DOI:
10.1039/D4VA00039K
(Critical Review)
Environ. Sci.: Adv., 2024, Advance Article
Exploring sustainable adsorbents to mitigate micro-/nano-plastic contamination: perspectives on electrospun fibrous constructs, biochar, and aerogels
Received
7th February 2024
, Accepted 11th June 2024
First published on 10th July 2024
Abstract
In recent years, the issues pertaining to the micro-/nano-plastics (MNP) pollution in urban water have escalated due to their detrimental environmental consequences, which not only disrupt aquatic habitats and harm marine life but also serve as vectors for toxic pollutants, potentially entering the food chain and posing risks to human health. Although conventional techniques such as filtration, sedimentation, and electrocoagulation have been extensively utilized for MNP removal, ongoing concerns persist regarding their effectiveness, sustainability, and cost implications. Hence, it is imperative to critically assess the performance of conventional techniques in addressing MNP-induced pollution and to shed light on the potential of some emerging technologies as promising next-generation solutions. However, the dearth of standardized approaches and the scarcity of comprehensive data contribute to the disturbing extent of uncertainties in utilizing such techniques to address MNP pollution. Therefore, the current review theoretically emphasizes on innovative use of biochar, electrospun fibers, and aerogels as sustainable adsorbents for managing MNP pollution. Further, it offers a systematic overview elucidating the foundational understanding pertaining to the sources, fate, and transport dynamics of microplastics within the environment. Apart from this, the article explores the integration of such adsorbents into existing water treatment systems and examines the associated challenges and future perspectives in real-world applications. Thus, the contextual review provides valuable insights into designing next-generation technologies aimed at controlling MNP pollution in water systems which not only enhances the understanding of the fate and transport mechanisms of emerging MNP pollutants treatment of water to make it portable but also assists material designers in evaluating and refining existing methodologies and thereby promoting a multi-faceted and synergistic approach to combat the complex issue of MNP pollution.
Environmental significance
The ever-increasing threat pertaining to micro-/nano plastics pollution has sparked alarm across the globe due to the severe repercussions for both the environment and human health. The persistence of these particles in the environment poses a concern due to their ability to absorb and transport toxic contaminants, resulting in bioaccumulation in aquatic creatures and subsequently influencing the food chain. These particles can harm marine life, ranging from plankton to bigger creatures, causing food chain disturbances and ecological imbalances. The assimilation of MPs by marine creatures can lead to physical harm, toxicity, and the transmission of pollutants up the food chain, thereby affecting human populations dependent on seafood. Thus, this comprehensive review article gives insights into the limitations of traditional methods for removing MNP and recommends a paradigm shift towards new sustainable adsorbents, especially biochar, electrospun fibrous constructions, and aerogels. The investigation of these novel materials as next-generation solutions represents a significant divergence from traditional options which not only advances our understanding of MNP pollution but also provides a strategic framework for future research, fostering a multifaceted and synergistic solution to protect the environment from the pervasive threat of MNP.
|
1. Introduction
The overexploitation of single-use plastics (>300 million tons each year) and their mismanagement led to the accumulation of micro and macro plastics in aquatic, terrestrial, and atmospheric surroundings, resulting in MNP-induced pollution as an emerging problem, considering the fact that it has percolated down through the soil streams all through and dispersed up above up to the cloud systems. Reportedly, plastic production globally exceeds ∼450 million metric tons (MMT) annually, and without intervention, it is projected to double or triple by 2060.1 As per the report obtained from the International Union for Conservation of Nature (IUCN), ∼3.2 million tons of plastic waste are dumped into the atmosphere in a yearly manner, while ∼1.5 million tons of which end up in the oceans. Consequently, the amount of plastic waste generated globally has not only risen up to ∼353 MMT in 2019 (OECD 2022) but is also estimated to surge ∼6-fold by 2060.2 Meanwhile, the prolonged persistence of plastics (which can last up to ∼1000 years) in the environment coupled with their potential to release harmful chemicals (such as bisphenol A and related chemicals, phthalates, per and poly-fluoroalkyl substances (PFAS), dioxins, toxic metal ions (lead and cadmium)) and/or other toxic additives during degradation affects the aesthetics of natural habitats but also disrupts thriving ecosystems. For instance, it has shown instances of devastating effects on marine life (such as seabirds, turtles, fish, and marine mammals) as they often mistake plastic debris for food, resulting in the ingestion and entanglement, ultimately causing injury, suffocation, and even death and decimation.3 The most significantly susceptible ecosystem component to microplastics (MPs) is water, which has a vicious nexus and non-escapable interdependence. Due to the invariably lesser density of MPs, generally, they incline to either settle down on the ground or disperse in the air depending on their size and substrate-specific functionality on MPs.4 However, most of the MPs eventually get transported to the water system which leaves the water extremely contaminated, to the extent of alarming malignancy.5
Further, with passing years, plastic waste can break down into smaller fragments (<5 μm) i.e., MPs (∼0.1 μm to 5 mm) and nano-particles (NPs) (0.001–0.1 μm) by the exposure to the ultraviolet (UV) irradiation and weathering processes, thereby causing its infiltration into aquatic and terrestrial environments.6 For example, polystyrene (PS)-based MPs (∼10 μm) can induce oxidative stress in epithelial cells as well as the human brain and adsorb other toxic organic pollutants (such as polycyclic aromatic hydrocarbons (PAHs), and persistent organic pollutants), which may eventually facilitate their transfer into different organs and tissues.7 NPs with smaller size, lesser density, and higher surface area, inherit the negative impacts of MPs on the aqueous environment and may exert more serious impacts, such as ingestion by different aquatic and terrestrial organisms, piling up in various body parts like liver, gills, blood streams of organisms which may cause metabolic disorders, severe infections and disruption of various cycles of the body.8 For instance, Wang et al. reported that PS-based MNP can not only decrease the cell viability of Caco-2 cells (derived from colon carcinoma) but also tend to induce cytotoxicity linked to oxidative stress, epithelial cell injury, disruption of intestinal barrier function, and altered detoxification processes.9 In another in vitro simulation experiment conducted by Fournier et al. cultured gut microbiota obtained from fecal samples observed a significant shift in the community composition of the gut microbiota after interaction with polyethylene terephthalate (PET)-based MNP, which had diameters ranging from ∼30 to ∼140 μm. Such interaction resulted in an increased proportion of clostridium and Bacteroides.10
Indeed, the concentration of MNP has experienced a significant increase (∼10-fold) in the last two decades (i.e. from 2000 to 2020), indicating a growing accumulation of these particles in the three functional components of our ecosystem (air, water, and soil). For example, Kawecki et al. conducted a study and developed a model with parameterization specific to Switzerland. The findings revealed that ∼540 ± 140 grams per capita per year (g cap−1 y−1) of seven analyzed polymers were released into the soil as macro- and MPs combined. In addition, the distribution of these polymers was further divided compositionally into ∼98 ± 50 g cap−1 y−1 of High-Density Polyethylene (HDPE), ∼94 ± 34 g cap−1 y−1 of Low-Density Polyethylene (LDPE), ∼24 ± 13 g cap−1 y−1 of PS, ∼16 ± 12 g cap−1 y−1 of EPS, ∼65 ± 36 g cap−1 y−1 of polyvinyl chloride (PVC), ∼200 ± 120 g cap−1 y−1 of PET and ∼126 ± 43 g cap−1 y−1 of PP.11 According to data published by the United Nations Educational Scientific and Cultural Organization (UNESCO), it is estimated that as of 2022, the ocean contained a staggering ∼50–75 trillion pieces of plastic and MPs.12 Meanwhile, even in remote areas like the Italian Alps, broken ice was found to contain a maximum of ∼74.4 MNP per kg, while on the exterior periphery of the glacier, it was projected to be ∼131 million MNP (even in the Tibetan Plateau, which boasts some of the highest altitudes in the world).13 Even in high-altitude places (like the Tibetan Plateau), with the highest elevations in the world (with an average height of >4000 meters), the presence of MNP has been identified in water streams like lakes and rivers,14 which suggests that MNP pollution is not limited by geographic boundaries and can reach even the most remote and seemingly isolated areas. In a recent study, researchers have discovered that the spread of MPs through atmospheric transport depends on their shape. In a model, fibers with a length of up to ∼1.5 mm were able to reach the most remote places on earth up to the stratosphere, while spheres of the same mass settled much closer to the respective regional plastic sources.15
The presence of NPs can disrupt the natural cycles and processes in the bodies of various organisms. For example, NPs can interfere with endocrine functions, affecting hormone production and regulation, which can have cascading effects on reproductive cycles, development, and behavior of organisms.16 Moreover, the COVID-19 pandemic has further exacerbated this issue by increasing the extensive use of single-use plastics, such as masks, gloves, and personal protective equipment (PPE), combined with the surge in packaging waste from online shopping and food delivery services.17 Globally, ∼8 million tonnes of pandemic-related plastic trash are created, with more than ∼25
000 tonnes entering the ocean.18 In another study, the global amount of face masks entering the oceans experienced a significant increase in 2020, with an estimated additional ∼1.56 billion masks contributing to marine pollution during that year alone.19
Conventional decontamination removal technologies including coagulation, dissolved air flotation, physical separation technologies, membrane disc-filters, sand filtration, coagulation, enhanced oxidation, magnetic separation, chemical agglomeration, and membrane filtration (such as ultrafiltration, microfiltration, nanofiltration, membrane bioreactors, and reverse osmosis) have demonstrated promising results in addressing the issue of MNP by exhibiting high removal efficiencies of >90%, along with the possibility of retrofitting at minimal cost compared to alternative techniques.20 However, it is critical to comprehend that the performance of the membrane filters is affected by different parameters such as materials used in membrane preparation, orientation, profile, size, wettability feature, and surface area.21 The pore sizes of conventional membranes may not be optimal for the removal of certain pollutants such as NPs due to their size scale fluctuations, thereby posing challenges in achieving adequate retention.22 Furthermore, conventional membrane filtration is associated with higher costs and limited process intensification, which can impact the setup and performance of the filtration system.23 Therefore, considering the challenges associated with conventional membrane-based technologies, there is an urgent need for further research on innovative, sustainable, and renewable technologies for the effective removal of MNP that can contribute to the development of efficient and eco-friendly solutions for the removal of MNP from various environments. In this context, Isaeva et al. summarized the report on modern carbon-based adsorbents, including graphene oxide (GO), carbon nanotubes (CNTs), metal–organic framework (MOF)–carbon composites, and MCs (microporous carbons) obtained through controlled pyrolysis of MOF-precursors for isolating MPs.24,25 In another study, Yang et al. investigated the performance of chitosan (CS)-based hydrogel systems for the removal of various types of pollutants especially MPs in an efficient manner.26 The 3D network structure of hydrogel possesses outstanding water holding and adsorption capacity along with reversible swelling ability enabling the removal of a wide variety of MNP. Recently, Goh et al. discussed the significance of multifunctional and flexible nanomaterials by recognizing and eradicating the MPs particles through different mechanistic approaches such as adsorption, advanced oxidation process, magnetic separation, membrane filtration, and many more.27 To resolve the complexity with efficacy and to explore the benefits of nanomaterials in MPs treatment processes in a more sustainable manner, the overview intends to stretch further to multifunctional adsorbents like aerogels, biochar, and electrospun mats. In this regard, Yon et al. synthesized durable zinc MOF-based composite material ZIF-8@aerogel by in situ grown ZIF-8 on wood aerogel fibers, and it is being utilized for the successful removal of MPs including poly(1,1-difluoroethylene) (60–110 nm) and PS (90–140 nm) from the aquatic medium with a removal efficiency of ∼91.4% and 85.8%, respectively.28 Further, Zhu et al. synthesized a modified porous cellulose nanofibrous-based aerogel through the chemical modification of bleached eucalyptus pulp by 2,2,6,6-tetra methyl piperidine-1-oxyl and 2,3-epoxy propyl trimethyl ammonium chloride. This aerogel demonstrated highly efficient separation of different PS-MPs, achieving near 100% efficiency for carboxylate-modified PS and ∼75% for amine-modified PS.29 Wang et al. have substantiated the effectiveness of corn straw and hardwood-derived biochar in MPs removal through experimental studies. The results revealed that biochar filters exhibit significant capacity for the removal and immobilization of 10 μm diameter MPs spheres (>95%), surpassing the performance of similar grain-sized sand filters studied.30 Rist et al. fabricated bio-based polyamide 6.9-based electrospun membrane as filters, prepared from plant oil-based azelaic acid and electrospun from chloroform/formic acid to produce self-standing electrospun nonwovens, with high mechanical properties and resistance to solvents. The electrospun mats demonstrated high efficiencies of up to 99.8% for the filtration of PS microparticles (PS-MPs) from water.31
A comprehensive overview of the previously published review articles related to MPs removal is listed in Table 1. To date, there has been no report investigating an overall systematic compilation on the understanding of such emerging frameworks that could be useful for potential MPs removal in water treatment. To address these critical knowledge gaps, the objectives of this work are to provide insights into the sources of nano- and MPs, the implications of pandemics such as COVID-19 on MPs pollution, the journey of such plastics, their environmental fate, followed by standards for the detection of MNP contaminants in water and soil. By critically evaluating the limitations of conventional techniques that are being used for the removal of MNP, the current review aims to shift the attention towards the exploration of the recently developed material-specific sustainable ways i.e., the use of biochar, electrospun micro/nanofibrous assemblies, and aerogels as emerging solutions in the field of MNP filtration from soil and water ecosystems. Additionally, it further delves into the challenges in implementing these materials for the removal of MNP and highlights the research gaps in this critical aspect of concern. Summatively, the paper offers strategies and recommendations for future research to improve the application of these adsorbents in real water treatment systems, thereby paving the way for a more comprehensive and synergistic approach to combatting the complex issue of MNP pollution.
