sample capillary of 250 μm i.d. and flow rates of 0.050–2 mL min−1), among others. Parallel path nebulizers have no suction, so liquids must be pumped.
The main types of spray chambers used in ICP-MS are the single-pass cylindrical type, the so-called Scott type (double-pass), and those based on cyclonic designs. Cyclonic spray chambers can be single-pass or double-pass (the central transfer tube of the double-pass acts as a secondary droplet filter to reduce the mean droplet size, thus reducing matrix effects and improving precision). The typical inner volumes of conventional chambers, around 50 mL, are reduced to 20 mL or less for micronebulizer coupling.
Alternatively, total sample consumption systems, enabling very low liquid flow rates and with a sample transport efficiency close to 100%, have become popular, offering competitive sensitivity and minimized matrix effects (interferences associated with the sample introduction system may be removed for analyte transport efficiency close to 100%).30 They consist of two main groups: (i) those for which the spray chamber is removed such as the direct injection nebulizer (DIN),31 or the direct injection high efficiency nebulizer (DIHEN)32 with variants such as the large bore DIHEN33,34 and the demountable DIHEN,35 and (ii) those having the micronebulizer associated with a very small single-pass spray chamber. In this case, almost 100% of the solvent is evaporated at the low flow delivery rates used. Such an approach has been called a “torch integrated sample introduction system” (TISIS).30 One of these systems is commercially available: DS-5 microflow concentric nebulizer, for very low-flow applications (3 to 10 μL min). The kit contains a low-volume spray chamber connected to the base of the ICP-MS torch.36 The heating of the chamber (hTISIS)37 and the addition of a sheathing gas flow30 to TISIS configurations can contribute to enhance the aerosol evaporation as well as to prevent the impact of droplets against the walls, thus increasing the sensitivity. The hTISIS has been proved appropriate for the analysis of samples of complex nature, such as whole blood.38
As has been mentioned in the introduction section, a current trend is the use of ICP-MS for sp and sc analysis. In the particular case of sc-ICP-MS it is crucial to employ sample introduction devices that keep cells intact and that allow a high cell transport efficiency. For such purposes it is important to prevent cell deposition on the inner walls of the spray chamber. Parameters such as cell size,39 morphology and stability (determined by the composition and structure of their cellular envelope) will facilitate or hinder their transport into the plasma.10 Different designs of microflow concentric nebulizers have been investigated, such as the MicroMist nebulizer in combination with a Scott spray chamber,40 or a parallel path design (Enya Mist) coupled to a total consumption low-volume spray chamber.41 Additionally, dedicated combinations of micronebulizer-spray chambers to transfer the highest percentage of cells into the ICP have been developed, such as the “high efficiency cell introduction system” (HECIS) consisting of a high performance concentric nebulizer and a 15 cm3 total consumption spray chamber with a sheath gas flow to minimize the deposition of cells.42 With such a system, transport efficiencies close to 100% have been reported. Nowadays, dedicated sc sample introduction systems based on pneumatic nebulizers for ICP-MS detection are marketed by companies such as Glass Expansion®, Meinhard®, Elemental Scientific® or PerkinElmer®, which typically offer pseudo-total consumption capabilities. An alternative to pneumatic nebulization is the generation of highly monodisperse microdroplets through a piezoelectric actuated quartz capillary and an internal carrier gas supply, offering high cell transport efficiencies;43 however, its effectiveness for the analysis of fragile cells seems not yet clear.10 Alternatively, some microfluidic platforms have been designed for sc manipulation and encapsulation in microdroplets before introduction into the ICP-MS,44 although the transport efficiency in this case may be not as high as desirable.
At first, the continuous introduction of a sample will allow for better signal stability. However, for complex samples the risk of nebulizer obstruction exists. An alternative option to the continuous aspiration is the introduction of tiny volumes (a few microliters38,45 or even nanoliters36) of samples in a discrete mode (flow injection in a liquid carrier, or segmented-flow injection in a continuously aspirated gas such as air), giving rise to transient signals. Due to the fast data acquisition capability of modern scanning ICP-MS devices (dwell time of a few ms), the transient signal when using a micronebulizer can reach a plateau for a few microliters (e.g. 2.5–10 μL) of solution injected (samples or standards), allowing for the analysis of several isotopes in each plug; of course, higher multielemental capabilities will be achieved with an ICP-TOFMS system.45 On the other hand, in the segmented-flow injection mode, as the carrier stream is not a liquid, the extent of sample re-nebulization from the chamber walls and the sample dispersion in liquid phase is reduced.37,38 Nonetheless, reported RSD values for 10 μL injections of 1
:
25 diluted blood samples were higher than those obtained by the continuous aspiration mode, these results being attributed to possible small changes in the sample volume injected into the air carrier stream.38
Other strategies for analysing tiny volumes of biological fluids include electrothermal vaporization (ETV),46 the sampling of dried droplets of biofluids by laser ablation (LA) with a high-energy pulsed laser,47–49 and the use of diode laser thermal vaporization (DLTV).50
In ETV-ICP-MS, a small amount of sample is placed in a conducting reservoir (typically a graphite furnace) which is then heated resistively to vaporize the sample. The vapour is subsequently transported into the ICP by a carrier gas (Ar) through an inert tube. ETV-ICP-MS is specially well suited for the direct analysis of solids (0.1–5 mg) and slurries,51 as well as for liquid samples (5 to 50 μL) such as oil or petroleum products,52 because the organic matrix is easily removed. ETV-ICP-MS can offer lower detection limits and less risk of interferences as compared with sample nebulization. In addition, it is very convenient for combination with micro-extraction procedures.53,54 An excellent critical review was published in 2009 about ETV-ICP-MS.46 In this paper it was stated that despite that ETV-ICP-MS was initially received with great enthusiasm by the scientific community owing to the potential advantages that the coupling could bring, it did not enjoy an increased success along the years. This continues to be the same more than ten years later. In any case, it is worth noting that some interesting applications have been recently developed for ETV-ICP-MS in the biological field, such as the analysis of trace elements in small amounts of cell samples without sample preparation.55
The analysis of dried matrix spots (DMS) on a filter paper, most commonly dried blood spots (DBS), has received attention for biochemical analysis. Development of the DBS methodology was related to a high-scale application of neonatal screening programs in hospitals because the analytical procedure requires a very small volume of blood (a few microliters), just requiring pricking the heel or finger.48 In addition, requirements for transportation and storage of DMS biological specimens are less strict than those for liquid samples, enabling home collection and posting them to the lab by ordinary mail. There are commercially available filter papers with different characteristics that can be used for clinical purposes. Two main requirements to achieve reproducible results in the analysis of a DMS are (i) that the capacity of absorption and dissemination of the sample on the filter paper is uniform, and (ii) that these properties are maintained between the same and different lots.48 Two main approaches can be followed for elemental and isotopic determination of DMS: (i) analyte extraction or sample digestion, or (ii) direct analysis of the DMS with techniques such as LA-ICP-MS.56–58 Examples of elemental and isotopic analysis of DBS specimens will be described in the applications section, collecting some pros and cons of the methodology. In addition to blood, other types of fluids, such as urine, have been analysed by LA-ICP-MS using the DMS methodology.59,60
On the other hand, the DLTV sample introduction approach employs a low-cost continuous-wave diode laser for analysis of very small amounts of sample (200 nL droplets deposited with a micropipette, or 65 pL with a piezoelectric dispenser).50,61 Laser power is sufficient to induce pyrolysis of a suitable substrate with a deposited sample leading to aerosol generation. This demonstrated its suitability for the determination of Pb and Cd in whole human blood without any sample treatment.50 However, to date, DLTV has been scarcely analytically exploited.
3. Applications for disease evaluation and/or diagnosis
In this section, representative applications of elemental and isotopic analysis focused on the use of low volumes of biological fluids are reviewed. For the samples easily accessible at relatively high volumes, focus has been exclusively on those examples where microsamples were analysed, or in method development aiming at the analysis of low sample volumes.
Sample preparation of biological fluids for ICP-MS can be considered as simple. Biofluids are usually either thermally digested or just diluted before analysis. For thermal digestion, samples are typically heated with a mixture of nitric acid and hydrogen peroxide.62 On the other hand, dilute acids (e.g. nitric acid) or alkali solutions (e.g. ammonium hydroxide or tetramethylammonium hydroxide) are commonly employed diluents for direct analysis of the biological fluids. The concentration of the acid must be low (typically below 0.5%) to prevent precipitation of proteins and cell debris, while in the case of using alkali solutions a complexing agent such as EDTA is also added to avoid metal precipitation in basic media. A surfactant such as Triton™ X is frequently incorporated into the samples to help rinse the spray chamber and to aid the solubilisation of proteins and cell membranes. In some cases, a small concentration of butanol (e.g. 1%) is included as a source of carbon to minimize differences between samples and standards.63
For the calibration of samples which have not been previously digested, the use of matrix-matched standards is typically preferred in an attempt to mimic the matrix of the sample. For complex sample matrices where external calibration with matrix-matched standards is found to be inadequate to correct for matrix effects, the method of standard additions may be used.64 In addition, as in most ICP-MS methods, an internal standard is usually added to standards and samples in order to correct for changes in instrument operating conditions and sample-specific matrix effects which may enhance or suppress the analyte signal.65 Finally, it must be noted that particular care must be taken in the introduction of non-digested samples into the ICP to avoid nebulizer and injector clogging. Also, deposition of carbon compounds in the torch and/or onto sampler and skimmer cones will cause destabilization or extinction of argon plasma. Such problems may be circumvented by the addition of oxygen to the composition of the plasma.66 However, in most cases this is not necessary. Considering that microsamples are introduced, a simple sample dilution is enough to work for long periods with ICP-MS.
Below, applications are divided into two groups, depending on whether an invasive sample collection procedure is required or not.
3.1. Biological fluids requiring invasive sample collection
Blood is the biological fluid where elemental and isotopic analysis has been most widely studied within a clinical context. Also, cerebrospinal fluid (CSF) has been rather deeply investigated. Among other types of human biological fluids, follicular fluid, amniotic fluid, fetal urine, peritoneal fluid, synovial fluid, and intraocular fluids such as vitreous and aqueous humours can be included.
3.1.1. Blood serum and whole blood.
Despite the invasive nature of sample collection, blood extraction by venipuncture is a very common procedure and an informed consent from individuals is usually easily accessible. The sample volume cannot be considered an issue, though there are exceptions, such as for new-borns/infants, or when repeated sampling is required (as for chronic patients). For these cases, the analysis of DBS on filter paper is an attractive approach. For instance, Moreda-Piñeiro et al.56 presented a method for the quantitative determination of several major (K, Na and S), minor (Ca, Cu, Fe, Mg, P, Rb and Zn) and trace elements (Al, As, Ba, I, Li, Pb, Sb, V and T) in DBS via LA-ICP-MS. Later, the same group published an article focused on analysis of DBS samples for metabolic disorder diagnosis in new-borns.57 Moreover, a methodology was developed for the quantitative analysis of Ga-based contrast agents (metallodrugs) based on DBS sampling and LA-ICP-MS measurements.58 Also, it is interesting to note that Aramendia et al.67 demonstrated the potential to couple a LA chamber simultaneously (by split-flow) to a MC-ICP-MS and a SF-ICP-MS, thus allowing both elemental (Co, Cu, Cd and Pb) and isotopic (Cu) information to be obtained from DBS samples.
