DOI:
10.1039/D3QM00730H
(Review Article)
Mater. Chem. Front., 2024,
8, 41-81
Critical challenges and opportunities for the commercialization of alkaline electrolysis: high current density, stability, and safety
Received
30th June 2023
, Accepted 15th September 2023
First published on 23rd September 2023
Abstract
Alkaline electrolysis technology, which enables the production of “green hydrogen,” holds significant importance in the global pursuit of carbon neutrality. The successful implementation and widespread adoption of this technology rely heavily on the development of efficient and durable electrocatalysts capable of operating at high current densities and ensuring safety. In recent years, there has been remarkable progress in research endeavors aimed at meeting industrial requirements, resulting in the design and synthesis of various catalysts tailored for current densities exceeding 200 mA cm−2 and possessing excellent stability. This review highlights recent advancements in the field, focusing on key factors influencing the catalytic performance in high-current-density electrocatalysis, including charge transfer, mass transport, and intrinsic activity. The mechanisms underlying catalytic degradation are discussed, and design strategies for creating highly stable electrocatalysts are presented. Furthermore, strategies for ensuring the safety of alkaline electrolysis are discussed. Finally, future directions and perspectives in this emerging field are provided, outlining potential areas of exploration and development.
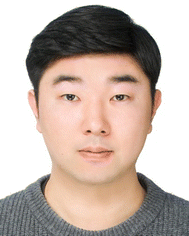
Jiseok Kwon
| Jiseok Kwon received his PhD degree from the Department of Energy Engineering at Hanyang University, Korea, in 2023. Starting from 2023, he is currently serving as a postdoctoral fellow at Hanyang University. His current research focuses on synthesis of nanomaterials for electrocatalysis, surface chemistry, and interfacial engineering. |
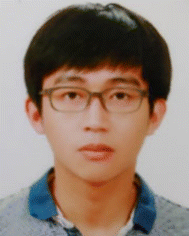
Seunggun Choi
| Seunggun Choi earned a BS degree from the Department of Energy Engineering at Hanyang University, Korea in 2018. His research interest is focused on electronic interactions between different materials and phases on electrocatalysts like electrodes for photo/electrochemical water splitting and CO2 reduction. |
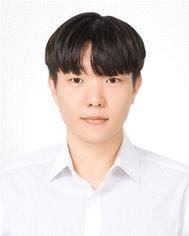
Chanjin Park
| Chanjin Park earned a BS degree from the Department of Energy Engineering at Hanyang University, Korea in 2023. His research interest is focused on electronic interactions between different materials and phases on electrocatalysts like electrodes for photo/electrochemical water splitting and CO2 reduction. |
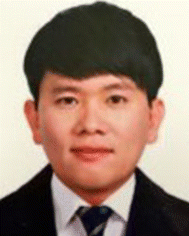
Hyuksu Han
| HyukSu Han received his PhD from the Department of Materials Science and Engineering at the University of Florida (USA) in 2014. Then he joined the University of Toulouse (France) as a postdoctoral fellow and worked in the field of nanomaterial synthesis. Currently, he is an assistant professor at Konkuk University. His research interest is mainly focused on the synthesis and characterization of nanostructured materials for catalysis. |
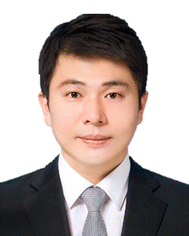
Taeseup Song
| Taeseup Song received his PhD degree from the Department of Materials Science and Engineering at Hanyang University, Korea, in 2012. He is currently an associate professor of the Department of Energy Engineering at Hanyang University. His research interest is mainly focused on the synthesis of nanostructured materials for energy device applications. |
1. Introduction
Net-zero has gained widespread attention as part of global efforts to address the problem of environment and climate change.1,2 Net-zero indicates that the total amount of greenhouse gas (GHG) emissions is equal to the amount of emissions removed, either by carbon capture or storage. Energy transition, which refers to changing the energy supply system from an unsustainable method based on fossil fuels and nuclear to a sustainable method using renewable energy, plays a critical role in achieving net-zero.3,4 However, renewable energy sources such as wind and solar are intermittent and can fluctuate depending on weather conditions.5 Therefore, “green hydrogen”, integrated with a renewable energy electricity system, is considered a key element in the transition to a sustainable energy system.6 Green hydrogen refers to hydrogen gas that is produced using renewable energy sources, such as solar or wind power, through a process called electrolysis.7 Green hydrogen has many potential applications, such as fuel for transportation, energy storage, and industrial processes, promoting the use of renewable energy in multiple sectors and contributing to a more sustainable and decarbonized energy system. Nevertheless, the production of green hydrogen through electrolysis is currently more expensive than traditional methods, such as steam methane reforming due to the low efficiency of the electrolysis system. Therefore, research and development efforts are focused on finding ways to increase the efficiency of the electrolysis system and thus reduce the cost of green hydrogen production and increase its availability.
Alkaline water electrolysis has undergone significant development and improvement over several decades, making it a well-established technology for commercial and industrial applications.8,9 In alkaline electrolyzers, transition metal-based catalysts, particularly nickel (Ni) catalysts, loaded on a current collector are commonly used as working electrodes instead of noble metals.10 Ni-based catalysts exhibit high stability in alkaline environments, contributing to the longevity of the system, which can exceed 20 years. However, alkaline electrolyzers typically operate at a cell voltage near 2 V and current densities of about 300 mA cm−2, resulting in a voltage efficiency of approximately 70%.11 In comparison, proton exchange membrane (PEM) electrolysis allows for current densities over 1–2 A cm−2, making it a more desirable option.12,13 The limited current density of alkaline electrolysis is mainly attributed to increased ohmic loss, heat generation according to Joule's law, and overall system inefficiency.14 Therefore, One of the foremost challenges of alkaline water electrolysis is the realization of high current density (HCD) over 1 A cm−2 in alkaline. To this end, it is necessary to reduce the resistance of the alkaline water electrolysis system and improve the efficiency to reduce ohmic loss and heat generation.
The typical resistance of an alkaline electrolyzer comprises electrical resistance (Relec), transport-related resistance (Rtrans), and electrochemical polarization resistance (Rp).15,16Relec is determined by Ohm's law and is influenced by electrode materials, electrolyte, and the electrical circuit (both internal and external). It is closely related to the charge transfer capability of the electrocatalysts and conductivity of electrolyte, including charge transfer between electrocatlayst–electrolyte and electrocatalyst–support (substrate). At high current density (HCD) conditions, strong polarization potential accelerates multi-electron catalytic reactions, leading to rapid electron consumption. In this scenario, electron transfer becomes the rate-determining step, especially for semiconductor materials that may experience an insufficient electron supply from the catalyst body to the active sites. This asymmetry in electron transfer and consumption contributes to an increase in Relec.
R
trans represents the physical resistance in the system, encompassing concentration polarization resistance (Rc), bubble resistance (Rbubble), and diaphragm resistance (Rdiaphragm).17,18Rc arises from the mass transport limitation of reactants and the concentration gradient of hydroxide ions (OH−) neat the surface of the catalyst. Under HCD conditions, fast reactant consumption and gas generation cause local ion depletion on the catalyst surface and create large concentration gradients, leading to an increase in resistance. Rbubble results from the formation of gas bubbles on the electrode surface, reducing the active electrode area. The presence of gas bubbles can impede the efficient flow of current and affect the overall performance of the electrolysis system. The bubbles can create a physical barrier on the electrode surface, hindering the contact between the electrode and the electrolyte. This leads to increased resistance and can result in voltage losses. This phenomenon is difficult to avoid in liquid electrolytes under high current density due to vigorous gas generation. Rdiaphragm indicates the resistivity and permeability of the diaphragm material.
R
p characterizes the polarization resistance encountered during electrochemical reactions, specifically pertaining to the hydrogen evolution reaction (HER) and oxygen evolution reaction (OER). Rp is associated with the overpotentials required to surpass the activation energy barrier for the HER and OER processes. The overpotentials for these reactions are primarily dictated by the intrinsic activity of the catalysts involved, which ultimately governs the kinetics of the electrochemical reactions. The intrinsic activity of the catalyst for HER and OER depends on its surface electronic structure and the adsorption/desorption behavior of reaction intermediates.19 High intrinsic catalytic activity is necessary to reduce overpotential under HCD conditions. However, the catalytic performance is sensitive to the local reaction environment, which is affected by the current density. For instance, under HCD condition, the increase in hydrogen coverage on the catalyst surface can impact the adsorption/desorption process of reaction intermediates and the intrinsic activity of the catalyst. The increase in hydrogen coverage (θH) can hinder the adsorption of hydrogen on the Pt surface, resulting in a significant increase in the free energy of hydrogen adsorption (ΔGH) when θH exceeds 1.20 This steric effect leads to decreased activity of Pt-based electrocatalysts, such as the commercially available benchmark Pt/C, under HCD conditions. Comparatively, α-MoB2 exhibits higher electrocatalytic activity for HER than Pt/C at current densities above 250 mA cm−2, requiring a much lower overpotential to achieve a current density of 1000 mA cm−2.21 The favorable performance of α-MoB2 can be attributed to its near-zero ΔGH at high hydrogen coverage, whereas Pt's surface struggles to adsorb additional hydrogen. To enable HCD operation of alkaline electrolyzers in industrial applications, it is essential to develop strategies that minimize the resistances described above. These strategies must aim to reduce ohmic loss, enhance the energy efficiency of water electrolysis, and improve overall system performance even under high current density conditions. Central to these efforts is the development of available and high-performance electrocatalysts, as they play a critical role in reducing ohmic losses and improving the efficiency and performance of alkaline electrolyzers, even at HCDs.
The stability of electrocatalysts plays a crucial role in determining their suitability for use in water electrolyzers for the HER and OER. However, compared to the optimization of catalytic performance, the exploration of stability and its underlying mechanisms has received relatively limited attention. Both chemical and mechanical stability are important factors in determining the overall stability of electrocatalysts, and their stability can be influenced by the current density. From a mechanical stability perspective, under HCD conditions, the vigorous evolution of gas occurs. As gas molecules are formed, adjacent bubbles tend to approach each other and merge into larger entities, reducing the system's surface energy. These bubbles adhere firmly to the catalyst's surface and gradually increase in size until they reach a critical point where they detach from the catalyst material. During this detachment process, the strong interfacial adhesion force between the electrocatalyst and the bubbles can lead to the peeling off of certain parts of the catalyst, resulting in a deterioration of its mechanical stability. For instance, Chen yang group observed that the RANEY® nickel electrode was peeled off from the electrode surface and entered the electrolyte solution during the HER process at a current density of 5 A cm−2.22 This peeling-off issue becomes more severe as the current density increases unless the interaction force between the catalyst and the support is stronger than the interfacial adhesion force between the catalyst and the bubbles. Chemical dissolution is another significant cause of catalytic degradation, particularly in the harsh conditions of a strong alkaline environment. During the catalytic process, electrocatalysts can undergo surface reconstruction, transitioning into reductive species during HER or amorphous metal oxyhydroxides during OER. This surface reconstruction process often leads to a high dissolution rate of the electrocatalyst components, resulting in catalytic instability known as “transient dissolution.” In contrast, the dissolution process of stable-phase catalysts is referred to as “steady-state dissolution.” Wang et al. found that the presence of a polysulfide structure (Sn2−) was responsible for excellent catalytic activity.23 However, this S-rich cobalt polysulfide showed continuous degradation of activity due to the loss of Sn2− during the HER process. Under HCD conditions, this dissolution process is accelerated, leading to the abrupt degradation of the catalytic electrode and increasing the replacement cost for electrolyzers. The understanding of stability mechanisms and the development of strategies to enhance the stability of electrocatalysts under HCD conditions are critical for improving the durability and cost-effectiveness of electrolyzers used in hydrogen and oxygen generation.
Safety concerns in alkaline water electrolyzer arise due to gas crossover, which is phenomenon of the unintended transport of gases across diaphragm in an electrolysis cell.24,25 Gas crossover involves the migration of hydrogen gas from anode to cathode and oxygen gas from cathode to anode, leading to the mixing of hydrogen and oxygen. Hydrogen gas is highly flammable and can form explosive mixtures with oxygen in certain concentrations, and thus gas crossover creates an explosive atmosphere within the electrolyzer or its surroundings.26,27 Ignition sources such as sparks or flames can trigger potentially hazardous explosions. To mitigate this risk, preventive measures must be implemented to minimize gas crossover and prevent the formation of explosive mixtures. To improve the safety of alkaline water electrolysis, researchers are developing technologies at the balance of plants (BOP) stage.28 These technologies aim to enhance safety by incorporating oxygen removal devices in the hydrogen stream. However, despite these advancements, the risk of explosion within the electrolytic cell remains due to gas mixing. Therefore, additional technologies are needed to remove oxygen from hydrogen and hydrogen from oxygen, ensuring a higher level of safety. Our group proposed a safety improvement technology for water electrolysis by integrating an oxygen reduction reaction (ORR) catalyst in the cathode electrode.29 This ORR catalyst serves to remove oxygen from the system, thereby increasing the purity of hydrogen within the electrolytic cell. By implementing such technologies, safety in water electrolysis can be enhanced, reducing the potential for gas crossover-related explosions and ensuring the production of high-quality hydrogen.
The aforementioned challenges encountered in alkaline water electrolysis are summarized in Fig. 1. Addressing these challenges necessitates a systematic approach to developing electrocatalysts that possess high efficiency, remarkable stability, and multi-functionality. Over the past few decades, significant advancements have been made in the field of electrocatalyst, particularly in exploring active sites and optimizing the adsorption/desorption process of reaction intermediates, which predominantly focus on the intrinsic activity of electrocatalysts.30–34 However, these investigations are typically carried out under laboratory conditions characterized by relatively low current densities ranging from 1 to 100 mA cm−2. This discrepancy highlights a substantial gap between current electrocatalyst studies, which predominantly emphasize low current density conditions, and the practical applications that require HCD. Consequently, there is a growing demand for research on electrocatalysts that are closely linked to practical applications in the field of water splitting. Accordingly, diverse research strategies for alkaline electrolyzer are being investigated to address the challenges associated with HCD, high stability, and safety concerns (Table 1). In view of recent advancements, this review article primarily focuses on high-performance electrodes with high current density (HCD), exceptional stability, and safety considerations, which are critical for the practical commercialization and widespread adoption of alkaline electrolyzers. The key emphasis of this review is to summarize and illustrate state-of-the-art design strategies that enable HCD, specifically addressing charge transfer, mass transfer, and intrinsic activity. Moreover, the review briefly delves into the mechanisms of mechanical and chemical degradation of electrocatalysts. And, we highlight representative studies on strengthening mechanical stability by engineering interactions between the electrocatalyst–substrate and electrocatalyst–bubble interfaces, as well as the manipulation of surface reconstruction to enhance chemical stability under harsh conditions (Fig. 1). Additionally, advanced electrolysis systems such as sea water electrolysis and anion exchange membrane (AEM) water electrolysis are discussed to showcase their significance. Finally, the review concludes with an outlook on the future development of electrocatalysts for alkaline electrolyzers.
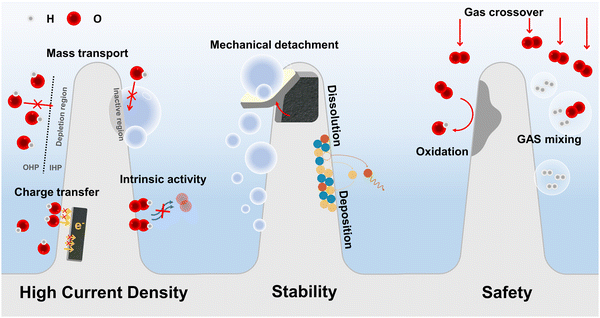 |
| Fig. 1 Schematic illustration of the main challenges encountered in alkaline water electrolysis. | |
Table 1 Design strategies of electrocatalysts for addressing challenges encountered in water electrolysis
2. Strategies to achieve high current densities
Urgent challenge encountered in alkaline water electrolysis is an achievement of HCD, which could be addressed by the design of a catalyst electrode capable of minimizing ohmic loss. Strategies for designing catalytic electrodes that can mitigate each resistance component involve considerations of charge transfer, mass transport, and intrinsic activity. Intrinsic activity is an indicator of the catalytic activity associated with optimizing electronic structure for reducing reaction barriers and is a prerequisite for enabling HCD operation. Catalyst design approaches aimed at enhancing intrinsic catalytic properties encompass various aspects such as surface chemistry, phase engineering, and heterointerface engineering. The studies that realized high current density with low overvoltage are summarized in Table 2. These design principles contribute to the development of catalyst electrodes capable of reducing ohmic losses and facilitating the realization of high current density in alkaline water electrolysis.
Table 2 Summary of electrocatalytic performance of recently reported electrocatalysts under high current densities
|
Materials |
Substrate |
Electrolyte |
HER-Overpotential@current density (mV@mA cm−2) |
OER-Overpotential@current density (mV@mA cm−2) |
Overall voltage@current density (V@mA cm−2) |
Ref. |
HER |
Ni5P4–Co2P |
Ni–Co foam |
1 M KOH |
267@1000 |
|
|
208
|
NC/Ni3Mo3N |
Ni foam |
1 M KOH |
954@1500 |
|
|
209
|
Se–Ps |
Ni foam |
1 M KOH |
261@400 |
|
|
210
|
1T-MoS2 QS/Ni(OH)2 |
|
1 M KOH |
185@500 |
|
|
211
|
Ce0.2–CoP/Ni3P |
Ni foam |
1 M KOH |
225@1000 |
|
|
212
|
Ni2P/NiTe2 |
Ni foam |
1 M KOH |
143@100 |
|
|
213
|
F, P-Fe3O4 |
Fe foam |
1 M KOH |
350@1500 |
|
|
214
|
NiCoP/NiCoSx |
Ni foam |
1 M KOH |
222@500 |
|
|
215
|
h-NiMoFe |
Ni foam |
1 M KOH |
97@1000 |
|
|
216
|
|
OER |
NiFe-60/Co3O4 |
Ni foam |
1 M KOH |
|
257@500 |
|
217
|
cMOF/LDH |
Carbon Cloth |
1 M KOH |
|
287@300 |
|
218
|
KT-Ni(0)@Ni-TPA |
Ni foam |
1 M KOH |
|
380@1500 |
|
219
|
(Ni,Fe)OOH |
Ni foam |
1 M KOH |
|
289@1000 |
|
81
|
Vertical graphene sheets/MoS2/ FeCoNi(OH)x |
Carbon fiber |
1 M KOH |
|
241@1000 |
|
220
|
3D Fe2O3@Ni2P/Ni(PO3)2 |
Ni foam |
1 M KOH |
|
340@1000 |
|
221
|
370@1000 |
|
Bifunctional |
IrNi–FeNi3 |
Ni foam |
1 M KOH |
289@1000 |
330@1000 |
1.47@10 |
222
|
np-NiCoFeMoMn |
|
1 M KOH |
150@1000 |
350@1000 |
1.47@10 |
223
|
Co4N–CeO2 |
Graphite plane |
1 M KOH |
190@500 |
390@500 |
1.51@10 |
224
|
MoNi4/SSW |
Stainless steel |
5 M KOH |
161@1000 |
233@1000 |
1.98@500 |
225
|
Ni/MoN |
Ni sheet |
1 M KOH |
514@500 |
533@200 |
2.4@300 |
226
|
Ni–P–B |
paper |
1 M KOH |
276@500 |
375@500 |
1.8@200 |
227
|
K2Fe4O7 |
Ni foam |
1 M KOH |
343@2000 |
421@2000 |
2.01@1500 |
228
|
Ni-CST, NiFeCo-CST |
Silk textile |
1 M KOH |
68@50 |
196@100 |
1.7@2000 |
229
|
NiCo@C–NiCoMoO |
Ni foam |
1 M KOH |
266@1000 |
390@1000 |
2.01@1000 |
230
|
NiMoN/NiFe LDH |
Ni foam |
1 M KOH |
205@1000 |
266@1000 |
1.77@1000 |
80
|
FeCoNiCuMn/CNF |
|
1 M KOH |
281@100 |
386@200 |
|
231
|
2.1 Improving charge transfer
The electrocatalytic process for water electrolysis is a multielectron transfer reaction.35 The electron transfer process involves the chemical bond formation/rupture, and charge accumulation/consumption, fast electron transport to support.36,37 Therefore, fast charge transfer and enough current-carrying capability are the guarantee of the subsequent multi-electron catalytic reactions and enhanced catalytic performance at HCD. The intrinsic electrical conductivity of electrocatalysts is closely linked to their ability to facilitate charge transfer. An electrocatalyst with high electrical conductivity is capable of supporting rapid electron transfer and can provide sufficient current-carrying capacity, both of which are crucial for efficient multi-electron catalytic reactions. When high current densities are applied, the strong polarization potential accelerates multi-electron catalytic reactions, leading to the rapid consumption of electrons. However, non-noble electrocatalysts for HER and OER show typical semiconductor properties with low electrical conductivity. Doping and vacancy engineering have been widely utilized to increase the charge carrier density and enhance the charge mobility, thus improving the intrinsic electrical conductivity of the catalyst. For example, CoO is a semiconductor in which the adjacent Co2+ ions are spin anti-parallel configuration, following the super exchange interaction.38,39 In the carrier transport process, the transfer of a hole between two adjacent Co ions necessitates spin flipping and spin rearrangement, requiring additional energy and leading to a reduction in carrier mobility. Therefore, Li et al. introduced the guest ion of V5+, which has no occupation of d orbital.40 Introduction of V5+ formed the local configuration of Co2+–O–V5+, enabling the double exchange interaction without spin-flip to increase carrier mobility (Fig. 2a). As-prepared CoV oxide catalyst exhibited 3.14 times higher conductivity compared to Co oxide (Fig. 2b). As shown in Fig. 2c, the CoV oxide with V Bridged Co–O possesses admirable HER and OER activity with an overpotential of 205 and 267 mV to drive 100 mA cm−2 current density, respectively. In a similar vein, layered double hydroxides (LDHs) have gained recognition as highly active catalysts for oxygen evolution reaction (OER), demonstrating a low overpotential and high electrolysis current.41–43 Despite these desirable characteristics, LDHs exhibit poor electrical conductivity, which has hindered their practical application and further advancement.44 NiFe LDH (layered double hydroxides) is a type of material that can exhibit semiconductor properties under certain conditions.45,46 LDHs are typically insulating materials, but their conductivity can be increased by introducing dopants or vacancies. In order to enhance the intrinsic conductivity of NiFe layered double hydroxides (LDHs), vanadium doping was introduced into the layered structure with a range of valence states (V3+, V4+, and V5+) in Fig. 2d.47 V doping was found to modulate the electronic structure of the pristine NiFe LDH and increase its electrical conductivity. The bandgap between the valence and conduction bands of NiFe LDH was measured to be approximately 1.56 eV (Fig. 2e). V doping predominantly altered the conduction band state of the NiFeV LDHs, leading to the narrowing of the bandgap and improving the electric conductivity of the active material. The sheet resistance of NiFe LDHs was measured to be 2.4 × 103 Ω sq−1, whereas that of NiFeV LDHs was found to be 1.3 × 103 Ω sq−1, providing direct evidence of the higher conductivity of NiFeV LDHs. Fig. 2f shows that this highly conducting NiFeV LDH requires the overpotential of 233 mV at 100 mA cm−2, which is smaller than that of NiFe LDH (280 mV). In addition, oxygen vacancy (VO) in LDH generates gap state in band structure, thereby modulating the electronic structure and enhancing the conductivity.48,49 VO-enriched NiFe LDH shows the smaller band gap of 0.17 eV compared to that of NiFe LDH, proving that introduction of VO can reduce the bandgap of NiFe LDH, and thus improving its conductivity.50 Furthermore, the d-band center is downshifted from −2.23 to −3.1 eV after increasing the concentration of VO. Tailored electronic structure weaken the adsorption energy of oxygen intermediate, exhibiting an overpotential of 255 mV at 100 mA cm−2. SrTi0.7Ru0.3O3−δ (STRO) with increased VO was reported by the research group led by Zongping Shao. This catalyst was synthesized using an unusual super-exchange interaction between adjacent Ti(III) and Ru(V) ions. The investigation of the Ti and Ru valence states within STRO revealed a charge redistribution phenomenon (Ti4+ + Ru4+ → Ti3+ + Ru5+), as depicted in Fig. 2g. Additionally, the substitution of Ti with Ru resulted in an increase in the number of oxygen vacancies, in accordance with charge neutrality (Fig. 2h). Consequently, the electrical conductivity of STRO, as shown in Fig. 2i, was enhanced (∼0.2 S cm−1) compared to that of STO (∼0 S cm−1). Density of states (DOS) profiles in Fig. 2j demonstrates that pristine STO behaves as a semiconductor with a significant bandgap. However, upon Ru doping, STRO exhibits metallic characteristics, primarily attributed to the Ru 4d orbital crossing the Fermi level, which accounts for the observed increase in conductivity.51
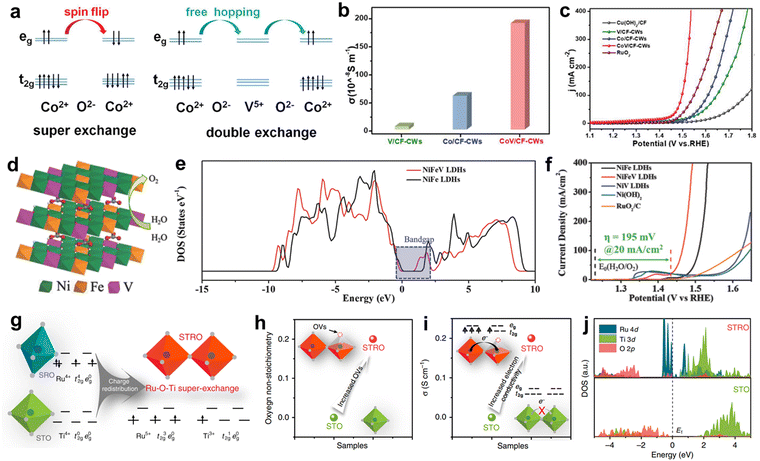 |
| Fig. 2 (a) Carrier hopping with spin-flip between adjacent Co2+ in CoO and free hopping between V bridged Co–O structure in CoV2O6. (b) The electrical conductivity of the electrocatalysts. (c) Corresponding polarization curves for OER. Reproduced with permission.40 Copyright 2020, Wiley. (d) The schematic diagram of NiFeV LDHs structure. (e) Total density of states (TDOS) curves of NiFeV LDHs and NiFe LDHs. (f) Polarization curves of NiFe and NiFe V LDH. Reproduced with permission.47 Copyright 2018, Wiley. (g) Schematic illustration of the super-exchange interaction in STRO. (h) The oxygen non-stoichiometry of STO and STRO. (i) The electronic conductivity of STO and STRO at room temperature. (j) Polarization curves of STO, STRO, SRO, and commercial Pt/C catalysts in 1 M KOH solution. Reproduced with permission.51 Copyright 2020, Springer Nature. | |
Another strategy to reduce charge transfer limitations is facilitating charge transfer between the substrates and active centers via hybridizing electrocatalysts with conductive materials, especially carbon-based materials. For example, reduced graphene oxide (rGO) is widely used as a conducting agent for the HER and OER due to its excellent electrical conductivity, high surface area, and chemical stability.52–55 Heteroatom dopant engineering on rGO could tune the electrical conductivity to overcome the charge transfer limitation of the catalyst.56–59 For example, Karmakar et al. reported the Ni3V2O8 anchored N-doped rGO.60 The presence of pyrrolic-N in carbon network is responsible for the high electrical conductivity of N-rGO, while pyridinic-N facilitates electrocatalytic activity. The introduction of N-rGO expose the more active site to electrolyte and increase the electrical conductivity, exhibiting a sufficiently high electrochemical active surface area (517.5 cm2) and remarkably low charge transfer resistance (1.6 Ω). Zhang et al. reported the synthesis of amorphous MoWSx/N-rGO hybrid material as a catalyst for electrocatalytic reactions.61 The authors optimized the conditions for preparing the catalyst material, conducting agent, and plasma treatment to achieve the desired electrocatalytic performance. The catalyst electrode prepared using the optimized conditions exhibited a low overpotential of 348 mV at a current density of 1000 mA cm−2, demonstrating the effectiveness of the amorphous MoWSx/N-rGO hybrid material as an electrocatalyst. Compared with traditional carbon-based materials, graphdiyne (GDY) shows many fascinating characteristics for electrocatalyst, including sp-/sp2-hybridized and large π-conjugated carbon with 2-dimensional network, high electrical conductivity, and stability.62–64 The unique incomplete charge transfer between GDY and metal atoms could increase the number and the stability of the active site. Benefitting from these superiorities, the catalyst hybridized with GDY has been widely studied.65–67 Li group reported the GDY-induced intercalation/exfoliation/decoration strategy of LDH catalyst (Fig. 3a).68 Due to the easy anion exchangeable characteristic in interlayers of bulk LDH material, hexaethylnylbenzene (HEB) monomer can easily react with anion, in this case CO3−. HEB enter the LDH gallery, where the subsequent polymerization of HEB occurs by forming graphdiyne (GDY). The intercalated GDY layers cause stress and deformation among LDH interlayers resulting in the exfoliation of LDH and the formation of nanoscale 2d sheets. Furthermore, GDY can provide additional active sites due to the affluent charged carbon atoms and electronic interaction between GDY and LDH layers enabling facile charge transfer from LDH to GDY layers. FeCo LDH with GDY exhibited 278 mV and 256 mV overpotential for OER and HER at current densities of 1000 mA cm−2, respectively. Chen et al. fabricated FeOOH@GDY heterostructure by in situ growth of GDY on the FeOOH nanowires, shown in Fig. 3b.69 An interface structure with electron-donor (GDY) and electron-acceptor (Fe) was formed, which showed the obvious facile charge transfer between GDY and catalyst at the interfaces. FeOOH@GDY requires overpotential of 411 mV at 100 mA cm−2 for OER and 161 mV at 1000 mA cm−2 for HER.
