DOI:
10.1039/C8SC02981D
(Edge Article)
Chem. Sci., 2018,
9, 8178-8183
Al(OCArF3)3 – a thermally stable Lewis superacid†‡
Received
6th July 2018
, Accepted 25th September 2018
First published on 2nd October 2018
Abstract
The adduct free Lewis superacid Al(OCArF3)3 was obtained by the reaction of ArF3COH with AlEt3 and fully characterized (ArF = C6F5). It comprises a high thermal stability up to 180 °C and a distinct reactivity towards Lewis bases, as exemplified by the isolation of the neutral adducts Al(OCArF3)·D (D = MeCN, THF, Et2O, pyridine, OPEt3), the fluoride complexes [Q][FAl(OCArF)3] (Q+ = Cs+, Ag+, Tl+, [S(NMe2)3]+, [Ph3C]+, Li+, [NBu4]+, [FeCp2]+) and the chloride complex [Ph3C][ClAl(OCArF)3].
Introduction
Lewis acids play a key role in many branches of chemistry. For instance, they are used as catalysts,1 as components in frustrated Lewis pairs (FLPs)2 and for the stabilization of unusual cations via formation of related weakly coordinating anions (WCAs).3 The strength of Lewis acids is usually estimated by the calculation of the fluoride ion affinity (FIA).4–8 The widely used B(C6F5)3 (BCF) exhibits a FIA of 452 kJ mol−1.8 Lewis superacids were defined as compounds exceeding the FIA of molecular SbF5 (501 kJ mol−1).9 Readily available aluminum halides, such as AlCl3 and AlBr3, are Lewis superacids in the gas phase, but exhibit a dramatically lower acidity in condensed phase due to dimerization. The key player in Lewis superacid chemistry is unarguably Krossing's Al(OC(CF3)3)3 (FIA = 547 kJ mol−1),9 which, however, has never been isolated.8 Due to its limited thermal stability it is usually prepared in situ and used as fluorobenzene adduct with a lower FIA (465 kJ mol−1).9 Another drawback is the tendency of the corresponding WCAs, [Al(OC(CF3)3)4]− or [FAl(OC(CF3)3)3]−, to suffer from severely disordered crystal structures.10 The vital interest in new Lewis superacids with more refined properties is highlighted by five recent publications: Mitzel et al. improved the Lewis acidity of BCF by substitution of its para-positions by CF3 groups resulting in the strongest single-site triorganoborane Lewis acid B(C6F4CF3)3.11 Riedel et al. reported on the isolation of the temperature sensitive superacid Al(OTeF5)3 and its acetonitrile adduct together with a series of salts containing the new WCA [Al(OTeF5)4]−.12 Sundermeyer and Krossing et al. described the extremely sensitive Lewis superacid Al(N(C6F5)2)3 with a high FIA of 555 kJ mol−1.13 Only recently the group of Greb published a neutral silicon Lewis superacid14 and a review article on Lewis superacidity.15
Results and discussion
The free Lewis acid Al(OCArF3)3
We identified the perfluorinated alcohol ArF3COH as a promising ligand for the preparation of new Lewis superacids (ArF = C6F5).16