A minimal blood sample volume can also be collected through volumetric absorptive microsampling (VAMS®), overcoming some pitfalls of DBS. The VAMS sampler consists of a holder on which an absorptive porous substrate is attached (see Fig. 3). This substrate takes up a fixed volume of specimen (e.g. 10 μL) when exposed to a liquid blood sample. After collection, samples can be dried and stored or directly analysed.68,69 In fact, the device resembles a pipette tip, so it can be easily integrated into an automated sample preparation procedure. VAMS shares the benefits of DBS sampling in terms of easiness, sample stability and transport efficiency. Since a constant and known volume is obtained, it becomes easier to carry out a quantitative analysis (classic DBS approaches required the use of a pipette in the sampling location to achieve that). In addition, VAMS has proven to overcome the variability associated with differences in viscosity and spreadability between blood samples spotted on filter paper from different patients.70 VAMS was applied for the determination of ultra-trace amounts of prosthesis-related metals by ICP-MS70,71 and for Fe elemental and isotopic analysis.72
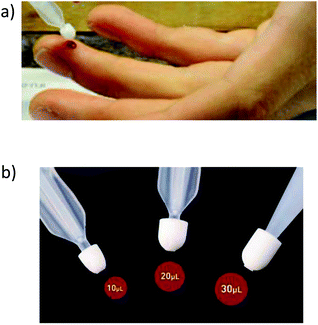 |
| Fig. 3 Volumetric absorptive microsampling. (a) Blood collection with vams®. (b) Tips for different blood volumes (https://www.neoteryx.com/mitra-clamshell-blood-collection-device). | |
Literature collection methods aim at multielemental analysis of low sample volumes, with or without acid digestion, before nebulization and ICP-MS measurement. For example, Fu et al.73 published an article focused on the rapid determination of Mn, Fe, Ni, Cu, Zn, and Se in the serum of hepatocellular carcinoma (HCC) patients by tandem ICP-MS. 100 μL serum specimens diluted to 2 mL with 1% (v/v) HNO3 were analyzed. The samples were introduced into the ICP source with a MicroMist glass concentric nebulizer and a Peltier-cooled quartz double-pass Scott-type spray chamber. N2O gas was chosen as the reaction gas, and the mass shift method was used to eliminate spectral interferences. Elemental analysis of 210 samples (105 inpatients and 105 healthy controls) revealed higher contents of Fe and Cu and lower contents of Zn and Se in the serum of HCC patients compared to controls, whereas for Mn and Ni significant differences were not found between the two cohorts. Another example requiring only 30 μL serum63 focused on Fe, Cu and Zn elemental determination with a 100-fold dilution of the serum specimens with 1.0% butanol, 0.5% v/v ammonia, 0.02% v/v Triton X-100 and 0.01% v/v HNO3. After validation, the method was applied to determine Zn, Cu and Fe in human serum samples from Alzheimer’s disease (AD) and age-related macular degeneration (AMD) patients. No significant differences were found for Zn and Cu levels between age matched controls, AD and AMD patients. Fe showed a larger overall variability as compared to Cu and Zn, Fe being marginally higher in the AMD group, compared with the AD group.
The concentrations of heavy metals in the sera of umbilical cord blood can provide information about prenatal exposure. The in utero exposure to metallic and metalloid elements was investigated by ICP-MS.74 The method required 100 μL of serum diluted 1
:
25 for direct analysis. Matrix-matched standards and kinetic energy discrimination were employed to eliminate mass matrix interferences and polyatomic ion interferences, respectively.
For isotopic analysis, most investigated elements so far are Fe, Cu and Zn, whose levels in whole blood/serum are accessible for MC-ICP-MS measurements. More recently, isotopic analysis of other metabolically relevant elements (e.g. S, Ca and Mg) has started to increase. Although much effort is made for method development for the measurement of accurate and precise isotopic composition, there is still work pending to extend its use for clinical purposes, especially analysing bigger cohorts. In this line, Table 1 shows applications focused on diagnosis/prognosis of different pathologies62,75–93 (examples focused on method development have not been considered). Most of the applications deal with Cu followed by Fe. Few examples can be found for S, Zn, Mg and Ca, but still they underpin the potential of isotopic analysis for diagnosis/prognosis of different pathologies.
Table 1 Isotopic determinations by MC-ICP-MS, in human blood, to assess differences between diseased people versus controls
Element |
Isotopic composition |
Pathology |
Sample introduction system |
Summary of results |
Ref. |
Cu |
δ65Cu |
Ovarian cancer |
— |
The Cu isotopic composition was lighter in ovarian cancer patients than in healthy subjects |
75
|
Age related macular degeneration (AMD) |
100 μL min−1 PFA nebulizer and a dual spray chamber, consisting of a cyclonic and a Scott-type sub-unit |
The Cu isotopic composition was lighter in AMD patients compared to controls |
62
|
Cholestatic liver disease |
100 μL min−1 PFA nebulizer and a dual spray chamber, consisting of a cyclonic and a Scott-type sub-unit |
The Cu isotopic composition of the patients with chronic liver disease was fractionated in favour of the lighter isotopes |
76
|
Liver transplant patients |
100 μL min−1 PFA nebulizer and a dual spray chamber, consisting of a cyclonic and a Scott-type sub-unit |
The isotopic composition in the blood serum of patients with end-stage liver disease was fractionated in favour of the lighter isotope |
77
|
Liver cirrhosis (LC) |
100 μL min−1 PFA nebulizer and a dual spray chamber, consisting of a cyclonic and a Scott-type sub-unit |
The Cu isotopic composition (δ65CuEXCH+UF and δ65Cuserum values) was lower in patients with alcoholic LC compared to controls |
78
|
The isotopic composition in the blood serum of patients with end-stage liver disease was fractionated in favour of the lighter isotope |
79
|
Cancer (breast and colorectal) |
— |
Breast and colorectal cancer patients showed lower δ65Cu values than healthy subjects |
80
|
Parkinsonism |
— |
Individual isotopic profiles showed that Cu metabolism is less controlled in patients compared to controls |
81
|
Wilson's disease |
Sample introduction was accomplished via a 100 μL min−1 PFA nebulizer mounted onto a double pass spray chamber (a combination of a Scott-type and a cyclonic spray chamber) |
Cu in serum samples from patients is significantly fractionated towards the lighter isotope when compared to healthy individuals |
82
|
Non-alcoholic fatty liver disease (NAFLD) |
200 μL min−1 quartz concentric nebulizer and a cyclonic spray chamber |
Serum δ65Cu values were lower in NAFLD compared to healthy controls |
83
|
Fe |
δ56Fe |
Ferroportin Q248H mutation/diabetes |
Low-flow PFA concentric nebulizer (100 μL min−1) and a quartz spray chamber with a cyclonic and a Scott-type sub-unit |
Significantly higher δ56Fe values in diabetic patients |
84
|
δ56Fe |
Chronic kidney disease (CKD) |
100 μL min−1 PFA concentric nebulizer and a high-stability dual spray chamber with cyclonic and Scott-type sub-units |
The serum Fe isotopic composition was heavier in patients with CKD and/or iron disorders than controls |
85
|
δ57Fe |
δ56Fe |
Hemochromatosis type I/anaemia of chronic disease (ACD) |
200 μl min−1 quartz concentric nebulizer and a cyclonic spray chamber |
The whole blood of patients with ACD shows a light Fe isotopic composition, whereas heavy Fe isotopic signature was obtained in the case of hemochromatosis patients. The results for hemochromatosis patients were demonstrated to coincide with low iron status, while those for ACD patients coincided with high iron status |
86
|
δ57Fe |
δ56Fe |
Hereditary hemochromatosis |
— |
Higher δ56Fe values were found in patients with hemochromatosis when compared with healthy individuals |
87
|
Fe, Cu & Zn |
δ65Cu |
Bariatric surgery |
100 μL min−1 PFA nebulizer and a dual spray chamber, consisting of a cyclonic and a Scott-type sub-unit |
Lower δ65Cu values were obtained in serum and whole blood after bariatric surgery (it became gradually lighter over time), while Δ66Zn (difference between serum and whole blood) was significantly increased at 6 months post-surgery |
88
|
δ56Fe |
δ66Zn |
Hematological malignancy |
— |
The Cu isotopic composition was significantly lower than the control group, whereas δ66Zn tended to increase in HM patients |
89
|
Mg |
δ25Mg |
Diabetes type 1 |
100 μL min−1 PFA nebulizer and a dual spray chamber, consisting of a cyclonic and a Scott-type sub-unit |
The serum Mg isotopic composition was significantly lighter in diabetes type 1 patients than for the reference population |
90
|
δ26Mg |
S |
δ34S |
Breast & colon and liver & prostate cancer/hepatitis and rheumatoid arthritis |
Cetac Aridus II desolvator (100 μL min−1 PFA nebulizer) |
The isotopic composition of patients with non-malignant liver pathologies tends to be light, whereas breast and prostate cancer and rheumatoid arthritis do not seem to affect the average δ34S serum values |
91
|
Cu and S |
δ65Cu |
Hepatocellular carcinoma patients |
— |
Lighter δ65Cu and δ34S values were reported in the blood of patients compared with that of control subjects |
92
|
δ34S |
Ca |
δ44Ca |
Osteoporosis |
APEX IR desolvating nebulizer |
The blood Ca isotopic composition of women suffering from osteoporosis was significantly lower than the control group |
93
|
3.1.2. Cerebrospinal fluid.
CSF is a main source of clinical information. However, the need for lumbar puncture to obtain CSF restricts its analysis except for well-justified evaluations. The CSF volume in adults is estimated to be about 100–150 mL, while typical collected volumes by lumbar puncture are around 3–10 mL.94–96 However, such volumes are frequently not available for research purposes, as CSF is mostly dedicated to clinical diagnosis. In any case, many examples of elemental determination by ICP-MS in CSF can be found in the literature to investigate different pathologies, including chronic neuropathic pain,97 amyotrophic lateral sclerosis,98–100 AD,101,102 or Niemann-Pick,103 among others.
The high sensitivity of ICP-MS allows most of the studies reported dealing with elemental alterations for a certain pathology made use of sample dilution prior to ICP-MS measurements. Still, efforts aimed at the analysis of micro-volumes of CSF were pursued. For instance, Theiner et al.45 developed a high-throughput quantitative multi-elemental method for CSF specimens by flow injection of undiluted CSF, requiring sample volumes of only 5 μL. Also, direct injection of small volumes of CSF employing total consumption systems operating at high temperature prior to the CRC-ICP-MS determination has been carried out.104 In particular, non-diluted 2.5 μL CSF volume was injected into a carrier gas stream in combination with a HEN, thus providing a transient signal that was integrated for data analysis. Signal stability, oxides and double charged ions and non-spectral interferences were evaluated using the developed approach for the elemental determination of 14 elements in CSF samples both by external calibration and single-point standard addition.
Among the applications within a clinical context, alterations of essential trace elements (Mn, Co, Cu, Zn and Se) as well as contaminant elements (Al, Ni, As, Sr, Cd, Ce, Pt, Pb and U) in blood, CSF and tissue samples of patients with malignant brain tumours by means of CRC-ICP-MS have been investigated.105 CSF samples from brain tumour patients (n = 61) were compared to CSF samples of hydrocephalus patients (n = 51). Only Cd and Sr showed lower and higher concentrations with respect to the control group (p < 0.05, Mann Whitney U-test). In addition, it is worth highlighting that particular attention was paid to amyotrophic lateral sclerosis. Vinceti et al.98,100 published several articles focused on analysis and speciation of Se by using ion-exchange chromatography, HPLC and ICP-MS, finding abnormal levels for this particular pathology.