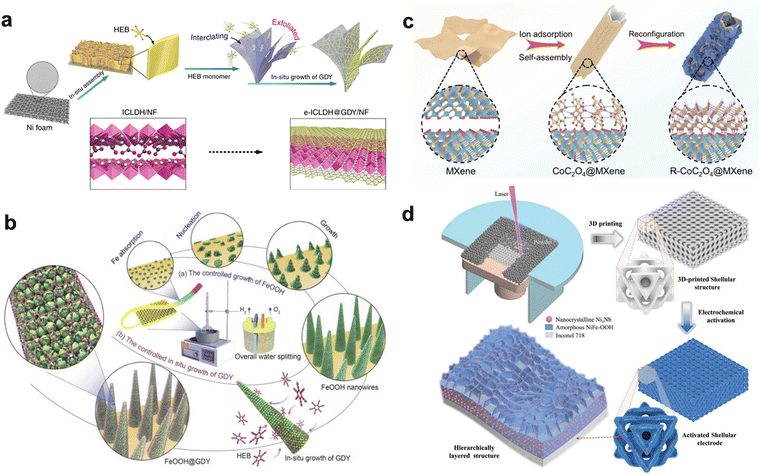 |
| Fig. 3 (a) Schematic representation of the preparation of e-ICLDH@GDY/NF structures. Reproduced with permission.68 Copyright 2020, Springer Nature. (b) Schematic representation of the controlled synthesis of FeOOH@GDY through the growth of FeOOH nanowires and the in situ growth of GDY. Reproduced with permission.69 Copyright 2023, Wiley. (c) Schematic illustration for the synthesis process of CoC2O4@MXene. Reproduced with permission.75 Copyright 2022, Springer Nature. (d) Schematic diagram of preparation of shellular electrode with amorphous NiFeOOH and embedded Ni3Nb through the 3D printing and in situ electrochemical activation process. Reproduced with permission.84 Copyright 2021, Wiley. | |
The class of two-dimensional (2D) transition metal carbides and nitrides, commonly known as MXenes, has gained significant attention in various research fields.70–72 These materials have a general chemical formula of Mn+1XnTx (n = 1–3, M is an early transition metal, X is carbon and/or nitrogen, and T represents surface functional groups such as –OH, –O, –Cl, and –F). MXenes have remarkable properties such as high electrical conductivity due to high electron density near the Fermi level, excellent hydrophilicity, and rich surface chemistry attributed to the presence of surface functional groups.73 Yu and coworkers fabricated macroporous 3D MXene frame with MXene coating on 3D Nickel foam. The NiFe LDH was electrodeposited onto this 3D MXene frame.74 Structural feature of NiFe LDH@MXene@NF is favorable to expose more edge and defect sites while shortening the charge transport pathway for electrocatalyic reactions. The presence of a natively conductive 3D MXene frame not only facilitates the fast charge transfer but also causes the electron extraction from NiFe-LDHs. The NiFe-LDH/MXene/NF enables commercially required high current density of 500 mA cm−2 at low overpotentials for both OER (300 mV) and HER (205 mV). Wang et al. reported the CoC2O4 nanorods self-assembled with MXene (Fig. 3c).75 The ultra-thin MXene with high conductivity provides an effective electronic path, which facilitates the rapid and deep self-reconfiguration process, transforming actual active species of Co(OH)2 from CoC2O4. The synergistic interaction between Co(OH)2 and MXene ensures remarkable HER activity even at a high current density. Specifically, the reconfigured CoC2O4@MXene delivers small overpotentials of 216 mV to reach current densities of 1000 mA cm−2 for HER in 1 M KOH.
The appropriate choice of a conductive substrate is crucial for optimizing the conductivity in the electrode system and reducing the ohmic loss within the electrode. Typically, a significant ohmic loss occurs between the substrate and the catalyst, which can negatively impact the catalyst's performance at HCD. Although the interface between the conductive substrate and the catalyst can be optimized for electron transfer in most metal substrates, such as metal mesh, metal foam, and carbon substrate.76 Based on this, self-supported catalysts/electrodes that use a support such as metal foam and carbon material with catalytic materials directly grown on its surface are intensively studied.32,77–79 Such intimate contact can not only guarantee rapid charge transfer but also prevent the detachment of active materials from substrates. For example, Zhai et al. reported hierarchical bimetal nitride/hydroxide (NiMoN/NiFe LDH) grown on Nickel foam, exhibiting 255 mV of overptential for generating 1000 mA cm−2.80 Zhou et al. utilized Nickel foam support and synthesized amorphous mesoporous Ni/Fe (oxy)hydroxide [(Ni, Fe)OOH] film on their surface by room-temperature.81 Directly grown (Ni, Fe)OOH yields current densities of 1000 mA cm−2 at overpotentials of only 289 mV in alkaline electrolyte. Li and coworker fabricated a hybrid heterostructure electrode based on Ni3N and NiFeP over Fe foam substrate, achieving the overpotential of 287 mV at 500 mA cm−2 in 1 M KOH.82 Besides commercial metal foam, 3D-printed metal and carbon substrates have the advantage of obtaining geometrically complex 3D structures (good flexibility), which can greatly increase the loading of catalysts and the number of catalytic active sites.83 Chang et al. obtained the Inconel 718 supports with shellular or planar structure through 3D printing technology and transformed them into highly active and robust catalytic electrodes via an in situ electrochemical activation strategy (Fig. 3d).84 The electrochemically activated shellular electrodes showed superior OER performance, achieving a high current density of 1500 mA cm−2 at a record-low overpotential of 261 mV. Peng et al. built 3D printed graphene/CNT (3DP GC) electrodes with high flexural strength and hierarchical porous structure via an extrusion-based 3D printing approach.85 3DP GC electrode integrated with a NiFeP nanosheets array exhibits showed superior OER performance than electrochemically activated planar electrodes. It is attributed that the 3DP GC electrodes offer an intrinsically high flexural strength that enables their large-scale applications and large surface area and interconnected channels, endowing fast mass and/or charge and ions transport rate.
2.2 Facilitating mass transport
Sluggish mass transfer becomes the bottleneck reaction of the whole electrochemical reaction kinetics. At HCD condition, a high-rate catalytic process rapidly consumes the reactants near the catalyst surface and generates products (H2 and O2), leading to the increase of concentration overpotential, which is caused by the of the reactants or the products in the bulk electrolyte and on the electrode surface. Due to limited mass transport, the reactant molecules cannot reach, or the product molecules cannot depart from, the reaction sites under the specified current, resulting in depletion of the reactants or accumulation of the products at the electrode surface. Accelerating the mass transport of reactant and product can be realized by enhancing the local electric field and the electrode surface wettability to facilitate the adsorption of polar reactants at the interface, and forming a gas-repellent surface by surface roughening and functionalization to enable fast gas bubble dissipation.
2.2.1 Ion transport.
OH− ions are the important reactants in the reaction steps of the water electrolysis in alkaline solution and the reaction rate is proportional to the reactants concentration.86 A higher concentration of OH− ions at the surface corresponds to a higher pH value, which, in turn, leads to a more negative change in free energy (ΔG) for each step of the OER. Local concentration of reactants can be modulated by tip-enhanced local electric field and construction of a local coordination environment to enrich the local reactant concentration in the vicinity of the catalytic sites.87–90 High-curvature structures can promote the aggregation of reactants around the surface of catalysts. It is attributed to the tip effect which refers to the aggregation of energy such as electrons, photons, and magnetism in the tip region with large curvature, resulting in the locally enhanced electric field at the sharp tip of a conductor. Liu et al. reported the NiFe nanocone array with sharp tips and optimal composition.91 The fabricated catalyst electrode, with optimized alloy composition, has a small overpotential of 190 mV at 10 mA cm−2 and 255 mV at 500 mA cm−2. To explore the tip effect and enhanced mass transfer, the NiFe nanocone catalyst with different tip coverture was prepared by annealing under different temperatures. The ECSA normalized polarization curve also shows decreased OER catalytic performance for the sample with reduced tip curvature radius, indicating that some factors related to tip curvature affect its intrinsic activity. Therefore, the role of the local electric field in enhancing reactant transfer and the alkaline OER rate has been identified by both finite element analysis (FEA) simulation and carefully tuning the apex curvature of NiFe nanocones. FEA simulation demonstrates that regions with sharp curvature promote the accumulation of positive charges (Fig. 4a). The local electric field strength in these regions is approximately three times greater than that observed on quasi-planar surfaces. Additionally, the average current density at sharp tips was found to be one order of magnitude larger than that observed on quasi-planar surfaces at a potential of 1.5 V. Fig. 4b shows that the tip enhances the local hydroxide ions (OH−) concentration at the reactive sites, and thus lowers the OER overpotential. This enhanced electric field to promote the mass transfer of reactants is also applicable to the HER catalyst. Zhang et al. designed the Ni0.2Mo0.8N/Ni hybrid electrocatalyst, which consists of Ni nanoparticles partially embedded in the Ni–Mo–N matrix on the surface.92 The tip-induced-like local electric field is enhanced, increasing the K-ion concentration in the inner Helmholtz plane (IHP) around the topmost embedded Ni nanoparticles (Fig. 4c). The surface-adsorbed K-ion can also slightly promote the HER kinetics of the Ni surface. Weakly hydrated K cations stabilize the transition state of the water dissociation step more favorably, boosting HER. And, increased concentration of hydrated cations leads to higher activity due to their stronger driving force to accumulate the reactant at the outer Helmholtz plane (OHP) and their consequently higher concentration at the interface, increasing the mass transfer (Fig. 4d). Furthermore, the embedded Ni nanoparticles tailor H adsorption strength of Ni–Mo–N matrix and provides the active sites for hydroxyl adsorption, reducing the water dissociation energy barrier. The resulting electrocatalyst shows excellent HER electrocatalytic performance with an overpotential of 70 mV at 300 mA cm−2.
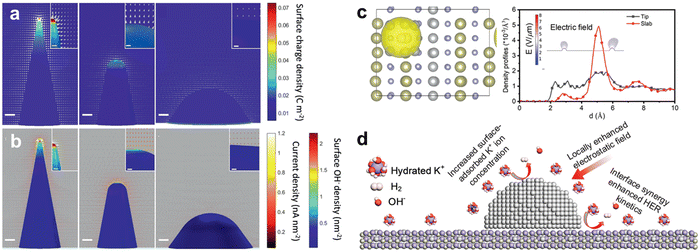 |
| Fig. 4 (a) Positive charge density distribution on the electrode surface. The arrows indicate the electrostatic field distribution around the electrode. (b) Surface OH− density distribution on the electrode surface. The arrows indicate OER current density and movement trend of the OH−. Reproduced with permission.91 Copyright 2021, Wiley. (c) The solvent-induced polarization charge and Tip-enhanced-like local electric field around the topmost Ni nanoparticles. (d) The dominant pathway with increased surface adsorbed K+ ion concentration. Reproduced with permission.92 Copyright 2021, Royal Society of Chemistry. | |
An alternative strategy to facilitate mass transport can be the introduction of additional reactant attractors around the catalysts. The reactant attractor should be conductive and has an efficient adsorption site towards OH− ions. Liu et al. reported N-doped carbon nanotubes (NCNT) as a conductive OH− concentration enhancement matrix.93 NCNT is incorporated with Fe-NiS2 catalyst to accelerate OER performance by promoting the mass transport process of OH−. OH adsorption energy (Eads) on the electropositive site of nitrogen-doped carbon (−1.48 eV) is higher than graphite carbon site (−1.22 eV), indicating the introduction of nitrogen-doping offers chemical active site facilitating the local attraction of OH−. In addition, a three-dimensional NCNT network with Fe-NiS2 induced a local electric field to accumulate the reactant near the surface of the catalysts. The zeta potential reflects the charges on the electrode surface, and therefore would provide additional information on the interaction between NCNT and OH−. Fe-NiS2/NCNT exhibits a more negative zeta potential (−25.8 mV) in 1 M KOH than Fe-NiS2 (−19.8 mV). This suggests more OH− ions are accumulated in the inner Helmholtz layer of Fe-NiS2/NCNT, which provides further evidence for the local OH− enrichment effect due to the presence of NCNT. Owing to the OH− enriched effect of NCNT, Fe-NiS2/NCNT shows 247 and 281 mV to reach the current densities of 100 and 200 mA cm−2, respectively, compared to that of Fe-NiS2 (330 and 392 mV).
2.2.2 Bubble transport.
The water electrolysis occurs at the liquid/solid interface, leading to the continuous formation of triple-phase boundaries (i.e., gas–liquid–solid interfaces) due to the evolution of gaseous H2 and O2.94,95 The aggregation of evolved gas bubble and attachment at the solid present a significant challenge in forming the catalyst/reactants interface, resulting in a reduced availability of active sites, hindered the mass transfer of the liquid phase reactant, and retarded charge transfer leading to inferior electrocatalytic activity and stability. The aggregation and attachment of evolved gas bubbles at the solid interface pose a notable obstacle in the establishment of an effective interface between the catalyst and reactants. This phenomenon has detrimental effects, including a diminished availability of active sites, impeded mass transport of the reactant, and slowed charge transfer processes. This challenge becomes even more pronounced in industrial-scale operations due to the vigorous gas bubbles, resulting that the mass transport is rate-limiting under the HCD.96,97 In this regard, bubble detachment from the catalyst surface has emerged as a novel research hotspot with great potential to pave the way for industrial-scale electrolysis. Qunlei Wen et al. have provided three design principles for engineering gas–liquid–solid interfaces through the following arguments.98 Considering a single bubble formed on the catalyst surface, ignoring the forces of external factors like electrolyte disturbance, the forces affecting the bubble are: the adhesion force (Fa), buoyant force (Fb), and gravity (G) (Fig. 5a). If we assume that the bubble is spherical, the following equation is established; | Fa = Fb − G = γ2π(R sin θb)sin θb | (1) |
| 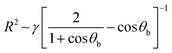 | (2) |
where γ is the surface tension of the catalyst, R is the bubble radius, θb is the bubble contact angle at the catalyst surface. From eqn (2), three design principles for engineering gas–liquid–solid interfaces are presented: (i) by regulating wettability with θb and γ, the bubble adhesion force and bubble size can be controlled. (ii) Discontinuous triple phase contact line (TPCL) greatly reduces the bubble adhesion force and enables the formation of small bubbles (Fig. 5b). Based on this, it is concluded that the wetting state of the bubble is a crucial indicator for evaluating the bubble adhesion force and size. Therefore, for facilitating bubble transport, the catalyst surface needs to be hydrophilic and aerophobic at the same time (Fig. 5c). According to Jingming Wang et al., the following relationship is established;where θb is the bubble contact angle at the catalyst surface and θw is the contact angle of water.99 This equation indicates that hydrophilicity and hydrophobicity are mutually indicative relationships with aerophobicity and aerophilicity, respectively. Evelyn N. Wang's group investigated the effect of hydrophilicity on electrolysis using PTFE deposition with different coverage (θPTFE = 0, 0.16, 0.55, 0.76) onto nickel foam.100 As θPTFE increased, the bubble size sharply increased. When θPTFE was 0, the bubble diameter was ∼200–700 μm, but when θPTFE increased to 0.76, the bubble size expanded up to about 4.5 mm (Fig. 5d). Mass transport overpotential (ηtrans) varied significantly depending on θPTFE. The lowest ηtrans at 400 mA cm−2 was observed when θPTFE was 0 (104 mV), whereas it increased more than three times when θPTFE increased to 0.76 (311 mV). It can be concluded that hydrophilicity plays a crucial role in facile bubble transport in the electrolysis process.
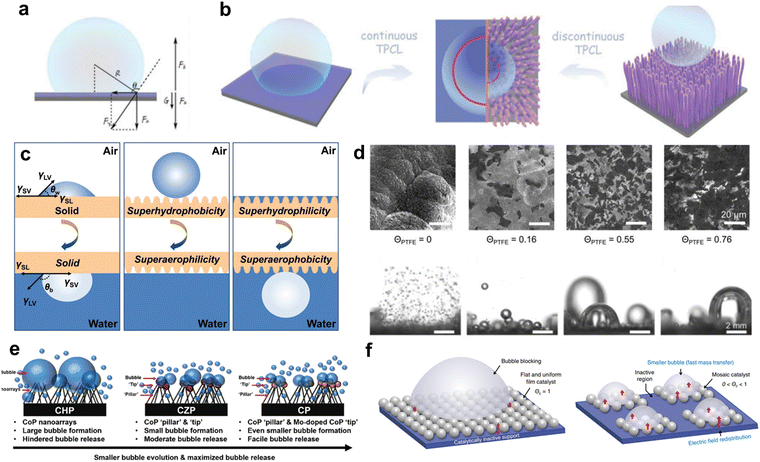 |
| Fig. 5 (a) Interaction force acting on a bubble with the surface of the catalyst. (b) Illustration of the adhesion behavior of a bubble on a surface with continuous TPCL versus a surface with discontinuous TPCL. Reproduced with permission.98 Copyright 2021, Wiley. (c) Illustration to demonstrate the gas–liquid–solid interfaces where the surface is flat (left), superhydrophobic/superaerophilic (middle), and superhydrophilic/superaerophobic (right). Reproduced with permission.99 Copyright 2017, Wiley. (d) SEM (top) and operando optical images (bottom) of nickel foams with varying PTFE coverage with scale bars of 20 mm and 2 mm for SEM and operando optical images, respectively. Reproduced with permission.100 Copyright 2021, Elsevier. (e) A schematic demonstration of bubble escape from CHP, CZP, and CP during the electrochemical reaction. Reproduced with permission.101 Copyright 2021, Elsevier. (f) A schematic representation of bubble behavior on a surface with a uniform catalyst layer (left) and a mosaic-patterned catalyst. Reproduced with permission.102 Copyright 2021, Wiley. | |
Another factor affecting the bubble adhesive force is the triple-phase contact line (TPCL). Compared with a flat surface that has continuous TPCL, a roughness-enhanced surface with decreasing discontinuous TPCL exhibits much lower bubble adhesive force and bubble size. Therefore, the adhesion force between electrocatalyst and the substrate is alleviated by structure and morphology engineering. For example, the research group of Yuanzhe Piao investigated the significant effect of the morphologies of the catalysts on the hydrophilicity of the catalysts by preparing 3 different catalysts of which structures and compositions were elaborately controlled.101Fig. 5e presents a schematic depiction encompassing three distinct catalyst configurations alongside their respective bubble transport phenomena. The catalytic entities comprise phosphorized cobalt hydroxide (CHP), featuring nanoarray morphologies of cobalt phosphide (CoP); phosphorized zeolitic imidazolate framework-67 (CZP), characterized by CoP nanoarrays possessing CoP-tip configurations; and molybdenum-doped CoP (CP), exemplifying CoP nanoarrays with Mo-doped CoP tips. Notably, CZP, distinguished by its 'pillar and tip' architecture, exhibits superior catalytic performance in comparison to CHP, wherein solely CoP nanoarrays are evident. As CHP and CZP are composed of very similar elements, the difference in electrochemical performance can be attributed to the morphologies, which determined hydrophilicity of the catalysts. In the contact angle measurement between the catalyst and water, the contact angle of CZP was lower (49.36°) compared to CHP (68.53°), and the observed bubble size was also smaller. Therefore, the hierarchical micro/nano structure could enhance hydrophilicity, reducing the size of bubbles, and facilitating bubble escape from the electrode surface. The researchers further improved bubble transport by doping Mo into catalysts with the hierarchical structure. Bilu Liu et al. designed a mosaic catalyst that could more effectively remove bubbles by successfully controlling the area of the catalyst where bubble nucleation occurs (Fig. 5f).102 In a mosaic catalyst, Pt islands with small sizes were loaded on the support via sputtering comprising a mosaic-like pattern. The mosaic-like pattern of Pt islands limited the size of hydrogen gas bubbles, requiring less than 100 mV of overpotential to deliver the high current density of 1000 mA cm−2.
Following their formation and detachment from the electrode surface, gas bubbles in an electrolysis system undergo upward migration driven by buoyancy. However, as these bubbles traverse through the complex and disordered three-dimensional (3D) structure of the system, they are prone to being obstructed and trapped. The entrapment of these bubbles leads to the creation of physical barriers between the active catalytic sites and the surrounding electrolyte. Consequently, these trapped bubbles contribute to notable Ohmic losses and a reduction in the number of accessible active catalytic sites. Therefore, Yat Li and coworkers fabricated C–Ni1−xO/3DPNi with nanorods on the surface by 3D printing (Fig. 6a–c).103 The lattice structure implemented via 3D printing provides interconnected bubble flow channels. The nanorod microstructure roughens the electrode surface, reducing the contact area between the bubbles and the solid catalyst by aiding the faster escape of bubbles. To understand the effect of pore arrangement on bubble escape, computer models corresponding to 3DPNi and nickel foam (NF) were constructed (Fig. 6d and e). The time needed for bubbles with different diameters to traverse the yz-plane was calculated (Fig. 6f). The maximum bubble diameter when escape from the periodic 3DPNi is available is 29, which is larger than 20 in the NF. As seen in Fig. 6d, if the bubble diameter exceeds the pore size of 3DPNi, the bubble can deform and pass through the pore channels of the periodic structure. Meanwhile, the bubble experiences more collisions and deformation in the NF owing to the randomized structures and tortuous paths, increasing the travel distance. The 3DPNi electrodes decorated with NiO achieve a high current density of 1000 mA cm−2 in 1.0 m KOH at overpotentials of 245 for HER and 425 mV for OER, respectively, which is superior to NF with NiO. Jian Zhang and coworkers created a hierarchical surface of porous nickel tube arrays (PNTA) to facilitate the bubble transport (Fig. 6g).104 NiFe-PZnin situ grown on the PNTA surface, not only enlarge the surface area but also facilitates electron transfer from the catalyst to the conductive support (Fig. 6h). The formation and escape of bubbles at the catalysts were observed with an in situ optical microscope. Bubbles formed at the NiFe-PZn@PNTA are rapidly discharged (Fig. 6i). The gas bubbles produced in the PNTA move upward by buoyancy and will fuse with each other in the rising process, resulting in the volume expansion of the bubbles and a larger buoyancy force, which could promote the efficient release of the bubbles. (Fig. 6j). After the discharge of the gas bubble, rapid replenishment of electrolytes occurred, accelerating OER activity by supplying the reactant to the active site. NiFe-PZn@PNTA exhibited a far higher catalytic activity than NiFe-PZn@NTA as expected (Fig. 6k).
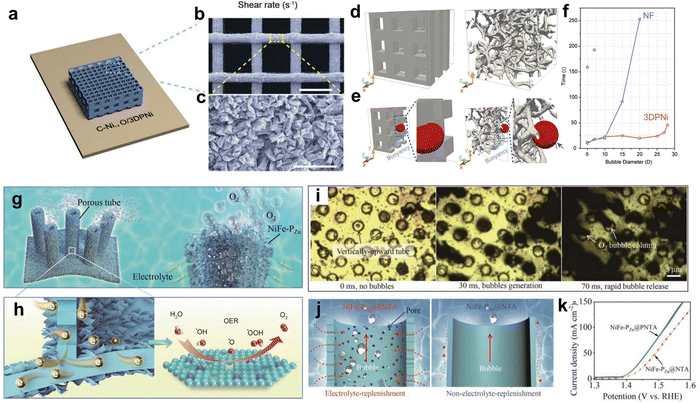 |
| Fig. 6 (a) Scheme illustration of the shape of periodic substrate of C–Ni1−xO/3DPNi. (b) and (c) SEM images capture the C–Ni1−xO/3DPNi lattice structure and a view with high magnification of an individual filament, respectively. Scale bars are 500 and 4 μm, respectively. The models of (d) 3DPNi and (e) NF structures used to showcase bubble shapes during escape. Arrow in (e) underlines the interaction of the bubble with the NF. (f) Bubble escape time without unit as a function of bubble diameter. Reproduced with permission.103 Copyright 2020, Wiley. (g) Schematic illustration of the microstructure of the tube sponge-like NiFe-PZn@PNTA, and (h) magnified illustration to demonstrate the facile electron transport and OER. (i) Operando optical microscope images of NiFe-PZn@PNTA during OER. (j) Visual illustrations of (left) NiFe-PZn@PNTA and (right) NiFe-PZn@NTA. (k) Polarization curves of NiFe-PZn@PNTA and NiFe-PZn@NTA. Reproduced with permission.104 Copyright 2020, Wiley. | |
2.3 Enhancing intrinsic activity
Obtaining affordable intrinsic activity of a catalyst is a prerequisite for realizing HCD of hydrogen production. The catalytic process involves the adsorption of reactants and the desorption of reaction intermediates, leading to gas evolution. The chemisorption behavior of the intermediates plays a crucial role in determining the catalytic reaction energy barrier.33 Ideal catalysts should exhibit moderate bonding strength with the intermediates to avoid unfavorable adsorption energy barriers and adverse desorption. The bonding strength between the electrocatalyst and the intermediate depends on the surface electronic structure of the catalyst.105 Therefore, regulating the surface electronic structure is an effective strategy for adjusting the reaction energy barrier for catalytic reactions. Surface chemistry engineering, phase engineering, and interface engineering are some of the valid strategies that can be employed to achieve this goal and will be discussed in detail.
