DOI:
10.1039/D3TB02740F
(Review Article)
J. Mater. Chem. B, 2024,
12, 4533-4552
Strategies to improve the performance of polyetheretherketone (PEEK) as orthopedic implants: from surface modification to addition of bioactive materials
Received
17th November 2023
, Accepted 4th March 2024
First published on 13th March 2024
Abstract
Polyetheretherketone (PEEK), as a high-performance polymer, is widely used for bone defect repair due to its homogeneous modulus of elasticity of human bone, good biocompatibility, excellent chemical stability and projectability. However, the highly hydrophobic surface of PEEK is biologically inert, which makes it difficult for cells and proteins to attach, and is accompanied by the development of infections that ultimately lead to failure of PEEK implants. In order to further enhance the potential of PEEK as an orthopedic implant, researchers have explored modification methods such as surface modification by physical and chemical means and the addition of bioactive substances to PEEK-based materials to enhance the mechanical properties, osteogenic activity and antimicrobial properties of PEEK. However, these current modification methods still have obvious shortcomings in terms of cost, maneuverability, stability and cytotoxicity, which still need to be explored by researchers. This paper reviews some of the modification methods that have been used to improve the performance of PEEK over the last three years in anticipation of the need for researchers to design PEEK orthopedic implants that better meet clinical needs.
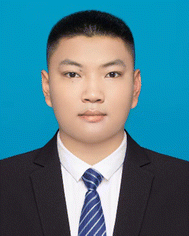
Huagui Huang
| Huagui Huang received his bachelor's degree. He graduated from Sichuan University. He is currently a graduate student at Dalian Medical University under the supervision of Prof. Zhonghai Li. His research interests mainly include intervertebral disc degeneration and the development of high performance bone repair materials. |
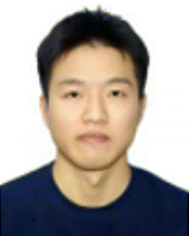
Xin Liu
| Xin Liu received his bachelor's degree. He is currently a graduate student at Dalian Medical University under the supervision of Prof. Zhonghai Li. His research interests mainly include organoid of bone and development of high performance bone repair materials. |
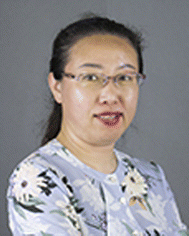
Honghua Wang
| Honghua Wang, PhD, is an associate researcher at Dalian Institute of Chemical Physics, Chinese Academy of Sciences. He has long been engaged in the molecular design, synthesis, modification of amorphous polyaryletherketone/sulfone and its application development in advanced composites, foams, coatings, 3D printing and other fields. |
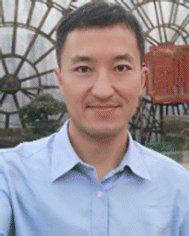
Chengde Liu
| Chengde Liu, PhD supervisor, is affiliated with the Department of Polymer Materials, School of Chemical Engineering, Dalian University of Technology. His main research work is the synthesis and surface modification of heteronaphthalene biphenyl polyaryl ether bone implant materials. Through molecular structure design, optimization of the synthesis method of polyaryl ethers and development of novel purification technology, we have obtained medical heteronaphthalene biphenyl polyaryl ethers with mechanical and biological properties that meet the requirements of polyaryl ether biomaterials. |
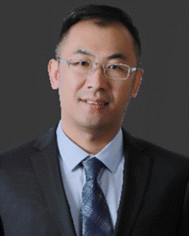
Zhonghai Li
| Dr Zhonghai Li received his PhD degree from the Second Military Medical University in 2012. He is currently a professor at the First Hospital of Dalian Medical University and a researcher at the Key Laboratory of Molecular Mechanisms of Repair and Remodeling of Orthopedic Diseases in Liaoning Province. His research interests include intervertebral disc degeneration, bone repair material development, and stem cell therapy. |
1. Introduction
Bone defects caused by trauma, tumors, infections and functional atrophy have become one of the major global health problems that seriously affect the function of patients' limbs and cause physical and psychological damage.1 For small bone defects, bone tissue can restore its basic structure and function on its own and usually does not require additional treatment. However, when the extent of the defect exceeds the critical size threshold (approximately >2 cm) or when more than 50% of the bone circumference is missing as well as when accompanied by inadequate blood supply, local infection, drug side effects, and malnutrition, the bone defect is difficult to recover and additional management is often required to aid in its recovery.2
Bone grafting is considered the gold standard for the treatment of bone defects, and it is the second most common tissue graft worldwide after blood transfusion.3,4 Currently, the commonly used grafts mainly include autologous bone, allogeneic bone, heterogeneous bone and synthetic grafts.5 Among them, autologous bone is the first choice of bone grafts, with excellent osteogenic properties and low histocompatibility.6 However, disadvantages such as limited sources, high treatment costs, high risk of immune rejection, complex bone handling techniques, and risk of infection and complications limit its clinical use.7 Homogeneous bone also has disadvantages such as genetic differences, anatomical variation, and disease transmission.8 Therefore, the search for alternative synthetic grafts has a wide prospect of applications.
The biomaterials commonly used in synthetic grafts include metals, ceramics, natural polymers and synthetic polymers.9 Metallic materials mainly include titanium (Ti), tantalum (Ta), cobalt (Co) and stainless steel. It is widely used as a replacement for load-bearing bone because of its excellent mechanical properties and corrosion resistance. However, the high stiffness of metal compared to natural bone can lead to loosening of the implant, and metal wear can produce toxic substances and by-products that can adversely affect bone tissue.10 Among all materials, bioceramics have the best biocompatibility because their composition and structure are highly similar to that of human bone.9 Nevertheless, the high bio-fragility is a major limitation for its clinical application.11 Natural materials such as collagen, chitosan, sodium alginate silk fibroin and hyaluronic acid, which can mimic the natural extracellular matrix (ECM), have advantages in terms of material acquisition, plasticity and biocompatibility, but their poor mechanical properties are difficult to meet the needs of bone tissue engineering.12
Polymers, on the other hand, are more readily available and can be processed under a variety of conditions to meet implant shape and porosity requirements, making them ideal for use as orthopedic implants.
With the development of synthetic polymeric materials, FDA-approved high-performance polyetheretherketone (PEEK) has been widely used in orthopedic implants. The investigation of modification strategies to enhance the performance of PEEK is currently a hot topic of research. At present, a variety of modification methods are used to improve the performance of PEEK materials, and Fig. 1 summarizes the relevant modification methods. In this paper, we review the changes in mechanical properties, osteogenic activity and antimicrobial properties of PEEK-based composites prepared by physically blending, chemically bonding and surface coating bioactive materials with PEEK over the past three years by various methods of physical and chemical modification of PEEK surfaces. It is expected to help researchers to find PEEK orthopedic implants with excellent osteogenic activity and antimicrobial properties to address the lack of PEEK bioactivity.
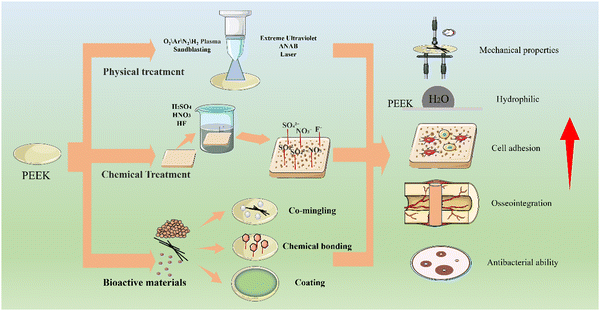 |
| Fig. 1 PEEK can be physically and chemically modified by surface modification and addition of bioactive materials to improve its physicochemical properties, bioactivity and antimicrobial capacity. | |
2. PEEK
PEEK, a key member of the polyaryletherketone family, is a high-temperature thermoplastic polymer consisting of aromatic backbone molecular chains interconnected by ketone and ether functional groups. In the late 1990s, PEEK gradually replaced metals as the new choice for orthopedic implants.13 As an orthopedic implant, PEEK has good biocompatibility, is less likely to cause allergic reactions and is not cytotoxic.14 The elastic modulus of PEEK is 3 to 4 GPa, similar to that of human bone, allowing PEEK to produce no stress shielding similar to that caused by the high elastic modulus of metal implants.15 The resonance stabilized chemical structure of PEEK results in delocalization of higher orbital electrons along the entire macromolecule, making it extremely unreactive and inherently resistant to chemical, thermal, and post-irradiation degradation. Except for concentrated sulfuric acid, PEEK is not damaged by exposure to solvents.13 PEEK is less affected by external temperature and PEEK requires processing temperatures to be exceeded to produce volatile degradation products.16 Despite the use of gamma and electron beam radiation it is also difficult to generate free radicals in PEEK.17 This allows the implanted PEEK to be stabilized in the body and provide effective long-term support. As a thermoplastic polymer, PEEK can be processed by melt 3D printing, which increases its advantages in the medical field. In addition, for patients requiring imaging in the clinic, PEEK materials possess good radiographic permeability and do not produce artifacts. These advantages make PEEK a suitable material for surgical implants. However, PEEK has a highly hydrophobic and biologically inert surface and has no antimicrobial properties, which is a major cause of infection and hinders bone repair.
Currently, PEEK materials are widely used in clinical settings. For example, spinal cages with good radioactivity and low modulus of elasticity,18 as well as carbon fiber reinforced PEEK that can meet the modulus of elasticity and friction properties required for dental and joint replacements.19 In addition, the application of PEEK in areas such as repair of cranial or maxillofacial defects and fixation devices is becoming more and more widespread.20 The advantages and disadvantages of PEEK bioactive materials and the current status of their application are shown in Fig. 2.
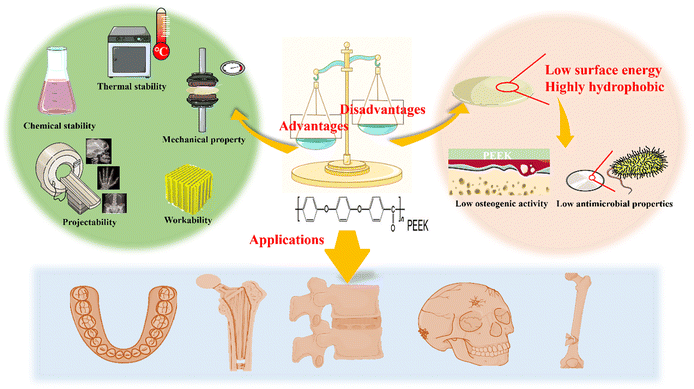 |
| Fig. 2 The advantages and disadvantages of PEEK as a medical implant and its application in the field of bone repair are summarized. | |
3. Surface modification
To overcome implantation failure due to highly hydrophobic and biologically inert PEEK surfaces, various physical and chemical surface modification methods have been used to improve their biocompatibility. After modification, PEEK surfaces roughness increases, surface energy decreases, hydrophilicity increases, and the chemical structure can change or even introduce new functional groups. This results in better cell adhesion and proliferation on the PEEK surface and good osseointegration of the surrounding tissues with the PEEK. The advantages and disadvantages of some surface modification techniques are summarized in Table 1.
Table 1 Advantages and disadvantages of some physical and chemical surface modification methods
Modification method |
Advantages |
Disadvantages |
ANAB, Accelerated neutral atom beam. |
Plasma treatment |
Increased roughness, hydrophilicity and surface energy, introduction of functional groups.24,25 |
Easy aging, poor stability, high temperature can lead to the destruction of the PEEK structure.26,27 |
Laser treatment |
Easy processing, high precision, high repeatability, low thermal damage, low oxidation effect.29,30 |
Nanopore clusters may be unevenly dispersed, and higher energy lasers can impair cell adhesion and migration.31 |
Sandblasting treatment |
Low cost, easy to operate, adjustable roughness.33 |
Physical change of roughness only, no introduction of functional groups.34 |
Extreme ultraviolet |
High absorbability, can be mixed with gas to increase the number of functional groups.35 |
Very limited depth of surface penetration, less formation of new chemical bonds, higher cost.36,37 |
ANAB |
Superior nanostructure and more chemical exposure without damaging the material surface.38 |
The penetration capacity is weak and the osteogenic induction potential is still limited.39 |
Sulfonation |
Low cost, simple operation, can introduce a large number of functional groups, with certain antibacterial ability.45–51 |
Residual sulfuric acid as well as newly generated sulfonate groups, oxygen radicals, sulfur dioxide and its derivatives can damage cells and DNA.41,44 |
Acid etching |
Less cytotoxicity of samples, diverse functional groups, low cost.53,54 |
Weak corrosion effect.55,56 |
3.1. Physical treatment
When applied to the surface of PEEK by physical means such as grinding, impact, and lasers, they can increase its roughness, wettability, mechanical properties, and even introduce various types of reactive groups to enhance bioactivity. A variety of physical methods have been used to physically modify PEEK, and we highlight a few of the most commonly used in current research.