To the best of the authors' knowledge, isotopic analysis of CSF has been tackled in two publications (see Table 2). One of them presents a method for Mg isotopic analysis via MC-ICP-MS using high-gain 1013 Ω Faraday cup amplifiers. The accuracy and the precision of the Mg isotope ratios measured at a 7 μg L−1 concentration level using 1013 Ω resistors for the 25Mg and 26Mg isotopes were only slightly deteriorated as compared to those of the conventional method. Accurate and precise Mg isotope ratios could be achieved using about 5 μL of human CSF.12 The method was applied for CSF collection from hydrocephalus patients. The other publication refers to the isotopic determination of Cu and Zn in patients with amyotrophic lateral sclerosis or Alzheimer’s disease.106
Table 2 Isotopic determination by MC-ICP-MS in human biological fluids, different than blood, to assess differences between diseased people versus controls
Element |
Isotopic composition |
Body fluid |
Pathology |
Sample introduction system |
Summary of results |
Ref. |
Mg |
δ26Mg |
Cerebrospinal (CSF) |
Hydrocephalus |
100 μL min−1 PFA concentric nebulizer mounted onto a double spray chamber with cyclonic and Scott-type sub-units for sample introduction into the plasma |
Hydrocephalus patients showed in CSF an isotopic composition of Mg similar to plasma values from healthy subjects. In contrast, this composition was found heavier than the quality control CSF material and lighter than the Seronorm reference material |
12
|
Cu and Zn |
δ65Cu |
|
Amyotrophic lateral sclerosis (ALS) and Alzheimer disease (AD) |
— |
In patients with AD, δ66Zn values were found slightly lighter in comparison with the control group. In the case of Cu, heavier delta values were observed in ALS patients |
106
|
δ66Zn |
Cu and Zn |
δ65Cu |
Aqueous humor |
Glaucoma disease |
— |
Patients with pseudoexfoliation glaucoma showed lower δ66Zn in comparison with healthy subjects. In contrast, no significant change in isotopic composition of Cu was detected |
107
|
δ66Zn |
3.1.3. Intraocular fluids.
Aqueous and vitreous humours are colourless intraocular fluids vital in the physiology of the eye. Aqueous humour helps to maintain intraocular pressure and structural integrity of the ocular globe, provides substrates and removes metabolites from the cornea, lens and trabecular meshwork, and participates in immune and antioxidant responses.107 Vitreous humour is a gel-like substance that fills the space between the lens and the retina, providing the round shape of the eye and also contributes to vision clarity.
Access to aqueous humour is enabled by using a needle placed in the anterior chamber of the eye and volumes collected are typically below 250 μL.108 Elemental concentrations of aqueous humour have been determined by ICP-MS to investigate ocular disorders such as glaucoma,109,110 AMD,111 cytomegalovirus retinitis,112 or pseudoexfolation syndrome.113 Changes in the aqueous humour isotopic composition may provide relevant information, complementary to that embedded in the element concentrations. A recent publication analysing aqueous humour of control and glaucoma individuals has shown statistical differences in terms of elemental concentrations of P and Mg (Na, K, Fe, Cu and Zn were investigated as well) and of the Zn isotopic composition (see Table 2).107
The volume of vitreous humour is higher than that of aqueous humour. Vitreous humour is accessible via surgical vitrectomies and biopsies for medical reasons. Given the invasiveness of vitreous sampling, collection of vitreous reflux after intravitreal injections has been explored. Very few publications are available within the scope of this review.114–116 Collected post-mortem vitreous humour has been analysed, and so publications are mostly focused on forensic and toxicological fields.115,117
3.1.4. Examples of other body fluids: follicular fluid, amniotic fluid, fetal urine, peritoneal fluid and synovial fluid.
Follicular fluid (FF) is a blood-plasma ultrafiltrate that envelops oocytes in the ovarian follicle. During in vitro treatment, oocytes and the surrounding FF are obtained via a transvaginal needle. The FF is separated from the oocyte(s) during processing. Elemental analysis of FF can be of interest to assess the trace element content as well as to determine potential exposure to toxic elements in women seeking in vitro fertilization treatment. For instance, Galusha et al.118 recently investigated variations of 4 elements at trace levels (Cu, Se, Sr, and Zn) and 7 at ultra-trace levels (As, Cd, Co, Mo, Mn, Hg, and Pb) in 197 human FF diluted specimens using a MicroMist nebulizer and tandem ICP-MS. The results showed a significantly lower concentration of Cd and Pb as compared to previous studies, this being attributed to the efforts to minimise sample contamination during the collection step.
Samples of amniotic fluid, the protective liquid that surrounds the fetus during pregnancy within the amniotic sac, can be obtained by amniocentesis. A few mL of liquid is extracted through a fine needle inserted into the uterus through the abdomen, under ultrasound guidance. Current knowledge of the concentration of elements in amniotic fluid and their role in fetal growth is limited. However, considering that the fetus swallows amniotic fluid, it may be an essential source of dietary components. In this line, determination of 18 essential and toxic elements in amniotic fluid samples by ICP-MS has been carried out.119 Samples were collected during cesarean section by transabdominal puncture just before delivery. An aliquot of each sample was digested with HNO3 and H2O2. A dynamic reaction cell (DRC) with two reaction gases, ammonia and oxygen, was employed in the measurement with ICP-MS to eliminate spectral interferences. Particular attention was focused on the validation of the procedure and evaluation of traceability and uncertainty. Analysis of amniotic fluid from 50 healthy Caucasian women at birth was carried out. Although the cohort is small, it contributes to further establish reference values for the concentration of selected essential and toxic elements in the amniotic fluid.
Regarding fetal urine, a method for the determination of 21 macro and trace elements in very low volumes (200–1000 μL) using a single analytical run by ICP-MS equipped with a DRC has been proposed.120 For sampling, a vesico–amniotic shunting procedure was applied under continuous ultrasound guidance using a shunt that was inserted through the maternal abdomen. A MicroMist nebulizer (0.1 mL min−1) and a low volume spray chamber (20 mL cyclonic Cinnabar) were employed. The samples were diluted with HNO3 and analysed without further treatment. The analytical procedure was applied to 58 samples collected from human foetuses. The comparison of measured concentrations of physiological elements with the literature provides no definitive conclusions. However, the concentration of toxic elements, As, Cd and Pb, was found lower in fetal urine than in the adult urine, which might be due to the additional mechanisms of protection from xenobiotics in the mother's placenta.
Peritoneal fluid is a serous fluid (commonly 5 to 20 mL total) in the abdominal cavity, which lubricates the surface of organs in the abdomen. Sampling of peritoneal fluid is generally performed by paracentesis. An interesting application of ICP-MS relates to the study of the evolution of the concentration of platinum after hyperthermic intraperitoneal perioperative chemotherapy (HIPC) with oxaliplatin in a patient diagnosed with peritoneal carcinomatosis.121 Plasma, plasma ultrafiltrate, urine and peritoneal fluid were sampled at 5 min time intervals. Specimens were diluted 1000-fold with 0.5% HNO3 and mixed on a vortex-mixer prior to ICP-MS analysis using a PFA microflow nebulizer and a quartz cyclonic (Peltier cooled) spray chamber. The method could be easily implemented in a routine setting for pharmacokinetic studies in patients treated with oxaliplatin-based HIPC.
Synovial fluid, a viscous fluid (with an egg white-like consistency) found in the knee joint, has a total volume usually below 3.5 mL. Its principal role is to reduce friction between the articular cartilage of synovial joints during movement. Sixteen elements in 250 μL synovial fluid samples taken from patients with rheumatoid arthritis were determined by ICP-MS.122 Before analysis, the samples were pretreated with 250 μL formic acid, and the mixture was shaken vigorously. Then, the flask was kept at 90 °C in a water bath for 30 min and the volume was completed to 2.5 mL with water and nitric acid to a final concentration of 1%. The use of a PFA microconcentric nebulizer operating at a low flow rate (200 μL min−1) and a cooled quartz cyclonic spray chamber enabled the introduction of sample solutions with high organic contents, thus, allowing the development of a fast sample pretreatment method to identify the elemental profile of human synovial fluid generated in joint disorders. Also, an ICP-MS method was developed to determine Co in synovial fluid to investigate leaching of implants containing this element.123
3.2. Biological fluids allowing non-invasive sample collection
In recent years, increasing attention is being paid to the determination of metals/isotopes in human biofluids not requiring invasive sampling, such as urine, saliva, lacrimal fluid, nasal exudate, seminal fluid, breast milk and sweat to establish correlations with the human body state or to detect particular diseases. Of course, collection procedures and available sample volumes are very different for each type of fluid. Below are presented examples for most of the fluids above collected, excepting seminal fluid and breast milk. Elemental concentrations of seminal fluids have been principally investigated to evaluate semen quality in terms of male reproductive parameters,124,125 or to correlate the concentrations of elements depending on donors' habits (e.g. Cd for smokers).126 Regarding breast milk, a lot of research has been done along the years with varied goals, such as to investigate the effect of maternal dietary intake on breast milk composition,127 the potential exposure of infants to toxicants through breast milk,128 or to establish reference values for elaboration of formula milk (baby formula or infant formula).129 Considering that this fluid is mostly used for feeding purposes, we consider that a detailed description of its characterization by ICP-MS is out of the scope of this review.
3.2.1. Urine and saliva.
The elemental and/or isotopic analysis of urine can provide information about body diseases,59,130 liberation of metals from implants,131 intoxication with heavy metals132,133 and their removal along treatment,134 contamination with actinides,36,135etc. In addition, sp-ICP-MS has been investigated for the characterization and determination of nanoparticles (NPs) made of titanium dioxide,136,137 silver,137 or platinum138 expelled in urine. On the other hand, elemental and/or isotopic analysis of saliva can be of interest to evaluate mouth diseases such as periodontitis139 and periodontal status,140 taste disorders,141 the liberation of metals from dental implants and orthodontic appliances,142 the effects of smoking,143 and illnesses such as diabetes mellitus.144 In addition, these fluids have been investigated as alternatives to blood for the routine control of workers at risk of occupational exposure (e.g. to lead).145
Sample uptake is easy in both cases, though some care must be taken to avoid the influence of recent food intake or digestion. Obviously, urine and saliva are fluids with few or no restrictions related to volume availability. In any case, some methods requiring low volumes have been developed, such as the analysis of dried urine spots by LA-ICP-MS either for the direct determination of several elements in urine,59 or for the determination of Cu isotope ratios in order to diagnose Wilson's disease.60 Also, the analysis of nanovolumes by flow injection,36 or the use of continuous sample introduction systems at low flow rates using a DIHEN nebulizer135 (0.06 μL min−1) and a LB-DIHEN132 (120 μL min−1), allowing the use of high volumes of contaminated samples135 and rinse-out times of elements susceptible to memory effects such as Hg to be reduced,132 has been proposed.
3.2.2. Lacrimal (tear) fluid.
Lacrimal (or tear) fluid consists of a thin film formed by an external lipid layer, a middle aqueous layer and an inner protein layer. It is mostly composed of water (90%) and to a much lesser extent by proteins, lipids, electrolytes and trace elements. Tear production is estimated to be at the 1 μL min−1 rate.146 Tears are collected either by a glass microcapillary of 5–10 μL inner volume (see Fig. 4a), with Schirmer strips (see Fig. 4b), or other absorbent materials such as minisponges, or flushing the eye with a saline solution that is later collected.108 In the first case, the microcapillary is placed at the inferior meniscus of the eye (blinking is recommended to prevent induction of reflex tearing).147 The Schirmer test consists of placing paper strips inside the lower eyelid (inferior fornix) of both eyes for a fixed time to measure production of tears. If required, analytes can then be leached out from the strip for (bio)chemical analysis (with the subsequent risk of contamination, or loss of the analytes due to not quantitative recoveries). On the other hand, in the case of using a saline solution to collect the tear, it must be taken into account that sample dilution is accomplished.
