DOI:
10.1039/C5EN00149H
(Tutorial Review)
Environ. Sci.: Nano, 2016,
3, 28-44
Recent advances in halloysite nanotube derived composites for water treatment
Received
9th July 2015
, Accepted 5th December 2015
First published on 7th December 2015
Abstract
Halloysite nanotubes (HNTs) are naturally occurring clay mineral with nanotubular structures and have found increasing potential applications in industrial fields. Here, after a brief introduction of the general structure, main properties and newly emerging applications of HNTs, particular attention is paid to HNT-derived applications in water treatment. We mainly review the recent progress in applications of HNT-derived nanocomposites in heavy metal ion, dye or organic pollutant removal from wastewater and HNT-containing membranes for water filtration. The HNT-derived composites exhibit superior properties for water treatment in various ways and are promising to be used in practical applications. Finally, we summarize the predominant mechanisms acting in the applications of water treatment and future prospects are discussed.
Nano impact
Halloysite nanotubes (HNTs) are one-dimensional nanomaterials with tubular structures and abundant deposits worldwide. In this article, recent progress in fabrication and applications of HNT-based composites is briefly reviewed. This is the first review paper to discuss the special applications of these inexpensive nanomaterials to address the problems of wastewater treatment. Critical issues and future prospects with respect to the practical applications in various types of wastewater recycling or treatment will be presented. We believe that HNTs with highly unusual geometry and surface chemistry will be potentially and effectively employed in massive removal of contaminants from wastewater in various ways in industries.
|
1. Introduction
Halloysite was first described in 1826 by Berthier as a dioctahedral 1
:
1 clay mineral of the kaolin group and extensive research began in the 1940s.1,2 Halloysite is widely found in both weathered rocks and soils worldwide. Countries like China, New Zealand, and Brazil have a considerable amount of halloysite deposits. The particles of halloysite adopt different morphologies including short tubular, spheroidal and platy shapes due to the variety of crystallization and geological occurrence. However, the predominant morphology of halloysite clay is elongated tubules, generally called halloysite nanotubes (HNTs). In this paper, “HNTs” is used hereafter for abbreviation to demonstrate the tubular halloysite clay, regardless of hydration and dehydration status, unless otherwise stated. Typically, the external diameter, lumen, and length of HNTs are 50–100 nm, 10–30 nm, and 100–2000 nm, respectively. These properties enable potential applications as nanoscale supports or functionalized nanomaterials and put HNTs back in the spotlight with the rapid development of nanoscience and nanotechnology in the past decade. Some other interesting characteristics of HNTs such as different internal and external surface chemistry, good biocompatibility and high mechanical strength will be discussed in detail in the following part. It is due to such a fact that HNTs have found increasing potential applications in various fields as reported in recent years. A survey of open publications in terms of “halloysite” in the past 10 years is presented in Fig. 1. These data, obtained based on a SciFinder Scholar search, obviously describe the increasing attention paid to halloysite, particularly in the past 4 years. Hence, an increasing number of publications are expected in the upcoming years.
 |
| Fig. 1 Annual number of scientific publications based on the word “halloysite” for the last decade (data analysis was done on 7th May, 2015). | |
Some interesting applications of HNTs were mainly focused on controlled/sustained release of drugs or bioactive molecules,3–10 cosmetics,11 medical implants,12,13 cancer cell isolation,14,15 and tissue engineering scaffolds,16–19 nanoreactors or nanotemplates20–22 due to cellular nontoxicity, environmental friendliness, biocompatibility properties as well as lumen space. Particularly, tremendous studies have focused on HNTs/polymer composites as an inexpensive and low-tech alternative, which provide exceptional mechanical, thermal and biological properties. Several researchers have reviewed the recent progress in the development of HNTs/polymer nanocomposites with their particular interests. Du et al. (2010)23 briefly reviewed the newly emerging applications of HNTs, emphasizing the fabrication of HNTs/polymer nanocomposites with remarkable reinforcing effects, enhanced flame retardancy and reduced thermal expansion properties. Lvov and Abdullayev (2013)24 presented recent studies on HNTs/polymer nanocomposites especially for the applications of controlled release. Liu and coworkers (2014)2 introduced recent advances in HNTs/polymer nanocomposites from the preparation, characterization to properties. In fact, besides the applications mentioned above, HNTs have always been functionalized for other various important applications. Previously, we have explored diverse useful applications of functionalized HNTs, such as immobilization of enzyme or antibacterial agents, proton conduction, super-absorbent resin, etc.25–35
With the rapid development of economy, the problem of pollution and shortage of water resource is becoming more serious, especially in developing countries. Among all the causes of water pollution, heavy metal ions, dyes, pesticide and some other organic pollutants take up a great proportion. In this regard, it is of significance to study approaches or inexpensive materials for wastewater treatment. As reported in very recent years, HNTs or HNT-based materials have also found many useful applications in treatment of drinking water or industrial wastewater. In addition, so far, no review articles on HNT-based materials have been published paying particular attention to applications in water treatment. Therefore, a comprehensive and critical review on this topic is necessary to provide a better understanding of the HNT-derived applications in water treatment. Accordingly, in this article, after briefly introducing the general structure and main properties of HNTs, we review the applications of HNT derived nanocomposites in fields of water treatment, paying special attention to heavy metal or dye removal from wastewater and membrane separation for water filtration.
2. General structure and main properties of halloysite nanotubes
HNTs, as rolled aluminosilicate sheets, have a similar theoretical formula to kaolinite expressed as Al2Si2O5(OH)4·nH2O, where n is equal to 2 and 0 for hydrated and dehydrated HNTs, respectively. Generally, the presence, or history, of interlayer water in HNTs is one of most significant characteristics that distinguish HNTs from kaolinite.37 The hydrated state (also called HNTs-10 Å) can transform into the dehydrated state (HNTs-7 Å) irreversibly even under ambient conditions (of temperature and humidity) or by moderate heating. It is therefore difficult, if not impossible, to handle HNTs-10 Å without inducing alteration in its hydration status.37 As the dominant morphology of halloysite, HNTs present different appearances, long or short, thin or stubby, as shown in Fig. 2a. HNTs have layered, well crystallized structure consisting of aluminum oxide octahedra in the inner layer and silicon dioxide tetrahedra in the outer layer (Fig. 2b). Fig. 2c presents the typical XRD patterns of HNTs powders including HNTs-10 Å and HNTs-7 Å states. Broad peaks corresponding to 9.934 Å and 7.326 Å are respectively found in HNTs-10 Å and HNTs-7 Å, assigned to the first order (001) basal reflections due to the less ordered multilayer structure. The high intensity and/or sharp peaks around 4.4 Å in either sample are indicative of halloysite,24,37 whereas just a quite weak and/or broad peak is observed for the kaolinite sample.