3.1.1. Plasma treatment.
Plasma treatment is one of the most commonly used physical treatments today. Plasma is an ionized gas produced in a closed reactor system.21 These gases mainly include argon, nitrogen, oxygen, ammonia, methane and water vapor.22 The plasma, by impacting the surface of the PEEK material, causes the breaking of chemical bonds, the replacement of atoms and the diffusion of electrons. This results in a variety of chemical reactions. In addition, the corrosive effect of the plasma on the material also changes a range of physical properties, including increased roughness and hydrophilicity.23,24 This leads to a significant improvement in the adhesion and proliferation of osteoblasts on the PEEK surface. The effect of plasma influence on the physicochemical properties of PEEK and its biological activity has been studied by many authors. Liu et al.25 applied argon cold plasma, nitrogen cold plasma and 90% argon and 10% nitrogen mixed cold plasma to PEEK, respectively. The comparison revealed that cold plasma significantly increased the roughness, hydrophilicity, osteogenic activity, and antimicrobial properties of PEEK. The nitrogen-treated PEEK had the roughest surface and higher hydrophilicity, and hybrid cold plasma treated PEEK has the strongest antimicrobial properties. Similarly, Fu et al.26 compared the effects of hydrogen plasma treatment, oxygen plasma, 66% hydrogen and 33% oxygen plasma on PEEK. Hydrophilicity, crystallinity, microhardness and cell density on the surface were significantly increased in the plasma-treated group compared to the untreated group. Plasma treatment with oxygen and H/O proved to be the most effective, whereas H/O plasma worked ten times faster to achieve the same effects. Han et al.27 found that most previous studies utilized polished PEEK as a substrate fabricated with traditional manufacturing processes, e.g., injection molding, to analyze the effects of plasma treatment. The effect of plasma treatment on the modification of PEEK implants is still unknown. They then applied plasma to the PEEK medical implants manufactured by fused filament fabrication 3D printing technology to verify its feasibility. However, this hydrophilicity lasts for a short period of time, and functional surfaces exposed to air may be buried beneath the polymer surface, making it extremely important for the storage of implants. In addition to directly improving the physicochemical properties, osteogenic activity, and antimicrobial ability of PEEK, the plasma-treated PEEK surface is useful for coating adhesion.28
3.1.2. Laser treatment.
In recent years, laser technology has been widely used for surface modification of PEEK materials to alter the surface morphology of the implant material, to guide the behavior of osteoblasts, and to further improve bone-implant integration. Femtosecond laser technology is widely used because it is significantly better than other laser technologies in terms of stability, reproducibility, operability, precision, and thermal effects.29 By characterizing femtosecond laser-treated PEEK, Luo et al.30 found that femtosecond laser etching can increase the surface energy, the content of active sites including amorphous carbon and carbon-hydroxyl on PEEK surfaces. In vitro validation experiments demonstrated that the samples etched with a femtosecond laser had a better ability to induce apatite deposition and cell proliferation than those treated with popular sulfonation modification, which would lead to better bioactivity and osteointegration. Xie et al.31 demonstrated that after femtosecond laser treatment of the PEEK surface, the micro-grooves on the surface and the nanopore clusters due to laser energy ablation can produce a synergistic effect, enhancing the osteogenic differentiation ability of cells, and improving the bone integration ability of PEEK materials. However, the PEEK surfaces co-treated with CO2 laser and plasma showed better cell proliferation and differentiation compared to laser treatment alone. CO2 laser formed carboxyl groups as well as microgrooves containing microporous or microcratered structures on the PEEK surfaces, and the plasma treatment did not have a significant effect on the microstructural morphology produced by laser microfabrication.32
3.1.3. Sandblasting treatment.
Sandblasting is an inexpensive form of modification, a process that physically roughens the surface of PEEK materials through abrasive particles. Sandblasting removes irregular surface defects and creates uniform micro-rough surfaces that support cell attachment and may enhance the integration of PEEK implants into the host tissue.33 When subjected to the same bonding process, sandblasting can significantly increase the bonding strength of PEEK compared to the manufacturing process (milled or heat-pressed).34
3.1.4. Extreme ultraviolet.
Polymer surfaces exposure to extreme ultraviolet (EUV) radiation results in surface ablation, surface geometry changes, and modification of their chemical structure. Moreover, additional usage of the low-temperature plasma induced by the EUV beam in a gas injected into the area surrounding the irradiated polymer surface causes the incorporation of new functional groups into the polymer surface.35,36 Czwartos et al.37 found that PEEK surfaces modified by EUV radiation and two low-temperature EUV-induced oxygen and nitrogen plasmas showed the appearance of new functional groups and the incorporation of a large number of atoms.
3.1.5. Accelerated neutral atom beam.
Accelerated neutral atom beam (ANAB) impinges on the PEEK surface by directing a neutral electron beam with an average energy in the range of a few electron volts to over 100 electron volts, leaving a depth of no more than 2 and 3 nm on the surface. This modification technique with extremely weak penetration does not damage the material surface compared to plasma and EUV radiation.38 ANAB-modified PEEK surfaces have superior nanostructures and more chemical exposure, which leads to increased osteoblast proliferation and differentiation, increased protein adhesion, and reduced bacterial colonization.39
3.2. Chemical treatment
3.2.1. Sulfonation.
Sulfonation is an electrophilic substitution reaction that is more commonly performed by attaching sulfonic acid groups to the aromatic rings of the PEEK backbone structure by using concentrated sulfuric acid or sulfur trioxide (SO3). The sulfonated PEEK (SPEEK) maintains excellent mechanical properties and significantly improves surface roughness and hydrophilicity, thereby increasing its osteogenic activity and antimicrobial properties.40 In addition, the use of simple, fast, and low-cost ultraviolet (UV)-initiated graft polymerization allows the benzophenone portion of the PEEK molecular structure to produce free radical substances on its surface. Subsequently, these radical substances initiate polymerization reactions with sulfonic acid-functional monomers to produce surface SPEEK.41 The functionalized surface-modified SPEEK produced by this new approach has good cell proliferation and differentiation as well as anti-inflammatory properties.42 However, it remains to be solved how to efficiently remove the residual sulfuric acid, how to avoid the impact on the pore size during the cleaning process and how to reduce the adverse effects of the newly generated sulfonate groups on human cells.43,44 Niu et al.45 modified the surface of PEEK/nanomagnesium silicate composite material with particle impact and concentrated sulfuric acid. In the electron microscope, large holes of about 150 microns in size caused by particle impact and small holes of about 2 microns caused by sulfonation were observed. This multi-level micropores and increased hydrophilicity have a significant role in promoting the adhesion, proliferation and differentiation of mesenchymal stem cells. For SPEEK, bioactive substances such as hydroxyapatite (HA) can be easily loaded onto the material surface.46 However, the water contact angle of sulfonated PEEK alone was 107.57° ± 3.52°, which was greater than that of PEEK (91.83° ± 3.10°).47 In contrast, the hydrophilic angle can be reduced from 112.5° ± 2.5° to 20° ± 2° by attaching the HA coating to SPEEK.48 In addition to inorganic materials, natural bioactive substances can also bind well to the sulfonated surface. Ekambaram et al.49 adhered aloe vera with natural skin-like properties to electrostatically spun PEEK nanofiber scaffolds that were sulfonated and treated for two hours. This composite scaffold promotes proliferation and differentiation of keratin-forming cells and fibroblasts, and has some antibacterial properties.
Roughness and –SO3H content can be well controlled by adjusting the contact time of concentrated sulfuric acid or SO3 with the PEEK surface. Significant differences in cell adhesion, spreading, proliferation and extracellular matrix (ECM) secretion were observed for different sulfonation levels of PEEK, and excessively high sulfonation levels were detrimental to cells. A series of tests have demonstrated that PEEK scaffolds sulfonated with concentrated sulfuric acid for 30 seconds have the best mechanical properties and biocompatibility.50,51 Furthermore, for sulfur trioxide, Wang et al.52 fabricated porous surfaces of PEEK implants by using gaseous SO3 in a controlled sulfonation method for different times (5, 15, 30, 60, and 90 min) and found that PEEK sulfonated for 15 min had the highest bioactivity.
3.2.2. Acid etching.
Composites of PEEK and Ti by acid etching allow the formation of micron and nanoscale structures on the surface. These structures can significantly promote the expression of early osteogenic markers in human bone marrow mesenchymal stem cells.53 Conventional sulfonation leaves sulfur-containing functional groups on the PEEK surface that are detrimental to the cells. Nitric acid is as strong an oxidizer as sulfuric acid, and the effects of acid etching of nitric acid as well as the addition of nitrocellulose on the properties of PEEK materials still need to be further explored. Ding et al.54 successfully obtained micro–nano porous structure by surface treatment of PEEK using a 1
:
1 volume ratio of nitric acid and concentrated sulfuric acid mixture. Based on this, the PEEK surface was further aminated by Schiff base reaction between ketone carbonyl and ethylenediamine to achieve a dual modification of the PEEK surface microstructure and chemical state. This not only confers good hydrophilicity and cytocompatibility to PEEK, but also provides high antimicrobial activity. The results of cytotoxicity experiments confirm that HNO3-treated PEEK is virtually non-toxic to cells.55 Co-treatment of PEEK with hydrofluoric acid and nitric acid introduces fluoride ions to the surface. This regulates macrophage polarization and stimulates osteoblast differentiation by inhibiting NF-κB pathway down-regulating the expression of pro-inflammatory factors.56
4. Bioactive material modified PEEK
Surface modification alone has a limited effect on enhancing the biocompatibility of PEEK and has little effect on the mechanical properties of PEEK. Therefore, a variety of inorganic and organic active materials at the micron/nano scale have been used to modify PEEK. They were loaded into PEEK by physical mixing, chemical bonding and surface coating, and the resulting composites showed good properties. The mechanical strength and stiffness of PEEK can be enhanced by the addition of bioactive fillers, and the resulting PEEK-based composites can be used to obtain orthopedic implants to meet different clinical needs by selecting the appropriate filler and content. The active materials incorporated as physical blends are usually prepared by the melt processing, and the resulting PEEK-based composites are significantly better than simple PEEK in terms of mechanical properties and biocompatibility. The active materials incorporated as physical blends are usually prepared by the melt processing, and the resulting PEEK-based composites are significantly better than simple PEEK in terms of mechanical properties and biocompatibility. Among the active materials, HA and carbon fiber (CF) are the most commonly used for blending with PEEK to prepare PEEK-based composites with excellent properties. In addition, the bioactive materials contain a large number of functional groups, which can be chemically bonded with the functional groups introduced by surface modification, and closely adhere to the PEEK surface. However, this change in chemical structure may lead to inactivation of bioactive materials. For this reason, researchers mostly adhere bioactive materials in the form of coatings, which can well help the release of bioactive materials.
4.1. Inorganic materials modified PEEK
4.1.1. Carbon fiber.
Pure PEEK materials have mechanical properties close to those of human cortical bone and can meet the needs of most bone defect repairs. However, for hip prostheses, femoral stems, and other load-bearing bones, there is still a need to further improve the mechanical properties of PEEK, of which CF is widely used as a filler in reinforced polymers because of its high strength and high modulus.57 By adjusting the type, length, orientation and mass fraction of CF, CF/PEEK composites can be obtained to meet different clinical conditions. Precursors commonly used in the manufacture of commercial CF include polyacrylonitrile (PAN) and pitch. Pitch-based CF usually has a larger diameter and lower strength than PAN-based CF.58 As the CF mass fraction increases, the modulus of elasticity and wear resistance of the material gradually increases. It has been found that graphene has a coordinated effect with CF in enhancing the wear resistance and tensile strength properties of PEEK, and that PEEK modified with a 20% CF and 10% graphene blend has the best mechanical properties.59
However, excellent mechanical properties are not enough for bone repair materials, and the low bioactivity still limits their clinical application. Yamane et al.60 used concentrated sulfuric acid and oxygen plasma to act on PEEK with 50% CF content and found that the addition of CF did not affect the generation of surface voids and the introduction of sulfonic and carboxyl groups. And this surface-modified CF\PEEK composite makes it easier for apatite to be generated on its surface. Although PEEK-based CF/PEEK composites exhibit satisfactory mechanical properties, their ability to improve bioactivity is limited. To address this issue, multiple classes of bioactive materials were further introduced into CF/PEEK composites. This multi-filler co-modification approach can fully utilize the properties of different materials to meet the needs of bone repair.
4.1.2. Graphite oxide.
Graphite oxide (GO) as a two-dimensional new carbon material, has been considered as an attractive bioactive material because of its unique lamellar structure, large specific surface area, sp2 carbon domains and abundant oxygen-containing function groups (hydroxyl, carboxyl, etc.) on its surface.61 By melt- extrusion nano-GO with PEEK, complexes with excellent properties can be obtained. Composites containing 1.0 wt% GO showed significant improvements in Young's modulus (25%), tensile strength (34%), elongation at break (31%), and energy storage modulus (61%) compared to pure PEEK.62 Also, cell adhesion and biological activity were significantly improved in cell experiments with the composite.63 In addition, GO can also adhere to the sulfonated PEEK surface in the form of a coating to limit the adverse effects of sulfur-containing groups on the cells and to exert the antibacterial activity of GO.64,65
4.1.3. Hydroxyapatite.
HA has the chemical formula Ca10(PO4)6(OH) and is the main component present in biological hard body tissues. Synthetic HA has similar chemical properties to inorganic components in the human body, so it shows a strong affinity for hard tissues. Therefore, synthetic HA has been widely used as a biomaterial for orthopedic and dental applications for the repair or replacement of hard tissues.66 70% of natural bone consists of nanocrystalline HA that is 20–80
nm long and 2–5
nm wide. However, the size of synthetic HA currently produced commercially is larger than the size of natural HA. Nanophase HA (nHA) is preferred over microphase HA because of its larger surface area, higher mechanical properties and better biocompatibility than microphase HA.67,68
The maximum values of tensile, compressive, flexural, and impact strengths of the composites obtained after blending nHA with PEEK at different mass fractions increased by about 16.2, 25, 54, and 21%, respectively, compared to pure PEEK69 with an increase in HA content from 10 wt% to 30 wt%, the elastic modulus of the composite increased from 2.36 GPa to 2.79 GPa, tensile strength decreased from 95 MPa to 74 MPa, and fracture elongation decreased from 63% to 23%, presenting brittle fracture failure. When the dispersion of particles was uniform, the elastic modulus was less affected by diameter variation, but the modulus anisotropic coefficient was greatly affected by the composition ratio, particle diameter, and dispersity.70 In addition to the excellent mechanical properties, in vivo and in vitro experiments have shown that the composite is non-cytotoxic and has higher osteogenicity and cell adhesion than pure PEEK materials.71 Sun et al.72 found that Ca2+ released from HA could activate the adhesion proteins FAK upon entry into the cell by increasing the level of HA the intracellular Ca2+ concentration was increased, thus promoting the expression of adhesion proteins/genes. For the fused filament fabrication (FFF) fabricated PEEK/HA scaffolds, HA particles are evenly distributed throughout the bulk and across the surface of the native 3D printed samples. By varying the pore size and HA content of the scaffold, the elastic modulus of the PEEK/HA scaffold can be widely adjusted in the range of 624.7–50.6 MPa.73,74 Limited by the high brittleness of ceramic materials, PEEK-based HA/PEEK composites, in spite of their good biological properties, still have a detrimental effect on the mechanical properties that cannot be ignored. Manzoor et al.75 found that although the ultimate tensile strength (∼14%) and Young's modulus (∼5%) of PEEK/HA were slightly reduced compared to pure PEEK, no significant difference was found with the addition of the inorganic phase to the polymer matrix. The composites after blending nHA with PEEK, although possessing excellent mechanical properties, nHA encapsulated inside the material is difficult to maximize the bone-enhancing effect. For this reason, zheng et al.76 constructed a degradable PLGA/nHA/alendronate(ALN) hybridized coating on the PEEK surface. The composites showed good biocompatibility and osteogenic ability, and the drugs could be released continuously for up to 98 days with the degradation of PLGA. Considering the different stages of bone repair, they subsequently grafted interleukin-4 to the outer surface of the hybrid coating. The early burst of interleukin-4 released by the hybrid coating switched peri-implant macrophages from a pro-inflammatory M1 phenotype to an anti-inflammatory M2 phenotype, thereby creating a favorable bone immunomodulatory microenvironment. Subsequently, slow and sustained release of from ALN and Ca2+ over the next few weeks inhibits excessive bone resorption and enhances bone formation to restore peri-implant bone homeostasis and promote bone implant integration in osteoporotic conditions.77 This strategy for constructing advanced bone implants with continuous function provides customizable and clinically viable therapies for patients with osteoporosis. Additionally, the biological properties of HA can be enhanced by doping it with other elements such as strontium (Sr), silicon (Si), magnesium (Mg) and zinc (Zn) for bone regeneration and biomineralization.78