2.3.1 Surface chemistry engineering.
Electrocatalysis is, in essence, a surface reaction, where the adsorption/desorption of reactants/products takes place at the catalyst surface region. Therefore, engineering surface chemistry of the catalyst tune catalytic performances for HCD water splitting. Engineering of surface chemistry refers to the deliberate modification or manipulation of the chemical and physical properties of a material's surface in order to achieve a specific desired effect or behavior.106 This can involve altering the surface composition, structure, morphology, or reactivity through various methods such as doping, vacancy formation, and surface functionalization.107 Engineering of surface chemistry modulates the electronic structure of active site near the surface, optimizing the adsorption and desorption process for the catalytic reaction. For example, Wang et al. presented a novel structure involving the incorporation of cobalt (Co) into ammonium lanthanum molybdate supported on Ni foams (referred to as Co-ALMO@NF).108 The introduction of Co into ALMO exerted a modulating effect on the electronic structure of molybdenum (Mo) and lanthanum (La), increasing the oxidation state. This effect could be attributed to electron transfer from ALMO@NF to the Co atom. The first-principles calculations revealed that the doped Co induced the charge redistribution around Mo and a negative shift in the d-band center, leading to a decrease in adsorption strength. The Co-ALMO@NF catalyst demonstrated excellent electrocatalytic performance, requiring 349 mV and 341 mV overpotentials to achieve a high current density of 600 mA cm−2 for the HER and OER, respectively. Mu et al. recently investigated the incorporation of Ru as a single-atom catalyst in FeCo-layered double hydroxide (LDH).109 These Ru atoms were embedded on the surface of FeCo-LDH, serving as isolated active sites and contributing to an optimal symmetry-breaking interface (Fig. 7a). The doping of a small amount of Ru resulted in the absence of scattering peaks corresponding to the Ru–Ru bond in the extended X-ray absorption fine structure (EXAFS) spectra, indicating the successful dispersion of Ru atoms as isolated entities within the FeCo-LDH structure (Fig. 7b). Specifically, the introduction of single atomic-site doping of the oxophilic-metal species Ru in FeCo-LDH facilitated the formation of an atomic Ru–O-transition metal (TM) symmetry-breaking interface structure. This atomic-scale symmetry breaking led to unsaturated bonding and the localized accumulation of excess charge. Theoretical calculation in Fig. 7c shows that he presence of these atom-scale symmetry-breaking interfaces on the FeCo-LDH surface promoted the coupling of oxygen atoms (O–O coupling) at the Ru–O active sites during the oxygen evolution reaction (OER), leading to the significant changes in the electronic structures of FeCo-LDH. Optimized electronic structure of FeCo-LDH and Ru active site resulted in a low overpotential of 194 mV at 1000 mA cm−2 for the OER process. Also, in addition to metal doping, the incorporation of non-metal elements through doping serves as an efficacious strategy. This approach engenders a consequential redistribution of electron density within the material system, thereby exerting a synergistic influence on the regulation of both the energy associated with water dissociation and the ΔGH parameter. Consequently, this intricate interplay results in the promotion of rapid water dissociation kinetics. In all alkaline electrolytes, water dissociation is always the most important step toward producing the hydrogen source needed for HER process. Enhanced water dissociation kinetics generates massive H3O+ intermediates within an electric double layer, resulting in an acid-like local environment under high [OH−] condition. This acid-like localized condition modulates the H adsorption behavior and energy barrier for HER, increasing the catalytic activity.110 The Miao group conducted a comprehensive investigation on the efficacy of non-metal dopants (specifically, B, N, O, and F) in Ni3S4 for HER.111 The introduction of non-metal dopants, particularly N, O, and F, was found to significantly modulate the electronic structure of the dual active site, resulting in a reduction in electron density and an elevation in the oxidation state of Ni and S. This phenomenon can be attributed to the higher electronegativity of non-metal elements compared to that of S. The adsorption energies of H2O and OH were found to be directly correlated with the d-band center (εd) of Ni, while the adsorption energy of H exhibited a linear dependence on the p-band center (εp) of the non-metal S (Fig. 7d and e). The free energy diagram in Fig. 7f revealed that the Volmer–Heyrovsky pathway predominantly governs the HER process on the F-Ni3S4 surface. Notably, the relatively low values of
and
indicated efficient water dissociation and adsorption/desorption of Hads, thus validating the accelerated Volmer and Heyrovsky steps. Fig. 7g displays that F-Ni3S4 electrocatalyst shows excellent HER performances due to tuned electronic structure and facile water dissociation.
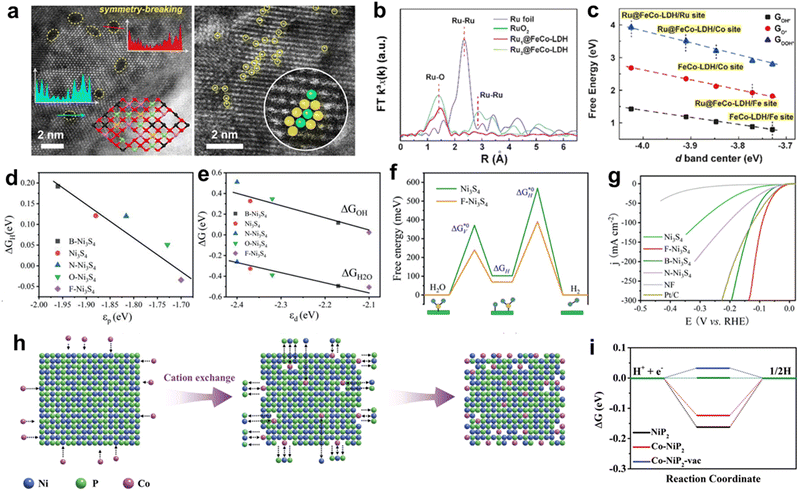 |
| Fig. 7 (a) HAADF-STEM images of Ru SACs@FeCo-LDH. The defects indicated by yellow dotted lines, along with their corresponding intensity profiles, and the bright spots identified by yellow circles correspond to individual Ru atoms. (b) The Fourier transform (FT) at the R space of Ru foil, RuO2 and Ru SACs@FeCo-LDH. (c) Relationship between ΔGOOH*, ΔGOH*, ΔGO* and d-band centers. Reproduced with permission.109 Copyright 2022, Royal Society of Chemistry. (d) Relationship between ΔGH and p-band center of S atom. (e) Relationship between ΔGH2O, ΔGOH and d-band center of Ni atom. (f) Free energy diagram of the pristine and F-doped Ni3S4. (g) Polarization curves of F-Ni3S4 with comparable electrocatalyst. Reproduced with permission.111 Copyright 2020, Wiley. (h) Schematic illustration of cation-exchange process of NixCo1−xP nanosheet array. (i) Free energy diagram of H adsorption for NiP2 systems. Reproduced with permission.113 Copyright 2022, Wiley. | |
The incorporation of vacancies within catalyst materials has been recognized as a means to expand the population of active sites with the presence of defective regions, which exhibit elevated distortion energy and diverse atomic configurations.112 Employing vacancy engineering manipulation of local charge density, enhancing the intrinsic activity of electrocatalyst. For example, Tao group conducted a study on the atomic-level surface engineering of NiP through a facile cation-exchange method employing Co ions (Fig. 7h).113 This process involves the replacement of Ni2+ ions with Co2+ ions, accompanied by the dissolution of some P anions, resulting in the formation of vacancies around the Co2+ ions. The combination of P vacancies and atomic-level Co doping facilitates electron transfer from Co and P to Ni. The modulation of the electronic structure through the presence of P vacancies and atomic-level Co doping weakens the interaction between the H adsorbate and the P surface atom, thereby optimizing the adsorption and desorption processes (Fig. 7i). Furthermore, the abundance of P vacancies in the outermost layer results in the exposure of excess active edge sites, leading to improved electrocatalytic activity. By precisely controlling the Co content, the optimal Ni0.96Co0.04P electrode demonstrates remarkable bifunctional electrocatalytic performance for overall water splitting, even at a high current density of 1000 mA cm−2. The overpotentials for the HER and the OER are measured to be 249.7 mV and 281.7 mV, respectively. Wei et al. investigated the utilization of ethaline-based deep eutectic solvent (DES) for electrodeposition, with the objective of achieving multi-vacancy engineering.114 By introducing NO3− ions during the electrodeposition, cation and anion vacancy-rich nickel hydroxide species were formed on the surface of the Ni-based catalyst. Density functional theory (DFT) calculations were performed to elucidate the coordinated regulation of these multi-vacancies, involving O2− and Ni2+ ions (specifically, Ni(Fe)OOH(VO + VNi)). It was hypothesized that this arrangement optimizes the adsorption or desorption behavior of intermediates on the Ni sites, thereby enhancing the overall catalytic activity. Experimental results demonstrated that the as-prepared NiFe catalyst, containing NO3− ions and deposited on Fe foam, exhibited a significantly lower overpotential of 320 mV at 200 mA cm−2 compared to the NiFe catalyst containing Cl− ions (355 mV).
2.3.2 Phase engineering.
The physicochemical characteristics of catalysts in the crystalline or amorphous states significantly influence their catalytic properties. Electrocatalysts featuring an amorphous phase exhibit enhanced catalytic activity compared to crystalline counterparts, which can be attributed to several factors.115–117 Firstly, the presence of randomly oriented bonding with defects at the surface increases the number of active sites available for catalytic reactions. This abundance of active sites promotes efficient interaction with reactants and enhances the overall catalytic performance. Secondly, the amorphous phase often exhibits an unsaturated electronic configuration, which optimizes both the adsorption and desorption reactions of intermediates. This electronic configuration facilitates the favorable binding and release of gas products, contributing to improved catalytic activity. Thirdly, the structural flexibility inherent to the amorphous phase allows for self-regulation in terms of the electronic structure and geometry of the active site in response to the applied potential. This adaptability enables the electrocatalyst to dynamically adjust its active site properties, optimizing the catalytic performance for the targeted reaction. Furthermore, the flexible local structure of the amorphous phase facilitates efficient charge transfer between the active site and reaction intermediates. In OER process, this accelerated charge transfer promotes the transformation of the electrocatalyst into the (oxy)hydroxide phase during the OER process, which is considered crucial for achieving high OER efficiency. In light of these considerations, our research group devised amorphous borophosphate materials through a mechanothermal method (Fig. 8a).118 By subjecting LiNiFe borophosphate (c-LNFBPO) to high-energy ball milling, the simultaneous application of mechanical and thermal energies induced a disordering of the crystalline lattice, resulting in the formation of fully amorphized LiNiFe borophosphate (a-LNFBPO). This amorphization process led to an increase in the valence state of the transition metals (Ni and Fe) due to the formation of an uncoordinated environment and defects within the structure. Additionally, the bond lengths of Ni–O and Fe–O in a-LNFBPO decreased, indicating the presence of strong metal–oxygen (M–O) covalent interactions. This strong M–O covalency in a-LNFBPO contributes to a lower charge transfer energy, thereby facilitating facile charge transfer between the catalyst and the electrolyte. The structural flexibility of a-LNFBPO, arising from the abundance of defects in the catalyst, reduces the energy required for the distortion of the active site. These unique features of a-LNFBPO play a crucial role in promoting the complete transformation of originally inert species into the active phase during the OER, whereas the active phase in c-LNFBPO remains confined to the surface. The a-LNFBPO@NF exhibited a significantly smaller overpotential of approximately 270 mV at a current density of 100 mA cm−2 compared to c-LNFBPO, which necessitated an overpotential of approximately 350 mV. Wu et al. designed B-doping-induced amorphization of crystalline layered double hydroxide (a-NiCo LDH) for HER catalyst (Fig. 8b).119 The doping process involved replacing the charged balanced carbonate anion (CO32−) with borate units (BO33−). This displacement resulted in the disruption of the original crystalline structure, leading to the formation of an amorphous structure with a significant number of oxygen vacancies and unsaturated atoms, thereby increasing the exposure of catalytically active sites. The amorphization of NiCo LDH led to an increase in the oxidation state of the transition metal species, attributed to the high electronegativity of borate. This higher oxidation state facilitated the efficient transfer of charges from Ni and Co to neighbor. The resulting amorphized NiCo LDH exhibited excellent performance as a HER catalyst, achieving a high current density of 500 mA cm−2 at an overpotential of 286 mV, and 1000 mA cm−2 at an overpotential of 381 mV.
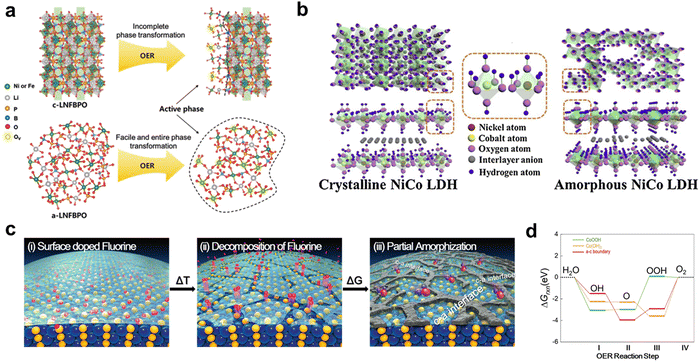 |
| Fig. 8 (a) Schematic illustration of surface reconstruction of c-LNFBPO and a-LNFBPO. Reproduced with permission.118 Copyright 2020, Wiley. (b) Schematic illustration of the crystal structure for the crystalline and amorphous NiCo LDH nanosheet arrays supported on the nickel foam. Reproduced with permission.119 Copyright 2020, Elsevier. (c) Schematic of the c–a interface via fluorine surface doping in Co2B. (d) Calculated free energy diagram of OH, O and OOH adsorption on the surface of CoOOH, Co(OH)2, and the c–a interface of Co2B. Reproduced with permission.122 Copyright 2019, Royal Society of Chemistry. | |
It should be noted that amorphous materials with a single component have intrinsically low electrical conductivity, which usually results in inferior catalytic activity than the crystalline counterparts.120,121 Therefore, the utilization of the advantages offered by both amorphous and crystalline structures through the formation of an amorphous and crystalline phase boundary is an effective strategy to design electrocatalyst for HCD. Taking into consideration these factors, Song group conducted a thorough investigation aimed at enhancing the catalytic performance of Co2B through the selective fluorination surface doping, thereby increasing the density of the crystalline–amorphous (c–a) phase boundary (Fig. 8c).122 Theoretical calculations revealed that the incorporation of F dopants was energetically favored exclusively on the surface of Co2B. At elevated temperatures, the F dopants decomposed into BF3 gas molecules, resulting in the formation of an amorphous phase on the surface. Consequently, the Co2B surface exhibited a significantly high density of c–a interfaces, which harnessed the combined advantages of the amorphous and crystalline phases, ultimately leading to enhanced oxygen OER activity. The Co2B catalyst with a high density of c–a interfaces on Nickel foam displayed remarkable OER performance, requiring only overpotential of 170 mV at a current density of 100 mA cm−2. The presence of the c–a interface enabled the amorphous phase to provide numerous adsorption sites for water or hydroxide molecules. Simultaneously, the crystalline Co2B phase facilitated the transport of electrons from the electrode to the adsorbed species, significantly enhancing the OER efficiency. Theoretical calculations in Fig. 8d further confirmed that the energy barrier associated with the rate-determining step of OER, specifically OOH* formation, was substantially reduced on the c–a interface compared to the amorphous or crystalline phases. These findings elucidate that high-density c–a interfaces effectively leverage the unique characteristics of both the amorphous and crystalline phases, leading to improved OER performance and a notable decrease in the energy barrier for the rate-determining step of OER. Similarly, Xu et al. reported the conversion strategy of nickel zeolite imidazolate framework (Ni-ZIF) nanorods into ultrathin Ni-ZIF/Ni-B nanosheets with abundant c–a phase boundaries via immersing NaBH4 solution.123 The crystalline Ni-ZIF is more favorable for the step from *O to *OOH, while the amorphous Ni-B has better catalytic activity for the formation of *OH. As expected, upon coupling crystalline Ni-ZIF with amorphous Ni-B, not only the energy barrier for *OH is effectively reduced but also the reaction free energy from *O to *OOH would be lowered, demonstrating the intrinsically enhanced activity in Ni-ZIF/Ni-B with c–a boundary. Also, the ΔGH* value is close to zero in Ni-ZIF/Ni-B with c–a phase boundary compared to crystalline and amorphous counterparts. Based on these, the Ni-ZIF/Ni-B nanosheet@NF showed superior electrocatalytic performances for HER and OER compared to crystalline and amorphous counterparts.
2.3.3 Heterointerface engineering.
Although many catalysts exhibit high intrinsic catalytic activity, they often suffer from certain limitations during practical applications, such as poor conductivity, a limited number of active sites, and sluggish reaction kinetics. To address these challenges, it has been observed that the combination of different catalysts or materials can result in synergistic effects and significantly enhance the overall performance beyond that of the individual components. Consequently, interface engineering by integrating different catalytically active components has emerged as a promising approach to tailor surface chemisorption properties for efficient water splitting.124 Through interface interactions, charge transfer processes occur, leading to surface electron reorganization. This, in turn, facilitates the modulation of the bonding strength between the active sites and reaction intermediates. Furthermore, the interface engineering approach holds the potential for achieving synergistic effects, wherein the combined materials or catalysts exhibit cooperative behaviors that surpass the individual contributions, resulting in superior catalytic performance. Therefore, the integration of different catalytically active components and the subsequent interface engineering approach offers a promising avenue for the development of highly efficient catalysts, enabling improved chemisorption properties, enhanced charge transfer, and the realization of synergistic effects. Zhang et al. reported a novel approach involving the implementation of a built-in electric field (BEF) strategy for the synthesis of heterogeneous Ni2P – Co6(CO3)2(OH)8·H2O [CoCH] arrays grown on carbon fiber paper (Ni2P-CoCH/CFP), as depicted in Fig. 9a.125 The design of the BEF relies on the combination of two hetero components possessing distinct Fermi levels, which effectively modifies the electronic structure of the active sites and achieves a balanced adsorption strength of crucial reaction intermediates. The difference in work function (ΔΦ) between Ni2P and CoCH generates a favorable driving force for electron flow from the high-level to the low-level region, leading to strong interfacial BEF from CoCH to Ni2P (Fig. 9b). Consequently, it induces the formation of an electron-rich Ni2P region and an electron-deficient CoCH region, thereby facilitating the adsorption processes of both hydrogen and oxygen intermediates. Furthermore, as illustrated in Fig. 9c, the interfacial interaction between Ni2P-CoCH offers optimized active sites that exhibit favorable adsorption properties towards hydrogen and oxygen intermediates. The Ni2P-CoCH/CFP composite, benefiting from the presence of a strong interfacial BEF, demonstrates excellent electrocatalytic performance at 100 mA cm−2 for both HER and OER with overpotentials of 143 mV and 320 mV, respectively.
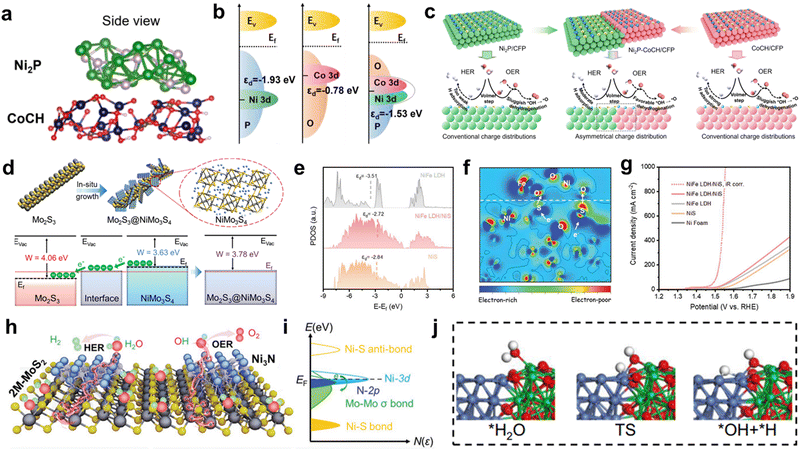 |
| Fig. 9 (a) The structure of Ni2P-CoCH. (b) Schematic illustration of d-band center of Ni2P, CoCH, and Ni2P-CoCH. (c) Schematic illustration of HER and OER mechanisms for Ni2P-CoCH/CFP electrocatalyst. Reproduced with permission.125 Copyright 2023, Wiley. (d) Energy band diagrams of Mo2S3 and NiMo3S4. Reproduced with permission.128 Copyright 2022, Wiley. (e) d orbital projected density of NiFe LDH, NiS and NiFe LDH/NiS. (f) The 2D data plot of Electron density difference for NiFe LDH/NiS. (g) Polarization curves of NiFe LDH/NiS. Reproduced with permission.129 Copyright 2022, Wiley. (h)Schematic illustration of water splitting process of the Ni3N@2M-MoS2. (i) Charge redistribution of active electronic states in Ni3N@2M-MoS2. Reproduced with permission.131 Copyright 2022, Wiley. (j) Atomic configurations of water dissociation process on the active sites of Ni(111)/Yb2O3(222) interface. Reproduced with permission.132 Copyright 2022, Springer Nature. | |
Similarly, Schottky heterojunctions, which arise from the interfaces between metals and semiconductors, exhibit a built-in electric field that facilitates electron transfer and separation processes.126 This electric field is established to balance the Fermi level difference between the metal and semiconductor components, allowing for spontaneous electron flow across the heterointerfaces and consequent adjustment of their electronic structures. Based on this, Ren et al. reported the Ni–MoO2 heterointerfaces, this built-in electric field drives the transfer of electrons, leading to a decrease in the Schottky resistance.127 The self-driven electron injection from Ni to MoO2 resulted in electronic redistribution at the interface. As a consequence, the electron density is enriched on the MoO2 side, while holes accumulate on the Ni side. Consequently, the reduced electron density in the Ni metal modifies the adsorption/desorption energy barriers for the reaction intermediates, achieving the small overpotential of 153 mV for HER at 100 mA cm−2. Fuqiang Huang and coworkers developed a sulfide-based metallic heterostructure composed of Mo2S3@NiMo3S4 to address the challenges related to electronic conductivity and high ohmic contact resistance typically encountered in heterostructures with ohmic barriers.128 The energy band diagrams depicted in Fig. 9d for Mo2S3, NiMo3S4, and Mo2S3@NiMo3S4 elucidated the favorable electron transfer from NiMo3S4 to Mo2S3 by the formation of the heterointerface. This electron transfer process promoted efficient charge transfer for rapid Volmer H and Heyrovsky H2 generation. Notably, Mo2S3@NiMo3S4 electrode demonstrated an overpotential of only 174 mV at a significant current density of 1000 mA cm−2. Wen et al. devised a novel Schottky heterojunction comprising dispersed NiFe LDH and ultrathin NiS nanosheets, denoted as NiFe LDH/NiS.129 The NiFe LDH component exhibited typical semiconductor behavior, characterized by a considerable band gap of approximately 1.51 eV. Remarkably, upon coupling with NiS, an augmented local density of states was observed, indicating a more conductive electronic structure for NiFe LDH/NiS. The d-band center of Ni(Fe) atoms in NiFe LDH/NiS shifted to a higher energy level compared to NiFe LDH and NiS, signifying an optimized binding strength for oxygen intermediates (Fig. 9e). 2D data plot of electron density difference in Fig. 9f demonstrates the alternating connection of electron accumulation and depletion regions, suggesting electron injection from NiS to NiFe LDH. The coupling of NiFe LDH induced electron redistribution at the interface, creating localized electrophilic/nucleophilic regions and modifying the surface chemisorption properties. The construction of Schottky heterojunction between NiS and NiFe LDH resulted in a high current density of 1000 mA cm−2 at an overpotential of 325 mV (Fig. 9g).
The combination of two catalysts, each possessing distinct reaction sites, leads to complementary effects that result in enhanced reaction rates and selectivity. This phenomenon arises when one catalyst demonstrates exceptional performance in the HER, while the other catalyst exhibits superior capability in OER. The coupling of these catalysts synergistically enhances the overall catalytic activity, leveraging their respective advantageous properties. For instance, Dutta et al. fabricated the NiFe LDH-NiSe catalyst for bi-functional HER and OER.130 The NiFe LDH is recognized as a promising OER catalyst in alkaline electrolysis, attributed to its large surface area, exposed active sites, and adjusted electronic structure through Fe incorporation. However, the poor intrinsic conductivity of NiFe LDH poses a limitation for HER, particularly at high current densities. On the other hand, nickel selenides have been explored as efficient catalysts for HER or even as bifunctional electrocatalysts due to their inherent high conductivity and long-term stability. Hence, the combination of NiFe LDH with NiSe offers the advantage of simultaneous HER and OER activities, requiring an overpotential of 240 mV for OER and 276 mV for HER at a current density of 100 mA cm−2. Wu et al. developed a novel metallic heterointerface, Ni3N@2M-MoS2, composed of Ni3N and monoclinic 2M-MoS2.131 Ni3N, a representative metal nitride, has a tendency to adsorb hydroxyl (OH−) species on the Ni surface, making it favorable for OER. However, due to its hydrophobic nature, Ni3N exhibits limited capability to adsorb and split water for HER in alkaline solutions. In contrast, 2M-MoS2 possesses superior hydrophilicity, enabling efficient mass transfer and refreshing of exposed active sites for water adsorption and dissociation. As shown in Fig. 9h, the Ni3N@2M-MoS2 composite promotes water dissociation, providing a hydrogen source with a lower energy barrier for HER. Furthermore, formation of a heterointerface between Ni and MoS2via Ni–S bonding leads to a tuned electronic structure of Ni in Fig. 9i, which enhances the adsorption of OER intermediates, thereby reducing the energy barrier for the rate-determining step (oxidation of *O to *OOH). The synergistic effect arising from the separated reaction sites and the heterointerface contributes to the outstanding HER and OER performance of Ni3N@2M-MoS2, with ultralow overpotentials (η) of 155 mV and 327 mV, respectively, at a current density of 1000 mA cm−2. Sun group employed the incorporation of oxophilic species in electrocatalysts to enhance the activity of HER.132 The introduction of oxophilic species promotes the cleavage of H–OH bonds in water molecules and facilitates the sluggish Volmer step of alkaline HER. Lanthanide oxides (Ln2O3), known for their high thermodynamic stability and oxophilicity, are considered promising promoters for water dissociation. Among them, Yb2O3 showed high oxophilicity and thermodynamic stability, which significantly increased the density of active sites, reduced the energy barrier for water dissociation, and optimized the free energy of hydrogen adsorption. Ni/Yb2O3 heterointerface induced the strong adsorption of OH and favorable water molecule adsorption and H–OH bond cleavage. Fig. 10j illustrates the mechanism whereby the oxygen atom of water is adsorbed on the Yb atom of Yb2O3, facilitating the subsequent breaking of the water molecule into hydroxyl and hydrogen intermediates. These intermediates are adsorbed by nearby Yb and Ni atoms, respectively. The screened Ni/Yb2O3 catalyst exhibited a low overpotential of 116 mV at 500 mA cm−2 and excellent long-term durability of 360 hours at 500 mA cm−2.
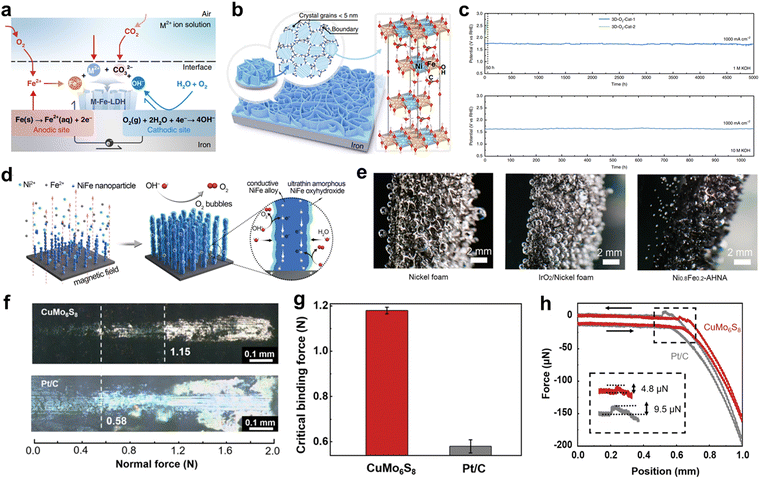 |
| Fig. 10 (a) Schematic illustration of reactions of corrosion engineering of iron substrates. (b) Grain boundary-enriched layered double hydroxide (LDH) nanosheet arrays prepared by corrosion engineering. (c) Stability test of 3D-O2-Cat-1 and 3D-O2-Cat-2 in 1 M KOH and in 10 M KOH at a current density of 1000 mA cm−2. Reproduced with permission.145 Copyright 2018, Springer Nature. (d) Schematic illustration of the synthesis of the NixFe1−x-AHNA and its self-activation process during OER. (e) Digital photos of the oxygen bubbles on the surface of nickel foam, IrO2/nickel foam and Ni0.8Fe0.2-AHNA during the OER process. Reproduced with permission.149 Copyright 2020, Royal Society of Chemistry. (f) The photos of micro-scratches of CuMo6S8 (top), Pt/C (bottom) adhesive on Cu foils. The dotted lines represent the critical adhesive forces. (g) Critical adhesive forces of CuMo6S8 and Pt/C. (h) Electrocatalyst-bubble interfacial adhesion force of CuMo6S8/Cu and Pt/C electrodes. Reproduced with permission.150 Copyright 2020, Springer Nature. | |
3. Strategies to achieve high stability
The stability of electrocatalysts plays a crucial role in evaluating their catalytic performance. Electrocatalysts are typically deposited, coated, or attached to a substrate electrode, and the interaction between the catalyst and the substrate electrode is essential for maintaining stability.133,134 Weakening of this interaction can result in catalyst detachment, leading to the loss of active sites and degradation of catalytic performance, particularly under HCD conditions where vigorous gas evolution and gas bubble interaction occur.98,135 The applied potential during electrochemical reactions can also impact catalyst stability. The oxidation or reduction of the catalysts at specific potentials can lead to the formation of unstable species, causing oxidative or reductive dissolution. These processes are considered the primary reasons for catalyst degradation and deactivation. The dissolution pathways of catalysts can vary depending on factors such as the crystalline facets of the catalyst, support substrates, and applied potentials.136,137 Furthermore, under anodic potentials, dissolved metal ions can redeposit onto the surface of the electrocatalyst through a dissolution–redeposition process.138 This process involves phase segregation which is the partitioning of a secondary phase from a primary phase. Phase segregation can significantly impact the long-term durability of catalysts, as it leads to dynamic cation redistribution and the loss of activity. In this section, we discuss the degradation mechanisms of electrocatalysts and explore strategies to mitigate degradation and design durable electrocatalysts. Understanding and addressing these degradation processes are crucial for the development of stable and efficient electrocatalytic systems. Table 3 summarizes the recent progress of electrocatalysts for high stability.