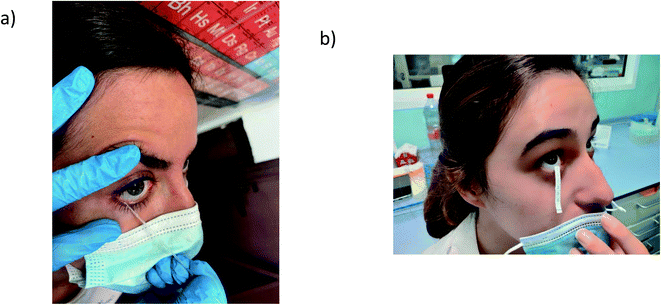 |
| Fig. 4 Collection of tears for analysis: (a) collection with a glass microcapillary. (b) Collection with schirmer strips. | |
Tear fluid has been analysed to investigate ocular disorders by means of proteomics and lipidomics.148,149 However, very few research articles can be found for trace elemental determination in human tears. In 2017, Cancarini et al.150 determined concentrations of Cu, Zn Se, Rb, Ba, Pb, Mn, Co and Cr in tear fluid and serum of Diabetes type II individuals (n = 47) with respect to a non-diabetic group (n = 50). 10 μL tear fluid was collected using Schirmer strips, and then subjected to acid exposure. A 1
:
500 dilution allowed for ICP-MS quantitative analysis by external calibration. Investigated elements were found to be at the ng mL−1 level. After statistical analysis, higher levels were found for Zn, Cr, Co, Mn, Ba and Pb in tear fluid from diabetic patients as compared to the control group. Following a similar methodology, lower levels of Pb and Ba and higher levels of As were found in tears of supposedly healthy individuals from rural areas as compared to urban living areas.151
3.2.3. Nostril fluids.
The elemental content of nostril fluids has not been much investigated. However, the recent description of the cerebral lymphatic drainage system, showing vessels draining to the nostrils and cervical lymph nodes,152 opened up new opportunities for obtaining information from the central nervous system. The olfactory nerve, which crosses the cribriform plate at the base of the ethmoid bone, leaves room for releasing soluble species and cells. Within this context, two studies153,154 have shown that the iron concentration in nasal exudates (sampling was carried out with swabs making circular movements in the area closest to the upper turbinate for 3 seconds in each nostril, stored at 4 °C and diluted in a 2% HNO3 solution before ICP-MS measurement) can allow differentiation between haemorrhagic and ischemic strokes. Thus, determination of metals and metalloids at trace and ultratrace levels in small nasal exudate samples deserves further studies because interesting information from the brain could be achieved.
In a forensic study gunshot residues (GSRs) in nasal samples were detected by monitoring 15 elements that are usually associated with GSRs. Nostrils were sampled by rubbing a swab (pre-moistened in a solution of 2% EDTA) in the same direction throughout the inner wall of the nostril to collect inorganic gunshot residues after shooting, and then the swabs were analysed by LA-ICP-MS.155 The results proved that the GSRs persisted for longer periods in nasal mucus than on the hands of the shooters, and that the LA method enabled the reduction of the swab analysis time.
3.2.4. Sweat.
Numerous factors can significantly impact the reliability of sweat analysis including anatomical site of sweat sampling, skin integrity and preparation, temperature and humidity at the sweat collection room, sweat collection method, etc.156 Procedures for sweat sampling after induction of perspiration or just passive sampling can be carried out. Commercial sweat collection devices are available such as the Macroduct® sweat collector (see Fig. 5a) or the PharmChek® sweat patch (see Fig. 5b). Of course, non-commercial techniques of sweat collection have also been described.156
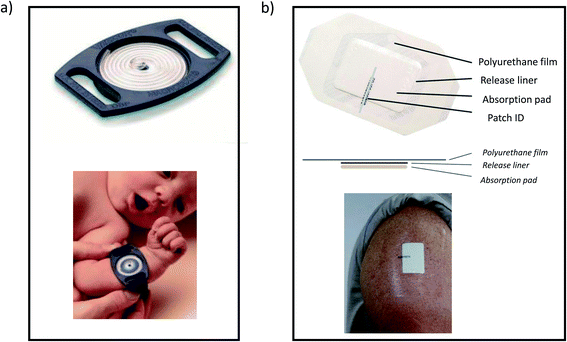 |
| Fig. 5 Examples of commercial sweat collection devices: (a) macroduct® sweat collector from elitechgroup (https://www.elitechgroup.com/germany/product/macroduct-sweat-collection-system-2), (b) pharmchek® sweat patch from pharmchem, inc. (https://www.pharmchek.com/products/pharmchek-patch). | |
The Macroduct® sweat collector employs a plastic capillary-coil device: sweat secreted by the sweat glands is forced from the ducts under hydraulic pressure and flows between the skin and the concave undersurface of the Macroduct collector and into the micro bore tubing spiral. The system allows an exact volume of sweat to be collected. The PharmCheck® sweat patch consists of a medical-grade absorption pad covered by a thin layer of polyurethane and an adhesive to fix against the skin. The adhesive film of the patch is a semi-permeable membrane that allows oxygen, carbon dioxide, and water vapour to pass through, while non-volatile species are trapped in the absorbent pad of the patch.
Several research studies related to elemental analysis of sweat by ICP-MS address studies of elimination of bioaccumulated toxic elements, comparing normothermic and hyperthermic conditions, or after physical exercise.157,158 In other studies, the release of essential elements such as Fe159 or Mg and P160 has been investigated. Although a non-metal, a few words must be reserved for the quantification of chloride in sweat of new-borns by ICP-MS to detect cystic fibrosis, the commonest, inherited life-shortening condition of childhood.161,162 Coulometric titration is commonly used for such purposes; however ICP-MS has proved to offer sensitivity for the recently reduced sweat Cl reference interval accepted for infants. Other advantages include the low sample volume requirements and the addition of an internal standard.
4. Conclusions
The high sensitivity provided by ICP-MS allows its application to many multielemental and isotopic analyses of biological fluids, just making use of a simple dilution step. However, for analysis of elements at very low concentrations this is not feasible because measured levels will be below detection limits. This makes it mandatory to resort to special microsampling introduction systems if very low sample volumes are available. For such purposes, strategies such as micronebulizers for continuous sample aspiration, flow injection, ETV-ICP-MS, LA sampling of DMS, etc., have been developed and applied, all of them with advantages and disadvantages. At present, efforts are being made to develop new or improved sample introduction systems, such as on-line coupling of microfluidic systems to ICP-MS. Over the last decade, this combination has been mainly exploited for the analysis of droplet encapsulated single cells and lysed cell populations.44 In addition, recent work addressed the accurate metal determination in any sample of limited volume using microfluidic chips connected to ICP-MS.163
To select the sample introduction method, not only the characteristics of the biological fluid, but also the intended application (e.g. number of samples, place of sample uptake, etc.) must be taken into account. Regarding this last issue, and especially for those biofluids for which it is not so common to carry out elemental/isotopic determination, particular care is needed to develop updated protocols of the sampling procedure and the storage, to avoid sample contamination with the sought elements.
Conflicts of interest
There are no conflicts to declare.
Acknowledgements
This work was supported through project PID2019-107838RB-I00/Agencia Estatal de Investigación (AEI)/10.13039/501100011033). E. Valencia-Agudo acknowledges the FPI Grant (Ref. BES-2017-080518) from the Ministry of Economy and Competitiveness. M. Aranaz acknowledges the “Plan de Apoyo y Promoción de la investigación” grant (Ref. PAPI-20-PF-04) of the University of Oviedo.
References
- M. A. Zoroddu, J. Aaseth, G. Crisponi, S. Medici, M. Peana and V. M. Nurchi, The essential metals for humans: a brief overview, J. Inorg. Biochem., 2019, 195, 120–129 CrossRef CAS PubMed.
- C. M. Weekley and C. He, Developing drugs targeting transition metal homeostasis, Curr. Opin. Chem. Biol., 2017, 37, 26–32 CrossRef CAS.
- M. Merola and S. Affatato, Materials for hip prostheses: a review of wear and loading considerations, Materials, 2019, 12, 495 CrossRef CAS.
- A. Sarmiento-González, J. M. Marchante-Gayón, J. M. Tejerina-Lobo, J. Paz-Jiménez and A. Sanz-Medel, ICP-MS multielemental determination of metals potentially released from dental implants and articular prostheses in human biological fluids, Anal. Bioanal. Chem., 2005, 382, 1001–1009 CrossRef.
- N. B. Ivanenko, A. A. Ganeev, N. D. Solovyev and L. N. Moskvin, Determination of trace elements in biological fluids, J. Anal. Chem., 2011, 66, 784–799 CrossRef CAS.
- R. S. Amais, G. L. Donati and M. A. Z. Arruda, ICP-MS and trace element analysis as tools for better understanding medical conditions, TrAC, Trends Anal. Chem., 2020, 133, 116094 CrossRef CAS.
- J. Hu, D. Deng, R. Liu and Y. Lv, Single nanoparticle analysis by ICPMS: a potential tool for bioassay, J. Anal. At. Spectrom., 2018, 33, 57–67 RSC.
- D. Mozhayeva and C. Engelhard, A critical review of single particle inductively coupled plasma mass spectrometry – a step towards an ideal method for nanomaterial characterization, J. Anal. At. Spectrom., 2020, 35, 1740–1783 RSC.
- H. Wang, M. He, B. Chen and B. Hu, Advances in ICP-MS-based techniques for trace elements and their species analysis in cells, J. Anal. At. Spectrom., 2017, 32, 1650–1659 RSC.
- M. Corte-Rodriguez, R. Álvarez-Fernández, P. García-Cancela, M. Montes-Bayón and J. Bettmer, Single cell ICP-MS using on line sample introduction systems: Current developments and remaining challenges, TrAC, Trends Anal. Chem., 2020, 132, 116042 CrossRef CAS.
- M. Costas-Rodriguez, L. Lobo and F. Vanhaecke, A novel approach to measure isotope ratios via multi-collector—inductively coupled plasma—mass spectrometry based on sample mixing with a non-enriched standard, Anal. Bioanal. Chem., 2014, 406, 4393–4399 CrossRef CAS PubMed.
- R. Grigoryan, M. Costas-Rodriguez, P. Santens and F. Vanhaecke, Multicollector inductively coupled plasma−mass spectrometry with 1013 Ω Faraday cup amplifiers for ultrasensitive Mg isotopic analysis of cerebrospinal fluid microsamples, Anal. Chem., 2020, 92, 15975–15981 CrossRef CAS.
- Q. Ma, M. Yang, H. Zhao, N. J. Evans, Z.-Y. Chu, L.-W. Xie, C. Huang, Z.-D. Zhao and Y.-H. Yang, Accurate and precise determination of Lu and Hf contents and Hf isotopic composition at the sub-nanogram level in geological samples using MC-ICP-MS, J. Anal. At. Spectrom., 2019, 34, 1256–1262 RSC.
- M. C. Garcia-Poyo, S. Berail, A. L. Ronzani, L. Rello, E. Garcia-Gonzalez, B. Lelievre, P. Cales, F. V. Nakadi, M. Aramendia, M. Resano and C. Pecheyran, Laser ablation of microdroplets for copper isotopic analysis via MC-ICP-MS. Analysis of serum microsamples for the diagnosis and follow-up treatment of Wilson’s disease, J. Anal. At. Spectrom., 2021, 36, 968–980 RSC.