Indeed, the reaction of triethylaluminum with three equivalents of ArF3COH provided the adduct free Al(OCArF3)3 (1), which crystallized directly from the reaction mixture in 66% yield (Scheme 1). Unlike Al(OC(CF3)3)3, 1 can be stored indefinitely under argon at room temperature. In fact, the thermal decomposition of 1 according to a DTA analysis occurs only above 180 °C. The molecular structure of Al(OCArF3)3 (1) reveals that the spatial arrangement of the Al atoms is distorted trigonal bipyramidal and defined by a O3 + F2 donor set (Fig. 1): the three equatorial Al–O bonds (1.681(1)–1.701(1) Å) are significantly shorter than the two axial Al⋯F contacts (2.083(1) and 2.153(1) Å). These contacts, arising from intramolecular coordination of F atoms situated in the ortho-positions of two perfluorophenyl groups, are probably the reason for the increased thermal stability. A similar κ-O3F2 configuration was found in the molecular structure of Al(OC(C5F10)C6F5)3 (Al⋯F: 2.085(2) and 2.113(2) Å)7 and Al(N(C6F5)2)3 (Al⋯F: 2.060(1) and 2.084(1) Å)13 and calculated for adduct free Al(OC(CF3)3)3 (Al⋯F: 2.143 and 2.155 Å).6
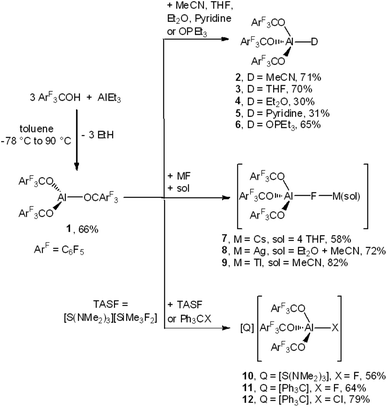 |
| Scheme 1 Synthesis of Al(OCArF3)3 (1) and reactivity towards Lewis bases. | |
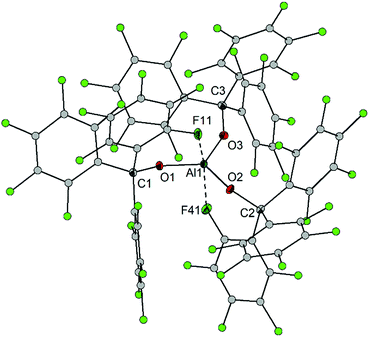 |
| Fig. 1 Molecular structure of Al(OCArF3)3 (1). Ellipsoids are set to 50% probability. Balls are drawn with an arbitrarily fixed radius. | |
Theoretical section
The computed gas phase structure of 1 closely resembles the solid state structure, however, the computed bond distances (Al–O: 1.698, 1.711 and 1.719 Å; Al⋯F: 2.131 and 2.173 Å) are slightly longer. In order to evaluate the Lewis acidity, the FIA of 1 and related Lewis acids have been evaluated using ab initio MP2/6-311++G(2d,2p) single point energies at B3LYP/6-311++G(2d,2p) optimized geometries (Fig. 2). In addition to FIA computed using the reaction via direct F− addition to LA, FIA values were also computed using the isodesmic reaction LA + COF3− = LAF− + COF2 and the experimental gas phase FIA value of COF2 (209 kJ mol−1).5 For example, this approach was recently used by Stephan and coworkers.17 The differences between FIA values obtained from F− addition reactions and from the isodesmic reactions are less than 4 kJ mol−1 (Table S3‡), indicating that the chosen approach allows to compute FIA via direct attachment of an F− ion. The FIA values for the reference Lewis acids AlCl3, SbF5, BF3, B(C6F5)3, and Al(OC(CF3)3)3 computed in this work are close to the values reported in the literature at different levels of theory.8 The computed FIA values of Al(OC(CF3)3)3 and 1 (Table S2‡) are close, but taking into account the fact that Al(OC(CF3)3)3 is isolable only as the fluorobenzene adduct Al(OC(CF3)3)3·PhF, 1 is expected to be a stronger Lewis superacid by 90 kJ mol−1 compared to the adduct.