Table 3 Summary of high stability of electrocatalyst recently reported under high current densities
|
Materials |
Substrate |
Electrolyte |
Stability test |
Ref. |
Method |
Current density (mA cm−2) |
Potential (V) |
Duration (h) |
HER |
Ni(OH)x/Ni3S2 |
Ni foam |
1 M KOH |
CA |
320 |
|
1000 |
232
|
|
Nickel nanopyramid arrays |
Ni foam |
1 M KOH |
CP |
1000 |
−1 |
7000 |
233
|
|
OER |
S-FeOOH |
Fe foam |
1 M KOH |
CP |
1000 |
∼2.7 |
1000 |
234
|
Bi/BiFeOxHY |
|
1 M KOH |
CP |
1000 |
1.58 |
1000 |
235
|
FeCoNiS |
Ni foam |
1 M KOH |
CP |
100 |
1.75 |
∼3000 |
236
|
Ni(OH)2/Fe2O3 |
|
|
CP |
1000 |
1.5 |
1500 |
237
|
Dealloyed FeNi |
Ni foam |
1 M KOH |
CP |
1000 |
1.5 |
1350 |
238
|
30 wt% KOH |
1.55 |
100 |
|
Bifunctional |
Porous Co-P |
Co foam |
1 M KOH |
CP |
(HER) 1000 |
−0.5 |
3000 |
239
|
(OER) 1000 |
1.7 |
3000 |
(Overall) 1000 |
2 |
4000 |
RuO2–NiO |
Ni foam |
1 M KOH |
CA |
(Overall) 1000 |
— |
2000 |
240
|
NiMoOx/NiMoS |
Ni foam |
1 M KOH |
CP |
(Overall) 500 |
1.75 |
500 |
241
|
FeCoNi(S) |
Ni foam |
1 M KOH |
CA |
(Overall) 500 |
— |
2000 |
242
|
3.1 Improving mechanical stability
The stability of electrocatalysts under HCD conditions is of utmost importance for practical large-scale water splitting applications. The mechanical stability of the catalyst is indicative of its ability to withstand electrochemical fatigue and external forces. Typically, materials are bonded onto the flat surface of a current collector using electrical insulating binding agents like Nafion, which may sacrifice a portion of the active sites.139 As discussed in Section 2.2, bubbles adhered to the catalyst exert a strong interfacial adhesion force when they detach, leading to the potential peeling-off of certain parts of the catalyst and compromising its mechanical stability. This peeling-off issue becomes more severe with increasing current density unless the interaction force between the catalyst and substrate surpasses the interfacial adhesion force between the catalyst and bubbles. Therefore, it is important to enhance the mechanical stability of electrodes by enhancing electrocatalyst–support and weakening electrocatalyst-bubble interfacial forces. Enhancing the interaction force between the catalyst and support is an effective strategy for achieving high durability of the electrocatalyst under HCD conditions. It is evident that powdery electrocatalysts are not well-suited for high current-density water splitting, as the destructive tensile and shaking forces experienced during operation present significant challenges.140 Therefore, self-supported electrocatalysts, consisting of nanoarrays integrated with 3D conducting substrates, have shown potential for sustaining high current density catalysis due to their improved mass transfer capacity and enhanced mechanical properties.141 Self-supported electrocatalysts are typically fabricated using electrodeposition and hydrothermal methods.142–144 Nevertheless, a significant ongoing challenge in electrocatalysis is the detachment of the electrocatalyst from the substrate, particularly under HCD. In the current state of research, many electrocatalysts grown on supports primarily rely on weak physical or chemical interactions for adhesion. These interactions include electrostatic adsorption, mechanical interlocking within porous structures, and intermolecular attraction governed by van der Waals forces. However, such weak interfaces between the electrocatalyst and support structure often prove inadequate to withstand the effects of bubbling, leading to poor stability of the electrode. To maintain a strong interaction between the catalyst and substrate, a novel approach involving the use of the substrate as a precursor is being employed. This method aims to ensure the stability of the catalyst–substrate interface and improve the overall durability of the electrocatalyst under HCD conditions. Liu et al. devised a facile corrosion engineering method to enhance the performance and stability of electrocatalysts.145 In their study, iron foam (IF) was immersed in an aqueous solution containing Ni2+, leading to the spontaneous formation of Fe-bearing layered double hydroxides (LDHs) on the surface of the IF, as illustrated in Fig. 10a. Unlike the commonly-formed iron rusts, the as-generated LDHs exhibited a well-oriented, grain boundary-enriched nanosheet array thin film structure, resulting in a significant proportion of highly active catalytic sites (Fig. 10b). The LDHs generated using IF (3D-O2-Cat-1) demonstrated superior electrocatalytic performance for OER compared to 3D-O2-Cat-2 prepared by electrodeposition. Specifically, 3D-O2-Cat-1 exhibited current densities of 500 and 1000 mA cm−2 at lower overpotentials of 300 and 340 mV, respectively, in a 1 M KOH solution. This improvement was attributed to the exposure of more unsaturated edge sites at the grain boundaries, which facilitated the adsorption and activation of reactants, enhancing the OER performance. Furthermore, the stability of 3D-O2-Cat-1 was evaluated under large current densities of 1000 mA cm−2 for an extended period of 5000 hours in a 1 M KOH solution, as shown in Fig. 10c. Remarkably, 3D-O2-Cat-1 exhibited ultra-high stability without significant degradation during this prolonged testing period. However, it was observed that 3D-O2-Cat-1 experienced serious deactivation after 50 hours under large current densities. The main reason for this deactivation was identified as the peeling of catalytic active species from the IF during the intense OER process under high current density conditions. This finding highlighted the importance of a strongly coupled interface between the LDH thin film and the substrate. Xin-Yu Zhang and coworkers reported the in situ formed anion-activated FeOOH via the electrochemical activation method of Iron foam (IF) at high current density (1000 mA cm−2).146 Of the seven common anions (VO33−, MoO42−, WO42−, S2−, H2PO4−, H2PO2− and F−), S2− was found to have the best regulatory effect on OER activity. S modified FeOOH/IF exhibits industrial-level OER current output of 1000 mA cm−2 with a low overpotential of 358 mV. Importantly, this catalyst can operate stably at 1000 mA cm−2 for at least 1000 h due to direct synthesis by adding trace anionic additive in the process of electrochemical test. Guo et al. designed the facile boronization strategy for transforming commercially available metal sheets into highly efficient and stable, corrosion-resistant, nonprecious electrodes.147 The catalyst peeling problem cannot be completely avoided, especially for long-time electrocatalysis due to self-supporting configuration and direct synthesis of metal sheets. Furthermore, the generation of metal sub-boride layers on the metal sheets via self-functionalization is responsible for the improved catalytic stability and corrosion resistance, showing the excellent stability at 500 mA cm−2 for 200 h under 30 wt% KOH solution.
In addition to the strong interaction between the electrocatalyst and the substrate, weakening the adhesion between the bubble and the electrocatalyst is also important for stable electrolysis under HCD. The adhesion force between the electrocatalyst and the substrate is alleviated by 3D nano array. When nano array heads upward, the triple-phase contact line (TPCL) is discontinued.148 The presence of gaps or interruptions in the TPCL can lead to a decrease in the adhesion force between the solid and gas phases. This weakened adhesion force can result in a reduced contact area and increased contact angle at the TPCL, leading to limited interaction between the phases and superaerophobicity. Based on these, Liang et al. successfully fabricated a binder-free NiFe-amorphous oxyhydroxide nanowire array (Ni0.8Fe0.2-AHNA) structure using a one-step chemical deposition method in the presence of a uniform magnetic field (Fig. 10d).149 This unique self-supported hierarchical nanowire array exhibits an upward orientation. The distinctive architecture of Ni0.8Fe0.2-AHNA plays a crucial role in reducing the contact area between the electrode and generated bubbles, thereby facilitating efficient bubble release and ion transfer processes. Moreover, the nanoscale confinement effect within the nanowire array restricts the valid growth region for NiFe oxyhydroxide to a narrow range of 1–5 nm. This confinement effect effectively mitigates the dissolution of catalytic components and degradation of catalytic activity. Experimental observations depicted in Fig. 10e demonstrate that oxygen bubbles exhibit strong adhesion to the surface of bare nickel foam and IrO2/nickel foam, leading to their substantial growth to sizes exceeding 800 mm. In contrast, the Ni0.8Fe0.2-AHNA surface enables easy detachment of bubbles, even when they are of small size. This upward nanoarray with small interaction of electrocatalyst-bubble requires the lowest overpotentials of 248 and 258 mV to reach large current densities of 500 and 1000 mA cm−2 and excellent stability over 120 hours. Furthermore, a dual interfacial engineering strategy targeting both the electrocatalyst–substrate and electrocatalyst–bubble interfaces was developed by Liu et al.150 In their work, a CuMo6S8/Cu electrode was fabricated through an in situ reaction between MoS2 and Cu, leading to a strong binding at the electrocatalyst–substrate interface and weak adhesion at the electrocatalyst–bubble interface. The critical binding forces of the CuMo6S8 and Pt/C electrodes were evaluated using a micro scratch tester, as depicted in Fig. 10f. The CuMo6S8 electrode exhibited peeling from the support at a load of 1.15 N, whereas Pt/C exhibited peeling at 0.58 N (Fig. 10g). To assess the interfacial adhesion force with bubbles (Fig. 10h), the CuMo6S8/Cu electrode demonstrated a significantly smaller force (4.8 μN) compared to the Pt/C electrode (9.5 μN). The CuMo6S8/Cu electrode, achieved through dual interfacial engineering, exhibited remarkable stability even at large current densities of 500, 1000, and 2500 mA cm−2. The electrode showed negligible degradation of performance within 300 h, particularly maintaining high stability at an ultra-large current density of 2500 mA cm−2 for over 100 h.
3.2 Improving chemical stability
The surface reconstruction process in transition metal-based electrocatalysts plays a crucial role in their electrochemical performance, particularly in the HER and OER.151 During this process, reductive species are formed on the catalyst surface during HER, while metal oxide and oxy-hydroxide phases are formed during OER.152,153 This in situ reconstruction process has the ability to modulate electrocatalytic behaviors such as adsorption, activation, and desorption, ultimately enhancing catalytic performance. However, it should be noted that the surface reconstruction process is accompanied by the transient dissolution of elements. This dissolution occurs due to disruptions in the crystal structure, which are caused by changes in the oxidation state of the transition metal (Fig. 11a). For example, the oxidation or reduction process involving the insertion or removal of oxygen atoms from the crystal structure can result in dissolution. Such disruptions often occur due to the presence of a low coordination number or metastable transition state, both of which contribute to the disturbance of the crystal structure. In the case of catalysts with weak stability, such as perovskites and metal–organic frameworks (MOFs), the reconstruction process can potentially lead to the destruction of the internal structure due to the severe dissolution of their components. This, in turn, results in the loss of active sites. Consequently, it is crucial to inhibit the dissolution of these active species while simultaneously preserving the reconstruction process, as it is key to maintaining the high catalytic activity and stability of the original catalysts. This challenge can be addressed through the introduction of additional elements or chemical groups to stabilize the bulk structure of the catalysts. In conclusion, it is imperative to exercise control over surface reconstruction to prevent compromising the entire bulk structure of the oxide catalyst, which serves as the template for creating a highly active surface. By managing the dissolution of active species and ensuring the stability of the catalyst's structure, the overall catalytic activity and stability can be effectively maintained. Tianze Wu and coworkers conducted a study to investigate the surface reconstruction of CoAl2O4 by partially substituting Fe.154 Their findings revealed that the introduction of Fe substitution facilitated and fine-tuned the surface reconstruction process. The O 2p energy level was identified as an influential factor in surface reconstruction. The incorporation of Fe led to an uplift of the O 2p band center, which in turn promoted the formation of vanadium–oxygen
species in spinel oxides and more favorably the oxidation of lattice oxygen in CoFe0.25Al1.75O4 (Fig. 11b). The participation of lattice oxygen species played a crucial role in accelerating the reconstruction process. Additionally, the leaching of Al was found to be closely associated with the reconstruction process. In the case of CoFe0.25Al1.75O4, the formation of Al vacancies after reconstruction process resulted in a decrease in the energy of the O 2p level, which induces the oxidation of lattice oxygen ceased, and the reconstruction process came to a halt, as no further
species were created (Fig. 11c). This termination mechanism contributed to the robustness of CoFe0.25Al1.75O4 as a catalyst, as it exhibited stable surface chemistry after reconstruction. Li et al. reported the Fe doping strategy in Ni3S2 Electrode.155 Incorporation of Fe inhibited S dissolution and facilitates the catalyst reconstruction. The incorporation of residual S in a newly reconstructed phase of NiOOH induced a longer Ni–O bond, which may correspond to a more open NiOOH lattice, yielding more facile transport to and from OER active sites (Fig. 11d). As shown in Fig. 11e, this tuned reconstructed phase with residual S showed remarkable stability for 3500 h at 100 mA cm−2.
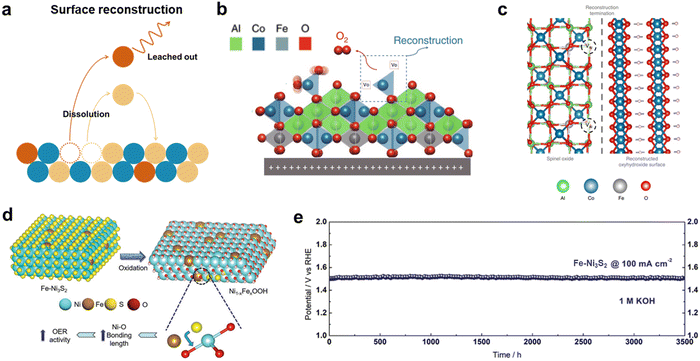 |
| Fig. 11 (a) Schematic presentation of dissolution–redeposition processes through surface reconstruction. (b) Surface reconstruction mechanism for CoFe0.25Al1.75O4 with lattice oxygen participation in OER process. (c) Schematic illustration of CoFe0.25Al1.75O4, which terminates its surface reconstruction due to the termination of Al vacancies. Reproduced with permission.154 Copyright 2019, Springer Nature. (d) Schematic illustration of the phase transformation with residual S and the influence of the residual S. (e) Stability test of Fe-Ni3S2 at a constant current density of 100 mA cm−2. Reproduced with permission.155 Copyright 2022, Wiley. | |
The dissolution process of the stable-phase catalysts during electrochemical reactions is referred to as steady-state dissolution (Fig. 12a). For example, the dissolution of Fe from Fe (oxy)hydroxide and NiFe oxide forming soluble FeO42− species has been observed due to anodic polarization in alkaline electrolytes. This dissolution process mainly causes the loss of active sites and degradation of catalytic performances. The research conducted by Qing Jiang and their team involved the fabrication of FeCo/CeO2−xNx laminate composite electrodes using alloying/dealloying of lamella nanostructured eutectic intermetallic compounds, followed by a thermal nitridation procedure (Fig. 12b).156 During the rigorous oxygen evolution reaction (OER), Fe dissolution is an unavoidable process in FeCo/CeO2−xNx. However, the dissolution rate of Fe in nanoporous FeCo/CeO2−xNx was found to be approximately 0.477 μg h−1, even at a high current density of approximately 1900 mA cm−2. The unique lamella nanostructure of the FeCo/CeO2−xNx composite electrode exhibited exceptional durability, maintaining a consistently high current density of approximately 1900 mA cm−2 over a period of 400 hours (Fig. 12c). In sharp contrast, nanoporous FeCo/Ce–O and FeCo electrodes exhibited a significant reduction in current densities over 100 hours, accompanied by higher dissolution rates of Fe (∼0.782 μg h−1 and ∼2.412 μg h−1, respectively). This difference in performance can be attributed to the electron transfer occurring at the heterointerface between CeO2−xNx and CoFeOOH, which substantially stabilizes the chemical states of the Fe component. This stabilization mechanism effectively alleviates the Fe leaching typically caused by the formation of soluble FeO42− species in the OER environment.
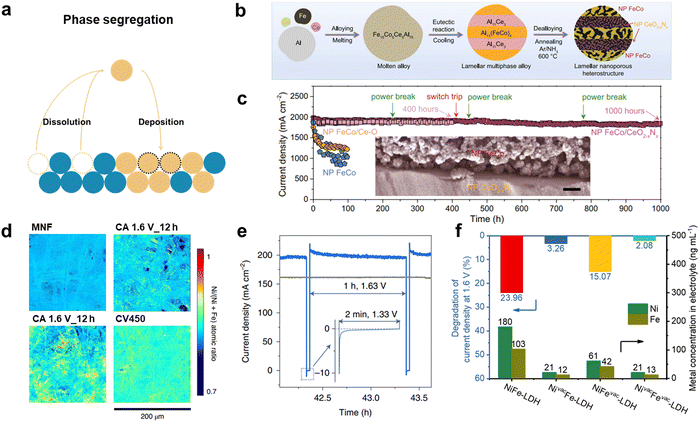 |
| Fig. 12 (a) Schematic presentation of phase segregation process. (b) Schematic diagram to illustrate fabrication procedure of lamellar nanoporous (NP) FeCo/CeO2−xNx electrode. (c) Stability test of NP FeCo/CeO2−xNx electrode at 1.54 V versus RHE for 400 and 1000 h, respectively. Reproduced with permission.156 Copyright 2023, Springer Nature. (d) Ni/(Ni + Fe) atomic ratio mapping of pristine mixed NiFe hydroxide (MNF), MNF after 12 h and 18 h of CA measurement at 1.6 VRHE, and after 450 CV cycles in the range 1.2–1.8 VRHE. (e) Continuous CA measurement (green line) leads to more current loss than the CA measurement with reduction protocol for 2 min (blue line). Reproduced with permission.157 Copyright 2020, Springer Nature. (f) The activity loss and metal dissolution in vacancy-engineered NiFe-LDH. Reproduced with permission.158 Copyright 2021, Wiley. | |
Another important aspect to consider is the influence of dissolved catalysts or ions in the electrolyte on catalytic performance, as well as their potential for redeposition during electrochemical reactions (Fig. 12a). Dissolution and redeposition processes play a crucial role in the long-term durability of catalysts, particularly due to phase segregation phenomena. Feng Lin and colleagues observed the development of Fe segregation in the Ni–Fe hydroxide host lattice during OER, leading to the formation of FeOOH and the subsequent interface between FeOOH and the host lattice.157 Through the use of X-ray fluorescence microscopy (XFM), it was revealed in Fig. 12d that the surface of the pristine mixed nickel–iron (MNF) catalyst underwent a phase segregation process after continuous 12 and 18 hours of chronoamperometric (CA) operation. In contrast, even after 450 cycles of cyclic voltammetry (CV), no obvious phase segregation was observed. This finding suggests that the intermittent electrochemical reduction protocol applied to the MNF catalyst could effectively reverse the phase segregation and extend the catalyst's lifetime (Fig. 12e). The application of the intermittent reduction protocol led to a significant improvement in catalytic performance for both thin and bulk MNF catalysts. For the thin MNF catalyst, a reduction step of 2 minutes at 1.33 V after every 1 hour of CA measurement at 1.63 V resulted in complete recovery of the catalytic performance. Such approaches offer promising avenues for enhancing the durability and stability of catalysts in various electrochemical applications. Peng et al. have proposed an effective strategy for suppressing dissolution and phase segregation in NiFe LDH by introducing cation vacancies in the basal plane.158 This approach enhances the binding energy between the metals and oxygen in the LDH sheets, while reducing lattice distortions. The rational introduction of cation vacancies significantly mitigates the dissolution of metals from NiFe-LDH under high oxidation potentials, leading to improved long-term stability during OER (Fig. 12f). DFT calculations demonstrated that the presence of cation vacancies increased the dissolution energies of metal atoms, particularly those located at edge sites. Consequently, the overall dissolution of metals and the undesired phase segregation resulting from selective dissolution–redeposition processes are suppressed.
4. Safety issues
Gas crossover is a noteworthy phenomenon observed in alkaline water electrolysis, wherein oxygen gas generated at the anode permeates through a porous diaphragm and traverses to the cathode, and vice versa. This crossover of gases poses significant safety concerns. When O2 and H2 gases combine, they can form an explosive mixture given specific conditions. This flammability range, commonly referred to as the explosive limits or flammability limits, delineates the concentration boundaries within which the mixture can ignite and sustain combustion. Typically, the explosive range of the O2–H2 gas mixture spans from 4% to 94% H2 by volume, with the remaining composition being O2. If an ignition source is present, the mixture can ignite and give rise to an explosion. Consequently, it is imperative to ensure that the presence of unnecessary crossover gases at the electrodes is maintained below the explosive range to mitigate risks. Another issue stemming from gas crossover is the occurrence of “reverse voltage,” which pertains to an unintentional reversal of the applied voltage polarity, resulting in a reversal of the current flow within the electrolysis cell.159,160 This phenomenon has the potential to disrupt the intended electrochemical reactions at the electrodes, leading to adverse effects on the efficiency of hydrogen and oxygen gas production, as well as introducing safety hazards or system damage.161 Few studies have been conducted to prevent oxygen gas crossover by the design of the electrode. Nevertheless, the development of such technology is crucial for achieving stable and widespread implementation of alkaline electrolysis. In this section, we will elucidate a study that has addressed safety concerns through the advancement of electrocatalysts.
4.1 Decoupled active site
Given the inherent presence of gas crossover in alkaline water electrolysis, which arises from the porous nature of the diaphragm, the most straightforward approach to prevent gas mixing-induced explosions is to eliminate the gas that permeates the diaphragm. One potential solution involves the removal of oxygen through ORR at the cathode. The cathode's operation in a reductive environment below 0 VRHE provides an opportunity to facilitate the removal of O2 gas through the ORR, which thermodynamically occurs below 1.23 VRHE. Consequently, if the electrocatalyst employed at the cathode exhibits exceptional ORR activity, it becomes feasible to prevent gas mixing beyond the explosion range at the cathode. By effectively curbing the presence of O2 gas through efficient ORR catalysis, the risks associated with gas crossover and the formation of explosive mixtures can be mitigated. However, unintended ORR at the cathode can have several detrimental effects on the electrolysis process Firstly, ORR competes with HER, leading to decreased efficiency in H2 production. This results in lower efficiency of overall electrolysis performance and reduced H2 generation rates. Furthermore, ORR at the HER electrode can cause additional voltage losses and reverse voltage. This can lead to increased energy consumption and undesired electrochemical reactions, impacting the stability and durability of the electrolysis system. Reverse voltage in polymer electrolyte membrane (PEM) fuel cell operation has been observed due to fuel starvation at the anode, leading to the corrosion of carbon components such as catalyst support, gas diffusion layer (GDL).162,163 To mitigate electrode damage caused by reverse voltage, a recent study proposed a novel approach that involves selectively promoting hydrogen oxidation reaction (HOR) by suppressing ORR and introducing OER electrocatalyst at the anode of the PEM fuel cell.164 The incorporation of OER electrocatalyst is beneficial as it competes with the carbon corrosion reaction. This study highlights the potential of multifunctional catalysts as a promising strategy to address dynamic electrochemical reactions, particularly in scenarios characterized by voltage reversal, as observed in water electrolysis. Based on this, therefore, to solve this problem, our research group fabricated a multifunctional electrocatalyst with decoupled active site to remove O2 gas at the cathode by implanting ORR activity into the HER catalyst (Fig. 13a).29 By incorporating pyridinic N, which is known to be ORR-active, into the NiFeP catalyst containing Niδ+ sites that are HER-active, an enhancement in the catalytic activity for ORRs at HER electrode was achieved.165,166 The pyridinic N is incorporated into in situ growth of zeolite imidazole framework (ZIF) onto NiFeP and subsequent pyrolysis at Ar atmosphere, forming core–shell structure, where tens-of-nanometer-sized NiFeP particles were encapsulated by N-doped carbon shell. The carbon shell on the surface of NiFeP facilitates the electron transfer for enhancing HER and suppressed the unintended oxidation of NiFe P due to O2 crossover. By altering the purging gas and analyzing chronopotentiometry, the gas crossover atmosphere is implemented and the reactivity of ORR and the issue of HER catalyst performance degradation due to ORR can be assessed (Fig. 13b and c). Upon O2 gas purging, the raised voltages above 0 VRHE indicates that the ORR is thermodynamically prioritized over HER. After ORR with O2 gas purging, increased HER overpotential by 36 mV was observed in pristine NiFe P due to unintended oxidation. On the contrary, only a 4 mV increase was exhibited in NiFeP@Ni_NC (Fig. 13c), confirming that the introduction of pyridinic N effectively mitigated the degradation of catalysis caused by unintended ORRs by the formation of decoupled active sites.