- J. Zhang, J. Li, L. Zhang, Z.-B. Wang, S.-L. Sun and Z.-Y. Luo, Precise determination of the molybdenum isotopic composition of urine by multiple collector inductively coupled plasma mass spectrometry, Rapid Commun. Mass Spectrom., 2020, 34, 8658 Search PubMed.
- M. Montes-Bayón, M. Sharar and M. Corte-Rodriguez, Trends on (elemental and molecular) mass spectrometry based strategies for speciation and metallomics, TrAC, Trends Anal. Chem., 2018, 104, 4–10 CrossRef.
- N. H. Bings, J. O. Orlandini von Niessen and J. N. Schaper, Liquid sample introduction in inductively
coupled plasma atomic emission and mass spectrometry—Critical review, Spectrochim. Acta, Part B, 2014, 100, 14–37 CrossRef CAS.
- J. L. Todoli and J. M. Mermet, Sample introduction systems for the analysis of liquid microsamples by ICP-AES and ICP-MS, Spectrochim. Acta, Part B, 2006, 61, 239–283 CrossRef.
- J. L. Todoli and J. M. Mermet, Elemental analysis of liquid microsamples through inductively coupled plasma spectrochemistry, TrAC, Trends Anal. Chem., 2005, 24, 107–116 CrossRef CAS.
- K. E. Lokits, P. A. Limbach and J. A. Caruso, Interfaces for capillary LC with ICPMS detection: A comparison of nebulizers/spray chamber configurations, J. Anal. At. Spectrom., 2009, 24, 528–534 RSC.
- B. Franze, I. Strenge and C. Engelhard, Separation and detection of gold nanoparticles with capillary electrophoresis and ICP-MS in single particle mode (CE-SP-ICP-MS), J. Anal. At. Spectrom., 2017, 32, 1481–1489 RSC.
- A. P. Vonderheide, M. V. Zoriy, A. V. Izmer, C. Pickhardt, J. A. Caruso, P. Ostapczuk, R. Hillec and J. S. Becker, Determination of 90Sr at ultratrace levels in urine by ICP-MS, J. Anal. At. Spectrom., 2004, 19, 675–680 RSC.
- A. W. Boom, Sample introduction techniques for atomic spectroscopy, Anal. Chem., 1984, 56, 875A–888A CrossRef.
- J. F. Staff, A.-H. Harding, J. Morton, K. Jones, E. A. Guice and T. McCormick, Investigation of saliva as an alternative matrix to blood for the biological monitoring of inorganic lead, Toxicol. Lett., 2014, 231, 270–276 CrossRef CAS PubMed.
- E. Pérez, F. M. Marco, P. Martínez-Peinado, J. Mora and G. Grindlay, Evaluation of different competitive immunoassays for aflatoxin M1 determination in milk samples by means of inductively coupled plasma mass spectrometry, Anal. Chim. Acta, 2019, 1049, 10–19 CrossRef.
-
https://www.meinhard.com/shop-by-product/nebulizers/meinhard-classic-nebulizers/meinhard-classic-high-efficiency-nebulizers
.
-
https://www.geicp.com/cgi-bin/site/wrapper.pl?c1=Products_nebs_bytype
.
-
https://www.icpms.com/products/nebulizers-pfa-microflow.php
.
-
https://burgener.com/NebulizerSelectionCriteria.html
.
- M. Grotti, F. Ardini and J. L. Todolí, Total introduction of microsamples in inductively coupled plasma mass spectrometry by high-temperature evaporation chamber with a sheathing gas stream, Anal. Chim. Acta, 2013, 767, 14–20 CrossRef CAS.
- A. C. S. Bellato, M. F. Giné and A. A. Menegário, Determination of B in body fluids by isotope dilution inductively coupled mass spectrometry with direct injection nebulization, Microchem. J., 2004, 77, 119–122 CrossRef CAS.
- B. W. Acon, J. A. McLean and A. Montaser, A direct injection high efficiency nebulizer interface for microbore high-performance liquid chromatography-inductively coupled plasma mass spectrometry, J. Anal. At. Spectrom., 2001, 16, 852–857 RSC.
- J. A. Mclean, B. W. Acon, A. Montaser, J. Singh, D. E. Pritchard and S. R. Patierno, Determination of chromium in human lung fibroblast cells using a large bore direct injection high-efficiency nebulizer with inductively coupled plasma mass spectrometry, Appl. Spectrosc., 2000, 54, 659–663 CrossRef CAS.
- C. S. Westphal, J. A. Mclean, S. J. Hakspiel, W. E. Jackson, D. E. Mcclain and A. Montaser, Determination of depleted uranium in urine via isotope ratio measurements using large-bore direct injection high efficiency nebulizer–inductively coupled plasma mass Spectrometry, Appl. Spectrosc., 2004, 58, 1044–1050 CrossRef CAS PubMed.
- C. S. Westphal, K. Kahen, W. F. Rutkowski, B. W. Acon and A. Montaser, Demountable direct injection high efficiency nebulizer for inductively coupled plasma mass spectrometry, Spectrochim. Acta, Part B, 2004, 59, 353–368 CrossRef.
- D. Schaumlöffel, P. Giusti, M. V. Zoriy, C. Pickhardt, J. Szpunar, R. Lobinski and J. S. Becker, Ultratrace determination of uranium and plutonium by nano-volume flow injection double-focusing sector field inductively coupled plasma mass spectrometry (nFI–ICP-SFMS), J. Anal. At.
Spectrom., 2005, 20, 17–21 RSC.
- R. Sánchez, J. L. Todolí, C. P. Lienemann and J. M. Mermet, Air-segmented, 5-μL flow injection associated with a 200 °C heated chamber to minimize plasma loading limitations and difference of behaviour between alkanes, aromatic compounds and petroleum products in inductively coupled plasma atomic emission spectrometry, J. Anal. At. Spectrom., 2010, 25, 1888–1894 RSC.
- Á. Cañabate, E. García-Ruiz, M. Resano and J.-L. Todolí, Analysis of whole blood by ICP-MS equipped with a high temperature total sample consumption system, J. Anal. At. Spectrom., 2017, 32, 78–87 RSC.
- S. Miyashita, A. S. Groombridge, S. Fujii, A. Minoda, A. Takatsu, A. Hioki, K. Chiba and K. Inagaki, Highly efficient single-cell analysis of microbial cells by time-resolved inductively coupled plasma mass spectrometry, J. Anal. At. Spectrom., 2014, 29, 1598–1606 RSC.
- S. Meyer, A. López-Serrano, H. Mitze, N. Jakubowski and T. Schwerdtle, Single-cell analysis by ICP-MS/MS as a fast tool for cellular bioavailability studies of arsenite, Metallomics, 2018, 10, 73–76 CrossRef CAS.
- M. C. Rodriguez, R. Álvarez-Fernández, E. Blanco, J. Bettmer and M. Montes-Bayón, Quantitative evaluation of cisplatin uptake in sensitive and resistant individual cells by single-cell ICP-MS (SC-ICP-MS), Anal. Chem., 2017, 89, 11491–11497 CrossRef.
- S. Miyashita, A. S. Groombridge, S. Fujii, A. Minoda, A. Takatsu, A. Hioki, K. Chiba and K. Inagaki, Highly efficient single-cell analysis of microbial cells by time-resolved inductively coupled plasma mass spectrometry, J. Anal. At. Spectrom., 2014, 29, 1598–1606 RSC.
- S. Gschwind, L. Flamigni, J. Koch, O. Borovinskaya, S. Groh, K. Niemax and D. Günther, Capabilities of inductively coupled plasma mass spectrometry for the detection of nanoparticles carried by monodisperse microdroplets, J. Anal. At. Spectrom., 2011, 26, 1166–1174 RSC.
- M. He, B. Chen, H. Wang and B. Hu, Microfluidic chip-inductively coupled plasma mass spectrometry for trace elements and their species analysis in cells, Appl. Spectrosc. Rev., 2019, 54, 250–263 CrossRef CAS.
- S. Theiner, A. Schoeberl, L. Fischer, S. Neumayer, S. Hann and G. Koellensperger, FI-ICP-TOFMS for quantification of biologically essential trace elements in cerebrospinal fluid – high-throughput at low sample volume, Analyst, 2019, 144, 4653–4660 RSC.
- M. Aramendia, M. Resano and F. Vanhaecke, Electrothermal vaporization–inductively coupled plasma-mass spectrometry: A versatile tool for tackling challenging samples: A critical review, Anal. Chim. Acta, 2009, 648, 23–44 CrossRef CAS.
- A. Limbeck, P. Galler, M. Bonta, G. Bauer, W. Nischkauer and F. Vanhaecke, Recent advances in quantitative LA-ICP-MS analysis: challenges and solutions in the life sciences and environmental chemistry, Anal. Bioanal. Chem., 2015, 407, 6593–6617 CrossRef CAS.
- M. Resano, M. A. Belarra, E. García-Ruiz, M. Aramendia and L. Rello, Dried matrix spots and clinical elemental analysis. Current status, difficulties, and opportunities, TrAC, Trends Anal. Chem., 2018, 99, 75–87 CrossRef CAS.
- P. J. Parsons, A. L. Galusha, Y. Cui, E. M. Faustman, J. C. Falman, J. D. Meeker and K. Kannan, A critical review of the analysis of dried blood spots for characterizing human exposure to inorganic targets using methods based on analytical atomic spectrometry, J. Anal. At. Spectrom., 2020, 35, 2092–2112 RSC.
- P. Foltynova, A. Bednarık, V. Kanicky and J. Preisler, Diode laser thermal vaporization ICP MS with a simple tubular cell for determination of lead and cadmium in whole blood, J. Anal. At. Spectrom., 2014, 29, 1585–1590 RSC.
- A. S. Henn, E. M. M. Flores, V. L. Dressler, M. F. Mesko, J. Feldmann and P. A. Mello, Feasibility of As, Sb, Se and Te determination in coal by solid sampling electrothermal vaporization inductively coupled plasma mass spectrometry, J. Anal. At. Spectrom., 2018, 33, 1384–1393 RSC.
- J. S. Silva, A. S. Henn, V. L. Dressler, P. A. Mello and E. M. M. Flores, Feasibility of rare earth element determination in low concentration in crude oil: Direct sampling electrothermal vaporization-inductively coupled plasma mass spectrometry, Anal. Chem., 2018, 90, 7064–7071 CrossRef CAS PubMed.
- L. Li, B. Hu, L. Xia and Z. Jiang, Determination of trace Cd and Pb in environmental and biological samples by ETV-ICP-MS after single-drop microextraction, Talanta, 2006, 70, 468–473 CrossRef CAS.
- J. C. Ramos and D. L. G. Borges, Evaluation of electrothermal vaporization as a sample introduction technique for the determination of trace elements in biological samples by inductively coupled plasma mass spectrometry, following dispersive liquid–liquid microextraction, J. Anal. At. Spectrom., 2014, 29, 304–314 RSC.
- H. Wang, M. He, B. Chen and B. Hu, Advances in ICP-MS-based techniques for trace elements and their species analysis in cells, J. Anal. At. Spectrom., 2017, 32, 1650–1659 RSC.
- J. Moreda-Piñeiro, A. Cantarero-Roldán, A. Moreda-Piñeiro, J. Á. Cocho and P. Bermejo-Barrera, Laser ablation inductively coupled plasma mass spectrometry for multi-elemental determination in dried blood spots, J. Anal. At. Spectrom., 2017, 32, 1500–1507 RSC.