4.1.4. Metals and their oxides.
Metal ions, such as Ag+, Zn2+ and Gu2+, show significant antimicrobial ability.79,80 Among them, silver in various structural forms has been the longest used and most widely used antimicrobial agent due to its lower price, industrial mass production and strong antimicrobial properties.81 However, how to get the metal ions to bind to PEEK stably and to control its slow release is the main problem that we are currently facing. For this reason, Lee et al.82 found that epoxy resin easily diffuses into PEKK to form a semi-interpenetrating polymer network, so silver nanoparticles (AgNPs) were doped into epoxy resin and then physically cross-linked with PEEK. AgNPs was uniformly distributed in the epoxy resin, and the roughness of the sample surface decreased with the increase of Ag concentration. An inhibition zone of 22.5 mm and an antibacterial rate of 83.47% were found for the PEKK coated with 0.5% AgNPs compared to an untreated PEEK substrate. In addition to the conventional surface grafting by coating, Siegel et al.83 anchored AgNPs to the PEEK surface in a purely physical form by laser-induced forward transfer technique. During directed movement of AgNPs, which is caused by the forward scattering force arising from the isotropic scattering of incoming electromagnetic radiation on spherical particles with sufficiently small diameter, the light absorption occurs at wavelengths close to the resonant conditions of metallic particles, leading to their simultaneous heating. Once the glass transition temperature of the semicrystalline polymer is overcome, nanoparticles are pushed into the polymer surface, creating a composite with considerably high concentration of particles in a close vicinity of the surface. In addition, metal–organic skeletal materials with large surface areas, extraordinary porosity and designable crystal structures are well suited to incorporate synergistic bacterial agents into their pores by linking inorganic metal ions or metal clusters with rigid organic ligands. Based on this, Yang et al.84 added AgNPs to a zeolitic imidazolate framework-8 (ZIF-8) with a tetrahedral structure formed by Zn2+ and imidazolate linkers and then combined with SPEEK to obtain composites with antimicrobial properties. This study provides a new idea for combining PEEK with Ag+ on the one hand, and demonstrates the synergistic effect of Ag and Zn in antibacterial properties on the other. Although Ag+ has excellent antibacterial properties, the toxicity of high concentrations of Ag+ to cells is something we cannot ignore.
In addition, among many antimicrobial metal ions, Zn is of wide interest because it also has excellent osteogenic properties. On the one hand, Zn can have good antibacterial potential through the production of reactive oxygen species (ROS) and its destructive interactions with bacterial membranes. On the other hand, Zn promotes bone formation by promoting alkaline phosphatase (ALP) activity, ECM mineralization and osteogenesis-related gene expression.85 In addition, it has been demonstrated that the addition of ZnO significantly enhances the anti-wear properties of PEEK and reduces polymer wear particle-induced inflammation and osteolysis by modulating the MEK-ERK-COX-2 axis.86,87 Sr is also an important trace element in the body and plays a dual role in bone metabolism, inhibiting bone resorption and stimulating bone formation. Sr+-modified PEEK has been shown to have multiple functions in osteogenesis, anti-inflammation and angiogenesis.88 In addition, Wang et al.89 found that the Zn–Sr–SPEEK implant demonstrated a strong ability to restore dynamic mitochondrial imbalance and dysfunction induced by hyperglycemia through downregulation of dynamin-related protein 1 (Drp1) gene, restoration of mitochondrial membrane potential (MMP) and elimination of ROS, ultimately enhancing osteogenicity of osteoblasts. This provides us with a solution to the problem of high complications and high failure rate of implantation due to mitochondrial dysfunction in hyperglycemia.
Other particles such as Ti,90,91 titanium dioxide,92 tantalum,93–95 tantalum pentoxide96 and niobium pentoxide97 can not only increase the hydrophilicity, surface energy, and surface roughness of PEEK materials, but also promote bone formation. Molybdenum disulfide and zirconium oxide can be used to increase the anti-wear properties of PEEK materials.98–100 In contrast, PEEK composites loaded with Mg101,102 and nickel103 have been reported to have significant pro-angiogenic ability. Table 2 summarizes the studies related to the modification of PEEK with inorganic materials.
Table 2 Inorganic material modified PEEK
Materials |
|
Physicochemical characterization |
In vivo and in vitro experiments |
Ref. |
CF, Carbon fiber; GO, Graphene oxide; GONPs, graphene oxide nanoplatelets; nHA, Nano hydroxyapatite; AgNPs, Silver nanoparticles; ZIF-8, zeolitic imidazolate framework-8; Gu, Copper; Ti, Titanium; Ta, Tantalum; Zn, Zinc; ZnO, Zinc oxide; TiO2, Titanium dioxide; Ta2O5, Tantalum pentoxide; Nb2O5, Niobium pentoxide; MoS2, molybdenum disulfide; Mg, Magnesium. ALN, Alendronate. |
CF |
CF |
Enhanced hydrophilicity and HA nucleation. |
Not available. |
60
|
CF + Graphite |
The coefficient of friction increased and the lowest coefficient of friction was found for 10% CFs + 20% graphite. |
Not available. |
59
|
GO |
GONPs |
Significant increase in storage modulus, tensile strength, Young's modulus and elongation at break. |
Not available |
62
|
GONPs |
Increased roughness and hydrophilicity. |
Improved cell adhesion and proliferation. |
63
|
GONPs |
Increased roughness and hydrophilicity. |
Promotes cell adhesion and proliferation with good antimicrobial properties. |
65
|
GONPs |
Formation of GO wrinkles on the surface and up-regulation of surface hydrophilicity. |
Enhanced bioactivity, osteointegration and antibacterial activity. |
64
|
HA |
nHA |
Uniform distribution of HA energy and increased mechanical properties. |
Increase cell proliferation, adhesion and osteogenic activity. |
69
|
nHA |
The more uniform the HA particle distribution, the higher the mechanical properties. |
Not available. |
70
|
nHA |
Increased mechanical properties, increased hydrophilicity. |
Increase cell proliferation, adhesion and osteogenic activity. |
71
|
nHA |
Increased mechanical properties. |
Aids in adhesion to soft tissues. |
72
|
nHA |
Increased crystallinity and mechanical strength. |
Not available. |
73
|
nHA |
The mechanical strength is similar to that of natural cancellous bone. |
Promotes cell attachment and mineralization. |
74
|
nHA + ALN |
Uniform distribution and reduced hydrophilic angle. |
Increase cell proliferation, adhesion and osteogenic activity. |
76
|
nHA + ALN + IL-4 |
Stable drug release with reduced hydrophilic angle. |
Modulates inflammatory response and promotes bone repair. |
77
|
nHA + Sr/Zn |
Slight reduction in mechanical strength. |
Increased formation of surface HA. |
78
|
Metals |
AgNPs |
AgNPs uniformly deposited on PEEK surface. |
Increased antimicrobial resistance. |
82
|
AgNPs |
AgNPs uniformly deposited on PEEK surface. |
Increased antimicrobial resistance. |
83
|
Ag + ZIF-8 |
Increased roughness and hydrophilicity. |
Ag and Zn possess synergistic antibacterial ability. |
84
|
Gu + Ti |
Excellent mechanical properties. |
Biocompatibility, osteogenic activity, increased angiogenesis. |
79
|
Gu + Ta |
No significant change in PEEK surface topography and roughness at the micron level. |
Increased cytocompatibility, osteogenic activity, antibacterial properties. |
80
|
Zn |
Enhanced hydrophilicity and effective loading and release of Zn2+. |
Increased osteogenic activity and antimicrobial activity. |
85
|
ZnO |
Increased resistance to pressure and abrasion. |
Good antibacterial properties. |
86
|
ZnO |
Not available. |
Attenuates polymer particle-induced inflammation and inflammatory osteolysis. |
87
|
Sr |
Not available. |
Promotes cell proliferation and differentiation and angiogenesis, inhibits inflammatory response. |
88
|
ZnO + Sr |
Not available. |
Increased osteogenic activity and antimicrobial activity. |
89
|
Ti |
Not available. |
Increased osteogenic activity and tighter bone apposition. |
90
|
Ti |
Not available. |
Increased biocompatibility and osteogenic activity. |
91
|
TiO2 |
Not available. |
Improved osseointegration strength. |
92
|
Ta |
Excellent mechanical properties, high surface energy. |
Accelerated osteogenesis and integration. |
93
|
Ta |
Enhanced physical and chemical properties. |
Good biocompatibility and pro-cell adhesion. |
94
|
Ta2O5 |
Increased roughness, hydrophilicity, surface energy and protein adsorption. |
Cell adhesion, proliferation, and increased osteogenesis. |
96
|
Nb2O5 |
Increased hydrophilicity, surface energy, surface roughness and protein absorption. |
Cell adhesion, proliferation, and increased osteogenesis. |
97
|
MoS2 |
Increased mechanical properties, hydrophilicity, roughness, abrasion resistance. |
Good biocompatibility. |
98
|
Mg |
Increased hydrophilicity. |
Promotes cell adhesion, proliferation and differentiation; promotes angiogenesis. |
101
|
Mg |
Not available. |
Upregulation contributes to bone macrophage polarization. |
102
|
Ni |
Not available. |
Pro-angiogenesis, cell proliferation, mineralization. |
103
|
4.2. Organic materials modified PEEK
4.2.1. Growth factors.
Various growth factors have positive effects on bone tissue formation, among which bone morphogenetic protein 2 (BMP-2) is the most promising bone growth stimulating factor.BMP-2 is a member of the transforming growth factor β superfamily, which induces differentiation of mesenchymal stem cells into osteoblasts, increases the activity and proliferation of osteoblasts at the site of bone defects, and promotes bone reconstruction and regeneration.104 Most of these growth factors are immobilized to the PEEK surface by chemical bonding or physical electrostatic adsorption. However, BMP-2 will inevitably deactivate during this process, so selecting a suitable loading method with sustained release capability is the first consideration when using them for modifying PEEK. Senatov et al.105 placed BMP-2 with PEEK/HA in a buffer followed by mechanical shaking and washing to physically adsorb BMP-2 to the PEEK surface. This approach resulted in a 98.6% percentage of protein binding to PEEK and the release of approximately 12% of the bound protein in the first 10 days. Subsequent ex vivo experiments confirmed the excellent osteogenic properties of this new implant. The sulfonated PEEK surface has surface micropores, charged sulfonic acid groups, and a microacidic environment all favoring the adhesion of BMP-2.106 In addition, degradable polymers such as (gelatin, poly (lactic-co-glycolic acid), etc.) are bonded to the PEEK surface in the form of a coating on BMP-2 to provide a functional coating with time-release capability.107,108 Liu et al.109 compared two modes of BMP-2 loading with covalent binding versus physical adsorption (electrostatic interaction). The results showed that, the loading efficiency was 79.24% for covalent immobilization and 72.36% for rhBMP-2 by heparin physisorption, with significantly lower water contact angles to 77.8° ± 2.2° and 58.0° ± 1.5°, respectively. rhBMP-2 physisorption was significantly higher than covalent binding in terms of osteogenic capacity.
In addition to BMP-2, researchers have explored other growth factors. Wang et al.110 addressed the problem of difficult integration of PEEK implants into soft tissue by loading PEEK with basic fibroblast growth factor, which aids mitosis and promotes vascular and wound healing. The platelet-derived growth factor BB can be used to promote chondrogenesis. Chitosan/mesoporous silica nanoparticles composite microspheres prepared by microfluidic method were used as a bifactorial carrier for sequential release of platelet-derived growth factor BB and kartogenin. By integrating SPEEK, a porous composite scaffold was constructed and promoted cell migration in vitro, enhanced chondrogenic differentiation of BMSCs, and promoted cartilage regeneration in vivo.111 Wang et al.112 found that Adiponectin, the most abundantly secreted by adipocytes, exhibited better osteogenic activity than BMP-2 in the presence of osteogenic inducers, and adhered Adiponectin with Sr to the surface of PEEK via dopamine. The results showed that Adiponectin with Sr increased cell adhesion, proliferation, ALP activity, formation of mineralized calcium nodules and expression levels of osteogenesis-related genes, and that there was a coordinated effect.
