DOI:
10.1039/C3BM60263J
(Paper)
Biomater. Sci., 2014,
2, 674-679
Fabrication of zeolite–polymer composite nanofibers for removal of uremic toxins from kidney failure patients†
Received
1st November 2013
, Accepted 19th December 2013
First published on 31st January 2014
Abstract
There is a need to develop a simple, cheap, and accessible method of treating patients with kidney failure, especially in resource-limited environments such as disaster areas and the developing world due to the inaccessibility of conventional hemodialysis treatments. In this study, we develop a zeolite–polymer composite nanofiber mesh to remove uremic toxins for blood purification. The nanofiber is composed of blood compatible poly(ethylene-co-vinyl alcohol) (EVOH) as the primary matrix polymer and zeolites which are capable of selectively adsorbing uremic toxins such as creatinine. The composite fiber meshes were produced by a cost-effective electrospinning method: electrospinning composite solutions of EVOH and zeolites. Scanning electron microscope (SEM) images revealed that the 7 w/v% EVOH solution produced non-woven fibers with a continuous and smooth morphology. The SEM also showed that over 90% of zeolites in the solution were successfully incorporated into the EVOH nanofibers. Although the barrier properties of the EVOH matrix lowered the creatinine adsorption capacity of the zeolites in the fiber when compared with adsorption to free zeolites, their adsorption capacity was still 67% of the free zeolites. The proposed composite fibers have the potential to be utilized as a new approach to removing nitrogenous waste products from the bloodstream without the requirement of specialized equipment.
Introduction
Kidney or renal failure is a debilitating condition in which the kidneys are no longer able to remove enough waste and excess fluid from the body. Failure of the kidney results in increased concentrations of these products in the body, which lead to adverse health effects. The most common treatments for kidney failure are kidney dialysis or kidney transplants. Worldwide, it is estimated that about 2.5 million patients undergo regular dialysis treatments.1,2 Although recently, both hemodialysis (HD) and peritoneal dialysis (PD) can be performed at home which saves patients some time, it is still an inconvenient, time consuming, and expensive process.3,4 In addition, of the world's population that receives dialysis treatments, 25% of them live in developing countries.1 The dialysis patient population growth rates in the developing countries are 12% per year due to increased access to dialysis treatments,1 meaning many people are not actually receiving treatment. In Sub-Saharan Africa, for example, economic and manpower factors have limited the regular maintenance HD treatments due to the absence of adequate technical support and frequent power outages.5 Thus worldwide, over one million people die per year due to end stage renal disease.6–8
Natural disasters also cause morbidity and mortality not only by the direct impact of trauma but also by interfering with the treatment of patients who have chronic diseases such as kidney failure because HD treatments are almost always performed in hospitals or in dialysis units.9 In addition, dialysis is a complex procedure that requires urban facilities such as water, electricity, and communication lines, which are also prone to damage by disasters. Disposable dialysis items (dialyzers, lines, needles, and concentrates) may also be destroyed or lost, and distribution of these items may be problematic as a result of transportation problems. It has been reported that the shortage of dialysate, water, and electricity created a dangerous situation for HD-dependent patients after a major earthquake struck northeastern Japan on 11 March 2011.10 Since the gigantic tsunami caused significant damage, particularly along the Pacific coast in the Tohoku region, dialysis patients in the affected region were sent to the dialysis facilities that had remained relatively intact. The capacity of these dialysis facilities was overwhelmed and they had to perform 2.5 hour HD treatments six times a day until other facilities recovered some functionality (HD is usually conducted over a 4 h duration three times per week).10 Therefore, there has been a great need to develop simpler and more accessible methods for the treatment of kidney failure.
The technique of HD is based on the principle of “diffusion”, which means clearance or removal of a high concentration of uremic toxins in the blood to a lower concentration solution through a semi-permeable membrane. Despite recent developments that have improved the permselective properties of the dialysis membranes,11–13 the convective diffusive technique is still an inconvenient, time consuming, and expensive process. From these perspectives, we focus on another principle of blood purification that utilizes a selective adsorption technique to remove uremic toxins from the patients’ blood. Recent improvements in water purification technologies have paved the way for achieving the efficient and selective removal of a wide range of molecules from a solution. Among them, zeolites have been looked at as a potential material to be able to selectively adsorb some uremic toxins and be used in applications as an artificial kidney.14–16 Zeolites are aluminosilicates with regular microporous structures that can be found naturally or produced synthetically.17 The pore size of a given type of zeolite is well defined and can thus specifically adsorb materials. These unique features, as well as the fact that zeolites are non-toxic and not degraded under physiological conditions, make zeolites an ideal candidate for use in artificial kidney applications.