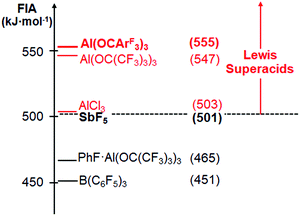 |
| Fig. 2 Fluoride ion affinities (FIAs) of selected Lewis acids (ArF = C6F5). | |
Reactivity of Al(OCArF3)3 towards Lewis bases
Having computationally established the high Lewis acidity of Al(OCArF3)3 (1), we directed our attention to its reactivity towards Lewis bases. In non-coordinating solvents, such as toluene or dichloromethane, the solubility of 1 at room temperature is very poor. When a solution of 1 in toluene is treated with neutral Lewis bases, the adducts Al(OCArF3)3·MeCN (2), Al(OCArF3)3·THF (3), Al(OCArF3)3·Et2O (4), Al(OCArF3)3·pyridine (5) and Al(OCArF3)3·OPEt3 (6) were obtained (Scheme 1). The 1H NMR chemical shifts (CD2Cl2) of the coordinating solvent molecules (e.g.: 2: δ = 2.57 ppm, 3: δ = 3.88 and 1.96 ppm) differ from those of the free solvents (MeCN: δ = 1.98 ppm, THF: δ = 3.68 and 1.82 ppm). The molecular structures of 2–6 show that the spatial arrangement of the Al atoms is distorted tetrahedral and defined by O3 + N and O3 + O donor sets, respectively (Fig. 3). Unlike 1, the adducts show no intramolecular Al⋯F contacts. The primary Al–O bond distances of 2–6 closely resemble those of 1. The molecular structure of 2 reveals an Al–N bond length of 1.941(1) Å and a C–N bond length of 1.134(2) Å which is shorter than in free acetonitrile (1.141(2) Å),18 which was also found for other Lewis acid base adducts (AlMe3·MeCN: 1.136(3) Å,19 B(C6F5)3·MeCN: 1.124(3) Å,18 [Cp3Zr(MeCN)][MeB(C6F5)3]: 1.126(5) Å
20). The Al–OTHF bonds of 3 (1.866(2) and 1.872(2) Å) are slightly longer than that of Al(OC(CF3)3)3·THF (1.824(2) Å).21
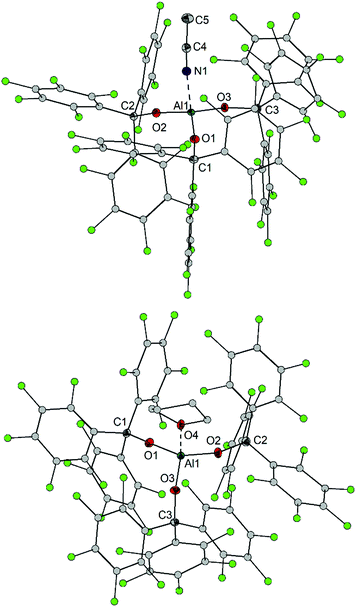 |
| Fig. 3 Molecular structures of Al(OCArF3)3·MeCN (2, top) and Al(OCArF3)3·THF (3, bottom; ellipsoids with 50% probability, balls with an arbitrarily fixed radius; hydrogen atoms, non-coordinating solvent molecules and a second molecular moiety in the THF adduct omitted for clarity). | |
The experimental Lewis acidity of Al(OCArF3)3
The Lewis acidity of 1 was investigated experimentally by three different methods: (1) considering the C–N stretching vibration of 2, (2) using the Gutmann–Beckett method and (3) in a competition experiment for fluoride ions with [SbF6]−.
(1) The C–N stretching vibration of Al(OCArF3)3·MeCN.
The C–N stretching vibration of 2 (2344 cm−1) is blue-shifted compared to free acetonitrile (2253 cm−1);18 more blue-shifted than other Lewis acid MeCN adducts including [Cp3Zr(MeCN)][MeB(C6F5)3] (2321 cm−1),20 SbF5·MeCN (2335 cm−1),22 and Al(OTeF5)3·MeCN (2340 cm−1),12 which correlates with the higher acid strength of 1 compared to other parent Lewis acids. However, it should be noted that the CN stretching mode of the acetonitrile adduct of the considerably less Lewis acidic BCF exhibits an even higher value of 2367 cm−1.18 The difficulty of the correlation of frequency of the C–N stretching mode with the Lewis acidity due to the Fermi coupling between ν(CN) and ν(CC) + δs(CH3) was pointed out by Krahl and Kemnitz.23 Hence, the adduct with deuterated acetonitrile was prepared also revealing a strong blueshift in the C–N stretching vibration (Al(OCArF3)3·CD3CN: 2338 cm−1, free CD3CN: 2258 cm−1,12 Al(OTeF5)3·CD3CN: 2328 cm−1).12 Greb suggested to restrict the comparison of the C–N stretching modes to compounds of high similarity.15
(2) The Gutmann–Beckett method.