Table 1 A comprehensive overview of the previously published review articles related to MPs removal
S. no. |
Paper title |
Highlights |
Aspects not discussed/limitations |
Ref. |
1 |
Review of MPs distribution, toxicity, analysis methods, and removal technologies |
Major MPs were reported from PET, polyurethane (PU), PS, polyvinyl alcohol (PVC), polypropyelene (PP), polyethyelene (PE), and polyamide (PA) |
Risk factors and toxicity of the MPs in the ecosystem and human body have not been mentioned fully |
32 |
Different sampling, pretreatment, and separation techniques (density-based), were discussed |
All the mentioned MPs removal techniques are not that efficient and the efficiency is only 70% |
Analytical analysis methods for different MPs applications have been discussed in detail |
Conventional removal techniques such as wastewater treatment plants (WWTPs), physical (flotation, sedimentation, and filtration), chemical, and biological technologies (activated sludge treatment, aerobic and anaerobic digestion, lagoons, and septic tanks) have been discussed in full detail |
2 |
A critical review of MPs removal from the environment |
The study focussed on the source and occurrence of MPs studies on the physical, chemical, and biological techniques for MPs removal from the environment have been critically carried out along with the advantages and limitations of each removal technique are identified |
Major research has been done on the dissemination pathway, environmental occurrence, and distribution in the air, soils, sediments, rivers, lakes, and oceans |
33 |
The lack of investigation on MPs ecotoxicity and remediation technologies has been profound and needs attention to address the research gaps |
3 |
MPs pollution focused on sources, distribution, contaminant interactions, analytical methods, and wastewater removal strategies: a review |
The evolution of MPs sources, the toxicological effect on the environment and human life, its extraction technique, and analysis have been briefly mentioned |
Standardization is not available, leading to the misidentification of MPs, future work on the precise sampling, treatment, solution preparations, and equipment was required for more precise evaluation |
34 |
A comprehensive study on the existing and conventional removal techniques such as WWTPs, biological wastewater treatment with microfiltration or ultrafiltration in a membrane bioreactor, electrocoagulation, and sol–gel method were discussed |
Several new hybrid and advanced treatment processes have not been explored properly, whereas the existing mentioned technology has been tested on a laboratory scale only with a few MPs |
A new approach to the utilization of coated Fe nanoparticles to magnetize plastics, allowing magnetic extraction and the isolation of MPs, and dynamic membrane technology was explored |
New treatment alternatives must continue to be explored and tested in real samples, where a mixture of MPs and different contaminants is present. It is also important to approach both lab tests and the industrial scale to ensure the viability of strategies to be implemented in future wastewater treatments |
4 |
A review of methods for extraction, removal, and stimulated degradation of MPs |
The extraction of MPs from water probes, techniques for identification of polymer types, and strategies for the stimulated natural and artificial degradation of the material are comprehensively discussed |
Sophisticated laboratory devices combining optical microscopy with FTIR or Raman as well as FTIR-TGA couples for the analysis of MPs are not explored |
35 |
Recent trends in the recycling of MPs and pollution control were explored in this study |
The review covers about two hundred recent sources and briefly explains the most appropriate techniques for a given task |
5 |
A review on MPs separation techniques from environmental media |
Separation techniques for MPs removal from environment matrices are explored |
Recovery rate, lack of comparative data, low fiber separation, contamination, quality assurance, and separation measures are not thoroughly discussed |
36 |
Different environmental media like soil, water, and sediments have been considered |
Lack of information about MPs separation from the soil |
Removal efficiency of the proposed system with advantages and limitations has been mentioned |
MPs separation helps to understand system performance and pollution estimation |
![[thin space (1/6-em)]](https://www.rsc.org/images/entities/char_2009.gif) |
Thematic focus of the present review |
Provides insights into the sources of nano- and micro-plastics, and implications of the pandemics such as COVID-19 on MPs pollution |
Describes environmental consequences followed by the conventional methods used for the removal of MNP contaminants in water and soil |
Explores some of the recently developed material-specific sustainable ways i.e., the use of biochar, electrospun micro/nanofibrous assemblies, and aerogels as emerging solutions in the field of MNP filtration |
Delves into the challenges in implementing these materials for the removal of MNP and highlights the research gaps in this critical aspect of concern |
Offers strategies and recommendations for future research to improve the application of these adsorbents in real water treatment systems |
2. Methodology
A systematic literature review was meticulously conducted over a span from December 2022 to November 2023, employing a three-step methodology that encompassed (i) identification and compilation of scholarly materials, (ii) screening of articles and meticulous data acquisition, and (iii) comprehensive reporting and widespread dissemination of findings. Various esteemed electronic and scientific databases such as Google Scholar, ACS Publication, ScienceDirect, Springer Link, and Web of Science were utilized to explore the originally published research and review articles from 2016 to 2023. The keywords/search terms such as the emerging level of MP, their harmful effect, toxicity assessment, and emerging adsorbents for MNP removal were used. Based on the title, keyword, and abstract of the extensive pool of scholarly works, similar types of articles (∼180) were selected for comparative analysis. The conventional techniques utilized for the removal of MNP are thoroughly analyzed, taking into account their limitations including sustainability, and efficacy parameters. Additionally, recently available relevant references pertaining to the utilization of electrospun fibers, biochar, and aerogels as potential adsorbent systems for MNP were meticulously curated and integrated into the review.
The book chapters, letters, opinions/perspectives, abstracts from conferences/seminars, encyclopedias, and viewpoints were not considered for the future purpose. Throughout the research endeavor, any kind of queries, disputes, and confusions were effectively resolved through collaborative group discussions, paper revisions, and laboratory meetings. Furthermore, the review, as well as the research article, were systematically categorized according to various aspects such as the escalating MNP from diverse origins, their direct and indirect consequences on the environment and living species, conventionally available methodology, and emerging techniques/systems for their treatment in eco-friendly/sustainable manner. The obtained articles were incorporated into Mendeley software for reference citation purposes, with duplicate results systematically removed. The schematic illustration of the performed screening process is shown in Fig. 1.
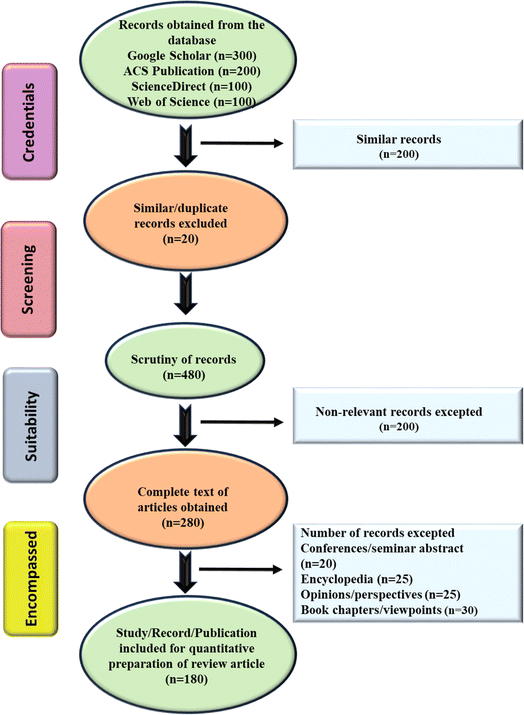 |
| Fig. 1 A schematic representation of the process involved in screening the databases and records which helped in the effective outcome of this study. | |
3. A quantitative outlook on the sources of MPs
MNP originate from a diverse range of sources, including adhesives, paints, coatings, pharmaceuticals, biomedical materials, electronics, magneto/optoelectronic devices, medical equipment, plastic sheets in the food department, marine fisheries, disposable materials for carriers, precursors used in industries and agricultural films.37 The schematic representation concerning the diverse sources of MNP generation is illustrated in Fig. 2a.
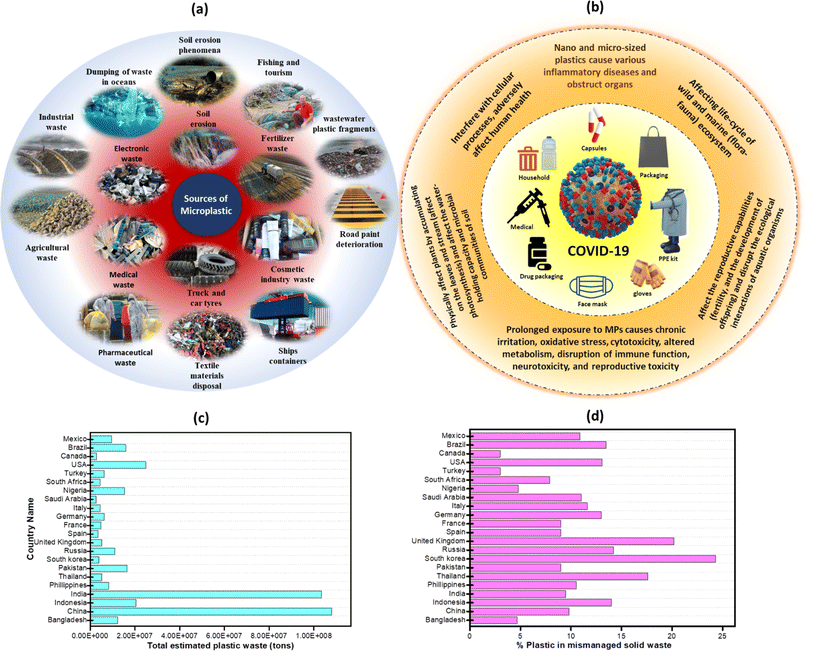 |
| Fig. 2 (a) Pictorial representation of various sources of MPs, (b) effect of the Covid-19 pandemic on MNP generation, (c) graphical representation of total estimated plastic waste (in tons)38,39 and (d) percentage of plastics in mismanaged solid waste.40 | |
3.1 MPs from packaging, cosmetics, and textile industries as fast-moving consumer goods (FMCG) sources
The cosmetics industry has posed a significant challenge concerning MPs pollution primarily attributed to the use of microbeads (composed of materials like polyethylene (PE) or polypropylene (PP)) in a wide range of personal care products such as scrubs, toothpaste, and shower gels. For example, a study conducted by Guerranti et al. revealed that cosmetics usage in the countries from the European Union is found to be ∼4000 tons of microbeads annually.41 Furthermore, research by Cheung and Fok in 2017 highlighted that the aquatic environment in mainland China is facing contamination with an estimated average number of microbeads of ∼210 trillion, which corresponds to ∼307 tons in weight.42 Additionally, synthetic textiles including polyester and nylon are major contributors to MPs pollution. For instance, Hernandez et al. presented the amount of MPs fiber generation from synthetic textiles (polyester) in terms of their mass and size while carrying out the imitated home washings taking into account washing parameters such as the utilization of detergent, washing time, consecutive washing and temperature condition along with fiber structure orientation.43 The study demonstrated that, of all the factors assessed the use of detergent exhibited the greatest effect on the overall amount of fibres released. However, detergent composition (liquid or powder) and overdose failed to impact MPs release significantly. Despite various release parameters while carrying out the insertion of surfactant (∼0.025 and ∼0.1 mg fibers per g textile washed out deprived of and with detergent, respectively), average MPs-based fabric orientation was found consistent regardless of wash conditions, with maximum of fibres found between ∼100 μm and ∼800 μm in length, regardless of the number of washing cycles.43
MPs can be generated from packaging films during everyday activities such as opening packages, consuming food, drinking from plastic bottles, or disposing insensitively into sewerage or garbage heaps. For instance, Sobhani et al. demonstrated that plastic packages from consumables in everyday life may result in a significant mass of MPs generation (i.e., within the range of ∼10 to ∼30 nanograms, which is equivalent to ∼14
000 to ∼75
000 isolated number of MPs).44 The quantity of MPs generated (ranging from ∼46 to ∼250 MPs per cubic centimeter (size of 10 μm × 10 μm × 10 μm), and/or ∼0.46 to ∼2.5 MPs per cubic centimeter (size of 1 mm × 10 μm × 10 μm)) depends on various factors, including the stiffness, thickness, anisotropy, density of the plastic materials, and the size of the MPs.44
3.2 MPs from agriculture, construction, and infrastructure demolition waste
MNP can also be deliberately introduced into the soil through the use of industrially produced chemical fertilizers such as diammonium phosphate and single superphosphate (which predominantly possess nitrogen, phosphorus, calcium, magnesium, and potassium elements) and pesticides such as herbicides, fungicides, nematicides, rodenticides, and insecticides for agriculture.45 These products often come with a polymer shell or coating, contributing to MNP contamination.46 For instance, China, being one of the largest producers and consumers of plastic globally, utilizes over ∼70 million tons of plastic products annually.47 Among these, mulch films account for ∼57.27% of agricultural film consumption, which has reached over 2.408 million tonnes.48 Research conducted in China has revealed significant stages of MPs contamination, with soil samples in localities where plastic mulching has been employed for consecutive ∼30 years showing MPs concentrations of ∼40.35 mg kg−1.49 A recent study in Japan found that plastic-based microcapsules from coated fertilizers collected in rice fields resulted in ∼144 mg of MPs per kg of soil.50 Additionally, the utilization of sewage sludge from WWTPs has been identified as a major source of MPs in soil used for agriculture.51 For instance, average MPs concentrations of ∼22.7 ± 12.1 × 103 particles per kg dry sludge were detected in sewage sludge from 28 WWTPs in China,52 which indicates a relatively high abundance of MPs in the sludge samples. In Europe, sludge reuse releases ∼63
000 to ∼430
000 tonnes of MNP into farmland each year, out of which only Finland uses up to 72% of sludge for agricultural use.53,54 Furthermore, the wear and tear of agricultural equipment (such as agrochemicals loaded plastic films coated crop seeds) including tire abrasion (from tractors and mechanical harvests), contribute to the presence of MPs in soil samples from agro-fields, with the accumulation of tire wear debris being a significant source of soil MNP.55 Afterward, Kole et al. estimated that ∼67% of tire constituent parts unconstrained in the surroundings in the Netherlands end up in the soil, emphasizing the importance of tire wear as a substantial contributor to soil MNP. Moreover, MNP can enter into the soil and aquatic ecosystem through road paint deterioration, truck and car tires, ship containers, and by disposal of textile materials.56 Further, massive numbers of MNP as fibers (synthetic and natural based), such as rayon, polyester, and carpets are released from the common textile garments industry during the domestic and industrial laundering processes.
3.3 MPs from aircraft, aviation, and other transport modes such as vehicular sources
The aviation sector has emerged as one of the newly realized and aggressive potential sources for the generation of MNP. The major envisaged constituents comprised of tire wear, cabin materials, and engine emissions. As documented, a single take-off and landing of an aircraft (not only commercial flights but also private jets and military aircraft) generates an average of up to ∼2.5 kg of MNP getting into the atmosphere dispersed and the majority of it primarily consists of rubber particles from tire abrasion.56 In the context of automotive sources, MPs most significantly arise from tire wear and road abrasion. Tires shed tiny plastic particles as they wear down due to friction with the road surface. As an example, Baensch-Baltruschat et al. used the specific emission factors per vehicle-kilometer approach to assess wear and tear on urban, rural, and highway roads. The cumulative estimated wear and tear for these three types of roads amounted to ∼17
300 tonnes annually.57 Worek et al. tested samples of road dust and soil from high-traffic areas, finding an average of ∼372 ± 50 MPs fragments/kg of dry weight in soil samples and ∼515 ± 20 fragments per kilogram of dry matter in road dust.58 Notably, the road dust samples included a larger concentration of MPs than the soil samples, possibly due to the direct action of braking, which causes a greater buildup of MPs at the emission source than in the surrounding soil. Furthermore, there is a clear variation in the size distribution of MPs fractions, as most instances in soil samples lie below ∼50 μm, whereas road dust samples largely consist of fractions between ∼50 and ∼200 μm.58 It must be noted that the extent of MPs-induced contamination in soil that can directly be attributed to tire dust or tire- or origin remains mostly unreported or scattered to the extent that it is hard to reproduce.
3.4 MPs from the pandemic, epidemic, healthcare and medical-safety products
The COVID-19 pandemic has led to a significant increase in single-use plastic pollution due to the extensive use of single-use-plastic-based biowaste and medical waste such as gloves, face masks, and PPE kits, hand sanitizer bottles, gloves, and water-logged masks. However, the persistence of plastics for prolonged periods, and improper disposal, including these single-use medical devices and PPE items, can contribute to their prevalence in the environment for many thousand years, thereby leading to detrimental effects on wildlife, including entanglement, ingestion, and habitat disruption.59,60 Fig. 2b provides an overview of the impact of plastic waste generation at the time of the pandemic. Reportedly, global demand for PPE has risen to ∼129 billion face masks and 65 billion gloves every month, ensuring the protection of the public and frontline workers globally.61 The total quantity of plastic garbage generated increased as a result of individual choices about utilizing online delivery services in order to address safety concerns about dining out or shopping during the pandemic or any other extended period of illness. As an example, the waste produced due to online shopping during the pandemic varied across countries, with the highest amounts recorded in China (∼402
000 tons), followed by Germany (∼36
500 tons), the USA (∼2700 tons), India (∼520 tons), and Italy (∼450 tons).62 Conversely, hospital waste generation, ranked from highest to lowest, was observed in India (∼100
865
000 tons), the USA (∼685
200 tons), Germany (∼500
300 tons), Italy (∼404
000 tons), and China (∼146
000 tons).38,39 A study by Peng and Kathuria estimated that ∼22–30 thousand tonnes of plastics related to the epidemic, including ∼12
000 tonnes of MPs, were released into worldwide seas. This highlights the significant contribution of the COVID-19 pandemic to the accumulation of MPs in marine and inland waters.40 Further, Saliu et al. revealed that in 2020, the worldwide manufacturing of masks was found to be range from ∼2.4 to ∼52 billion pieces, resulting in the generation of ∼7200 to ∼312
000 tons of waste containing MPs. Among these wastes, a significant portion, i.e., ∼1% to ∼10% was released into the ocean, which accounts for ∼72 to ∼31
200 tonnes of MNP waste.63 Further, Mintenig et al. demonstrated that facial cleanser beads exhibit a higher mass of MPs compared to other personal care products (PCPs). For instance, they found that facial cleanser beads contained ∼0.05 grams of MNP per gram or ∼2450 particles per gram, whereas shower gels exhibited only ∼0.02 grams per gram or ∼2.15 particles per gram.64 Plastic particles in shower gel exhibited an average diameter of ∼419 μm, whereas those in face cleanser beads showed an average diameter of ∼197 μm. A previous study conducted in China on characterizing MPs in facial cleanser beads indicated that WWTP was responsible for >80% of MPs entering the aquatic environment. The residual portion (∼20%) may be ascribed to direct emissions, of which ∼18.2% come from cities and ∼0.5% from rural regions.42,65 The detailed outline of the total estimated plastic waste in various countries is shown in Fig. 2c and d.