In this study, we develop a zeolite–polymer composite nanofiber mesh as a wearable blood purification system without the requirement of specialized equipment (Fig. 1). The nanofiber is composed of poly(ethylene-co-vinyl alcohol) (EVOH) as the primary matrix polymer which prevents the zeolites from being released into the bloodstream. EVOH has already been used for many medical applications due to its blood compatibility.18–20 The composite fiber meshes were produced by a cost-effective electrospinning method using an EVOH solution blended with zeolites. Various zeolites are incorporated into the fibers to test their ability to adsorb creatinine from solution. The creatinine adsorption capability of the fibers is also investigated.
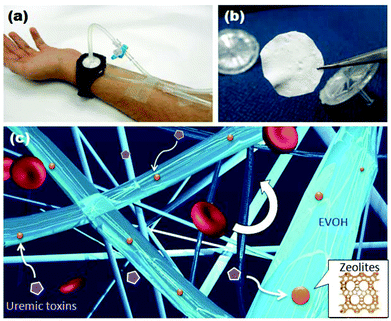 |
| Fig. 1 Photographs of a wearable blood purification system (a) and a zeolite–polymer composite nanofiber mesh (b). (c) The nanofiber is composed of blood compatible poly(ethylene-co-vinyl alcohol) as the primary matrix polymer and zeolites which are capable of selectively adsorbing uremic toxins. | |
Experimental section
Materials
1,1,1,3,3,3-Hexafluoroisopropanol (HFIP) was purchased from Sigma Aldrich (Tokyo, Japan). Hydrochloric acid, isopropanol (IP), poly(vinyl alcohol) (PVA), and creatinine were purchased from Wako Pure Chemicals (Tokyo, Japan). EVAL E105A (EVOH), with 44 ethylene mol% (from 1H-NMR), was kindly supplied by KURARAY (Okayama, Japan). HSZ-series zeolites 320-HOA (USY type), 500-KOA (L), 640-HOA and 690-HOA (Mordenite, whose Si/Al ratios are 18 and 240, respectively), 720-KOA (Ferrierite), 840-HOA (ZSM-5), 940-HOA and 980-HOA (Beta, whose Si/Al ratios are 37 and 500, respectively) were purchased from TOSOH (Yamaguchi, Japan).
Fiber fabrication
Electrospinning solutions were prepared using a solvent system of either a 70
:
30 IP–water mixture (labelled IP–water) or HFIP. EVOH was added in various concentrations of 5, 7, 8.5, and 10 w/v%. Solutions prepared in the 70
:
30 IP–water system were mixed at 70 °C while solutions prepared in HFIP were mixed at room temperature. The solutions containing zeolites were ultrasonicated in order to disperse the particles. Additionally, PVA–water solutions were prepared in a 80 mg L−1 concentration as a sacrificial layer. Electrospinning was performed in a Nanon-01A (MECC, Fukuoka, Japan). First, PVA nanofibers were fabricated as a sacrificial layer. Spinning parameters were kept constant at a 30 kV applied voltage, 0.8 mL h−1 solution flow rate, 15 cm working distance, and a 25 gauge pointed needle. For the EVOH or EVOH–zeolite composite fibers, the spinning parameters were kept constant at a 25 kV applied voltage, 1.0 mL h−1 solution flow rate, 10 cm working distance, and a 25 gauge pointed needle. The fibers were electrospun onto a sheet of aluminium foil on a stationary plate collector. Prior to characterization, the fibers were extracted from the foil in warm water to dissolve and wash off the sacrificial layer.
Fiber characterization
The fiber morphologies were observed using a Hitachi S-4800 field emission scanning electron microscope (SEM) (Hitachi, Tokyo, Japan). Prior to imaging, the samples were sputter coated with ∼3 nm platinum using an E-1030 Ion Sputterer (Hitachi). Lower resolution images were obtained using a NEOScope JCM-5000 table top SEM (JEOL, Tokyo, Japan). The fiber diameters were measured using the ImageJ software with ave. ± standard deviations (n = 40). The content of zeolites in the fibers was measured by TG-DTA analyses (TG-DTA6200, Seiko Instruments Inc., Chiba, Japan), which were conducted on 3 mg fiber meshes at a heating rate of 10 °C min−1 and set at 500 °C for 2 h. The incorporation of the zeolites in the nanofiber was also determined by energy dispersive X-ray spectroscopy (EDX, equipped in SEM, HORIBA, Kyoto, Japan).