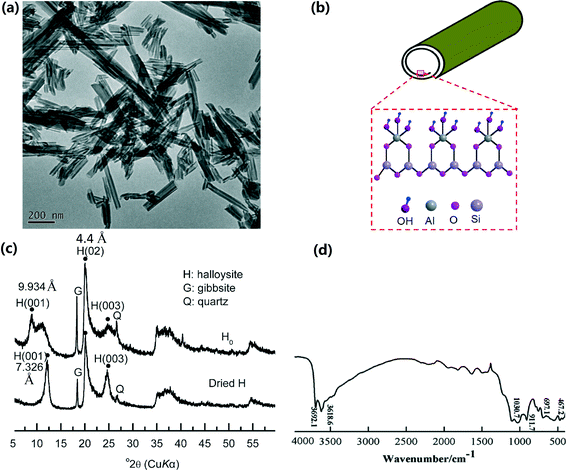 |
| Fig. 2 (a) TEM image, (b) crystalline structure, (c) XRD pattern36 and (d) FTIR curve42 of natural HNTs. | |
HNTs show a moderate BET surface area ranging from 22.1 to 81.6 m2 g−1 with a 10.7–39% lumen space and a relatively lower density of 2.14–2.59 g cm−3.38–40 The big lumen space provides advantages for the loading of active substances under vacuum by immersion in an appropriate solution. In addition, it is possible to enlarge the lumen space by selective acid corrosion of aluminum oxide as described by Lvov (see Fig. 3).41 The outer surface of HNTs shows different chemical and electrical properties from the inner surface because of the different crystal structures. The outer surface possesses a relatively lower hydroxyl group density (Si–OH) and a negative charge, while the inner surface has abundant hydroxyl groups (Al–OH) and a positive charge. In fact, the outer surface of HNTs is mostly occupied by Si–O–Si groups with a few Si–OH groups exposed on the edges of the sheets or possible defects.2 Therefore, the strong electronegativity of oxygen atoms makes the outer surface negatively charged. Meanwhile, the lower density of hydroxyl groups on the outermost surface along with the charged properties endows HNTs with good dispersion ability in nonpolar polymers, offering enormous advantages for the preparation of HNTs/polymer nanocomposites. In addition, the abundant Al–OH groups in the inner surface with high activity facilitate the selective modification or immobilization of guest molecules in the lumen space. These surface groups can be confirmed by FTIR results as presented in Fig. 2d. The peaks at 3692.1 cm−1 and 3618.6 cm−1 are attributed to Al2–OH stretching vibrations and the peak at 911.7 cm−1 is ascribed to the corresponding bending vibrations.37 The very strong absorption peak at 1030.7 cm−1 is assigned to the stretching vibrations of Si–O–Si groups.37 Additionally, HNTs are also considered as reinforcing and flame retardancy materials when in HNTs/polymer nanocomposites because of the high aspect ratio (length/diameter) and the barriers against heat and mass transport.2
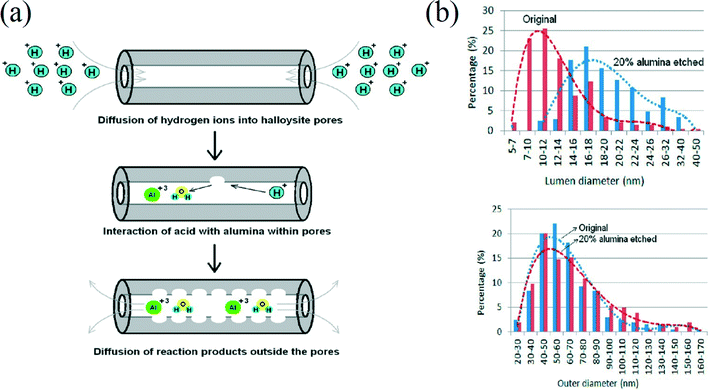 |
| Fig. 3 (a) Illustration of acid etching of alumina inner layers from HNT lumen and (b) histogram of lumen and outer diameters for original and alumina etched HNTs.41 | |
3. HNT-derived applications in removal of heavy metal ions
As mentioned above, the lumens of HNTs could be loaded with various active chemicals and the surfaces (both innermost and outermost surfaces) are readily non-covalently or covalently functionalized. HNTs have been considered as ideal alternatives for the preparation of adsorbents for removal of different kinds of heavy metal ions from wastewater, such as Cu(II), Pb(II), Cd(II), Zn(II), Cr(IV), and Co(II). The removal of hazardous heavy metals from aqueous media including drinking water, industrial or domestic wastewater, and soil solutions is of environmental importance to reduce the problem of water pollution and/or shortage worldwide. Generally, natural HNTs without any modification can remove heavy metal ions from aqueous media through the mechanisms of physical and/or chemisorption, site geometry, metal speciation, etc. However, in order to enhance the affinity and loading capacity for heavy metal ions, HNTs were often decorated with some interesting nanomaterials and/or functional groups to endow them with the extra mechanism of complexation. Table 1 summarizes the HNT-derived applications in removal of heavy metal ions from aqueous media. Because of the layer structure, natural HNTs were always intercalated with active molecules to improve the loading capacity and reusability. For example, Khelifa and coworkers developed intercalated HNTs with sodium acetate at various contact times and found that the most intercalated sample was more effective as an adsorbent of Cu(II) from aqueous solutions with a removal capacity of around 50 mg g−1.43 Subsequently, Khelifa and coworkers compared the variation of Cu(II) adsorption capacity for a series of intercalated HNTs with Na+, NH4+ and Pb2+ acetate, in which they believed that the Cu(II) adsorption capacity was not only related to the intercalated fraction of acetate but also partly attributed to cation exchange on the negative surface sites.44 Another approach to fabricating intercalated HNTs was proposed by Matusik et al. with diethanolamine and triethanolamine molecules as shown in Fig. 4.45 The tailored HNTs adsorption capacity in terms of Pb(II), Cd(II), Zn(II), and Cu(II) was significantly improved due to the two-step gradual diffusion of the metals into the interlayer space and subsequent binding to the amine nitrogen of the grafted amino alcohol.
Table 1 HNT-derived application in removal of heavy metals ions from aqueous media
Materials |
Heavy metals |
Feed conditionsa |
Removal capacity (mg g−1) |
Ref. |
Feed conditions demonstrate the initial concentration and pH of test heavy metal ions in aqueous solution.
HBA represents the abbreviation of 2-hydroxybenzoic acid.
PSA represents the abbreviation of N-2-pyridylsuccinamic acid.
PPy represents the abbreviation of polypyrrole.
HDTMA represents the abbreviation of hexadecyltrimethylammonium bromide.
KH-792 represents the abbreviation of N-β-aminoethyl-γ-aminopropyl trimethoxysilane.
|
HNTs/CH3COO− |
Cu(II) |
10–200 mg L−1; pH, 6 |
∼50 |
43
|
HNTs/CH3COONa |
Cu(II) |
10–200 mg L−1; pH, 6 |
52.3 |
44
|
HNTs/amino alcohols |
Pb(II), Cd(II), Zn(II), Cu(II) |
0.005–5.0 mmol L−1; pH, 2.0–6.5 |
— |
45
|
HNTs/HBAb |
Fe(III) |
10 mg L−1; pH, 4 |
45.54 |
46
|
HNTs/murexide |
Pd(II) |
1 mg L−1; pH, 1–7 |
42.86 |
47
|
HNTs/PSAc |
Pb(II) |
1 mg mL−1; pH, 1–7 |
23.58 |
48
|
HNTs/PPyd |
Cr(VI) |
1000 mg L−1; pH, 2–11 |
149.25 |
49
|
HNTs/HDTMAe |
Cr(VI) |
25–300 mg L−1; pH, 3–10 |
6.611 |
50
|
HNTs/KH-792f |
Cr(VI) |
0–400 mg L−1; pH, 2–9 |
37.25 |
51
|
HNTs/alginate |
Cu(II) |
50–100 mg L−1 |
74.13 |
52
|
Natural HNTs |
Zn(II) |
10 mg L−1; pH, 2–9 |
9.87 |
53
|
Natural HNTs |
Co(II) |
10 mg L−1; pH, 3–11 |
∼6 |
54
|
Natural HNTs |
Ag(I) |
∼50 mg L−1 |
3–6 |
55
|
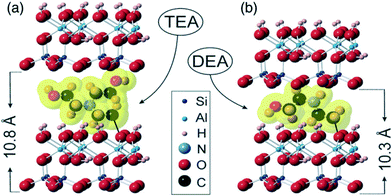 |
| Fig. 4 Approximate structure model of (a) triethanolamine and (b) diethanolamine intercalated HNTs.45 | |
Besides the aforementioned studies on non-covalently modified HNTs, there are more emerging works on covalently functionalized HNTs. Chang et al.46 reported 2-hydroxybenzoic acid (HBA) modified HNTs as a selective sorbent in solid phase exaction for separation and preconcentration of trace Fe(III) in aqueous media. The functionalized HNTs offered excellent selective solid phase exaction for Fe(III) with an enrichment factor of 75 and the maximum adsorption capacity was found to be 45.54 mg g−1 under optimum conditions. As solid phase exaction has become one of the most popular preconcentration/separation methods, He et al.48 also demonstrated acid-functionalized HNTs with N-2-pyridylsuccinamic acid for solid phase exaction of Pb(II). In their work, an enrichment factor of 65 and a maximum adsorption capacity of 25.38 mg g−1 for Pb(II) were obtained. For the removal of Cr(VI) from aqueous solutions, Mishra and Maity et al.49 fabricated a polypyrrole-coated HNTs nanocomposite via in situ polymerization of pyrrole onto HNTs. The authors reported a maximum adsorption capacity of 149.25 mg g−1 for Cr(VI) at pH 2.0 at 25 °C and the final nanocomposite was reused three times without loss of its original removal efficiency. Apart from the adsorption capacity, the adsorption rate is another important factor for evaluating HNT-based adsorbents. To increase the adsorption rate for Cr(VI), Zhang et al.50 proposed a novel HNT-based adsorbent modified with the surfactant hexadecyltrimethylammonium bromide. The modified HNTs exhibited a rapid adsorption rate for chromates and were capable of approaching 90% maximum adsorption capacity within 5 min. The effects of pH and ionic strength on the adsorption capacity were also investigated in their work and the modified HNTs were recovered by eluents after adsorption of Cr(VI). Additionally, in another work, Zhang et al. prepared silane coupling agent grafted HNTs for adsorption of Cr(VI) and also studied the rapid adsorption properties.51 Interestingly and particularly, a continuous fixed bed column for adsorption of Cu(II) was developed by Zhang et al. using HNTs/alginate hybrid beads, which is shown in Fig. 5.52 At the optimized bed height, influent concentration and flow rate conditions, the hybrid beds showed an excellent removal capacity of 74.13 mg g−1 for Cu(II) and regeneration properties with favorable stability.