4. Gross overview of physical consequences of MPs on the “soil–water–atmosphere” nexus
4.1 Physical consequences of MPs in water
The occurrence of MNP in earthly and marine environments raises concerns due to their potential impacts on organisms, ecosystems, and human health assisted by various mechanistic routes such as ingestion, contamination, infection, inflammation, assimilation, blockage of various tracts in active biological systems such as plants and animals (both aquatic and terrestrial).66 For example, MPs can be consumed by a variety of organisms, ranging from small invertebrates to larger marine animals, and have the ability to accumulate and magnify in the food chain. Such undesirable effects may cause nutritional imbalances, and changes in feeding behavior, leading to related growth facts, reproduction malfunction, and eventually threatening the overall growth of such species.67 Further, nanoparticles (NPs) can be ingested by a diverse range of aquatic organisms, including invertebrates, fish, and plankton through various pathways, such as filtration feeding or direct absorption across cellular membranes. Once ingested, NPs can accumulate in different body parts and tissues, including the gills, liver, intestines, and blood.68 While keeping this fact in mind, Goh et al. explored detailed profiling of nanoparticles for MPs remediation from aquatic environments which may have long-persisting issues like depth-specific susceptibility of marine creatures/microorganisms.27 Additionally, it has been observed that copepods, when exposed to high concentrations of MPs, exhibited reduced algal feeding, as highlighted in a study conducted by Piyal Bhattacharya.69 Furthermore, research by Moos et al. demonstrated that mussels ingesting MPs experienced a pronounced inflammatory response.70 Further, the regions with elevated MPs concentrations such as certain coastal areas and bodies of water often experience diminished water clarity due to the scattering of light by these particles, resulting in a cloud/hazy or murky appearance.71 Consequently, this can disrupt the process of photosynthesis in underwater plants due to a significant drop in photon flux density with increased concentration of MPs and alter the visual hunting tactics of marine underwater species employed by certain species.
The higher exposure of humans to freshwater environments increases the significance of MNP as a threat in these ecosystems compared to marine environments. For example, in the urban waters of Changsha, Hunan Province, China, surface sediments were found to contain MNP concentrations ranging from ∼270 to 866 items per kg.72 In Qinghai Lake, the largest inland lake in China, MNP concentrations in the lake water ranged from ∼0.05 × 105 to 7.58 × 105 particles per km2, while sediment samples contained ∼50 to ∼1292 particles per m2.73 Similarly, in the Wei River in northwestern China, MPs concentrations in surface waters ranged from ∼4 to ∼11 items per L, while sediment samples had concentrations of ∼360 to ∼1320 items per kg.74 In humans, the MPs incorporation takes place via gastrointestinal tracts followed by their permeation into both the lymph and circulatory systems of unborn fetuses through the placentas, as well as being absorbed in the lungs when inhaled, which can trigger various biological responses from the immune system and have detrimental effects on the health of body cells. Reportedly, MPs smaller than <150 mm may translocate across the gut epithelium.75
4.2 Consequences of MPs across soil–water interface
Contamination of soil by MNP can occur through diverse pathways, such as the incorporation of MPs in sewage sludge or compost direct interference, the fragmentation of larger plastic objects into smaller particles (fracture and collapse of structural integrity), or direct deposition from the atmosphere (settlement of contaminated MNP aerosols).76 They have several effects on soil environments as they can enhance water evaporation rates by influencing soil porosity, potentially leading to increased water loss, increasing amount of dissolved nitrogen, phosphorus, and other organic carbonous compounds in soil through enzymatic stimulation activity, altering the structure and diversity of the bacterial community, and eventually leading to significant extent of soil–moisture interaction imbalance over time.77 Additionally, MNP in the soil can adsorb various pollutants like antibiotics, heavy metals, and persistent organic substances (also known as “forever chemicals”) and release internal and non-bonded additives through dynamic leaching activity, which in turn may cause pollution and alter the movement of pollutants in the soil, thereby endangering the soil quality and its associated microorganisms.78 Further, the presence of MNP in the soil environment can alter the overall bulk density (making it lighter) and pH of the soil. For instance, Tan et al. noticed that the pH decreased with increasing levels of MPs in a pot experiment, which may account for the increase in soil aggregate size, soil porosity, and aeration when the MPs were put into the soil.79
Similar to the marine environment, MNP pollution in soil has reportedly increased the uptake of MNP by various soil organisms such as earthworms, and rhizobium. For example, PS particles can induce a malfunctioning of intestinal microflora, impair intestinal boundary function, and thereby causing metabolic disorders in mice as a secondary consequence.80 Earthworms, which are important contributors to soil health, also experience inhibited growth when exposed to PE-based MPs, and higher concentrations of MPs that can even lead to increased mortality rates in these organisms. Similarly, nematodes, another important group of soil organisms, efficiently ingest MNP, which can be detected in their offspring as well. MNP is extremely dangerous to plant species, causing growth suppression, intestinal damage, immune system disturbance, reproductive consequences, and death. MNP can also inhibit the ability of roots to absorb water and nutrients, affecting plant development and germination.81 Similarly, MPs in soil may induce a new microbial habitat with its consequences on bulk soil microbiomes.82 MPs tend to accumulate in pores indeed capsules and also impact seed water adsorption and may thus delay germination and root growth. Thus, MPs in soil may disturb the soil nutrient cycle alter the microbial structure, and may even ultimately affect crop growth.83 Through the complex food chain and species interactions, MNP can accumulate and transfer through trophic levels, ultimately posing a threat to human health via bioaccumulation.84
4.3 Consequences of MPs across atmosphere–water interface
MNP possess the potential to remain airborne due to their corresponding tiny size and lower density values, which can function as carriers for different other contaminants present in the air, and their transportation with more time is helped by wind.85 While rivers and coastal areas are recognized as major sources of marine MNP, present research indicates that atmospheric deposition may also contribute to the presence of MNP in the ocean and their concentrations in the atmosphere can vary across different regions depending on climate conditions. For example, MNP concentrations ranged from ∼4.18 items per m3 in Shanghai, China, to ∼14.1 items per m3 in Beijing, and up to ∼275 items per m3 in Germany.86–88 Indoor air tests from 12 countries globally revealed a range of PET MPs concentrations from ∼38 to ∼120
000 μg g−1, with the average value in Colombia.89 MPs can indirectly influence climate by modifying the reflectivity (albedo) of the surfaces they settle on, thereby not only impacting local temperature and climate patterns but also acting as radiative surfaces. Further, Zhang et al. addressed the pressing issue of anthropogenic pollutants, specifically focusing on the MPs in cryosphere regions where they conducted experiments in snow, river, glaciers, lake water, and sea ice with a primary focus on the physical and chemical characteristics of MPs and their transportation dynamics in these fragile ecosystems.90 However, there has been relatively minimal attention directed toward the significant consequences of cryosphere changes so far. Revell et al. observed that atmospheric MPs had an effective radiative forcing of ∼0.044 ± 0.399 μW m−2 in the ecosystem, presuming a uniform surface concentration of one MPs particle per cubic meter and a vertical distribution up to ∼10 km height.91 Despite the significant uncertainty involved with these findings, the research provides an innovative perspective on the potential impact of MPs on the climate and environment, especially in the context of global warming and increasing plastic production and consumption. It further highlighted that deposited MPs on glacier surfaces are transported over long distances through wet and dry deposition, which can have significant effects, particularly when they possess dark colors like black, grey, or brown.92 These MPs can absorb solar radiation, leading to the heating of surface snow, a phenomenon akin to the impact of carbon black in snow. This suggests that MPs in snow may lower glacier surface albedo and, consequently disrupt the energy balance in cryosphere regions. Furthermore, the accelerated melting of glaciers could introduce MPs into downstream rivers and lakes, creating threats to ecosystems.64 Unfortunately, existing studies have failed to quantify the impacts of airborne MPs deposition on surface snow.93
Human exposure to MNP through inhalation poses a significant risk to human health. Inhalation is recognized as one of the primary routes of exposure to MNP, and it is estimated that the intake of MNP through the air exceeds other exposure routes.94 When inhaled, MNP can deposit in the lungs, and natural mechanisms such as cilia movement, sneezing, and phagocytosis aid in the removal of inhaled particles. Consequently, MNP has the potential to accumulate in the body over time, leading to chronic inflammation, which may increase the risk of cancer.95 Fig. 3a highlights the numerous phases of the plastic life cycle, including manufacture, use, and final disintegration, with an emphasis on the formation of MPs.
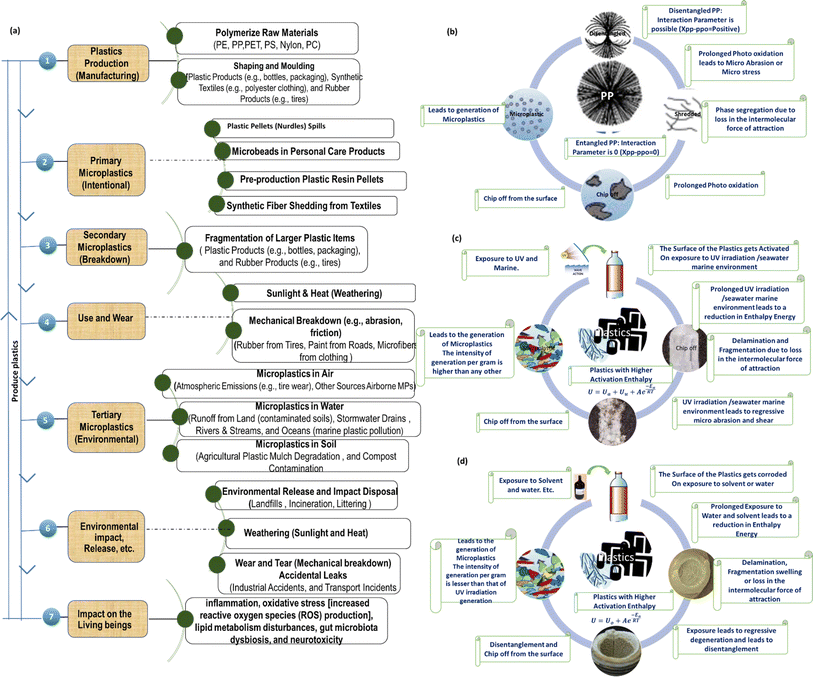 |
| Fig. 3 (a) Schematic representation showing a comprehensive overview of the stages/life cycle of plastic products and its disorientation, followed by disintegration into different stages of MPs through various environmental, mechanical, and human factors along with their adverse impact on human life. (b) Effect of photo-oxidation on the structure of the polymer for MNP generation, (c) effect of UV-irradiation and marine environment on the structure of the polymer for MNP generation, and (d) effect of solvent or water on the structure of the polymer for MNP generation. | |
Understanding the various mechanisms contributing to MPs generation is crucial for comprehending their pervasive presence in various environments. Thus, the paper further gives insights into various factors influencing MNP generation, namely the influence of photo-oxidation, UV-irradiation, and different solvent systems on polymer structures.96 The entire lifespan of plastic items starting from critical phases of production to use to final environmental degradation exhaustion of utility shelf life is shown in Fig. 3, which highlights the critical stages at which structural changes take place and, as a result of various environmental stresses (including photooxidation, exposure to ultraviolet light, and contact with solvents and aquatic environments), macroscopic plastic entities break down fragment with their MPs counterparts. These stressors include both human activity and natural factors, which create an environment that is favourable for the disintegration of plastics into components of MPs. When exposed to sunlight, high-energy UV photons are absorbed by polymer molecules and it generates free radicals (which are reactive entities with unpaired electrons), which further combine with ambient oxygen, thereby triggering oxidative chain reaction.97 Oxygen radicals attack the polymer backbone, causing molecular bond breaking and the creation of smaller fragments like MNP. In marine environments, the combination of UV exposure and ocean dynamics, such as temperature variations and wave activity, accelerates the breakdown of plastics. Temperature variations cause polymers to expand and compress, increasing their susceptibility to mechanical stress.98 Furthermore, wave motion exposes plastic waste to ongoing physical abrasion from other materials and surfaces, hastening the fragmentation process.99 Solvents, which are commonly employed in industrial processes and products, can weaken the polymer matrix, allowing larger plastic objects to break down into micro-sized particles. For example, Ghatge et al. evaluated the influence of organic solvents on the breakdown of PE, a widely used plastic polymer.100 The results showed that exposure to solvents enhanced PE fragmentation, resulting in micro- and NPs. Water has an important role in plastic transport and transformation, altering structural integrity and contributing to MPs dispersion across ecosystems. Arhant et al. investigated the hydrolytic breakdown of PET and discovered that water contact induced considerable chemical changes and structural damage to the polymer.101
5. Conventional methods for the removal of MNP from contaminated water
As the existence of MNP in the ecosystem causes great concern in the current circumstances, numerous traditional strategies for eliminating these new plastic pollutants have previously been thoroughly researched. As previously documented, the bulk of MPs are >20 μm and easily removed using filtering and Membrane Bioreactor (MBR).102 These removal methods are classified based on the nature of the removal process, such as (i) capture and surface attachment methods: coagulation, flocculation, and sedimentation (CFS), electrocoagulation, adsorption, magnetization, and microorganism aggregation, (ii) filtration and separation methods, which include filtration and separation via membrane and constructed wetlands, and (iii) degradation methods, which include photocatalytic degradation, microorganism degradation, and thermal degradation.103,104
Indeed, filtration and separation methods are commonly employed for the removal of MNP from the aqueous environment due to their practicality and effectiveness.105 However, it is important to note that the efficiency of these methods can vary depending on the size and nature of the particles. When it comes to small-sized and fibrous MNP especially those that are in the nanometer range, the efficiency of conventional filtration techniques may be relatively low. This may be attributed to the fact that smaller particles have a higher tendency to pass through filter media or pores, resulting in reduced removal efficiency.106 The small size and mobility of these particles make them more challenging to capture and separate effectively. To address this limitation, alternative techniques, and advanced filtration systems are being explored. For example, hydrogels, MOFs, and membranes have gained widespread acceptance in usage for the removal of MPs from various components of the environment.77,107 Despite their numerous advantages, these materials come with certain limitations, such as selectivity issues, fouling problems, challenges in scaling up, and complex treatment processes, which restrict their potential application for MPs removal.102 To impress upon the scenario, various systems (hydrogel, membrane, and MOF), their respective synthesis methods, the solvents utilized, and their flocculation activity concerning MPs are listed in Table 2.
