DOI:
10.1039/D3TB02471G
(Review Article)
J. Mater. Chem. B, 2024,
12, 872-894
A new era in cancer treatment: harnessing ZIF-8 nanoparticles for PD-1 inhibitor delivery
Received
19th October 2023
, Accepted 11th December 2023
First published on 11th December 2023
Abstract
This review delves into the potential of zeolitic imidazolate framework-8 (ZIF-8) nanoparticles in augmenting the efficacy of cancer immunotherapy, with a special focus on the delivery of programmed cell death receptor 1 (PD-1) inhibitors. The multifunctional nature of ZIF-8 nanoparticles as drug carriers is emphasized, with their ability to encapsulate a range of therapeutic agents, including PD-1 inhibitors, and facilitate their targeted delivery to tumor locations. By manipulating the pore size and surface characteristics of ZIF-8 nanoparticles, controlled drug release can be realized. The strategic use of ZIF-8 nanoparticles to deliver PD-1 inhibitors presents a precise and targeted modality for cancer treatment, reducing off-target impacts and enhancing therapeutic effectiveness. This combined strategy addresses the existing challenges and constraints of current immunotherapy techniques, with the ultimate goal of enhancing patient outcomes in cancer therapy.
I. Introduction
Immunotherapy, a groundbreaking approach in the fight against cancer, leverages the body's own immune system to detect and destroy cancer cells. Unlike traditional methods such as chemotherapy and radiation, immunotherapy boosts the body's natural defenses against cancer. It has shown success in treating a variety of cancers, including melanoma, lung cancer, and blood-related malignancies.1–6 The main focus of immunotherapy is on modulating immune checkpoints, molecules that regulate the immune response. One such checkpoint is the programmed cell death ligand 1 (PDL1), often overexpressed in cancer cells. PDL1 interacts with its receptor, programmed cell death protein 1 (PD1), on immune cells, suppressing the immune response and allowing cancer cells to evade detection and destruction by the immune system.7,8 Cancer cells with a high concentration of PD-L1 can deactivate T cells, preventing them from attacking the cancerous cells. This evasion mechanism allows cancer cells to continue their growth and proliferation.9–11 Despite the promise of immunotherapy, challenges remain. One hurdle is the lack of tumor specificity, which means the treatment might not exclusively target cancer cells, potentially leading to off-target effects and systemic toxicity.3,12 Additionally, not all patients respond to immunotherapy due to inherent or developed resistance mechanisms.13,14 Delivering therapeutic agents directly to the tumor site is also challenging. Systemic administration often results in less-than-ideal drug concentrations at the tumor site, requiring precision and control for effective treatment.15–18
ZIF-8's high surface area and adjustable pore size allow it to encapsulate various therapeutic agents. This encapsulation protects the agents from degradation and ensures their targeted delivery to the tumor site.19 ZIF-8's high surface area and adjustable pore size allow it to encapsulate various therapeutic agents. This encapsulation protects the agents from degradation and ensures their targeted delivery to the tumor site.20 In essence, ZIF-8 acts as a personal bodyguard for these therapeutic agents, increasing the effectiveness of the treatment while minimizing potential side effects.19 Researchers have found that ZIF-8 can be modified to enhance its performance. For instance, ZIF-8 nanoparticles modified with Pluronic F127 and incorporated with CCCP have demonstrated the ability to stimulate anti-cancer immune responses. This modification alters the immunosuppressive nature of the tumor environment, resulting in highly effective inhibition of tumor growth.21 The integration of ZIF-8 into immunotherapy could potentially overcome some of the current challenges faced in treating cancer. It could guide therapeutic agents directly to the tumor site, increasing the effectiveness of the treatment and minimizing side effects. ZIF-8 is being explored as a targeted delivery system. It is pictured as a tiny sponge with a high surface area and adjustable pore size, making it an ideal candidate for drug delivery (Fig. 1). ZIF-8 can encapsulate various therapeutic agents, including those targeting PD-L1, ensuring they reach their destination – the tumor site.22–25 Hence, the integration of PD-L1 targeting and novel delivery systems like ZIF-8 represents a promising strategy to enhance the effectiveness of immunotherapy.
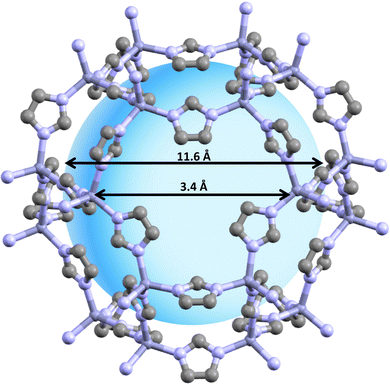 |
| Fig. 1 This figure illustrates the structure of ZIF-8, a type of nanoparticle. ZIF-8 is characterized by its high surface area and adjustable pore size. The ZIF-8 structure can be characterized as a three-dimensional network akin to a diamond, where each Zn ion is linked to six adjacent imidazole ligands, forming octahedral-shaped cages. This ZIF-8 framework is composed of two distinct types of cavities, both made up of octahedral cages. The main cavities, which consist of 8-member cages, have an approximate diameter of 1.16 nm and are interconnected with 6-member cages that have an approximate diameter of 0.34 nm. These properties make ZIF-8 an ideal candidate for drug delivery.26–28 | |
The combination of ZIF-8 with PDL1 inhibitors could potentially enhance the antitumor effects of immunotherapy. The inhibition of PDL1 reignites the immune system's response against cancer cells. ZIF-8 serves as a reliable carrier for PDL1 inhibitors, ensuring their controlled release and targeted delivery to the tumor microenvironment (TME). This integration could enhance the efficacy and safety of immunotherapy, providing a solution to the limitations of current strategies. This review explores recent advancements in the integration of ZIF-8, PDL1, and targeted delivery systems in immunotherapy. It discusses the underlying mechanisms, preclinical and clinical studies, challenges, and future perspectives of this approach. Understanding this strategy could pave the way for the development of more effective and personalized immunotherapeutic approaches in cancer treatment.
II. Understanding ZIF-8 as a versatile nanoparticle for drug delivery
ZIF-8, a nanoparticle that falls under the category of metal–organic frameworks (MOFs), has been the subject of considerable interest in the sphere of drug delivery. This nanoparticle is a complex assembly of metal ions that are meticulously coordinated with organic linkers, culminating in a porous crystalline structure (Fig. 1). The distinguishing features of ZIF-8 are its unique properties. It possesses a high surface area, a characteristic that is indispensable in drug delivery applications. Furthermore, the pore size of ZIF-8 is not fixed but can be adjusted, providing an extraordinary degree of adaptability. In addition to these attributes, ZIF-8 exhibits remarkable stability, an essential quality for its intended applications. These combined properties render ZIF-8 a compelling candidate for drug delivery applications, explaining the significant attention it has garnered in this field.19,20,26–28
ZIF-8, with its high surface area, is capable of accommodating a substantial drug-loading capacity. This allows for the encapsulation of a diverse range of therapeutic agents, including but not limited to small molecules, proteins, nucleic acids, and imaging agents.20,29 The versatility of ZIF-8 is further exemplified by its tunable pore size. This feature can be adjusted to accommodate different drug molecules, thereby providing a high degree of flexibility in drug delivery systems.20,30 Moreover, ZIF-8 is not just about capacity and versatility. It also shines in terms of stability. Under physiological conditions, ZIF-8 maintains excellent stability. This ensures the protection of the encapsulated drugs and controls their release, further enhancing its suitability for drug delivery applications.20,31,32
ZIF-8 has been recognized as a promising carrier for therapeutic agents in the fight against cancer (Fig. 2). The nanoparticle's porous structure serves as an efficient vessel for the encapsulation of anticancer drugs, protecting them from degradation and enhancing their solubility and stability. The release of drugs from ZIF-8 is not a random process. Instead, it can be meticulously controlled by adjusting the pore size and surface properties of the nanoparticles. This allows for a sustained release of the drugs directly at the tumor site, providing a targeted approach to cancer treatment.33 Fascinatingly, ZIF-8 has been suggested as a potential platform for delivering drugs in anti-cancer treatments, owing to its ability to break down in acidic environments. This idea is based on the targeted release of the encapsulated drug molecules near tumor tissues, which are known to secrete substances with a higher level of acidity. This feature has become a point of particular interest in ZIF-8 as a platform for targeted anticancer drug carriers.34 Thus, ZIF-8 is not just a passive carrier; it's an active participant in enhancing the effectiveness of cancer treatment. Its potential in this field is immense and continues to be explored in ongoing research.
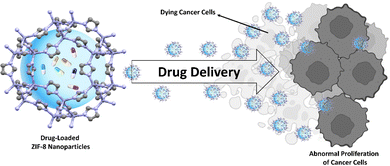 |
| Fig. 2 This figure illustrates the ZIF-8 nanoparticle, a promising carrier for therapeutic agents in the field of drug delivery. The nanoparticle's porous structure, depicted here, allows for efficient encapsulation and release of various anticancer drugs. | |
In addition to its inherent properties, ZIF-8 can be functionalized to enhance its targeting capabilities. Surface modifications, such as the incorporation of targeting ligands or antibodies, facilitate specific recognition and binding to cancer cells. This specificity improves the selectivity and efficacy of drug delivery, positioning ZIF-8 as a potent tool in the field of cancer treatment.20,35,36 This targeted approach aims not only to improve efficacy but also to minimize unintended effects. By concentrating on cancer cells, off-target effects are mitigated, leading to reduced systemic toxicity. The ultimate objective is to enhance therapeutic outcomes. Essentially, ZIF-8 is not merely a drug delivery system; it represents a promising avenue for more effective cancer treatments.20,28,34
ZIF-8 has been used as a drug delivery system for various types of cancer treatment, such as chemotherapy, phototherapy, immunotherapy and others. Here are some examples of how ZIF-8 can be applied in these methods:
Chemotherapy
Chemotherapy is the use of drugs to kill cancer cells or stop their growth. ZIF-8 can be functionalized with targeting ligands or drugs to enhance their specificity and efficacy. For instance, Wang et al. synthesized ZIF-8 on ZnO surface and loaded it with ciprofloxacin, a widely used antibiotic for bacterial infections. They showed that the ZIF-8@ZnO nanocomposite could improve the drug loading efficiency and release kinetics of ciprofloxacin in vitro and in vivo. The ZIF-8@ZnO nanocomposite could also reduce the side effects of ciprofloxacin by increasing its solubility and stability.20
Phototherapy
Phototherapy is the use of light to treat cancer cells or tumors. ZIF-8 can be modified with photosensitizers or light absorbers to increase its sensitivity and response to light. For example, Sanaei-Rad et al. synthesized a novel ternary ZIF-8/GO/MgFe2O4 nanocomposite and loaded it with tetracycline, an antibiotic that can inhibit bacterial growth. They showed that the tetracycline-loaded nanocomposite could be sensitized by visible light (λ > 400 nm) to release tetracycline in acidic pH (pH < 5), which mimics the tumor microenvironment. The tetracycline-loaded nanocomposite could also exhibit antibacterial activity against both Gram-positive and Gram-negative bacteria.37
Immunotherapy
Immunotherapy is the use of substances that stimulate or enhance the immune system to fight cancer cells or tumors. ZIF-8 can be combined with immunomodulators or adjuvants to improve its immunogenicity and efficacy. For instance, Li et al. synthesized a core–shell structure of ZnO@ZIF-8@AuNPs@RGD peptides, where AuNPs are gold nanoparticles that can act as contrast agents for magnetic resonance imaging (MRI), RGD peptides are arginine–glycine–aspartic acid peptides that can bind to integrin receptors on cancer cells, and ZIF-8 is a porous MOF that can load anticancer drugs such as doxorubicin (DOX). They showed that the core–shell structure could enhance the MRI detection of tumors, increase the uptake of DOX by cancer cells via RGD-mediated endocytosis, and improve the therapeutic effect of DOX by reducing its toxicity.20
Stem cell therapy