4.2.2. Bioactive peptides modified PEEK and its composites.
Proteins are limited in their application in implants due to their short half-life, low stability and high immunogenicity. Short amino acid sequences derived from the active structural domains of proteins have shown strong potential for application due to their lower cost, ease of synthesis, and protein-like function.113
An osteoinductive oligopeptide called bone-forming peptide (BFP, in a sequence of GQGFSYPYKAVFSTQ) originated from bone morphogenetic protein-7 (BMP-7) was proved to have excellent osteogenic activity and bone formation activity, even better than BMP-7.114 Yu et al.115 formed a carboxymethyl chitosan film on the PEEK surface by spin coating with the aim of controlling the release of silver ions on the surface. Meanwhile, BFP was modified onto the PEEK surface by 1-(3-dimethylaminopropyl)-3-ethylcarbodiimide hydrochloride/N-hydroxy succinimide. This functional material has both antimicrobial and osteogenic activity. Similarly, Wang et al.116 prepared the same multifunctional implant material with GO, a 2D nanocoating on the surface of PEEK formed by polydopamine to provide photodisinfection, and BFP to provide osteogenic activity. In addition, osteogenic growth peptide (OGP), which also has osteogenic activity, is a naturally occurring tetrapeptide with the same C-terminal amino acid sequence as histone H4. OGP can regulate osteogenic differentiation of bone marrow mesenchymal stem cells in its free or bound state and further promote stromal mineralization.117
AMP is a peptide with antimicrobial activity, an important component of innate immunity to pathogens in most organisms, with excellent broad-spectrum antimicrobial activity and low biotoxicity. AMP can bind to negatively charged bacterial cell membranes through nonspecific electrostatic interactions, leading to cell death by disrupting the cell membrane.118 This antibacterial mechanism makes it difficult for bacteria to develop drug resistance. HHC36 (KRWWKWWRR) is a highly effective AMP containing nine amino acids predicted and designed by the artificial neural network. It has high antibacterial activity against a variety of multi-resistant “superbugs,” such as MRSA. It exhibits better antibacterial properties than conventional antibiotics (tobramycin, ciprofloxacin, imipenem, ceftazidime) and clinical candidate AMPs (such as MX226 and hLF1-11). HHC36 was successfully loaded onto the SPEEK surface by a simple solvent evaporation method. The sample had excellent antibacterial properties and good cytocompatibility, which significantly reduced bacterial survival and inflammatory response in vivo.119 Small molecule antimicrobial peptides with the sequence Arg(R)-Trp(W)-Arg(R)-Trp(W)-Arg(R)-Trp(W) and beta-defensin-14 have also been shown to enhance the antimicrobial properties of PEEK.120,121 In addition to its broad-spectrum antimicrobial effects, KR-12 (Ac-KRIVQRIKDFLR-NH2), the active unit derived from the antimicrobial peptide LL-37, immobilizes the antimicrobial peptide KR-12 on the surface of a PEEK implant with the help of polydopamine (PDA), which inhibits bacterial infections and promotes osteogenic differentiation through activation of BMP/SMAD signaling pathway.122 However, for implants a single osteogenic activity and antimicrobial properties are difficult to meet the requirements of patients. Li et al.123 utilized a mussel foot protein mimetic peptide with clickable stacked ends to bio-orthogonally click antimicrobial peptide (AMP) and OGP onto stacked modified PEEK biomaterials. To enable PEEK to obtain dual effects of host defense and tissue repair.
In addition to various direct osteogenic peptides, some peptides aid in osteogenesis by promoting cell adhesion. The tripeptide Arg-Gly-Asp (RGD), derived from the ECM protein fibronectin, can mediate cell adhesion by acting as a binding module for integrin receptors, and RGD can be coupled to PEEK surface via polydopamine at controlled density and orientation while retaining its biological activity.124 However, it is difficult to adhere RGD to the PEEK surface by conventional means. It was found that the carbonyl group in benzophenone can effectively reduce the hydroxyl group on the PEEK surface, and the cell adhesion peptide Gly-Arg-Gly-Asp can be stably immobilized by further silylation.125 Nonapeptide of human vitronectin (HVP), can promote osteoblast adhesion machinery mediated by cell surface glycosaminoglycans. Cassari et al.126 covalently bound this adhesion peptide to the PEEK surface. The presence of the HVP peptide doubled the adhesion of the cantilever tip compared to PEEK alone, while the reverse dimer of HVP increased the adhesion twofold compared to the control. This may be due to the longer sequence of the inverse dimer compared to HVP.
4.2.3. Polysaccharide and matrix protein modified PEEK.
In recent years, some researchers have added polysaccharides and matrix proteins to polymers to mimic natural extracellular matrices and improve the biocompatibility of PEEK. Type I collagen is one of the main components of the bone matrix. It is evident for the adhesion and proliferation of osteoblasts, and fibrin is also used to determine the orientation of apatite and the mechanical strength of bone. In addition, collagen stimulates the proliferation of vascular endothelial cells, synergistically promoting bone repair by coupling osteogenesisand angiogenesis.127 In addition collagen also aids in the adsorption of basement membrane proteins secreted by epithelial cells, allowing PEEK implants to bind tightly to the tissue to aid in osteogenesis.128 However, PEEK is chemically inert, so tethering collagen to the PEEK surface requires a multi-step process. Kim et al.129 first placed PEEK in a mixture of sulfuric and nitric acids used to prepare a rough surface, followed by adhesion of PDA to the surface-modified PEEK, followed by chemical bonding of type I collagen to PDA. Rapid attachment and proliferation of cells on the composite due to specific recognition between osteoblast membrane integrins and the RGD sequence of immobilized collagen. Arisaka et al.130 used ultraviolet light to irradiate the PEEK surface to generate free radicals in the benzophenone units of the PEEK molecular chain, which triggered photopolymerization. This method can tether type I collagen to the PEEK surface without the need for additional initiators, condensation reagents, or surface activation. In addition, gelatin is a product of collagen hydrolysis, which has excellent biocompatibility with collagen and is superior to collagen in terms of cost and immunogenicity. Gelatin-coated plasma-treated PEEK surfaces exhibit high hydrophilic and cell adhesion and proliferation promoting properties.131
In addition to matrix proteins, polysaccharides are also important components of the extracellular matrix. Among them, hyaluronic acid is as the most acidic mucopolysaccharide that has been used to modify PEEK and is widely present in connective tissue. It is involved in many natural processes, such as biological signaling, wound repair, and morphogenesis.132 However, its low residence time and rapid degradability limit its applicability. Photocrosslinking of hyaluronic acid derivatives such as methacrylated hyaluronic acid can be considered as one of the possible strategies to immobilize hyaluronic acid on biomedical implants and produce hydrophilic, lubricious and biocompatible surfaces.133 Methacrylating hyaluronic acid can be cross-linked on PEEK scaffolds by ultraviolet light can improve the mechanical properties, osteogenic differentiation and ECM mineralization of the scaffold.134 Liu et al.135 first prepared photoinduced electrospun TiO2 that mimics the topology of cortical bone fibers and used it to fabricate composites with PEEK through the stable chemical bonding of hyaluronic acid methacrylate. This one-step composite has a surface rich in nanofibers with the roughness of homologous bone and is well suited to promote cell adhesion, proliferation and differentiation in vitro. In addition to its direct bone-enabling effects, hyaluronic acid can also be used for drug delivery. Liu et al.136 doped LAPONITE®, which can be used to attenuate glucocorticoid-induced implant loosening, into a deposition system consisting of hyaluronic acid and chitosan, which was subsequently encapsulated on a PEEK surface to help prevent implant failure in glucocorticoid-treated patients. Zheng et al.137 chemically bonded PEEK with another natural glycosaminoglycan (chondroitin sulfate) by using ethylenediamine as an intercalating reactant. The results showed that the introduction of chondroitin sulfate increased the angiogenic, anti-inflammatory, and osteogenic capacity of PEEK. Table 3 summarizes the studies related to the modification of PEEK with organic materials.
Table 3 Organic material modified PEEK
Biomolecules |
|
Load method |
In vivo and in vitro experiments |
Ref. |
Abbreviation: BMP, Bone morphogenetic protein; ALP, Alkaline phosphatase; PDA, polydopamine; bFGF, Basic fibroblast growth factor; PDGF-BB, Platelet-derived growth factor BB; EDC/NHS, 1-(3-dimethylaminopropyl)-3-ethylcarbonimide hydrochloride/N-hydroxy succinimide; OGP, Osteogenic growth peptide; AMP, Antimicrobial peptide; RGD, Tripeptide Arg-Gly-Asp; UV, Ultraviolet; PLGA, Poly (lactic acid)-glycolic acid. |
Growth factors |
BMP |
Physical adsorption |
There was a significant increase in the amount of bone tissue in the implanted area and an increase in the value of bone volume/tissue volume on micro CT. |
105
|
BMP |
Physical adsorption |
Enhanced cell adhesion and spreading, improved collagen secretion, extracellular matrix mineralization and ALP activity. |
106
|
BMP |
Gelatin covalent binding |
Significantly promotes cell adhesion, proliferation and osteogenic differentiation. |
107
|
BMP |
PLGA microsphere encapsulation |
Significantly promotes cell adhesion, proliferation and osteogenic differentiation. |
108
|
BMP |
Covalent fixation and heparin binding |
Exhibits good cytocompatibility and promotes cell differentiation. |
109
|
bFGF |
PDA coating |
Promotes cell proliferation, adhesion and migration and exhibits good biocompatibility. Exhibits excellent performance in improving the growth and adhesion of surrounding soft tissues |
110
|
PDGF-BB |
Chitosan/silica nanoparticles composite microsphere loading |
It has good biocompatibility, promotes cell migration, enhances cartilage differentiation of BMSCs in vitro and promotes cartilage regeneration in vivo. |
111
|
Adiponectin |
PDA coating |
Significant increase in cell proliferation and differentiation. |
112
|
Bioactive peptides |
BFP-1 |
EDC/NHS coupling |
It has good inhibitory effect on Gram-negative and Gram-positive bacteria. And it has better biological activity to promote cell proliferation and osteogenic differentiation. |
105
|
BFP-1 |
GONPs and PDA load |
Produces a synergistic photothermal/photodynamic treatment effect that produces a powerful antimicrobial phototherapy effect. |
116
|
OGP |
Covalent bonding |
Promotes cell attachment, proliferation, ALP activity and, mineralization and new bone formation. |
117
|
HHC36 |
Physical adsorption |
No significant effect on cell proliferation and viability. Reduced bacterial survival on the sample surface and inflammatory response in the soft tissue surrounding the sample. |
119
|
Beta-defensin-14 |
Physical adsorption |
Excellent osteointegration and durable broad-spectrum antimicrobial activity. |
120
|
AMP |
Physical adsorption |
Better inhibition of bacteria, upregulation of osteogenesis-related gene/protein expression, and accelerated osseointegration at the implant bone interface. |
121
|
KR-12 |
PDA coating |
Excellent antibacterial activity, better adhesion, proliferation, osteogenic differentiation and osseointegration. |
122
|
AMP + OGP |
Mussel foot protein mimetic peptide loading |
Inhibits bacterial growth, stabilizes bone homeostasis and promotes bone regeneration at the interface. |
123
|
RGD |
PDA coating |
Promote cell proliferation, osteogenic differentiation, and significantly improve HA formation. |
124
|
GRGD |
Silanized coating |
Promote cell proliferation, adhesion and differentiation. |
125
|
ECM |
Type I collagen |
EDC/NHS coupling |
Enhanced adsorption to basement membrane proteins, enhanced cell viability and diffusion. |
128
|
Type I collagen |
PDA coating |
Biocompatibility is improved and cell proliferation and differentiation are promoted. |
129
|
Type I collagen |
Chemical bonding |
Increased cell adhesion and proliferation, increased expression of osteogenic genes and mineralization. |
130
|
Gelatin |
Physical adsorption |
Higher hydrophilicity and biocompatibility to promote cell proliferation. |
131
|
Hyaluronic acid |
Ultraviolet light crosslinking |
Promotes cell adhesion and proliferation, contributing to osteogenic differentiation and extracellular matrix mineralization. |
134
|
Hyaluronic acid |
Photo-induced polymerization under UV light irradiation |
Enhanced cell adhesion, proliferation and osteogenic differentiation. |
1135 |
Hyaluronic acid |
Physical adsorption |
Enhanced cell viability, adhesion and osteogenic differentiation, and osseointegration in rats in vivo. |
136
|
Chondroitin sulfate |
Chemical bonding |
Significantly promotes cell adhesion and spreading, increases osteogenic and angiogenic activity, and reduces inflammatory responses. |
137
|
5. Antibiotic modified PEEK
For PEEK implants, infection is the main cause of implant failure,138 and Staphylococcus aureus is the most common pathogen after orthopedic surgery, followed by Escherichia coli,139 so it is essential to enhance the antimicrobial activity of PEEK implants. When treating bone infections by systemic intravenous antimicrobials, a series of problems will occur due to the lack of vascularity in the area of the bone defect. Previously, we described that sulfonation, metals and their oxides, GO and antimicrobial peptides can enhance the antimicrobial activity of PEEK. In addition, antibacterial activity can be achieved by direct addition of antibiotics. Table 4 summarizes the studies related to antibiotic-modified PEEK.
Table 4 Antibiotic modified PEEK
Category |
Load method |
Antibacterial activity |
Osteogenic activity |
Ref |
GS, Gentamicin; Van, Vancomycin; HA, Hydroxyapatite |
GS |
PDA coating |
Continuous antibacterial power. |
It promotes ALP secretion, enhances cell proliferation and new bone formation in vivo. |
141
|
GS + Ag + Silk fibroin |
PDA coating |
Ag and GS exhibited synergistic antibacterial ability. |
Improved cell adhesion, proliferation and osteogenic capacity. |
142
|
GS + Calcium phosphate |
Layer-by-layer |
Excellent sustained antimicrobial performance. |
Acceptable biocompatibility and pro-osteogenic differentiation of cells |
143
|
Tannic acid + GS + Pluronic F127 |
Layer-by-layer |
Exhibited sustained antibacterial activity. |
Enhanced osteoblast differentiation, angiogenesis and osseointegration. |
144
|
Van gelatin nanoparticles |
PDA coating |
Significantly improves antibacterial performance against Staphylococcus aureus and Streptococcus pyogenes. |
Non-cytotoxic and interacts well with osteoblasts. |
145
|
Phage + Van |
Biofilm |
Van and staphylococcal phages were able to reduce the total bacterial load independently and both had a synergistic effect. |
Not available. |
146
|
ZnO + Van |
Physical adsorption |
Exhibited sustained antibacterial activity. |
Not available. |
147
|
Cefuroxime sodium salt + HA |
Physical adsorption |
Adequate antibacterial effect against Staphylococcus aureus |
Not available. |
148
|
Cyclosporine |
Chitosan/phospholipid-sterol, peptide hybrid coating |
Stable release of cyclosporine inhibits bacterial growth. |
HA formation, albumin adsorption, and increased osteogenesis in vitro. |
149
|
Minocycline + Dexamethasone |
PDA coating |
Certain antibacterial properties. |
Promotes osteogenic differentiation and osseointegration, good cytocompatibility. |
150
|
Gentamicin (GS) is a broad-spectrum antibiotic with low toxicity and few side effects that can be used to treat common pathogens in the skeletal system. GS-modified implants can treat defects in bone infections with considerable osseointegration.140 GS exhibited good antimicrobial properties when adhered to the PEEK surface via PDA. SPEEK-PDA-GS showed almost 100% inhibition of S. aureus and E. coli on the first day. In contrast, untreated PEEK and sulfonated PEEK had almost no antimicrobial ability. SPEEK-PDA-GS lost its antimicrobial activity on the fourth day.141 However, Yan et al.142 reported that GS doping alone did not significantly improve the antibacterial (release killing and anti-adhesive) activity during the first 24 h. The synergistic effect between AgNPs and GS was greatly enhanced by the simultaneous doping of AgNPs and GS. Potential mechanisms for the synergistic effect between AgNPs and GS include increased bacterial membrane permeability, promotion of cellular ROS production and damage to DNA and proteins. Ag and GS exhibit pH-responsive release behavior, as faster and greater amounts of Ag and GS release are achieved at lower pH conditions. pH is a relevant stimulus for antimicrobial coatings, as many bacteria metabolically acidify their local environment. Layer-by-layer (LBL), an efficient modification method for implants, performs on material surface through the assembly procedure using electrostatic force, hydrogen bonding, and other means. By depositing GS with calcium phosphate143 and tannic acid144 on the PEEK surface by LBL, composites with both antimicrobial activity and excellent osseointegration properties can be obtained.