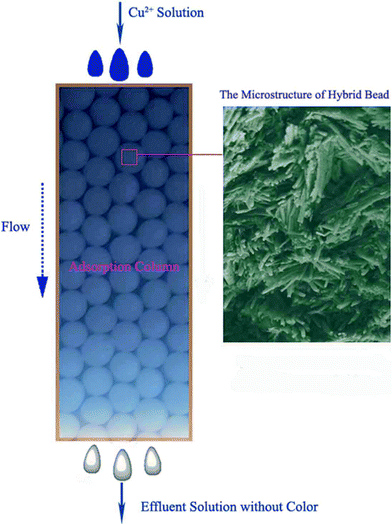 |
| Fig. 5 The schematic of HNTs/alginate fixed bed column for Cu(II) adsorption.52 | |
In some other works, the removal properties of natural HNTs without any treatment for Zn,53 Co,54 Ag55 were also systematically investigated. Compared to chemical methods for removing heavy metal ions from aqueous solutions, HNT-derived adsorbents showed great advantages such as lower cost, easy operation, reusability, non-secondary pollution, etc. Meanwhile, functionalized HNTs provided a much higher removal capacity (6.611–149.25 mg g−1) for heavy metal ions in marked contrast to natural HNTs (less than 10 mg g−1), which is clearly displayed in Table 1.
4. HNT-derived applications in removal of dyes
Dyes are extensively used in fields like the paper, plastic, leather, cosmetic, textile and food industries; however, dye-containing wastewater in these industries has to be treated carefully before discharging. The dye-containing wastewater, if discharged into natural streams, may cause various serious environmental problems, such as disturbance of aquatic photosynthesis and damage of the ecosystem. Therefore, the removal of dyes from wastewater has received considerable attention over the past few decades.56–58 Similar to the adsorption capacity for heavy metal ions, HNTs or HNT-derived composites also present superior removal capacity for dyes from dye-contaminated wastewater. Natural HNTs are capable of adsorbing both cationic and anionic dyes because of the negative Si–O–Si on the outermost surface and the positive Al–OH on the inner lumen surface (pH 4–9). Recently, a number of studies on removal of cationic, neutral and anionic dyes from aqueous solution onto natural HNTs have been reported.59–65 These works were based on the adsorption mechanisms including physical and chemisorption, site geometry, etc. and showed moderate adsorption capacity (26–113.46 mg g−1) and reusability.
In some other methods, HNTs were modified with Fe3O4 nanoparticles to combine magnetic separation technology with the adsorption process for dye removal from aqueous media.66–68 Magnetically modified HNTs are easier to separate from target aqueous solution after adsorption of dyes. Zhang and Liu et al.67 developed a magnetic nanocomposite of Fe3O4–HNTs by a chemical precipitation method. The resulted adsorbent maintained a high adsorption capacity for methyl violet of 88.32 mg g−1 and also exhibited good magnetic properties for magnetic isolation. Liu and coworkers described a HNTs/Fe3O4/carbon nanocomposite through in situ growth of Fe3O4 nanoparticles and a hydrothermal carbonization process of glucose on the surface of HNTs, which is shown in Fig. 6.68 The authors reported that the as-prepared nanocomposite is a fast, separable and superparamagnetic adsorbent with enhanced adsorption ability (57.13 mg g−1), where the values for natural HNTs and HNTs/Fe3O4 were 38.73 mg g−1, 29.33 mg g−1, respectively, under the same optimized conditions. Generally, magnetically modified HNTs may lose some adsorption capacity to a certain degree compared to pristine HNTs as confirmed by reports in the literature.66–68
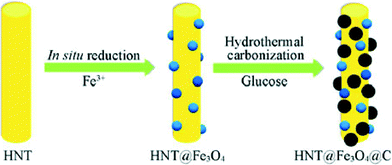 |
| Fig. 6 Schematic showing of synthesis of HNTs/Fe3O4/carbon nanocomposite.68 | |
For strengthening the adsorption capacity, chitosan,69 alginate,70 graphene71,72 and the like have been adopted to construct HNT-based adsorbents for the removal of dyes from aqueous media. Liu and Zhou et al.69 prepared composite hydrogel beads containing chitosan and HNTs by a dropping and pH-precipitation method (as shown in Fig. 7). The composite hydrogel beads were employed to remove methylene blue and malachite green from aqueous solutions, exhibiting improved thermal stability and reinforced adsorption ability (72.60 mg g−1 for methylene blue and 276.9 mg g−1 for malachite green). Liu and Zhang et al.71 described an HNTs@rGO composite (HGC) through homogeneous loading of HNTs on the surface of reduced graphene oxide sheets via an electrostatic self-assembly process (as shown in Fig. 8). The composite (HGC) showed a moderate adsorption capacity (45.4 mg g−1) for rhodamine B (RhB) and high performance (23.6 F g−1) as a supercapacitor. In another work, Zhang et al.72 reported a spherical zeolite/reduced graphene oxide composite established via blending HNT-derived zeolite with graphene oxide nanosheets (see Fig. 9). Although the tubular structure of HNTs was destroyed after hydrothermal reaction and regenerated a cubic or spherical zeolite as described in their work, the synthesized composite still showed an adsorption capacity of 53.3 mg g−1 for methylene blue and a smaller value of 48.6 mg g−1 for malachite green.
 |
| Fig. 7 Appearance of chitosan and HNTs-chitosan hydrogel beds in wet and dry state.69 | |
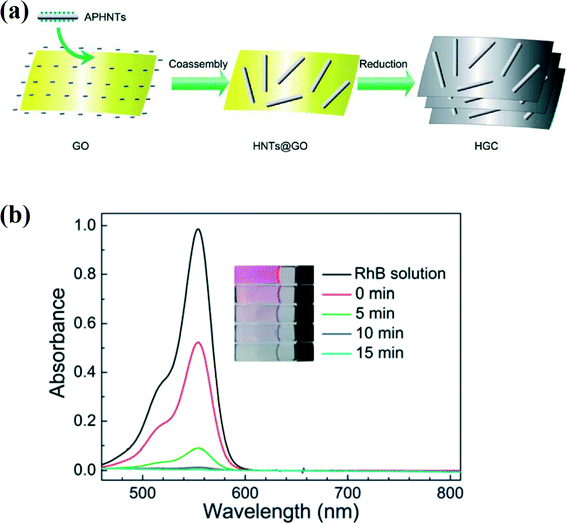 |
| Fig. 8 (a) Construction process of HGC and (b) UV-vis spectra of RhB solution and those after treatment with HGC at different times.71 | |
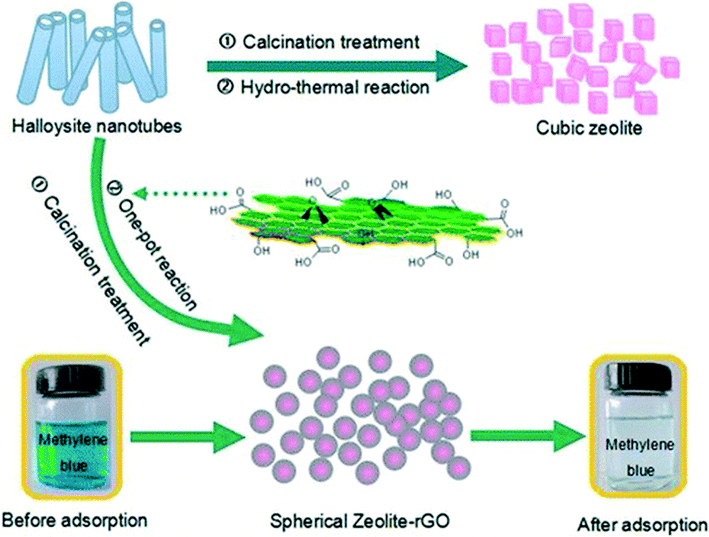 |
| Fig. 9 Schematic showing the synthesis of zeolite/rGO composite.72 | |
Theoretically, the works mentioned above in applications for dye removal are based on the adsorption mechanism. However, some researchers also proposed TiO2-,73–76 ZnO-,76 Ag-77,78 and CeO2-78 functionalized HNTs for the removal of dyes from aqueous media based on the dual mechanism of adsorption and photodegradation. Zheng et al.73 deposited anatase TiO2 on the surface of HNTs for adsorption and photodegradation of methylene blue. As expected, the HNTs/TiO2 composite exhibited an efficient photocatalytic activity in the decomposition of methylene blue. At a calcination temperature of 300 °C, methylene blue was degraded to a degree of 81.6% after 4 h of treatment of UV irradiation. Chuan and coworkers74 also fabricated a TiO2 mounted HNT composite by the hydrolysis of tetrabutyl titanate at room temperature and it was found that the resulted composite was capable of decreasing the concentration of methylene blue in aqueous solution rapidly due to the synthetic action of adsorption and photodecomposition. The photodecomposition activity test showed that almost 50% of methylene blue decomposed in 6 h and most decomposed in 48 h. In addition, a carbon-doped TiO2/HNTs hybrid nanofiber with enhanced visible-light photocatalytic performance was described by Liu et al. (as shown in Fig. 10).75 The visible-light photocatalytic efficiency of the nanofiber in the degradation of methyl blue was greatly reinforced with a moderate HNT doping amount of 8%, far greater than that of commercial anatase TiO2. Yang et al.76 reported a HNTs/MO/carbon nanocomposite by the deposition of metal oxide (MO) nanoparticles (ZnO, TiO2) onto carbon-coated HNTs for photodegradation of methylene blue (see Fig. 11). They investigated the effect of carbon on the photocatalytic activity and the results indicated that graphitic carbon could improve the conductivity of HNTs and lead to a significant improvement in photocatalytic properties (see Fig. 12). Du et al.77 reported a type of HNT supported Ag nanoparticles for photocatalytic decomposition of methylene blue using tea polyphenols as reductant (see Fig. 13), which exhibited good catalytic activity and high removal capacity for methylene blue. Within 60 min, nearly 90% of MB had been decomposed photo-catalytically by the HNTs/AgNPs catalyst. Ni et al.78 prepared a HNT supported hybrid CeO2–AgBr nanocomposite by a microwave mediated method (see Fig. 14a). The synergistic effect of CeO2 and AgBr greatly promoted the photocatalytic activity and the introduction of AgBr species was found to extend the spectral response from the UV to the visible region (see Fig. 14b).