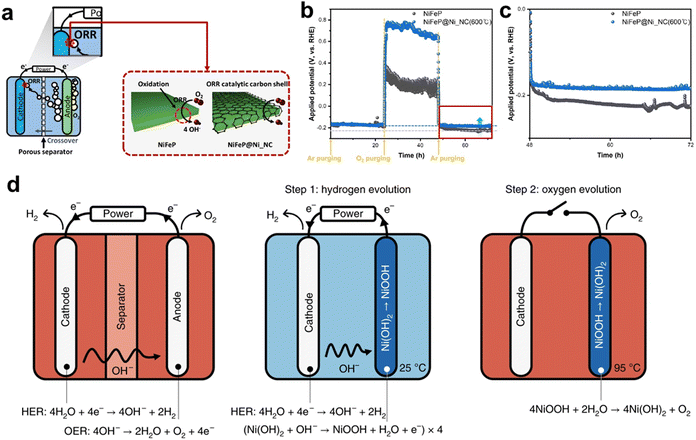 |
| Fig. 13 (a) Schematic illustration of the oxidation process of NiFeP and NiFeP@Ni_NC at the cathode driven by oxygen gas crossover in alkaline electrolysis. (b) Chronopotentiometry designed for evaluation of the stability against oxidation induced by oxygen gas crossover. (c) Enlarged view of the red box in Fig. 13b. Reproduced with permission.29 Copyright 2021, Elsevier. (d) Typical alkaline water electrolysis, which typically takes place at elevated temperatures (50–80 °C) and electrochemical-thermally activated chemical (E-TAC) water splitting proceeds in two consecutive steps. Reproduced with permission.169 Copyright 2019, Springer Nature. | |
4.2 Decoupled electrolysis
Decoupled electrolysis presents an effective approach to prevent gas crossover by avoiding the simultaneous generation of H2 and O2 in the same location. This strategy involves the use of solid-state decoupling agents as auxiliary electrodes in decoupled electrolysis cells. Among these agents, Ni(OH)2 has gained significant attention due to its exceptional stability in alkaline environments.167,168 Gideon S. Grader research group, for instance, reported decoupled water electrolysis reactions by dividing the process into two steps (Fig. 13d).169 The first step involves an electrochemical process where water is reduced at the cathode and oxidized at the anode. This is followed by a spontaneous chemical step, which occurs at a faster rate at higher temperatures and facilitates the anodic regeneration by oxidizing water. In this scheme, HER remains unchanged as it occurs at the cathode. This decoupled electrolysis modifies OER at the anode. In this process, water is reduced to hydrogen gas at the cathode, resulting in the liberation of OH− ions. The conventional four-electron OER, which typically takes place at the anode in traditional electrolysis, is transformed into a series of consecutive one-electron oxidation reactions of nickel hydroxide (Ni(OH)2) anode. Subsequently, spontaneous oxygen evolution and anode regeneration occur in a thermally activated chemical step. This two-step electrochemical-thermally activated chemical (E-TAC) cycle enables the formation of the O–O bond during the second step. In this step, the oxidized anode of NiOOH is spontaneously reduced to Ni(OH)2, resulting in the generation of O2 and the regeneration of the anode. As a result, the overall water-splitting reaction is completed. Through this E-TAC process, a current density of 200 mA cm−2 was achieved at 1.6 V, and the system demonstrated stability during 100 consecutive cycles at a nominal current density of 50 mA cm−2. Gideon S. Grader research group improved the performance through the introduction of electrospun core/shell Ni/Ni(OH)2 nanofibers with a high surface area at anode.170 The E-TAC performance was further increased to 113 mA cm−2 at 1.48 VRHE with nearly 100% Faraday efficiency. This decoupled electrolysis with separate HER and OER steps has several advantages. Firstly, this approach prevents the mixing of product gases over a range of current densities, leading to improved gas handling and increased operational flexibility. This feature makes alkaline electrolytic cells well-suited to be powered by sustainable energy sources. Secondly, the absence of a membrane in this device architecture allows for the production of highly pure H2 and O2. This eliminates the need for additional purification steps, thereby reducing the overall cost of alkaline water electrolysis technology. Lastly, the separate production of H2 and O2 requires different driving voltages or power inputs. This implies that sustainable energy sources, such as solar or wind power, can be flexibly utilized based on the varying output of these unstable power sources. This adaptability enables efficient utilization of renewable energy for either H2 or O2 production, depending on the availability and fluctuation of the sustainable energy sources.
5. Next-generation alkaline water electrolysis
Despite concerted efforts to mitigate the drawbacks of alkaline water electrolysis, numerous challenges persist that are not readily addressable via advancements in catalyst alone. Providing large quantities of limited freshwater resources for electrolysis can be challenging, and regional disparities may render commercial water electrolysis impractical in arid area.171 Desalination technologies are intensely studied and currently under development to convert seawater into usable fresh water,172–175 however, additional costs will inevitably deteriorate the economic viability of AWE. The utilization of liquid electrolytes also causes a set of issues. The operational current density is low (<0.5 A cm−2), and impurities, such as water vapor, compromise the purity of the produced hydrogen.176 Moreover, the inability to produce pressurized hydrogen exacerbates challenges related to hydrogen storage and transportation.177 Furthermore, the inflexible nature of power load alterations impedes the ability to promptly respond to renewable energy load fluctuations.178 To tackle these problems, alternative water electrolysis systems are being developed. The two most promising alternatives are seawater electrolysis and AEMWE. Seawater electrolysis can significantly enhance the cost-effectiveness of AWE by utilizing seawater as an electrolyte, although potential side reactions triggered by anions such as Cl− in seawater pose challenges.179 AEMWE provides a solution to the issues posed by liquid electrolytes via the deployment of membrane electrolytes, although this technology is still in the early stages. This chapter will discuss the research progress and challenges of these two alternative technologies.
5.1 Seawater electrolysis
Seawater, comprising 96.5% of global water resources, holds great potential as an electrolyte for water electrolysis. Utilizing seawater as an electrolyte for water electrolysis could significantly augment the cost-effectiveness of electrolysis systems, mitigating the requirement for a desalination procedure. Additionally, when integrated with a fuel cell, it could supplement fresh water in regions experiencing freshwater scarcity. The conductivity of seawater (5 S m) vastly surpasses that of pure water (5.5 × 10−6 S m), underscoring its utility as an electrolyte. Furthermore, electrolysis systems situated along coastlines could be more easily integrated with marine renewable energies.171 However, saltwater electrolysis can corrode catalyst electrodes due to the dissolved Cl− in the electrolyte, and chlorine evolution reaction (CER) can generate toxic Cl2 gas in acidic electrolytes, while the production of hypochlorite can accelerate the corrosion of catalyst electrodes in neutral and alkaline electrolytes.179 Therefore, to address these issues, many researchers focus on theoretical and empirical exploration. The Strasser research group utilized a theoretical framework to discern the optimal conditions for seawater electrolysis.180 A variety of reactions are possible depending on the pH and the concentration of chloride ions. Fig. 14a displays the Pourbaix diagram of aquatic chlorochemistry at a Cl− concentration of 0.5 M which is similar to the Cl− concentration in seawater. In an acidic solution, a CER can occur, | 2Cl− = Cl2 + 2e−E0 = +1.36 VSHE | (4) |
which competes with the OER under the potential region of E > 1.36 VSHE. Despite the OER being thermodynamically more favorable, the faster reaction kinetics of the CER may be predominant, attributed to being a two-electron reaction with a single reaction intermediate. In contrast, in an alkaline environment with pH > 7.5, the formation of hypochlorite must be considered: | 2Cl− + 2OH− = ClO− + H2O 2e− | (5) |
| E0 = +0.89 VSHE at pH 14 | (6) |
pH 7.5 is the pKa of hypochlorous acid, below which the formation of hypochlorous acid becomes dominant over hypochlorite. The standard electrode potential of hypochlorite formation is pH dependent, akin to the OER, resulting in a fixed potential difference between the OER and hypochlorite formation at approximately 0.480 V in an alkaline medium. If the electrocatalyst operates at an overpotential (η) lower than this value, hypochlorite formation does not occur, and the OER does not compete with the faster CER. In an acidic medium, the smaller potential difference between the CER and OER and unfavorable kinetic for OER than in alkaline electrolytes obstruct achieving high current densities at electrode potentials where the CER is thermodynamically impossible (Fig. 14b). Therefore, an alkaline environment is most suitable for seawater oxidation.180 Equilibrium potential of OER and Cl− redox reaction is dependent on the catalyst, and this deviation originates from differences in the activation energy barriers.181 Given that OER generally exhibits slower kinetics than Cl− redox reaction, the actual OER-only range, as seen in Fig. 14c, is approximately 200 mV for IrO2.182–184 High current density operation within a potential range of 200 mV is a challenge. Also, the extended operation may result in diminished catalyst activity which could increase the voltage required for seawater electrolysis, reaching a potential area where Cl− can react. Therefore, it is necessary to devise a catalyst with high corrosion resistance to Cl−, alongside utilizing wider thermodynamic potential ranges. Xiaoxin Zou group developed a catalyst, consisting of an OER-active oxidized NiFeBx layer on the surface, a NiFeBx intermediate layer, and a NiFe alloy substrate (Fig. 14d).185Via DFT calculations, a wide OER-only potential range of (Ni, Fe)OOH was revealed due to weak Cl− adsorption. Further, corrosion resistance of the borate layer was demonstrated by chronopotentiometry analysis, exhibiting stable operation for over 100 h at a high current density of 500 mA cm−2 in a 30 wt% KOH + 0.5 M NaCl electrolyte and OER faradaic efficiency of 100%. In addition, the corrosion potential of the catalyst was higher than that of NiFe, and the corrosion current was smaller than NiFe.
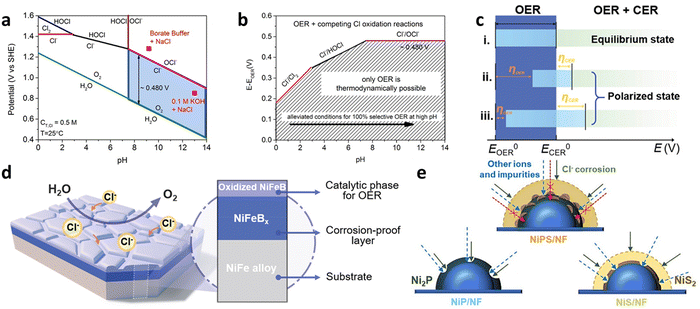 |
| Fig. 14 (a) The Pourbaix diagram exhibiting the possible reactions for seawater electrolysis. The temperature and the concentration of Cl− is illustrated. The region with 0.480 V of OER-only potential is highlighted with the blue shade. (b) Illustration of the theoretical maximum overpotential only allowing OER. Reproduced with permission.180 Copyright 2016, Wiley. (c) Scheme outlining (light-blue) the OER-only potential windows under (i) equilibrium and (ii) (iii) polarized state where Cl− is present in aqueous environments. (d) A visual representation of MOEE. Reproduced with permission.185 Copyright 2021, Wiley. (e) Scheme depicting the increased stability of NiPS/NF in OER with seawater as electrolytes. Reproduced with permission.187 Copyright 2022, Elsevier. | |
Sulfide electrocatalysts can form multivalent anions that block and repel Cl− from the surface.186 Zhong-Yong Yuan and researchers synthesized a Ni2P/NiS2 (NiPS) catalyst to prevent corrosion due to Cl− in seawater electrolysis (Fig. 14e).187 NiS2 can greatly enhance corrosion resistance against seawater by forming multivalent anions that repel Cl−, while Ni2P forms a stable, compact layer on the catalyst surface, enhancing resistance to other ions than Cl− and impurities in seawater. NiPS/NF maintained stable seawater electrolysis operation for over 60 h at a current density of 200 mA cm−2 in alkaline seawater electrolyte. Utilizing multivalent anion layers to enhance Cl− corrosion resistance may impose restrictions on the choice of potential catalyst materials. Liang Chen and co-workers were able to enhance Cl− corrosion resistance using multivalent anion layers without severely limiting the selection of catalyst materials by employing extremely cost-effective sulfate salts as an additive (Fig. 15a).188 Higher the concentration of Na2SO4 was, the longer NF operated stably (Fig. 15b). Further, Ecorr, Icorr, and Rct were significantly reduced after the addition of Na2SO4, implying the corrosion of the catalyst electrode due to Cl− was suppressed by adding SO42− additives to the electrolyte. SO42− adsorbed on the catalyst surface pushes out Cl− and mitigates the corrosion process. In molecular dynamics (MD) simulations, Cl− tended to accumulate abundantly over the electrode surface in the absence of SO42− (Fig. 15c). After introducing Na2SO4 into the electrolyte, despite sulfate concentration being much lower than that of Cl−, divalent SO42− preferred to adsorb onto the electrode surface. Consequently, electrical repulsion pushes Cl− away from the electrode surface. The ion distribution demonstrated that SO42− presence hindered Cl− from approaching within 0.5 nm of the electrode surface and diminished the amount of Cl− within 1 nm (Fig. 15d). OH− quantity within 1 nm remained unaffected, indicating that OER activity would not be impeded by the inclusion of SO42− additives, which could be attributed to the strong hydrogen bonding force between OH− and the NF surface.189 The researchers validated the MD results with the results of ion chromatography and potentiometric titration systems. The amount of attached Cl− decreased by 47% due to the addition of SO42−, while OH− decreased by 7%. Therefore, sulfate was successfully employed as an additive to enhance the seawater electrolysis stability of the catalyst by repelling Cl− away from the catalyst surface without severely limiting the pool of catalyst materials.
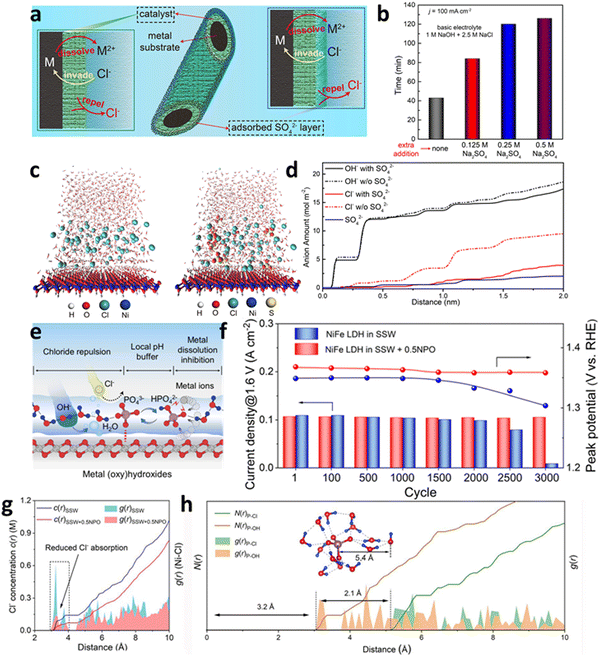 |
| Fig. 15 (a) Schematic illustration of the optimized catalysts (left) and electrolytes (right) for protecting the metal substrate from Cl− corrosion. (b) The stability of NF in the electrolytes with different Na2SO4 concentration. (c) Visualized MD simulations of electrolyte on the surface of the catalysts. (d) The distribution of Cl−, OH−, and SO42− as a function of distance from the surface of the catalysts. Reproduced with permission.188 Copyright 2022, Elsevier. (e) Graphical illustration of the anti-corrosion mechanism of network formed by H2O and adsorbed PO43− on the surface of the catalysts. (f) Current densities at the 1.6 VRHE and reduction peak potentials. (g) The radial distribution function of g(r) (Ni–Cl) and c(r) (Cl− concentration) as a function of the distance from the surface of the catalysts with and without 0.5NPO (0.5 M Na3PO4). (h) The g(r) and N(r) (integration) of P–Cl and P–O in SSW + 0.5NPO. Structure of hydrated PO43− interacting with OH− is depicted in inset. Reproduced with permission.190 Copyright 2022, Elsevier. | |
Intense research interests have been focused on the theoretical investigations to introduce an anion layer that can more effectively repel Cl− during seawater electrolysis. Fangyi Cheng suggested that the PO43− is the most suitable anion for efficient and stable seawater electrolysis.190 The PO43− and H2O adsorbed on the catalyst surface form a complex hydrogen bond network as illustrated in Fig. 15e, which is beneficial for the delivery of OH−. On the other hand, because Cl− is a weak hydrogen bond acceptor, the coulomb repulsion between PO43− and Cl− becomes dominant, effectively suppressing the diffusion of Cl− in the network. Furthermore, PO43− has high electrochemical stability and stabilizes metal cations. Also, the conversion process between PO43− and HPO42− serves as a local buffer, compensating for pH decreases under high current densities and further improving electrode stability. OER performance begins to deteriorate from 500 cycles when phosphate ions are absent, while no performance degradation was observed in electrolyte with phosphate ions (Fig. 15f). A significant correlation was observed between the peak potential and the decrease in current density. The shift in the reduction peak was attributed to the enhancement of the reducibility of Ni due to the introduction of neighboring Fe.191,192 Thus, the dissolution or phase separation of Fe due to Cl− led to a decrease in current density. In MD simulations, the approach of Cl− was inhibited when phosphoric acid was added, due to the electrical repulsion from the adsorbed PO43− (Fig. 15g). According to the graph shown in Fig. 15h, the minimal distance between OH− and PO43− is much closer than P–Cl and smaller than the radius of the hydrated PO43−. Therefore, the PO43− exhibits a more distinct repulsion against Cl− compared to the OH−. This could be due to the formation of hydrogen bonds between the hydrated PO43− and surrounding OH− (inset of Fig. 15h). Therefore, the integration of PO43− inhibits the adsorption of Cl−, and due to the weak repulsion with OH−, thus, electrode corrosion decreases while catalyst activity is maintained.
However, introducing additives incurs additional costs193 and potentially leads to unexpected side reactions.194 Jin et al. presented a strategy to enhance the activity of the catalyst by introducing a Lewis acid layer on the catalyst surface, thereby engineering the local reaction environment.179 The surface of Cr2O3–CoOx exhibits a localized alkaline environment due to the in situ generation of OH−, preventing detrimental chlorine chemistry and leading to increased activity. The pH of the anode surface of Cr2O3–CoOx and CoOx varies with current density. The pH on the CoOx surface is lower than that of bulk seawater and decreases as the current density increases. In contrast, the pH on the Cr2O3–CoOx surface is higher than bulk seawater, initially increasing and then decreasing as current density increases, indicating the Cr2O3 layer can create a localized alkaline environment atop the catalyst. The resulting Lewis acid layer can generate a large amount of OH−, which participates in OER to form a stable electrical double layer and effectively resist Cl− migration even at high anodic potentials. In situ IR spectroscopy revealed that the locally generated OH− on the anode surface originates from the dissociation of water molecules adsorbed on Cr2O3 which has a smaller energy barrier for water dissociation compared to CoO2. This strategy of introducing a Lewis acid layer into the catalyst for seawater electrolysis enhances the activity and stability of the electrolysis system, suggesting a wide range of applicability for various catalysts.
Although the main issue of seawater electrolysis is preventing the reaction of Cl− at the anode, it is worth mentioning the research aimed at promoting HER. If HER efficiency improves, the overpotential applied to the electrolysis system is reduced, preventing the electrochemical reaction of Cl−. Qiao group has been paying attention to the ability of N atom to effectively tune the electronic structure of metal atoms with active sites in HER catalysts.195 Accordingly, a catalyst was reported prepared by an unsaturated nitridation process resulting in encapsulated nickel surface nitrides (Ni-SN@C) in a carbon shell as HER catalyst for seawater electrolysis.196 The highly selective surface nitridation of Ni-SN@C is attributed to the confinement provided by the carbon shell, preventing extended nitridation of Ni at high temperatures. The Ni of nickel surface nitride is metallic with unsaturated Ni–N bonding and charge redistribution, with no bulk nickel nitride phase detected. The reaction mechanism on the catalyst surface was elucidated by in situ Raman analysis using isotopes and Tafel slope analysis. Due to its unique electronic structure, Ni-SN@C promotes H2O adsorption and weakens H adsorption, generating hydronium ions during alkaline HER. The hydronium ions in Ni-SN@C originate from the binding of H* to water molecules under negative potential due to the saturated active sites with underpotentially deposited H. The creation of hydronium ions reduces the HER barrier, rendering the Heyrovsky step the RDS. In tests combining hydrazine oxidation at the anode to reduce cell potential, H2 was produced at a current density of 1 A cm−2 with a cell voltage of just 0.7 V. The two electrode systems also displayed excellent stability without significant current variations over 24 hours, and the H2 production rate was around 0.21 mL cm−2 min−1, recording a 100% faradaic efficiency.
5.2. Anion exchange membrane water electrolysis
Anion exchange membrane water electrolysis (AEMWE) employs a membrane that conducts OH− as an electrolyte in a water electrolysis system. During the AEMWE, water is converted to hydrogen and OH− after the HER at the cathode, and the generated OH− is delivered to the anode via the AEM, where it is oxidized to water and oxygen after the OER (Fig. 16a).197 AEMWE can produce high-purity H2 at high current densities owing to the use of a membrane, reduce the size of the electrolysis cell to increase volumetric energy density and operate under pressurized conditions.171 Moreover, it can implement low-concentration alkaline electrolyte or even pure water as the feed water, thus obviating the need for highly corrosion-resistant precious metal-based catalysts or hardware.177 However, AEMWE also has limitations making long-term operation challenging, such as catalyst particle agglomeration, catalyst detachment, and catalyst element leaching (Fig. 16b).198
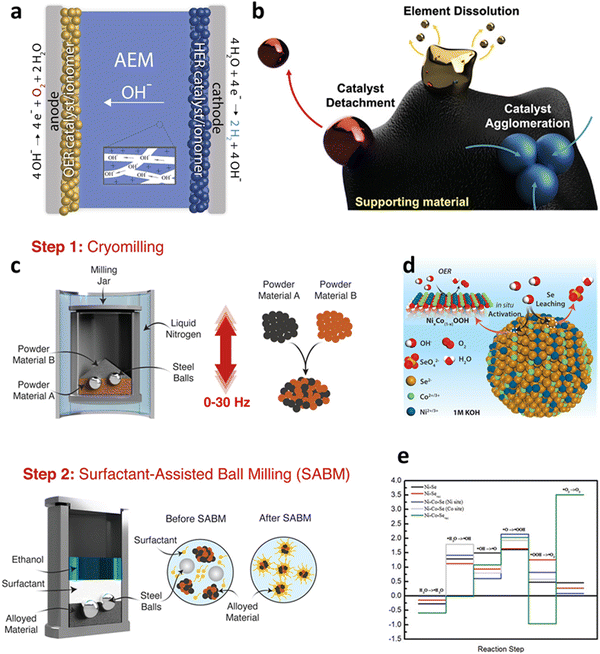 |
| Fig. 16 (a) Graphical illustration of AEMWE. Reproduced with permission.197 Copyright 2020, Elsevier. (b) Proposes degradation mechanisms of catalysts in AEMWE. Reproduced with permission.198 Copyright 2020, Wiley. (c) Illustration of the two-step synthesis process. Step 1: low-temperature milling to produce Ni–Co alloys. Step 2: SABM to modify the alloy particle size via implementing surfactant. (d) Scheme to depict the electrochemical activation process of Ni–Co–Se alloys. (e) The calculated potential energy of each reaction step for OER in Ni–Se, Ni–VSe (Sevac), Ni–Co–Se, and Ni–Co–VSe (101) plane. Reproduced with permission.201 Copyright 2021, Wiley. | |
According to the preceding report, a significant amount of Fe leaches out during OER of Ni, Fe-based catalyst in alkaline electrolyte.199 Therefore, Sargent and his co-workers presented (NiCo)3Se4 as an OER catalyst for AEMWE, aiming to replace Fe.200 The synthesis of (NiCo)3Se4 was accomplished through a two steps ball milling process followed by electrochemical oxidization of the surface (Fig. 16c and d). Low-temperature ball milling was used to prepare amorphous alloy particles, and high-temperature ball milling was used to control the size of the alloy particles while concurrently forming a surfactant on the particle surface. Then, referring to the previous research reporting the instability of metal selenides during OER in alkaline environments,201 the catalyst was synthesized by reconstructing the surface of (NiCo)3Se4 to an OER-active surface as Se leaches out through electrochemical oxidation. When the OER activity of oxidized (NiCo)3Se4 was evaluated utilizing an AEM electrolysis cell, the voltage required to generate a high current density of 2 A cm−2 was considerably low (2 V). The high OER activity of (NiCo)3Se4 was examined via DFT (Fig. 16e). For the OER to occur, two O atoms need to be adsorbed and subsequently desorbed. After leaching of Se atoms leaving Se vacancies
on the surface, O atom adsorption at
near Ni and Co was thermodynamically favorable. When an O atom is adsorbed on
, the second O atom also interacts with two metal atoms, thereby the O2 desorption energy was very high and was the rate-determining step (RDS) for OER. Therefore, the oxygen adsorbed on
forms an active oxide layer without O2 desorption. This study successfully replaced Fe with non-precious metal elements and provided the methodology for activating O atoms in the lattice.
AEMWE is technologically less mature than other low-temperature water electrolysis such as AWE or PEMWE, and thus, there also can be technical obstacles in components other than catalysts, such as ionomer and membrane.202,203 Therefore, issues arising in AEMWE are caused by complex factors, making it difficult to anticipate or identify limiting factors. Even the problems summarized in Fig. 16b are significantly affected by interactions between the catalyst and the support, as well as by the durability and characteristics of the support.198 The priorities set during the early stages of technology such as AEMWE often change as researchers come across breakthroughs. The subsequent part of this section emphasizes the dynamic aspects of AEMWE research, introducing cases where performance has been enhanced by addressing overlooked issues in AEMWE. Park group significantly improved electrochemical performance in AEMWE by engineering the porosity of the catalysts, thereby optimizing the gas–liquid–solid interface, which was not considered a limiting factor in AEMWE.204 Catalysts with different pore sizes were prepared by synthesizing a catalyst-template composite in a solution dispersed with silica templates of different particle sizes and subsequent leaching of silica particles in 5 M KOH (Fig. 17a and b). In an OER test, oxygen gas generated by OER blocked the catalyst surface, causing a rapid increase in potential (Fig. 17c). However, samples with larger pores took a longer time to experience the swift potential increase. Fig. 17d illustrates bubble escape behavior depending on the pore size. As reported earlier,205,206 faster bubble escape is possible in the catalysts with larger pores, since bubble escape in the catalysts with smaller pores can be delayed due to the interference of catalyst particles. The porous catalysts demonstrated a current density of 846.8 mA cm−2 at 1.8 V in AEMWE cell at 70 °C, significantly surpassing the catalysts without template. Consequently, this study accomplished significant performance improvements in AEMWE by controlling the gas–liquid–solid interface, which was overlooked as not being rate-limiting. Furthermore, controlling the morphologies of catalysts can regulate the concentration of OH− in the anode, creating a favorable environment for OER. Shuifen Xie et al. synthesized nanosheets, introducing Fe3+ which could etch Pd crystals to obtain subnanometer interlayer spacing (Fig. 17e and f).207 Confining the reaction channel to a subnanometer size increases the availability of the reactants and catalyst, which is advantageous for both HER and OER. In catalysts with confined structures, a high local OH− concentration can be obtained (Fig. 17g) as it was found through DFT that IrOx is the site which attracts OH− through DFT. However, in catalysts with open structures, no significant change in OH− concentration is observed, as the surface of the catalysts cannot maintain a high local OH− concentration due to the diffusion of OH− to the electrolyte. Nanosheets showed high activity while generating a high current density of 0.5 A cm−2 at the voltage of 1.6 V (Fig. 17h). No significant overpotential increase was observed for 40 hours in chronopotentiometry, when a constant current density of 0.5 A cm−2 was applied (Fig. 17i). This study enhanced the HER and OER activity of the catalysts in AEMWE by controlling the morphologies of the catalyst to confine the electrolyte.
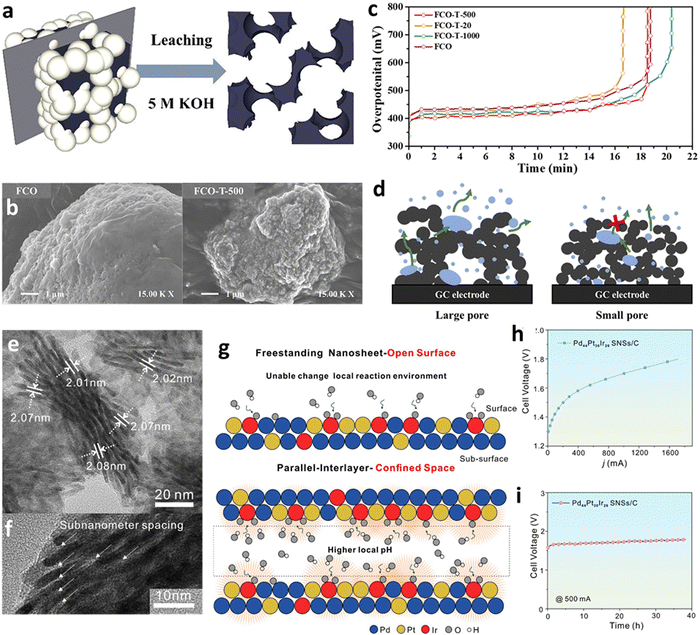 |
| Fig. 17 (a) Illustration of the process of preparing the catalysts with engineered pore size by silica template and leaching. (b) SEM images of FCO (Fe-doped Co3O4) and FCO-T-500. (c) CP curves at a current density of 20 mA cm−2 when RDE was not rotating. (d) Graphical illustration of bubble behavior in the catalysts with different pore sizes. Reproduced with permission.204 Copyright 2023, Elsevier. (e) TEM and (f) HR-TEM images of ASNSs (assembled supernanosheets). (g) Schematic illustration of the reactant behavior in (top) freestanding NSs and (bottom) ASNSs. (h) The LSV of an AEM electrolyzer and (i) Chronopotentiometry at 0.5 A of ASNSs at 80 °C. Reproduced with permission.207 Copyright 2022, American Chemical Society. | |
6. Summary and outlook
This review delves into the essential research strategies aimed at facilitating the commercialization of alkaline electrolysis. Unlike conventional studies that often assess electrocatalyst performance at low current densities (10 mA cm−2), engineering electrocatalysts for HCD operation in alkaline electrolysis mandates an alternative approach. This review expounds upon the design principles for electrocatalysts and outlines research strategies focused on augmenting activity during HCD operations within alkaline electrolysis. Such strategies encompass aspects such as charge transfer, mass transport, and intrinsic activity. Moreover, the review discerns degradation mechanisms and recent advancements that bolster stability in alkaline HCD, differentiating between mechanical and chemical aspects. In addition, a noteworthy concern is the pervasive issue of oxygen gas crossover, which directly impacts the safety of AWE. In response, the exploration of research avenues involving decoupled active sites and decoupled electrolysis is discussed. Furthermore, this review encompasses emerging trends in next-generation alkaline water electrolysis systems, encompassing seawater electrolysis and AEMWE. These innovations hold the potential to surmount prevailing limitations associated with AWE. However, despite the considerable strides in electrocatalyst development for AWE, the realization of industrial applications necessitates sustained endeavors in devising electrocatalysts capable of accommodating high current densities while maintaining stability. From an electrocatalytic systems perspective, this review highlights numerous challenges and prospects that warrant pursuit in future research endeavors.