Adsorption studies of creatinine
The adsorption capacity of the zeolites and fibers was measured using a V-650 UV-Visible spectrophotometer (JASCO, Tokyo, Japan). A UV calibration curve for creatinine in water was prepared based on the 234 nm absorption peak. Creatinine solutions of 190 μM concentrations were prepared in millipore water. The solution was separated into different vials with 5 mL aliquots into which zeolites or fibers were mixed. The vials were shaken at 37 °C in a TAITEC PERSONAL-11 water bath shaker (TAITEC, Saitama, Japan) for at least 12 h. For the free zeolite samples, the vials were centrifuged in order to settle the zeolite particles out of suspension. The UV absorption spectra of the samples were then measured and the amount of creatinine adsorbed calculated. The adsorption assay was also conducted under continuous flow with a 1 mL h−1 flow rate. Punched pieces of nanofiber membranes with a diameter of 10 mm were positioned in a syringe filter cartridge and sealed.21 Creatinine solution (190 μM) was introduced into the inlet of the cartridge to flow through the membrane and exit through the outlet. The UV absorption spectra of the circulating solution were continuously measured.
Results and discussion
Fabrication of EVOH nanofiber
During the last decade increased attention has been given to polymeric nanofibers because the nano-scale features provide an extremely large surface area and porosity, while the macroscopic features enable facile manipulation as a bulk matter.22–24 Among them, polymeric nanofibers fabricated by electrospinning have gained popularity because of their versatile and cost-effective fabrication method.25,26 Electrospinning is applicable to almost any soluble or fusible polymers and can yield a variety of continuous fibers with uniform diameters ranging from micrometers to nanometers. In general, the viscosity of the electrospun solution has a dominant effect on the jet behaviour of electrospinning. The 70
:
30 IP–water solvent system is commonly used in the literature for electrospinning of EVOH.27 As reported in the literature, it was found that these solutions could only be electrospun for a couple of hours after being removed from heating (70 °C) as the EVOH would start to come out of solution. In addition, the solution would readily harden on the needle tip, causing occasional clogging. In comparison, the solutions dissolved in HFIP, which the authors have not yet seen reported in the literature, could be electrospun very easily over many days and produced more consistent fibers.
The morphology of the fibers electrospun with various concentrations of EVOH in the IP–water or HFIP solvent systems is shown in Fig. 2(a–f). The 5 w/v% EVOH IP–water fibers (a) had a beaded morphology while all the other formulations produced fibers free of beading defects (c–f). In general, the fibers produced in the HFIP solvent system were thinner and possessed overall improved morphologies. Fig. 2(g) shows the average diameters of the EVOH nanofibers produced under various conditions. As is typical, the fiber diameter increased with the polymer concentrations of the electrospinning solution. With the IP–water solvent system, solutions with EVOH concentrations of 10 w/v% or greater could not be produced due to rapid precipitation upon removal from heat. Based on the samples produced, it was determined that the 7 w/v% EVOH HFIP electrospinning solution was optimal for producing EVOH based fibers with a defect-free morphology and small fiber diameters. Thus, this solution was used as the base solution for all subsequent experiments.