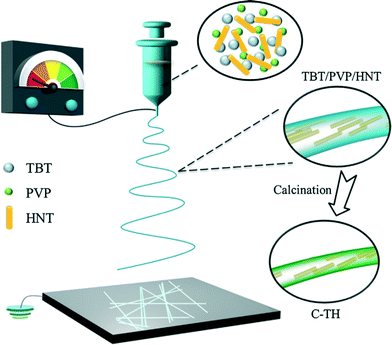 |
| Fig. 10 Schematic illustration of the synthesis of carbon-doped TiO2/HNTs hybrid nanofibers.75 | |
 |
| Fig. 11 Schematic of the fabrication of HNTs/MO/carbon nanocomposite.76 | |
 |
| Fig. 12 Proposed mechanisms for enhanced photocatalytic activity of HNTs/MO/carbon nanocomposite without (a) and with (b) carbon coating.77 | |
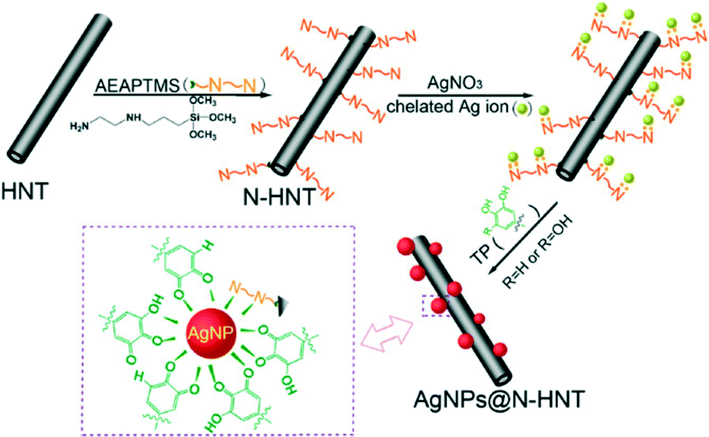 |
| Fig. 13 Illustration of synthesis of HNT supported AgNPs.77 | |
 |
| Fig. 14 (a) Scheme of photocatalytic degradation mechanism of HNTs/CeO2/AgBr nanocomposite and (b) photocatalytic degradation of methyl orange in aqueous solution under solar light irradiation.78 | |
Overall, different from removal of heavy metals, there are two main mechanisms for removal of dyes from dye-containing wastewater, namely, the single role of adsorption and the dual role of adsorption and degradation. Generally, the dual-role mechanism is more efficient than the single-role mechanism because of its sustainability. For single-role based adsorbents, the adsorption capacity is losing with using circles and a possible regeneration process is necessary to recover the capacity (Table 2).
Table 2 HNT-derived application in removal of dyes from aqueous media
Materials |
Dyes |
Mechanism |
Feed concentrationa (mg L−1) |
Removal capacity (mg g−1) |
Ref. |
Feed concentration demonstrates the initial concentration of test dyes in aqueous solution, which is not the optimized concentration.
rGO represents the abbreviation of reduced graphene oxide nanosheets.
Zeolite here was fabricated from natural HNTs by hydrothermal reaction.
AgNPs represents the abbreviation of silver nanoparticles.
|
Natural HNTs |
Methylene blue |
Adsorption |
100–300 |
84.32 |
59
|
Natural HNTs |
Methyl violet |
Adsorption |
50–400 |
113.64 |
60
|
Natural HNTs |
Malachite green |
Adsorption |
20–100 |
99.6 |
61
|
Natural HNTs |
Rhodamine 6G & chrome azurol |
Adsorption |
300 |
43.6, 38.7 |
62
|
Natural HNTs |
Methyl orange & Congo red |
Adsorption |
300 |
~26 |
63
|
Natural HNTs |
Neutral red |
Adsorption |
50–400 |
65.45 |
64
|
Activated HNTs |
Methylene blue |
Adsorption |
50–500 |
103.63 |
65
|
HNTs/Fe3O4 |
Methylene blue & neutral red & methyl orange |
Adsorption |
0.1 mmol L−1 |
18.44 |
66
|
HNTs/Fe3O4 |
Methyl violet |
Adsorption |
50–400 |
88.2 |
67
|
HNTs/Fe3O4/carbon |
Methylene blue |
Adsorption |
10–70 |
57.13 |
68
|
HNTs/chitosan |
Methylene blue & malachite green |
Adsorption |
20–400 |
72.60, 276.9 |
69
|
HNTs/alginate |
Methylene blue |
Adsorption |
50–500 |
250 |
70
|
HNTs/rGOb |
Rhodamine B |
Adsorption |
0.01 mmol L−1 |
45.4 |
71
|
Zeolite/rGOc |
Methylene blue & malachite green |
Adsorption |
50–300 |
53.3, 48.6 |
72
|
HNTs/TiO2 |
Methylene blue |
Adsorption & degradation |
0.1 mmol L−1 |
52.49 |
73
|
HNTs/TiO2 |
Methylene blue |
Adsorption & degradation |
40 |
29.64 |
74
|
HNTs/TiO2/carbon |
Methyl blue |
Degradation |
20 |
— |
75
|
HNTs/MO/carbon |
Methylene blue |
Adsorption & degradation |
5 |
~50 |
76
|
HNTs/AgNPsd |
Methyl blue |
Degradation |
0.0267 mmol L−1 |
— |
77
|
HNTs/CeO2/AgBr |
Methyl orange |
Degradation |
20 |
— |
78
|
5. HNT-derived applications in membrane separation
HNTs have been proved to be capable of improving the mechanical strength, thermal stability and separation properties of HNT-containing polymer membranes. Various types of membranes including ultrafiltration (UF), nanofiltration (NF), forward osmosis (FO), reverse osmosis (RO), membrane reactor (MR), nanofiber, microfiber membranes, etc. have been reported adopting HNTs as inorganic fillers to endow them with special functions (as summarized in Table 3).79–95 For instance, natural HNTs were incorporated to UF,79 FO (see Fig. 15),92 RO93 or nanofiber95 membrane matrix to reinforce the mechanical strength and improve the hydrophilicity. As reported, all the mentioned membranes exhibited improved water flux compared to HNT-free membranes due to the interesting characteristics of natural HNTs. One important factor is the hydrophilicity of natural HNTs, which may result in an increase in solubilization and diffusion of water molecules into the membrane matrix, thus facilitating water permeation into membranes. In addition, the formation of voids at the interface of embedded HNTs and the presence of lumen of HNTs possibly provide more short pathways for permeation of water molecules. In some other works, natural HNTs were functionalized with Ag nanoparticles,80,82 Cu(II) ions,81N-halamine,87 lysozyme,88etc. to prepare antimicrobial hybrid ultrafiltration membranes as reported by Zhang et al. In these membranes, functionalized HNTs provided efficient antibacterial performance against both Gram-negative (E. coli) and Gram-positive (S. aureus) bacteria in the water filtration process (see Fig. 17 and 18). The antimicrobial phenomenon is mainly based on a contact-destruction mechanism, where the HNT supported antibacterial reagents appear on the membrane surface and/or the pore wall of hybrid membranes. In order to improve the anti-protein-fouling properties, 2-methacryloyloxyethyl phosphorylcholine (MPC) (see Fig. 19),85 dextran,86etc. grafted HNTs were also blended to polymer membrane matrix to prepare anti-protein-fouling membranes, which showed improved pure water flux and antifouling properties. The antifouling properties of hybrid membranes benefit from the improvement of membrane surface hydrophilicity after the introduction of modified HNTs. For the applications of dye/salt separation in aqueous media, Zhang and Liu et al. proposed charged “loose” nanofiltration membranes fabricated through introducing charged functionalized HNTs into the membrane matrix.89,90 This type of “loose” nanofiltration membrane was confirmed to maintain relatively higher dye rejection and “near-zero” salt rejection as shown in Fig. 20. There membranes therefore may find potential applications for direct separation of dyes/salts from dye-containing wastewater. Ismail et al.94 reported a type of photocatalytic membrane reactor by the incorporation of TiO2 modified HNTs into the PVDF membrane matrix. The obtained nanocomposite membrane played the roles of both degradation and separation for bilge water. In fact, many other types HNT-containing films/membranes have been reported in the literature in recent years. Here, however, only membranes used for water treatment were reviewed (Fig. 16).