(i) Most of the currently developed catalysts are “pre-catalysts,” which exhibit partial or complete reconstruction into actual active species contingent upon distinct conditions related to the HER, OER, or working condition (electrolyte, temperature, current density). Encompassing alterations in morphology, electronic attributes, as well as interfacial and bulk structure, in conjunction with the substrate–catalyst interrelationship within in situ states, holds the potential to furnish researchers with an enhanced comprehension of reconstruction process. This heightened understanding, in turn, can inform the judicious design of electrocatalyst that align more congruously with intended performance benchmarks. Nevertheless, it merits recognition that the phenomenon of surface reconstruction in electrocatalysts operating at current densities reaching the ampere scale is considerably more pronounced and rapid when contrasted with instances at lower current densities. Attaining efficacious dominion over this reconstruction process bears direct implications for refining the characteristics of real active sites. While the reconstruction dynamics of OER have garnered relatively more attention, it is imperative that commensurate consideration be extended to the HER reconstruction, which has garnered comparatively scant investigation. Given the disparate responses evinced by micro- and nanostructural attributes, crystal phases, and elemental composition across varying current densities, elaborate monitoring of the reconstruction process yields profound insights into the performance and stability aspects germane to elevated current densities. The development of in situ measurement methodologies tailored to interrogating the surface dynamics of electrocatalysts under the rigors of ampere-level HCD assumes significance.
(ii) A substantial body of research endeavors has been dedicated to the exploration of adsorption energy and free energy utilizing the configuration of pre-catalytic, within DFT calculations. Nonetheless, to ensure a level of computational accuracy commensurate with empirical reality, it becomes imperative to progress beyond the purview of calculations that exclusively involve the configuration of reconstructed active species. Instead, a rigorous approach necessitates the incorporation of computations encompassing the actual active layer subsequent to surface reconstruction, alongside a cognizant consideration of the dynamic interaction between the bulk and the active layer. Furthermore, it merits attention that the underpinning dynamic of HCD conditions precipitates a pronounced augmentation in oxygen and hydrogen coverage across the catalyst surface, thereby engendering a discernible influence upon the adsorption/desorption kinetics of reaction intermediates, and by extension, the intrinsic catalytic activity itself. Hence, to comprehensively encapsulate the mechanistic intricacies inherent to such catalytic systems, it becomes incumbent to undertake computation under conditions of heightened hydrogen and oxygen coverage, reflective of ampere-level current densities. This computational approach holds the promise of affording a more comprehensive and authentic representation of the intricate interaction of catalytic phenomena operating at HCD.
(iii) The profound elucidation of the foundational intricacies inherent to the process of water electrolysis has made rapid strides. However, the ongoing absence of precise mechanism underpinning catalyst deactivation, primarily arising from the intricate chemical alterations experienced by the catalyst during the course of electrocatalysis, underscores a critical gap. To this end, the intricate catalyst deactivation at a molecular level should be raveled with concerted employment of theoretical simulations and sophisticated in situ characterization methodologies. It is pivotal that forthcoming investigations encompass the dynamic process of active site dissolution and subsequent redeposition, offering invaluable insights into the phenomenon of surface reconstruction. In this vein, a cogent and strategic trajectory involves delving into the dissolution and redeposition dynamics of metal ions in the crucible of anodic potentials. Moreover, a nuanced comprehension of the segregation of active metal constituents stands as an avenue necessitating thoughtful interpretation in future studies. In essence, the holistic and systematic unraveling of these underlying mechanisms augments our grasp of catalyst dynamics and lays the groundwork for judicious strategies in advancing the efficiency and longevity of catalytic systems.
(iv) Furthermore, it is imperative to acknowledge the potential amplification of the dissolution–redeposition process, which could exert a heightened influence, particularly within batch systems or configurations featuring recyclable electrolytes. It is worth noting that this phenomenon might manifest differently within the context of an electrolyzer operating within a flow system, wherein the concentration of dissolved metal ions could be comparably lower, thereby potentially mitigating the extent of ion-mediated effects. that could catalyze advancements within the domain of catalytic stability. Up to now, scant attention has been directed toward establishing a coherent linkage between the stability performances observed at laboratory scales and those exhibited within commercial-scale implementations. An augmented understanding of the intricate interaction between lab-scale and commercial-scale stability outcomes holds the promise of furnishing insights that could catalyze advancements within the domain of catalytic stability.
(v) A lot of research has been done on improving efficiency and durability in terms of catalysts and systems, but gas crossover, which is the most problematic issue, has not been studied. Of course, separators are being developed to prevent gas crossover, but in terms of using porous separators, the dissolution of gas into the electrolyte and gas crossover must be a phenomenon that cannot be prevented. Therefore, research should be conducted to remove the dissolved gas from the electrode end. In addition, Decoupled water splitting is a new and growing field focused on exploring how separating the electrochemical oxygen- and hydrogen-evolution reactions can benefit electrolysis systems used for generating carbon-neutral hydrogen. Membrane separators have been replaced with cheaper alternatives or removed entirely, depending on the system. OER has been replaced with other value-added oxidation reactions, with mediators further implemented in completely modular electrochemical synthesis schemes. The intrinsic safety and operational flexibility of electrolysis have been substantially improved as well.
(vi) Considerable research efforts have been directed toward enhancing the efficacy and durability of catalysts and electrochemical systems. However, a conspicuous gap remains in the exploration of a vexing predicament – gas crossover. While strides have been made in developing diaphragm aimed at mitigating gas crossover, the utilization of porous diaphragm introduces a concomitant challenge: the inevitable occurrence of gas dissolution into the electrolyte and the subsequent phenomenon of gas crossover. Consequently, investigations should be undertaken to address the extraction of dissolved gas from the electrode interface. Furthermore, the emerging realm of decoupled water electrolysis has garnered increasing attention. This burgeoning field centers on dissecting how the segregation of electrochemical oxygen-evolution and hydrogen-evolution reactions, whether through spatial, temporal, or dual modalities, can confer advantages to electrolysis systems intended for carbon-neutral hydrogen generation. In this vein, membrane separators have yielded ground to cost-efficient alternatives or have been altogether eliminated, contingent upon the unique system configuration. Moreover, innovative approaches have seen the substitution of oxygen evolution with alternative value-added oxidation reactions, coupled with the integration of mediators within wholly modular electrochemical synthesis frameworks. This transformative trajectory has yielded marked enhancements in the intrinsic safety and operational adaptability of electrolysis processes. In this continuum of progress, however, the mitigation of the pervasive issue of gas crossover assumes a central role in realizing the full potential of these advancements.
(vii) Several noteworthy electrocatalysts have demonstrated commendable stability under HCD condition. However, a limitation hampering their broader application pertains to the inherent complexity and challenges associated with their synthesis procedures and cost, which tend to hinder their feasibility for large-scale production. This predicament is further compounded by the substantial dimension characteristic of electrodes operational within commercial alkaline electrolyzer. Conventionally employed laboratory techniques, such as solvent heat treatment and electrodeposition, generally fall short of achieving a uniform growth of catalysts across expansive electrode surfaces. Consequently, a proactive consideration of the scalability quotient within the experimental design becomes imperative. Mitigating the aforementioned challenges can be approached through the adaptation and enhancement of extant preparation methodologies and electrode constituents. This involves integrating laboratory-proven catalysts into electrodes via established commercial thermal spray processes. Simultaneously, the augmentation of commercial electrodes through subsequent processing to elevate performance represents a promising avenue. Beyond this, the pursuit of novel synthetic procedures holds promise in realizing large-scale production. Wet chemistry techniques and three-dimensional (3D) printing technologies emerge as potentially facile and scalable routes for catalyst synthesis. In tandem with considerations of synthesis scalability, economic and environmental evaluations are essential for the viable commercialization of catalysts. Attaining economic viability mandates a thorough assessment of cost expenditures incurred during electrode preparation, coupled with meticulous scrutiny of environmental ramifications precipitated by production processes.
Conflicts of interest
There are no conflicts to declare.
Acknowledgements
This work was supported by the National Research Foundation of Korea (NRF) grant funded by the Korea government (MSIT) (no. 2022R1A5A1032539 and 2023R1A2C2004923).
References
- S. Fankhauser, S. M. Smith, M. Allen, K. Axelsson, T. Hale, C. Hepburn, J. M. Kendall, R. Khosla, J. Lezaun and E. Mitchell-Larson, The meaning of net zero and how to get it right, Nat. Clim. Change, 2022, 12, 15–21 CrossRef
.
- L. Rosa, D. L. Sanchez and M. Mazzotti, Assessment of carbon dioxide removal potential via BECCS in a carbon-neutral Europe, Energy Environ. Sci., 2021, 14, 3086–3097 RSC
.
- A. Millot, A. Krook-Riekkola and N. Maïzi, Guiding the future energy transition to net-zero emissions: Lessons from exploring the differences between France and Sweden, Energy Policy, 2020, 139, 111358 CrossRef CAS
.
- A. Ahmed, T. Ge, J. Peng, W.-C. Yan, B. T. Tee and S. You, Assessment of the renewable energy generation towards net-zero energy buildings: A review, Energy Build., 2022, 256, 111755 CrossRef
.
- G. Gowrisankaran, S. S. Reynolds and M. Samano, Intermittency and the value of renewable energy, J. Polit. Econ., 2016, 124, 1187–1234 CrossRef
.
- V. Panchenko, Y. V. Daus, A. Kovalev, I. Yudaev and Y. V. Litti, Prospects for the production of green hydrogen: Review of countries with high potential, Int. J. Hydrogen Energy, 2023, 48, 4551–4571 CrossRef CAS
.
- S. Atilhan, S. Park, M. M. El-Halwagi, M. Atilhan, M. Moore and R. B. Nielsen, Green hydrogen as an alternative fuel for the shipping industry, Curr. Opin. Chem. Eng., 2021, 31, 100668 CrossRef
.
- Z. Y. Yu, Y. Duan, X. Y. Feng, X. Yu, M. R. Gao and S. H. Yu, Clean and affordable hydrogen fuel from alkaline water splitting: past, recent progress, and future prospects, Adv. Mater., 2021, 33, 2007100 CrossRef CAS
.
- M. David, C. Ocampo-Martínez and R. Sánchez-Peña, Advances in alkaline water electrolyzers: A review, J. Energy Storage, 2019, 23, 392–403 CrossRef
.
- C. Xiang, K. M. Papadantonakis and N. S. Lewis, Principles and implementations of electrolysis systems for water splitting, Mater. Horiz., 2016, 3, 169–173 RSC
.
- S. Marini, P. Salvi, P. Nelli, R. Pesenti, M. Villa, M. Berrettoni, G. Zangari and Y. Kiros, Advanced alkaline water electrolysis, Electrochim. Acta, 2012, 82, 384–391 CrossRef CAS
.
- S. Grigoriev, V. Porembsky and V. Fateev, Pure hydrogen production by PEM electrolysis for hydrogen energy, Int. J. Hydrogen Energy, 2006, 31, 171–175 CrossRef CAS
.
- Q. Wu, Y. Wang, K. Zhang, Z. Xie, K. Sun, W. An, X. Liang and X. Zou, Advances and status of anode catalysts for proton exchange membrane water electrolysis technology, Mater. Chem. Front., 2023, 7, 1025–1045 RSC
.
- R. Phillips, A. Edwards, B. Rome, D. R. Jones and C. W. Dunnill, Minimising the ohmic resistance of an alkaline electrolysis cell through effective cell design, Int. J. Hydrogen Energy, 2017, 42, 23986–23994 CrossRef CAS
.
- Z. Abdin, C. Webb and E. M. Gray, Modelling and simulation of an alkaline electrolyser cell, Energy, 2017, 138, 316–331 CrossRef CAS
.
- M. M. Bakker and D. A. Vermaas, Gas bubble removal in alkaline water electrolysis with utilization of pressure swings, Electrochim. Acta, 2019, 319, 148–157 CrossRef CAS
.
- K. Zouhri and S.-y Lee, Evaluation and optimization of the alkaline water electrolysis ohmic polarization: exergy study, Int. J. Hydrogen Energy, 2016, 41, 7253–7263 CrossRef CAS
.
- J. Haverkort and H. Rajaei, Voltage losses in zero-gap alkaline water electrolysis, J. Power Sources, 2021, 497, 229864 CrossRef CAS
.
- Q. Kang, D. Lai, W. Tang, Q. Lu and F. Gao, Intrinsic activity modulation and structural design of NiFe alloy catalysts for an efficient oxygen evolution reaction, Chem. Sci., 2021, 12, 3818–3835 RSC
.
- E. Skúlason, V. Tripkovic, M. E. Björketun, S. Gudmundsdóttir, G. Karlberg, J. Rossmeisl, T. Bligaard, H. Jónsson and J. K. Nørskov, Modeling the electrochemical hydrogen oxidation and evolution reactions on the basis of density functional theory calculations, J. Phys. Chem. C, 2010, 114, 18182–18197 CrossRef
.
- Y. Chen, G. Yu, W. Chen, Y. Liu, G.-D. Li, P. Zhu, Q. Tao, Q. Li, J. Liu and X. Shen, Highly active, nonprecious electrocatalyst comprising borophene subunits for the hydrogen evolution reaction, J. Am. Chem. Soc., 2017, 139, 12370–12373 CrossRef CAS PubMed
.
- Z. Lei, P. Liu, X. Yang, P. Zou, A. Nairan, S. Jiao, R. Cao, W. Wang, F. Kang and C. Yang, Monolithic Nickel Cata Featured with High-Density Crystalline Steps for Stable Hydrogen Evolution at Large Current Density, Small, 2023, 2301247 CrossRef CAS
.
- C. Wang, T. Wang, J. Liu, Y. Zhou, D. Yu, F. Han, Q. Li, J. Chen and Y. Huang, Facile synthesis of silk-cocoon S-rich cobalt polysulfide as an efficient catalyst for the hydrogen evolution reaction, Energy Environ. Sci., 2018, 11, 2467–2475 RSC
.
- R. Qi, X. Gao, J. Lin, Y. Song, J. Wang, Y. Qiu and M. Liu, Pressure control strategy to extend the loading range of an alkaline electrolysis system, Int. J. Hydrogen Energy, 2021, 46, 35997–36011 CrossRef CAS
.
- H. In Lee, D. T. Dung, J. Kim, J. H. Pak, S. K. Kim, H. S. Cho, W. C. Cho and C. H. Kim, The synthesis of a Zirfon-type porous separator with reduced gas crossover for alkaline electrolyzer, Int. J. Energy Res., 2020, 44, 1875–1885 CrossRef
.
- N. Armaroli and V. Balzani, The hydrogen issue, ChemSusChem, 2011, 4, 21–36 CrossRef CAS PubMed
.
- M. T. Janicke, H. Kestenbaum, U. Hagendorf, F. Schüth, M. Fichtner and K. Schubert, The controlled oxidation of hydrogen from an explosive mixture of gases using a microstructured reactor/heat exchanger and Pt/Al2O3 catalyst, J. Catal., 2000, 191, 282–293 CrossRef CAS
.
- H. Becker, J. Murawski, D. V. Shinde, I. E. Stephens, G. Hinds and G. Smith, Impact of impurities on water electrolysis: a review, Sustain, Energy Fuels, 2023, 7, 1565–1603 CAS
.
- S. Choi, J. Kwon, S. Jo, S. Kim, K. Park, S. Kim, H. Han, U. Paik and T. Song, Highly efficient and stable bifunctional electrocatalysts with decoupled active sites for hydrogen evolution and oxygen reduction reactions, Appl. Catal., B, 2021, 298, 120530 CrossRef CAS
.
- R. Zhao, Q. Li, X. Jiang, S. Huang, G. Fu and J.-M. Lee, Interface engineering in transition metal-based heterostructures for oxygen electrocatalysis, Mater. Chem. Front., 2021, 5, 1033–1059 RSC
.
- L. Lin, M. Chen and L. Wu, Hierarchical MoP/NiFeP hybrid hollow spheres as highly efficient bifunctional electrocatalysts for overall water splitting, Mater. Chem. Front., 2021, 5, 375–385 RSC
.
- J. Kwon, H. Han, S. Choi, K. Park, S. Jo, U. Paik and T. Song, Current Status of Self-Supported Catalysts for Robust and Efficient Water Splitting for Commercial Electrolyzer, ChemCatChem, 2019, 11, 5898–5912 CrossRef CAS
.
- J. Li, X. Feng, W. Zhong, M. Zhou, M. Qian, J. Xu, H. Pang, L. Xu, J. Yang and Y. Tang, Engineering Co/Co2P Mott-Schottky heterostructure encased in hollow N-doped carbon polyhedron as an advanced nanoreactor towards high-efficiency electrocatalytic oxygen evolution, Mater. Chem. Front., 2023, 7, 3641–3649 RSC
.
- K. Park, J. Kwon, S. Jo, S. Choi, E. Enkhtuvshin, C. Kim, D. Lee, J. Kim, S. Sun and H. Han, Simultaneous electrical and defect engineering of nickel iron metal-organic-framework via co-doping of metalloid and non-metal elements for a highly efficient oxygen evolution reaction, Chem. Eng. J., 2022, 439, 135720 CrossRef CAS
.
- L. Giordano, B. Han, M. Risch, W. T. Hong, R. R. Rao, K. A. Stoerzinger and Y. Shao-Horn, pH dependence of OER activity of oxides: current and future perspectives, Catal. Today, 2016, 262, 2–10 CrossRef CAS
.
- H. Lyle, S. Singh, M. Paolino, I. Vinogradov and T. Cuk, The electron-transfer intermediates of the oxygen evolution reaction (OER) as polarons by in situ spectroscopy, Phys. Chem. Chem. Phys., 2021, 23, 24984–25002 RSC
.
- W. T. Hong, K. A. Stoerzinger, Y.-L. Lee, L. Giordano, A. Grimaud, A. M. Johnson, J. Hwang, E. J. Crumlin, W. Yang and Y. Shao-Horn, Charge-transfer-energy-dependent oxygen evolution reaction mechanisms for perovskite oxides, Energy Environ. Sci., 2017, 10, 2190–2200 RSC
.
- J. C. Papaioannou, G. S. Patermarakis and H. S. Karayianni, Electron hopping mechanism in hematite (α-Fe2O3), J. Phys. Chem. Solids, 2005, 66, 839–844 CrossRef CAS
.
- T. Satoh, R. Iida, T. Higuchi, Y. Fujii, A. Koreeda, H. Ueda, T. Shimura, K. Kuroda, V. Butrim and B. Ivanov, Excitation of coupled spin–orbit dynamics in cobalt oxide by femtosecond laser pulses, Nat. Commun., 2017, 8, 638 CrossRef PubMed
.
- Z. Li, J. Yang, Z. Chen, C. Zheng, L. Q. Wei, Y. Yan, H. Hu, M. Wu and Z. Hu, V “Bridged” Co O to Eliminate Charge Transfer Barriers and Drive Lattice Oxygen Oxidation during Water-Splitting, Adv. Funct. Mater., 2021, 31, 2008822 CrossRef CAS
.
- P. M. Bodhankar, P. B. Sarawade, G. Singh, A. Vinu and D. S. Dhawale, Recent advances in highly active nanostructured NiFe LDH catalyst for electrochemical water splitting, J. Mater. Chem. A, 2021, 9, 3180–3208 RSC
.
- K. R. Park, J. Jeon, H. Choi, J. Lee, D.-H. Lim, N. Oh, H. Han, C. Ahn, B. Kim and S. Mhin, NiFe layered double hydroxide electrocatalysts for an efficient oxygen evolution reaction, ACS Appl. Energy Mater., 2022, 5, 8592–8600 CrossRef CAS
.
- S. Y. Jung, K. M. Kim, S. Mhin, Y. K. Kim, J. H. Ryu, E. Enkhtuvshin, S. J. Kim, N. T. T. Thao, S. Choi and T. Song, Computational atomic-scale design and experimental verification for layered double hydroxide as an efficient alkaline oxygen evolution reaction catalyst, Int. J. Energy Res., 2022, 46, 11972–11988 CrossRef CAS
.
- A. Karmakar, K. Karthick, S. S. Sankar, S. Kumaravel, R. Madhu and S. Kundu, A vast exploration of improvising synthetic strategies for enhancing the OER kinetics of LDH structures: a review, J. Mater. Chem. A, 2021, 9, 1314–1352 RSC
.
- S. Nayak and K. Parida, Superactive NiFe-LDH/graphene nanocomposites as competent catalysts for water splitting reactions, Inorg. Chem. Front., 2020, 7, 3805–3836 RSC
.
- L. Du, G. Shi, Y. Zhao, X. Chen, H. Sun, F. Liu, F. Cheng and W. Xie, Plasmon-promoted electrocatalytic water splitting on metal–semiconductor nanocomposites: the interfacial charge transfer and the real catalytic sites, Chem. Sci., 2019, 10, 9605–9612 RSC
.
- P. Li, X. Duan, Y. Kuang, Y. Li, G. Zhang, W. Liu and X. Sun, Tuning electronic structure of NiFe layered double hydroxides with vanadium doping toward high efficient electrocatalytic water oxidation, Adv. Energy Mater., 2018, 8, 1703341 CrossRef
.
- H. Liao, G. Ni, P. Tan, Y. Liu, K. Chen, G. Wang, M. Liu and J. Pan, Borate narrowed band gap of nickel–iron layer double hydroxide to mediate rapid reconstruction kinetics for water oxidation, Appl. Catal., B, 2022, 317, 121713 CrossRef CAS
.
- S. Liu, J. Zhu, M. Sun, Z. Ma, K. Hu, T. Nakajima, X. Liu, P. Schmuki and L. Wang, Promoting the hydrogen evolution reaction through oxygen vacancies and phase transformation engineering on layered double hydroxide nanosheets, J. Mater. Chem. A, 2020, 8, 2490–2497 RSC
.
- S. Liu, H. Zhang, E. Hu, T. Zhu, C. Zhou, Y. Huang, M. Ling, X. Gao and Z. Lin, Boosting oxygen evolution activity of NiFe-LDH using oxygen vacancies and morphological engineering, J. Mater. Chem. A, 2021, 9, 23697–23702 RSC
.
- J. Dai, Y. Zhu, H. A. Tahini, Q. Lin, Y. Chen, D. Guan, C. Zhou, Z. Hu, H.-J. Lin and T.-S. Chan, Single-phase perovskite oxide with super-exchange induced atomic-scale synergistic active centers enables ultrafast hydrogen evolution, Nat. Commun., 2020, 11, 5657 CrossRef CAS PubMed
.
- H. J. Lee, S. W. Lee, H. Hwang, S. I. Yoon, Z. Lee and H. S. Shin, Vertically oriented MoS 2/WS 2 heterostructures on reduced graphene oxide sheets as electrocatalysts for hydrogen evolution reaction, Mater. Chem. Front., 2021, 5, 3396–3403 RSC
.
- W. Zhang, Y. Li and S. Peng, Template-free synthesis of hollow Ni/reduced graphene oxide composite for efficient H2 evolution, J. Mater. Chem. A, 2017, 5, 13072–13078 RSC
.
- J. Ding, R. Yue, X. Zhu, W. Liu, H. Pei, S. He and Z. Mo, Flower-like Co3Ni1B nanosheets based on reduced graphene oxide (rGO) as an efficient electrocatalyst for the oxygen evolution reaction, New J. Chem., 2022, 46, 13524–13532 RSC
.
- R. K. Singh, R. Kumar and D. P. Singh, Graphene oxide: strategies for synthesis, reduction and frontier applications, RSC Adv., 2016, 6, 64993–65011 RSC
.
- S. Wu, Z. Yin, Q. He, G. Lu, X. Zhou and H. Zhang, Electrochemical deposition of Cl-doped n-type Cu 2 O on reduced graphene oxide electrodes, J. Mater. Chem., 2011, 21, 3467–3470 RSC
.
- W. Song, P. Chen, J. Yan, W. Zhu and H. Ji, The tribological properties of reduced graphene oxide doped by N and B species with different configurations, ACS Appl. Mater. Interfaces, 2020, 12, 29737–29746 CAS
.
- L. K. Putri, B.-J. Ng, W.-J. Ong, H. W. Lee, W. S. Chang and S.-P. Chai, Heteroatom nitrogen-and boron-doping as a facile strategy to improve photocatalytic activity of standalone reduced graphene oxide in hydrogen evolution, ACS Appl. Mater. Interfaces, 2017, 9, 4558–4569 CrossRef CAS PubMed
.
- H. Han, K. M. Kim, H. Choi, G. Ali, K. Y. Chung, Y.-R. Hong, J. Choi, J. Kwon, S. W. Lee and J. W. Lee, Parallelized reaction pathway and stronger internal band bending by partial oxidation of metal sulfide–graphene composites: important factors of synergistic oxygen evolution reaction enhancement, ACS Catal., 2018, 8, 4091–4102 CrossRef CAS
.
- A. Karmakar and S. K. Srivastava, In situ fabricated nickel vanadate/N-doped reduced graphene oxide hybrid as an advanced electrocatalyst in alkaline hydrogen evolution reaction, J. Mater. Chem. A, 2019, 7, 15054–15061 RSC
.
- D. Zhang, F. Wang, W. Zhao, M. Cui, X. Fan, R. Liang, Q. Ou and S. Zhang, Boosting Hydrogen Evolution Reaction Activity of Amorphous Molybdenum Sulfide Under High Currents Via Preferential Electron Filling Induced by Tungsten Doping, Adv. Sci., 2022, 9, 2202445 CrossRef CAS PubMed
.
- X. Gao, H. Liu, D. Wang and J. Zhang, Graphdiyne: synthesis, properties, and applications, Chem. Soc. Rev., 2019, 48, 908–936 RSC
.
- Y. Fang, Y. Liu, L. Qi, Y. Xue and Y. Li, 2D graphdiyne: an emerging carbon material, Chem. Soc. Rev., 2022, 51, 2681–2709 RSC
.
- X. Chen, M. Wang and M. Yuan, Recent advances of graphdiyne: synthesis, functionalization, and electrocatalytic applications, Mater. Chem. Front., 2021, 5, 7964–7981 RSC
.
- G. Shi, Z. Fan, L. Du, X. Fu, C. Dong, W. Xie, D. Zhao, M. Wang and M. Yuan, In situ construction of graphdiyne/CuS heterostructures for efficient hydrogen evolution reaction, Mater. Chem. Front., 2019, 3, 821–828 RSC
.