Vancomycin (Van) is a glycopeptide antibiotic that inhibits the synthesis of peptides and phospholipids in bacterial cell walls and interferes with cell wall formation, thereby inhibiting bacterial growth and reproduction. Van showed no cytotoxicity after loading on PEEK via PDA and possesses good osteogenic activity and antibacterial properties.145 However, Van alone may lead to resistance, and to reduce the concentration of Van on the PEEK surface, combined Van loading on the PEEK surface with bacteriophages lacking cross-resistance to antibiotics synergistically reduces live bacteria in static and dynamic biofilms, even at subtherapeutic vancomycin levels (i.e., 33.75 μg mL−1).146 To address the problem of difficulty in the attachment of antibiotic agents to PEEK surfaces lacking functional groups such as hydroxyl, carboxyl and amines, Chen et al.147 used a chemical synthesis method to grow ZnO nanorod arrays as carriers of antibiotic agents on PEEK substrates. Van possessed a stable drug release profile and resulted in higher concentrations of antibiotic agents in buffer solutions than the, minimum inhibitory concentration 90% value of Staphylococcus aureus within 96 h. The bioactivity activity of Van loaded on ZnO/PEEK substrates was maintained for at least 28 days. Van possessed a stable drug release profile and resulted in higher concentrations of antibiotic agents in buffer solutions than the MIC 90 of Staphylococcus aureus within 96 h. AbouAitah et al.148 obtained an antimicrobial coating with stable release by coating HA and cefuroxime sodium salt by simple ultrasonic coating. The absorption of cephalosporin antibiotics up to 1 mg cm−2 was achieved. An initial burst of 96% to 24% of the drug was released within 86 h, followed by a linear, stable release. Cefuroxime sodium salt coating had sufficient antibacterial effect against S. aureus. In addition, cyclosporine149 and minocycline150 can also improve their antibacterial and osteogenic abilities by binding to PEEK.
6. Summary and prospect
Among the many implants used for bone defect repair, PEEK, as an FDA-approved implant material, is widely used due to its mechanical properties close to those of human bone, stable chemical properties, high processability, and permeability. However, its high biological inertness and surface hydrophobicity can hinder the attachment of proteins and cells, which is detrimental to tissue repair. For this reason, various surface modifications and modification methods based on the addition of various bioactive substances have been widely studied. These modification methods can greatly improve the physical and chemical properties of PEEK, biocompatibility and antimicrobial properties, but at the same time, some of the modification methods also have obvious shortcomings. By using a combination of various modification methods, the unfavorable effects can be well mitigated.
With the in-depth research on PEEK modification, the research on improving the performance of PEEK has gone from simple optimization of mechanical properties, enhancement of biocompatibility, promotion of cell proliferation and differentiation to postoperative bacteriostasis and immunomodulation. However, bone defect repair is a dynamic process, and the requirements for PEEK composites are different for different stages of repair. How to give the composites multifunctionality to match this dynamic process should be the next priority for researchers to consider. Moreover, as researchers use various kinds of drugs on PEEK surfaces, it is necessary to establish an ideal drug delivery system. Burst release or non-release of the drug may even have a detrimental effect on bone repair. Researchers need more in-depth design and exploration to find the best modification. From the material science point of view, due to the chemical stability of PEEK, the implanted composite will exist in the defect site for a long time, which will hinder the growth of new bone tissue to some extent. Some researchers have proposed that degradable polymers can be combined with PEEK to improve its degradability, and the composites can be prepared by combining the excellent mechanical properties of PEEK with the degradability and high biocompatibility of degradable polymers. This novel PEEK-based composite still needs further research to verify its scientific validity at this time, but it provides us with new ideas. Finally, personalized implants for bone defects due to different reasons are an important trend in future medicine. Although PEEK implants with different shapes and physicochemical properties can be achieved by adjusting various parameters of multiple 3D printing methods, it is still very difficult to determine the parameters for personalized printing.
Author contributions
Huagui Huang: conceptualization, investigation, funding acquisition, writing – original draft. Xin Liu: investigation, writing. Jinzuo Wang: investigation. Moran Suo: investigation. Jing Zhang: investigation. Tianze Sun: writing – review & editing. Honghua Wang: supervision, writing – review & editing. Chengde Liu: writing – review & editing. Zhonghai Li: supervision, funding acquisition, writing – review & editing.
Conflicts of interest
The authors declare no competing financial interest.
Acknowledgements
This study was supported by the Science and Technology Innovation Foundation of Dalian (2022JJ12SN045), the Natural Science Foundation of Liaoning Province (2022-MS-322) and the Medical-Industrial Joint Innovation Funding Project of First Hospital of Dalian Medical University and Dalian Institute of Chemical Physics (DMU-1&DICP UN202311). The funders had no role in the study design, data collection and analysis, decision to publish, or preparation of the manuscript.
References
- S. Chang, S. Wang, Z. Liu and X. Wang, Advances of Stimulus-Responsive Hydrogels for Bone Defects Repair in Tissue Engineering, Gels, 2022, 8(6), 389 CrossRef CAS PubMed.
- N. Xue, X. Ding, R. Huang, R. Jiang, H. Huang, X. Pan, W. Min, J. Chen, J. A. Duan, P. Liu and Y. Wang, Bone Tissue Engineering in the Treatment of Bone Defects, Pharmaceuticals, 2022, 15(7), 879 CrossRef CAS PubMed.
- D. Tang, R. S. Tare, L. Y. Yang, D. F. Williams, K. L. Ou and R. O. Oreffo, Biofabrication of bone tissue: approaches, challenges and translation for bone regeneration, Biomaterials, 2016, 83, 363–382 CrossRef CAS PubMed.
- V. Campana, G. Milano, E. Pagano, M. Barba, C. Cicione, G. Salonna, W. Lattanzi and G. Logroscino, Bone substitutes in orthopaedic surgery: from basic science to clinical practice, J. Mater. Sci.: Mater. Med., 2014, 25(10), 2445–2461 CrossRef CAS PubMed.
- P. Baldwin, D. J. Li, D. A. Auston, H. S. Mir, R. S. Yoon and K. J. Koval, Autograft, Allograft, and Bone Graft Substitutes: Clinical Evidence and Indications for Use in the Setting of Orthopaedic Trauma Surgery, J. Orthop. Traumatol., 2019, 33(4), 203–213 Search PubMed.
- M. A. Flierl, W. R. Smith, C. Mauffrey, K. Irgit, A. E. Williams, E. Ross, G. Peacher, D. J. Hak and P. F. Stahel, Outcomes and complication rates of different bone grafting modalities in long bone fracture nonunions: a retrospective cohort study in 182 patients, J. Orthop. Surg. Res., 2013, 8, 33 CrossRef PubMed.
- M. A. Fernandez-Yague, S. A. Abbah, L. McNamara, D. I. Zeugolis, A. Pandit and M. J. Biggs, Biomimetic approaches in bone tissue engineering: Integrating biological and physicomechanical strategies, Adv. Drug Delivery Rev., 2015, 84, 1–29 CrossRef CAS PubMed.
- J. R. Perez, D. Kouroupis, D. J. Li, T. M. Best, L. Kaplan and D. Correa, Tissue Engineering and Cell-Based Therapies for Fractures and Bone Defects, Front. Bioeng. Biotechnol., 2018, 6, 105 CrossRef PubMed.
- L. Ricciotti, A. Apicella, V. Perrotta and R. Aversa, Geopolymer Materials for Bone Tissue Applications: Recent Advances and Future Perspectives, Polymers, 2023, 15(5), 1087 CrossRef CAS PubMed.
- M. P. Ginebra, M. Espanol, Y. Maazouz, V. Bergez and D. Pastorino, Bioceramics and bone healing, EFORT Open Rev., 2018, 3(5), 173–183 CrossRef PubMed.
- X. Gu, W. Lin, D. Li, H. Guo, P. Li and Y. Fan, Degradation and biocompatibility of a series of strontium substituted hydroxyapatite coatings on magnesium alloys, RSC Adv., 2019, 9(26), 15013–15021 RSC.
- A. Bharadwaz and A. C. Jayasuriya, Recent trends in the application of widely used natural and synthetic polymer nanocomposites in bone tissue regeneration, Mater. Sci. Eng., C, 2020, 110, 110698 CrossRef CAS PubMed.
- S. M. Kurtz and J. N. Devine, PEEK biomaterials in trauma, orthopedic, and spinal implants, Biomaterials, 2007, 28(32), 4845–4869 CrossRef CAS PubMed.
- L. Ouyang, M. Chen, D. Wang, T. Lu, H. Wang, F. Meng, Y. Yang, J. Ma, K. W. K. Yeung and X. Liu, Nano Textured PEEK Surface for Enhanced Osseointegration, ACS Biomater. Sci. Eng., 2019, 5(3), 1279–1289 CrossRef CAS PubMed.
- X. Han, D. Yang, C. Yang, S. Spintzyk, L. Scheideler, P. Li, D. Li, J. Geis-Gerstorfer and F. Rupp, Carbon Fiber Reinforced PEEK Composites Based on 3D-Printing
Technology for Orthopedic and Dental Applications, J. Clin. Med., 2019, 8(2), 240 CrossRef CAS PubMed.
- H. Li, R. Fouracre, M. Given, H. Banford, S. Wysocki and S. Karolczak, The effects on polyetheretherketone and polyethersulfone of electron and/spl gamma/irradiation, IEEE Trans. Dielectr. Electr. Insul., 1999, 6(3), 295–303 CrossRef CAS.
- T. Sasuga and M. Hagiwara, Radiation deterioration of several aromatic polymers under oxidative conditions, Polymer, 1987, 28(11), 1915–1921 CrossRef CAS.
- W. Jacobs, P. C. Willems, M. Kruyt, J. van Limbeek, P. G. Anderson, P. Pavlov, R. Bartels and C. Oner, Systematic review of anterior interbody fusion techniques for single- and double-level cervical degenerative disc disease, Spine, 2011, 36(14), E950–E960 CrossRef PubMed.
- N. Bonnheim, F. Ansari, M. Regis, P. Bracco and L. Pruitt, Effect of carbon fiber type on monotonic and fatigue properties of orthopedic grade PEEK, J. Mech. Behav. Biomed. Mater., 2019, 90, 484–492 CrossRef CAS PubMed.
- M. He, Y. Huang, H. Xu, G. Feng, L. Liu, Y. Li, D. Sun and L. Zhang, Modification of polyetheretherketone implants: From enhancing bone integration to enabling multi-modal therapeutics, Acta Biomater., 2021, 129, 18–32 CrossRef CAS PubMed.
- D. Wang, Y. Zou, L. Tao, Y. Zhang, Z. Liu, S. Du, S. Zang and S. Wang, Low-temperature plasma technology for electrocatalysis, Chin. Chem. Lett., 2019, 30(4), 826–838 CrossRef CAS.
- A. H. Poulsson, D. Eglin, S. Zeiter, K. Camenisch, C. Sprecher, Y. Agarwal, D. Nehrbass, J. Wilson and R. G. Richards, Osseointegration of machined, injection moulded and oxygen plasma modified PEEK implants in a sheep model, Biomaterials, 2014, 35(12), 3717–3728 CrossRef CAS PubMed.
- N. Fukuda, A. Tsuchiya, Sunarso, R. Toita, K. Tsuru, Y. Mori and K. Ishikawa, Surface plasma treatment and phosphorylation enhance the biological performance of poly(ether ether ketone), Colloids Surf., B, 2019, 173, 36–42 CrossRef CAS PubMed.
- M. Fedel, V. Micheli, M. Thaler and F. Awaja, Effect of nitrogen plasma treatment on the crystallinity and self-bonding of polyetheretherketone (PEEK) for biomedical applications, Polym. Adv. Technol., 2020, 31(2), 240–247 CrossRef CAS PubMed.
- C. Liu, J. Bai, Y. Wang, L. Chen, D. Wang, S. Ni and H. Liu, The effects of three cold plasma treatments on the osteogenic activity and antibacterial property of PEEK, Dent. Mater., 2021, 37(1), 81–93 CrossRef CAS PubMed.
- Q. Fu, M. Gabriel, F. Schmidt, W. D. Müller and A. D. Schwitalla, The impact of different low-pressure plasma types on the physical, chemical and biological surface properties of PEEK, Dent. Mater., 2021, 37(1), e15–e22 CrossRef CAS PubMed.
- X. Han, N. Sharma, S. Spintzyk, Y. Zhou, Z. Xu, F. M. Thieringer and F. Rupp, Tailoring the biologic responses of 3D printed PEEK medical implants by plasma functionalization, Dent. Mater., 2022, 38(7), 1083–1098 CrossRef CAS PubMed.