The Gutmann–Beckett method uses the change of the 31P NMR shift of OPEt3 after coordination to a Lewis acidic center resulting in the so-called acceptor number (AN) as a benchmark for Lewis acidity.24 Al(OCArF3)3·OPEt3 exhibits a 31P NMR shift of 73.9 ppm in CD2Cl2 corresponding to a low AN of 72.7 compared to Lewis acids with a lower FIA (e.g. B(C6F5)3: 78.1 (CD2Cl2),25 B(OC6F5)3: 88.2 (C6D6),26 BF3·Et2O: 84.0 (CDCl3))27. However, the induced change of the 31P NMR shift does not only depend on the Lewis acidity, but also on HSAB and sterical effects. The large OC(C6F5)3 moieties provide sterical shielding of the aluminum center not allowing a small Al–O distance. Thus, the Gutmann–Beckett method seems unsuitable for the evaluation of the Lewis acidity of 1.
(3) A competition experiment.
As an experimental prove for Lewis superacidity, a competition experiment between 1 and [NBu4][SbF6] was performed in toluene (Scheme 2). It revealed the formation of [FAl(OCArF3)3]−via19F NMR spectroscopy28 suggesting a higher fluoride affinity of 1 compared to SbF5.
 |
| Scheme 2 Abstraction of a fluoride ion from SbF6− by Al(OCArF3)3. | |
As discussed in the literature,15 method (1) and (2) gave ambiguous results concerning the Lewis acidity of 1 and seem unsuitable in this context. However, the competition experiment (3) is in agreement with the calculated trend of the fluoride ion affinity and proves the Lewis superacidity of 1.
The weakly coordinating anions [FAl(OCArF3)3]− and [ClAl(OCArF3)3]−
The utility of Lewis acids goes hand in hand with their ability to form metallates, which can act as weakly coordinating anions.29 The reaction of 1 with several fluoride sources gave rise to a series of salts containing the WCA [FAl(OCArF3)3]− with different countercations (Scheme 1, 7–11): The conversion of 1 with [S(NMe2)3][Me3SiF2] in THF gave [S(NMe2)3][FAl(OCArF3)3] (10). It revealed 19F NMR chemical shifts of δ = −140.6, −159.6, −167.0 and −178.4 ppm (CD2Cl2) with the aluminum bound fluorine atom exhibiting the strongest highfield shift ([Ag(Me2SiO)6][FAl(OC(CF3)3)3]: δ = −176.3 ppm,30 [Cs(Tol)3][FAl(N(C6F5)2)3]: δ = −157.4 ppm).13 The trityl salt [Ph3C][FAl(OCArF3)3] (11) was prepared by the reaction of Al(OCArF3)3 (1) with Ph3CF in toluene in 64% yield.13 An analogous procedure using Ph3CCl gave the corresponding chloroaluminate [Ph3C][ClAl(OCArF3)3] (12) in 79% yield. Trityl salts of WCAs are frequently used as hydride or methyl group abstraction reagents, e.g. for the in situ generation of metallocene cations for the catalytic polymerization of alkenes31 and are also used as mild one-electron oxidants.32 The metal salts [M(sol)][FAl(OC(CF3)3)3] (M = Cs, Ag, Tl; sol = THF, Et2O, MeCN) were obtained from reacting 1 with CsF, AgF and TlF in acetonitrile. The silver and thallium salts of the WCA have the potential to generate reactive cations by halide ion abstraction, whereby the driving force is the precipitation of sparingly soluble silver or thallium halides. Silver salts of WCAs serve also as mild oxidants (Scheme 3). The use of the silver salt 8 was demonstrated in the reactions with lithium bromide and tetrabutyl ammonium bromide in which the precipitation of silver bromide gave rise to [Li(THF)2][FAl(OCArF3)3] 13 and [NBu4][FAl(OCArF3)3] 14, respectively (Scheme 3). 13 is suitable for salt metathesis reactions. Furthermore, 8 is able to oxidize ferrocene in dichloromethane to the ferrocenium salt [FeCp2][FAl(OCArF3)3] (15) which could act as a milder oxidant.