- H. Sun, X. Xu, C. Jing, W. Shang, Y. Wang, M. Zeng and Z. Jia, Composite clusters: Co 5.7 Ni 2.3 W 12 O 42 (OH) 4@ fluoro-graphdiyne as a stable electrode for sustained electrochemical oxygen evolution under high current conditions, Mater. Chem. Front., 2021, 5, 7666–7674 RSC
.
- H. Yu, Y. Xue, L. Hui, C. Zhang, Y. Zhao, Z. Li and Y. Li, Controlled growth of MoS2 nanosheets on 2D N-doped graphdiyne nanolayers for highly associated effects on water reduction, Adv. Funct. Mater., 2018, 28, 1707564 CrossRef
.
- L. Hui, Y. Xue, B. Huang, H. Yu, C. Zhang, D. Zhang, D. Jia, Y. Zhao, Y. Li and H. Liu, Overall water splitting by graphdiyne-exfoliated and-sandwiched layered double-hydroxide nanosheet arrays, Nat. Commun., 2018, 9, 5309 CrossRef CAS PubMed
.
- X. Chen, D. Zhang, X. Zheng, C. Zhang, Y. Gao, C. Xing, S. Chen, H. Wu, Y. Xue and Y. Li, Overall water electrolysis on a graphdiyne-iron oxyhydroxide heterostructure, J. Mater. Chem. A, 2023, 11, 9824–9828 RSC
.
- H. Wang and J.-M. Lee, Recent advances in structural engineering of MXene electrocatalysts, J. Mater. Chem. A, 2020, 8, 10604–10624 RSC
.
- L. Yan and B. Zhang, Rose-like, ruthenium-modified cobalt nitride nanoflowers grown in situ on an MXene matrix for efficient and stable water electrolysis, J. Mater. Chem. A, 2021, 9, 20758–20765 RSC
.
- Z. Kang, M. A. Khan, Y. Gong, R. Javed, Y. Xu, D. Ye, H. Zhao and J. Zhang, Recent progress of MXenes and MXene-based nanomaterials for the electrocatalytic hydrogen evolution reaction, J. Mater. Chem. A, 2021, 9, 6089–6108 RSC
.
- C. Tsounis, P. V. Kumar, H. Masood, R. P. Kulkarni, G. S. Gautam, C. R. Müller, R. Amal and D. A. Kuznetsov, Advancing MXene electrocatalysts for energy conversion reactions: surface, stoichiometry, and stability, Angew. Chem., Int. Ed., 2023, 62, e202210828 CrossRef CAS
.
- M. Yu, Z. Wang, J. Liu, F. Sun, P. Yang and J. Qiu, A hierarchically porous and hydrophilic 3D nickel–iron/MXene electrode for accelerating oxygen and hydrogen evolution at high current densities, Nano Energy, 2019, 63, 103880 CrossRef CAS
.
- L. Wang, Y. Hao, L. Deng, F. Hu, S. Zhao, L. Li and S. Peng, Rapid complete reconfiguration induced actual active species for industrial hydrogen evolution reaction, Nat. Commun., 2022, 13, 5785 CrossRef CAS PubMed
.
- H. Yang, M. Driess and P. W. Menezes, Self-supported electrocatalysts for practical water electrolysis, Adv. Energy Mater., 2021, 11, 2102074 CrossRef CAS
.
- J. Zhang, C. Si, T. Kou, J. Wang and Z. Zhang, Recent progress in self-supported two-dimensional transition metal oxides and (oxy) hydroxides as oxygen evolution reaction catalysts, Sustain, Energy Fuels, 2020, 4, 2625–2637 CAS
.
- C. Wang, B. Yan, Z. Chen, B. You, T. Liao, Q. Zhang, Y. Lu, S. Jiang and S. He, Recent advances in carbon substrate supported nonprecious nanoarrays for electrocatalytic oxygen evolution, J. Mater. Chem. A, 2021, 9, 25773–25795 RSC
.
- S. Li, E. Li, X. An, X. Hao, Z. Jiang and G. Guan, Transition metal-based catalysts for electrochemical water splitting at high current density: current status and perspectives, Nanoscale, 2021, 13, 12788–12817 RSC
.
- P. Zhai, C. Wang, Y. Zhao, Y. Zhang, J. Gao, L. Sun and J. Hou, Regulating electronic states of nitride/hydroxide to accelerate kinetics for oxygen evolution at large current density, Nat. Commun., 2023, 14, 1873 CrossRef CAS
.
- H. Zhou, F. Yu, Q. Zhu, J. Sun, F. Qin, L. Yu, J. Bao, Y. Yu, S. Chen and Z. Ren, Water splitting by electrolysis at high current densities under 1.6 volts, Energy Environ. Sci., 2018, 11, 2858–2864 RSC
.
- J. Li, M. Song, Y. Hu, Y. Zhu, J. Zhang and D. Wang, Hybrid Heterostructure Ni3N| NiFeP/FF Self-Supporting Electrode for High-Current-Density Alkaline Water Electrolysis, Small Methods, 2023, 7, 2201616 CrossRef CAS PubMed
.
- S. Chang, X. Huang, C. Y. A. Ong, L. Zhao, L. Li, X. Wang and J. Ding, High loading accessible active sites via designable 3D-printed metal architecture towards promoting electrocatalytic performance, J. Materi. Chem. A, 2019, 7, 18338–18347 RSC
.
- S. Chang, Y. Zhang, B. Zhang, X. Cao, L. Zhang, X. Huang, W. Lu, C. Y. A. Ong, S. Yuan and C. Li, Conductivity modulation of 3D-printed shellular electrodes through embedding nanocrystalline intermetallics into amorphous matrix for ultrahigh-current oxygen evolution, Adv. Energy Mater., 2021, 11, 2100968 CrossRef CAS
.
- M. Peng, D. Shi, Y. Sun, J. Cheng, B. Zhao, Y. Xie, J. Zhang, W. Guo, Z. Jia and Z. Liang, 3D printed mechanically robust graphene/CNT electrodes for highly efficient overall water splitting, Adv. Mater., 2020, 32, 1908201 CrossRef CAS
.
-
A. J. Bard, L. R. Faulkner and H. S. White, Electrochemical methods: fundamentals and applications, John Wiley & Sons, 2022 Search PubMed
.
- W. Liu, X. Li, Y. Wang, D. Yang, Z. Guo, M. Liu and J. Wang, Multi-branched AgAuPt nanoparticles for efficient electrocatalytic hydrogen evolution: Synergism of tip-enhanced electric field effect and local electric field effect, J. Energy Chem., 2023, 81, 339–348 CrossRef CAS
.
- D. Liu, X. Li, S. Chen, H. Yan, C. Wang, C. Wu, Y. A. Haleem, S. Duan, J. Lu and B. Ge, Atomically dispersed platinum supported on curved carbon supports for efficient electrocatalytic hydrogen evolution, Nat. Energy, 2019, 4, 512–518 CrossRef CAS
.
- Y. Chen, G. Zhang, H. Liu, Y. Wang, Z. Chen, Q. Ji, H. Lan, R. Liu and J. Qu, Tip-Intensified Interfacial Microenvironment Reconstruction Promotes an Electrocatalytic Chlorine Evolution Reaction, ACS Catal., 2022, 12, 14376–14386 CrossRef CAS
.
- Y. Liu, H. Jiang and Z. Hou, Local Field Induced Mass Transfer: New Insight into Nano-electrocatalysis, Chem. – Eur. J., 2021, 27, 17726–17735 CrossRef CAS PubMed
.
- P. Liu, B. Chen, C. Liang, W. Yao, Y. Cui, S. Hu, P. Zou, H. Zhang, H. J. Fan and C. Yang, Tip-Enhanced Electric Field: A New Mechanism Promoting Mass Transfer in Oxygen Evolution Reactions, Adv. Mater., 2021, 33, 2007377 CrossRef CAS
.
- B. Zhang, L. Zhang, Q. Tan, J. Wang, J. Liu, H. Wan, L. Miao and J. Jiang, Simultaneous interfacial chemistry and inner Helmholtz plane regulation for superior alkaline hydrogen evolution, Energy Environ. Sci., 2020, 13, 3007–3013 RSC
.
- X. Liu, X. Zhao, S. Cao, M. Xu, Y. Wang, W. Xue and J. Li, Local hydroxyl enhancement design of NiFe sulfide electrocatalyst toward efficient oxygen evolution reaction, Appl. Catal., B, 2023, 331, 122715 CrossRef CAS
.
- M. Wang, X. Yu, Z. Wang, X. Gong, Z. Guo and L. Dai, Hierarchically 3D porous films electrochemically constructed on gas–liquid–solid three-phase interface for energy application, J. Mater. Chem. A, 2017, 5, 9488–9513 RSC
.
- R. Shi, L. Shang, C. Zhou, Y. Zhao and T. Zhang, Interfacial wettability and mass transfer characterizations for gas–liquid–solid triple-phase catalysis, Exploration, 2022, 2, 20210046 CrossRef PubMed
.
- X. Yu, M. Wang, X. Gong, Z. Guo, Z. Wang and S. Jiao, Self-Supporting Porous CoP-Based Films with Phase-Separation Structure for Ultrastable Overall Water Electrolysis at Large Current Density, Adv. Energy Mater., 2018, 8, 1802445 CrossRef
.
- A. Angulo, P. van der Linde, H. Gardeniers, M. Modestino and D. F. Rivas, Influence of bubbles on the energy conversion efficiency of electrochemical reactors, Joule, 2020, 4, 555–579 CrossRef CAS
.
- Q. Wen, Y. Zhao, Y. Liu, H. Li and T. Zhai, Ultrahigh-Current-Density and Long-Term*Durability Electrocatalysts for Water Splitting, Small, 2022, 18, 2104513 CrossRef CAS PubMed
.
- C. Yu, P. Zhang, J. Wang and L. Jiang, Superwettability of Gas Bubbles and Its Application: From Bioinspiration to Advanced Materials, Adv. Mater., 2017, 29, 1703053 CrossRef PubMed
.
- R. Iwata, L. Zhang, K. L. Wilke, S. Gong, M. He, B. M. Gallant and E. N. Wang, Bubble growth and departure modes on wettable/non-wettable porous foams in alkaline water splitting, Joule, 2021, 5, 887–900 CrossRef CAS
.
- D. Kim, X. Qin, B. Yan and Y. Piao, Sprout-shaped Mo-doped CoP with maximized hydrophilicity and gas bubble release for high-performance water splitting catalyst, Chem. Eng. J., 2021, 408, 127331 CrossRef CAS
.
- Y. Luo, S. W. Chiang, L. Tang, Z. Zhang, F. Yang, Q. Yu, B. Ding and B. Liu, Manipulating Electrocatalysis using Mosaic Catalysts, Small Science, 2021, 1, 2000059 CrossRef CAS
.
- T. Kou, S. Wang, R. Shi, T. Zhang, S. Chiovoloni, J. Q. Lu, W. Chen, M. A. Worsley, B. C. Wood, S. E. Baker, E. B. Duoss, R. Wu, C. Zhu and Y. Li, Periodic Porous 3D Electrodes Mitigate Gas Bubble Traffic during Alkaline Water Electrolysis at High Current Densities, Adv. Energy Mater., 2020, 10, 2002955 CrossRef CAS
.
- Y. Zhou, N. Jin, Y. Ma, Y. Cui, L. Wang, Y. Kwon, W.-K. Lee, W. Zhang, H. Ge and J. Zhang, Tube-Sponge-Inspired Hierarchical Electrocatalysts with Boosted Mass and Electron Transfer for Efficient Oxygen Evolution, Adv. Mater., 2023, 35, 2209500 CrossRef CAS
.
- V. Stamenkovic, B. S. Mun, K. J. Mayrhofer, P. N. Ross, N. M. Markovic, J. Rossmeisl, J. Greeley and J. K. Nørskov, Changing the activity of electrocatalysts for oxygen reduction by tuning the surface electronic structure, Angew. Chem., Int. Ed., 2006, 45, 2897–2901 CrossRef CAS PubMed
.
- Y. Luo, L. Tang, U. Khan, Q. Yu, H.-M. Cheng, X. Zou and B. Liu, Morphology and surface chemistry engineering toward pH-universal catalysts for hydrogen evolution at high current density, Nat. Commun., 2019, 10, 269 CrossRef PubMed
.
-
G. A. Somorjai and Y. Li, Introduction to surface chemistry and catalysis, John Wiley & Sons, 2010 Search PubMed
.
- J. Wang, J. Feng, Y. Li, F. Lai, G. C. Wang, T. Liu, J. Huang and G. He, Multilayered Molybdate Microflowers Fabricated by One-Pot Reaction for Efficient Water Splitting, Adv. Sci., 2023, 10, 2206952 CrossRef CAS PubMed
.
- X. Mu, X. Gu, S. Dai, J. Chen, Y. Cui, Q. Chen, M. Yu, C. Chen, S. Liu and S. Mu, Breaking the symmetry of single-atom catalysts enables an extremely low energy barrier and high stability for large-current-density water splitting, Energy Environ. Sci., 2022, 15, 4048–4057 RSC
.
- M. Raccaud, E. T. Friman, A. B. Alber, H. Agarwal, C. Deluz, T. Kuhn, J. C. M. Gebhardt and D. M. Suter, Mitotic chromosome binding predicts transcription factor properties in interphase, Nat. Commun., 2019, 10, 487 CrossRef CAS PubMed
.
- J. Wang, Z. Zhang, H. Song, B. Zhang, J. Liu, X. Shai and L. Miao, Water dissociation kinetic–oriented design of nickel sulfides via tailored dual sites for efficient alkaline hydrogen evolution, Adv. Funct. Mater., 2021, 31, 2008578 CrossRef CAS
.
- S. Choi, Y. Park, H. Yang, H. Jin, G. M. Tomboc and K. Lee, Vacancy-engineered catalysts for water electrolysis, CrystEngComm, 2020, 22, 1500–1513 RSC
.
- X. Lv, S. Wan, T. Mou, X. Han, Y. Zhang, Z. Wang and X. Tao, Atomic-Level Surface Engineering of Nickel Phosphide Nanoarrays for Efficient Electrocatalytic Water Splitting at Large Current Density, Adv. Funct. Mater., 2023, 33, 2205161 CrossRef CAS
.
- Z. Wei, M. Guo and Q. Zhang, Scalable electrodeposition of NiFe-based electrocatalysts with self-evolving multi-vacancies for high-performance industrial water electrolysis, Appl. Catal., B, 2023, 322, 122101 CrossRef CAS
.
- T. Guo, L. Li and Z. Wang, Recent development and future perspectives of amorphous transition metal-based electrocatalysts for oxygen evolution reaction, Adv. Energy Mater., 2022, 12, 2200827 CrossRef CAS
.
- T. Liu, Q. Liu, A. M. Asiri, Y. Luo and X. Sun, An amorphous CoSe film behaves as an active and stable full water-splitting electrocatalyst under strongly alkaline conditions, Chem. Commun., 2015, 51, 16683–16686 RSC
.
- S. Anantharaj and S. Noda, Amorphous catalysts and electrochemical water splitting: an untold story of
harmony, Small, 2020, 16, 1905779 CrossRef CAS PubMed
.
- J. Kwon, H. Han, S. Jo, S. Choi, K. Y. Chung, G. Ali, K. Park, U. Paik and T. Song, Amorphous nickel–iron borophosphate for a robust and efficient oxygen evolution reaction, Adv. Energy Mater., 2021, 11, 2100624 CrossRef CAS
.
- H. Yang, Z. Chen, P. Guo, B. Fei and R. Wu, B-doping-induced amorphization of LDH for large-current-density hydrogen evolution reaction, Appl. Catal., B, 2020, 261, 118240 CrossRef CAS
.
- N. Tran, Y. Chang, D. Faragalli, S. Roberts, J. Josefowicz and Y. Shing, GeTe thin films: Amorphous and crystalline characteristics, J. Vac. Sci. Technol., 1983, 1, 345–347 CrossRef CAS
.
- P. I. Scheurle, A. Mähringer, A. C. Jakowetz, P. Hosseini, A. F. Richter, G. Wittstock, D. D. Medina and T. Bein, A highly crystalline anthracene-based MOF-74 series featuring electrical conductivity and luminescence, Nanoscale, 2019, 11, 20949–20955 RSC
.
- H. Han, H. Choi, S. Mhin, Y.-R. Hong, K. M. Kim, J. Kwon, G. Ali, K. Y. Chung, M. Je, H. N. Umh, D.-H. Lim, K. Davey, S.-Z. Qiao, U. Paik and T. Song, Advantageous crystalline–amorphous phase boundary for enhanced electrochemical water oxidation, Energy Environ. Sci., 2019, 12, 2443–2454 RSC
.
- H. Xu, B. Fei, G. Cai, Y. Ha, J. Liu, H. Jia, J. Zhang, M. Liu and R. Wu, Boronization-induced ultrathin 2D nanosheets with abundant crystalline–amorphous phase boundary supported on nickel foam toward efficient water splitting, Adv. Energy Mater., 2020, 10, 1902714 CrossRef CAS
.
- Q. Xu, J. Zhang, H. Zhang, L. Zhang, L. Chen, Y. Hu, H. Jiang and C. Li, Atomic heterointerface engineering overcomes the activity limitation of electrocatalysts and promises highly-efficient alkaline water splitting, Energy Environ. Sci., 2021, 14, 5228–5259 RSC
.
- S. Zhang, C. Tan, R. Yan, X. Zou, F.-L. Hu, Y. Mi, C. Yan and S. Zhao, Constructing Built-in Electric Field in Heterogeneous Nanowire Arrays for Efficient Overall Water Electrolysis, Angew. Chem., 2023, e202302795 CAS
.
- X.-H. Li and M. Antonietti, Metal nanoparticles at mesoporous N-doped carbons and carbon nitrides: functional Mott–Schottky heterojunctions for catalysis, Chem. Soc. Rev., 2013, 42, 6593–6604 RSC
.
- J. T. Ren, L. Wang, L. Chen, X. L. Song, Q. H. Kong, H. Y. Wang and Z. Y. Yuan, Interface Metal Oxides Regulating Electronic State around Nickel Species for Efficient Alkaline Hydrogen Electrocatalysis, Small, 2023, 19, 2206196 CrossRef CAS PubMed
.
- T. Wu, S. Xu, Z. Zhang, M. Luo, R. Wang, Y. Tang, J. Wang and F. Huang, Bimetal modulation stabilizing a metallic heterostructure for efficient overall water splitting at large current density, Adv. Sci., 2022, 9, 2202750 CrossRef CAS
.
- Q. Wen, K. Yang, D. Huang, G. Cheng, X. Ai, Y. Liu, J. Fang, H. Li, L. Yu and T. Zhai, Schottky heterojunction nanosheet array achieving high-current-density oxygen evolution for industrial water splitting electrolyzers, Adv. Energy Mater., 2021, 11, 2102353 CrossRef CAS
.
- S. Dutta, A. Indra, Y. Feng, T. Song and U. Paik, Self-supported nickel iron layered double hydroxide-nickel selenide electrocatalyst for superior water splitting activity, ACS Appl. Mater. Interfaces, 2017, 9, 33766–33774 CrossRef CAS
.
- T. Wu, E. Song, S. Zhang, M. Luo, C. Zhao, W. Zhao, J. Liu and F. Huang, Engineering metallic heterostructure based on Ni3N and 2M-MoS2 for alkaline water electrolysis with industry-compatible current density and stability, Adv. Mater., 2022, 34, 2108505 CrossRef CAS
.
- H. Sun, Z. Yan, C. Tian, C. Li, X. Feng, R. Huang, Y. Lan, J. Chen, C.-P. Li and Z. Zhang, Bixbyite-type Ln2O3 as promoters of metallic Ni for alkaline electrocatalytic hydrogen evolution, Nat. Commun., 2022, 13, 3857 CrossRef CAS
.
- D. Chanda, J. Hnát, M. Paidar, J. Schauer and K. Bouzek, Synthesis and characterization of NiFe2O4 electrocatalyst for the hydrogen evolution reaction in alkaline water electrolysis using different polymer binders, J. Power Sources, 2015, 285, 217–226 CrossRef CAS
.
- P. Wang and B. Wang, Interface Engineering of Binder-Free Earth-Abundant Electrocatalysts for Efficient Advanced Energy Conversion, ChemSusChem, 2020, 13, 4795–4811 CrossRef CAS PubMed
.
- A. Maljusch, O. Conradi, S. Hoch, M. Blug and W. Schuhmann, Advanced evaluation of the long-term stability of oxygen evolution electrocatalysts, Anal. Chem., 2016, 88, 7597–7602 CrossRef CAS PubMed
.
- A. R. Akbashev, V. Roddatis, C. Baeumer, T. Liu, J. T. Mefford and W. C. Chueh, Probing the stability of SrIrO3 during active water electrolysis via operando atomic force microscopy, Energy Environ. Sci., 2023, 16, 513–522 RSC
.
- L. Liu, W. Li, X. He, J. Yang and N. Liu,
In Situ/Operando Insights into the Stability and Degradation Mechanisms of Heterogeneous Electrocatalysts, Small, 2022, 18, 2104205 CrossRef CAS PubMed
.
- F.-Y. Chen, Z.-Y. Wu, Z. Adler and H. Wang, Stability challenges of electrocatalytic oxygen evolution reaction: From mechanistic understanding to reactor design, Joule, 2021, 5, 1704–1731 CrossRef CAS
.
- D. M. Morales, J. Villalobos, M. A. Kazakova, J. Xiao and M. Risch, Nafion-Induced Reduction of Manganese and its Impact on the Electrocatalytic Properties of a Highly Active MnFeNi Oxide for Bifunctional Oxygen Conversion, ChemElectroChem, 2021, 8, 2979–2983 CrossRef CAS PubMed
.
- T. Y. Ma, S. Dai and S. Z. Qiao, Self-supported electrocatalysts for advanced energy conversion processes, Mater. Today, 2016, 19, 265–273 CrossRef CAS
.
- J. Li, J. Zhang, J. Shen, H. Wu, H. Chen, C. Yuan, N. Wu, G. Liu, D. Guo and X. Liu, Self-supported electrocatalysts for the hydrogen evolution reaction, Mater. Chem. Front., 2023, 7(4), 567–606 RSC
.
- M. Yao, H. Hu, B. Sun, N. Wang, W. Hu and S. Komarneni, Self–Supportive Mesoporous Ni/Co/Fe Phosphosulfide Nanorods Derived from Novel Hydrothermal Electrodeposition as a Highly Efficient Electrocatalyst for Overall Water Splitting, Small, 2019, 15, 1905201 CrossRef CAS PubMed
.
- M. Etesami, A. A. Mohamad, M. T. Nguyen, T. Yonezawa, R. Pornprasertsuk, A. Somwangthanaroj and S. Kheawhom, Benchmarking superfast electrodeposited bimetallic (Ni, Fe, Co, and Cu) hydroxides for oxygen evolution reaction, J. Alloys Compd., 2021, 889, 161738 CrossRef
.
- J. Kim, H. Kim, G. H. Han, S. Hong, J. Park, J. Bang, S. Y. Kim and S. H. Ahn, Electrodeposition: An efficient method to fabricate self-supported electrodes for electrochemical energy conversion systems, Exploration, 2022, 2, 20210077 CrossRef PubMed
.
- Y. Liu, X. Liang, L. Gu, Y. Zhang, G.-D. Li, X. Zou and J.-S. Chen, Corrosion engineering towards efficient oxygen evolution electrodes with stable catalytic activity for over 6000 hours, Nat. Commun., 2018, 9, 2609 CrossRef PubMed
.
- X.-Y. Zhang, F.-T. Li, Y.-W. Dong, B. Dong, F.-N. Dai, C.-G. Liu and Y.-M. Chai, Dynamic anion regulation to construct S-doped FeOOH realizing 1000 mA cm−2-level-current-density oxygen evolution over 1000 h, Appl. Catal., B, 2022, 315, 121571 CrossRef CAS
.
- F. Guo, Y. Wu, H. Chen, Y. Liu, L. Yang, X. Ai and X. Zou, High-performance oxygen evolution electrocatalysis by boronized metal sheets with self-functionalized surfaces, Energy Environ. Sci., 2019, 12, 684–692 RSC
.
- L. Zhang, Z. Shi, Y. Lin, F. Chong and Y. Qi, Design strategies for large current density hydrogen evolution reaction, Front. Chem., 2022, 10, 866415 CrossRef CAS PubMed
.
- C. Liang, P. Zou, A. Nairan, Y. Zhang, J. Liu, K. Liu, S. Hu, F. Kang, H. J. Fan and C. Yang, Exceptional performance of hierarchical Ni–Fe oxyhydroxide@ NiFe alloy nanowire array electrocatalysts for large current density water splitting, Energy Environ. Sci., 2020, 13, 86–95 RSC
.
- H. Liu, R. Xie, Y. Luo, Z. Cui, Q. Yu, Z. Gao, Z. Zhang, F. Yang, X. Kang and S. Ge, Dual interfacial engineering of a Chevrel phase electrode material for stable hydrogen evolution at 2500 mA cm−2, Nat. Commun., 2022, 13, 6382 CrossRef CAS PubMed
.
- Y. Zeng, M. Zhao, Z. Huang, W. Zhu, J. Zheng, Q. Jiang, Z. Wang and H. Liang, Surface reconstruction of water splitting electrocatalysts, Adv. Energy Mater., 2022, 12, 2201713 CrossRef CAS
.
- W. Zou, C. Sun, K. Zhao, J. Li, X. Pan, D. Ye, Y. Xie, W. Xu, H. Zhao and L. Zhang, Surface reconstruction of NiCoP pre-catalysts for bifunctional water splitting in alkaline electrolyte, Electrochim. Acta, 2020, 345, 136114 CrossRef CAS
.
- L. Su, X. Cui, T. He, L. Zeng, H. Tian, Y. Song, K. Qi and B. Y. Xia, Surface reconstruction of cobalt phosphide nanosheets by electrochemical activation for enhanced hydrogen evolution in alkaline solution, Chem. Sci., 2019, 10, 2019–2024 RSC
.
- T. Wu, S. Sun, J. Song, S. Xi, Y. Du, B. Chen, W. A. Sasangka, H. Liao, C. L. Gan and G. G. Scherer, Iron-facilitated dynamic active-site generation on spinel CoAl2O4 with self-termination of surface reconstruction for water oxidation, Nat. Catal., 2019, 2, 763–772 CrossRef CAS
.
- D. Li, W. Wan, Z. Wang, H. Wu, S. Wu, T. Jiang, G. Cai, C. Jiang and F. Ren, Self-Derivation and Surface Reconstruction of Fe-Doped Ni3S2 Electrode Realizing High-Efficient and Stable Overall Water and Urea Electrolysis, Adv. Energy Mater., 2022, 12, 2201913 CrossRef CAS
.
- S.-P. Zeng, H. Shi, T.-Y. Dai, Y. Liu, Z. Wen, G.-F. Han, T.-H. Wang, W. Zhang, X.-Y. Lang and W.-T. Zheng, Lamella-heterostructured nanoporous bimetallic iron-cobalt alloy/oxyhydroxide and cerium oxynitride electrodes as stable catalysts for oxygen evolution, Nat. Commun., 2023, 14, 1811 CrossRef CAS
.
- C. Kuai, Z. Xu, C. Xi, A. Hu, Z. Yang, Y. Zhang, C.-J. Sun, L. Li, D. Sokaras and C. Dong, Phase segregation reversibility in mixed-metal hydroxide water oxidation
catalysts, Nat. Catal., 2020, 3, 743–753 CrossRef CAS
.
- L. Peng, N. Yang, Y. Yang, Q. Wang, X. Xie, D. Sun-Waterhouse, L. Shang, T. Zhang and G. I. Waterhouse, Atomic Cation-Vacancy Engineering of NiFe-Layered Double Hydroxides for Improved Activity and Stability towards the Oxygen Evolution Reaction, Angew. Chem., Int. Ed., 2021, 60, 24612–24619 CrossRef CAS PubMed
.
- B. K. Hong, P. Mandal, J.-G. Oh and S. Litster, On the impact of water activity on reversal tolerant fuel cell anode performance and durability, J. Power Sources, 2016, 328, 280–288 CrossRef CAS
.