- K. Przykaza, M. Jurak, G. Kalisz, R. Mroczka and A. E. Wiącek, Characteristics of Hybrid Bioglass-Chitosan Coatings on the Plasma Activated PEEK Polymer, Molecules, 2023, 28(4), 1729 CrossRef CAS PubMed.
- D. Xie, C. Xu, C. Ye, S. Mei, L. Wang, Q. Zhu, Q. Chen, Q. Zhao, Z. Xu, J. Wei and L. Yang, Fabrication of Submicro-Nano Structures on Polyetheretherketone Surface by Femtosecond Laser for Exciting
Cellular Responses of MC3T3-E1 Cells/Gingival Epithelial Cells, Int. J. Nanomed., 2021, 16, 3201–3216 CrossRef CAS PubMed.
- F. Luo, R. Mao, Y. Huang, L. Wang, Y. Lai, X. Zhu, Y. Fan, K. Wang and X. Zhang, Femtosecond laser optimization of PEEK: efficient bioactivity achieved by synergistic surface chemistry and structures, J. Mater. Chem. B, 2022, 10(36), 7014–7029 RSC.
- H. Xie, C. Zhang, R. Wang, H. Tang, M. Mu, H. Li, Y. Guo, L. Yang and K. Tang, Femtosecond laser-induced periodic grooves and nanopore clusters make a synergistic effect on osteogenic differentiation, Colloids Surf., B, 2021, 208, 112021 CrossRef CAS PubMed.
- Y. Zheng, C. Xiong, Z. Wang, X. Li and L. Zhang, A combination of CO2 laser and plasma surface modification of poly(etheretherketone) to enhance osteoblast response, Appl. Surf. Sci., 2015, 344, 79–88 CrossRef CAS.
- N. Limaye, L. Veschini and T. Coward, Assessing biocompatibility & mechanical testing of 3D-printed PEEK versus milled PEEK, Heliyon, 2022, 8(12), e12314 CrossRef CAS PubMed.
- D. Gouveia, M. E. Razzoog, M. Sierraalta and M. F. Alfaro, Effect of surface treatment and manufacturing process on the shear bond strength of veneering composite resin to polyetherketoneketone (PEKK) and polyetheretherketone (PEEK), J. Prosthet. Dent., 2022, 128(5), 1061–1066 CrossRef CAS PubMed.
- J. Czwartos, B. Budner, A. Bartnik, P. Wachulak, H. Fiedorowicz and Z. Mierczyk, Physico-Chemical Surface Modifications of Polyetheretherketone (PEEK) Using Extreme Ultraviolet (EUV) Radiation and EUV-Induced Nitrogen Plasma, Materials, 2020, 13(19), 4466 CrossRef CAS PubMed.
- S. Okawa, N. Taka and Y. Aoyagi, Effect of Modification with Helium Atmospheric-Pressure Plasma and Deep-Ultraviolet Light on Adhesive Shear Strength of Fiber-Reinforced Poly(ether-ether-ketone) Polymer, J Funct Biomater, 2020, 11(2), 27 CrossRef CAS PubMed.
- J. Czwartos, B. Budner, A. Bartnik, P. Wachulak, B. A. Butruk-Raszeja, A. Lech, T. Ciach and H. Fiedorowicz, Effect of Extreme Ultraviolet (EUV) Radiation and EUV Induced, N(2) and O(2) Based Plasmas on a PEEK Surface’s Physico-Chemical Properties and MG63 Cell Adhesion, Int. J. Mol. Sci., 2021, 22(16), 8455 CrossRef CAS PubMed.
- S. Ajami, M. J. Coathup, J. Khoury and G. W. Blunn, Augmenting the bioactivity of polyetheretherketone using a novel accelerated neutral atom beam technique, J. Biomed. Mater. Res., Part B, 2017, 105(6), 1438–1446 CrossRef CAS PubMed.
- T. J. Webster, J. R. Shallenberger, E. R. Edelman and J. Khoury, Accelerated Neutral Atom Beam (ANAB) Modified Poly-Ether-Ether-Ketone for Increasing In Vitro Bone Cell Functions and Reducing Bacteria Colonization Without Drugs or Antibiotics, J. Biomed. Nanotechnol., 2022, 18(3), 788–795 CrossRef PubMed.
- Y. Li, J. Wang and D. He, GuoxiongZhu; Wu, G.; Chen, L., Surface sulfonation and nitrification enhance the biological activity and osteogenesis of polyetheretherketone by forming an irregular nano-porous monolayer, J. Mater. Sci.: Mater. Med., 2019, 31(1), 11 CrossRef PubMed.
- Y. Zheng, L. Liu, L. Xiao, Q. Zhang and Y. Liu, Enhanced osteogenic activity of phosphorylated polyetheretherketone via surface-initiated grafting polymerization of vinylphosphonic acid, Colloids Surf., B, 2019, 173, 591–598 CrossRef CAS PubMed.
- L. Liu, W. Zhang, L. Yuan, Y. Liu and Y. Zheng, Ameliorative antibacterial, anti-inflammatory, and osteogenic activity of sulfonate-bearing polyetheretherketone toward orthopedic and dental implants, Mater. Lett., 2021, 305, 130774 CrossRef CAS.
- Z. Zheng, P. Liu, X. Zhang, X. Jingguo, W. Yongjie, X. Zou, X. Mei, S. Zhang and S. Zhang, Strategies to improve bioactive and antibacterial properties of polyetheretherketone (PEEK) for use as orthopedic implants, Mater. Today Bio, 2022, 16, 100402 CrossRef CAS PubMed.
- T. Miyazaki, C. Matsunami and Y. Shirosaki, Bioactive carbon-PEEK
composites prepared by chemical surface treatment, Mater. Sci. Eng., C, 2017, 70(1), 71–75 CrossRef CAS PubMed.
- Y. Niu, L. Guo, F. Hu, L. Ren, Q. Zhou, J. Ru and J. Wei, Macro-Microporous Surface with Sulfonic Acid Groups and Micro-Nano Structures of PEEK/Nano Magnesium Silicate Composite Exhibiting Antibacterial Activity and Inducing Cell Responses, Int. J. Nanomed., 2020, 15, 2403–2417 CrossRef CAS PubMed.
- M. Escobar, J. C. M. Souza, G. M. O. Barra, M. C. Fredel, M. Özcan and B. Henriques, On the synergistic effect of sulfonic functionalization and acidic adhesive conditioning to enhance the adhesion of PEEK to resin-matrix composites, Dent. Mater., 2021, 37(4), 741–754 CrossRef CAS PubMed.
- H. Xin, Q. Shi, X. Ning, Y. Chen, X. Jia, Z. Zhang, S. Zhu, Y. Li, F. Liu and L. Kong, Biomimetic Mineralized Fiber Bundle-Inspired Scaffolding Surface on Polyetheretherketone Implants Promotes Osseointegration, Macromol. Biosci., 2023, 23(4), e2200436 CrossRef PubMed.
- N. Addai Asante, Y. Wang, S. Bakhet, S. Kareem, K. A. Owusu, Y. Hu and M. Appiah, Ambient temperature sulfonated carbon fiber reinforced PEEK with hydroxyapatite and reduced graphene oxide hydroxyapatite composite coating, J. Biomed. Mater. Res., Part B, 2021, 109(12), 2174–2183 CrossRef CAS PubMed.
- R. Ekambaram and S. Dharmalingam, Fabrication and evaluation of electrospun biomimetic sulphonated PEEK nanofibrous scaffold for human skin cell proliferation and wound regeneration potential, Mater. Sci. Eng., C, 2020, 115, 111150 CrossRef CAS PubMed.
- Z. Yuan, T. Long, J. Zhang, Z. Lyu, W. Zhang, X. Meng, J. Qi and Y. Wang, 3D printed porous sulfonated polyetheretherketone scaffold for cartilage repair: Potential and limitation, J. Orthop. Transl., 2022, 33, 90–106 Search PubMed.
- J. Zhang, Y. Yi, C. Wang, L. Ding, R. Wang and G. Wu, Effect of Acid-Etching Duration on the Adhesive Performance of Printed Polyetheretherketone to Veneering Resin, Polymers, 2021, 13(20), 3509 CrossRef CAS PubMed.
- T. Wan, Z. Jiao, M. Guo, Z. Wang, Y. Wan, K. Lin, Q. Liu and P. Zhang, Gaseous sulfur trioxide induced controllable sulfonation promoting biomineralization and osseointegration of polyetheretherketone implants, Bioact. Mater., 2020, 5(4), 1004–1017 Search PubMed.
- N. Bloise, E. I. Waldorff, G. Montagna, G. Bruni, L. Fassina, S. Fang, N. Zhang, J. Jiang, J. T. Ryaby and L. Visai, Early Osteogenic Marker Expression in hMSCs Cultured onto Acid Etching-Derived Micro- and Nanotopography 3D-Printed Titanium Surfaces, Int. J. Mol. Sci., 2022, 23(13), 7070 CrossRef CAS PubMed.
- R. Ding, T. Chen, Q. Xu, R. Wei, B. Feng, J. Weng, K. Duan, J. Wang, K. Zhang and X. Zhang, Mixed Modification of the Surface Microstructure and Chemical State of Polyetheretherketone to Improve Its Antimicrobial Activity, Hydrophilicity, Cell Adhesion, and Bone Integration, ACS Biomater. Sci. Eng., 2020, 6(2), 842–851 CrossRef CAS PubMed.
- J. Ma, Q. Liang, W. Qin, P. O. Lartey, Y. Li and X. Feng, Bioactivity of nitric acid and calcium chloride treated carbon-fibers reinforced polyetheretherketone for dental implant, J. Mech. Behav. Biomed. Mater., 2020, 102, 103497 CrossRef CAS PubMed.
- S. Huo, X. Meng, S. Zhang, B. Yue, Y. Zhao, T. Long, B. Nie and Y. Wang, Hydrofluoric acid and nitric acid cotreatment for biofunctionalization of polyetheretherketone in M2 macrophage polarization and osteogenesis, J. Biomed. Mater. Res., Part A, 2021, 109(6), 879–892 CrossRef CAS PubMed.
- X. Gu, X. Sun, Y. Sun, J. Wang, Y. Liu, K. Yu, Y. Wang and Y. Zhou, Bioinspired Modifications of PEEK Implants for Bone Tissue Engineering, Front. Bioeng. Biotechnol., 2020, 8, 631616 CrossRef PubMed.
- C. Liao, Y. Li and S. C. Tjong, Polyetheretherketone and Its Composites for Bone Replacement and Regeneration, Polymers, 2020, 12(12), 2858 CrossRef CAS PubMed.
- Y. Li, Y. Chen, Y. Guo, D. Bian and Y. Zhao, Tribological Behavior of PEEK/PTFE Composites Reinforced with Carbon Fibers and Graphite, Materials, 2022, 15(20), 7078 CrossRef CAS PubMed.
- Y. Yamane, T. Yabutsuka, Y. Takaoka, C. Ishizaki, S. Takai and S. Fujibayashi, Surface Modification of Carbon Fiber-Polyetheretherketone Composite to Impart Bioactivity by Using Apatite Nuclei, Materials, 2021, 14(21), 6691 CrossRef CAS PubMed.
- S. F. Kiew, L. V. Kiew, H. B. Lee, T. Imae and L. Y. Chung, Assessing biocompatibility of graphene oxide-based nanocarriers: A review, J. Controlled Release, 2016, 226, 217–228 CrossRef CAS PubMed.
- S. Yaragalla, M. Zahid, J. K. Panda, N. Tsagarakis, R. Cingolani and A. Athanassiou, Comprehensive Enhancement in Thermomechanical Performance of Melt-Extruded PEEK Filaments by Graphene Incorporation, Polymers, 2021, 13(9), 1425 CrossRef CAS PubMed.
- Z. Su, J. Zhang, P. Tan, S. Zhu and N. Jiang, Selective Polyetheretherketone Implants Combined with Graphene Cause Definitive Cell Adhesion and Osteogenic Differentiation, Int. J. Nanomed., 2022, 17, 5327–5338 CrossRef CAS PubMed.
- W. Qin, Y. Li, J. Ma, Q. Liang, X. Cui, H. Jia and B. Tang, Osseointegration and biosafety of graphene oxide wrapped porous CF/PEEK composites as implantable materials: The role of surface structure and chemistry, Dent. Mater., 2020, 36(10), 1289–1302 CrossRef CAS PubMed.
- C. Guo, R. Lu, X. Wang and S. Chen, Antibacterial activity, bio-compatibility and osteogenic differentiation of graphene oxide coating on 3D-network poly-ether-ether-ketone for orthopaedic implants, J. Mater. Sci.: Mater. Med., 2021, 32(11), 135 CrossRef CAS PubMed.
- A. Szcześ, L. Hołysz and E. Chibowski, Synthesis of hydroxyapatite for biomedical applications, Adv. Colloid Interface Sci., 2017, 249, 321–330 CrossRef PubMed.
- S. M. Zakaria, S. H. Sharif Zein, M. R. Othman, F. Yang and J. A. Jansen, Nanophase hydroxyapatite as a biomaterial in advanced hard tissue engineering: a review, Tissue Eng., Part B, 2013, 19(5), 431–441 CrossRef CAS PubMed.
- H. Zhou and J. Lee, Nanoscale hydroxyapatite particles for bone tissue engineering, Acta Biomater., 2011, 7(7), 2769–2781 CrossRef CAS PubMed.
- X. Yu, S. Yao, C. Chen, J. Wang, Y. Li, Y. Wang, A. Khademhosseini, J. Wan and Q. Wu, Preparation of Poly(ether-ether-ketone)/Nanohydroxyapatite Composites with Improved Mechanical Performance and Biointerfacial Affinity, ACS Omega, 2020, 5(45), 29398–29406 CrossRef CAS PubMed.
- J. Kang, J. Zheng, Y. Hui and D. Li, Mechanical Properties of 3D-Printed PEEK/HA Composite Filaments, Polymers, 2022, 14(20), 4293 CrossRef CAS PubMed.