Stem cell therapy is a type of treatment that uses stem cells, which are cells that can change into different kinds of cells, to repair or replace damaged tissues or organs. ZIF-8 is a material that has a lot of tiny pores and can hold other substances inside it. ZIF-8 can be used as a scaffold or a carrier for stem cells, which means it can provide a structure or a space for the stem cells to grow and function. For example, ZIF-8 can be used to wrap around dental pulp stem cells (DPSCs) and deliver growth factors to them.38 Growth factors are proteins that help the stem cells to multiply and differentiate into osteoblasts, which are cells that make bone. ZIF-8 can also be used to wrap around mesenchymal stem cells (MSCs) and deliver anti-inflammatory cytokines to them. Anti-inflammatory cytokines are molecules that reduce inflammation and help the stem cells to modulate their function, which means they can change their behavior according to the situation.20,39–43
Radiochemotherapy
ZIF-8 can be modified with drugs that can enhance their delivery and effectiveness in radiotherapy. For example, Du et al. designed a novel drug delivery system, [Dbait-ADM@ZIF-8]OPM, which uses osteosarcoma-platelet hybrid membranes to encapsulate ZIF-8 coated with Dbait and Adriamycin. Dbait is a radiosensitizer that can increase the radiosensitivity of tumor cells by generating reactive oxygen species (ROS). Adriamycin is a chemotherapeutic agent that can induce apoptosis of tumor cells by inhibiting microtubule formation. The combination of Dbait and Adriamycin showed potent anti-tumor effects in tumor-bearing mice with almost no significant biotoxicity.44
Gene therapy
Gene therapy is a promising field of medicine that involves the use of genetic material to alter or correct the function of cells or tissues. One such method involves the use of zeolitic imidazolate framework-8 (ZIF-8), a type of metal–organic framework, as a gene delivery vehicle. ZIF-8 can carry various types of genes, including miRNA, siRNA, mRNA, and DNA. For instance, researchers have synthesized ZIF-8 on a ZnO surface and loaded it with miR-491-5p, a small non-coding RNA known to inhibit tumor growth by targeting oncogenes. This ZIF-8@ZnO nanocomposite was found to release miR-491-5p stably over an extended period, enhancing the efficiency of miR-491-5p in inhibiting tumor growth both in vitro and in vivo.20
Targeted therapy
In the targeted therapy, ZIF-8 can be modified with specific ligands or antibodies to target specific diseases, including cancer. For example, ZIF-8 can be loaded with doxorubicin (DOX) and verapamil (VER), two drugs used in cancer treatment, to enhance the anti-cancer effect and overcome multidrug resistance.45 Additionally, ZIF-8 can be conjugated with folate to deliver methotrexate (MTX), a drug used to treat leukemia.46 As for targeted radiotherapy, ZIF-8 can also be modified with specific ligands or antibodies to target cancer cells or other diseases. Similar to the gene therapy application, ZIF-8@ZnO nanocomposite loaded with miR-491-5p has been shown to stably release miR-491-5p over a long period and greatly improve the efficiency of miR-491-5p in inhibiting tumor growth in vitro and in vivo.20 In summary, ZIF-8 holds great potential in the fields of gene therapy, targeted therapy, and targeted radiotherapy due to its versatility and ability to be modified for specific applications. However, more research is needed to fully realize its potential and address any challenges that may arise. ZIF-8 can be employed to encapsulate photosensitizers, enabling targeted and controlled delivery. For example, a research study outlined a therapeutic agent, ZIF-8@Ce6-HA, which was created by combining glucose oxidase (GOX) and gold nanospheres (AuNPs) loaded with Chlorin e6 (Ce6), a photosensitizer frequently used, with zeolitic imidazolate framework-8 (ZIF-8). This platform is designed to leverage the unique micro-environment of osteosarcoma (OS) cells, characterized by low pH and high reactive oxygen species (ROS), to achieve precise drug delivery.47
Starvation therapy and photothermal therapy
A nanoparticle platform, referred to as ZIF-8@GA, was developed by encapsulating glucose oxidase (GOX) and gold nanospheres (AuNPs) loaded with dihydroartemisinin (DHA) within ZIF-81. This platform is designed to leverage the unique micro-environment of osteosarcoma (OS) cells, characterized by low pH and high reactive oxygen species (ROS), to achieve precise drug delivery.37
Chemotherapy and photodynamic therapy
In a recent study, ZIF-8 was synthesized on a ZnO surface, resulting in a ZnO@ZIF-8 structure. This structure demonstrated a high drug-loading efficacy of ciprofloxacin within the pore network of ZIF-82, leading to a controlled release and high antimicrobial efficiency of ciprofloxacin.48
III. Unveiling the secrets of synthesis of ZIF-8 nanoparticles
Room temperature solution reaction method
The room temperature solution reaction method for synthesizing ZIF-8. This method is quite straightforward. The first step involves preparing two separate solutions. The first solution is made by dissolving zinc nitrate in deionized water. The second solution is made by dissolving 2-methylimidazole in ammonium hydroxide. The next step is to slowly add the zinc nitrate solution to the 2-methylimidazole solution while stirring. This ensures that the two solutions are thoroughly mixed together. After about an hour of stirring, the mixture turns into a milky suspension. This change in appearance indicates that NZIF-8 nanoparticles have formed. These nanoparticles are the desired product of this synthesis method. An interesting feature of these NZIF-8 nanoparticles is their ability to be loaded with certain drugs, such as gentamicin. This is done by immersing the nanoparticles in a solution of the drug, allowing the drug molecules to diffuse into the pores of the nanoparticles. Once loaded with the drug, these nanoparticles can release the drug in response to changes in pH. This makes them potentially useful for targeted drug delivery applications, where the drug is only released when the nanoparticles reach a specific part of the body with a certain pH level. This method is advantageous because it can be carried out at room temperature, making it energy-efficient. Additionally, the use of water as a solvent makes this method environmentally friendly. The resulting NZIF-8 nanoparticles have potential applications in the field of medicine, particularly in targeted drug delivery.20
Solvothermal method
The process begins by preparing two separate solutions in methanol: one of zinc nitrate and the other of 2-methylimidazole. These two solutions are then mixed together under ultrasonic conditions. The use of ultrasonic waves helps to ensure a thorough mixing of the two solutions. After mixing, the solution is centrifuged. The product of the centrifugation is then dispersed in methanol to create a new solution. This new solution is then mixed with another methanol solution of zinc nitrate. This step is crucial for the formation of the ZIF-8 structure. The mixed solution is transferred to a Teflon-lined stainless steel autoclave, where it undergoes a solvothermal reaction. This reaction occurs under specific temperature and pressure conditions within the autoclave. The final product of this process is a hollow ZIF-8 with a good crystal structure. The hollow structure of the ZIF-8 allows it to be loaded with various substances. For instance, the hollow ZIF-8 can be loaded with drugs which makes the ZIF-8 a potential carrier for targeted drug delivery applications.49
Microwave-assisted method
The process begins by preparing two separate solutions in methanol: one of zinc nitrate and the other of 2-methylimidazole. These two solutions are then mixed together in a microwave reactor. The use of microwave energy helps to ensure a thorough mixing of the two solutions and accelerates the reaction process. After the microwave-assisted reaction, the solution is centrifuged. The product of the centrifugation is then washed to remove any unreacted materials or by-products. The final product of this process is ZIF-8 nanoparticles. These nanoparticles are the desired product of this synthesis method. The Microwave-assisted method is advantageous because it can significantly reduce the reaction time and improve the yield of ZIF-8. This is due to the fact that microwave energy can provide uniform and rapid heating, which can accelerate the reaction process and improve the efficiency of the synthesis.50
Sonochemical method
The process begins by dissolving zinc nitrate in water. Then, 2-methylimidazole is added to an organic solvent. The two solutions are then mixed together and sonicated. Sonication is the act of applying sound energy to agitate particles in a solution. The time and temperature of sonication can be adjusted depending on the specific requirements of the synthesis. After sonication, the mixture is centrifuged. Centrifugation is a process that involves the use of the centrifugal force for the separation of mixtures. The product of the centrifugation is then washed to remove any unreacted materials or by-products. The final product of this process is ZIF-8 nanoparticles. These nanoparticles are the desired product of this synthesis method. The Sonochemical method is advantageous because it can enhance the crystallinity and stability of ZIF-8. The extent of crystallinity has a big influence on hardness, density, transparency and diffusion.20
Mechanochemical method
The process begins by grinding zinc nitrate with 2-methylimidazole. This is done in an organic solvent under the application of mechanical energy. The mechanical energy helps to break down the materials and mix them together at a molecular level. The grinding process leads to a reaction between zinc nitrate and 2-methylimidazole, resulting in the formation of ZIF-8. The product of this reaction is in the form of nanoparticles. After the reaction, the mixture is filtered to separate the ZIF-8 nanoparticles from the solvent. The nanoparticles are then washed to remove any unreacted materials or by-products. The ZIF-8 nanoparticles produced by this method have a high surface area and porosity. These characteristics make them suitable for various applications, such as gas storage and separation, and drug delivery. The Mechanochemical method is advantageous because it is a simple and efficient way to produce ZIF-8. It does not require any special equipment or conditions, and it can be easily scaled up for industrial production.51
Microfluidic synthesis method
The process begins by preparing two separate solutions in methanol: one of zinc nitrate and the other of 2-methylimidazole. These solutions are then injected into a microfluidic reactor. A microfluidic reactor is a device that allows for precise control over fluid flow at a very small scale. This can enhance the mixing of the solutions and the efficiency of the reaction. The product suspension that forms in the reactor is continuously collected. This suspension is then centrifuged, a process that separates the ZIF-8 nanoparticles from the rest of the solution. The result of the centrifugation is ZIF-8 nanoparticles. These nanoparticles are the desired product of this synthesis method. This method can also be used to prepare ZIF-8@SiO2 composite nanoparticles. These composite nanoparticles combine the properties of ZIF-8 and silica (SiO2), resulting in particles with good stability and biocompatibility. The microfluidic synthesis method is advantageous because it allows for precise control over the reaction conditions, which can lead to a higher quality product. It also allows for the continuous production of ZIF-8 nanoparticles, making it suitable for large-scale production.52
IV. Developing strategies for functionalizing ZIF-8 for targeted delivery
A multitude of strategies have been investigated to functionalize ZIF-8 for targeted drug delivery in cancer treatment. One such approach involves the conjugation of targeting ligands, such as antibodies or peptides, onto the surface of ZIF-8 nanoparticles.20,53 These ligands are not just decorative elements; they serve a crucial function. They can specifically recognize and bind to receptors that are overexpressed on cancer cells, acting like a homing beacon that guides the nanoparticles to their intended destination. This promotes the selective uptake and internalization of the nanoparticles. In other words, it's not just about getting the drugs to the tumor site; it's about getting them into the cancer cells themselves. This targeted approach is like having a GPS for drug delivery, ensuring that the therapeutic agents reach their intended destination and do not get lost along the way.54,55 For instance, a study demonstrated that ZIF-8 nanoparticles could be readily functionalized with targeting ligands for the identification and absorption of particular cancer cells, enhancing the efficacy of chemotherapeutic medicines and reducing adverse effects.56 Another research highlighted that when a nanocarrier modified with specific targeting ligands is introduced, it can be readily detected by the receptor that is overexpressed on tumor cells. These cells can then identify, bind to, and quickly internalize the nanocarrier through processes such as receptor-mediated endocytosis or tumor cell phagocytosis.56 Hence, functionalizing ZIF-8 with targeting ligands is like equipping it with a sophisticated navigation system. It's not just about getting from point A to point B; it's about ensuring that every step of the journey is as efficient and effective as possible.