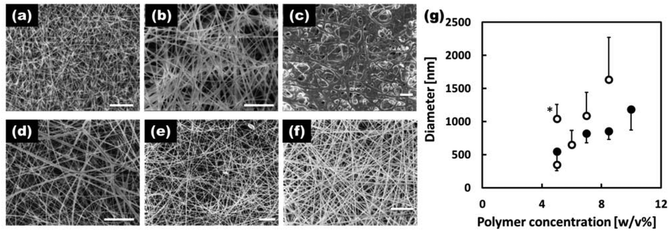 |
| Fig. 2 SEM images of electrospun EVOH nanofibers produced from IP–water with the polymer concentration of 5 w/v% (a), 7 w/v% (b), and 8.5 w/v% (c), and HFIP with the polymer concentration of 5 w/v% (d), 7 w/v% (e), and 8.5 w/v% (f) (scale bar: 20 μm). (g) Average diameters of electrospun EVOH nanofibers produced from IP–water (open) and HFIP (closed) under various polymer concentrations (n = 40). *Includes the size of beads. | |
Fabrication of zeolite–EVOH nanofiber composite
Another advantage of electrospinning is its capability of creating composite nanofibers. For example, functionalized composite nanofibers have been developed by electrospinning polymers blended with nanoparticles,28 carbon nanotubes,29 ceramics30 or biomolecules.31 We have recently developed hyperthermia nanofibers by electrospinning temperature-responsive polymers blended with magnetic nanoparticles and anti-cancer drugs.32 Both the generated heat from magnetic nanoparticles and released drugs successfully induced apoptosis of cancer cells by hyperthermic and chemotherapeutic effects, respectively. To prepare these composite nanofibers, however, electrospinning conditions have to be carefully controlled during the whole process because it can undergo clogging of the needle tip. In this study, the polymer–zeolites mixture was simply ultrasonicated for over 1 h prior to electrospinning. Fig. 3(a) shows SEM images of the EVOH fibers blended with 940 HOA zeolites. Because the average particle size of zeolites (approximately 2–3 μm) was significantly larger than the fiber diameter (Fig. S1†), bead-like formations were observed. Since the size of beads was found to be 2–20 μm, zeolite particles may partially form aggregates in the fiber. However, between the beads, smooth and continuous fibrous structures were still maintained and each zeolite particle was coated with EVOH (no free zeolites were observed). To determine the successful incorporation of zeolites within the fibers, SEM/EDX mapping images of the fibers were obtained (Fig. 3(b and c)). The elemental mapping images of Si and O atoms, which correspond to zeolite components, show that zeolites are successfully incorporated within the fibers. The amounts of the incorporated zeolites were also determined by TG-DTA analyses. It was determined that over 90% of zeolites were successfully incorporated into nanofibers (Fig. S2†). Fig. 4 plots the zeolite content against feed concentration. There is a good correlation between incorporated amounts and feeding amounts. These results indicated that a nanofiber composite composed of adsorbent particles and a blood compatible polymer was successfully fabricated using electrospinning techniques.
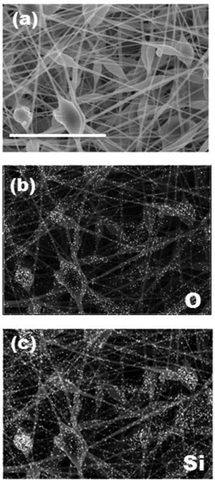 |
| Fig. 3 SEM/EDX mapping images of beta-type 940-HOA zeolite (10 wt%)–EVOH nanofiber composite produced from 7 w/v% HFIP (scale bar: 8 μm). (a) SEM, (b) O-mapping, and (c) Si-mapping. | |
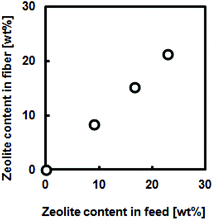 |
| Fig. 4 Relationship between zeolite contents in the fiber and their feed concentrations. The weight percentages of zeolites in nanofibers were calculated from the weight loss of nanofiber composites by thermogravimetric analysis. | |
Creatinine adsorption capacity of zeolite–EVOH nanofiber composite
Initially, the adsorption capacities of the various zeolites under varying conditions were measured. Table S1† summarizes the pore size and composition of zeolites used in this study. The 320-HOA and 500-HOA zeolites have smaller pore sizes than that of creatinine (0.71 nm × 0.80 nm × 0.30 nm). Therefore, they were non-adsorbent to creatinine (Fig. S3†). The 980-HOA and 720-KOA zeolites were also found to have very low creatinine adsorption capacities while the 640-HOA and 690-HOA zeolites have mid-range 130 μmol g−1 adsorptions. The zeolites with the greatest creatinine adsorption capacities (220 μmol g−1) were found to be the 840-HOA and 940-HOA zeolites (ZSM-5 and Beta type, respectively). They have similar [Si]/[Al] ratios of around 37–38. Therefore, the [Si]/[Al] ratio is also important for creatinine adsorption. The reusability of the zeolites was also tested by first saturating the zeolites with creatinine, washing the saturated zeolites in 1 M HCl, and then testing the adsorption capacity of the washed zeolites as with the as-received zeolites. It was found that the 940-HOA retained much more of its adsorption capacity after the simple washing process than the 840-HOA and thus a higher reusability (Fig. S3†).