- N. Wang, D. Qi, L. Liu, Y. Zhu, H. Liu and S. Zhu, Fabrication of In Situ Grown Hydroxyapatite Nanoparticles Modified Porous Polyetheretherketone Matrix Composites to Promote Osteointegration and Enhance Bone Repair, Front. Bioeng. Biotechnol., 2022, 10, 831288 CrossRef PubMed.
- C. Sun, H. Zhao, L. Wang, J. Zhang, J. Zheng, Z. Yang, L. Huang, L. Wang, C. Liu, D. Li and Q. Li, Additive manufactured polyether-ether-ketone composite scaffolds with hydroxyapatite filler and porous structure promoted the integration with soft tissue, Biomater. Adv., 2022, 141, 213119 CrossRef CAS PubMed.
- K. Rodzeń, P. K. Sharma, A. McIlhagger, M. Mokhtari, F. Dave, D. Tormey, R. Sherlock, B. J. Meenan and A. Boyd, The Direct 3D Printing of Functional PEEK/Hydroxyapatite Composites via a Fused Filament Fabrication Approach, Polymers, 2021, 13(4), 545 CrossRef PubMed.
- J. Zheng, H. Zhao, E. Dong, J. Kang, C. Liu, C. Sun, D. Li and L. Wang, Additively-manufactured PEEK/HA porous scaffolds with highly-controllable mechanical properties and excellent biocompatibility, Mater. Sci. Eng., C, 2021, 128, 112333 CrossRef CAS PubMed.
- F. Manzoor, A. Golbang, S. Jindal, D. Dixon, A. McIlhagger, E. Harkin-Jones, D. Crawford and E. Mancuso, 3D printed PEEK/HA composites for bone tissue engineering applications: Effect of material formulation on mechanical performance and bioactive potential, J. Mech. Behav. Biomed. Mater., 2021, 121, 104601 CrossRef CAS PubMed.
- Y. Zheng, H. Zhou, M. Li, J. Fu, J. Dong, Y. Liu and L. Liu, Polyetheretherketone surface engineered with a degradable hybrid coating for accelerating osteogenesis, Mater. Lett., 2023, 331, 133515 CrossRef CAS.
- Y. Zheng, A. Gao, J. Bai, Q. Liao, Y. Wu, W. Zhang, M. Guan, L. Tong, D. Geng, X. Zhao, P. K. Chu and H. Wang, A programmed surface on polyetheretherketone for sequentially dictating osteoimmunomodulation and bone regeneration to achieve ameliorative osseointegration under osteoporotic conditions, Bioact. Mater., 2022, 14, 364–376 CAS.
- F. Manzoor, A. Golbang, D. Dixon, E. Mancuso, U. Azhar, I. Manolakis, D. Crawford, A. McIlhagger and E. Harkin-Jones, 3D Printed Strontium and Zinc Doped Hydroxyapatite Loaded PEEK for Craniomaxillofacial Implants, Polymers, 2022, 14(7), 1376 CrossRef CAS PubMed.
- Y. Guo, C. Chen, S. Zhang, L. Ren, Y. Zhao and W. Guo, Mediation of mechanically adapted TiCu/TiCuN/CFR-PEEK implants in vascular regeneration to promote bone repair in vitro and in vivo, J. Orthop. Transl., 2022, 33, 107–119 Search PubMed.
- R. Wan, X. Wang, L. Lei, G. Hu, H. Tang and H. Gu, Enhanced anti-microbial activity and osseointegration of Ta/Cu co-implanted polyetheretherketone, Colloids Surf., B, 2022, 218, 112719 CrossRef CAS PubMed.
- S. Chernousova and M. Epple, Silver as antibacterial agent: ion, nanoparticle, and metal, Angew. Chem., Int. Ed., 2013, 52(6), 1636–1653 CrossRef CAS PubMed.
- W. F. Lee, L. Y. Wang, T. Y. Renn, J. C. Yang, L. S. Fang, Y. H. Lee and P. W. Peng, Characterization and Antibacterial Properties of Polyetherketoneketone Coated with a Silver Nanoparticle-in-Epoxy Lining, Polymers, 2022, 14(14), 2906 CrossRef CAS PubMed.
- J. Siegel, B. Vyhnálková, T. Savenkova, J. Pryjmaková, P. Slepička, M. Šlouf and T. Hubáček, Surface Engineering of AgNPs-Decorated Polyetheretherketone, Int. J. Mol. Sci., 2023, 24(2), 1432 CrossRef CAS PubMed.
- X. Yang, H. Chai, L. Guo, Y. Jiang, L. Xu, W. Huang, Y. Shen, L. Yu, Y. Liu and J. Liu, In situ preparation of porous metal-organic frameworks ZIF-8@Ag on poly-ether-ether-ketone with synergistic antibacterial activity, Colloids Surf., B, 2021, 205, 111920 CrossRef CAS PubMed.
- Y. Zhang, H. Wu, B. Yuan, X. Zhu, K. Zhang and X. Zhang, Enhanced osteogenic activity and antibacterial performance in vitro of polyetheretherketone by plasma-induced graft polymerization of acrylic acid and incorporation of zinc ions, J. Mater. Chem. B, 2021, 9(36), 7506–7515 RSC.
- T. Wu, X. Zhang, K. Chen, Q. Chen, Z. Yu, C. Feng, J. Qi and D. Zhang, The antibacterial and wear-resistant nano-ZnO/PEEK composites were constructed by a simple two-step method, J. Mech. Behav. Biomed. Mater., 2022, 126, 104986 CrossRef CAS PubMed.
- X. Meng, W. Zhang, Z. Lyu, T. Long and Y. Wang, ZnO nanoparticles attenuate polymer-wear-particle induced inflammatory osteolysis by regulating the MEK-ERK-COX-2 axis, J. Orthop. Transl., 2022, 34, 1–10 Search PubMed.
- L. Hu, Y. Ge, Z. Cao, Y. Tian, Q. Sun, Z. Li, J. Ma, Y. Wu, N. Wang and B. Tang, Strontium-modified porous polyetheretherketone with the triple function of osteogenesis, angiogenesis, and anti-inflammatory for bone grafting, Biomater. Adv., 2022, 143, 213160 CrossRef CAS PubMed.
- H. Wang, X. Fu, J. Shi, L. Li, J. Sun, X. Zhang, Q. Han, Y. Deng and X. Gan, Nutrient Element Decorated Polyetheretherketone Implants Steer Mitochondrial Dynamics for Boosted Diabetic Osseointegration, Adv. Sci., 2021, 8(20), e2101778 CrossRef PubMed.
- B. C. Cheng, S. Jaffee, S. Averick, I. Swink, S. Horvath and R. Zhukauskas, A comparative study of three biomaterials in an ovine bone defect model, Spine J., 2020, 20(3), 457–464 CrossRef PubMed.
- C. Liu, Y. Zhang, L. Xiao, X. Ge, F. C. Öner and H. Xu, Vacuum plasma sprayed porous titanium coating on polyetheretherketone for ACDF improves the osteogenic ability: An in vitro and in vivo study, Biomed. Microdevices, 2021, 23(2), 21 CrossRef CAS PubMed.
- M. Hayashi, T. Shimizu, M. Imamura, S. Fujibayashi, S. Yamaguchi, K. Goto, B. Otsuki, T. Kawai, Y. Okuzu and S. Matsuda, Optimizing the layer thickness of sol-gel-derived TiO(2) coating on polyetheretherketone, Sci. Rep., 2021, 11(1), 15875 CrossRef CAS PubMed.
- C. Q. Jia, Z. Zhang, S. Q. Cao, T. J. Wang, H. C. Yu, W. X. Wang, B. M. Guo, X. Y. Qiu, Y. G. You, F. Q. Hu, J. Zhao and X. S. Zhang, A biomimetic gradient porous cage with a micro-structure for enhancing mechanical properties and accelerating osseointegration in spinal fusion, Bioact. Mater., 2023, 23, 234–246 CAS.
- L. Ren, S. Tang, X. Shen, C. Gao, Y. K. Jung, D. Wang, A. He, L. Yang, J. W. Shin and J. Wei, Influences of sodium tantalite submicro-particles in polyetheretherketone based composites on behaviors of rBMSCs/HGE-1 cells for dental application, Colloids Surf., B, 2020, 188, 110723 CrossRef CAS PubMed.
- K. Yuan, K. Zhang, Y. Yang, Y. Lin, F. Zhou, J. Mei, H. Li, J. Wei, Z. Yu, J. Zhao and T. Tang, Evaluation of interbody fusion efficacy and biocompatibility of a polyetheretherketone/calcium silicate/porous tantalum cage in a goat model, J. Orthop. Transl., 2022, 36, 109–119 Search PubMed.
- Z. Pang, Z. Pan, M. Ma, Z. Xu, S. Mei, Z. Jiang and F. Yin, Nanostructured Coating of Non-Crystalline Tantalum Pentoxide on Polyetheretherketone Enhances RBMS Cells/HGE Cells Adhesion, Int. J. Nanomed., 2021, 16, 725–740 CrossRef PubMed.
- J. Ge, F. Wang, Z. Xu, X. Shen, C. Gao, D. Wang, G. Hu, J. Gu, T. Tang and J. Wei, Influences of niobium pentoxide on roughness, hydrophilicity, surface energy and protein absorption, and cellular responses to PEEK based composites for orthopedic applications, J. Mater. Chem.
B, 2020, 8(13), 2618–2626 RSC.
- X. Lv, X. Wang, S. Tang, D. Wang, L. Yang, A. He, T. Tang and J. Wei, Incorporation of molybdenum disulfide into polyetheretherketone creating biocomposites with improved mechanical, tribological performances and cytocompatibility for artificial joints applications, Colloids Surf., B, 2020, 189, 110819 CrossRef CAS PubMed.
- N. Kakkad, N. S. Yadav, P. Hazari, S. Narwani, K. Somkuwar, S. Basha, V. Verma, S. Arora, O. Aldowah, A. Heboyan and M. I. Karobari, Comparative Evaluation of Tensile Bond Strength of Poly Ether Ether Ketone (PEEK) and Zirconia Copings Using Resin Cement with or without Adhesive: An In Vitro Study, Materials, 2022, 15, 12 CrossRef PubMed.
- P. Ayyadanveettil, V. Thavakkara, N. Latha, M. Pavanan, A. Saraswathy and M. S. Kuruniyan, Randomized clinical trial of zirconia and polyetheretherketone implant abutments for single-tooth implant restorations: A 5-year evaluation, J. Prosthet. Dent., 2022, 128(6), 1275–1281 CrossRef CAS PubMed.
- X. Wei, W. Zhou, Z. Tang, H. Wu, Y. Liu, H. Dong, N. Wang, H. Huang, S. Bao, L. Shi, X. Li, Y. Zheng and Z. Guo, Magnesium surface-activated 3D printed porous PEEK scaffolds for in vivo osseointegration by promoting angiogenesis and osteogenesis, Bioact. Mater., 2023, 20, 16–28 CAS.
- F. Wang, P. Sun, E. Xie, Y. Ji, Y. Niu, F. Li and J. Wei, Phytic acid/magnesium ion complex coating on PEEK fiber woven fabric as an artificial ligament with anti-fibrogenesis and osteogenesis for ligament-bone healing, Biomater. Adv., 2022, 140, 213079 CrossRef CAS PubMed.
- T. Dong, C. Duan, S. Wang, X. Gao, Q. Yang, W. Yang and Y. Deng, Multifunctional Surface with Enhanced Angiogenesis for Improving Long-Term Osteogenic Fixation of Poly(ether ether ketone) Implants, ACS Appl. Mater. Interfaces, 2020, 12(13), 14971–14982 CrossRef CAS PubMed.
- H. Yu, Y. Chen, M. Mao, D. Liu, J. Ai and W. Leng, PEEK-biphasic bioceramic composites promote mandibular defect repair and upregulate BMP-2 expression in rabbits, Mol. Med. Rep., 2018, 17(6), 8221–8227 CAS.
- F. Senatov, A. Maksimkin, A. Chubrik, E. Kolesnikov, P. Orlova, M. Krivozubov, K. Nikitin, A. Gromov and A. Karyagina, Osseointegration evaluation of UHMWPE and PEEK-based scaffolds with BMP-2 using model of critical-size cranial defect in mice and push-out test, J. Mech. Behav. Biomed. Mater., 2021, 119, 104477 CrossRef CAS PubMed.
- Z. Sun, L. Ouyang, X. Ma, Y. Qiao and X. Liu, Controllable and durable release of BMP-2-loaded 3D porous sulfonated polyetheretherketone (PEEK) for osteogenic activity enhancement, Colloids Surf., B, 2018, 171, 668–674 CrossRef CAS PubMed.
- J. Wu, L. Li, C. Fu, F. Yang, Z. Jiao, X. Shi, Y. Ito, Z. Wang, Q. Liu and P. Zhang, Micro-porous polyetheretherketone implants decorated with BMP-2 via phosphorylated gelatin coating for enhancing cell adhesion and osteogenic differentiation, Colloids Surf., B, 2018, 169, 233–241 CrossRef CAS PubMed.
- S. Qin, Z. Lu, K. Gan, C. Qiao, B. Li, T. Chen, Y. Gao, L. Jiang and H. Liu, Construction of a BMP-2 gene delivery system for polyetheretherketone bone implant material and its effect on bone formation in vitro, J. Biomed. Mater. Res., Part B, 2022, 110(9), 2075–2088 CrossRef CAS PubMed.
- W. Liu, H. Wang, C. Liu, J. Wang, X. Cheng, C. Liu, L. Qiao, S. Zhang and X. Jian, RhBMP-2 immobilized on poly(phthalazinone ether nitrile ketone) via chemical and physical modification for promoting in vitro osteogenic differentiation, Colloids Surf., B, 2020, 194, 111173 CrossRef CAS PubMed.
- X. Wang, N. Ma, L. Feng, M. Shen, Y. Zhou, X. Zhang, R. Huang, L. Zhou, S. Ji, Y. Lou and Z. Zhu, Fabrication of bFGF/polydopamine-loaded PEEK implants for improving soft tissue integration by upregulating Wnt/β-catenin signaling, Heliyon, 2023, 9(4), e14800 CrossRef CAS PubMed.