An innovative approach that has been explored involves the modification of ZIF-8 with components that respond to stimuli. This strategy incorporates linkers or coatings that are responsive to specific stimuli. For instance, according to Wang et al., ZIF-8 materials can be designed for single-stimulus and multi-stimulus response release of drugs. In a study by Utonova et al., it was found that the degradation rate of ZIF-8 microcrystals was affected by the presence of lactic acid, a substance particularly secreted by tumor cells. This suggests that ZIF-8 could potentially be modified to respond to the acidic conditions within the tumor microenvironment (TME), triggering the release of encapsulated drugs. In another study by Mazloum-Ardakani et al., ZIF-8 was successfully loaded with the hydrophobic anti-cancer drug 5-fluorouracil (5-FU), and the hydrophilic drugs ciprofloxacin (CF) and metronidazole (MZ) using a one-pot process. The 5-FU-loaded ZIF-8 composites demonstrated pH-sensitive properties, releasing the drug in response to the acidic conditions in the TME. These examples illustrate the versatility of ZIF-8 as a drug delivery system. By responding to the unique conditions of the TME, ZIF-8 ensures targeted and efficient drug delivery, potentially improving therapeutic outcomes and minimizing side effects.20,34,57
Studies have shown that ZIF-8 nanoparticles can be strategically altered with components that react to specific stimuli, thereby enhancing their capacity to identify and bind to certain cancer cells. This modification technique amplifies the potency of chemotherapy drugs and reduces side effects. For example, in research conducted by Zhang et al., ZIF-8 was utilized as a drug delivery mechanism for the transport and discharge of the telomerase inhibitor BIBR 1532. The study discovered that ZIF-8 could modify the permeability of the lysosomal membrane, likely due to the protonation effect of the imidazole ring. This modification enabled the cellular uptake and discharge of BIBR 1532, resulting in a higher accumulation of the drug in the nucleus. Consequently, the ZIF-8 encapsulated BIBR 1532 exhibited a more pronounced inhibition of cancer cell growth compared to free BIBR 1532. This research highlights the potential of ZIF-8 as a drug delivery vehicle for enhancing the transport, release, and effectiveness of water-insoluble drugs.48 When a nanocarrier that responds to specific stimuli is introduced, it can be readily identified by the receptor that is overexpressed on tumor cells. These cells can then identify, bind to, and quickly internalize the nanocarrier through processes such as receptor-mediated endocytosis or tumor cell phagocytosis. This method allows for the release of drugs at specific sites, further enhancing therapeutic effectiveness.20,34,57 As previously stated, ZIF-8, responsive to single and multi-stimulus, can be modified to react to TME's acidic conditions due to lactic acid. A study loaded ZIF-8 with 5-FU, CF, and MZ, demonstrating pH-sensitive drug release in the TME. This underscores ZIF-8's potential in enhancing therapeutic outcomes and reducing side effects.
In addition to its standalone capabilities, ZIF-8 can also be combined with other nanomaterials, such as liposomes or polymeric nanoparticles, to create hybrid systems that exhibit synergistic properties. These hybrid systems are not just a simple sum of their parts; they leverage the advantages of both ZIF-8 and the additional nanomaterials.20,33,58–60 A recent study has shown that ZIF-8 nanoparticles can be effectively combined with other nanomaterials for the detection and capture of specific cancer cells. This enhances the effectiveness of chemotherapy drugs and minimizes side effects.20 Another study emphasized that when a hybrid nanocarrier is introduced, it can be readily detected by the receptor that is overexpressed on tumor cells. These cells can then identify, bind to, and quickly internalize the nanocarrier through processes such as receptor-mediated endocytosis or tumor cell phagocytosis.58 This enables enhanced drug loading capacity, improved stability, and targeted delivery. In essence, combining ZIF-8 with other nanomaterials is like creating a super team; each member brings its unique strengths to the table, resulting in a system that is more effective than any of its individual components.
In summary, ZIF-8 is a versatile nanoparticle with unique properties that make it an excellent candidate for drug delivery in cancer treatment. Its high surface area, tunable pore size, and stability allow for efficient encapsulation and controlled release of therapeutic agents. By functionalizing ZIF-8, targeted drug delivery can be achieved, enhancing the selectivity and efficacy of cancer treatment. The integration of ZIF-8 in immunotherapy holds great promise for advancing the field and improving patient outcomes.
V. Examining PDL1 as an important immune checkpoint in cancer
PD-L1 is a pivotal player in the complex game of immune evasion and tumor progression.61,62 Often, cancer cells, as if donning a cloak of invisibility, upregulate PD-L1 as a result of various genetic and epigenetic alterations.8,63 This upregulation is not a random act but a strategic move in the battle against the body's immune system. When PD-L1 interacts with its receptor, PD-1, on immune cells, it's akin to throwing a wrench in the works of the immune response. This interaction promotes immune tolerance towards cancer cells, effectively turning off the alarm system that would typically alert the body to the presence of these rogue cells.61,62 The interaction between PD-L1 and PD-1 is not a benign handshake; it leads to the suppression of T-cell activity. Imagine T-cells as soldiers on the front lines of defense against cancer cells. The PD-L1 and PD-1 interaction effectively disarms these soldiers, preventing the immune system from effectively recognizing and eliminating cancer cells.61,62 This immune evasion mechanism allows tumors to grow and progress unchecked, much like a wolf in sheep's clothing. Moreover, PD-L1 doesn’t just help cancer cells hide; it also paints a target on them. PD-L1 expression in the TME is associated with poor prognosis and resistance to conventional cancer therapies.63,64 It's like a double-edged sword - while it helps cancer cells evade immediate detection, its presence could also signal more aggressive disease and resistance to treatment. Hence, understanding the role and regulation of PD-L1 in cancer is akin to deciphering enemy tactics in warfare. It provides valuable insights into how cancer cells evade the immune system and offers potential targets for more effective cancer therapies.
VI. Addressing challenges in PD-L1/PD-1 blockade cancer immunotherapy
PD-L1 has emerged as a significant factor in immune evasion in the cancer immunotherapy, thereby becoming an essential target. The interaction mechanism between PD-L1 and PD-1 enables cancer cells to slip past the immune system. By blocking this interaction, the immune system's ability to identify and combat cancer cells is restored. Monoclonal antibodies, specifically engineered as immune checkpoint inhibitors, have been created to target the PD-L1/PD-1 pathway.65,66 Two such inhibitors, pembrolizumab and nivolumab, have demonstrated substantial effectiveness. They inhibit the interaction between PD-L1 and PD-1, thus empowering the immune system to identify and attack cancer cells. However, the efficacy of these treatments is not uniform among patients due to the intricate relationship between the immune system and cancer.67 To boost the therapeutic effectiveness of PD-L1/PD-1 blockade, we need to investigate novel strategies. One such strategy involves the use of ZIF-8, a metal–organic framework, which could potentially act as a delivery system for these treatments, enhancing their stability and targeted delivery to tumor cells.20
Moreover, the combination of PD-L1 inhibitors with other treatments such as chemotherapy, radiation, or other immunotherapies can lead to a synergistic effect, thereby enhancing the overall antitumor response. These combinations, along with targeted delivery systems, could potentially overcome the challenges presented by resistance mechanisms, such as the activation of alternative immune checkpoints and tumor heterogeneity.61 While PD-L1/PD-1 blockade has revolutionized cancer treatment, resistance to this therapy remains a significant challenge. However, with the application of synergistic approaches, ZIF-8, and targeted delivery, we could potentially overcome these challenges and pave the way for a new era of effective cancer immunotherapy.68,69
Despite these advancements, ongoing research is necessary to improve these treatments and extend their efficacy to a wider range of patients. The rise of resistance mechanisms and the complex nature of tumor heterogeneity present significant challenges to the effectiveness of PD-L1 inhibition. However, with the application of synergistic approaches and targeted delivery, we could potentially overcome these challenges and usher in a new era of effective cancer immunotherapy.17
VII. Exploring benefits of combining ZIF-8 and PDL1 inhibition in cancer treatment
Integrating ZIF-8 with PDL1 inhibition holds great potential for improving the outcomes of cancer treatment. By combining ZIF-8, a versatile nanoparticle for drug delivery, with PDL1 inhibitors, several benefits can be achieved.7,48,70 Firstly, ZIF-8 can serve as a carrier for PDL1 inhibitors, protecting them from degradation and facilitating their targeted delivery to the tumor site.48,70 This targeted delivery approach can enhance the accumulation of PDL1 inhibitors in the TME, maximizing their therapeutic effects while minimizing systemic toxicity.7,70 Secondly, ZIF-8 can be functionalized to enhance the selectivity and specificity of PDL1 inhibition. Surface modifications of ZIF-8 nanoparticles with targeting ligands or antibodies can facilitate the specific recognition and binding to cancer cells overexpressing PDL1.71,72 This targeted approach increases the local concentration of PDL1 inhibitors at the tumor site, improving their efficacy in blocking the PDL1/PD1 interaction and restoring the immune response against cancer cells.7,73 Furthermore, the combination of ZIF-8 and PDL1 inhibition can potentially overcome resistance mechanisms associated with PDL1/PD1 blockade alone. By delivering PDL1 inhibitors directly to the tumor site using ZIF-8, alternative immune checkpoints or heterogeneous tumor populations can be targeted more effectively, enhancing the overall antitumor immune response.73–75 In conclusion, targeting PDL1 in cancer immunotherapy has shown significant clinical benefits. By integrating ZIF-8 with PDL1 inhibition, the targeted delivery and enhanced efficacy of PDL1 inhibitors can be achieved, potentially overcoming resistance mechanisms and improving patient outcomes. The combination of ZIF-8 and PDL1 inhibition represents a promising approach in advancing cancer treatment and harnessing the full potential of immunotherapy.
VIII. Investigating targeted delivery systems for immunotherapy
Targeted delivery systems play a crucial role in improving the efficacy and safety of immunotherapy in cancer treatment. These systems aim to selectively deliver therapeutic agents, such as ZIF-8 and PDL1 inhibitors, to the tumor site while minimizing off-target effects.33,76 Various approaches have been developed to achieve targeted delivery, including passive targeting,20,77 active targeting,7,76,77 and stimuli-responsive systems.34,78–81 Passive targeting relies on the enhanced permeability and retention (EPR) effect, which takes advantage of the leaky vasculature and impaired lymphatic drainage in tumors. Nanoparticles, such as ZIF-8, can accumulate in the tumor through passive targeting, allowing for prolonged drug release and increased drug concentrations at the tumor site.20,77 Active targeting involves the functionalization of nanoparticles with ligands or antibodies that specifically recognize and bind to receptors overexpressed on cancer cells. This approach enhances the specificity and selectivity of drug delivery, improving the therapeutic index of immunotherapy. Ligands or antibodies targeting PDL1 can be conjugated to ZIF-8 nanoparticles, facilitating the targeted delivery of PDL1 inhibitors to PDL1-expressing cancer cells.7,76,77 Stimuli-responsive delivery systems utilize external or internal triggers to release therapeutic agents at the tumor site. These triggers can include changes in pH, temperature, or enzyme activity in the TME. By incorporating stimuli-responsive components into ZIF-8 nanoparticles, controlled drug release can be achieved, ensuring that PDL1 inhibitors are released specifically in the TME.34,78–81
Targeted delivery systems have emerged as a game-changer in the field of immunotherapy, offering a multitude of advantages.17,82,83 One of the key benefits is the enhancement of the therapeutic index, a measure of a drug's safety in relation to its effectiveness.84,85 By increasing drug concentrations specifically at the tumor site and reducing off-target effects, targeted delivery systems can significantly improve this index.17,82,83 Moreover, these systems pave the way for the administration of lower drug doses. This approach not only minimizes systemic toxicity but also improves patient tolerability, striking a balance between efficacy and safety.86,87 Perhaps one of the most groundbreaking aspects of targeted delivery systems is their ability to overcome biological barriers, such as the blood–brain barrier. These barriers have traditionally posed significant challenges in delivering therapeutic agents to certain sites within the body. However, with targeted delivery systems, it is now possible to deliver these agents to previously inaccessible sites, opening new frontiers in disease treatment.84,88
Targeted delivery systems, particularly those utilizing nanoparticles, have shown immense potential in the realm of cancer treatment. However, these systems are not without their challenges.84,89,90 One of the primary hurdles lies in the complexity of designing and synthesizing nanoparticles with the desired properties. The creation of nanoparticles requires careful consideration of factors such as size, stability, and surface functionality.91,92 For instance, studies by Hashemzadeh et al. highlighted the intricate process of nanoparticle synthesis and the challenges associated with achieving the desired physicochemical properties.93–98 Another challenge stems from the inherent heterogeneity of tumors and variability in receptor expression. Tumors are not uniform entities; they consist of diverse cell populations with different molecular and phenotypical profiles. This diversity can affect the efficacy of targeted delivery, as the nanoparticles may not uniformly bind to or be taken up by all cancer cells.99,100 Furthermore, the clearance of nanoparticles by the reticuloendothelial system (RES) poses another significant challenge. The RES, which includes immune cells like macrophages, is responsible for removing foreign substances from the body. When nanoparticles are introduced into the body, they are often quickly recognized and cleared by the RES. This clearance can limit the circulation time of the nanoparticles and reduce their accumulation at the tumor site.101,102 Thus, while targeted delivery systems hold great promise for improving cancer treatment, it is crucial to address these challenges to fully realize their potential. Future research should focus on developing strategies to overcome these hurdles and optimize the design and functionality of nanoparticle-based delivery systems.