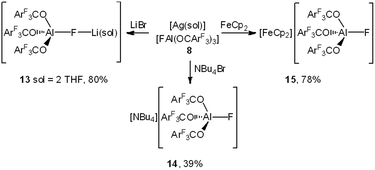 |
| Scheme 3 Reaction of the silver salt 8 with LiBr, NBu4Br and ferrocene. | |
The aluminates 7–9 and 11–15 were structurally characterized by X-ray crystallography (Fig. 4 and S5–S10‡). Like in the neutral Lewis acid base adducts 2–6, the spatial arrangement of 7–9 and 11–15 is tetrahedral. The Al–O distances found in the anions [FAl(OCArF3)3]− (e.g. 1.726(1), 1.745(1) and 1.745(1) Å in 11) are slightly elongated compared to the free Lewis acid 1 (1.681(1)–1.701(1) Å). The Al–F distances range from 1.678(1) Å in [NBu4][FAl(OCArF3)3] to 1.759(2) Å in [Li(Et2O)2][FAl(OCArF3)3] being considerably shorter than the hemilabile Al⋯F contacts of the free Lewis acid 1 (2.083(1) and 2.153(1) Å). In 7–9 and 13, there are weak cation⋯anion contacts between the metal ions and the Al–F (Cs⋯F: 2.886(2) and 2.869(2) Å, Ag⋯F: 2.314(1) and 2.224(4) Å, Tl⋯F: 2.506(2) Å, Li⋯F: 1.828(6) Å), which are absent in the [Ph3C]+, [S(NMe2)3]+, [NBu4]+ and [FeCp2]+ salts. The latter compounds tend to exhibit slightly shorter Al–F bond lengths. In the calculated [FAl(OCArF3)3]− anion, the Al–O distances (1.756 Å) and the Al–F distance (1.699 Å) are somewhat longer than the experimental values,33 but comparable to the Al–F distances found for [Ag(Me2SiO)6][FAl(OC(CF3)3)3] (1.677(4) Å)30 and [Cs(Tol)3][FAl(N(C6F5)2)3] (1.689(2) Å).13 The Al–Cl distance in [Ph3C][ClAl(OCArF3)3] is 2.1748(6) Å.
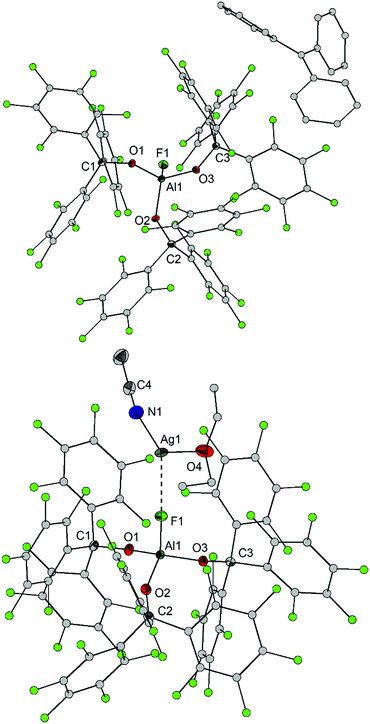 |
| Fig. 4 Molecular structure of [Ph3C][FAl(OCArF3)3] (Al1–F1 1.683(1) Å) (left) and [Ag(MeCN)(Et2O)][FAl(OCArF3)3] (Al1–F1 1.698(1) Å, F1⋯Ag1 2.310(1) Å, F1⋯Ag1a 2.224(4) Å) (right) (ellipsoids with 50% probability, balls with a arbitrarily fixed radius; hydrogen atoms and non-coordinating solvent molecules omitted for clarity; a positional disorder found for the Ag atom with an occupancy ratio of 0.85 : 015 is not displayed). | |
The stability of [FAl(OCArF3)3]− towards oxidation was investigated by cyclic voltammetry in acetonitrile revealing no oxidative processes at potentials of up to 2.2 V (vs. Fc/Fc+). In order to evaluate the coordination ability of the WCAs, the electrostatic potential was calculated (Fig. 5). In contrast to [FAl(OC(CF3)3)3]− (Fig. 5a) where the F atom bound to Al carries considerable negative charge and is quite open for the attack of the electrophile due to a lack of protection by OC(CF3)3 groups, the corresponding fluorine atom in [FAl(OCArF3)3]− (Fig. 5c) and chlorine atom in [ClAl(OCArF3)3]− (Fig. 5d) carry smaller charges and are shielded by the bulky OC(C6F5)3 groups. Their electrostatic potentials resemble that of Krossing's classical WCA [Al(OC(CF3)3)4]− (Fig. 5b).