- J. Moreda-Piñeiro, J. A. Cocho, M. L. Couce, A. Moreda-Piñeiro and P. Bermejo-Barrera, Trace elements in dried blood spots as potential discriminating features for metabolic disorder diagnosis in newborns, Metallomics, 2021, 13, mfab018 CrossRef PubMed.
- S. Kröger, M. Sperling and U. Karst, Quantitative dried blood spot analysis for metallodrugs by laser ablation-inductively coupled plasma-mass spectrometry, J. Trace Elem. Med. Biol., 2019, 51, 50–56 CrossRef PubMed.
- M. Aramendia, L. Rello, F. Vanhaecke and M. Resano, Direct trace-elemental analysis of urine samples by laser ablation-inductively coupled plasma mass spectrometry after sample deposition on clinical filter papers, Anal. Chem., 2012, 84, 8682–8690 CrossRef CAS PubMed.
- M. Resano, M. Aramendia, L. Rello, M. L. Calvo, S. Bérail and C. Pécheyran, Direct determination of Cu isotope ratios in dried urine spots by means of fs-LA-MC-ICPMS. Potential to diagnose Wilson's disease, J. Anal. At. Spectrom., 2013, 28, 98–106 RSC.
- P. Foltynova, V. Kanicky and J. Preisler, Diode Laser Thermal Vaporization Inductively Coupled Plasma Mass Spectrometry, Anal. Chem., 2012, 84, 2268–2274 CrossRef CAS PubMed.
- M. Aranaz, M. Costas-Rodriguez, L. Lobo, H. Gonzalez-Iglesias, F. Vanhaecke and R. Pereiro, Pilot study of homeostatic alterations of mineral elements in serum of patients with age-related macular degeneration via elemental and isotopic analysis using ICP-mass spectrometry, J. Pharm. Biomed. Anal., 2020, 177, 112857 CrossRef CAS.
- T. N. Abduljabbar, B. L. Sharp, H. J. Reid, N. Barzegar-Befroeid, T. Petod and I. Lengyel, Determination of Zn, Cu and Fe in human patients' serum using microsampling ICP-MS and sample dilution, Talanta, 2019, 204, 663–669 CrossRef CAS PubMed.
- S. C. Wilschefski and M. R. Baxter, Inductively coupled plasma mass spectrometry: Introduction to analytical aspects, Clin. Biochem. Rev., 2019, 40, 115–133 Search PubMed.
- J. L. M. de Boer, R. Ritsema, S. Piso, H. van Staden and W. van den Beld, Practical and quality-control aspects of multi-element analysis with quadrupole ICP–MS with special attention to urine and whole blood, Anal. Bioanal. Chem., 2004, 379, 872–880 CrossRef CAS PubMed.
- R. S. Amais, E. E. Garcia, M. R. Monteiro, A. R. A. Nogueira and J. A. Nóbrega, Direct analysis of biodiesel microemulsions using an inductively coupled plasma mass spectrometry, Microchem. J., 2010, 96, 146–150 CrossRef CAS.
- M. Aramendıa, L. Rello, S. Berail, A. Donnard, C. Pecheyrand and M. Resano, Direct
analysis of dried blood spots by femtosecond-laser ablation-inductively coupled plasma-mass spectrometry. Feasibility of split-flow laser ablation for simultaneous trace element and isotopic analysis, J. Anal. At. Spectrom., 2015, 30, 296–309 RSC.
- M. G. M. Kok and M. Fillet, Volumetric absorptive microsampling: current advances and applications, J. Pharm. Biomed. Anal., 2018, 147, 288–296 CrossRef CAS.
- M. Protti, R. Mandrioli and L. Mercolini, Tutorial: Volumetric absorptive microsampling (VAMS), Anal. Chim. Acta, 2019, 1046, 32–47 CrossRef CAS PubMed.
- E. Bolea-Fernandez, K. Phan, L. Balcaen, M. Resano and F. Vanhaecke, Determination of ultra-trace amounts of prosthesis-related metals in whole blood using volumetric absorptive micro-sampling and tandem ICP – Mass spectrometry, Anal. Chim. Acta, 2016, 941, 1–9 CrossRef CAS PubMed.
- S. Capiaua, E. Bolea-Fernandez, L. Balcaen, C. Van Der Straeten, A. G. Verstraete, F. Vanhaecke and C. P. Stove, Development, validation and application of an inductively coupled plasma – mass spectrometry method to determine cobalt in metal-on-metal prosthesis patients using volumetric absorptive microsampling, Talanta, 2020, 208, 120055 CrossRef.
- Y. Anoshkina, M. Costas-Rodrıguez and F. Vanhaecke, Iron isotopic analysis of finger-prick and venous blood by multi-collector inductively coupled plasma-mass spectrometry after volumetric absorptive microsampling, J. Anal. At. Spectrom., 2017, 32, 314–321 RSC.
- L. Fu, H. Xie, J. Huang and L. Chen, Rapid determination of trace elements in serum of hepatocellular carcinoma patients by inductively coupled plasma tandem mass spectrometry, Anal. Chim. Acta, 2020, 1112, 1–7 CrossRef CAS.
- C. Liang, Z. Li, X. Xia, Q. Wang, R. Tao, Y. Tao, H. Xiang, S. Tong and F. Tao, Determine multiple elements simultaneously in the sera of umbilical cord blood samples—A very simple method, Biol. Trace Elem. Res., 2017, 177, 1–8 CrossRef CAS PubMed.
- B. Toubhans, A. T. Gourlan, P. Telouk, K. Kutchman-Singh, L. W. Francis, R. S. Conlan, L. Margarit, D. Gonzalez and L. Charlet, Cu isotope ratios are meaningful in ovarian cancer diagnosis, J. Trace Elem. Med. Biol., 2020, 62, 126611 CrossRef CAS PubMed.
- M. Costas-Rodriguez, S. V. Campenhout, A. A. M. B. Hastuti, L. Devisscher, H. V. Vlierberghe and F. Vanhaecke, Body distribution of stable copper isotopes during the progression of cholestatic liver disease induced by common bile duct ligation in mice, Metallomics, 2019, 11, 1093–1103 CrossRef CAS PubMed.
- S. Lauwens, M. Costas-Rodriguez, H. V. Vlierberghe and F. Vanhaecke, Cu isotopic signature in blood serum of liver transplant patients: a follow-up study, Sci. Rep., 2016, 6, 20683 CrossRef PubMed.
- S. Lauwens, M. Costas-Rodriguez, J. Delanghe, H. V. Vlierberghe and F. Vanhaecke, Quantification and isotopic analysis of bulk and of exchangeable and ultrafiltrable serum copper in healthy and alcoholic cirrhosis subjects, Talanta, 2018, 189, 332–338 CrossRef CAS PubMed.
- M. Costas-Rodriguez, Y. Anoshkina, S. Lauwens, H. V. Vlierberghe, J. Delanghe and F. Vanhaecke, Isotopic analysis of Cu in blood serum by multi-collector ICP-mass spectrometry: a new approach for the diagnosis and prognosis of liver cirrhosis, Metallomics, 2015, 7, 491–498 CrossRef CAS.
- P. Télouk, A. Puisieux, T. Fujii, V. Balter, V. Bondanese, A. P. Morel, G. Clapisson, A. Lamboux and F. Albarede, Copper isotope effect in serum of cancer patients. A pilot study, Metallomics, 2014, 7, 299–308 CrossRef.
- F. Larner, B. Sampson, M. RehkÄmper, D. J. Weiss, J. R. Dainty, S. O'Riordan, T. Panetta and P. G. Bain, High precision isotope measurements reveal poor control of copper metabolism in Parkinsonism, Metallomics, 2013, 5, 125–132 CrossRef CAS PubMed.
- M. Aramendía, L. Rello, M. Resano and F. Vanhaecke, Isotopic analysis of Cu in serum samples for diagnosis of Wilson's disease: a pilot study, J. Anal. At. Spectrom., 2013, 28, 675–681 RSC.
- S. V. Campenhout, A. A. M. B. Hastuti, S. Lefere, H. V. Vlierberghe, F. Vanhaecke, M. Costas-Rodriguez and L. Devisscher, Lighter serum copper isotopic composition in patients with early non-alcoholic fatty liver disease, BMC Res. Notes, 2020, 13, 225 CrossRef PubMed.
- J. C. Cikomola, M. R. Flórez, M. Costas-Rodriguez, Y. Anoshkina, K. Vandepoele, P. B. Katchunga, A. S. Kishabongo, M. M. Speeckaert, F. Vanhaecke and J. Delanghe, Whole blood Fe isotopic signature in a sub-Saharan African population, Metallomics, 2017, 9, 1142–1149 CrossRef CAS.
- Y. Anoshkina, M. Costas-Rodriguez, M. Speeckaert, W. V. Biesen, J. Delanghe and F. Vanhaecke, Iron isotopic composition of blood serum in anemia of chronic kidney disease, Metallomics, 2017, 9, 517–524 CrossRef CAS PubMed.
- L. V. Heghe, J. Delanghe, H. V. Vlierberghe and F. Vanhaecke, The relationship between the iron isotopic composition of human whole blood and iron status parameters, Metallomics, 2013, 5, 1503 CrossRef PubMed.
- P. A. Krayenbuehl, T. Walczyk, R. Schoenberg, F. V. Blanckenburg and G. Schulthess, Hereditary hemochromatosis is reflected in the iron isotope composition of blood, Blood, 2005, 105, 3812–3816 CrossRef CAS PubMed.
- A. A. M. B. Hastuti, M. Costas-Rodriguez, Y. Anoshkina, T. Parnall, J. A. Madura and F. Vanhaecke, High-precision isotopic analysis of serum and whole blood Cu, Fe and Zn to assess possible homeostasis alterations due to bariatric surgery, Anal. Bioanal. Chem., 2020, 412, 727–738 CrossRef CAS PubMed.
- A. A. M. B. Hastuti, M. Costas-Rodriguez, A. Matsunaga, T. Ichinose, S. Hagiwara, M. Shimura and F. Vanhaecke, Cu and Zn isotope ratio variations in plasma for survival prediction in hematological malignancy cases, Sci. Rep., 2020, 10, 16389 CrossRef PubMed.
- R. Grigoryan, M. Costas-Rodriguez, S. V. Laecke, M. Speeckaert, B. Lapauw and F. Vanhaecke, Multi-collector ICP-mass spectrometry reveals changes in the serum Mg isotopic composition in diabetes type I patients, J. Anal. At. Spectrom., 2019, 34, 1514–1521 RSC.
- E. Albalat, P. Telouk, V. Balter, T. Fujii, V. P. Bondanese, M.-L. Plissonnier, V. Vlaeminck-Guillem, J. Baccheta, N. Thiam, P. Miossec, F. Zoulim, A. Puisieux and F. Albarède, Sulfur isotope analysis by MC-ICP-MS and application to small medical samples, J. Anal. At. Spectrom., 2016, 31, 1002–1011 RSC.
- V. Balter, A. Nogueira da Costa, V. P. Bondanese, K. Jaouen, A. Lamboux, S. Sangrajrang, N. Vincent, F. Fourel, P. Télouk, M. Gigou, C. Lécuyer, P. Srivatanakul, C. Bréchot, F. Albarède and P. Hainaut, Natural variations of copper and sulfur stable isotopes in blood of hepatocellular carcinoma patients, Proc. Natl. Acad. Sci., 2015, 112, 982–985 CrossRef CAS PubMed.