Table 3 HNT-derived application in membrane separation
Materials |
Matrix |
Membrane process or type |
Functiona |
Ref. |
Function demonstrates the key role played by HNT-based materials in the membrane matrix.
PES represents the abbreviation of polyether sulfone.
UF represents the abbreviation of ultrafiltration.
CuNPs represents the abbreviation of copper nanoparticles.
MPC represents the abbreviation of 2-methacryloyloxyethyl phosphorylcholine.
PSS represents the abbreviation of poly(sodium-4-styrenesulfonate).
NF represents the abbreviation of nanofiltration.
PIL represents the abbreviation of poly(ionic liquid).
PA represents the abbreviation of polyamide.
FO represents the abbreviation of forward osmosis.
RO represents the abbreviation of reverse osmosis.
PVDF represents the abbreviation of polyvinylidene fluoride.
MR represents the abbreviation of membrane reactor.
PAN represents the abbreviation of polyacrylonitrile.
|
Natural HNTs |
PESb |
UFc |
Hydrophilic modification |
79
|
HNTs/AgNPs |
PES |
UF |
Antimicrobial |
80
|
HNTs/Cu(II) |
PES |
UF |
Antimicrobial |
81
|
HNTs/Cs/AgNPs |
PES |
UF |
Antimicrobial |
82
|
HNTs/CuNPsd |
PES |
UF |
Antimicrobial |
83
|
HNTs/rGO/AgNPs |
PES |
UF |
Antimicrobial |
84
|
HNTs/MPCe |
PES |
UF |
Antifouling |
85
|
HNTs/dextran |
PES |
UF |
Antifouling |
86
|
HNTs/N-halamine |
PES |
UF |
Antimicrobial |
87
|
HNTs/lysozyme |
PES |
UF |
Antimicrobial |
88
|
HNTs/PSSf |
PES |
NFg |
Ion-exchange |
89
|
HNTs/PILh |
PES |
NF |
Ion-exchange |
90
|
Sulfonated HNTs |
PES |
NF |
Ion-exchange |
91
|
Natural HNTs |
PAi |
FOj |
Antifouling |
92
|
Natural HNTs |
PA |
ROk |
Antifouling |
93
|
HNTs/TiO2 |
PVDFl |
MRm |
Photocatalyst |
94
|
Natural HNTs |
PANn |
Nanofiber |
Reinforcement |
95
|
 |
| Fig. 15 Schematic diagram of HNTs/PA thin film composite forward osmosis membrane using PSf substrate.92 | |
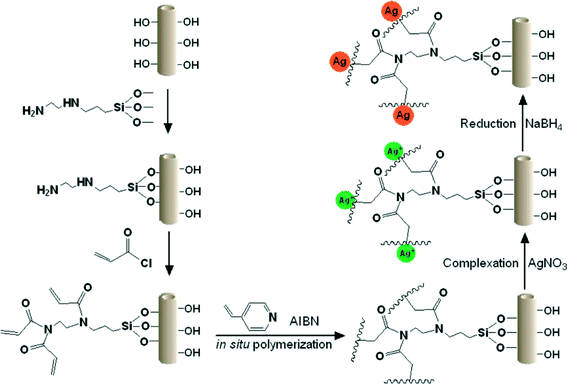 |
| Fig. 16 Reaction principle of HNTs–AgNPs for fabrication of antimicrobial membrane.80 | |
 |
| Fig. 17 Photographs showing the bacterial culture plates of (a) E. coli and (b) S. aureus attached to PES and PES/HNTs–AgNPs membranes.80 | |
 |
| Fig. 18 (a) SEM images of E. coli attached to PES membrane and N-halamine@HNTs/PES membrane; (b) bacteriostasis rate measurement of PES membrane and N-halamine@HNTs/PES membrane.87 | |
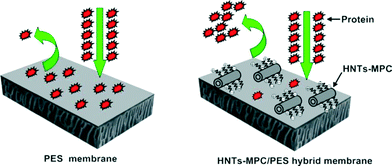 |
| Fig. 19 Schematic diagram of HNTs–MPC/PES anti-protein-fouling ultrafiltration membrane.85 | |
 |
| Fig. 20 (a) Schematic showing the fabrication process of HNTs-PIL/PES positively charged nanofiltration membrane and (b) rejections for dyes and salts of HNTs-PIL/PES membranes as a function of HNTs-PIL content.90 | |
In summary, polymer membranes containing HNTs are generally endowed with relatively higher hydrophilicity, water flux, mechanical strength and thermal stability. The significant properties of membranes, particularly for water treatment, like antimicrobial, antifouling, ion-exchange, photocatalysis, etc. can also be obtained by inducing functionalized HNTs, as shown in Table 3.
6. Other HNT-derived applications for water treatment
Herein, we have discussed the applications of HNT-derived composites as adsorbents in heavy metal ion and dye adsorption/degradation. In fact, due to the tubular structure and multiple surface groups, HNTs have gradually found many other applications for contaminant adsorption/degradation. As listed in Table 4, the adsorption/degradation of tetracycline,96–100 ammonium ions,101–104 2,4-dichlorophenoxyacetic acid,105 chloroanilines,106 phenol-based contaminant107,108 5-aminosalicylic acid,109etc. by HNT-based composites from aqueous solutions has been reported. Li et al.96 proposed highly-controllable core–shell nanorods for selective recognition and rapid adsorption of tetracycline by loading of Fe3O4 into lumen of HNTs and imprinted polymerization at the surface. The tunable nanoshell thickness would affect the saturation adsorption capacity for tetracycline and the largest saturation adsorption capacity appeared at a thickness of 35 nm. Wang et al.97 also reported a magnetic HNT-based composite of HNTs/CoFe3O4 for the removal of tetracycline hydrochloride from aqueous solutions. The authors investigated the effects of pH, temperature, initial concentrations and reaction time on the adsorption capacity for tetracycline hydrochloride. For degradation of tetracycline, TiO2-,98 CdS-,99,100etc. functionalized HNTs were always adopted to realize the photodegradation properties. Conducting polymers, carbon or metal ions were frequently introduced to metal oxide functionalized HNTs to enhance the electrical conductivity for photocatalysis.
Table 4 Other HNT-derived application for water treatment
Materials |
Applications |
Ref. |
MIP represents the abbreviation of molecularly imprinted polymer.
PNIPAM represents the abbreviation of poly(N-isopropylacrylamide).
Zeolite was synthesized from natural HNTs by hydrothermal reaction.
CTS, GTA, and HRP represent the abbreviations of chitosan, glutaraldehyde, and horseradish peroxidase, respectively.