Fig. 5(a–d) shows SEM images of the EVOH fibers blended with 720-KOA (Ferrierite), 840-HOA (ZSM-5), 940-HOA (beta), and 980-HOA (beta), respectively. The composite nanofiber meshes were successfully produced regardless of zeolite species tested in this study. Fig. 5(e) compares the creatinine adsorption capacity of free zeolites and those in the fiber by zeolite mass. Although the barrier properties of the EVOH matrix lowered the creatinine adsorption capacity of the zeolites in the fiber when compared with adsorption to free zeolites, the adsorption capacities increase according to the same trend as free zeolites: 720-KOA < 980-HOA < 840-HOA < 940-HOA. This result indicates that zeolites still keep the adsorbent ability of creatinine in the fibers.
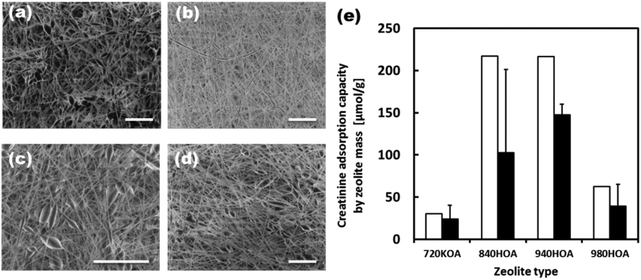 |
| Fig. 5 SEM images of zeolite–EVOH nanofiber composites with various types of zeolites (10 wt%). (a) 720-KOA (Ferrite), (b) 840-HOA (ZSM-5), (c) 940-HOA (beta), and (d) 980-HOA (beta) (scale bar: 20 μm). (e) Creatinine adsorption capacities of free zeolites (open) and those in the fiber by zeolite mass (closed) after 24 h of immersion (n = 3). | |
Although the barrier properties of the EVOH matrix lowered the creatinine adsorption capacity of the zeolites in the fiber when compared with adsorption to free zeolites, their adsorption capacity of 940-HOA composite fiber was still 67% of the free zeolites because EVOH is known as a semi-crystalline polymer.33 Thus, the 940-HOA zeolite was chosen as the optimal zeolite to be used in the zeolite–EVOH composite fibers. Fig. 6 shows the creatinine adsorption capacity of the composite fibers with various 940-HOA zeolite contents. The adsorption capacity of the fiber mass was proportionally increased by increasing the zeolite content in nanofibers (open bars in Fig. 6(a)). On the other hand, the adsorption capacity of the zeolite mass was decreased by increasing the zeolite content (open bars in Fig. 6(b)). In other words, adsorption efficiency became low when large amounts of zeolite were incorporated into the nanofibers. This may be due to aggregation of the zeolites at higher concentrations, lowering their surface area for adsorption. These results indicate that not only zeolite's type but also electrospinning conditions (e.g., concentration or solvent) have to be carefully controlled to obtain a higher capacity of creatinine adsorption. We have also demonstrated the adsorption assay of creatinine under a continuous flow condition (closed bars in Fig. 6(a and b)). The absorbance capacity became a plateau within a few hours. Unfortunately, the value is approximately 20% of that obtained in the absence of flow. This result was unexpected because there are higher possibilities for creatinine to interact with zeolite in the presence of flow. A possible explanation for this result is that although continuous flow may promote creatinine to interact with the fibers when passing through membrane, it may also prevent the creatinine from entering into the zeolites within. In other words, the pores on the surface of zeolite particles were quickly saturated in the presence of flow and thus, creatinine was prevented from diffusing into the deep pores. This result indicates that the design of the filter setup including flow rate and area of membrane is very important to maximize the adsorption capacity of the zeolite–fiber composite.