- A. Eisenhauer, M. Müller, A. Heuser, A. Kolevica, C. C. Glüer, M. Both, C. Laue, U. V. Hehn, S. Kloth, R. Shroff and J. Schrezenmeir, Calcium isotope ratios in blood and urine: a new biomarker for the diagnosis of osteoporosis, BoneKEy Rep., 2019, 10, 100200 CrossRef CAS PubMed.
- L. Gerhardsson, T. Lundh, L. Minthon and E. Londos, Metal concentrations in plasma and cerebrospinal fluid in patients with Alzheimer's disease, Dementia Geriatr. Cognit. Disord., 2008, 25, 508–515 CrossRef CAS.
- B. Michalke and V. Nischwitz, Review on metal speciation analysis in cerebrospinal fluid—current methods and results: A review, Anal. Chim. Acta, 2010, 682, 23–36 CrossRef CAS.
- F. Maass, B. Michalke, D. Willkommen, C. Schulte, L. Tönges, M. Boerger, I. Zerra, M. Bähr and P. Lingor, Selenium speciation analysis in the cerebrospinal fluid of patients with Parkinson's disease, J. Trace Elem. Med. Biol., 2020, 57, 110–115 CrossRef.
- M. Korvela, A.-L. Lind, M. Wetterhall, T. Gordh, M. Andersson and J. Pettersson, Quantification of 10 elements in human cerebrospinal fluid from chronic pain patients with and without spinal cord stimulation, J. Trace Elem. Med. Biol., 2016, 37, 1–7 CrossRef CAS PubMed.
- J. Mandrioli, B. Michalke, N. Solovyev, P. Grill, F. Violi, C. Lunetta, A. Conte, V. A. Sansone, M. Sabatelli and M. Vinceti, Elevated levels of selenium species in cerebrospinal fluid of amyotrophic lateral sclerosis patients with disease-associated gene mutations, Neurodegener. Dis., 2017, 17, 171–180 CrossRef CAS PubMed.
- M. Vinceti, T. Filippini, J. Mandrioli, F. Violi, A. Bargellini, J. Weuve, N. Fini, P. Grill and B. Michalke, Lead, cadmium and mercury in cerebrospinal fluid and risk of amyotrophic lateral sclerosis: A case-control study, J. Trace Elem. Med. Biol., 2017, 43, 121–125 CrossRef CAS PubMed.
- M. Vinceti, N. Solovyev, J. Mandrioli, C. M. Crespi, F. Bonvicini, E. Arcolin, E. Georgoulopoulou and B. Michalke, Cerebrospinal fluid of newly diagnosed amyotrophic lateral sclerosis patients exhibits abnormal levels of selenium species including elevated selenite, Neurotoxicology, 2013, 38, 25–32 CrossRef CAS PubMed.
- B. R. Cardoso, D. J. Hare, A. I. Bush, Q.-X. Li, C. J. Fowler, C. L. Masters, R.-N. Martins, K. Ganio, A. Lothian, S. Mukherjee, E. A. Kapp and B. R. Roberts, The AIBL research group, Selenium levels in serum, red blood cells, and cerebrospinal fluid of Alzheimer's disease patients: A report from the Australian Imaging, Biomarker & Lifestyle Flagship study of ageing (AIBL), J. Alzheimer's Dis., 2017, 57, 183–193 CAS.
- B. R. Roberts, J. D. Doecke, A. Rembach, L. F. Yévenes, C. J. Fowler, C. A. McLean, M. Lind, I. Volitakis, C. L. Masters, A. I. Bush and D. J. Hare, Rubidium and potassium levels are altered in Alzheimer's disease brain and blood but not in cerebrospinal fluid, Acta Neuropathol. Commun., 2016, 4, 119 CrossRef PubMed.
- Y. H. Hung, N. G. Faux, D. W. Killile, N. Yanjanin, S. Firnkes, I. Volitakis, G. Ganio, M. Walterfang, C. Hastings, F. D. Porter, D. S. Ory and A. I. Bush, Altered transition metal homeostasis in Niemann–Pick disease, type C1, Metallomics, 2014, 6, 542–553 CrossRef CAS PubMed.
- A. Cañabate, E. García-Ruiz, M. Resano and J.-L. Todolí, Cerebrospinal fluid elemental analysis by using a total sample consumption system operated at high temperature adapted to inductively coupled plasma mass spectrometry, J. Anal. At. Spectrom., 2017, 32, 1916–1924 RSC.
- A. Stojsavljević, L. Vujotić, B. Rovčanin, S. Borković-Mitić, M. Gavrović-Jankulović and D. Manojlović, Assessment of trace metal alterations in the blood, cerebrospinal fluid and tissue samples of patients with malignant brain tumors, Sci. Rep., 2020, 10, 3816 CrossRef PubMed.
- L. Sauzéat, E. Bernard, A. Perret-Liaudet, I. Quadrio, A. Vighetto, P. Krolak-Salmon, E. Broussolle, P. Leblanc and V. Balter, Isotopic evidence for disrupted copper metabolism in amyotrophic lateral sclerosis, iScience, 2018, 6, 264–271 CrossRef PubMed.
- M. Aranaz, M. Costas-Rodriguez, L. Lobo, M. García, H. González-Iglesias, R. Pereiro and F. Vanhaecke, Homeostatic alterations related to total antioxidant capacity, elemental concentrations and isotopic compositions in aqueous humour of glaucoma patients, Anal. Bioanal. Chem., 2021 DOI:10.1007/s00216-021-03467-5.
- M. Tamhane, S. Cabrera-Ghayouri, G. Abeliana and V. Viswanath, Review of biomarkers in ocular matrices: Challenges and opportunities, Pharm. Res., 2019, 36, 40 CrossRef PubMed.
- B. Bocca, G. Forte, A. Pisano, C. Farace, E. Giancipoli, A. Pinna, S. Dore and R. Madeddu, A pilot study to evaluate the levels of aqueous humour trace elements in open-angle glaucoma, J. Trace Elem. Med. Biol., 2020, 61, 126560 CrossRef CAS PubMed.
- B. Hohberger, M. A. Chaudhri, B. Michalke, M. Lucio, K. Nowomiejska, U. Schlötzer-Schrehardt, P. Grieb, R. Rejdak and A. G. M. Jünemann, Levels of aqueous humour trace elements in patients with open-angle glaucoma, J. Trace Elem. Med. Biol., 2018, 45, 150–155 CrossRef CAS PubMed.
- A. G. M. Junemann, P. Stopa, B. Michalke, A. Chaudhri, U. Reulbach, C. Huchzermeyer, U. Schlotzer-Schrehardt, F. E. Kruse, E. Zrenner and R. Rejdak, Levels of aqueous humour trace elements in patients with non-exudative age-related macular degeneration: A case-control study, PLoS One, 2013, 8, e56734 CrossRef PubMed.
- Y. Zhou, F. Hong, X. Wang, J. Feng, D. Zhu, Y. Xu and Y. Tao, Abnormal levels of aqueous humour trace elements in patients with cytomegalovirus retinitis, Eye, 2019, 33, 1606–1612 CrossRef CAS PubMed.
- K. Yavuzer and Y. Akinay, Microstructure and chemical analysis in pseudoexfoliation syndrome, Curr. Eye Res., 2021, 46, 490–495 CrossRef CAS PubMed.
- M. Vázquez, I. Ibarra, A. Sibón and M. D. Pérez-Cárceles, Multi-element Analysis of Vitreous Humour Samples by ICP-MS and ICP-OES, At. Spectrosc., 2017, 38, 124–132 CrossRef.
- J. C. S. Junior, P. C. M. Filho, R. B. F. Guidugli, M. N. Eberlin, G. de Souza Pesso, E. Gomes da Silva, M. A. Z. Arruda and N. F. Hoehr, Metals and (metallo)proteins identification in vitreous humour focusing on post-mortem biochemistry, Metallomics, 2014, 6, 1801–1807 CrossRef PubMed.
- R. González de Vega, M. L. Fernández-Sánchez, H. G. Iglesias, M. C. Prados and A. Sanz-Medel, Quantitative selenium speciation by HPLC-ICP-MS(IDA) and simultaneous activity measurements in human vitreous humour, Anal. Bioanal. Chem., 2016, 407, 2405–2413 CrossRef PubMed.
- A. Wójtowicz, R. Wietecha-Posłuszny and M. Snamina, Contemporary trends in drug analysis of vitreous humour: a critical review, TrAC, Trends Anal. Chem., 2020, 129, 115935 CrossRef.
- A. L. Galusha, A. C. Haig, M. S. Bloom, P. C. Kruger, A. McGough, N. Lenhart, R. Wong, V. Y. Fujimoto, E. Mok-Lind and P. J. Parsons, Ultra-trace element analysis of human follicular fluid by ICP-MS/MS: pre-analytical challenges, contamination control, and matrix effects, J. Anal. At. Spectrom., 2019, 34, 741–752 RSC.
- B. Markiewicz, A. Sajnóg, W. Lorenc, A. Hanć, I. Komorowic, J. Suliburska, R. Kocyłowski and D. Barałkiewicz, Multielemental analysis of 18 essential and toxic elements in amniotic fluid samples by ICP-MS: Full procedure validation and estimation of measurement uncertainty, Talanta, 2017, 174, 122–130 CrossRef CAS PubMed.
- A. Sajnóg, M. Tkaczyk, M. Stanczyk, K. Szaflik, J. Suliburska, R. Kocyłowski and D. Barałkiewicz, A new procedure for the determination of 21 macro- and trace elements in human fetal urine using an inductively coupled plasma mass spectrometry with dynamic reaction cell (ICP-DRC-MS) equipped with a micro-flow nebulizer, Talanta, 2021, 222, 121672 CrossRef PubMed.
- L. Lemoine, E. Thijssen, J.-P. Noben, P. Adriaensens, R. Carleer and K. Van der Speeten, A validated inductively coupled plasma mass spectrometry (ICP-MS) method for the quantification of total platinum content in plasma, plasma ultrafiltrate, urine and peritoneal fluid, J. Pharm. Biomed. Anal., 2018, 152, 39–46 CrossRef CAS PubMed.
- F. Moyano, E. Verni, H. Tamashiro, S. Digenaro, L. D. Martinez and R. A. Gil, Single-step procedure for trace element determination in synovial fluid by dynamic reaction cell-inductively coupled plasma mass spectrometry, Microchem. J., 2014, 112, 17–24 CrossRef CAS.
- B. D. Kerger, R. Gerads, H. Gurleyuk, A. Urban and D. J. Paustenbach, Total cobalt determination in human blood and synovial fluid using inductively coupled plasma mass spectrometry: method validation and evaluation of performance variables affecting metal hip implant patient samples, Toxicol. Environ. Chem., 2015, 97, 1145–1163 CrossRef CAS.
- P. Li, Y. Zhong, X. Jiang, C. Wang, Z. Zuo and A. Sha, Seminal plasma metals concentration with respect to semen quality, Biol. Trace Elem. Res., 2012, 148, 1–6 CrossRef CAS PubMed.
- M. Katayama, S. Kaneko, K. Takamatsu, T. Tsukimura and T. Togawa, Determination of trace metals in human seminal plasma using inductively coupled plasma mass spectrometry and multivariate statistical analyses for sperm parameters, J. Mol. Biomarkers Diagn., 2013, 4, 1000147 Search PubMed.
- T. Zivkovic, B. Tariba and A. Pizent, Multielement analysis of human seminal plasma by octopole reaction cell ICP-MS, J. Anal. At. Spectrom., 2014, 29, 2114–2126 RSC.