RB represents the abbreviation of Rose bengal.
|
HNTs/Fe3O4/MIPa |
Adsorption of tetracycline |
96
|
HNTs/CoFe2O4 |
Adsorption of tetracycline hydrochloride |
97
|
HNTs/TiO2/Mn+ |
Degradation of tetracycline |
98
|
HNTs/CdS/PNIPAmb |
Degradation of tetracycline |
99
|
HNTs/CdS–Mn+ |
Degradation of tetracycline |
100
|
NaA zeolitec |
Adsorption of ammonium |
101
|
Zeolite Xc |
Adsorption of ammonium |
102
|
NaA zeolite/chitosanc |
Adsorption of ammonium |
103
|
Zeolite Yc |
Adsorption of ammonium |
104
|
HNTs/Fe3O4/MIP |
Enrichment of 2,4-dichlorophenoxyacetic acid |
105
|
Acid-activated HNTs |
Adsorption of chloroanilines |
106
|
HNTs–CTS–GTA–HRPd |
Degradation of phenol |
107
|
HNTs/RBe |
Degradation of phenol-based pesticide |
108
|
Natural HNTs |
Adsorption of 5-aminosalicylic acid |
109
|
Considering the removal of ammonium ions from aqueous solutions, Zhang and coworkers developed a series of zeolite, such as NaA zeolite,101,103 zeolite X,102 and zeolite Y104 from natural HNTs by a hydrothermal method. The tubular structure of HNTs changed to cubic or spherical forms with high crystallinity and uniform pore channels after the hydrothermal reaction (see Fig. 21 and 22). The synthesized zeolite or hybrid beads showed higher adsorption capacity and fast adsorption rate for ammonium ions (NH4+) and the authors believed that such low cost adsorbents could be utilized for effective and environmentally-friendly removal of NH4+ pollutants from wastewater.
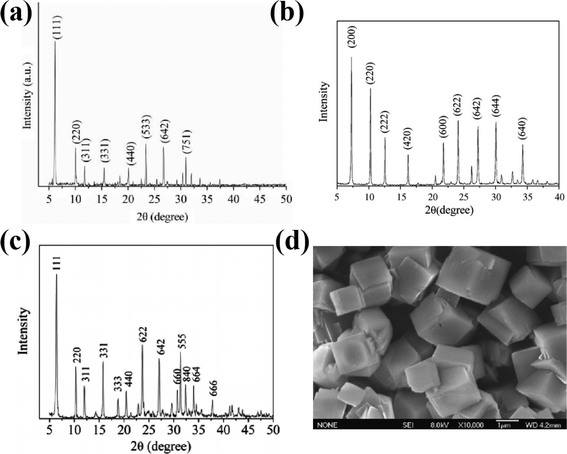 |
| Fig. 21 XRD patterns of (a) zeolite X, (b) NaA zeolite, (c) zeolite Y and (d) SEM image of NaA zeolite synthesized from natural HNTs.101–104 | |
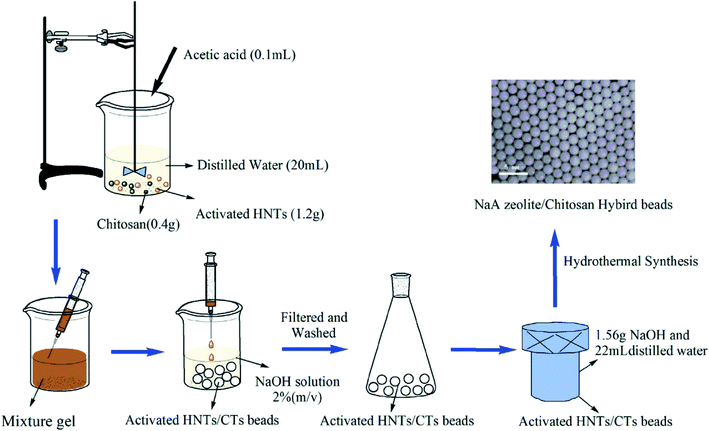 |
| Fig. 22 Schematic showing the fabrication process of NaA zeolite/chitosan hybrid beads.103 | |
Phenol-based pesticides are widely used in agriculture and considered as one of the most important endocrine disrupting chemicals present in the environment. Some of them, for instance, nonylphenol compounds, are stable in water and exhibit aquatic toxicity and estrogenic activity even at very low concentrations. HNTs were also employed as basis materials to prepare some special adsorbents for adsorption/degradation of phenol-based pollutants. Zhang and coworkers107 synthesized HNTs/chitosan hybrid nanotubes through assembly onto HNTs and then immobilized horseradish peroxidase (HRP) on the hybrid nanotubes for the degradation of phenol (see Fig. 23). The immobilized HRP did not demonstrate activity loss but exhibited commendable removal efficiency for phenol from wastewater. Szczubiałka et al.108 prepared a hybrid photosensitizer by the incorporation of Rose Bengal (RB) into HNTs for the photodegradation of phenol-based pesticide. The photosensitizer was found to be efficient for singlet oxygen generation and combine adsorption ability with photocatalytic properties.
 |
| Fig. 23 Schematic illustration of (a) the preparation of chitosan–halloysite hybrid nanotubes and (b) the immobilization of horseradish peroxidase.107 | |
7. Conclusions and future prospects
In this review, we summarized the general structure and main properties of HNTs and provided an overview of very recent research studies on HNT-derived nanocomposites for water treatment. Increasing exciting applications have been discovered due to the interesting characteristics of HNTs such as nanoscale lumen, high aspect ratio, different internal and external surface chemistry, and abundant deposition. HNT-derived applications in water treatment were mainly focused on removal of heavy metal ions and dyes from aqueous media and preparation of all kinds of mixed matrix membranes. There are three main mechanisms involved in this review for water treatment:
(1) Adsorption-predominant. The removal of heavy metal ions, some dyes or some organic pollutants from polluted water resources is based on this adsorption-predominant mechanism. The adsorption process of HNT-based materials is complicated, which may include physi- and/or chemisorption, site geometry, metal speciation and complexation effect.
(2) Combination of adsorption and degradation. For removal of some degradable dyes or organic pollutants from wastewater, both adsorption and degradation (mostly, photodegradation) effects are the critical factors for the removal efficiency. TiO2, Ag, CdS, enzyme, etc. were loaded onto the lumen or external surface of HNTs to endow them with additional degradation properties. The dual mechanism of both adsorption and degradation showed advantages compared to either single mechanism. The dual mechanism is more efficient for the removal of degradable pollutants and is promising for future applications.
(3) Interfacial interaction and reinforcement. For membrane preparation, natural HNTs or functionalized HNTs were selected as nanofillers to reinforce membrane performance, such as mechanical strength, thermal stability and permeation properties. In addition, the incorporated functional reagents on the surface of HNTs are capable of endowing hybrid membranes with novel properties, such as antifouling, antimicrobial, catalytic performance, etc.
Overall, HNTs are promising and cost-effective materials in the future for preparation of functional organic/inorganic nanocomposites. The significant progress in HNT-derived applications in water treatment may provide more opportunities for recycling and purification of industrial or domestic wastewater. Future studies should pay more attention to the improvement of operability, regeneration and processing capacity in practical applications.
Acknowledgements
This work was financially sponsored by the National Natural Science Foundation of China (No. 21376225 and 21476215) and Excellent Youth Development Foundation of Zhengzhou University (No. 1421324066).
References
- P. Berthier, Ann. Chim. Phys., 1826, 32, 332–335 Search PubMed.
- M. Liu, Z. Jia, D. Jia and C. Zhou, Prog. Polym. Sci., 2014, 39, 1498–1525 CrossRef CAS.
- D. Fix, D. V. Andreeva, Y. M. Lvov, D. G. Shchukin and H. Möhwald, Adv. Funct. Mater., 2009, 19, 1720–1727 CrossRef CAS.
- H. Schmitt, N. Creton, K. Prashantha, J. Soulestin, M. Lacrampe and P. Krawczak, Polym. Eng. Sci., 2015, 55, 573–580 CAS.
- S. A. Garea, A. Ghebaur and E. Vasile, Mater. Plast., 2014, 51, 124–129 CAS.
- H. Wu, Y. Shi, C. Huang, Y. Zhang, J. Wu, H. Shen and N. Jia, J. Biomater. Appl., 2014, 28, 1180–1189 CrossRef PubMed.
- P. áLo Meo, J. Mater. Chem. B, 2014, 2, 7732–7738 RSC.
- L. Fan, B. Li, Q. Wang, A. Wang and J. Zhang, Adv. Mater. Interfaces, 2014, 1 Search PubMed.
- G. Cavallaro, G. Lazzara, S. Milioto, G. Palmisano and F. Parisi, J. Colloid Interface Sci., 2014, 417, 66–71 CrossRef CAS PubMed.