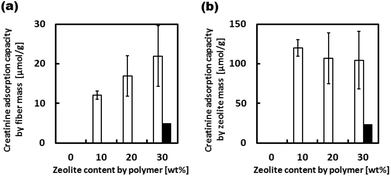 |
| Fig. 6 Creatinine adsorption capacity of 940-HOA zeolite nanofibers by fiber mass (a) and zeolite mass (b) in the absence (open bar) or presence (closed bar) of flow (n = 3). The absorption capacities were obtained after 24 h or 5 h for batch (no flow) or circulating (under flow) experiments, respectively. | |
The human body produces roughly 4 mmol of creatinine per hour (148 μmol kg−1 day−1
34). It can be estimated that around 17 g of 940-HOA zeolite or 170 g of the zeolite–EVOH nanofiber composite would be needed to adsorb a daily production of creatinine. Theoretically, this can be achieved by preparing 10 cm3 filter membranes using the zeolite–EVOH nanofiber mesh. Although this membrane size is still large for a wearable system, there are several potential approaches to achieve higher absorption capacities. Possible ways to achieve this include increasing the ability of creatinine to penetrate into the fibers and to increase the effective surface area. The surface area could be increased by either reducing the fiber diameter or using a sacrificial polymer blended with the EVOH to increase porosity. Future work will explore these various possibilities of zeolite–polymer composite fibers for their practical uses in the human body.
Conclusions
In summary, this study investigated the fabrication of zeolite–polymer composite fibers by the electrospinning process for the adsorption of creatinine. The carrier polymer was chosen to be an EVOH with an ethylene content of 44 mol%. It was found that the best base polymer solution was 7 w/v% EVOH in HFIP. The zeolite with the highest creatinine adsorption capacity was found to be the beta type 940-HOA zeolite whose Si/Al ratio is 37. The incorporation of zeolites into nanofibers possesses both nano- and macro-scale advantages; the nano-scale features provide extremely large surface area and porosity while the macroscopic features enable facile manipulation as bulk matter. The fabricated composite fibers were capable of adsorbing creatinine from solution. Although the adsorption capacity is still not high enough, the proposed composite fibers have the potential to be utilized as a new wearable blood purification system, especially in low infrastructure areas such as disaster sites and the developing world.
Acknowledgements
The authors would like to express their gratitude to the Grants-in-Aid for Young Scientists (Start-up) (24800085) from the Ministry of Education, Culture, Sports, Science and Technology (MEXT), Japan. The authors are grateful to Dr Yusuke Yamauchi (NIMS) for helpful discussions on zeolites.
Notes and references
-
Fresenius Medical Care Deutschland GmbH, ESRD Patients in 2012 - A Global Perspective, 2013.
- R. J. Glassock and C. Winearls, Nephron. Clin. Pract., 2008, 110, c39 CrossRef PubMed.
- A. Harris, B. A. Cooper, J. J. Li, L. Bulfone, P. Branley, J. F. Collins, J. C. Craig, M. B. Fraenkel, D. W. Johnson, J. Kesselhut, G. Luxton, A. Pilmore, M. Rosevear, D. J. Tiller, C. A. Pollock and D. C. Harris, Am. J. Kidney Dis., 2011, 57, 707 CrossRef PubMed.
- K. Baboolal, P. McEwan, S. Sondhi, P. Spiewanowski, J. Wechowski and K. Wilson, Nephrol. Dial. Transplant., 2008, 23, 1982 CrossRef PubMed.
- E. L. Bamgboye, Kidney Int., 2003, 63, S93 CrossRef.
- W. G. Couser, G. Remuzzi, S. Mendis and M. Tonelli, Kidney Int., 2011, 80, 1258 CrossRef PubMed.
- A. K. Bello, E. Nwankwo and A. M. El Nahas, Kidney Int., 2005, 68, S11 CrossRef PubMed.
- A. M. El Nahas and A. K. Bello, Lancet, 2005, 22, 365 Search PubMed.
- M. S. Sever, E. Erek, R. Vanholder, A. Kalkan, N. Guney, N. Usta, C. Yilmaz, C. Kutanis, R. Turgut and N. Lameire, J. Am. Soc. Nephrol., 2004, 15, 1071 CrossRef.
- M. Nangaku and T. Akizawa, Kidney Int., 2011, 79, 1037 CrossRef PubMed.
-
High-Performance Membrane Dialyzers, Contribution to Nephrology, ed. A. Saito, H. Kawanishi, A. C. Yamashita and M. Mineshima, Karger, Basel, 2011 Search PubMed.
- K. Namekawa, M. Matsuda, M. Fukuda, A. Kaneko and K. Sakai, J. Artif. Organs, 2012, 15, 185 CrossRef CAS PubMed.
- K. Namekawa, A. Kaneko, K. Sakai, S. Kunikata and M. Matsuda, J. Artif. Organs, 2011, 14, 52 CrossRef CAS PubMed.