- S. S. Tan, G. L. Khor, E. Stoutjesdijk, K. W. T. Ng, I. Khouw, M. Bragt, A. Schaafsmac, D. A. J. Dijck-Brouwer and F. A. J. Muskiet, Case study of temporal changes in maternal dietary intake and the association with breast milk mineral contents, J. Food Compos. Anal., 2020, 89, 103468 CrossRef CAS.
- M. Levi, C. Hjelm, F. Harari and M. Vahter, ICP-MS measurement of toxic and essential elements in human breast milk. A comparison of alkali dilution and acid digestion sample preparation methods, Clin. Biochem., 2018, 53, 81–87 CrossRef CAS PubMed.
- M. L. Astolfi, D. Marotta, V. Cammalleri, E. Marconi, A. Antonucci, P. Avino, S. Canepari, M. Vitali and C. Protano, Determination of 40 elements in powdered infant formulas and related risk assessment, Int. J. Environ. Res. Public Health, 2021, 18, 5073 CrossRef CAS PubMed.
- S. Subashchandrabose, E. R. Pereira-Filho and G. L. Donati, Trace element analysis of urine by ICP-MS/MS to identify urinary tract infection, J. Anal. At. Spectrom., 2017, 32, 1590–1594 RSC.
- A. Sarmiento-González, J. M. Marchante-Gayón, J. M. Tejerina-Lobo, J. Paz-Jiménez and A. Sanz-Medel, High-resolution ICP–MS determination of Ti, V, Cr, Co, Ni, and Mo in human blood and urine of patients implanted with a hip or knee prosthesis, Anal. Bioanal. Chem., 2008, 391, 2583–2589 CrossRef PubMed.
- M. G. Minnich, D. C. Miller and P. J. Parsons, Determination of As, Cd, Pb, and Hg in urine using inductively coupled plasma mass spectrometry with the direct injection high efficiency nebulizer, Spectrochim. Acta, Part B, 2008, 63, 389–395 CrossRef.
- D. R. Jones, J. M. Jarrett, D. Stukes, A. Baer, M. McMichael, K. Wallon, G. Xiao and R. L. Jones, Development and validation of a biomonitoring method to measure As, Cr, and Ni in human urine samples by ICP-UCT-MS, Int. J. Hyg. Environ. Health, 2021, 234, 113713 CrossRef CAS PubMed.
- E. Robotti, F. Quasso, M. Manfredi, F. Gosetti, E. Mazzucco, C. Isidoro and E. Marengo, Determination by ICP-MS and multivariate data analysis of elemental urine excretion profile during the EDTA chelation therapy: A case study, J. Trace Elem. Med. Biol., 2020, 62, 126608 CrossRef CAS PubMed.
- M. V. Zoriy, C. Pickhardt, P. Ostapczuk, R. Hille and J. S. Becker, Determination of Pu in urine at ultratrace level by sector field inductively coupled plasma mass spectrometry, Int. J. Mater. Sci., 2004, 232, 217–224 CAS.
- S. Salou, D. Larivière, C.-M. Cirtiu and N. Fleury, Quantification of titanium dioxide nanoparticles in human urine by single-particle ICP-MS, Anal. Bioanal. Chem., 2021, 413, 171–181 CrossRef CAS PubMed.
- K. Badalova, P. Herbello-Hermelo, P. Bermejo-Barrera and A. Moreda-Piñeiro, Possibilities of single particle—ICP-MS for determining/characterizing titanium dioxide and silver nanoparticles in human urine, J. Trace Elem. Med. Biol., 2019, 54, 55–61 CrossRef CAS PubMed.
- S. Fernández-Trujillo, M. Jiménez-Moreno, A. Ríos and R. C. R. Martín-Doimeadios, A simple analytical methodology for platinum nanoparticles control in complex clinical matrices via SP-ICP-MS, Talanta, 2021, 231, 122370 CrossRef PubMed.
- F. Romano, A. Castiblanco, F. Spadotto, F. Di Scipio, M. Malandrino, G. N. Berta and M. Aimetti, ICP-mass-spectrometry ionic profile of whole saliva in patients with untreated and treated periodontitis, Biomedicines, 2020, 8, 354 CrossRef CAS PubMed.
- E. Inonu, S. S. Hakki, S. A. Kayis and F. H. Nielsen, The Association Between Some Macro and Trace Elements in Saliva and Periodontal Status, Biol. Trace Elem. Res., 2020, 197, 35–42 CrossRef CAS PubMed.
- M. Watanabe, M. Asatsuma, A. Ikui, M. Ikeda, Y. Yamada, S. Nomura and A. Igarashi, Measurements of several metallic elements and matrix metalloproteinases (MMPs) in saliva from patients with taste disorder, Chem. Senses, 2005, 30, 121–125 CrossRef CAS PubMed.
- H. Reis de Souza Schacher and L. Macedo de Menezes, Metal ion quantification in the saliva of patients with lingual arch appliances using silver solder, laser, or TIG welding, Clinical Oral Investigations, 2020, 24, 2109–2120 CrossRef PubMed.
- Y.-J. Kim, Y.-K. Kim and H.-S. Kho, Effects of smoking on trace metal levels in saliva, Oral Dis., 2010, 16, 823–830 CrossRef PubMed.
- L. M. Martínez, D. M. Pagán and P. L. Jornet, Trace elements in saliva as markers of type 2 diabetes mellitus, Biol. Trace Elem. Res., 2018, 186, 354–360 CrossRef PubMed.
- J. F. Staff, A.-H. Harding, J. Morton, K. Jones, E. Guice and T. McCormick, Investigation of saliva as an alternative matrix to blood for the biological monitoring of inorganic lead, Toxicol. Lett., 2014, 231, 270–276 CrossRef CAS PubMed.
- E. P. Duktiewicz and P. L. Urban, Quantitative mass spectrometry of unconventional human biological matrices, Philos. Trans. R. Soc., A, 2016, 374, 20150380 CrossRef PubMed.
- W. Ngo, J. Chen, S. Panthi, K. K. Nichols and J. J. Nichols, Comparison of collection methods for the measure of human meibum and tear film-derived lipids using mass spectrometry, Curr. Eye Res., 2018, 43, 1244–1252 CrossRef CAS PubMed.
- E. Ponzini, C. Santambrogio, A. De Palma, P. Mauri, S. Tavazzi and R. Grandori, Mass spectrometry-based tear proteomics for non-invasive biomarker discovery, Mass Spectrom. Rev., 2021, 1–19 Search PubMed.
- I. Cicalini, C. Rossi, D. Pieragostino, L. Agnifili, L. Mastropasqua, M. di Ioia, G. De Luca, M. Onofrj, L. Federici and P. Del Boccio, Integrated lipidomics and metabolomics analysis of tears in multiple sclerosis: An insight into diagnostic potential of lacrimal fluid, Int. J. Mol. Sci., 2019, 20, 1265 CrossRef CAS PubMed.
- A. Cancarini, J. Fostinelli, L. Napoli, M. E. Gilberti, P. Apostoli and F. Semeraro, Trace elements diabetes: Assessment of levels in tears and serum, Exp. Eye Res., 2017, 154, 47–52 CrossRef CAS PubMed.
- F. Semeraro, C. Costagliola, A. Cancarini, E. Gilberti, E. Tosco and P. Apostoli, Defining reference values of trace elements in the tear film: Diagnostic methods and possible applications, Ecotoxicol. Environ. Saf., 2012, 80, 190–194 CrossRef CAS PubMed.
- A. Louveau, I. Smirnov, T. J. Keyes, J. D. Eccles, S. J. Rouhani, J. D. Peske, N. C. Derecki, D. Castle, J. W. Mandell, K. S. Lee, T. H. Harris and J. Kipnis, Structural and functional features of central nervous system lymphatic vessels, Nature, 2015, 523, 337–341 CrossRef CAS PubMed.
- C. García-Cabo, P. Llano-Suárez, L. Benavente-Fernández, S. Calleja-Puerta, M. T. Fernández-Abedul and J. M. Costa-Fernández, Obtaining information from the brain in a non-invasive way: determination of iron in nasal exudate to differentiate hemorrhagic and ischemic strokes, Clin. Chem. Lab. Med., 2020, 58, 847–853 Search PubMed.
- C. García-Cabo, P. Llano-Suárez, L. B. Fernández, J. M. Costa-Fernández, M. T. Fernández-Abedul and S. Calleja-Puerta, Iron measured in nasal exudate samples as a new and useful biomarker in the differential diagnosis of patients with acute stroke, Cerebrovasc. Dis., 2020, 49, 625–631 CrossRef PubMed.
- M. Sampedro, M. Aliste, S. Arranz, S. Alicia, N. Unceta, G. Alberto, A. Vallejo, M. Aliste, S. Arranz, A. Sánchez-Ortega, M. C. Sampedro, N. Unceta, A. Gómez-Caballero, A. Vallejo, M. A. Goicolea and R. J. Barrio, Particle analysis for the detection of gunshot residue (GSR) in nasal samples using scanning laser ablation and inductively coupled plasma-mass spectrometry, J. Forensic Sci., 2020, 65, 1094–1101 CrossRef.
- J. N. Hussain, N. Mantri and M. M. Cohen, Working Up a Good Sweat – The challenges of standardising sweat collection for metabolomics analysis, Clin. Biochem. Rev., 2017, 38, 13–34 Search PubMed.
- S. J. Genuis, D. Birkholz, I. Rodushkin and S. Beesoon, Blood, urine, and sweat (BUS) study: Monitoring and elimination of bioaccumulated toxic elements, Arch. Environ. Contam. Toxicol., 2011, 61, 344–357 CrossRef CAS PubMed.
- J. Siquier-Coll, I. Bartolomé, M. Pérez-Quintero, D. Muñoz, M. C. Robles and M. Maynar-Mariño, Effect of exposure to high temperatures in the excretion of cadmium and lead, J. Therm. Biol., 2020, 89, 102545 CrossRef CAS PubMed.
- T. Saran, M. Zawadka, S. Chmiel and A. Mazur, Sweat iron concentration during 4-week exercise training, AAEM, 2018, 25, 500–503 CAS.
- J. Siquier-Coll, I. Bartolomé, M. Pérez-Quintero, F. J. Grijota, D. Muñoz and M. Maynar-Mariño, Effect of heat exposure and physical exercise until exhaustion in normothermic and hyperthermic conditions on serum, sweat and urinary concentrations of magnesium and phosphorus, J. Therm. Biol., 2019, 84, 176–184 CrossRef CAS PubMed.
- J. T. Collie, R. J. Massie, O. A. H. Jones, P. D. Morrison and R. F. Greaves, A candidate reference method using ICP-MS for sweat chloride quantification, Clin. Chem. Lab. Med., 2016, 54, 561–567 CAS.
- A. Marvelli, B. Campi, G. Mergni, M. E. Di Cicco, P. Turini, P. Scardina, R. Zucchi, M. Pifferi, G. Taccetti, A. Paolicchi, G. la Marca and A. Saba, Sweat chloride assay by inductively coupled plasma mass spectrometry: a confirmation test for cystic fibrosis diagnosis, Anal. Bioanal. Chem., 2020, 412, 6909–6916 CrossRef CAS PubMed.
- E. Mavrakis and S. A. Pergantis, Chip-based microfluidics on-line with inductively coupled plasma - mass spectrometry for standard dilution analysis, Anal. Chim. Acta, 2021, 1179, 338830 CrossRef CAS PubMed.
Footnote |
† Both authors contributed equally to this work. |
|
This journal is © The Royal Society of Chemistry 2022 |