- D. Liang, Q. Shen, M. Hou, Z. Shao and B. Yi, Study of the cell reversal process of large area proton exchange membrane fuel cells under fuel starvation, J. Power Sources, 2009, 194, 847–853 CrossRef CAS
.
- X. Wang, W. Ma, Z. Xu, H. Wang, W. Fan, X. Zong and C. Li, Metal phosphide catalysts anchored on metal-caged graphitic carbon towards efficient and durable hydrogen evolution electrocatalysis, Nano Energy, 2018, 48, 500–509 CrossRef CAS
.
- H.-E. Kim, J. Kwon and H. Lee, Catalytic approaches towards highly durable proton exchange membrane fuel cells with minimized Pt use, Chem. Sci., 2022, 13, 6782–6795 RSC
.
- T. Joo, L. Hu, B. K. Hong, J.-G. Oh and S. Litster, On the origin of deactivation of reversal-tolerant fuel cell anodes under voltage reversal conditions, J. Power Sources, 2020, 472, 228439 CrossRef CAS
.
- S.-M. Jung, S.-W. Yun, J.-H. Kim, S.-H. You, J. Park, S. Lee, S. H. Chang, S. C. Chae, S. H. Joo and Y. Jung, Selective electrocatalysis imparted by metal–insulator transition for durability enhancement of automotive fuel cells, Nat. Catal., 2020, 3, 639–648 CrossRef CAS
.
- Y. Yin, X. Sun, M. Zhou, X. Zhao, J. Qin, S.-Z. Qiao, X.-W. Du and J. Yang, Laser-Induced Pyridinic-Nitrogen-Rich Defective Carbon Nanotubes for Efficient Oxygen Electrocatalysis, ChemCatChem, 2019, 11, 6131–6138 CrossRef CAS
.
- L. Trotochaud, S. L. Young, J. K. Ranney and S. W. Boettcher, Nickel–Iron Oxyhydroxide Oxygen-Evolution Electrocatalysts: The Role of Intentional and Incidental Iron Incorporation, J. Am. Chem. Soc., 2014, 136, 6744–6753 CrossRef CAS PubMed
.
- L. Chen, X. Dong, Y. Wang and Y. Xia, Separating hydrogen and oxygen evolution in alkaline water electrolysis using nickel hydroxide, Nat. Commun., 2016, 7, 11741 CrossRef CAS PubMed
.
- W. Zhang, M. Liu, X. Gu, Y. Shi, Z. Deng and N. Cai, Water Electrolysis toward Elevated Temperature: Advances, Challenges and Frontiers, Chem. Rev., 2023, 123(11), 7119–7192 CrossRef CAS PubMed
.
- H. Dotan, A. Landman, S. W. Sheehan, K. D. Malviya, G. E. Shter, D. A. Grave, Z. Arzi, N. Yehudai, M. Halabi and N. Gal, Decoupled hydrogen and oxygen evolution by a two-step electrochemical–chemical cycle for efficient overall water splitting, Nat. Energy, 2019, 4, 786–795 CrossRef CAS
.
- A. Landman, S. Hadash, G. E. Shter, A. Ben-Azaria, H. Dotan, A. Rothschild and G. S. Grader, High performance core/shell Ni/Ni (OH)2 electrospun nanofiber anodes for decoupled water splitting, Adv. Funct. Mater., 2021, 31, 2008118 CrossRef CAS
.
- J. Liu, S. Duan, H. Shi, T. Wang, X. Yang, Y. Huang, G. Wu and Q. Li, Rationally Designing Efficient Electrocatalysts for Direct Seawater Splitting: Challenges, Achievements, and Promises, Angew. Chem., Int. Ed., 2022, 61, e202210753 CrossRef CAS PubMed
.
- F. Liu, L. Wang, R. Bradley, B. Zhao and W. Wu, Highly efficient solar seawater desalination with environmentally friendly hierarchical porous carbons derived from halogen-containing polymers, RSC Adv., 2019, 9, 29414–29423 RSC
.
- X. Liu, Y. Tian, Y. Wu, A. Caratenuto, F. Chen, S. Cui, J. A. DeGiorgis, Y. Wan and Y. Zheng, Seawater desalination derived entirely from ocean biomass, J. Mater. Chem. A, 2021, 9, 22313–22324 RSC
.
- T. Hu, L. Li, Y. Yang and J. Zhang, A yolk@shell superhydrophobic/superhydrophilic solar evaporator for efficient and stable desalination, J. Mater. Chem. A, 2020, 8, 14736–14745 RSC
.
- I. A. Said, N. Fuentes, Z. He, R. Xin, K. Zuo and Q. Li, Low-cost desalination of seawater and hypersaline brine using nanophotonics enhanced solar energy membrane distillation, Environ. Sci.: Water Res. Technol., 2020, 6, 2180–2196 RSC
.
- Z.-Y. Yu, Y. Duan, X.-Y. Feng, X. Yu, M.-R. Gao and S.-H. Yu, Clean and Affordable Hydrogen Fuel from Alkaline Water Splitting: Past, Recent Progress, and Future Prospects, Adv. Mater., 2021, 33, 2007100 CrossRef CAS PubMed
.
- D. Li, E. J. Park, W. Zhu, Q. Shi, Y. Zhou, H. Tian, Y. Lin, A. Serov, B. Zulevi, E. D. Baca, C. Fujimoto, H. T. Chung and Y. S. Kim, Highly quaternized polystyrene ionomers for high performance anion exchange membrane water electrolysers, Nat. Energy, 2020, 5, 378–385 CrossRef CAS
.
- R. A. Krivina, M. Zlatar, T. N. Stovall, G. A. Lindquist, D. Escalera-López, A. K. Cook, J. E. Hutchison, S. Cherevko and S. W. Boettcher, Oxygen Evolution Electrocatalysis in Acids: Atomic Tuning of the Stability Number for Submonolayer IrOx on Conductive Oxides from Molecular Precursors, ACS Catal., 2023, 13, 902–915 CrossRef CAS
.
- J. Guo, Y. Zheng, Z. Hu, C. Zheng, J. Mao, K. Du, M. Jaroniec, S.-Z. Qiao and T. Ling, Direct seawater electrolysis by adjusting the local reaction environment of a catalyst, Nat. Energy, 2023, 8, 264–272 CAS
.
- F. Dionigi, T. Reier, Z. Pawolek, M. Gliech and P. Strasser, Design Criteria, Operating Conditions, and Nickel–Iron Hydroxide Catalyst Materials for Selective Seawater Electrolysis, ChemSusChem, 2016, 9, 962–972 CrossRef CAS
.
- J. G. Vos, T. A. Wezendonk, A. W. Jeremiasse and M. T. M. Koper, MnOx/IrOx as Selective Oxygen Evolution Electrocatalyst in Acidic Chloride Solution, J. Am. Chem. Soc., 2018, 140, 10270–10281 CrossRef CAS PubMed
.
- Z. Xie, S. Yu, X.-B. Fan, S. Wei, L. Yu, Y. Zhong, X.-W. Gao, F. Wu and Y. Zhou, Wavelength-sensitive photocatalytic H2 evolution from H2S splitting over g-C3N4 with S,N-codoped carbon dots as the photosensitizer, J. Energy Chem., 2021, 52, 234–242 CrossRef CAS
.
- X. Ren, Y. Zhai, Q. Zhou, J. Yan and S. Liu, Fabrication of nanoporous Ni and NiO via a dealloying strategy for water oxidation catalysis, J. Energy Chem., 2020, 50, 125–134 CrossRef
.
- H.-Y. Wang, C.-C. Weng, J.-T. Ren and Z.-Y. Yuan, An overview and recent advances in electrocatalysts for direct seawater splitting, Front. Chem. Sci. Eng., 2021, 15, 1408–1426 CrossRef
.
- J. Li, Y. Liu, H. Chen, Z. Zhang and X. Zou, Design of a Multilayered Oxygen-Evolution Electrode with High Catalytic Activity and Corrosion Resistance for Saline Water Splitting, Adv. Funct. Mater., 2021, 31, 2101820 CrossRef CAS
.
- Y. Kuang, M. J. Kenney, Y. Meng, W.-H. Hung, Y. Liu, J. E. Huang, R. Prasanna, P. Li, Y. Li, L. Wang, M.-C. Lin, M. D. McGehee, X. Sun and H. Dai, Solar-driven, highly sustained splitting of seawater into hydrogen and oxygen fuels, Proc. Natl. Acad. Sci. U. S. A., 2019, 116, 6624–6629 CrossRef CAS PubMed
.
- H.-Y. Wang, J.-T. Ren, L. Wang, M.-L. Sun, H.-M. Yang, X.-W. Lv and Z.-Y. Yuan, Synergistically enhanced activity and stability of bifunctional nickel phosphide/sulfide heterointerface electrodes for direct alkaline seawater electrolysis, J. Energy Chem., 2022, 75, 66–73 CrossRef CAS
.
- T. Ma, W. Xu, B. Li, X. Chen, J. Zhao, S. Wan, K. Jiang, S. Zhang, Z. Wang, Z. Tian, Z. Lu and L. Chen, The Critical Role of Additive Sulfate for Stable Alkaline Seawater Oxidation on Nickel-Based Electrodes, Angew. Chem., Int. Ed., 2021, 60, 22740–22744 CrossRef CAS PubMed
.
- Y. Shi, W. Du, W. Zhou, C. Wang, S. Lu, S. Lu and B. Zhang, Unveiling the Promotion of Surface-Adsorbed Chalcogenate on the Electrocatalytic Oxygen Evolution Reaction, Angew. Chem., Int. Ed., 2020, 59, 22470–22474 CrossRef CAS PubMed
.
- M. Yu, J. Li, F. Liu, J. Liu, W. Xu, H. Hu, X. Chen, W. Wang and F. Cheng, Anionic formulation of electrolyte additive towards stable electrocatalytic oxygen evolution in seawater splitting, J. Energy Chem., 2022, 72, 361–369 CrossRef CAS
.
- C. Kuai, C. Xi, A. Hu, Y. Zhang, Z. Xu, D. Nordlund, C.-J. Sun, C. A. Cadigan, R. M. Richards, L. Li, C.-K. Dong, X.-W. Du and F. Lin, Revealing the Dynamics and Roles of Iron Incorporation in Nickel Hydroxide Water Oxidation Catalysts, J. Am. Chem. Soc., 2021, 143, 18519–18526 CrossRef CAS
.
- M. W. Louie and A. T. Bell, An Investigation of Thin-Film Ni–Fe Oxide Catalysts for the Electrochemical Evolution of Oxygen, J. Am. Chem. Soc., 2013, 135, 12329–12337 CrossRef CAS PubMed
.
- Z. Zhao, J. Sun and X. Meng, Recent advances in transition metal-based electrocatalysts for seawater electrolysis, Int. J. Energy Res., 2022, 46, 17952–17975 CrossRef CAS
.
- J. Chang, G. Wang, Z. Yang, B. Li, Q. Wang, R. Kuliiev, N. Orlovskaya, M. Gu, Y. Du, G. Wang and Y. Yang, Dual-Doping and Synergism toward High-Performance Seawater Electrolysis, Adv. Mater., 2021, 33, 2101425 CrossRef CAS PubMed
.
- H. Jin, H. Yu, H. Li, K. Davey, T. Song, U. Paik and S.-Z. Qiao, MXene Analogue: A 2D Nitridene Solid Solution for High-Rate Hydrogen Production, Angew. Chem., Int. Ed., 2022, 61, e202203850 CrossRef CAS PubMed
.
- H. Jin, X. Wang, C. Tang, A. Vasileff, L. Li, A. Slattery and S.-Z. Qiao, Stable and Highly Efficient Hydrogen Evolution from Seawater Enabled by an Unsaturated Nickel Surface Nitride, Adv. Mater., 2021, 33, 2007508 CrossRef CAS PubMed
.
- G. A. Lindquist, Q. Xu, S. Z. Oener and S. W. Boettcher, Membrane Electrolyzers for Impure-Water Splitting, Joule, 2020, 4, 2549–2561 CrossRef CAS
.
- H. Jin, B. Ruqia, Y. Park, H. J. Kim, H.-S. Oh, S.-I. Choi and K. Lee, Nanocatalyst Design for Long-Term Operation of Proton/Anion Exchange Membrane Water Electrolysis, Adv. Energy Mater., 2021, 11, 2003188 CrossRef CAS
.
- F. D. Speck, K. E. Dettelbach, R. S. Sherbo, D. A. Salvatore, A. Huang and C. P. Berlinguette, On the Electrolytic Stability of Iron-Nickel Oxides, Chem, 2017, 2, 590–597 CAS
.
- J. Abed, S. Ahmadi, L. Laverdure, A. Abdellah, C. P. O’Brien, K. Cole, P. Sobrinho, D. Sinton, D. Higgins, N. J. Mosey, S. J. Thorpe and E. H. Sargent, In Situ Formation of Nano Ni–Co Oxyhydroxide Enables Water Oxidation Electrocatalysts Durable at High Current Densities, Adv. Mater., 2021, 33, 2103812 CrossRef CAS PubMed
.
- W. Li, D. Xiong, X. Gao and L. Liu, The oxygen evolution reaction enabled by transition metal phosphide and chalcogenide pre-catalysts with dynamic changes, Chem. Commun., 2019, 55, 8744–8763 RSC
.
- M. S. Cha, J. E. Park, S. Kim, S.-H. Han, S.-H. Shin, S. H. Yang, T.-H. Kim, D. M. Yu, S. So, Y. T. Hong, S. J. Yoon, S.-G. Oh, S. Y. Kang, O.-H. Kim, H. S. Park, B. Bae, Y.-E. Sung, Y.-H. Cho and J. Y. Lee, Poly(carbazole)-based anion-conducting materials with high performance and durability for energy conversion devices, Energy Environ. Sci., 2020, 13, 3633–3645 RSC
.
- A. Khataee, A. Shirole, P. Jannasch, A. Krüger and A. Cornell, Anion exchange membrane water electrolysis using Aemion™ membranes and nickel electrodes, J. Mater. Chem. A, 2022, 10, 16061–16070 RSC
.
- M.-H. Kim, D.-H. Park, J.-H. Byeon, D.-M. Lim, Y.-H. Gu, S.-H. Park and K.-W. Park, Fe-doped Co3O4 nanostructures prepared via hard-template method and used for the oxygen evolution reaction in alkaline media, J. Ind. Eng. Chem., 2023, 123, 436–446 CrossRef CAS
.
- Y. J. Kim, A. Lim, J. M. Kim, D. Lim, K. H. Chae, E. N. Cho, H. J. Han, K. U. Jeon, M. Kim, G. H. Lee, G. R. Lee, H. S. Ahn, H. S. Park, H. Kim, J. Y. Kim and Y. S. Jung, Highly efficient oxygen evolution reaction via facile bubble transport realized by three-dimensionally stack-printed catalysts, Nat. Commun., 2020, 11, 4921 CrossRef CAS PubMed
.
- S. Assavapanumat, M. Ketkaew, P. Garrigue, V. Lapeyre, S. Reculusa, C. Wattanakit and A. Kuhn, Hierarchical Multiporous Nickel for Oxygen Evolution Reaction in Alkaline Media, ChemCatChem, 2019, 11, 5951–5960 CrossRef CAS
.
- Z. Lyu, X. Zhang, X. Liao, K. Liu, H. Huang, J. Cai, Q. Kuang, Z. Xie and S. Xie, Two-Dimensionally Assembled Pd–Pt–Ir Supernanosheets with Subnanometer Interlayer Spacings toward High-Efficiency and Durable Water Splitting, ACS Catal., 2022, 12, 5305–5315 CrossRef CAS
.
- S. Ma, X. Qu, J. Huang, C. Zhang, G. Chen, W. Chen, T. Li, T. Shao, K. Zheng, J. Tian, C. Li and K. Ostrikov, Compositional and crystallographic design of Ni–Co phosphide heterointerfaced nanowires for high-rate, stable hydrogen generation at industry-relevant electrolysis current densities, Nano Energy, 2022, 95, 106989 CrossRef CAS
.
- Y. Chen, J. Yu, J. Jia, F. Liu, Y. Zhang, G. Xiong, R. Zhang, R. Yang, D. Sun, H. Liu and W. Zhou, Metallic Ni3Mo3N Porous Microrods with Abundant Catalytic Sites as Efficient Electrocatalyst for Large Current Density and Superstability of Hydrogen Evolution Reaction and Water Splitting, Appl. Catal., B, 2020, 272, 118956 CrossRef CAS
.
- Y. Liu, Q. Feng, W. Liu, Q. Li, Y. Wang, B. Liu, L. Zheng, W. Wang, L. Huang, L. Chen, X. Xiong and Y. Lei, Boosting interfacial charge transfer for alkaline hydrogen evolution via rational interior Se modification, Nano Energy, 2021, 81, 105641 CrossRef CAS
.
- W. Chen, J. Gu, Y. Du, F. Song, F. Bu, J. Li, Y. Yuan, R. Luo, Q. Liu and D. Zhang, Achieving Rich and Active Alkaline Hydrogen Evolution Heterostructures via Interface Engineering on 2D 1T-MoS2 Quantum Sheets, Adv. Funct. Mater., 2020, 30, 2000551 CrossRef CAS
.
- F. Zhang, X. Wang, W. Han, Y. Qian, L. Qiu, Y. He, L. Lei and X. Zhang, The Synergistic Activation of Ce-Doping and CoP/Ni3P Hybrid Interaction for Efficient Water Splitting at Large-Current-Density, Adv. Funct. Mater., 2023, 33, 2212381 CrossRef CAS
.
- Y. Li, X. Tan, H. Tan, H. Ren, S. Chen, W. Yang, S. C. Smith and C. Zhao, Phosphine vapor-assisted construction of heterostructured Ni2P/NiTe2 catalysts for efficient hydrogen evolution, Energy Environ. Sci., 2020, 13, 1799–1807 RSC
.
- X.-Y. Zhang, F.-T. Li, R.-Y. Fan, J. Zhao, B. Dong, F.-L. Wang, H.-J. Liu, J.-F. Yu, C.-G. Liu and Y.-M. Chai, F, P double-doped Fe3O4 with abundant defect sites for efficient hydrogen evolution at high current density, J. Mater. Chem. A, 2021, 9, 15836–15845 RSC
.
- W. Han, F. Zhang, L. Qiu, Y. Qian, S. Hao, P. Li, Y. He and X. Zhang, Interface engineering of hierarchical NiCoP/NiCoSx heterostructure arrays for efficient alkaline hydrogen evolution at large current density, Nanoscale, 2022, 14, 15498–15506 RSC
.
- Y. Luo, Z. Zhang, F. Yang, J. Li, Z. Liu, W. Ren, S. Zhang and B. Liu, Stabilized hydroxide-mediated nickel-based electrocatalysts for high-current-density hydrogen evolution in alkaline media, Energy Environ. Sci., 2021, 14, 4610–4619 RSC
.
- J. Lv, L. Wang, R. Li, K. Zhang, D. Zhao, Y. Li, X. Li, X. Huang and G. Wang, Constructing a Hetero-interface Composed of Oxygen Vacancy-Enriched Co3O4 and Crystalline–Amorphous NiFe-LDH for Oxygen Evolution Reaction, ACS Catal., 2021, 11, 14338–14351 CrossRef CAS
.
- Y. Wang, L. Yan, K. Dastafkan, C. Zhao, X. Zhao, Y. Xue, J. Huo, S. Li and Q. Zhai, Lattice Matching Growth of Conductive Hierarchical Porous MOF/LDH Heteronanotube Arrays for Highly Efficient Water Oxidation, Adv. Mater., 2021, 33, 2006351 CrossRef CAS
.
- Q. Hu, Z. Wang, X. Huang, Y. Qin, H. Yang, X. Ren, Q. Zhang, J. Liu, M. Shao and C. He, Integrating well-controlled core-shell structures into “superaerophobic” electrodes for water oxidation at large current densities, Appl. Catal., B, 2021, 286, 119920 CrossRef CAS
.
- X. Ji, Y. Lin, J. Zeng, Z. Ren, Z. Lin, Y. Mu, Y. Qiu and J. Yu, Graphene/MoS2/FeCoNi(OH)x and Graphene/MoS2/FeCoNiPx multilayer-stacked vertical nanosheets on carbon fibers for highly efficient overall water splitting, Nat. Commun., 2021, 12, 1380 CrossRef CAS PubMed
.
- X. Cheng, Z. Pan, C. Lei, Y. Jin, B. Yang, Z. Li, X. Zhang, L. Lei, C. Yuan and Y. Hou, A strongly coupled 3D ternary Fe2O3@Ni2P/Ni(PO3)2 hybrid for enhanced electrocatalytic oxygen evolution at ultra-high current densities, J. Mater. Chem. A, 2019, 7, 965–971 RSC
.
- Y. Wang, G. Qian, Q. Xu, H. Zhang, F. Shen, L. Luo and S. Yin, Industrially promising IrNi–FeNi3 hybrid nanosheets for overall water splitting catalysis at large current density, Appl. Catal., B, 2021, 286, 119881 CrossRef CAS
.
- H. Liu, H. Qin, J. Kang, L. Ma, G. Chen, Q. Huang, Z. Zhang, E. Liu, H. Lu, J. Li and N. Zhao, A freestanding nanoporous NiCoFeMoMn high-entropy alloy as an efficient electrocatalyst for rapid water splitting, Chem. Eng. J., 2022, 435, 134898 CrossRef CAS
.
- H. Sun, C. Tian, G. Fan, J. Qi, Z. Liu, Z. Yan, F. Cheng, J. Chen, C.-P. Li and M. Du, Boosting Activity on Co4N Porous Nanosheet by Coupling CeO2 for Efficient Electrochemical Overall Water Splitting at High Current Densities, Adv. Funct. Mater., 2020, 30, 1910596 CrossRef CAS
.
- V. R. Jothi, K. Karuppasamy, T. Maiyalagan, H. Rajan, C.-Y. Jung and S. C. Yi, Corrosion and Alloy Engineering in Rational Design of High Current Density Electrodes for Efficient Water Splitting, Adv. Energy Mater., 2020, 10, 1904020 CrossRef CAS
.
- Y. Chen, Y. Wang, J. Yu, G. Xiong, H. Niu, Y. Li, D. Sun, X. Zhang, H. Liu and W. Zhou, Underfocus Laser Induced Ni Nanoparticles Embedded Metallic MoN Microrods as Patterned Electrode for Efficient Overall Water Splitting, Adv. Sci., 2022, 9, 2105869 CrossRef CAS PubMed
.
- W. Hao, R. Wu, H. Huang, X. Ou, L. Wang, D. Sun, X. Ma and Y. Guo, Fabrication of practical catalytic electrodes using insulating and eco-friendly substrates for overall water splitting, Energy Environ. Sci., 2020, 13, 102–110 RSC
.
- J. Jian, W. Chen, D. Zeng, L. Chang, R. Zhang, M. Jiang, G. Yu, X. Huang, H. Yuan and S. Feng, Metal-ionic-conductor potassium ferrite nanocrystals with intrinsic superhydrophilic surfaces for electrocatalytic water splitting at ultrahigh current densities, J. Mater. Chem. A, 2021, 9, 7586–7593 RSC
.
- J. Mo, Y. Ko, Y. S. Yun, J. Huh and J. Cho, A carbonization/interfacial assembly-driven electroplating approach for water-splitting textile electrodes with remarkably low overpotentials and high operational stability, Energy Environ. Sci., 2022, 15, 3815–3829 RSC
.
- G. Qian, J. Chen, T. Yu, L. Luo and S. Yin, N-Doped Graphene-Decorated NiCo Alloy Coupled with Mesoporous NiCoMoO Nano-sheet Heterojunction for Enhanced Water Electrolysis Activity at High Current Density, Nano-Micro Lett., 2021, 13, 77 CrossRef PubMed
.
- H. Zhu, S. Sun, J. Hao, Z. Zhuang, S. Zhang, T. Wang, Q. Kang, S. Lu, X. Wang, F. Lai, T. Liu, G. Gao, M. Du and D. Wang, A high-entropy atomic environment converts inactive to active sites for electrocatalysis, Energy Environ. Sci., 2023, 16, 619–628 RSC
.
- W. He, R. Zhang, D. Cao, Y. Li, J. Zhang, Q. Hao, H. Liu, J. Zhao and H. L. Xin, Super-Hydrophilic Microporous Ni(OH)x/Ni3S2 Heterostructure Electrocatalyst for Large-Current-Density Hydrogen Evolution, Small, 2023, 19, 2205719 CrossRef CAS PubMed
.
- Z. Lei, P. Liu, X. Yang, P. Zou, A. Nairan, S. Jiao, R. Cao, W. Wang, F. Kang and C. Yang, Monolithic Nickel Catalyst Featured with High-Density Crystalline Steps for Stable Hydrogen Evolution at Large Current Density, Small, 2023, 19, 2301247 CrossRef CAS PubMed
.
- X.-Y. Zhang, F.-T. Li, Y.-W. Dong, B. Dong, F.-N. Dai, C.-G. Liu and Y.-M. Chai, Dynamic anion regulation to construct S-doped FeOOH realizing 1000 mA cm−2-level-current-density oxygen evolution over 1000 h, Appl. Catal., B, 2022, 315, 121571 CrossRef CAS
.
- S. Jo, W. B. Park, K. B. Lee, H. Choi, K.-S. Lee, D. Ahn, Y.-W. Lee, K.-S. Sohn, J. Hong and J. I. Sohn, Bi/BiFe(oxy)hydroxide for sustainable lattice oxygen-boosted electrocatalysis at a practical high current density, Appl. Catal., B, 2022, 317, 121685 CrossRef CAS
.
- Y. Huang, L.-W. Jiang, H. Liu and J.-J. Wang, Electronic structure regulation and polysulfide bonding of Co-doped (Ni, Fe)1+xS enable highly efficient and stable electrocatalytic overall water splitting, Chem. Eng. J., 2022, 441, 136121 CrossRef CAS
.
- D. Yu, Y. Hao, S. Han, S. Zhao, Q. Zhou, C.-H. Kuo, F. Hu, L. Li, H.-Y. Chen, J. Ren and S. Peng, Ultrafast Combustion Synthesis of Robust and Efficient Electrocatalysts for High-Current-Density Water Oxidation, ACS Nano, 2023, 17, 1701–1712 CrossRef CAS
.
- W. Peng, Y. Li, B. Yuan, R. Hu, Z. Luo and M. Zhu, A dealloyed bulk FeNi pattern with exposed highly active facets for cost-effective oxygen evolution, Appl. Catal., B, 2023, 323, 122171 CrossRef CAS
.
- Y. Li, B. Wei, Z. Yu, O. Bondarchuk, A. Araujo, I. Amorim, N. Zhang, J. Xu, I. C. Neves and L. Liu, Bifunctional Porous Cobalt Phosphide Foam for High-Current-Density Alkaline Water Electrolysis with 4000-h Long Stability, ACS Sustainable Chem. Eng., 2020, 8, 10193–10200 CrossRef CAS
.
- G. Zhang, B. Wang, L. Li, S. Yang, J. Liu and S. Yang, Tailoring the electronic structure by constructing the heterointerface of RuO2–NiO for overall water splitting with ultralow overpotential and extra-long lifetime, J. Mater. Chem. A, 2020, 8, 18945–18954 RSC
.
- P. Zhai, Y. Zhang, Y. Wu, J. Gao, B. Zhang, S. Cao, Y. Zhang, Z. Li, L. Sun and J. Hou, Engineering active sites on hierarchical transition bimetal oxides/sulfides heterostructure array enabling robust overall water splitting, Nat. Commun., 2020, 11, 5462 CrossRef CAS
.
- L. Chen, Y. Wang, X. Zhao, Y. Wang, Q. Li, Q. Wang, Y. Tang and Y. Lei, Trimetallic oxyhydroxides as active sites for large-current-density alkaline oxygen evolution and overall water splitting, J. Mater. Sci. Technol., 2022, 110, 128–135 CrossRef CAS
.
|
This journal is © the Partner Organisations 2024 |
Click here to see how this site uses Cookies. View our privacy policy here.