Table 2 An overview of the compilation of different systems (hydrogel, membrane, and MOF) along with their respective synthesis method, solvent media, and flocculation activity towards MPs
Hydrogel |
System |
Synthesis method |
Solvent used |
Flocculation activity |
Ref. |
Bacterial cellulose hydrogel |
Biofilm formation method |
Distilled water |
∼88.6%: ∼1.77 g L−1 removal of MPs |
108 |
Polyethyleneimine and polydopamine copolymer, graphene oxide nanosheet, and poly(N-isopropyl acrylamide) hydrogel |
Self-polymerization reaction |
Deionized water |
∼97.09% efficacy for ferric ion adsorbed MPs compound polymer |
109 |
Ferrofluid/aminated alginate/psyllium hydrogel |
Chemical method |
Acetone |
∼94% removal of PMMA (coagulation adsorption) |
110 |
Sericin–CS-based hydrogel |
Crosslinking and chemical method |
Organic solvents |
Removal of PET by degradation method |
111 |
Membrane system |
System |
Synthesis method |
Removal efficiency |
Mechanism |
Ref. |
Membrane bioreactors |
Membrane unit and ultrafiltration process |
13 different polymers found in MPs (mainly polyester and PE) ∼99.9% removal efficiency |
Entrapment of MPs fiber due to their size and morphology |
112 |
Pilot scale membrane bioreactors |
Membrane formation method |
Removal of ∼99.4% MPs in activated sludge |
Opting for different processing steps |
113 |
Membrane microfiltration (polyvinylidene fluoride used as a substrate) |
Membrane formation method |
— |
∼100% removal efficiency |
114 |
Polycarbonate, cellulose acetate, and polytetrafluoroethylene membrane |
Filtration method |
Distilled water |
Removal of polyamide and PS MPs (∼94%) |
21 |
Silica-based ceramic hollow fiber microporous membrane by using Guinea cornhusk ash |
Membrane formation method and phase inversion |
Absolute ethanol and ammonia |
Polyacrylonitrile (PAN), PVC, polyvinylpyrrolidone (PVP), and polymethyl methacrylate (PMMA) MPs (∼99%) |
115 |
Holey Ti3C2 nanosheet-based membrane |
Membrane method |
Deionized water and hydrochloric acid |
∼99.3% removal (water flux 196.7 L h−1 m−2 kPa−1) |
116 |
Wastewater treatment plant samples were utilized |
Microfiltration membrane technique |
— |
∼98% MPs recovery in the form of fibers, pellets |
117 |
![[thin space (1/6-em)]](https://www.rsc.org/images/entities/char_2009.gif) |
MOF |
Zr-based MOF (UiO-66-OH@MF-3) |
Chemical method |
Acetone, ethanol, and dimethylformamide (DMF) |
∼95.5% removal efficiency |
77 |
ZIF-67 MOF |
Chemical method |
Deionized water, concentrated HCl, and NaOH |
∼92.1% adsorption ratio (PS MPs) |
118 |
Zinc-based MOF composite (ZIF-8@aergel by in situ growing ZIF-8 on wood aerogel fibers) |
Chemical method |
Deionized water and nitric acid solution |
Micro/nano plastic (poly(1,1-difluoroethylene and PS)) = ∼91.4 and ∼85.8% removal efficiency |
28 |
MOF (MIL-100) and Fe nanoparticles were incorporated into polysulfone matrix (PSF)-PSF/MIL-100 (Fe) nanoparticle composite membrane |
Chemical method and membrane formation method |
Deionized water |
∼99% rejection efficiency for methylene blue dye adsorbed in MPs |
119 |
In situ formation of Ag2O/Fe–MOF |
Chemical precipitation method |
Deionized water |
Conversion of MPs into useful chemical (acetic acid) and hydrogen production |
120 |
Cr-based MOF (cr–MOF/MIL-101) |
Hydrothermal method |
HF acid + water (pseudo-second-order kinetic) |
∼96% removal efficiency of PS nano-plastic (∼800 mg g−1) follows |
121 |
Nano-pillared structure composed of 2D MOF separated by carbon-encapsulated FeO nanoparticle |
Hydrothermal method |
Distilled water |
∼100% removal of MPs |
122 |
Zirconium MOF |
Hydrothermal method |
DMF used as a solvent |
∼95.5% removal efficiency of MPs |
77 |
Composite membrane made up of PAN with the addition of reduced graphene oxide |
Chemical and membrane formation method |
DMF, H2SO4 and H2O2 |
>82% removal efficiency of MPs |
123 |
6. Emerging techniques for the removal of MNP: new-directions and alternative material frameworks as adsorbents
The most emerging technologies cover the functional usage of biochar, electrospun fibrous constructs, and aerogels as they demonstrate significant potential in tackling the challenges posed by MNP pollution. These newer framework approaches offer distinct characteristics (specific to the chemical structure of MPs, specific to functional groups, specific to absorbability, specific to surface capability) and novel strategies (trapping, sequestration, coagulation, sedimentation, filtration, degradation) for effective MNP removal from water and wastewater.29,124 Biochar, renowned for its extensive surface area (∼250–500 m2 g−1) and exceptional adsorption capacity, exhibits promising ability in sequestering MNP.30 Electrospun fibrous constructs, on the other hand, feature high porosity (controlled pore size, allows size-specific MNP trapping) and customizable surface functionalities, enabling efficient capture of MNP.31 Aerogels, characterized by their remarkably low density (∼0.01–0.4 g cm−3) and large surface area (∼30–600 m2 g−1), present opportunities for advanced MNP adsorption and removal.125 Therefore, by harnessing the potential of biochar, electrospun fibrous constructs, and aerogels, the challenges associated with MNP pollution including mitigating their detrimental impacts on human health and the environment may be effectively addressed.
6.1 Biochar-based adsorbents
Biochar, a pyrogenic carbonaceous substance with a high surface-to-volume ratio, and the ability to functionalize its surface, porosity, and chemical composition, has sparked widespread attention as a bio-based sustainable adsorbent for the removal of MNP. The conventionally adopted procedure to make biochar is pyrolysis, which involves heating the feedstock (e.g., biomass waste, plant waste, animal waste) under minimally aerated (low oxygen) conditions.126 The utilization of precursor materials, temperature, pressure conditions, and pyrolysis technology, have a significant impact on the various properties of biochar, including adsorption capabilities and the elemental composition of the adsorbent of the resulting biochar.127 In this context, Chen et al. demonstrated that increasing the pyrolytic temperatures showed a direct impact on the degree of carbonization, which in turn led to improvements in the structural, compositional, and morphological aspects of the resulting biochar, accompanied by improved sorption capabilities.128 Furthermore, the abundance of feedstocks for the production of biochar, such as biomass of agricultural waste and solid waste, makes it a renewable resource with immense potential to remove MNP.129 For instance, Singh et al. developed eco-friendly iron-nanoparticles surface-modified biochar adsorbents with improved magnetic and surface properties to remove NPs under various pH conditions. The results showed that iron-modified biochar achieved ∼100% removal efficiency compared to ∼75% removal efficiency with untreated biochar.130 Further, Siipola et al. utilized pine and fir bark for the development of activated biochar by slow pyrolysis at ∼475 °C and steam activation at 800 °C. Such modification tends to alter the shape of the biochar and improve its adsorption capabilities and was found to retain ∼100% of cylindrical pieces of PE, including spherical microbeads (∼10 μm), cylindrical pieces of PE (∼2–3 mm), and fleece woven fibers.131
Employing magnetic adsorbents made from zinc/magnesium-modified biochar (Zn/Mg-MBC) has been explored for the removal/separation of Wang et al.132 The study demonstrated that even after undergoing five consecutive cycles of adsorption, the elimination efficiency using Mg-MBC, Zn-MBC, and MBC remained consistently high at ∼94.6%, ∼95.8%, and ∼95.0%, respectively. The primary mechanisms responsible for pollutant retention are chemical binding and electrostatic interaction between biochar and MP. Table 3 summarizes various reported methods of adsorptive removal of MPs using biochar. For example, Shi et al. developed biochar by applying the pyrolysis method of rape straw for MPs separation applications.136 Further, the magnetic biochar (MB) was prepared by using ferric chloride salt as an agent through the ultrasonication method. The performance of magnetic biochar was further improved by modifying biochar with CTAB surfactant (CMB) (Fig. 4a). The developed magnetic biochar system was utilized to isolate and segregate NPs from the environment surrounding in the form of PS (PS) and carboxylate-modified PS (CPS) nanoparticles (Fig. 4b). The maximum removal efficacy of ∼90% was attained by using the modified magnetic biochar system with quantitative values of ∼163.4 and ∼159.6 mg g−1 for PS and CPS respectively through an aggregation-based mechanistic approach. The prepared system was found recyclable in nature for up to five cycles suggesting their excellent selectivity and sensitivity (Fig. 4c). The system was highly effective at neutral pH and highly selective in the presence of a wide variety of metal ions (Fig. 4d and e). Further, Shi et al. utilized pinewood sawdust as a precursor for the fabrication of biochar and modified the biochar to develop a magnetic biochar system.137 The magnetically active biochar system was utilized to remove different types of PS such as unmodified, amine-modified, aged under UV radiation, and carboxylate-modified systems (as dummy MPs representation) with a maximum removal efficiency of ∼95%. The Langmuir adsorption isotherm for the system elicited an adsorption capacity of ∼107.71 mg g−1 and it followed the H-bonding and electrostatic interaction-based mechanisms.
Table 3 Representation of biochar system in MPs removal along with their solvent, mechanism, and removal efficiency
Biochar |
System |
Experimental medium of MPs dispersion |
Mechanism |
Removal efficiency |
Ref. |
Straw magnetic biochar with porosity and graphitization |
Ultrapure water |
Magnetic force |
Isolation of ∼31.29 mg g−1 MPs |
126 |
Corn straw and hardwood biochar (pyrolysis method) |
— |
Stuck, trapped, and entangled mechanism |
∼10 μM MPs spheres (∼90% adsorptive removal of MPs) |
30 |
Waste rice husk-derived magnetic biochar (impregnation pyrolysis) |
Deionized water |
Electrostatic interaction and complexation |
∼99.96% removal of MPs |
133 |
Biochar of bark of Scots pine and spruce (slow pyrolysis) |
Deionized water |
Adsorption phenomena |
∼90% removal of MPs |
131 |
Woodchip-derived biochar (pyrolysis) |
Ultrapure water |
Adsorption mechanism |
∼90% removal of MPs from sand columns |
134 |
Magnetic corncob biochar (hydrothermal) |
Ultrapure water |
Hydrophobic and electrostatic interaction |
∼90% removal of polyamide MPs |
127 |
Mg/Zn modified magnetic biochar (impregnation method) |
Deionized water and ethanol |
Adsorption and thermal degradation |
∼99.46% removal of PS MPs |
132 |
(Cow manure and sawdust) livestock manure biochar (biodegradation method) |
Deionized water |
Biological degradation through different species |
∼90% removal of polyhydroxyalkanoates MPs |
135 |
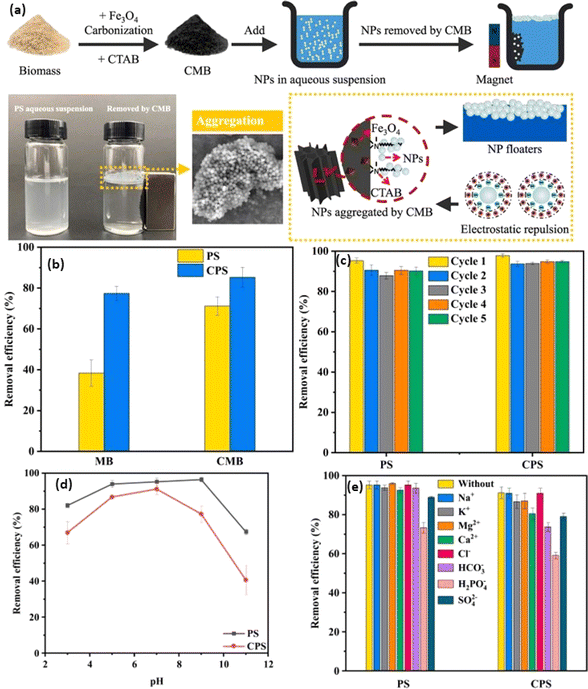 |
| Fig. 4 (a) Synthesis of magnetic biochar (MB) and CTAB modified biochar (CMB), (b) removal efficiency of PS (PS) and carboxylate modified PS (CPS), (c) reusability, (d) pH stability, and (e) highly selective in the presence of a wide variety of metal ions.136 | |
6.2 Electrospun fiber-based adsorbents
Electrospun mats/membranes have attracted substantial consideration in the field of MNP separation and segregation due to their extraordinary properties such as excellent surface area (∼8.93 m2 g−1) depending upon the porosity, wide range of diameters (ranging from ∼40 to ∼2000 nm) permeability, easy surface functionalization process, controllable surface charge along with exceptional pliability/flexibility as a macrostructural framework.138 Additionally, the highly interrelated and porous network structure of such electrospun mat-based systems results in a very low resistance toward water transport (permeability/diffusibility) which ultimately enhances the membrane flux to a favorable desired value (Table 4). Further, electrospun nanofibrous membranes (when suitably designed as mats) found domination as compared to conventionally reported membranes in terms of hydrophilicity, mechanical stability, selectivity, flexibility, resistance to water flow, and surface area.31,147 An interesting aspect of this method is the production of electrospun nanofiber mats of various sizes and shapes with porous properties, which allows them to eradicate smaller contaminant particles isolation via trapping, such as PM 2.5 (<2.5 μm), PM10 (<10 μm), and PM1.0 (<1 μm), over the last few decades owing to their size exclusion mechanism.148 The performance and adsorption sieving characteristics of mats could be improved through functionalization/modification mode (chemical grafting, layer by layer, self-assembly, co-axial spinning, side-by-side deposition, and nanomaterial implementation via electrospraying) and incorporation of polymer matrix and chemical agents.149 Few reports have dealt with the electrospun mats, concerning their potential for MNP removal. For instance, Wang et al. fabricated low-pressure-driven and highly porous electrospun PAN membrane by adopting a layer-by-layer assembly protocol (where three layers i.e. polyethyleneimine (PEI), poly(acrylic acid) (PAA) and PAN were deposited over one another139 (as shown in Fig. 5a). The electrospun mat was hydrolyzed to add carboxylic groups that were negatively charged, so as to initiate the easy formation of LbL assembly, as seen in Fig. 5b. The prepared system reflected outstanding mechanical strength (∼58.5 MPa ± 20.1) and hydrophilicity (water contact angle = 38.3 ± 2.3°) at a neutral pH (pH = 7) condition and subsequently acted as an efficient model for the removal of ∼89.9% PS (PS) based micro-/nanosphere with its size in the range of ∼50–500 nm. As seen in Fig. 5c, all of the modified membranes exhibited retention rates >99%. The presence of electrostatic attraction mainly contributes to the isolation/removal of MNP in an effective manner from the micro-plastic dispersed polluted water. Further, Risch and Adlhart developed CS–glutaraldehyde/PE electrospun nanofiber by applying a high-throughput nozzle free-surface electrospinning methodology (Fig. 5d). The CS-based nano-sponge is highly biocompatible in nature and possesses outstanding bulk density and porosity. The nano-sponge was found to be highly selective towards the adsorption of PET-based MPs from the micro-plastic-induced pollution in water and wastewater mediums.140 The turbidity test of MPs was performed on nanofiber which decreases with the time of measurement and concentration of MPs (Fig. 5e). The non-linear fit of data for the adsorbed amount indicated Lagergren's pseudo-first-order model (Fig. 5f). A maximum of ∼99.49% removal was observed for MPs which suggests their application in tackling environmental challenges (Fig. 5g).