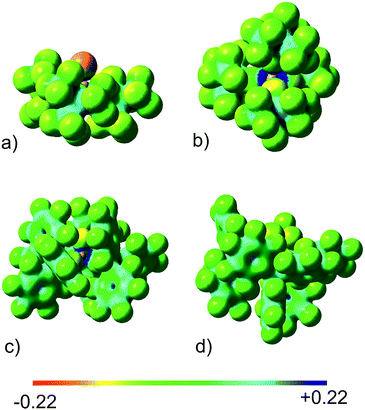 |
| Fig. 5 Projection of the calculated electrostatic potential on the 0.025 e− bohr−3 isodensity surface. (a) [FAl(OC(CF3)3)3]−; (b) [Al(OC(CF3)3)4]−; (c) [FAl(OCArF3)3]−; (d) [ClAl(OCArF3)3]−. B3LYP/6-311++G(2d,2p) level of theory. | |
In summary, the presented WCA salts can be utilized in broad range of applications: as oxidants (8, 11, 12, 15), in salt metathesis reactions (7–9, 13), in hydride or alkyl elimination reactions (11 and 12) or as supporting electrolytes (14) according to the scheme presented in ref. 29d.
Conclusions
Synthetic protocols for the synthesis of the new Lewis superacid Al(OCArF3)3 (1), and its corresponding WCAs [FAl(OCArF3)3]− and [ClAl(OCArF3)3]− were developed. Compared to literature known Lewis superacids, 1 stands out due to its high FIA. Its high thermal stability allows the adduct free isolation as crystalline solid and storage under argon at room temperature. Furthermore, the WCAs [FAl(OCArF3)3]− and [ClAl(OCArF3)3]− show favorable crystallization behavior due to the absence of disorder and we present a versatile series of their salts with different countercations as a toolbox for potential users. We hope these beneficial properties will encourage chemists from different fields to make use of these easily accessible compounds.
Conflicts of interest
There are no conflicts to declare.
Acknowledgements
We thank Prof. Dr Jörg Sundermeyer and Sebastian Ullrich for providing access to IR spectroscopic measurements under inert conditions. We thank Sarah Gansemer and Prof. Dr Anne Staubitz for TGA measurements and Daniel Duvinage and Dr Marian Olaru for support with the NMR spectroscopic measurements and cyclic voltammetry (D. D.) We thank Artem Zavgorodnii for his synthetic contribution. A. Y. T is grateful to RSF grant 18-13-00196.
Notes and references
-
(a) A. Corma and H. Garcia, Chem. Rev., 2003, 103, 4307 CrossRef CAS PubMed;
(b) S. Kobayashi and K. Manabe, Pure Appl. Chem., 2000, 72, 1373 CAS;
(c) F. Fringuelli, O. Piermatti, F. Pizzo and L. Vaccaro, Eur. J. Org. Chem., 2001, 439 CrossRef CAS;
(d) J. Faller and J. Jonathan, Curr. Org. Chem., 2006, 10, 151 CrossRef CAS.
-
(a) C. Welch, R. R. San Juan, J. D. Masuda and D. W. Stephan, Science, 2006, 314, 1124 CrossRef PubMed;
(b) G. C. Welch, L. Cabrera, P. A. Chase, E. Hollink, J. D. Masuda, P. Wei and D. W. Stephan, Dalton Trans., 2007, 3407 RSC. For recent reviews see
(c) D. W. Stephan, J. Am. Chem. Soc., 2015, 137, 10018 CrossRef CAS PubMed;
(d) D. W. Stephan and G. Erker, Angew. Chem., 2015, 127, 6498 (
Angew. Chem. Int. Ed.