- N. G. Veerabadran, D. Mongayt, V. Torchilin, R. R. Price and Y. M. Lvov, Macromol. Rapid Commun., 2009, 30, 99–103 CrossRef CAS PubMed.
- Y. Suh, D. Kil, K. Chung, E. Abdullayev, Y. Lvov and D. Mongayt, J. Nanosci. Nanotechnol., 2011, 11, 661–665 CrossRef CAS PubMed.
- H. Kelly, P. Deasy, E. Ziaka and N. Claffey, Int. J. Pharm., 2004, 274, 167–183 CrossRef CAS PubMed.
- S. Feitosa, J. Palasuk, K. Kamocki, S. Geraldeli, R. Gregory, J. Platt, L. Windsor and M. Bottino, J. Dent. Res., 2014, 93, 1270–1276 CrossRef CAS PubMed.
- A. D. Hughes and M. R. King, Langmuir, 2010, 26, 12155–12164 CrossRef CAS PubMed.
- A. D. Hughes, J. Mattison, J. D. Powderly, B. T. Greene and M. R. King, J. Visualized Exp., 2012, 64, e4248 Search PubMed.
- M. Liu, L. Dai, H. Shi, S. Xiong and C. Zhou, Mater. Sci. Eng., C, 2015, 49, 700–712 CrossRef CAS PubMed.
- M. Liu, C. Wu, Y. Jiao, S. Xiong and C. Zhou, J. Mater. Chem. B, 2013, 1, 2078–2089 RSC.
- G. Nitya, G. T. Nair, U. Mony, K. P. Chennazhi and S. V. Nair, J. Mater. Sci.: Mater. Med., 2012, 23, 1749–1761 CrossRef CAS PubMed.
- W. Y. Zhou, B. Guo, M. Liu, R. Liao, A. B. M. Rabie and D. Jia, J. Biomed. Mater. Res., Part A, 2010, 93, 1574–1587 Search PubMed.
- D. G. Shchukin, G. B. Sukhorukov, R. R. Price and Y. M. Lvov, Small, 2005, 1, 510–513 CrossRef CAS PubMed.
- Y. Fu, L. Zhang and J. Zheng, J. Nanosci. Nanotechnol., 2005, 5, 558–564 CrossRef CAS PubMed.
- A. Wang, F. Kang, Z. Huang, Z. Guo and X. Chuan, Microporous Mesoporous Mater., 2008, 108, 318–324 CrossRef CAS.
- M. Du, B. Guo and D. Jia, Polym. Int., 2010, 59, 574–582 CAS.
- Y. Lvov and E. Abdullayev, Prog. Polym. Sci., 2013, 38, 1690–1719 CrossRef CAS.
- J. Jiang, Y. Zhang, D. Cao and P. Jiang, Chem. Eng. J., 2013, 215, 222–226 CrossRef.
- L. Yu, Y. Zhang, B. Zhang and J. Liu, Sci. Rep., 2014, 4, 4551–4555 Search PubMed.
- H. Zhang, T. Zhang, J. Wang, F. Pei, Y. He and J. Liu, Fuel Cells, 2013, 13, 1155–1165 CrossRef CAS.
- H. Bai, H. Zhang, Y. He, J. Liu, B. Zhang and J. Wang, J. Membr. Sci., 2014, 454, 220–232 CrossRef CAS.
- H. Zhang, C. Ma, J. Wang, X. Wang, H. Bai and J. Liu, Int. J. Hydrogen Energy, 2014, 39, 974–986 CrossRef CAS.
- R. Zhai, B. Zhang, L. Liu, Y. Xie, H. Zhang and J. Liu, Catal. Commun., 2010, 12, 259–263 CrossRef CAS.
- L. Yan, J. Jiang, Y. Zhang and J. Liu, J. Nanopart. Res., 2011, 13, 6555–6561 CrossRef CAS.
- Y. Zhang, Y. Chen, H. Zhang, B. Zhang and J. Liu, J. Inorg. Biochem., 2013, 118, 59–64 CrossRef CAS PubMed.
- C. Liu, L. Yu, Y. Zhang, B. Zhang, J. Liu and H. Zhang, RSC Adv., 2013, 3, 13756–13763 RSC.
- C. Chao, J. Liu, J. Wang, Y. Zhang, B. Zhang, Y. Zhang, X. Xiang and R. Chen, ACS Appl. Mater. Interfaces, 2013, 5, 10559–10564 CAS.
- X. Ding, H. Wang, W. Chen, J. Liu and Y. Zhang, RSC Adv., 2014, 4, 41993–41996 RSC.
- A.-P. Wang, F. Kang, Z.-H. Huang and Z. Guo, Clays Clay Miner., 2006, 54, 485–490 CrossRef CAS.
- E. Joussein, S. Petit, J. Churchman, B. Theng, D. Righi and B. Delvaux, Clay Miner., 2005, 40, 383–426 CrossRef CAS.
- P. Pasbakhsh, G. J. Churchman and J. L. Keeling, Appl. Clay Sci., 2013, 74, 47–57 CrossRef CAS.
- U. A. Handge, K. Hedicke-Höchstötter and V. Altstädt, Polymer, 2010, 51, 2690–2699 CrossRef CAS.
- A. Alhuthali and I. M. Low, J. Mater. Sci., 2013, 48, 4260–4273 CrossRef CAS.
- E. Abdullayev, A. Joshi, W. Wei, Y. Zhao and Y. Lvov, ACS Nano, 2012, 6, 7216–7226 CrossRef CAS PubMed.
- F. Dong, J. Wang, Y. Wang and S. Ren, J. Mater. Chem., 2012, 22, 11093–11100 RSC.
- S. Mellouk, A. Belhakem, K. Marouf-Khelifa, J. Schott and A. Khelifa, J. Colloid Interface Sci., 2011, 360, 716–724 CrossRef CAS PubMed.
- S. Mellouk, S. Cherifi, M. Sassi, K. Marouf-Khelifa, A. Bengueddach, J. Schott and A. Khelifa, Appl. Clay Sci., 2009, 44, 230–236 CrossRef CAS.
- J. Matusik and A. Wścisło, Appl. Clay Sci., 2014, 100, 50–59 CrossRef CAS.
- R. Li, Z. Hu, S. Zhang, Z. Li and X. Chang, Int. J. Environ. Anal. Chem., 2013, 93, 767–779 CrossRef CAS.
- R. Li, Q. He, Z. Hu, S. Zhang, L. Zhang and X. Chang, Anal. Chim. Acta, 2012, 713, 136–144 CrossRef CAS PubMed.
- Q. He, D. Yang, X. Deng, Q. Wu, R. Li, Y. Zhai and L. Zhang, Water Res., 2013, 47, 3976–3983 CrossRef CAS PubMed.
- N. Ballav, H. J. Choi, S. B. Mishra and A. Maity, Appl. Clay Sci., 2014, 102, 60–70 CrossRef CAS.
- J. Wang, X. Zhang, B. Zhang, Y. Zhao, R. Zhai, J. Liu and R. Chen, Desalination, 2010, 259, 22–28 CrossRef CAS.
- P. Luo, J.-S. Zhang, B. Zhang, J.-H. Wang, Y.-F. Zhao and J.-D. Liu, Ind. Eng. Chem. Res., 2011, 50, 10246–10252 CrossRef CAS.
- Y. Wang, X. Zhang, Q. Wang, B. Zhang and J. Liu, Water Sci. Technol., 2014, 70, 192–199 CrossRef CAS PubMed.
- Y. Dong, Z. Liu and L. Chen, J. Radioanal. Nucl. Chem., 2012, 292, 435–443 CrossRef CAS.
- J. Li, F. Wen, L. Pan, Z. Liu and Y. Dong, J. Radioanal. Nucl. Chem., 2013, 295, 431–438 CrossRef CAS.
- G. Kiani, Appl. Clay Sci., 2014, 90, 159–164 CrossRef CAS.
- X. S. Wang, Y. Zhou, Y. Jiang and C. Sun, J. Hazard. Mater., 2008, 157, 374–385 CrossRef CAS PubMed.
- R. O. Cristóvão, A. P. Tavares, L. A. Ferreira, J. M. Loureiro, R. A. Boaventura and E. A. Macedo, Bioresour. Technol., 2009, 100, 1094–1099 CrossRef PubMed.
- V. S. Mane, I. D. Mall and V. C. Srivastava, Dyes Pigm., 2007, 73, 269–278 CrossRef CAS.
- M. Zhao and P. Liu, Microporous Mesoporous Mater., 2008, 112, 419–424 CrossRef CAS.
- R. Liu, B. Zhang, D. Mei, H. Zhang and J. Liu, Desalination, 2011, 268, 111–116 CrossRef CAS.