Table 4 Representation of electrospun material systems in MPs removal along with different parameters (solvent, method, and pH)
Electrospun system |
Solvent |
Method |
pH |
Removal efficiency |
Ref. |
Highly porous electrospun PAN membrane |
Ultrapure water and organic solvent |
Layer-by-layer assembly protocol |
Physiological pH = 7.2 |
PS (PS) microspheres (89.9% removal through electrostatic attraction) |
139 |
Graphene oxide incorporated PU-based electrospun composite membrane |
Tetrahydrofuran (THF), dimethylformamide (DMF) |
Chemical and electrospun method |
Neutral pH = 7 |
Removal of micro and nano plastic of butadiene styrene (BS), PS, and poly(methyl methacrylate) (PMMA) (cake formation and blocking mechanism) |
124 |
CS–polyethylene oxide (PEO) nanofiber electrospun mats |
Methanol, ethanol, and acetic acid |
Cross-linking method |
pH = 8 |
91% removal of PET MP (hydrostatic filtration) |
140 |
Tubular nanofiber electrospun membrane of PAN polymer |
DMF |
Bottom-up electrospinning method |
— |
— |
141 |
PAN electrospun nanofiber membrane |
DMF |
Hot pressing method |
— |
Removal of 0.2 μM MPs through cake formation mechanism |
142 |
Polyvinyl alcohol (PVA) electrospun membrane followed by cross-linking with glutaraldehyde |
HCl, acetone, and Milli-Q water |
Conventional non-woven micro fibrous approach |
Wide pH range |
98% removal of polycarboxylate microsphere particles through particle retention scheme |
143 |
Electrospun polyimide (PI) nanofiber membranes |
Deionized water |
Dead-end filtration system |
— |
0.22 μM microfiltration membrane |
144 |
Polyvinylidene fluoride (PVDF) ultrafiltration membranes |
Millipore, Milli-Q water, and other systems |
Top-down approach by considering the electrospinning method |
— |
Removal of PET MPs |
145 |
Silica-based ceramic-supported thin membrane and Guinea corn husk were utilized as precursor |
N-Methyl 2-pyrilidone |
Phase inversion, interfacial polymerization, and sintering approach |
Neutral pH |
Removal of PVC, PMMA, PAN, and PVP |
115 |
Ultrafiltration membranes of PET nanofibers and organic contaminants |
Ethanol, trifluoroacetic acid, hydrochloric acid and acetonitrile |
Electrospinning method for nanofiber preparation and cryo-sectioning through freezing agent |
pH = 7 |
Removes 75% of nano/microfibers |
146 |
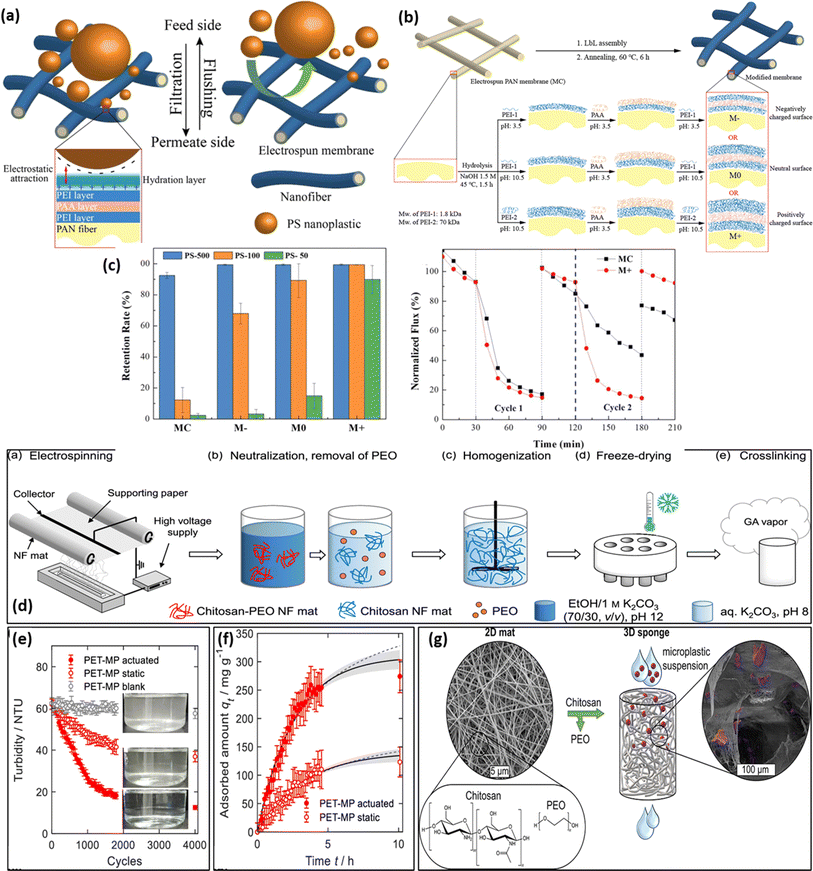 |
| Fig. 5 (a) Surface modification of electrospun mats using a Layer-by-Layer (LbL) approach. (b) Membrane surface modification process (c) retention rates of the prepared membranes for PS microspheres and two filtration cycles of MC and M+ to assess the removal efficiency of PS-500 MPs.139 (d) Schematic representation showing the synthesis of CS-based electrospun nanofiber, (e) turbidity reduction test of MPs, (f) adsorbed amount of MPs, and (g) highest concentration with surface area of MPs on nanaofibers.140 | |
A highly economical silica-based ceramic-supported thin hollow fibrous membrane via phase inversion, interfacial polymerization, and sintering approaches has been developed by Yogarathinam et al. (as shown in Fig. 6a). The prepared silica membrane was utilized for the eradication of various types of MPs from the aquatic environment such as PVC, polymethyl methacrylate (PMMA), PAN and PVP to a greater extent approximated to >90%.115 The order for the removal of MPs was removed as PVP (∼97.2%) > (∼93.7%) > PMMA (∼92.1%) > PVC (∼88.8%). In a recent study, Juraji et al. developed a polyurethane (PU)-based electrospun composite membrane by the incorporation of graphene oxide montmorillonite hybrid filters (Fig. 6d). The developed membrane exhibited super hydrophilicity (due to no/zero water contact angle and presence of various hydrophilic groups (carboxylic and hydroxyl)), maximum extent of pressure-driven as well as gravity-driven water flux, and outstanding capacity in the removal of PS (PS), acrylonitrile butadiene styrene (ABS), and PMMA type MNP.124 The mechanistic behaviour is characterized by individual and combined impacts of the size exclusion effect and adsorption phenomena (Fig. 6e). The maximum adsorption capacity was ∼417 mg g−1, following the pseudo-second-order kinetic model and Langmuir adsorption isotherm. Removal effectiveness was ∼95% for various MPs (PMMA, ABS, and PS) (Fig. 6f). The present electrospun composite was found satisfactory for up to ten cycles for the removal of MPs (Fig. 6g). The time-dependent gravity flux of different MPs was found to be reduced as compared to the water system due to the fouling of MPs (Fig. 6h). The fabricated PU-composite membrane acts as an efficient approach for the removal of MNP from polluted water.
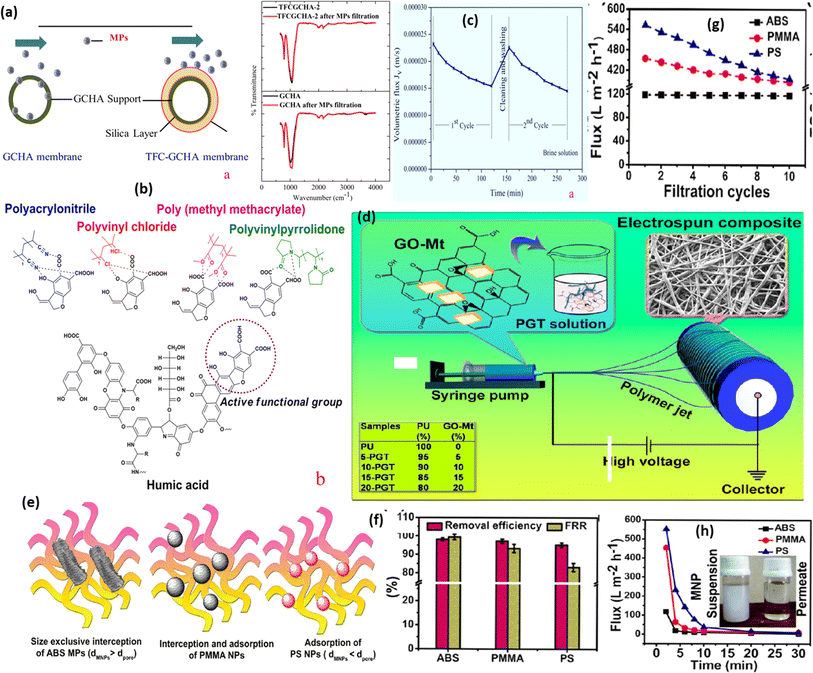 |
| Fig. 6 (a) Fouling analysis MPs fouling in (Guinea cornhusk ash) GCHA and TFC-GCHA (thin-film composite Guinea cornhusk ash) membranes with their FTIR spectra of before and after MPs analysis, (b) schematic of the interaction of MPs-HA, (c) brine solution filtration analysis, (d) schematic representation showing the synthesis of a PU-based electrospun composite, (e) mechanistic representation showing the adsorption of ABS, PMMA, and PS nano plastics, (f) removal efficacy of MPs, (g) reusability of the electrospun composite after ten cycles, and (h) time-dependent flux of different MNP.124 | |
6.3 Aerogels as adsorbents
Aerogel-based adsorbents have gained significant attention due to their remarkably high specific surface area (30 m2 g−1 to as high as 600 m2 g−1) and adjustable surface chemistry, which is approximate for the removal and segregation of MNP.106 Aerogels are essentially highly porous structures with a high percentage of air, that can be derived from different types of precursors, including organic, inorganic, or hybrid molecules.150 Aerogels are available in various forms such as powders, monoliths, and films, and exhibit different types of porosity, including mesoporous, microporous, or mixed porous structures. The porous structure of aerogels enables the physical entrapment of MPs particles, while the surface chemistry can be suitably tailored to enhance the adsorption affinity for a specific plastic.151,152 Additionally, the lightweight nature of aerogels facilitates their deployment and handling in water treatment systems. Furthermore, the regeneration capabilities of aerogels make them appealing for the treatment of MP-contaminated water. After adsorption, the aerogel-based adsorbents can undergo regeneration processes to recover the trapped plastics and restore their adsorption capacity.
Several studies have explored the potential of aerogel-based adsorbents for MNP removal, as listed in Table 5. For instance, Zheng et al. fabricated coral-inspired environmentally durable aerogels by utilizing polydopamine-enhanced magnetic chitosan (PDA-MCS) (Fig. 7a).106 The adsorption and removal/separation capacity of different CS, magnetic chitosan (MCS), and PDA-MCS-based systems were investigated (Fig. 7b and c). The advanced aerogel was found to be highly efficient in the adsorption of PET, PE, and PS type MPs from the contaminated water under different pH conditions (pH = 6–9) with ∼94.6%, ∼92.3%, and ∼97.3% removal efficiency, respectively (Fig. 7d and e). The developed aerogel-based system was found acceptable for up to four recycles in the removal of MPs (Fig. 7f). The mechanism behind the formation of aerogel-assisted MPs disposed of water involves electrostatic interactions and physical adhesion. Different reaction conditions such as no light, daylight, and UV light were explored to assess the adsorption of MPs through such aerogel systems (Fig. 7g). Further, Zhuang et al. selected cellulose nanofiber as a standard matrix material for the preparation of modified cellulose nanofibers-based aerogel.125 Here, 2,3-epoxypropyl trimethyl ammonium chloride acts as a modifier whereas polyvinyl alcohol acts as a crosslinker and liquid nitrogen freezing method was used during the preparation of these aerogels. The prepared aerogel system possesses a very high tendency to adsorb small-size MPs (∼2 to 5.5 μm) with an adsorption capacity of ∼146.38 mg g−1.125 The adsorption phenomena follow the pseudo-second-order kinetic model and Langmuir adsorption isotherm acts as an efficient model to be operational mechanistically in removing MPs from contaminated water. In another study, Meng et al. developed facile crosslinking of brittle oxide-based nanofibers into 3D-based aerogels on the basis of smart screening.157 The methodology exhibited high efficiency in evaporating water from MP-polluted seawater sources powered through solar energy which basically enables the vapor to condense naturally and harvest the same in an electricity-free system. A 100% removal efficiency was observed for the MPs from contaminated water by using the aerogel system, further, it can be reused in pressure sensing post-solvent evaporation. Very recently, Zhuang et al. developed a highly modified aerogel system where cellulose nanofiber acts as a matrix and 3-glycidoxypropyltrimethoxysilane/polyethyleneimine as a modifier.156 The fabricated aerogel system was successful in the removal/isolation/separation of MPs from water with an adsorption capacity of ∼117.04 mg g−1 at room temperature conditions. The adsorption phenomenon followed the pseudo-second-order kinetic model and the Langmuir isotherm, suggesting that MPs particles are adsorbed as a single molecular layer on an aerogel's non-homogeneous surface.
Table 5 Representation of various types of aerogel systems in MPs removal along with different parameters
Aerogel |
System |
Model system employed for efficacy assessment of the system |
Underlying mechanistic principle |
Removal efficiency |
Ref. |
Cellulose nanofiber aerogel modified by quaternary ammonium salt (chemical synthesis) |
Deionized water |
Adsorption mechanism/electrostatic action |
∼146.38 mg g−1 adsorption capacity of MPs by fluorescence characteristics |
125 |
Zinc MOF-based wood aerogel (in situ method) |
Deionized water |
Electrostatic interaction |
∼91.4 and 85.8% removal of polydifluoroethylene and PS MNP |
28 |
Coral bio-inspired aerogel (polydopamine and CS) (self-polymerization and freeze-drying) |
Ethanol |
Surface and intra-pore adsorption |
∼91.6% removal of PET MPs |
106 |
Egg protein-derived ultralightweight hybrid monolithic aerogel (pyrolysis) |
Water |
Chemical interconnection |
∼99.9% MNP contaminants |
153 |
Microporous carbon aerogels (fast polycondensation of dialdehyde and silsesquioxane) |
Methanol, ethanol, HF |
Electrocatalyst support/adsorption |
Rapid removal of ∼90% MPs in <10 s |
154 |
Cyclodextrin–graphene oxide aerogel microspheres (electrospraying, freeze casting, and self-assembly) |
Organic solvents |
π–π and adsorptive chemical interaction |
∼17 mg g−1 removal of organic micropollutants (2,4-dichlorophenol) |
155 |
Modified aerogel system where cellulose nanofiber acts as a matrix and 3-glycidoxypropyltrimethoxysilane/polyethyleneimine acts as a modifier |
Acetic acid and KOH |
Ultrasonic and freeze-drying methods |
∼117.04 mg g−1 adsorption capacity for MPs (pseudo-second-order kinetic model) |
156 |
Lanthanum-modified k-carrageenan/sodium alginate aerogel system |
Deionized water |
Sol–gel technique |
Removal of ciprofloxacin hydrochloride antibiotic along with existing MPs |
150 |
Facile crosslinking brittle oxide nanofibers into elastic 3D fibrous aerogels |
Organic solvents |
Smart screening |
100% removal efficiency |
157 |
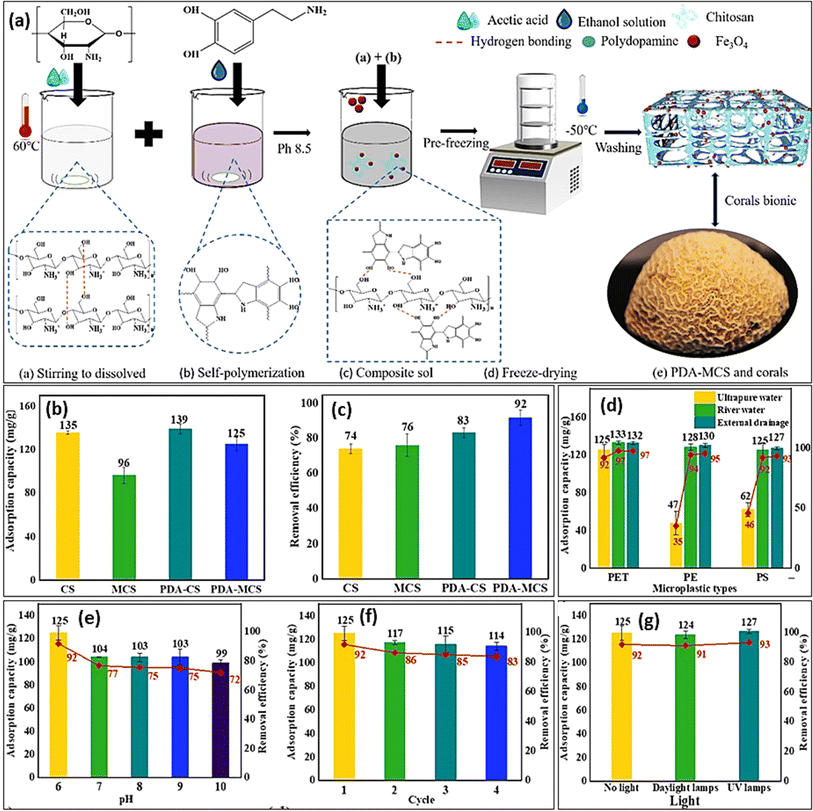 |
| Fig. 7 (a) Synthesis of polydopamine-enhanced magnetic chitosan (PDA-MCS) aerogel and corals, (b) adsorption and (c) removal efficiency of different synthesized systems, (d) adsorption capacity in different water media for various MPs, (e) adsorption capacity and removal efficiency of developed aerogel at different pH values, (f) after various cycles of reuse, and (g) in the presence of different light conditions.106 | |
However, research on the application of aerogel-based adsorbents for MPs and NPs removal/isolation/separation is still in its early stages, and further investigations are needed to optimize the adsorption performance via a complete understanding of the underlying mechanisms. The properties of the aerogels, including their surface chemistry, pore structure, and composition, should be tailored specifically to target the unique selective characteristics of MNP. A comparative analysis of the conventional as well as our proposed emerging frameworks in terms of their efficacy, intrinsic properties, and operational efficiencies is shown in Fig. 8a and b. Moreover, it addresses the increased effectiveness and specificity demonstrated by emerging adsorption methods in comparison to traditional ones, supporting their incorporation into contemporary water treatment systems. The comparative analysis presented here provides insightful information about how pollutant removal technologies are developing and steers the field of water purification efforts in the direction of greater sustainability and environmental stewardship.