The incorporation of targeting ligands or antibodies in targeted delivery systems indeed presents a significant challenge due to the potential for immunogenicity and toxicity. These foreign substances may be recognized by the immune system, triggering an immune response. This reaction can lead to adverse effects, emphasizing the need for careful selection of ligands or antibodies. The selection process must ensure that these entities exhibit specificity, stability, and compatibility with the immune system.103–105 Moreover, in addition to these considerations, it becomes imperative to comprehensively evaluate the long-term safety and biocompatibility of precision delivery systems. Since these systems employ nanoparticles, they interact with various biological systems within the body. Therefore, it is of paramount importance to assess their long-term effects on health and potential interactions with other biological systems.106,107 Nanoparticles possess remarkable capabilities due to their small size and distinctive properties, enabling them to breach biological barriers and interact with cells at the molecular level.108 While this enhances the precise delivery of therapeutic agents, it also raises concerns regarding unforeseen long-term health implications.109 For instance, the accumulation of nanoparticles in specific organs or tissues may lead to unforeseen health complications over time.110,111 Hence, ensuring the safety and efficacy of these precision delivery systems requires stringent testing and vigilant monitoring. This encompasses conducting in-depth in vitro studies, utilizing animal models, and eventually designing meticulously controlled clinical trials.112,113 By addressing these challenges, the full potential of precision delivery systems in enhancing patient outcomes can be realized.
In conclusion, targeted delivery systems play a crucial role in improving the efficacy and safety of immunotherapy in cancer treatment. Passive targeting, active targeting, and stimuli-responsive systems can offer different approaches for achieving targeted delivery of ZIF-8 and PDL1 inhibitors. While these strategies offer advantages in enhancing drug delivery to the tumor site, challenges related to design complexity, tumor heterogeneity, and potential immunogenicity need to be addressed. Further research and development in targeted delivery systems are necessary to optimize their performance and maximize their therapeutic potential in immunotherapy.
IX. Utilizing ZIF-8 for targeted delivery of PD-L1 in immunotherapy
Analyzing different ZIF-8/PDL1 delivery systems in immunotherapy
The utilization of ZIF-8-based nanoparticles as a drug carrier in the context of cancer therapy, with a specific focus on their potential in the realm of immunotherapy, is a subject of great significance (Table 1). ZIF-8, characterized by its porous nature, high drug loading capacity, and sensitivity to pH-induced degradation, emerges as a promising candidate for facilitating targeted drug delivery within the intricate landscape of cancer treatment. The unique attributes of ZIF-8 nanoparticles, encompassing their remarkable drug-loading capabilities and pH-responsive degradation, make them a compelling choice for targeted drug delivery. These nanoparticles can be synthesized via in situ synthesis and physical adsorption techniques, offering versatility in their production. In the context of this study, ZIF-8 nanoparticles served as an instrumental drug carrier for precise and targeted delivery in the realm of cancer therapy.23,114–122
Table 1 Overview of studies highlighting different ZIF-8/PDL1 delivery systems in immunotherapy
Nanoparticle |
Drug |
Goal |
Cellular model |
Cellular uptake |
Animal model |
Animal |
Outcome |
Ref. |
ZIF-8 |
PDL1 siRNA |
Combination of ZIF-8 nanoparticles and PDL1 inhibition in cancer immunotherapy to enhance antitumor effects and modulate the TME |
4T1/GFP/FlucII cell line |
Confocal laser scanning microscopy (CLSM) |
Subcutaneous tumor and lung metastasis models |
8-week-old female C57BL/6 mic |
Improved antitumor effects, immune responses, and modulation of the TME; enhanced tumor growth inhibition, immune cell activation, and increased apoptosis and necrosis rates were observed; the integrated approach of ZIF-8, PDL1 inhibition, and targeted delivery holds promise for advancing immunotherapy in cancer treatment |
123
|
Free miR-34a, ZIF-8, OMV@ZIF-8@miR-34a nanoparticles |
miR-34a |
The effectiveness of OMV@ZIF-8@miR-34a nanoparticles for targeted miRNA delivery and enhanced tumor therapy |
4T1 cells (murine breast cancer cell line) |
Fluorescence imaging |
Mice were injected with 4T1 cells to induce tumor growth |
Female BALB/c mice (6 weeks old, 16–22 g) |
The engineered nanoparticles had high miRNA delivery efficiency and accurate tumor targeting; the combination of miR-34a payloads in the nanoparticles with immune activation and checkpoint inhibition resulted in synergistic tumor therapeutic efficacy; the nanoparticles showed improved antitumor effects, survival rates, immune cell activation, and modulation of the TME; the nanoparticles exhibited stability, efficient cellular uptake, and distribution within tumor tissues |
124
|
EPI, EZ, GHZ, EHGZ, mEHGZ nanoparticles |
EPI, Gox, hemin, anti-PD-L1 antibody |
The development of a nanoparticle-based drug delivery system for cancer therapy and evaluate its effectiveness in inhibiting tumor growth and inducing ICD |
4T1 murine breast cancer cell line |
Flow cytometry, CLSM, immunofluorescence staining |
4T1-bearing mic |
Male C57BL/6 mice at 4–6 weeks |
mEHGZ nanoparticles exhibited enhanced cellular uptake efficiency compared to other formulations; treatment with mEHGZ nanoparticles resulted in significant inhibition of tumor growth; mEHGZ nanoparticles induced ICD, as indicated by increased CRT expression; Combination of mEHGZ nanoparticles with anti-PD-L1 antibody activated the immune response |
148
|
ZIF-8 with bone/tumor dual-targeted ligand HA/ALN (CLALN) |
Curcumin (Cur) |
The evaluation of the potential application of bone and tumor dual-targeted liquid metal nanoparticles (LM NPs) in treating bone metastasis |
4T1 cells |
Fluorescence imaging |
Bone metastasis model |
BALB/c mice |
Improved tumor therapeutic effects; inhibition of PD-L1 protein expression; the modulation of the immunosuppressive microenvironment |
149
|
KN046@19 F-ZIF-8 |
Rhodamine B, KN046 |
The enhancement of the immune response rate, reduce side effects, and improve the therapeutic efficacy of KN046 in cancer treatment |
Murine B16F10 melanoma cell line |
CLSM, 19F MRI |
Subcutaneous B16F10 melanoma tumors |
BALB/c mice (6–8 weeks old, 18–20 g) |
The design, synthesis, and characterization of the nanoprobe, as well as its potential benefits in enhancing the immune response and reducing side effects; improved therapeutic efficacy; 19F MRI – fluorine signal turned on when ZIF-8 nanoparticles were destroyed, indicating antibody release – increased 19F MRI signal intensity with decreased pH and increased GSH concentration, demonstrating nanoprobe responsiveness |
23
|
ZIF-8 nanoparticles coated with polydopamine and PEG |
EGCG |
Efficient delivery of EGCG, a potent antioxidant found in tea, to the TME |
Human malignant melanoma cells (A375 cells), tumor-conditioned media (CM) from A375 cells |
— |
B16F10 melanoma mouse model |
C57BL/6J mice |
Significant inhibition of PD-L1 expression in tumor cells; effective reduction of IL-1β secretion in the TME; significant accumulation of nanoparticles in tumor tissue; suppression of tumor growth and improved survival rates in animal models; decrease in myeloid-derived suppressor cells and tumor-associated macrophages; increase in T regulatory cells, DCs, and CD3+ T cells |
150
|
AZ-P and AZ-P@P |
Anti-PD-L1 antibodies, gold nanorods (AuNRs) |
Efficient delivery of anti-PD-L1 antibodies; PTT; controlled release of drugs |
4T1 murine breast tumor cell, bone marrow-derived DCs (BMDCs) |
Inductively coupled plasma mass spectrometry (ICP-MS) |
4T1-Luc tumor-bearing mice |
Naive BALB/c mic |
Nanosystem functions: PTT, controlled release of anti-PD-L1 antibodies, tumor antigen capture; blocking PD-L1/PD-1 interaction, preventing immune evasion, activating cytotoxic T lymphocytes; NIR-triggered heating demonstrated cytotoxicity against cancer cells; ATP-triggered release of anti-PD-L1 antibodies from the nanosystem; strong antigen-capturing capability of the nanosystem; enhanced immune response and inhibition of tumor growth in mice models |
155
|
ZIF-8 nanoparticles have been recently used as carriers for PDL1 siRNA, using a blend of in situ synthesis and physical adsorption. The resulting compound, IM@ZP, allows for the simultaneous delivery of PDL1 siRNA and ZIF-8 nanoparticles to the tumor site, aiming to enhance the antitumor immune response (Fig. 3). The study's hypothesis is based on the interaction between ZIF-8 and PDL1, which could amplify the antitumor immune response. The research involved cellular and animal models to evaluate the effects of ZIF-8-based nanoparticle delivery and PDL1 inhibition. The 4T1 cell line was used to assess the uptake and intracellular distribution of ZIF-8 nanoparticles (Fig. 4). Animal models were used to validate these findings and assess the therapeutic outcomes of ZIF-8-based nanoparticle delivery coupled with PDL1 inhibition (Fig. 5).123
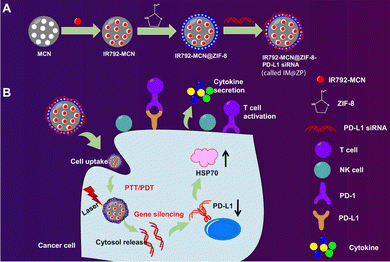 |
| Fig. 3 ZIF-8 nanoparticles are portrayed as having a porous structure with a high loading capacity, allowing for efficient encapsulation and delivery of therapeutic agents. (A) The synthesis process of the nanoparticles is shown, with PDL1 siRNA being loaded onto the ZIF-8 nanoparticles through in situ synthesis and physical adsorption methods. The uptake of the nanoparticles by 4T1 cancer cells is emphasized, demonstrating their therapeutic potential. (B) The release behavior of siRNA from the nanoparticles is depicted, indicating a rapid release under acidic conditions and a stable release over time. Enhanced photothermal effects resulting from the combination of mesoporous carbon nanocomposite (MCN) and IR792 molecules, a near-infrared (NIR) laser dye, are highlighted, suggesting that exposure to NIR laser irradiation generates heat, leading to increased cell death in cancer cells. The therapeutic efficacy of the ZIF-8-based nanoparticle system combined with PDL1 inhibition is demonstrated, showing increased cell death in cancer cells under NIR laser irradiation and an enhanced immune response.123 (Wang Y, Wang H, Song Y, Lv M, Mao Y, Song H, et al. Journal of Nanobiotechnology, 2022, 20(1) Licensed under a Creative Commons Attribution 4.0 International License (CC BY 4.0). [http://creativecommons.org/licenses/by/4.0/].). | |
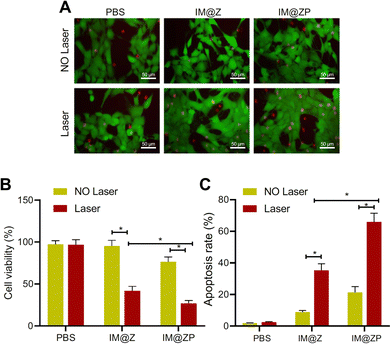 |
| Fig. 4 The effects of the IR792-MCN@ZIF-8-PD-L1 siRNA (IM@ZP) nanoparticle system on cancer cells. Figure (A) compares the photothermal killing ability of different treatment groups, demonstrating that IM@ZP, especially when combined with PD-L1 siRNA, significantly reduces cell viability compared to other groups, indicating enhanced photothermal killing ability. Figure (B) presents the results of a CCK-8 assay measuring cell viability. It shows good biocompatibility with IM@Z, but a slight decrease in cell viability with IM@ZP, suggesting that PD-L1 siRNA may slightly impact cell viability. Figure (C) illustrates the combined effect of photothermal therapy (PTT) and PD-L1 silencing on tumor growth inhibition. The most significant reduction in tumor volume is observed in the IM@ZP + NIR group, indicating that combining PTT and PD-L1 silencing improves therapeutic efficacy in inhibiting tumor growth.123 (Wang Y, Wang H, Song Y, Lv M, Mao Y, Song H, et al. Journal of Nanobiotechnology, 2022, 20(1) Licensed under a Creative Commons Attribution 4.0 International License (CC BY 4.0). [http://creativecommons.org/licenses/by/4.0/].). | |