- Z. Yuan, Z. Lyu, W. Zhang, J. Zhang and Y. Wang, Porous Bioactive Prosthesis With Chitosan/Mesoporous Silica Nanoparticles Microspheres Sequentially and Sustainedly Releasing Platelet-Derived Growth Factor-BB and Kartogenin: A New Treatment Strategy for Osteoarticular Lesions, Front. Bioeng. Biotechnol., 2022, 10, 839120 CrossRef PubMed.
- S. Wang, Y. Yang, Y. Li, J. Shi, J. Zhou, L. Zhang, Y. Deng and W. Yang, Strontium/adiponectin co-decoration modulates the osteogenic activity of nano-morphologic polyetheretherketone implant, Colloids Surf., B, 2019, 176, 38–46 CrossRef CAS PubMed.
- L. Oliver-Cervelló, H. Martin-Gómez, N. Mandakhbayar, Y. W. Jo, E. A. Cavalcanti-Adam, H. W. Kim, M. P. Ginebra, J. H. Lee and C. Mas-Moruno, Mimicking Bone Extracellular Matrix: From BMP-2-Derived Sequences to Osteogenic-Multifunctional Coatings., Adv. Healthcare Mater., 2022, 11(20), e2201339 CrossRef PubMed.
- H. K. Kim, J. H. Kim, D. S. Park, K. S. Park, S. S. Kang, J. S. Lee, M. H. Jeong and T. R. Yoon, Osteogenesis induced by a bone forming peptide from the prodomain region of BMP-7, Biomaterials, 2012, 33(29), 7057–7063 CrossRef CAS PubMed.
- Y. Yu, Y. Sun, X. Zhou, Y. Mao, Y. Liu, L. Ye, L. Kuang, J. Yang and Y. Deng, Ag and peptide co-decorate polyetheretherketone to enhance antibacterial property and osteogenic differentiation, Colloids Surf., B, 2021, 198, 111492 CrossRef CAS PubMed.
- S. Wang, C. Duan, W. Yang, X. Gao, J. Shi, J. Kang, Y. Deng, X. L. Shi and Z. G. Chen, Two-dimensional nanocoating-enabled orthopedic implants for bimodal therapeutic applications, Nanoscale, 2020, 12(22), 11936–11946 RSC.
- M. Yakufu, Z. Wang, Y. Wang, Z. Jiao, M. Guo, J. Liu and P. Zhang, Covalently functionalized poly(etheretherketone) implants with osteogenic growth peptide (OGP) to improve osteogenesis activity, RSC Adv., 2020, 10(17), 9777–9785 RSC.
- Q. Y. Zhang, Z. B. Yan, Y. M. Meng, X. Y. Hong, G. Shao, J. J. Ma, X. R. Cheng, J. Liu, J. Kang and C. Y. Fu, Antimicrobial peptides: mechanism of action, activity and clinical potential, Mil. Med. Res., 2021, 8(1), 48 CAS.
- W. Gao, X. Han, D. Sun, Y. Li, X. Liu, S. Yang, Z. Zhou, Y. Qi, J. Jiao and J. Zhao, Antibacterial properties of antimicrobial peptide HHC36 modified polyetheretherketone, Front. Microbiol., 2023, 14, 1103956 CrossRef PubMed.
- X. Yuan, L. Ouyang, Y. Luo, Z. Sun, C. Yang, J. Wang, X. Liu and X. Zhang, Multifunctional sulfonated polyetheretherketone coating with beta-defensin-14 for yielding durable and broad-spectrum antibacterial activity and osseointegration, Acta Biomater., 2019, 86, 323–337 CrossRef CAS PubMed.
- N. Li, J. Bai, W. Wang, X. Liang, W. Zhang, W. Li, L. Lu, L. Xiao, Y. Xu, Z. Wang, C. Zhu, J. Zhou and D. Geng, Facile and Versatile Surface Functional Polyetheretherketone with Enhanced Bacteriostasis and Osseointegrative Capability for Implant Application, ACS Appl. Mater. Interfaces, 2021, 13(50), 59731–59746 CrossRef CAS PubMed.
- X. Meng, J. Zhang, J. Chen, B. Nie, B. Yue, W. Zhang, Z. Lyu, T. Long and Y. Wang, KR-12 coating of polyetheretherketone (PEEK) surface via polydopamine improves osteointegration and antibacterial activity in vivo, J. Mater. Chem. B, 2020, 8(44), 10190–10204 RSC.
- M. Li, J. Bai, H. Tao, L. Hao, W. Yin, X. Ren, A. Gao, N. Li, M. Wang, S. Fang, Y. Xu, L. Chen, H. Yang, H. Wang, G. Pan and D. Geng, Rational integration of defense and repair synergy on PEEK osteoimplants via biomimetic peptide clicking strategy, Bioact. Mater., 2022, 8, 309–324 CAS.
- Y. Zhu, Z. Cao, Y. Peng, L. Hu, T. Guney and B. Tang, Facile Surface Modification Method for Synergistically Enhancing the Biocompatibility and Bioactivity of Poly(ether ether ketone) That Induced Osteodifferentiation, ACS Appl. Mater. Interfaces, 2019, 11(31), 27503–27511 CrossRef CAS PubMed.
- Y. Zheng, C. Xiong, X. Li and L. Zhang, Covalent attachment of cell-adhesive peptide Gly-Arg-Gly-Asp (GRGD) to poly(etheretherketone) surface by tailored silanization layers technique, Appl. Surf. Sci., 2014, 320, 93–101 CrossRef CAS.
- L. Cassari, A. Zamuner, G. M. L. Messina, M. Marsotto, H. Chen, G. Gonnella, T. Coward, C. Battocchio, J. Huang, G. Iucci, G. Marletta, L. Di Silvio and M. Dettin, Bioactive PEEK: Surface Enrichment of Vitronectin-Derived Adhesive Peptides, Biomolecules, 2023, 13(2), 246 CrossRef CAS PubMed.
- N. Marín-Pareja, E. Salvagni, J. Guillem-Marti, C. Aparicio and M. P. Ginebra, Collagen-functionalised titanium surfaces for biological sealing of dental implants: effect of immobilisation process on fibroblasts response, Colloids Surf., B, 2014, 122, 601–610 CrossRef PubMed.
- A. Saad, C. Penaloza Arias, M. Wang, O. ElKashty, D. Brambilla, F. Tamimi and M. Cerruti, Biomimetic Strategy to Enhance Epithelial Cell Viability and Spreading on PEEK Implants, ACS Biomater. Sci. Eng., 2022, 8(12), 5129–5144 CrossRef CAS PubMed.
- H. Kim, Y. H. Lee, N. K. Kim and I. K. Kang, Immobilization of Collagen on the Surface of a PEEK Implant with Monolayer Nanopores, Polymers, 2022, 14(9), 1633 CrossRef CAS PubMed.
- Y. Arisaka, H. Masuda, T. Yoda and N. Yui, Phototethering of Collagen onto Polyetheretherketone Surfaces to Enhance Osteoblastic and Endothelial Performance, Macromol. Biosci., 2022, 22(8), e2200115 CrossRef PubMed.
- M. Mehdizadeh Omrani, H. Kumar, M. G. A. Mohamed, K. Golovin, S. M. A, A. Hadjizadeh and K. Kim, Polyether ether ketone surface modification with plasma and gelatin for enhancing cell attachment, J. Biomed. Mater. Res., Part B, 2021, 109(5), 622–629 CrossRef CAS PubMed.
- W. Y. Chen and G. Abatangelo, Functions of hyaluronan in wound repair, Wound Repair Regen., 1999, 7(2), 79–89 CrossRef CAS PubMed.
- J. A. Burdick and G. D. Prestwich, Hyaluronic acid hydrogels for biomedical applications, Adv. Mater., 2011, 23(12), H41–56 CrossRef CAS PubMed.
- L. Ferroni, U. D’Amora, S. Leo, E. Tremoli, M. G. Raucci, A. Ronca, L. Ambrosio and B. Zavan, PEEK and Hyaluronan-Based 3D Printed Structures: Promising Combination to Improve Bone Regeneration, Molecules, 2022, 27(24), 8749 CrossRef CAS PubMed.
- S. Liu, Y. Zhu, H. Gao, P. Ge, K. Ren, J. Gao, Y. Cao, D. Han and J. Zhang, One-step fabrication of functionalized poly(etheretherketone) surfaces with enhanced biocompatibility and osteogenic activity, Mater. Sci. Eng., C, 2018, 88, 70–78 CrossRef CAS PubMed.
- Z. Liu, Q. Tang, R. T. Liu, M. Z. Yu, H. Peng, C. Q. Zhang, Z. Z. Zhu and X. J. Wei, LAPONITE® intercalated biomimetic multilayer coating prevents glucocorticoids induced orthopedic implant failure, Bioact. Mater., 2023, 22, 60–73 CAS.
- Z. Zheng, L. Hu, Y. Ge, J. Qi, Q. Sun, Z. Li, L. Lin and B. Tang, Surface Modification of Poly(ether ether ketone) by Simple Chemical Grafting of Strontium Chondroitin Sulfate to Improve its Anti-Inflammation, Angiogenesis, Osteogenic Properties., Adv. Healthcare Mater., 2022, 11(13), e2200398 CrossRef PubMed.
- W. Liu, J. Li, M. Cheng, Q. Wang, Y. Qian, K. W. K. Yeung, P. K. Chu and X. Zhang, A surface-engineered polyetheretherketone biomaterial implant with direct and immunoregulatory antibacterial activity against methicillin-resistant Staphylococcus aureus, Biomaterials, 2019, 208, 8–20 CrossRef CAS PubMed.
- O. H. Cho, I. G. Bae, S. M. Moon, S. Y. Park, Y. G. Kwak, B. N. Kim, S. N. Yu, M. H. Jeon, T. Kim, E. J. Choo, E. J. Lee, T. H. Kim, S. H. Choi, J. W. Chung, K. C. Kang, J. H. Lee, Y. M. Lee, M. S. Lee and K. H. Park, Therapeutic outcome of spinal implant infections caused by Staphylococcus aureus: A retrospective observational study, Medicine, 2018, 97(40), e12629 CrossRef PubMed.
- J. Martínez-Moreno, S. Mir-Palomo, V. Merino, A. Nácher and M. Merino-Sanjuán, Development of antibiotic loaded biodegradable matrices to prevent superficial infections associated to total knee arthroplasty, Colloids Surf., B, 2019, 181, 1–5 CrossRef PubMed.
- A. Sun, X. Lin, Z. Xue, J. Huang, X. Bai, L. Huang, X. Lin, S. Weng and M. Chen, Facile surface functional polyetheretherketone with antibacterial and immunoregulatory activities for enhanced regeneration toward bacterium-infected bone destruction, Drug Delivery, 2021, 28(1), 1649–1663 CrossRef CAS PubMed.
- J. Yan, W. Zhou, Z. Jia, P. Xiong, Y. Li, P. Wang, Q. Li, Y. Cheng and Y. Zheng, Endowing polyetheretherketone with synergistic bactericidal effects and improved osteogenic ability, Acta Biomater., 2018, 79, 216–229 CrossRef CAS PubMed.
- Z. Xue, Z. Wang, A. Sun, J. Huang, W. Wu, M. Chen, X. Hao, Z. Huang, X. Lin and S. Weng, Rapid construction of polyetheretherketone (PEEK) biological implants incorporated with brushite (CaHPO(4)·2H(2)O) and antibiotics for anti-infection and enhanced osseointegration, Mater. Sci. Eng., C, 2020, 111, 110782 CrossRef CAS PubMed.
- J. Huang, S. Lin, X. Bai, W. Li, R. Zhang, C. Miao, X. Zhang, Z. Huang, M. Chen and S. Weng, Decorated Polyetheretherketone Implants with Antibacterial and Antioxidative Effects through Layer-by-Layer Nanoarchitectonics Facilitate Diabetic Bone Integration with Infection, ACS Appl. Mater. Interfaces, 2022, 14(47), 52579–52598 CrossRef CAS PubMed.
- T. Chen, Q. Chen, H. Fu, D. Wang, Y. Gao, M. Zhang and H. Liu, Construction and performance evaluation of a sustained release implant material polyetheretherketone with antibacterial properties, Mater. Sci. Eng., C, 2021, 126, 112109 CrossRef CAS PubMed.
- H. Joo, S. M. Wu, I. Soni, C. Wang-Crocker, T. Matern, J. P. Beck and C. Loc-Carrillo, Phage and Antibiotic Combinations Reduce Staphylococcus aureus in Static and Dynamic Biofilms Grown on an Implant Material, Viruses, 2023, 15(2), 460 CrossRef CAS PubMed.
- D. W. Chen, K. Y. Lee, M. H. Tsai, T. Y. Lin, C. H. Chen and K. W. Cheng, Antibacterial Application on Staphylococcus aureus Using Antibiotic Agent/Zinc Oxide Nanorod Arrays/Polyethylethylketone Composite Samples, Nanomaterials, 2019, 9(5), 713 CrossRef CAS PubMed.
- K. AbouAitah, M. Bil, E. Pietrzykowska, U. Szałaj, D. Fudala, B. Woźniak, J. Nasiłowska, A. Swiderska-Sroda, M. Lojkowski, B. Sokołowska, W. Swieszkowski and W. Lojkowski, Drug-Releasing Antibacterial Coating Made from Nano-Hydroxyapatite Using the Sonocoating Method, Nanomaterials, 2021, 11(7), 1690 CrossRef CAS PubMed.
- K. Przykaza, M. Jurak, A. E. Wiącek and R. Mroczka, Characteristics of hybrid chitosan/phospholipid-sterol, peptide coatings on plasma activated PEEK polymer, Mater. Sci. Eng., C, 2021, 120, 111658 CrossRef CAS PubMed.
- X. Xu, Y. Li, L. Wang, Y. Li, J. Pan, X. Fu, Z. Luo, Y. Sui, S. Zhang, L. Wang, Y. Ni, L. Zhang and S. Wei, Triple-functional polyetheretherketone surface with enhanced bacteriostasis and anti-inflammatory and osseointegrative properties for implant application, Biomaterials, 2019, 212, 98–114 CrossRef CAS PubMed.
Footnote |
† These authors contributed equally to this study. |
|
This journal is © The Royal Society of Chemistry 2024 |