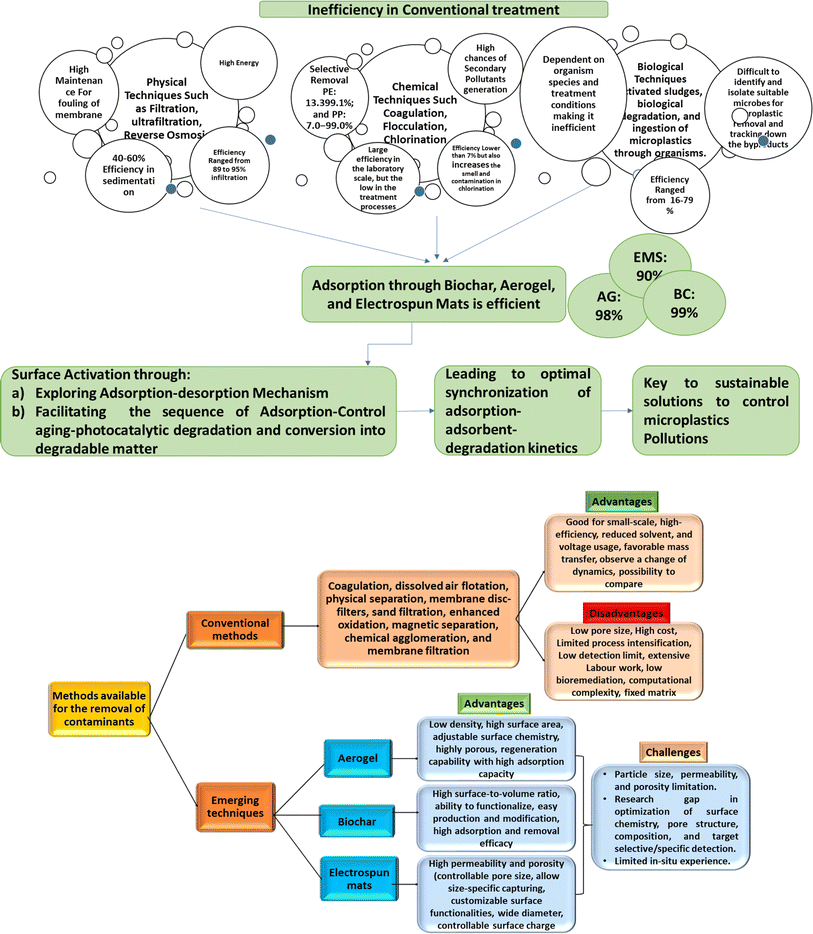 |
| Fig. 8 Schematic representation highlighting the inefficiency of conventional treatment and efficiency (in terms of effectiveness, properties, and removal efficacy) of biochar, aerogel, and electrospun mats for the removal of MPs. | |
Thus, the present review offers an in-depth investigation of the theoretical and methodological issues concerning the removal of MNP from diverse environmental matrices using aerogels, electrospun frameworks, and biochar-based constructs. Further theoretical exploration and empirical investigations are needed to validate performance under varying environmental conditions, assess long-term effectiveness, and explore synergistic effects when combining these materials. To address the underlying causes of plastic pollution, policy interventions may also focus on consumer behaviour, waste management techniques, and the manufacturing process of plastics. Collaborative approaches should be given priority in future research and policy endeavors in order to expedite the conversion of scientific information into practical solutions for ensuring sustainability.
7. Challenges and future perspectives
Aerogels, biochar, and electrospun micro-/nano fibrous constructs though offer potential solutions for the removal of MNP from various environments. Studies on this perspective are still in the preliminary stages with a broad set of open questions. In the coming decades, the demand for advanced smart, and efficient systems dealing with MNP contamination in water will likely increase as water scarcity and its associated environmental sustainability issues become more pressing.30,151 Such efforts may lead to the creation of new and improved classes of hybrid adsorbents to be employed in a range of domains, from large-scale WWTPs to small-scale household water management systems. Meanwhile, at this nascent stage, the utilization of biochar, aerogel, and electrospun-based adsorbent systems for MNP removal/separation/isolation faces several challenges that hinder the development of more efficient, sustainable, and viable technologies of which scalability, cost-effectiveness, and long-term environmental impacts prior to their integrated implementation as full-scale technology are the significant ones. Therefore, it is crucial to conceptually address the following aspects in their further exploration and development while dealing with technical challenges pertaining to (a) their practical implications, (b) their long-term interactions with the water ecosystem with regard to their influence on aquatic micro-organisms, and (c) the post-removal/segregation strategy to handle/neutralize such MNP. Some of the challenges associated with these techniques are as below.
7.1 Particle size, porosity, and permeability limitations of adsorbent systems
The effectiveness of aerogels, beads, and electrospun constructs in removing MNP depends on their pore dimensions, pore structure, and surface area. Aerogels are typically expected to capture a wide range of MPs particles and rather non-selectivity where designed with suitable shapes along with pore size, and their application mostly may remain regiospecific and in a certain locality of concern. In contrast, micro/nano-sized and micro/nanoporous biochar particles with a significant surface area are envisaged to be able to effectively capture and retain smaller anisotropic particles, considering the fact that the MNP capturing mechanism involves site-specific locking. For example, Wang et al. developed corn straw biochar with ∼808.3 ± 81.9 m2 g−1 total specific surface area, ∼1.318 mg m−3 particle density value, and ∼47.28 mL pore volume. The formed biochar was found effective in the removal of ∼95% of MPs spheres.158 Reportedly, biochar is effective in the removal of MPs spheres, provided biochar particle size remains appropriate and consistent since size-scale incompatibility between pores and MPs size may impede such a process. In contrast, electrospun fibrous constructs are used to favourably alter the passage of water and unwanted smaller particles while selectivity capturing MNP. For instance, Wang et al. developed a low-pressure driven electrospun membrane for the removal of PS. The electrospun membrane possesses outstanding mechanical stability as it was highly dependent upon the tensile strength (∼8 MPa) and tensile strain (∼58.5 ± 20.1%). Their high values suggest mechanical strength augmentation along with durability.139 Hence, apart from the porosity of such frameworks striking the right balance between capturing efficiency and water permeability may be a challenge. The typical mechanism behind the functional role of the electrospun membrane remains (a) permeation-assisted trapping and (b) ligand-induced selective binding of the membrane.159
7.2 Heterogeneity and polydispersity (size and nature) of MNP
MPs and NPs come in various shapes, sizes, and chemical compositions making their chemical functionality specific and size-selective removal/separation a complex task. The differential affinity of a diverse range of MNP to biochar functionality makes the process of separation adsorption/absorption extent dependent. In an effort to demonstrate the same, for example, Wu et al. developed rice straw-derived biochar with an excellent specific surface area of ∼327.65 m2 g−1.160 The result showed that the developed biochar with remarkable surface area and porosity offers excellent removal of MPs with a diameter of ∼300 nm from contaminated water. Similarly, for instance, Juraij et al. electrospun PU nanofiber membranes for the complete removal and separation of MPs and NPs (PS, ABS, and PMMA) from aqueous mediums.124 The prepared membrane possesses a fouling nature that follows a standard blocking model where foulants adhere to the inner wall of fiber and help in the removal of MNP.
7.3 Adsorption capacity selectivity and specificity
High adsorption capacity to effectively capture and retain MNP without compromising much in their chemical functionality and other desirable attributes such as mechanical stability or liability or hydrolytic stability may be extremely essential. For example, Ozden et al. developed egg protein-derived ultra-lightweight hybrid monolithic aerogel for the removal of MP. The adsorption capacity of developed aerogel was found ∼32.6 mg g−1 for the effective removal of MPs.153 However, the adsorption capacity of biochar for MNP may be limited by other substances present in water. Natural organic matter, dissolved ions, and other contaminants can compete with plastic particles for adsorption sites on biochar surfaces, reducing the overall removal efficiency. For instance, when biochar is introduced to a substrate over a specified concentration, it may have the effect of agglomeration, which lowers the specific surface area overall, preventing binding sites from being exposed and subsequently weakening the adsorption capacity.161 To demonstrate this effect, it has been observed that the adsorption of diclofenac by biochar fell from around 107 to approximately 25% when the quantity of biochar was raised from ∼2 to ∼20 g L−1.161 Similarly, in electrospun construct, the selective capture of MNP generally remains controlled by their network structure and is challenging in complex water matrices with diverse particle sizes and compositions.
7.4 Scale-up and cost-effectiveness
The scalability and cost-effectiveness of aerogels and biochar-based adsorbent systems for large-scale water treatment/decontamination with regard to MNP involve severe manufacturing engineering challenges. The design, operation, and maintenance of such decontamination-aiming filtration systems remain complex due to factors such as energy-intensive supercritical drying process as necessary for aerogels or the necessary optimization to ensure maximized efficiency while keeping the flow rate, contact time, and system stability in view for biochar-based set-ups. In the case of electrospun constructs as nanofibrous membranes, the large-scale manufacturing costs need to be connected by enhanced efficiency. For example, Risch and Adlhart developed a highly cost-effective CS/polyethylene oxide nanofiber sponge through the electrospinning method. The fabricated sponge was highly effective in the removal of poly(ethylene terephthalate) MPs up to ∼99.6%.140 The nanofiber sponge was found to be easy to synthesize in bulk amounts which suggests their outstanding scalability.
7.5 Stability, durability, regeneration and reusability
The adsorption capacity of aerogels, biochar, or even electrospun materials tends to suffer from fouling over a period of time, attributed to the saturation of the adsorption sites by the MPs and other contaminants. Therefore, suitable methods need to be adopted to ensure the regeneration and reusability of such frameworks. For instance, Wu et al. developed a rice straw-modified biochar system for the effective removal of MPs from the water. The formed biochar can be easily recovered through thermal treatment after the adsorption of MPs and can be reused again in the same manner. The system works efficiently and acts as regenerative in nature for up to >3 cycles.160 Similarly, in electrospun frameworks surface characteristics modulation via premodification during membrane fabrication by incorporating functional agents into spinning solution and post modification as the deposited membrane surface may enhance anti-fouling performance. Interestingly, aerogels may get fouled by water organic matter and other minerals hindering their ability to capture MNP. Hence, strategies to mitigate such surface fouling by smarter approaches may be necessary.
7.6 Impact on the environment
The environmental consequences of using such material frameworks need to be carefully assessed in such a manner that they should not enhance secondary MNP concentration post-use or production. Aerogels remain highly porous lightweight materials whose manufacturing, use, and disposal may leave their footprints. For example, Zheng et al. coral inspired environmental durability aerogel for the eradication of MPs from the aquatic environment.106 The formed aerogel system was found highly biocompatible, reusable, and environmentally friendly/durable in nature. Similarly, biochar may require a significant amount of biomass and hence sustainable approach is necessary. Disposal of spent biochar post MNP removal/separation must be assessed while aligning to maximized benefits of life-cycle analysis. Electrospun nanoporous membrane systems should also be looking from the angle that the membranes themselves do not become the source of MNP. Therefore, the utilization of biopolymers and non-toxic solvents in electrospinning referred to as bio-electrospinning, offers a promising avenue for developing efficient nanofibrous membranes for removal of MNP, aligning with sustainability considerations.
Furthermore, one of the primary sources of error lies in the accuracy of MNP quantification (in size, volume, and chemical constitutions) methods. Another source of error stems from assumptions about MNP behavior in aqueous environments, exhibiting complex transport dynamics influenced by factors such as particle size, surface properties, and environmental conditions. For example, Zhang et al. developed coagulant-coated bubbles for the removal of MPs and organic matter. Here the saline condition of media plays a significant role in the separation of MPs whereas the interaction behavior of MPs and organic matter influences the synergistic removal in different water mediums.162 Additionally, the effectiveness of the proposed emerging adsorbents, such as biochar, aerogel, and electrospun fiber, for MNP removal may be influenced by various factors such as adsorbent morphology, surface chemistry, and the presence of other dyes and heavy metal ions. For instance, the work reported by Li et al. involves the removal of MPs by considering different aging times through magnetic corncob biochar under the specified environmental condition.127 Recently, Zhuang et al. carried out the preparation of cellulose-based aerogels with structural direction through the freezing mechanism of liquid nitrogen. The developed aerogel can significantly adsorb MPs by reaching the stage of equilibrium in 20 min.156 Further, Juraji et al. (2023) developed electrospun PU nanofiber for the removal of MPs and nanoplastic. The mechanistic behavior depends upon the adsorptive separation and size-exclusive interception analysis respectively. Here, Langmuir adsorption isotherm defines the adsorption behavior of MNP.124 Assumptions regarding the adsorption mechanisms and kinetics of MNP onto adsorbents should be validated through experimental studies conducted under specified controlled conditions. For example, Heo et al. designed magnetic iron oxide nanoparticles for the removal of PS-based MPs through various adsorption isotherms and kinetic modeling. However, the maximum adsorption capacity was observed through the Langmuir adsorption isotherm model and pseudo-seconder kinetic model.163 Characterizing the adsorption capacity and affinity of adsorbents for different MNP types can improve the understanding of adsorption processes and optimize the adsorbent design for enhanced MNP removal efficiency. For example, Wang et al. performed the thermal degradation and adsorption of MNP through Mg/Zn modified magnetic biochar adsorbents. The modified ability of biochar highly augments the removal efficacy in a significant manner and through adsorbent regeneration/recyclability.132 In this regard, integrating advanced analytical techniques, computational modeling approaches, and experimental validation studies can provide comprehensive insights into MNP behavior and facilitate the development of effective strategies for MNP pollution mitigation. For instance, Mendonca et al. fabricated bacterial cellulose based hydrogel as a significant bio-flocculants for the eradication of MPs through computational analysis which implement the ratio and grinding times acts as potential variables during reliability and flocculation rate.108
8. Conclusion
The review paper delves into the escalating concerns on MNP pollution, along with its detrimental impacts on both the environment and human health. A systematic overview of the limitations of conventional techniques like filtration, sedimentation, and electrocoagulation, which have been widely employed for MNP removal, exposed challenges related to their efficacy, sustainability, and cost-effectiveness. Considering these challenges, further development has been stressed regarding the effective removal of MNP, contributing to the development of eco-friendly solutions. In this context, the current attempt aims at a comprehensive analysis of the three promising materials including biochar, electrospun fibrous constructs, and aerogels, that not only offer promising avenues for the effective removal of MNP from diverse environments but also align with the principles of circularity by utilizing waste materials, reducing the demand for single-use plastics, and promoting circular economy practices. However, it is important to note that further research, innovation, and collaboration are essential to optimize their performance and scale up production to fully exploit their long-term environmental advantages. Challenges such as scalability, cost-effectiveness, and long-term environmental impacts need to be addressed to ensure the practical implementation and commercial viability of these solutions, thereby realizing effective and implementable solutions for MNP removal. Thus, the current review offers a visionary glimpse into the transformative potential of these innovative adsorbent materials in combating the escalating crisis of plastic pollution, thereby beckoning material designers toward a future where environmental sustainability and cutting-edge technology join forces to safeguard the planet from such threats. Conclusively, future directions for developing and implementing such sustainable framework-based adsorbent systems to mitigate micro- and nano-plastic pollution encompass advancing innovative material development, comprehensively understanding adsorption mechanisms, rigorously evaluating real-world performance while ensuring environmental safety, optimizing procedures for scalability, and integrating further interdisciplinary research.