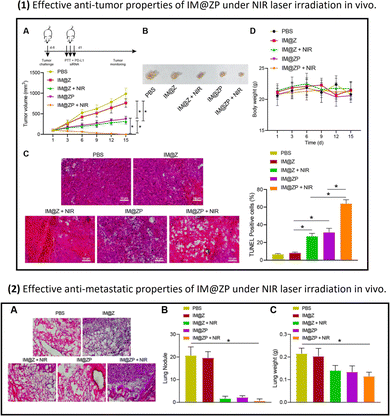 |
| Fig. 5 Part (1): The anti-tumor properties of IM@ZP (nanoparticles with PD-L1 siRNA) under NIR laser irradiation in vivo: Panel A shows that the IM@ZP + NIR group exhibits the most significant reduction in tumor volume, suggesting enhanced therapeutic efficacy. Panel B visually demonstrates the reduction in tumor size in the IM@ZP + NIR group. Panel C indicates that treatment with IM@Z + NIR or IM@ZP, especially IM@ZP + NIR, induces a higher percentage of cell apoptosis in tumor tissues. Part (2): The anti-metastatic properties of IM@ZP (nanoparticles with PD-L1 siRNA) under NIR laser irradiation in vivo. Panel A shows HE staining analysis of lung metastasis, revealing fewer metastatic nodules in the IM@ZP + NIR group, indicating reduced lung metastasis. Panel B provides quantitative data on lung metastatic nodules, demonstrating that the IM@ZP + NIR group has the fewest, suggesting effective inhibition of lung metastases formation. Panel C presents lung weight data, showing significantly lower weight in the IM@ZP + NIR group, indicating reduced metastatic burden in the lungs.123 (Wang Y, Wang H, Song Y, Lv M, Mao Y, Song H, et al. Journal of Nanobiotechnology, 2022, 20(1) Licensed under a Creative Commons Attribution 4.0 International License (CC BY 4.0). [http://creativecommons.org/licenses/by/4.0/].). | |
The combination of IM@Z and NIR laser irradiation, along with PD-L1 siRNA, promotes immune responses both in vitro and in vivo. In vitro, it enhanced the maturation of DCs, proliferation of CD8+ T cells, and production of pro-inflammatory cytokines. In vivo, it increased the infiltration of CD8+ and CD4+ T cells into the tumor tissues. These findings highlight the potential of the nanoparticle system in enhancing the immune response for cancer immunotherapy. The combined approach showed promise in enhancing antitumor effects, elevating survival rates, activating immune cells, and modulating the TME. This study underscores the potential of this innovative combination strategy, offering a pathway toward improved cancer immunotherapy outcomes.123
Another study investigated the use of microRNA (miRNA) for cancer treatment, with a focus on improving miRNA delivery and targeting within tumors. ZIF-8 was used as a carrier for miRNA, and the process involved mixing zinc nitrate hexahydrate and 2-methylimidazole in an aqueous solution at pH 8, facilitating the binding of pre-miR-34a. The mixture was dried at 60 °C under reduced pressure to produce ZIF-8 nanoparticles loaded with pre-miR-34a. The efficiency of pre-miR-34a encapsulation within ZIF-8 was assessed using varying concentrations of FAM-miR-34a. Outer membrane vesicles (OMVs) displaying PD1 were coated onto the ZIF-8 nanoparticles, creating OMV@ZIF-8@miR-34a nanoparticles capable of targeting tumor cells. ZIF-8 nanoparticles, being pH-sensitive, respond to the acidic environment in lysosomes, facilitating the release of the encapsulated miR-34a into the cytoplasm of cancer cells. The released miR-34a inhibits the expression of the target protein SIRT1, preventing the proliferation of breast cancer tissue. The OMVs stimulate immune cells to secrete interferon-gamma (IFN-γ), inducing an antitumor immune response and enhancing the anticancer activity of the nanoparticles. The interaction between PD1 on the OMVs and PD-L1 on tumor cells can block the PD1/PD-L1 pathway, further amplifying the anticancer activity. The study demonstrated the efficiency of OMV@ZIF-8@miR-34a nanoparticles in delivering miRNA with precision, leading to enhanced efficacy in cancer therapeutics. The approach was tested using a murine breast cancer model employing the 4T1 cell line, with cell viability evaluated using a CCK-8 assay and cellular uptake visualized using fluorescence imaging techniques.124
Comparing three distinct ZIF-8-based delivery systems (in Section A)
In the Section A, three distinct ZIF-8-based delivery systems have been explored:
1. ZIF-8/PDL1 siRNA (IM@ZP) nanoparticles.
This system leverages ZIF-8 nanoparticles as vehicles for PDL1 siRNA. The siRNA is affixed to the nanoparticles via in situ synthesis and physical adsorption techniques. The resulting compound, known as IM@ZP, facilitates the concurrent delivery of PDL1 siRNA and ZIF-8 nanoparticles to the tumor site. The main goal of this dual-delivery strategy is to exploit synergistic effects, thereby enhancing the antitumor immune response.
Strengths.
This system capitalizes on the ability of ZIF-8 nanoparticles to carry PDL1 siRNA, enabling concurrent delivery to the tumor site. This dual-delivery approach aims to exploit synergistic effects, potentially enhancing the antitumor immune response and boosting the overall effectiveness of immunotherapy.
Weaknesses.
The success of this system hinges on the efficiency of siRNA loading and the stability of the nanoparticles in the physiological environment. Moreover, the reliance on two methods (in situ synthesis and physical adsorption) for siRNA loading could make the production process more complex.
2. ZIF-8/Pre-miR-34a nanoparticles.
This system uses ZIF-8 as a vehicle for pre-miR-34a. The synthesis process involves the combination of zinc nitrate hexahydrate and 2-methylimidazole in a water-based solution at a specific pH level, which aids in the binding of pre-miR-34a. The resulting mixture is then dried, yielding ZIF-8 nanoparticles loaded with pre-miR-34a.
Strengths.
This system capitalizes on the ability of ZIF-8 nanoparticles to carry PDL1 siRNA, enabling concurrent delivery to the tumor site. This dual-delivery approach aims to exploit synergistic effects, potentially enhancing the antitumor immune response and boosting the overall effectiveness of immunotherapy.
Weaknesses.
The success of this system hinges on the efficiency of siRNA loading and the stability of the nanoparticles in the physiological environment. Moreover, the reliance on two methods (in situ synthesis and physical adsorption) for siRNA loading could make the production process more complex.
3. OMV@ZIF-8@miR-34a nanoparticles.
This system entails the engineering of OMVs to display PD1 on their surface. These PD1-displaying OMVs are subsequently coated onto the surface of ZIF-8 nanoparticles, resulting in OMV@ZIF-8@miR-34a nanoparticles. This setup allows for the specific targeting of tumor cells, as PD1 can specifically bind to PD-L1 present on the surfaces of these cells.
Strengths.
This system involves the engineering of outer membrane vesicles (OMVs) to display PD1 on their surface, allowing for specific targeting of tumor cells. The OMVs in this formulation have immunostimulatory properties, effectively stimulating immune cells to secrete interferon-gamma (IFN-γ), which induces a potent antitumor immune response.
Weaknesses.
The effectiveness of this system would depend on the successful display of PD1 on the OMVs and the stability of the nanoparticles. Also, the efficiency of IFN-γ secretion could impact the system's effectiveness.
Each of these systems harnesses the unique properties of ZIF-8 nanoparticles to deliver therapeutic agents (PDL1 siRNA or miR-34a) to tumor cells. However, they differ in several key areas:
• Type of therapeutic agent.
The ZIF-8/PDL1 siRNA system carries PDL1 siRNA, while the ZIF-8/Pre-miR-34a and OMV@ZIF-8@miR-34a systems carry miR-34a.
• Method of loading.
The ZIF-8/PDL1 siRNA system loads the siRNA onto the nanoparticles through in situ synthesis and physical adsorption methodologies. The ZIF-8/Pre-miR-34a system uses a specific pH level to facilitate the binding of pre-miR-34a.
• Additional components.
The OMV@ZIF-8@miR-34a system uses OMVs to enhance targeting and therapeutic efficacy.
These differences could potentially impact the effectiveness of each system in a clinical setting. For example, the type of therapeutic agent carried could influence the system's ability to target specific types of cancer cells. The method of loading could affect the efficiency of drug delivery, and the use of additional components like OMVs could enhance the system's targeting capabilities.
However, more research is needed to fully comprehend the implications of these differences. It's also vital to consider potential side effects and long-term impacts of these treatment approaches. For instance, factors such as the body's immune response to the nanoparticles, the potential for off-target effects, and the long-term stability of the nanoparticles are all important considerations. As with any new therapeutic approach, these systems would need to undergo rigorous testing in preclinical and clinical trials to assess their safety and efficacy.
Understanding immune checkpoint therapy
One of the possible applications of ZIF-8 is to deliver immune checkpoint inhibitors (ICIs), which are drugs that block the signals that prevent the immune system from attacking cancer cells.34,45,125,126 ICIs are a type of immunotherapy that can boost the immune response against tumors by releasing the brakes on T cells, which are white blood cells that can recognize and kill cancer cells. However, some cancer cells have evolved mechanisms to evade or suppress the immune system, such as expressing proteins called immune checkpoints on their surface. These proteins act as brakes on T cells, preventing them from activating and attacking the cancer cells. By blocking these proteins, ICIs can unleash the full potential of the immune system to fight cancer.125,126 ZIF-8 can be used as a carrier for ICIs by loading them into its pores or coating them with ZIF-8 material. This way, ZIF-8 can protect the ICIs from degradation or clearance by the body's enzymes or immune system, and also control their release in response to stimuli such as pH, temperature, light, or magnetic fields. ZIF-8 can also be designed to target specific types of cancer cells that express certain immune checkpoints, such as PD-L1 or CTLA-4 (cytotoxic T-lymphocyte-associated protein 4), by using antibodies or peptides that bind to these receptors.34,45 Immune checkpoint inhibitors are a type of immunotherapy that can boost the immune response against tumors by releasing the brakes on T cells, which are white blood cells that can recognize and kill cancer cells. However, some cancer cells have evolved mechanisms to evade or suppress the immune system, such as expressing proteins called immune checkpoints on their surface. These proteins act as brakes on T cells, preventing them from activating and attacking the cancer cells. By blocking these proteins, ICIs can unleash the full potential of the immune system to fight cancer.125,126 As also depicted in Fig. 6, It achieves this by targeting specific proteins called immune checkpoints that play a crucial role in regulating the activity of immune cells known as T cells. T cells are responsible for identifying and eliminating cancerous cells in the body. Two pivotal immune checkpoints that have garnered significant attention in cancer therapy are PD-1 and CTLA-4. These proteins essentially serve as “brakes” for the immune system, preventing T cells from mistakenly attacking healthy cells and helping maintain overall immune balance (Fig. 6).127–134
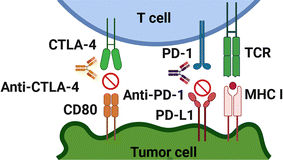 |
| Fig. 6 This figure illustrates the mechanism of immune evasion by tumor cells and the role of immune checkpoint inhibitors in reversing this process. Tumor cells upregulate PD-L1 and CD80 to evade immune surveillance. PD-1 and CTLA-4 are immune checkpoints expressed by T cells that inhibit immune responses. The interaction of these checkpoints with their ligands on tumor cells leads to immune evasion. Immune checkpoint inhibitors, such as anti-CTLA-4 and anti-PD1, can reverse this inhibition, thereby activating the immune response against tumor cells. | |