, 2015
, 54
, 6400
) CrossRef.
- T. A. Engesser, M. R. Lichtenthaler, M. Schleep and I. Krossing, Chem. Soc. Rev., 2016, 45, 789 RSC.
- T. E. Mallouk, G. L. Rosenthal, G. Mueller, R. Brusasco and N. Bartlett, Inorg. Chem., 1984, 23, 3167 CrossRef CAS.
- K. O. Christe, D. A. Dixon, D. McLemore, W. W. Wilson, J. Sheehy and J. A. Bootz, J. Fluorine Chem., 2000, 101, 151 CrossRef CAS.
- L. O. Müller, D. Himmel, J. Stauffer, G. Steinfeld, J. Slattery, G. Santiso-Quiñones, V. Brecht and I. Krossing, Angew. Chem., 2008, 120, 7772 (
Angew. Chem. Int. Ed.
, 2008
, 47
, 7659
) CrossRef.
- A. Kraft, N. Trapp, D. Himmel, H. Böhrer, P. Schlüter, H. Scherer and I. Krossing, Chem.–Eur. J., 2012, 18, 9371 CrossRef CAS PubMed.
- H. Böhrer, N. Trapp, D. Himmel, M. Schleep and I. Krossing, Dalton Trans., 2015, 44, 7489 RSC.
- Calculated within this work (vide infra).
- For examples see:
(a) A. Reisinger, N. Trapp, I. Krossing, S. Altmannshofer, V. Herz, M. Presnitz and W. Scherer, Angew. Chem., 2007, 119, 8445 (
Angew. Chem. Int. Ed.
, 2007
, 46
, 8295
) CrossRef;
(b) I. Raabe, C. Röhr and I. Krossing, Dalton Trans., 2007, 36, 5376 RSC;
(c) D. Aris, J. Beck, A. Decken, I. Dionne, J. Schmedt auf der Günne, W. Hoffbauer, T. Köchner, I. Krossing, J. Passmore, E. Rivard, F. Steden and X. Wang, Dalton Trans., 2011, 40, 5865 RSC;
(d) H. Poleschner and K. Seppelt, Angew. Chem., 2013, 125, 13072 (
Angew. Chem. Int. Ed.
, 2013
, 52
, 12838
) CrossRef.
- L. A. Körte, J. Schwabedissen, M. Soffner, S. Blomeyer, C. G. Reuter, Y. V. Vishnevskiy, B. Neumann, H.-G. Stammler and N. W. Mitzel, Angew. Chem., 2017, 129, 8701 (
Angew. Chem. Int. Ed.
, 2017
, 56
, 8578
) CrossRef.
-
(a) A. Wiesner, T. W. Gries, S. Steinhauer, H. Beckers and S. Riedel, Angew. Chem., 2017, 129, 8375 (
Angew. Chem. Int. Ed.
, 2017
, 56
, 8263
) CrossRef;
(b) K. F. Hoffmann, A. Wiesner, N. Subat, S. Steinhauer and S. Riedel, Z. Anorg. Allg. Chem., 2018 DOI:10.1002/zaac.201800174.
- J. F. Kögel, D. A. Sorokin, A. Khvorost, M. Scott, K. Harms, D. Himmel, I. Krossing and J. Sundermeyer, Chem. Sci., 2018, 9, 245 RSC.
- R. Maskey, M. Schädler, C. Legler and L. Greb, Angew. Chem., 2018, 130, 1733 (
Angew. Chem. Int. Ed.
, 2018
, 57
, 1717
) CrossRef CAS Also see: A. L. Liberman-Martin, R. G. Bergman and T. D. Tilley, J. Am. Chem. Soc., 2015, 137, 5328 CrossRef PubMed.
- L. Greb, Chem.–Eur. J., 2018 DOI:10.1002/chem.201802698.
-
(a) R. D. Chambers and D. J. Spring, J. Chem. Soc. C, 1968, 2394 RSC;
(b) S. V. Kulkarni, R. Schure and R. Filler, J. Am. Chem. Soc., 1973, 95, 1859 CrossRef CAS.