Data availability
No primary research results, software or code have been included and no new data were generated or analysed as part of this review.
Author contributions
Krishna Priyadarshini Das: conceptualization; writing – original draft & editing; Pooja Chauhan: writing – review & editing; Ulrike Staudinger: writing – review & editing; Bhabani Kumar Satapathy: conceptualization, resources; writing – review & editing.
Conflicts of interest
There are no conflicts to declare.
References
- M. Bergmann, B. C. Almroth, S. M. Brander, T. Dey, D. S. Green, S. Gundogdu, A. Krieger, M. Wagner and T. R. Walker, Science, 2022, 376, 469–470 Search PubMed.
- S. Afzal, A. Singh, S. R. Nicholson, T. Uekert, J. S. DesVeaux, E. C. D. Tan, A. Dutta, A. C. Carpenter, R. M. Baldwin and G. T. Beckham, Green Chem., 2023, 25, 5068–5085 Search PubMed.
- S. Hohn, E. Acevedo-Trejos, J. F. Abrams, J. Fulgencio de Moura, R. Spranz and A. Merico, Sci. Total Environ., 2020, 746, 141115 Search PubMed.
- S. Vijayaraman, P. Mondal, A. Nandan and N. A. Siddiqui, Presence of Microplastic in Water Bodies and Its Impact on Human Health, 2020, pp. 57–65, DOI:10.1007/978-981-15-0954-4_4.
- M. N. Issac and B. Kandasubramanian, Environ. Sci. Pollut. Res., 2021, 28, 19544–19562 Search PubMed.
- S. N. Dimassi, J. N. Hahladakis, M. N. D. Yahia, M. I. Ahmad, S. Sayadi and M. A. Al-Ghouti, Arabian J. Chem., 2022, 15, 104262 Search PubMed.
- M. S.-L. Yee, L.-W. Hii, C. K. Looi, W.-M. Lim, S.-F. Wong, Y.-Y. Kok, B.-K. Tan, C.-Y. Wong and C.-O. Leong, Nanomaterials, 2021, 11, 496 Search PubMed.
- M. Zhang, J. Yang, Z. Cai, Y. Feng, Y. Wang, D. Zhang and X. Pan, Environ. Sci.: Nano, 2019, 6, 709–735 Search PubMed.
- Q. Wang, J. Bai, B. Ning, L. Fan, T. Sun, Y. Fang, J. Wu, S. Li, C. Duan, Y. Zhang, J. Liang and Z. Gao, Chemosphere, 2020, 254, 126788 Search PubMed.
- E. Fournier, J. Ratel, S. Denis, M. Leveque, P. Ruiz, C. Mazal, F. Amiard, M. Edely, V. Bezirard, E. Gaultier, B. Lamas, E. Houdeau, E. Engel, F. Lagarde, L. Etienne-Mesmin, M. Mercier-Bonin and S. Blanquet-Diot, J. Hazard. Mater., 2023, 443, 130383 Search PubMed.
- D. Kawecki, P. R. W. Scheeder and B. Nowack, Environ. Sci. Technol., 2018, 52, 9874–9888 Search PubMed.
- S. S. Paul, R. Anirud, B. Bahl, K. Maheshwari and A. Banerjee, in Visualization Techniques for Climate Change with Machine Learning and Artificial Intelligence, Elsevier, 2023, pp. 77–100 Search PubMed.
- R. Ambrosini, R. S. Azzoni, F. Pittino, G. Diolaiuti, A. Franzetti and M. Parolini, Environ. Pollut., 2019, 253, 297–301 Search PubMed.
- C. Jiang, L. Yin, Z. Li, X. Wen, X. Luo, S. Hu, H. Yang, Y. Long, B. Deng, L. Huang and Y. Liu, Environ. Pollut., 2019, 249, 91–98 Search PubMed.
- D. Tatsii, S. Bucci, T. Bhowmick, J. Guettler, L. Bakels, G. Bagheri and A. Stohl, Environ. Sci. Technol., 2024, 58, 671–682 Search PubMed.
- I. Iavicoli, L. Fontana, V. Leso and A. Bergamaschi, Int. J. Mol. Sci., 2013, 14, 16732–16801 Search PubMed.
- A. L. Patrício Silva, J. C. Prata, T. R. Walker, A. C. Duarte, W. Ouyang, D. Barcelò and T. Rocha-Santos, Chem. Eng. J., 2021, 405, 126683 Search PubMed.
- Y. Peng, P. Wu, A. T. Schartup and Y. Zhang, Proc. Natl. Acad. Sci. U. S. A., 2021, 118(47) DOI:10.1073/pnas.2111530118.
- S. Dey, P. Samanta, D. Dutta, D. Kundu, A. R. Ghosh and S. Kumar, Environ. Sci. Pollut. Res., 2023, 30, 93363–93387 Search PubMed.
- R. Y. Krishnan, S. Manikandan, R. Subbaiya, N. Karmegam, W. Kim and M. Govarthanan, Sci. Total Environ., 2023, 858, 159681 Search PubMed.
- A. R. P. Pizzichetti, C. Pablos, C. Álvarez-Fernández, K. Reynolds, S. Stanley and J. Marugán, Case Stud. Chem. Environ. Eng., 2021, 3, 100075 Search PubMed.
- S. Bolan, L. P. Padhye, T. Jasemizad, M. Govarthanan, N. Karmegam, H. Wijesekara, D. Amarasiri, D. Hou, P. Zhou, B. K. Biswal, R. Balasubramanian, H. Wang, K. H. M. Siddique, J. Rinklebe, M. B. Kirkham and N. Bolan, Sci. Total Environ., 2024, 909, 168388 Search PubMed.
- S. H. Joo, Y. Liang, M. Kim, J. Byun and H. Choi, Environ. Challenges, 2021, 3, 100042 Search PubMed.
- V. I. Isaeva, M. D. Vedenyapina, A. Y. Kurmysheva, D. Weichgrebe, R. R. Nair, N. P. T. Nguyen and L. M. Kustov, Molecules, 2021, 26, 6628 Search PubMed.
- D. Pedrero, C. Edo, F. Fernández-Piñas, R. Rosal and S. Aguado, Sep. Purif. Technol., 2024, 333, 125816 Search PubMed.
- J. Yang, X. Chen, J. Zhang, Y. Wang, H. Wen and J. Xie, Int. J. Biol. Macromol., 2021, 189, 53–64 Search PubMed.
- P. S. Goh, H. S. Kang, A. F. Ismail, W. H. Khor, L. K. Quen and D. Higgins, Chemosphere, 2022, 299, 134418 Search PubMed.
- D. You, Y. Zhao, W. Yang, Q. Pan and J. Li, Chem. Res. Chin. Univ., 2022, 38, 186–191 Search PubMed.
- G. Zhu, J. Wang, X. Wang, Y. Liu, W. Wu, H. Xiao, L. Huang, H. Dai, X. Zhou and H. Bian, ACS Sustain. Chem. Eng., 2023, 11, 13928–13938 Search PubMed.
- Z. Wang, M. Sedighi and A. Lea-Langton, Water Res., 2020, 184, 116165 Search PubMed.
- M. Rist and A. Greiner, RSC Applied Polymers, 2024 10.1039/D3LP00201B.
- H. Park and B. Park, Water, 2021, 13, 2736 Search PubMed.
- R. Ahmed, A. K. Hamid, S. A. Krebsbach, J. He and D. Wang, Chemosphere, 2022, 293, 133557 Search PubMed.
- S. D. Martinho, V. C. Fernandes, S. A. Figueiredo and C. Delerue-Matos, Int. J. Environ. Res. Public Health, 2022, 19, 5610 Search PubMed.
- T. A. Lastovina and A. P. Budnyk, Journal of Water Process Engineering, 2021, 43, 102209 Search PubMed.
- I. Nabi, A.-U.-R. Bacha and L. Zhang, J. Cleaner Prod., 2022, 337, 130458 Search PubMed.
- V. Kumar, M. Umesh, P. Chakraborty, P. Sharma, S. Sarojini, T. Basheer, K. Kaur, R. Pasrija and D. Barcelo, TrAC, Trends Anal. Chem., 2024, 170, 117392 Search PubMed.
- N. Parashar and S. Hait, Sci. Total Environ., 2021, 759, 144274 Search PubMed.
- N. U. Benson, O. H. Fred-Ahmadu, D. E. Bassey and A. A. Atayero, J. Environ. Chem. Eng., 2021, 9, 105222 Search PubMed.
- M. W. Peng and N. Kathuria, Journal of Management Studies, 2021, 58, 1431–1435 Search PubMed.
- C. Guerranti, T. Martellini, G. Perra, C. Scopetani and A. Cincinelli, Environ. Toxicol. Pharmacol., 2019, 68, 75–79 Search PubMed.
- P. K. Cheung and L. Fok, Water Res., 2017, 122, 53–61 Search PubMed.
- E. Hernandez, B. Nowack and D. M. Mitrano, Environ. Sci. Technol., 2017, 51, 7036–7046 Search PubMed.
- Z. Sobhani, Y. Lei, Y. Tang, L. Wu, X. Zhang, R. Naidu, M. Megharaj and C. Fang, Sci. Rep., 2020, 10, 4841 Search PubMed.
- M. G. A. Barros, R. dos Santos Grignet, S. P. B. Bernal, C. D. C. S. Gonçalves, M. R. Z. Passarini and J. R. Ottoni, in Microbial Inoculants, Elsevier, 2023, pp. 207–228 Search PubMed.
- J. Wang, X. Liu, Y. Li, T. Powell, X. Wang, G. Wang and P. Zhang, Sci. Total Environ., 2019, 691, 848–857 Search PubMed.
- Y. Chen, A. K. Awasthi, F. Wei, Q. Tan and J. Li, Sci. Total Environ., 2021, 752, 141772 Search PubMed.
- Y. Zhang, Y. Li, F. Su, L. Peng and D. Liu, Sci. Total Environ., 2022, 802, 149658 Search PubMed.
- W. Li, R. Wufuer, J. Duo, S. Wang, Y. Luo, D. Zhang and X. Pan, Sci. Total Environ., 2020, 749, 141420 Search PubMed.
- N. Katsumi, T. Kusube, S. Nagao and H. Okochi, Chemosphere, 2021, 267, 129185 Search PubMed.
- R. R. Hurley and L. Nizzetto, Current Opinion in Environmental Science & Health, 2018, 1, 6–11 Search PubMed.
- J. Li, H. Liu and J. Paul Chen, Water Res., 2018, 137, 362–374 Search PubMed.
- M. Bläsing and W. Amelung, Sci. Total Environ., 2018, 612, 422–435 Search PubMed.
- D. R. da Silva Souza, L. D. Caminhas, J. P. de Mesquita and F. V. Pereira, Mater. Chem. Phys., 2018, 203, 148–155 Search PubMed.
- N. Khalid, M. Aqeel, A. Noman and Z. Fatima Rizvi, J. Hazard. Mater., 2023, 445, 130455 Search PubMed.
- P. J. Kole, A. J. Löhr, F. Van Belleghem and A. Ragas, Int. J. Environ. Res. Public Health, 2017, 14, 1265 Search PubMed.
- B. Baensch-Baltruschat, B. Kocher, F. Stock and G. Reifferscheid, Sci. Total Environ., 2020, 733, 137823 Search PubMed.
- J. Worek, X. Badura, A. Białas, J. Chwiej, K. Kawoń and K. Styszko, Energies, 2022, 15, 2816 Search PubMed.
- M. Lee and H. Kim, Nanomaterials, 2022, 12, 851 Search PubMed.
- F. Haque and C. Fan, J. Cleaner Prod., 2022, 367, 133027 Search PubMed.
- J. C. Prata, J. P. da Costa, I. Lopes, A. C. Duarte and T. Rocha-Santos, Sci. Total Environ., 2020, 702, 134455 Search PubMed.
- N. U. Benson, D. E. Bassey and T. Palanisami, Heliyon, 2021, 7, e06343 Search PubMed.
- F. Saliu, M. Veronelli, C. Raguso, D. Barana, P. Galli and M. Lasagni, Environ. Adv., 2021, 4, 100042 Search PubMed.
- S. M. Mintenig, I. Int-Veen, M. G. J. Löder, S. Primpke and G. Gerdts, Water Res., 2017, 108, 365–372 Search PubMed.
- J. Sun, Z.-R. Zhu, W.-H. Li, X. Yan, L.-K. Wang, L. Zhang, J. Jin, X. Dai and B.-J. Ni, Water Res., 2021, 190, 116784 Search PubMed.
- S. Agarwal, Macromol. Chem. Phys., 2020, 221(6) DOI:10.1002/macp.202000017.
- Y. Xiang, L. Jiang, Y. Zhou, Z. Luo, D. Zhi, J. Yang and S. S. Lam, J. Hazard. Mater., 2022, 422, 126843 Search PubMed.
- T. J. Baker, C. R. Tyler and T. S. Galloway, Environ. Pollut., 2014, 186, 257–271 Search PubMed.
- P. Bhattacharya, Acta Biomedica Scientia, 2016, 3, 47–52 Search PubMed.
- N. von Moos, P. Burkhardt-Holm and A. Köhler, Environ. Sci. Technol., 2012, 46, 11327–11335 Search PubMed.
- W. Luo, L. Su, N. J. Craig, F. Du, C. Wu and H. Shi, Environ. Pollut., 2019, 246, 174–182 Search PubMed.
- L. Yin, C. Jiang, X. Wen, C. Du, W. Zhong, Z. Feng, Y. Long and Y. Ma, Int. J. Environ. Res. Public Health, 2019, 16, 1650 Search PubMed.
- X. Xiong, K. Zhang, X. Chen, H. Shi, Z. Luo and C. Wu, Environ. Pollut., 2018, 235, 899–906 Search PubMed.
- L. Ding, R. Fan Mao, X. Guo, X. Yang, Q. Zhang and C. Yang, Sci. Total Environ., 2019, 667, 427–434 Search PubMed.
- D. Barceló, Y. Picó and A. H. Alfarhan, Environ. Toxicol. Pharmacol., 2023, 101, 104204 Search PubMed.