Unfortunately, cancer cells can manipulate these checkpoints to avoid detection and evade destruction by the immune system.135,136 In immune checkpoint therapy, specialized antibodies are used to disrupt the interaction between PD-1 or CTLA-4 and their respective ligands, PD-L1 or B7.135–137 These ligands are proteins found on the surfaces of either cancer cells or antigen-presenting cells (APCs).137 By interrupting these interactions, the therapy essentially removes the brakes on the immune system, enabling T cells to more effectively recognize and combat cancer cells.135,136 While targeting PD-1 or CTLA-4 individually has shown promise in treating specific cancers, combining antibodies that target both PD-L1 and CTLA-4 has demonstrated even more encouraging results. These combination therapies work synergistically, enhancing the immune response against cancer cells by addressing different checkpoints and activating distinct pathways within the immune system.138 However, it's important to acknowledge the limitations of these combination therapies, including their high costs and potential side effects. Producing and administering monoclonal antibodies can be expensive, making them less accessible to a wider population.139,140 Additionally, the immune activation triggered by these therapies can lead to immune-related adverse events, which can range from mild to severe and affect various organs in the body. ZIF-8 can be used as a carrier for ICIs by loading them into its pores or coating them with ZIF-8 material. This way, ZIF-8 can protect the ICIs from degradation or clearance by the body's enzymes or immune system, and also control their release in response to stimuli such as pH, temperature, light, or magnetic fields. ZIF-8 can also be designed to target specific types of cancer cells that express certain immune checkpoints, such as PD-L1 or CTLA-4, by using antibodies or peptides that bind to these receptors.34,45 To address these limitations, researchers are exploring innovative strategies, such as the use of smart delivery systems like ZIF-8, to enhance the efficacy and safety of immune checkpoint therapies. These delivery systems enable precise delivery of therapeutic agents to tumor sites, minimizing off-target effects and potentially reducing the required dosage, thereby mitigating side effects and lowering treatment costs.141,142 ZIF-8, in particular, serves as a smart delivery system designed to safely and effectively transport therapeutic agents, such as KN046, to tumor locations. ZIF-8 is a biocompatible and biodegradable nanoparticle that safeguards the active components of immunotherapy and ensures their accurate delivery to the tumor site.143 KN046 is an innovative recombinant humanized bispecific single-domain antibody-Fc fusion protein that targets PD-L1 and CTLA-4. It is designed to bind effectively to both PD-L1 and CTLA-4, thereby amplifying the immune response against cancer cells (Fig. 7).23,144–147
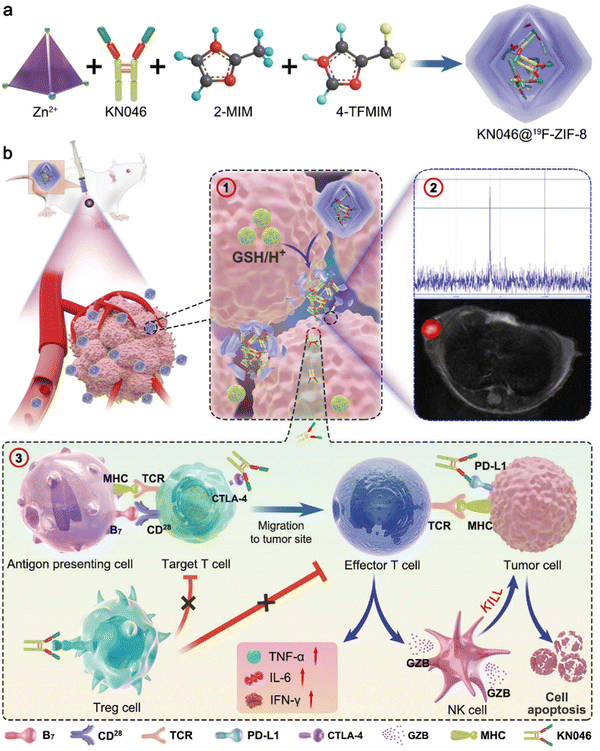 |
| Fig. 7 A schematic illustration of the formation of the KN046@19F-ZIF-8 nanoplatform. The figure is divided into two parts: (a) the formation of the nanoplatform and (b) the mechanism of dual-blockade of an immune checkpoint to enhance melanoma immunotherapy. In part (a), the formation of the nanoplatform is depicted. The process starts with the synthesis of the 19F-ZIF-8 nanoparticles, which are represented as hexagonal structures. These nanoparticles serve as the carrier for the therapeutic agent KN046. The schematic shows that KN046 is encapsulated within the 19F-ZIF-8 nanoparticles, indicated by the presence of KN046 molecules inside the hexagonal structures. This encapsulation is achieved through a surface modification of KN046 using polyvinylpyrrolidone (PVP). The modified KN046 is then loaded into the 19F-ZIF-8 nanoparticles. In part (b), the mechanism of dual-blockade of an immune checkpoint to enhance melanoma immunotherapy is illustrated. The figure shows the interaction between the nanoplatform and the TME. The nanoplatform, represented by the 19F-ZIF-8 nanoparticles loaded with KN046, is delivered to the tumor site. Upon reaching the tumor, the nanoplatform releases KN046, which can bind to both PD-L1 and CTLA-4 effectively. This dual-blockade of immune checkpoints aims to enhance the immune response against melanoma cells. The figure suggests that the nanoplatform can activate the immune system and promote an effective antitumor immune response.23 (Jiang C, Zhang L, Xu X, Qi M, Zhang J, He S, et al. Advanced Science, 2021, 8(20) Licensed under a Creative Commons Attribution 4.0 International License (CC BY 4.0). [http://creativecommons.org/licenses/by/4.0/].). | |
KN046@19F-ZIF-8, a combination of KN046 and ZIF-8, offers several advantages in cancer treatment. It boosts the immune response by activating T cells to target cancer cells more effectively. ZIF-8 serves as a delivery system, reducing side effects by ensuring KN046 reaches the tumor site safely and efficiently. The compound is designed to respond to the Tumor Microenvironment (TME), allowing for controlled release of KN046, which improves its effectiveness. The combination also enhances the pharmacokinetic efficiency, protecting the active components from immune clearance, leading to prolonged exposure and potentially better treatment outcomes. Experimental results, as shown in Fig. 8, confirm its enhanced immune response, reduced toxicity, and excellent antitumor efficacy.23
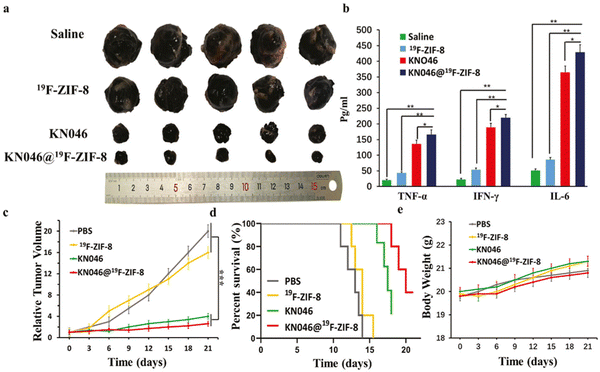 |
| Fig. 8 (a) This image displays tumors that were removed from various treatment groups after a 14-day period (n = 5). The visual comparison of tumor sizes can provide insights into the effectiveness of the treatments. (b): This chart shows the concentrations of TNF-α, IFN-γ, and IL-6 in the serum of mice from each group (n = 5). These cytokines are markers of immune response and potential inflammation. High levels may suggest an activated immune system, which is beneficial for antitumor efficacy, but excessively high levels could indicate an overactive immune response and potential side effects. (c) and (d) These charts depict the relative tumor volumes and survival rates of mice in each group (n = 5). The relative tumor volumes show the change in tumor size over time, reflecting the antitumor efficacy of the treatments. The survival rates represent the percentage of mice that survived throughout the experiment, indicating the overall impact of the treatments on the mice's well-being. (e) This chart illustrates the changes in body weight of mice from each group during the treatment period. Monitoring body weight is crucial to assess potential side effects and overall well-being. Significant weight loss or gain can indicate toxicity or adverse reactions to the treatments.23 (Jiang C, Zhang L, Xu X, Qi M, Zhang J, He S, et al. Advanced Science, 2021, 8(20) Licensed under a Creative Commons Attribution 4.0 International License (CC BY 4.0). [http://creativecommons.org/licenses/by/4.0/].). | |
The KN046@19F-ZIF-8 nanoplatform uses 19F, a non-natural isotope of fluorine, for imaging and tracking purposes. It allows real-time monitoring of the therapeutic agent, KN046, within the tumor site. The 19F signal can be released in response to specific stimuli in the tumor microenvironment (TME), enhancing antitumor efficacy and survival rates.23
Hence, the incorporation of 19F into the formulation of the KN046@19F-ZIF-8 nanoplatform enables non-invasive imaging, accurate quantification, and responsive imaging capabilities, thereby creating a valuable tool for tracking and evaluating the efficacy of the nanoplatform in cancer therapy. Overall, the integration of ZIF-8, PD-L1 inhibition, and targeted delivery holds promise for enhancing the effectiveness of immunotherapy in cancer treatment. The study utilized a cellular model involving B16F10 melanoma cells and an animal model using BALB/c mice with implanted B16F10 melanoma tumors to evaluate the therapeutic efficacy of KN046@19F-ZIF-8. The results support the potential of this approach for immunotherapy in cancer treatment.23
In the realm of cancer therapy, research has honed in on immunotherapy, particularly immune checkpoint inhibitors like anti-PD-L1 antibodies. However, these have shown limited success, especially in immunosuppressed cancers such as triple-negative breast cancer. To enhance their effectiveness, the concept of Immune Cell Death (ICD) has been introduced, aiming to kill tumor cells and activate the immune system. A study focuses on mEHGZ, a biomimetic nanoparticle carrier, encapsulating epirubicin, glucose oxidase, and hemin within ZIF-8 nanoparticles. These nanoparticles are unique, coated with calreticulin from over-expressed tumor cell membranes. ZIF-8's appeal lies in its large surface area, complex porosity, and pH-triggered biodegradation. The nanoparticles, with a tetrahedral shape and diameter of 110 nm, are further enhanced by a 10 nm thick outer layer of the mEHGZ nanoparticle, made of the tumor cell membrane. This design leverages ZIF-8's drug-carrying capabilities for targeted drug delivery within the Tumor Microenvironment (TME) (Fig. 9).148
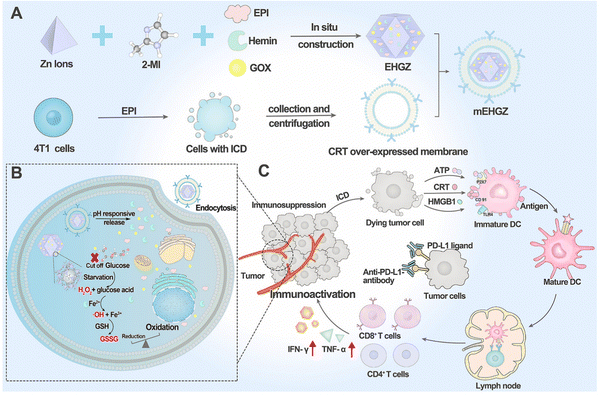 |
| Fig. 9 The construction of a self-amplified biomimetic nanosystem to ICD and activate an immune microenvironment for boosting the therapeutic effect of anti-PD-L1 antibody is illustrated. (A) The process begins with the preparation of mEHGZ nanoparticles. This involves encapsulating EPI (chemotherapeutic agent), Gox, and hemin (catalyst) within ZIF-8 nanoparticles. The nanoparticles are then coated with the tumor cell membrane that overexpresses CRT, a protein involved in immunogenic signaling. (B) After the mEHGZ nanoparticles are endocytosed by tumor cells, the encapsulated EPI is released in response to the low pH within the cells. This leads to the ICD of tumor cells, causing the release of antigens and damage-associated molecular patterns (DAMPs). Gox and hemin within the nanoparticles initiate a cascade reaction, generating hydroxyl free radicals (˙OH) and depleting intracellular GSH. This induces strong stress on the endoplasmic reticulum (ER), further enhancing the ICD effect. (C) The released antigens and DAMPs resulting from ICD promote the maturation of dendritic cells (DCs), infiltration of CD8+ T cells, and secretion of cytokines. This creates an immunosupportive microenvironment that enhances the therapeutic effect of anti-PD-L1 antibody.148 (Li Z, Cai H, Li Z, Ren L, Ma X, Zhu H, et al. Bioactive Materials. 2023;21:299–312 Licensed under a Creative Commons Attribution 4.0 International License (CC BY 4.0). [http://creativecommons.org/licenses/by/4.0/].). | |
The study used the 4T1 murine breast cancer cell line to test various formulations, including mEHGZ nanoparticles. The cellular uptake efficiency of mEHGZ nanoparticles was impressive at 91.04%, outperforming free EPI and EHGZ nanoparticles. The cytotoxicity of mEHGZ nanoparticles was minimal in APCs like RAW264.7 and DC2.4 cells, indicating a negligible impact on their viability. mEHGZ nanoparticles also induced a higher level of intracellular ROS production in 4T1 cells compared to free EPI. The study showed enhanced cellular uptake of mEHGZ nanoparticles and robust CRT transportation from the ER to the cell membrane, providing evidence of mEHGZ's ability to trigger ICD. In animal models, female mice were used to establish a tumor-bearing model. Once the tumor volume reached 50–75 mm 3, the mice were assigned to various treatment groups. The therapeutic efficacy of different formulations, including EPI, EZ, GHZ, EHGZ, mEHGZ, and an anti-PD-L1 antibody, was evaluated (Fig. 10).148
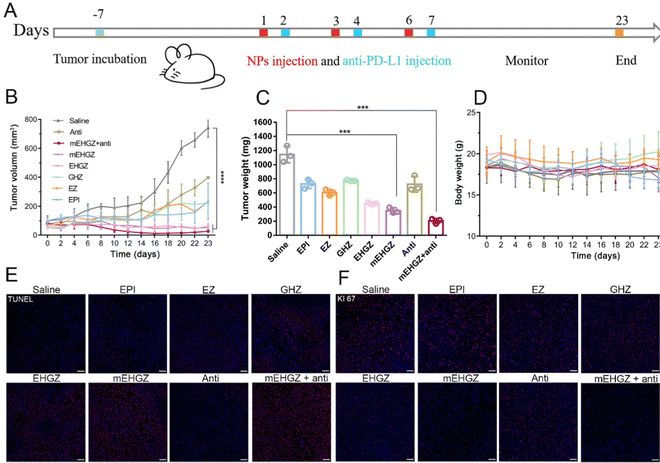 |