- D. Bergé-Lefranc, C. Vagner, R. Calaf, H. Pizzala, R. Denoyel, P. Brunet, H. Ghobarkar and O. Schäf, Microporous Mesoporous Mater., 2012, 153, 288 CrossRef PubMed.
- D. Bergé-Lefranc, H. Pizzala, J. L. Pailaud, O. Schäf, C. Vagner, P. Boulet, B. Kuchta and R. Denoyel, Adsorption, 2008, 14, 377 CrossRef PubMed.
- V. Wernert, O. Schäf, H. Ghobarkar and R. Denoyel, Microporous Mesoporous Mater., 2005, 83, 101 CrossRef CAS PubMed.
-
S. M. Auerbach, K. A. Carrado and P. K. Dutta, Handbook of Zeolite Science and Technology, CRC press, 2003 Search PubMed.
- S. Itoh, C. Suzuki and T. Tshuji, J. Biomed. Mater. Res. A, 2006, 77, 294 CrossRef PubMed.
- V. Sirolli, E. Ballone, S. Di Stante, L. Amoroso and M. Bonomini, Int. J. Artif. Organs, 2002, 25, 529 CAS.
- M. Boromini, S. Stuard, M. P. Carreno, N. Settefrati, P. Santarelli, N. Haeffner-Cavaillon and A. Albertazzi, Nephron, 1997, 75, 402 CrossRef PubMed.
- A. L. Golden, C. F. Battrell, S. Pennell, A. S. Hoffman, J. J. Lai and P. S. Stayton, Bioconjugate Chem., 2010, 21, 1820 CrossRef CAS PubMed.
- Y.-J. Kim, M. Ebara and T. Aoyagi, Angew. Chem., Int. Ed., 2012, 51, 10537 CrossRef CAS PubMed.
- G. D. Fu, L. Q. Xu, F. Yao, K. Zhang, X. F. Wang, M. F. Zhu and S. Z. Nie, Appl. Mater. Interfaces, 2009, 1, 239 CrossRef CAS PubMed.
- Z.-M. Huang, Y.-Z. Zhang, M. Kotaki and S. Ramakrishna, Composite. Sci. Technol., 2003, 63, 2223 CrossRef CAS.
- S. Ramakrishna, K. Fujihara, W.-E. Teo, T. Yong, Z. Ma and R. Ramaseshan, Mater. Today, 2006, 9, 40 CrossRef CAS.
- Y. Zhang, C. T. Lim, S. Ramakrishna and Z. M. Huang, Recent development of polymer nanofibers for biomedical and biotechnological applications, J. Mater. Sci. Mater. Med., 2005, 16, 933 CrossRef CAS PubMed.
- E.-R. Kenawy, J. M. Layman, J. R. Watkins, G. L. Bowlin, J. A. Matthews, D. G. Simpson and G. E. Wnek, Biomaterials, 2003, 24, 907 CrossRef CAS.
- Y. Duan, J. Jia, S. Wang, W. Yan, L. Jin and Z. Wang, J. Appl. Polym. Sci., 2007, 15, 1208 CrossRef.
- K. Saeed, S.-Y. Park, H.-J. Lee, J.-B. Baek and W.-S. Huh, Polymer, 2006, 47, 8019 CrossRef CAS PubMed.
- J. R. Venugopal, S. Low, A. T. Choon, A. B. Kumar and S. Ramakrishna, Artif. Organs, 2008, 32, 388 CrossRef CAS PubMed.
- Z.-G. Wang, L.-S. Wan, Z.-M. Liu, X.-J. Huang and Z.-K. Xu, J. Mol. Catal. B: Enzym., 2009, 56, 189 CrossRef CAS PubMed.
- Y.-J. Kim, M. Ebara and T. Aoyagi, Adv. Funct. Mater., 2013, 23, 5753 CrossRef CAS.
- S. Aucejo, C. Marco and K. Gavara, J. Appl. Polym. Sci., 1999, 74, 1201 CrossRef CAS.
- W. E. Mitch, V. U. Collier and M. Walser, Clin. Sci., 1980, 58, 327 CAS.
Footnote |
† Electronic supplementary information (ESI) available: Particle size distribution from DLS measurement on the 940-HOA zeolite, thermogravimetry curves for zeolite–EVOH nanofiber composites produced from 7 w/v% HFIP, properties of zeolites used in this study and creatinine adsorption capacity of various zeolites. See DOI: 10.1039/c3bm60263j |
|
This journal is © The Royal Society of Chemistry 2014 |