- R. Jupp, T. C. Johnstone and D. W. Stephan, Dalton Trans., 2018, 47, 7029 RSC.
- H. Jacobsen, H. Berke, S. Döring, G. Kehr, G. Erker, R. Fröhlich and O. Meyer, Organometallics, 1999, 18, 1724 CrossRef CAS.
- M. R. Kopp and B. Neumüller, Z. Anorg. Allg. Chem., 1999, 625, 739 CrossRef CAS.
- T. Brackemeyer, G. Erker, R. Fröhlich, J. Prigge and U. Peuchert, Chem. Ber./Recl., 1997, 130, 899 CrossRef CAS.
- B. von Ahsen, B. Bley, S. Proemmel, R. Wartchow, H. Willner and F. Aubke, Z. Anorg. Allg. Chem., 1998, 624, 1225 CrossRef CAS.
- T. Krahl and E. Kemnitz, J. Fluorine Chem., 2006, 127, 663–678 CrossRef CAS.
- A. Bihlmeier, M. Gonsior, I. Raabe, N. Trapp and I. Krossing, Chem.–Eur. J., 2004, 10, 5041 CrossRef CAS PubMed.
-
(a) U. Mayer, V. Gutmann and W. Gerger, Monatsh. Chem., 1975, 106, 1235 CrossRef CAS;
(b) M. A. Beckett, G. C. Strickland, J. R. Holland and K. S. Varma, Polymer, 1996, 37, 4629 CrossRef CAS.
- A. E. Ashley, T. J. Herrington, G. G. Wildgoose, H. Zaher, A. L. Thompson, N. H. Rees, T. Krämer and D. O'Hare, J. Am. Chem. Soc., 2011, 133, 14727 CrossRef CAS PubMed.
- G. J. P. Britovsek, J. Ugolotti and A. J. P. White, Organometallics, 2005, 24, 1685 CrossRef CAS.
- E. L. Myersa, C. P. Buttsa and V. K. Aggarwal, Chem. Commun., 2006, 4434 RSC.
- Also the formation of free (C6F5)3COH was detected as a consequence of the formation of HF from the reaction between SbF5 and toluene. G. A. Olah, P. Schilling and I. M. Gross, J. Am. Chem. Soc., 1974, 96, 876 CrossRef CAS.
-
(a) S. H. Strauss, Chem. Rev., 1993, 93, 927 CrossRef CAS;
(b) I. Krossing and A. Reisinger, Coord. Chem. Rev., 2006, 250, 2721 CrossRef CAS;
(c) A. B. A. Rupp and I. Krossing, Acc. Chem. Res., 2015, 48, 2537 CrossRef CAS PubMed;
(d) I.
M. Riddlestone, A. Kraft, J. Schaefer and I. Krossing, Angew. Chem., 2018 DOI:10.1002/ange.201710782;
Angew. Chem. Int. Ed., DOI:10.1002/anie.201710782.
- T. S. Cameron, A. Decken, I. Krossing, J. Passmore, J. M. Rautiainen, X. Wang and X. Zeng, Inorg. Chem., 2013, 52, 3113 CrossRef CAS PubMed.
- D. S. McGuinness, A. J. Rucklidge, R. P. Tooze and A. M. Z. Slawin, Organometallics, 2007, 26, 2561 CrossRef CAS.
- E. F. van der Eide, W. E. Piers, M. Parvez and R. McDonald, Inorg. Chem., 2007, 46, 14 CrossRef CAS PubMed.
- B3LYP/6-311++G(2d,2p) level of theory (see ESI‡ for details).
Footnotes |
† ArF = C6F5. |
‡ Electronic supplementary information (ESI) available: Experimental procedures as well as computational and crystallographic details are reported in the electronic supplement. CCDC 1818681–1818687, 1863847–1863852 and 1866467. For ESI and crystallographic data in CIF or other electronic format see DOI: 10.1039/c8sc02981d |
|
This journal is © The Royal Society of Chemistry 2018 |
Click here to see how this site uses Cookies. View our privacy policy here.