| Fig. 10 The impact of mEHGZ nanoparticles combined with an anti-PD-L1 antibody on tumor growth in a 4T1-bearing mice model. (A) Fig. 6A presents a schematic diagram of the treatment plan. The mice received intratumoral injections of various treatments, including EPI (a chemotherapy drug), EZ (empty ZIF-8 nanoparticles), GHZ (Gox and hemin-loaded ZIF-8 nanoparticles), EHGZ (EPI, Gox, and hemin-loaded ZIF-8 nanoparticles), mEHGZ (CRT-coated EHGZ nanoparticles), and an anti-PD-L1 antibody. (B) Post-treatment, tumor growth and lung metastasis were assessed in the 4T1-bearing mice model. The findings revealed that EPI treatment alone had minimal effect on tumor growth. However, the use of EHGZ and mEHGZ nanoparticles resulted in a significant delay in tumor growth, indicating the effective inhibition of tumor proliferation by mEHGZ nanoparticles. (C) Tumor weights were recorded at the end of the treatments. The lightest tumor weight was observed in the group treated with mEHGZ nanoparticles combined with the anti-PD-L1 antibody, confirming the effectiveness of this combination treatment in inhibiting tumor cell proliferation. (D) The therapeutic effect was further evaluated by examining the tumor sections. Remarkable shrinkage of tumors was observed in the group treated with mEHGZ nanoparticles combined with the anti-PD-L1 antibody compared to other treatment groups. This suggests that the induced tumor cell death and activated tumor immune microenvironment by mEHGZ nanoparticles favorably potentiate the treatment effect of the anti-PD-L1 antibody. (E) Immunohistochemistry staining was performed to evaluate the proliferation marker KI67 in tumor tissues. The weakest KI67 signal was displayed in the group treated with mEHGZ nanoparticles and anti-PD-L1 antibody, indicating reduced proliferation of tumor cells and further supporting the inhibitory effect of the combination treatment on tumor growth. (F) TUNEL (terminal deoxynucleotidyl transferase dUTP nick-end labeling) staining was performed on the tumor tissue sections to assess apoptosis. A higher number of TUNEL-positive cells were observed in the group treated with mEHGZ nanoparticles combined with anti-PD-L1 antibody compared to other treatment groups, suggesting that the combination treatment induces apoptosis in tumor cells.148 (Li Z, Cai H, Li Z, Ren L, Ma X, Zhu H, et al. Bioactive Materials. 2023;21:299–312 Licensed under a Creative Commons Attribution 4.0 International License (CC BY 4.0). [http://creativecommons.org/licenses/by/4.0/].). | |
The study evaluated tumor growth, lung metastasis, survival rates, and other key metrics to assess treatment effectiveness. The combination of mEHGZ nanoparticles and anti-PD-L1 antibody showed a significant tumor growth inhibition rate of 82.02%, outperforming EPI treatment alone (36.41%). Notably, no lung metastasis was detected in groups treated with mEHGZ nanoparticles, either alone or combined with anti-PD-L1 antibody. The highest survival rate was observed in the group treated with the combined regimen, indicating improved overall survival. The treatment also led to an increase in mature dendritic cells within tumors, and heightened CRT exposure on the cell membrane, signifying potent ICD induction by mEHGZ nanoparticles. Additionally, the combined treatment enhanced CD8+ T cell infiltration into tumor tissue, indicating a strengthened adaptive immune response. Histological examination revealed minimal pathological changes in major organs in groups treated with mEHGZ nanoparticles, highlighting the safety and biocompatibility of the mEHGZ delivery system. The combined treatment group exhibited the highest ratio of TUNEL-positive cells, indicating significant tumor cell apoptosis. Furthermore, this group displayed the weakest KI67 signal, suggesting effective suppression of tumor cell proliferation. In conclusion, the study highlights the potential of mEHGZ nanoparticles as an effective platform for ICD-based cancer therapy. The combination of mEHGZ nanoparticles with anti-PD-L1 antibodies demonstrated exceptional therapeutic efficacy, paving the way for future advancements in cancer treatment strategies.148
Photothermal therapy (PTT) uses light to generate heat, selectively killing cancer cells. A new nanosystem enhances PTT with gold nanorods (AuNRs), ZIF-8 nanoparticles, and anti-PD-L1 antibodies (AZ-P). AuNRs absorb near-infrared light, converting it into heat to destroy cancer cells. The ZIF-8 shell protects AZ-P and releases them in response to stimuli like ATP. AZ-P blocks the PD-L1/PD-1 interaction on cancer cells, promoting T cell activation. The nanosystem's components work synergistically to enhance the immune response against tumors. Another study suggests a liquid metal-based system for mild-PTT to treat deep tumors. ZIF-8 is functionalized with a bone/tumor dual-targeted ligand (HA/ALN), targeting tumor cells at bone metastases sites. HA targets CD44 receptors on tumor cells, while ALN accumulates in the bone microenvironment. This dual-targeting strategy enhances nanoparticle accumulation in the tumor and bone regions, improving treatment efficacy. The functionalization of ZIF-8 with HA/ALN can inhibit PD-L1 protein expression and recruit active immune T cells, potentially enhancing immunotherapy efficacy. The combination of ZIF-8-based nanoparticle delivery and PD-L1 inhibition is a promising strategy for cancer immunotherapy. Experimentally, a bone metastasis model was established to evaluate the potential application of the bone and tumor dual-targeted liquid metal nanoparticles. The outcomes include improved tumor therapeutic effects, inhibition of PD-L1 protein expression, and modulation of the immunosuppressive microenvironment.149
A recent study also explored the potential of ZIF-8 nanoparticles, coated with polydopamine and PEG, in immuno-oncotherapy. The nanoparticles were used as carriers for EGCG, a tea antioxidant known for inhibiting tumor growth. The study found that the nanoparticles effectively reduced levels of the inflammatory cytokine IL-1β and suppressed PD-L1 expression, enhancing the immune response against tumors. Cellular experiments with human and mouse melanoma cells showed significant inhibition of PD-L1 expression by the nanoparticles and EGCG. Animal experiments with a melanoma mouse model demonstrated successful nanoparticle accumulation in tumor tissue, significant suppression of tumor growth, and improved survival rates. The study also revealed changes in the immune cell composition within the tumor microenvironment (TME), indicating an immune response modulation. These findings highlight the potential of ZIF-8 nanoparticles in immuno-oncotherapy.150
Proposing mechanisms for enhanced antitumor effects with integrated approach
The enhanced antitumor effects observed above-mentioned studies with the integrated approach of ZIF-8 and PDL1 inhibition can be attributed to several underlying mechanisms: (1) improved drug delivery: ZIF-8 nanoparticles act as carriers for PDL1 inhibitors, protecting them from degradation and facilitating their targeted delivery to the tumor site. This targeted delivery approach ensures a higher concentration of PDL1 inhibitors in the TME, maximizing their therapeutic effects. (2) Restoration of immune response: PDL1 inhibitors block the interaction between PDL1 on cancer cells and PD1 on immune cells, preventing immune suppression. This restoration of the immune response allows immune cells, such as T cells, to recognize and attack cancer cells more effectively. (3) Modulation of the TME: ZIF-8 nanoparticles can modulate the TME by promoting immune cell infiltration and reducing immunosuppressive factors. This modulation creates a more favorable environment for the antitumor immune response, enhancing the efficacy of PDL1 inhibition. It is known that ZIF-8 nanoparticles possess the ability to release zinc ions when exposed to acidic environments, such as those found within tumor cells. These zinc ions play a crucial role in the activation of immune cells, including macrophages and neutrophils, which are capable of destroying cancer cells through phagocytosis or the release of cytotoxic chemicals.20,45,151 Another characteristic of ZIF-8 nanoparticles is their ability to decrease the concentration of immunosuppressive factors within the tumor microenvironment. These factors, produced by some tumor cells, inhibit the immune system's ability to attack cancer cells. This is presented in a nanozyme which was composed of Fe3O4@ZIF-8/GOx@MnO2.45,143,152–154 (4) Synergistic effects on immune checkpoint pathways: the combination of ZIF-8 and PDL1 inhibition may have synergistic effects on other immune checkpoint pathways. By targeting multiple immune checkpoints simultaneously, the integrated approach can overcome resistance mechanisms and enhance the overall antitumor immune response. Overall, the integrated approach of combining ZIF-8 and PDL1 inhibition in immunotherapy demonstrates synergistic effects, leading to enhanced antitumor effects. The improved drug delivery, restoration of immune response, modulation of the TME, and synergistic effects on immune checkpoint pathways contribute to the observed enhanced antitumor effects in preclinical studies.
X. Discussing clinical applications and future perspectives
The potential integration of ZIF-8, PDL1 inhibitors, and targeted delivery systems in immunotherapy could significantly improve cancer treatment outcomes (Fig. 11). However, it's important to note that this field is still in its early stages and, as of now, there are no ongoing clinical trials exploring this integrated approach. The concept of using ZIF-8 nanoparticles as carriers for PDL1 inhibitors in various cancer types is being investigated. The aim is to evaluate the targeted delivery of PDL1 inhibitors to the tumor site, enhance the antitumor immune response, and potentially improve patient outcomes. Moreover, the combination of ZIF-8, PDL1 inhibitors, and other immunotherapeutic agents such as immune checkpoint inhibitors or adoptive cell therapies is a subject of interest. The goal is to assess the synergistic effects of combining multiple immunotherapeutic approaches and targeted delivery systems to achieve enhanced antitumor responses and improved patient survival rates. However, it's crucial to emphasize that these are areas of research interest and not yet subjects of ongoing clinical trials. As the field progresses, we can anticipate more comprehensive studies and clinical trials in the future.
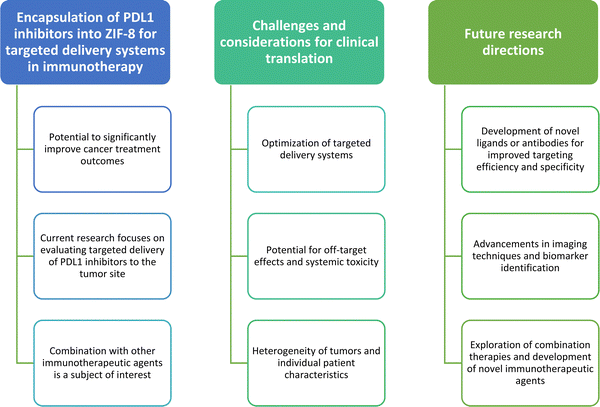 |
| Fig. 11 PDL1 inhibitors in ZIF-8 could improve cancer treatments. Challenges include delivery optimization and off-target effects. Future research aims to enhance targeting and explore combination therapies. | |
The potential integration of ZIF-8, PDL1 inhibitors, and targeted delivery systems in immunotherapy could significantly improve cancer treatment outcomes, as outlined in Table 1. However, several challenges and considerations need to be addressed for successful translation into clinical practice. Firstly, the optimization of targeted delivery systems is a significant challenge. This includes ensuring efficient and specific delivery of PDL1 inhibitors to the tumor site, which involves the selection of appropriate ligands or antibodies for targeting, as well as designing nanoparticles with optimal size, stability, and surface functionality. Secondly, the potential for off-target effects and systemic toxicity is a crucial consideration. A careful evaluation of the safety profile of this integrated approach is necessary to minimize adverse reactions and ensure patient well-being. Lastly, the heterogeneity of tumors and individual patient characteristics pose challenges in achieving consistent and effective targeted delivery. Personalized approaches may be required to account for tumor-specific factors and patient variability. These challenges highlight the complexity of translating this promising approach into clinical practice.