- D. Gu, P. Zhang, L. Zhang, H. Liu, Z. Pu and S. Shang, Opt. Mater., 2018, 83, 272–278 Search PubMed.
- Y.-J. Chen, Y. Chen, C. Miao, Y.-R. Wang, G.-K. Gao, R.-X. Yang, H.-J. Zhu, J.-H. Wang, S.-L. Li and Y.-Q. Lan, J. Mater. Chem. A, 2020, 8, 14644–14652 Search PubMed.
- S. Dong, Q. Ji, Y. Wang, H. Liu and J. Qu, J. Environ. Sci., 2020, 89, 102–112 Search PubMed.
- J. Tan, Y. Chen, Z. Mo, C. Tan, R. Wen, Z. Chen and H. Tian, Environ. Sci. Pollut. Res., 2022, 29, 61534–61546 Search PubMed.
- G. Zhou, Q. Wang, J. Li, Q. Li, H. Xu, Q. Ye, Y. Wang, S. Shu and J. Zhang, Sci. Total Environ., 2021, 752, 141837 Search PubMed.
- N. Khalid, M. Aqeel and A. Noman, Environ. Pollut., 2020, 267, 115653 Search PubMed.
- S. Kublik, S. Gschwendtner, T. Magritsch, V. Radl, M. C. Rillig and M. Schloter, Front. Environ. Sci., 2022, 10 DOI:10.3389/fenvs.2022.989267.
- M. Sajjad, Q. Huang, S. Khan, M. A. Khan, Y. Liu, J. Wang, F. Lian, Q. Wang and G. Guo, Environ. Technol. Innovation, 2022, 27, 102408 Search PubMed.
- C. Wang, J. Zhao and B. Xing, J. Hazard. Mater., 2021, 407, 124357 Search PubMed.
- Y. Zhang, T. Gao, S. Kang and M. Sillanpää, Environ. Pollut., 2019, 254, 112953 Search PubMed.
- Z. Wang, Y. Qin, W. Li, W. Yang, Q. Meng and J. Yang, Environ. Chem. Lett., 2019, 17, 1821–1830 Search PubMed.
- Z. Li, Q. Li, R. Li, Y. Zhao, J. Geng and G. Wang, Environ. Sci. Pollut. Res., 2020, 27, 30306–30314 Search PubMed.
- M. Klein and E. K. Fischer, Sci. Total Environ., 2019, 685, 96–103 Search PubMed.
- J. Zhang, L. Wang, L. Trasande and K. Kannan, Environ. Sci. Technol. Lett., 2021, 8, 989–994 Search PubMed.
- Y. Zhang, T. Gao, S. Kang, H. Shi, L. Mai, D. Allen and S. Allen, Earth-Sci. Rev., 2022, 226, 103924 Search PubMed.
- L. E. Revell, P. Kuma, E. C. Le Ru, W. R. C. Somerville and S. Gaw, Nature, 2021, 598, 462–467 Search PubMed.
- X. Li, S. Yao, Z. Wang, X. Jiang, Y. Song and S. X. Chang, Environ. Pollut., 2022, 315, 120433 Search PubMed.
- Y.-L. Zhang, S.-C. Kang and T.-G. Gao, Advances in Climate Change Research, 2022, 13, 455–458 Search PubMed.
- J. C. Prata, A. L. P. Silva, J. P. da Costa, C. Mouneyrac, T. R. Walker, A. C. Duarte and T. Rocha-Santos, Int. J. Environ. Res. Public Health, 2019, 16, 2411 Search PubMed.
- X. Chen, X. Chen, Y. Zhao, H. Zhou, X. Xiong and C. Wu, Sci. Total Environ., 2020, 719, 137276 Search PubMed.
- M. Zhang, K. Lin, X. Li, L. Wu, J. Yu, S. Cao, D. Zhang, L. Xu, S. J. Parikh and Y. S. Ok, Environ. Pollut., 2022, 293, 118521 Search PubMed.
- V. Pillay, A. Seedat, Y. E. Choonara, L. C. du Toit, P. Kumar and V. M. K. Ndesendo, AAPS PharmSciTech, 2013, 14, 692–711 Search PubMed.
- I. E. Gilani, S. Sayadi, N. Zouari and M. A. Al-Ghouti, Bioresour. Technol. Rep., 2023, 24, 101606 Search PubMed.
- R. C. Hale, A. E. King and J. M. Ramirez, in Microplastic Contamination in Aquatic Environments, Elsevier, 2024, pp. 1–31 Search PubMed.
- S. Ghatge, Y. Yang, J.-H. Ahn and H.-G. Hur, Appl. Biol. Chem., 2020, 63, 27 Search PubMed.
- M. Arhant, M. Le Gall, P.-Y. Le Gac and P. Davies, Polym. Degrad. Stab., 2019, 161, 175–182 Search PubMed.
- T. Poerio, E. Piacentini and R. Mazzei, Molecules, 2019, 24(22), 4148 Search PubMed.
- L. Feng, Q. Zhang, F. Ji, L. Jiang, C. Liu, Q. Shen and Q. Liu, Chem. Eng. J., 2022, 430, 132754 Search PubMed.
- L. Liu, M. Xu, Y. Ye and B. Zhang, Sci. Total Environ., 2022, 806, 151312 Search PubMed.
- M. Rashid, N. T. Price, M. Á. Gracia Pinilla and K. E. O'Shea, Water Res., 2017, 123, 353–360 Search PubMed.
- B. Zheng, B. Li, H. Wan, X. Lin and Y. Cai, J. Hazard. Mater., 2022, 431, 128611 Search PubMed.
- M. Malankowska, C. Echaide-Gorriz and J. Coronas, Environ. Sci.: Water Res. Technol., 2021, 7, 243–258 Search PubMed.
- I. Mendonça, J. Sousa, C. Cunha, M. Faria, A. Ferreira and N. Cordeiro, Chemosphere, 2023, 314, 137719 Search PubMed.
- Q. Guo, Y. Liu, J. Liu, Y. Wang, Q. Cui, P. Song, X. Zhang and C. Zhang, Chem. Mater., 2022, 34, 5165–5175 Search PubMed.
- M. E. Mahmoud, M. F. Amira, S. Daniele, M. E. Abouelanwar and B. M. Morcos, J. Taiwan Inst. Chem. Eng., 2023, 146, 104793 Search PubMed.
- A. Ullah, G.-J. Lee, H. Kim, H. T. Kwon and S. I. Lim, Int. J. Biol. Macromol., 2023, 242, 124698 Search PubMed.
- J. Talvitie, A. Mikola, A. Koistinen and O. Setälä, Water Res., 2017, 123, 401–407 Search PubMed.
- K. Gurung, M. C. Ncibi and J. M. Fontmorin, J. Membr. Sci. Technol., 2016, 6(3) DOI:10.4172/2155-9589.1000158.
- C. Akarsu, H. Kumbur and A. E. Kideys, Water Sci. Technol., 2021, 84, 1648–1662 Search PubMed.
- L. T. Yogarathinam, J. Usman, M. H. D. Othman, A. F. Ismail, P. S. Goh, A. Gangasalam and M. R. Adam, J. Hazard. Mater., 2022, 424, 127298 Search PubMed.
- L. Yang, X. Cao, J. Cui, Y. Wang, Z. Zhu, H. Sun, W. Liang, J. Li and A. Li, J. Colloid Interface Sci., 2022, 617, 673–682 Search PubMed.
- N. Yahyanezhad, M. J. Bardi and H. Aminirad, Water Practice & Technology, 2021, 16, 782–792 Search PubMed.
- H. Wan, J. Wang, X. Sheng, J. Yan, W. Zhang and Y. Xu, Toxics, 2022, 10, 70 Search PubMed.
- G. Gnanasekaran, A. G and Y. S. Mok, Sep. Purif. Technol., 2021, 277, 119655 Search PubMed.
- J. Qin, Y. Dou, F. Wu, Y. Yao, H. R. Andersen, C. Hélix-Nielsen, S. Y. Lim and W. Zhang, Appl. Catal., B, 2022, 319, 121940 Search PubMed.
- S. Modak, M. Kasula and M. R. Esfahani, ACS Appl. Eng. Mater., 2023, 1, 744–755 Search PubMed.
- M. Haris, M. W. Khan, A. Zavabeti, N. Mahmood and N. Eshtiaghi, Chem. Eng. J., 2023, 455, 140390 Search PubMed.
- B. Fryczkowska and L. Przywara, Desalin. Water Treat., 2021, 214, 252–262 Search PubMed.
- K. Juraij, S. P. Ammed, C. Chingakham, B. Ramasubramanian, S. Ramakrishna, S. Vasudevan and A. Sujith, ACS Appl. Nano Mater., 2023, 6, 4636–4650 Search PubMed.
- J. Zhuang, N. Rong, X. Wang, C. Chen and Z. Xu, Sep. Purif. Technol., 2022, 293, 121133 Search PubMed.
- S. Ye, M. Cheng, G. Zeng, X. Tan, H. Wu, J. Liang, M. Shen, B. Song, J. Liu, H. Yang and Y. Zhang, Water Res., 2020, 179, 115876 Search PubMed.
- J. Li, X. Chen, S. Yu and M. Cui, Sci. Total Environ., 2023, 875, 162647 Search PubMed.
- T. Chen, Y. Zhang, H. Wang, W. Lu, Z. Zhou, Y. Zhang and L. Ren, Bioresour. Technol., 2014, 164, 47–54 Search PubMed.
- M. Verma, P. Singh and M. Dhanorkar, J. Chem. Technol. Biotechnol., 2024, 99, 330–342 Search PubMed.
- N. Singh, N. Khandelwal, Z. A. Ganie, E. Tiwari and G. K. Darbha, Chem. Eng. J., 2021, 418, 129405 Search PubMed.
- V. Siipola, S. Pflugmacher, H. Romar, L. Wendling and P. Koukkari, Appl. Sci., 2020, 10, 788 Search PubMed.
- J. Wang, C. Sun, Q.-X. Huang, Y. Chi and J.-H. Yan, J. Hazard. Mater., 2021, 419, 126486 Search PubMed.
- J. Wu, C. Yang, H. Zhao, J. Shi, Z. Liu, C. Li and F. Song, Environ. Sci. Pollut. Res., 2022, 30, 26914–26928 Search PubMed.
- L. Hsieh, L. He, M. Zhang, W. Lv, K. Yang and M. Tong, Water Res., 2022, 221, 118783 Search PubMed.
- Y. Sun, S. M. Shaheen, E. F. Ali, H. Abdelrahman, B. Sarkar, H. Song, J. Rinklebe, X. Ren, Z. Zhang and Q. Wang, Environ. Pollut., 2022, 306, 119339 Search PubMed.
- Y. Shi, J. Du, T. Zhao, B. Feng, H. Bian, S. Shan, J. Meng, P. Christie, M. H. Wong and J. Zhang, Environ. Pollut., 2023, 318, 120897 Search PubMed.
- Q. Shi, S. Guo, J. Tang, H. Lyu, C. Ri and H. Sun, Environ. Pollut., 2023, 316, 120696 Search PubMed.
- M. N. Pervez, M. R. Mishu, M. E. Talukder, G. K. Stylios, A. Buonerba, S. W. Hasan, Y. Cai, Y. Zhao, A. Figoli, T. Zarra, V. Belgiorno and V. Naddeo, Water Emerging Contam. Nanoplast., 2022, 1, 10 Search PubMed.
- R. Wang, L. Zhang, B. Chen and X. Zhu, J. Membr. Sci., 2020, 614, 118470 Search PubMed.
- P. Risch and C. Adlhart, ACS Appl. Polym. Mater., 2021, 3, 4685–4694 Search PubMed.
- T. Aslan, S. Arslan, M. Eyvaz, S. Güçlü, E. Yüksel and İ. Koyuncu, J. Membr. Sci., 2016, 520, 616–629 Search PubMed.
- Z. Wang, C. Crandall, R. Sahadevan, T. J. Menkhaus and H. Fong, Polymer, 2017, 114, 64–72 Search PubMed.
- Y. Liu, R. Wang, H. Ma, B. S. Hsiao and B. Chu, Polymer, 2013, 54, 548–556 Search PubMed.
- A. K. Gautam, C. Lai, H. Fong and T. J. Menkhaus, J. Membr. Sci., 2014, 466, 142–150 Search PubMed.
- C. Hachemi, M. Enfrin, A. O. Rashed, V. Jegatheesan, P. D. Hodgson, D. L. Callahan, J. Lee and L. F. Dumée, Chemosphere, 2023, 310, 136891 Search PubMed.
- M. Enfrin, C. Hachemi, D. L. Callahan, J. Lee and L. F. Dumée, Sep. Purif. Technol., 2021, 278, 119592 Search PubMed.
- F. Zhu, Y.-M. Zheng, B.-G. Zhang and Y.-R. Dai, J. Hazard. Mater., 2021, 401, 123608 Search PubMed.
- R. Senthil, V. Sumathi, A. Tamilselvi, S. B. Kavukcu and A. W. Aruni, Sci. Rep., 2022, 12, 8411 Search PubMed.
- H. Chen, M. Huang, Y. Liu, L. Meng and M. Ma, Sci. Total Environ., 2020, 739, 139944 Search PubMed.
- F. Yu, Z. Yang, X. Zhang, P. Yang and J. Ma, Environ. Technol. Innovation, 2021, 24, 102052 Search PubMed.
- F. Liu, Y. Zhang, L. Zhong, Q. Feng, Z. Dong and Z. Xu, Int. J. Biol. Macromol., 2024, 256, 128326 Search PubMed.
- Q. Liu and S. M. Khor, TrAC, Trends Anal. Chem., 2024, 170, 117465 Search PubMed.
- S. Ozden, S. Monti, V. Tozzini, N. S. Dutta, S. Gili, N. Caggiano, A. J. Link, N. M. Pugno, J. Higgins, R. D. Priestley and C. B. Arnold, Mater. Today, 2022, 59, 46–55 Search PubMed.
- Z. Chen, S. Liu, L. Chen, J. Huang, B. Zheng, W. Huang, S. Li, Y. Lu and R. Fu, Chem. Eng. J., 2021, 418, 129315 Search PubMed.
- Z.-J. Nie, Q.-F. Guo, H. Xia, M.-M. Song, Z.-J. Qiu, S.-T. Fan, Z.-H. Chen, S.-X. Zhang, S. Zhang and B.-J. Li, J. Environ. Chem. Eng., 2021, 9, 104749 Search PubMed.
- J. Zhuang, M. Pan, Y. Zhang, F. Liu and Z. Xu, Int. J. Biol. Macromol., 2023, 235, 123884 Search PubMed.
- X. Meng, X. Peng, Y. Wei, S. Ramakrishna, Y. Sun and Y. Dai, Chem. Eng. J., 2022, 437, 135444 Search PubMed.
- H. Wang, H. Teng, X. Wang, J. Xu and L. Sheng, J. Environ. Manage., 2022, 310, 114758 Search PubMed.
- Z. Meng, L. Zhu, X. Wang and M. Zhu, Acc. Mater. Res., 2023, 4, 180–192 Search PubMed.
- W. Wu, M. Yang, Q. Feng, K. McGrouther, H. Wang, H. Lu and Y. Chen, Biomass Bioenergy, 2012, 47, 268–276 Search PubMed.
- M. Dong, L. He, M. Jiang, Y. Zhu, J. Wang, W. Gustave, S. Wang, Y. Deng, X. Zhang and Z. Wang, Int. J. Environ. Res. Public Health, 2023, 20, 1679 Search PubMed.
- M. Zhang, J. Yang, Z. Kang, X. Wu, L. Tang, Z. Qiang, D. Zhang and X. Pan, J. Hazard. Mater., 2021, 404, 124095 Search PubMed.
- Y. Heo, E.-H. Lee and S.-W. Lee, Chemosphere, 2022, 307, 135672 Search PubMed.
|
This journal is © The Royal Society of Chemistry 2024 |