- G. Kiani, M. Dostali, A. Rostami and A. R. Khataee, Appl. Clay Sci., 2011, 54, 34–39 CAS.
- Y. Zhao, E. Abdullayev, A. Vasiliev and Y. Lvov, J. Colloid Interface Sci., 2013, 406, 121–129 CrossRef CAS PubMed.
- H. Chen, J. Zhao, J. Wu and H. Yan, RSC Adv., 2014, 4, 15389–15393 RSC.
- P. Luo, Y. Zhao, B. Zhang, J. Liu, Y. Yang and J. Liu, Water Res., 2010, 44, 1489–1497 CrossRef CAS PubMed.
- P. Luo, B. Zhang, Y. Zhao, J. Wang, H. Zhang and J. Liu, Korean J. Chem. Eng., 2011, 28, 800–807 CrossRef CAS.
- Y. Xie, D. Qian, D. Wu and X. Ma, Chem. Eng. J., 2011, 168, 959–963 CrossRef CAS.
- J. Duan, R. Liu, T. Chen, B. Zhang and J. Liu, Desalination, 2012, 293, 46–52 CrossRef CAS.
- L. Jiang, C. Zhang, J. Wei, W. Tjiu, J. Pan, Y. Chen and T. Liu, Chem. Res. Chin. Univ., 2014, 30, 971–977 CrossRef CAS.
- Q. Peng, M. Liu, J. Zheng and C. Zhou, Microporous Mesoporous Mater., 2015, 201, 190–201 CrossRef CAS.
- L. Liu, Y. Wan, Y. Xie, R. Zhai, B. Zhang and J. Liu, Chem. Eng. J., 2012, 187, 210–216 CrossRef CAS.
- Y. Liu, X. Jiang, B. Li, X. Zhang, T. Liu, X. Yan, J. Ding, Q. Cai and J. Zhang, J. Mater. Chem. A, 2014, 2, 4264–4269 CAS.
- J. Zhu, Y. Wang, J. Liu and Y. Zhang, Ind. Eng. Chem. Res., 2014, 53, 13711–13717 CrossRef CAS.
- Y. Du and P. Zheng, Korean J. Chem. Eng., 2014, 31, 2051–2056 CrossRef CAS.
- X. Lu, X. Chuan, A. Wang and F. Kang, Acta Geol. Sin., 2006, 80, 278–284 Search PubMed.
- L. Jiang, Y. Huang and T. Liu, J. Colloid Interface Sci., 2015, 439, 62–68 CrossRef CAS PubMed.
- Y. Zhang, J. Ouyang and H. Yang, Appl. Clay Sci., 2014, 95, 252–259 CrossRef CAS.
- M. Zou, M. Du, H. Zhu, C. Xu and Y. Fu, J. Phys. D: Appl. Phys., 2012, 45, 325302 CrossRef.
- X. Li, C. Yao, X. Lu, Z. Hu, Y. Yin and C. Ni, Appl. Clay Sci., 2015, 104, 74–80 CrossRef CAS.
- J. Zhang, Y. Zhang, Y. Chen, S. Yi, B. Zhang, H. Zhang and J. Liu, Adv. Sci. Lett., 2012, 11, 57–62 CrossRef CAS.
- J. Zhang, Y. Zhang, Y. Chen, L. Du, B. Zhang, H. Zhang, J. Liu and K. Wang, Ind. Eng. Chem. Res., 2012, 51, 3081–3090 CrossRef CAS.
- Y. Chen, Y. Zhang, J. Liu, H. Zhang and K. Wang, Chem. Eng. J., 2012, 210, 298–308 CrossRef CAS.
- Y. Chen, Y. Zhang, H. Zhang, J. Liu and C. Song, Chem. Eng. J., 2013, 228, 12–20 CrossRef CAS.
- L. Duan, Q. Zhao, J. Liu and Y. Zhang, Environ. Sci.: Water Res. Technol., 2015, 1, 874–881 CAS.
- Q. Zhao, J. Hou, J. Shen, J. Liu and Y. Zhang, J. Mater. Chem. A, 2015, 3, 18696–18705 CAS.
- Z. Wang, H. Wang, J. Liu and Y. Zhang, Desalination, 2014, 344, 313–320 CrossRef CAS.
- H. Yu, Y. Zhang, X. Sun, J. Liu and H. Zhang, Chem. Eng. J., 2014, 237, 322–328 CrossRef CAS.
- L. Duan, W. Huang and Y. Zhang, RSC Adv., 2015, 5, 6666–6674 RSC.
- Q. Zhao, C. Liu, J. Liu and Y. Zhang, RSC Adv., 2015, 5, 38646–38653 RSC.
- J. Zhu, N. Guo, Y. Zhang, L. Yu and J. Liu, J. Membr. Sci., 2014, 465, 91–99 CrossRef CAS.
- L. Yu, Y. Zhang, H. Zhang and J. Liu, Desalination, 2015, 359, 176–185 CrossRef CAS.
- Y. Wang, J. Zhu, G. Dong, Y. Zhang, N. Guo and J. Liu, Sep. Purif. Technol., 2015, 150, 243–251 CrossRef CAS.
- M. Ghanbari, D. Emadzadeh, W. Lau, S. Lai, T. Matsuura and A. Ismail, Desalination, 2015, 358, 33–41 CrossRef CAS.
- M. Ghanbari, D. Emadzadeh, W. Lau, T. Matsuura and A. Ismail, RSC Adv., 2015, 5, 21268–21276 RSC.
- A. Moslehyani, A. Ismail, M. Othman and T. Matsuura, RSC Adv., 2015, 5, 14147–14155 RSC.
- M. Makaremi, R. T. De Silva and P. Pasbakhsh, J. Phys. Chem. C, 2015, 119, 7949–7958 CAS.
- J. Dai, X. Wei, Z. Cao, Z. Zhou, P. Yu, J. Pan, T. Zou, C. Li and Y. Yan, RSC Adv., 2014, 4, 7967–7978 RSC.
- W. Guan, X. Wang, J. Pan, J. Lei, Y. Zhou, C. Lu and Y. Yan, Adsorpt. Sci. Technol., 2012, 30, 579–592 CrossRef CAS.
- X. Yu, Z. Lu, N. Si, W. Zhou, T. Chen, X. Gao, M. Song, Y. Yan, P. Huo and C. Yan, Appl. Clay Sci., 2014, 99, 125–130 CrossRef CAS.
- W. Xing, L. Ni, X. Liu, Y. Luo, Z. Lu, Y. Yan and P. Huo, RSC Adv., 2013, 3, 26334–26342 RSC.
- W. Xing, L. Ni, X. Liu, Y. Luo, Z. Lu, Y. Yan and P. Huo, Desalin. Water Treat., 2015, 53, 794–805 CrossRef CAS.
- Y. Zhao, B. Zhang, X. Zhang, J. Wang, J. Liu and R. Chen, J. Hazard. Mater., 2010, 178, 658–664 CrossRef CAS PubMed.
- Y. Zhao, B. Zhang, X. Zhang, J. Wang, J. Liu and R. Chen, Water Sci. Technol., 2010, 62, 937–946 CrossRef CAS PubMed.
- K. Yang, X. Zhang, C. Chao, B. Zhang and J. Liu, Carbohydr. Polym., 2014, 107, 103–109 CrossRef CAS PubMed.
- Y. Zhao, B. Zhang, Y. Zhang, J. Wang, J. Liu and R. Chen, Sep. Sci. Technol., 2010, 45, 1066–1075 CrossRef CAS.
- S. Zhong, C. Zhou, X. Zhang, H. Zhou, H. Li, X. Zhu and Y. Wang, J. Hazard. Mater., 2014, 276, 58–65 CrossRef CAS PubMed.
- B. Szczepanik, P. Słomkiewicz, M. Garnuszek and K. Czech, Appl. Clay Sci., 2014, 101, 260–264 CrossRef CAS.
- R. Zhai, B. Zhang, Y. Wan, C. Li, J. Wang and J. Liu, Chem. Eng. J., 2013, 214, 304–309 CrossRef CAS.
- D. Bielska, A. Karewicz, T. Lachowicz, K. Berent, K. Szczubiałka and M. Nowakowska, Chem. Eng. J., 2015, 262, 125–132 CrossRef CAS.
- M. Viseras, C. Aguzzi, P. Cerezo, C. Viseras and C. Valenzuela, Microporous Mesoporous Mater., 2008, 108, 112–116 CrossRef CAS.
|
This journal is © The Royal Society of Chemistry 2016 |
Click here to see how this site uses Cookies. View our privacy policy here.