Future research should focus on addressing these issues to maximize the potential benefits of integrating ZIF-8, PDL1 inhibitors, and targeted delivery systems in cancer immunotherapy. This includes the development of novel ligands or antibodies for improved targeting efficiency and specificity. Advancements in imaging techniques and biomarker identification could enable better patient stratification and selection for targeted delivery approaches. This could help identify patients who are most likely to benefit from the integrated approach, thereby improving treatment outcomes. Moreover, the combination of ZIF-8, PDL1 inhibitors, and other immunotherapeutic agents holds promise for achieving synergistic effects and overcoming resistance mechanisms. Further exploration of combination therapies and the development of novel immunotherapeutic agents may lead to improved treatment strategies. These future directions highlight the potential for significant advancements in this field. As research progresses, we can anticipate more comprehensive studies and clinical trials that will further our understanding and application of these promising approaches in cancer immunotherapy.
XI. Conclusion
In this review, the integration of ZIF-8 nanoparticles, PDL1 inhibitors, and targeted delivery systems in the field of immunotherapy is explored. Several key points are emphasized by the findings. Firstly, the versatility of ZIF-8 nanoparticles as a drug delivery platform is highlighted, with their ability to safeguard therapeutic agents and facilitate their precise delivery to the tumor site. Secondly, the crucial role of PDL1 inhibitors in restoring the immune response against cancer cells by blocking the PDL1/PD1 interaction is underscored. Furthermore, the potential enhancement of immunotherapy's antitumor effects through the integration of ZIF-8 with PDL1 inhibition is discussed in the review. This integration shows promise in overcoming resistance mechanisms and improving patient outcomes. Additionally, the improvement in the specificity and selectivity of drug delivery achieved through targeted delivery systems, such as ligand-conjugated ZIF-8 nanoparticles, is acknowledged in the review. This advancement enhances the therapeutic index of immunotherapy. The implications of integrating ZIF-8, PDL1 inhibitors, and targeted delivery systems for advancing immunotherapy are outlined. Firstly, the delivery and concentration of PDL1 inhibitors at the tumor site can be improved, thereby enhancing the efficacy of immunotherapy. Secondly, resistance mechanisms associated with PDL1/PD1 blockade alone can be overcome, leading to improved treatment outcomes. Thirdly, targeted delivery of therapeutic agents specifically to the TME can minimize off-target effects and systemic toxicity. Lastly, treatment approaches can be personalized based on individual patient characteristics by selecting appropriate ligands or antibodies for targeted delivery. The review also presents this integrated approach as a promising avenue in cancer treatment. Immunotherapy can be revolutionized by addressing the challenges and limitations of current strategies through the improvement in the delivery and concentration of therapeutic agents at the tumor site. This can result in an enhanced antitumor immune response and improved patient outcomes. However, it is important to note that further research and clinical trials are necessary to fully understand the safety, efficacy, and feasibility of this integrated approach. Challenges related to optimization, patient variability, and potential toxicities must be carefully addressed. With the continued advancements in targeted delivery systems and personalized medicine, the potential impact of this integrated approach in cancer treatment is substantial. Great promise is held by the integration of ZIF-8, PDL1 inhibitors, and targeted delivery systems for advancing immunotherapy and improving cancer treatment outcomes. This approach has the potential to enhance the efficacy, specificity, and safety of immunotherapy, paving the way for more effective and personalized cancer treatments in the future.
Conflicts of interest
There are no conflicts to declare.
References
- C. Peterson, N. Denlinger and Y. Yang, Cancers, 2022, 14, 3972 CrossRef CAS PubMed.
- X. Zhao, T. Starr and S. Subramanian, Cancers, 2023, 15, 4197 CrossRef PubMed.
- Q. Sun, G. Melino, I. Amelio, J. Jiang, Y. Wang and Y. Shi, Discover Oncol., 2021, 12, 27 CrossRef PubMed.
- C. Liu, M. Yang, D. Zhang, M. Chen and D. Zhu, Front. Immunol., 2022, 13, 961805 CrossRef CAS PubMed.
- M. Tajaldini, A. Poorkhani, T. Amiriani, A. Amiriani, H. Javid, P. Aref, F. Ahmadi, S. Sadani and V. Khori, Eur. J. Pharmacol., 2023, 957, 175991 CrossRef CAS PubMed.
- H. Javid, F. Attarian, T. Saadatmand, N. Rezagholinejad, A. Mehri, H. Amiri and M. Karimi-Shahri, J. Cell. Biochem., 2023, 124, 477–494 CrossRef CAS PubMed.
- J. Liu, Z. Chen, Y. Li, W. Zhao, J. Wu and Z. Zhang, Front. Pharmacol., 2021, 12, 731798 CrossRef CAS PubMed.
- Z. Liu, X. Yu, L. Xu, Y. Li and C. Zeng, Exp. Hematol. Oncol., 2022, 11, 44 CrossRef CAS PubMed.
- X. Yu, X. Zhai, J. Wu, Q. Feng, C. Hu, L. Zhu and Q. Zhou, Biochim. Biophys. Acta Mol. Basis Dis., 2024, 1870 Search PubMed.
- M. Rui, W. Zhang, K. Mi, H. Ni, W. Ji, X. Yu, J. Qin and C. Feng, Int. J. Biol. Macromol., 2023, 253, 126811 CrossRef CAS PubMed.
- Q. Geng, Y. Dong, P. Jin, X. Mu, N. Zhao, H. Cui, Q. Yang, B. Xiao, F. Zhao, Y. Lv, G. Su, G. Wu and P. Jiao, J. Mol. Struct., 2023, 1294, 136487 CrossRef CAS.
- C. Pan, H. Liu, E. Robins, W. Song, D. Liu, Z. Li and L. Zheng, J. Hematol. Oncol., 2020, 13, 29 CrossRef PubMed.
- D. Frisone, A. Friedlaender, A. Addeo and P. Tsantoulis, Front. Oncol., 2022, 12, 817548 CrossRef CAS PubMed.
- S. Wang, K. Xie and T. Liu, Front. Immunol., 2021, 12, 690112 CrossRef CAS PubMed.
- W. Mu, Q. Chu, Y. Liu and N. Zhang, Nano-Micro Lett., 2020, 12, 142 CrossRef CAS PubMed.
- D. Varshney, S. Y. Qiu, T. P. Graf and K. J. McHugh, AAPS J., 2021, 23, 90 CrossRef CAS PubMed.
- Z. Zhao, L. Zheng, W. Chen, W. Weng, J. Song and J. Ji, J. Hematol. Oncol., 2019, 12, 126 CrossRef PubMed.
- M. Karimi-Shahri, A. Alalikhan, P. Hashemian, A. Hashemzadeh and H. Javid, Nanotechnology, 2023, 34, 212001 CrossRef PubMed.
- S. K. Alsaiari, S. S. Qutub, S. Sun, W. Baslyman, M. Aldehaiman, M. Alyami, A. Almalik, R. Halwani, J. Merzaban, Z. Mao and N. M. Khashab, Sci. Adv., 2021, 7, eabe7174 CrossRef CAS PubMed.
- Q. Wang, Y. Sun, S. Li, P. Zhang and Q. Yao, RSC Adv., 2020, 10, 37600–37620 RSC.
- B. Ding, H. Chen, J. Tan, Q. Meng, P. Zheng, P. A. Ma and J. Lin, Angew. Chem., Int. Ed., 2023, 62, e202215307 CrossRef CAS PubMed.
- H. Zhang, J. Zhang, Q. Li, A. Song, H. Tian, J. Wang, Z. Li and Y. Luan, Biomaterials, 2020, 245, 817548 Search PubMed.
- C. Jiang, L. Zhang, X. Xu, M. Qi, J. Zhang, S. He, Q. Tian and S. Song, Adv. Sci., 2021, 8, 2102500 CrossRef CAS PubMed.
- S. Dutta, Biomater. Sci., 2021, 9, 6391–6402 RSC.
- M. Ding, W. Liu and R. Gref, Adv. Drug Delivery Rev., 2022, 190, 114496 CrossRef CAS PubMed.
- N. A. H. M. Nordin, A. F. Ismail, A. Mustafa, R. S. Murali and T. Matsuura, RSC Adv., 2014, 4, 52530–52541 RSC.
- M. S. Tahir, J.-W. Lee, I. Rabani, F. Afzal, Y.-J. Han, H.-Y. Park, J. Lee and Y. S. Seo, J. Radioanal. Nucl. Chem., 2023, 332, 3967–3975 CrossRef CAS.
- S. Kouser, A. Hezam, M. J. N. Khadri and S. A. Khanum, J. Porous Mater., 2022, 29, 663–681 CrossRef CAS.
- V. A. Tran and S.-W. Lee, RSC Adv., 2021, 11, 9222–9234 RSC.
- L. Yan, X. Chen, Z. Wang, X. Zhang, X. Zhu, M. Zhou, W. Chen, L. Huang, V. A. L. Roy, P. K. N. Yu, G. Zhu and W. Zhang, ACS Appl. Mater. Interfaces, 2017, 9, 32990–33000 CrossRef CAS PubMed.
- M. d J. Velásquez-Hernández, R. Ricco, F. Carraro, F. T. Limpoco, M. Linares-Moreau, E. Leitner, H. Wiltsche, J. Rattenberger, H. Schröttner, P. Frühwirt, E. M. Stadler, G. Gescheidt, H. Amenitsch, C. J. Doonan and P. Falcaro, CrystEngComm, 2019, 21, 4538–4544 RSC.
- X. Liu, Y. Li, Y. Ban, Y. Peng, H. Jin, H. Bux, L. Xu, J. Caro and W. Yang, Chem. Commun., 2013, 49, 9140–9142 RSC.
- H. Xie, X. Liu, Z. Huang, L. Xu, R. Bai, F. He, M. Wang, L. Han, Z. Bao, Y. Wu, C. Xie and Y. Gong, Cancers, 2022, 14, 3935 CrossRef CAS PubMed.
- S. A. Butonova, E. V. Ikonnikova, A. Sharsheeva, I. Y. Chernyshov, O. A. Kuchur, I. S. Mukhin, E. Hey-Hawkins, A. V. Vinogradov and M. I. Morozov, RSC Adv., 2021, 11, 39169–39176 RSC.
- S. Bhattacharjee, M.-S. Jang, H.-J. Kwon and W.-S. Ahn, Catal. Surv. Asia, 2014, 18, 101–127 CrossRef CAS.
- J. Bi, Y. Lu, Y. Dong and P. Gao, J. Nanomater., 2018, 2018, 1357812 Search PubMed.
- M. Lei, W. Cai, L. Zou, B. Yu, L. Chen, Y. Cao, S. Xiang, C. Song, J. Lei, W. Jiang and Z. Hu, Nano Res., 2023, 19, 1–3, DOI:10.1007/s12274-023-5979-1.
- X. Duan, Y. Luo, R. Zhang, H. Zhou, W. Xiong, R. Li, Z. Huang, L. Luo, S. Rong and M. Li, Mater. Today Adv., 2023, 17, 100336 CrossRef CAS.
- X. Xia, X. Song, Y. Li, W. Hou, H. Lv, F. Li, Y. Li, J. Liu and X. Li, Front. Bioeng. Biotechnol., 2022, 10, 1026743 CrossRef PubMed.
- E. Issaka, J. N. O. Amu-Darko, M. Adams, S. Yakubu, E. Gyimah, N. Ali, J. Cui and M. Bilal, Catal. Lett., 2023, 153, 2083–2106 CrossRef CAS.
- T. L.-B. Pham, D. P.-H. Nguyen, T. T.-T. Luu, L. S. Nguyen, N. T. Binh, Q. D. Nguyen and P. A. Tran, Biomedicines, 2023, 11, 1158 CrossRef CAS PubMed.
- X. Xia, X. Song, Y. Li, W. Hou, H. Lv, F. Li, Y. Li, J. Liu and X. Li, Front. Bioeng. Biotechnol., 2022, 10, 1026743 CrossRef PubMed.
- H. N. Abdelhamid, Biointerface Res. Appl. Chem., 2021, 11, 8283–8297 CAS.
- L. Du, G. Zhu, Y. Xu, B. Han, Y. Wang, M. Zhu, Y. Meng, H. Chen and Z. Yu, Front. Bioeng. Biotechnol., 2023, 11, 1147064 CrossRef PubMed.
- J. Gong, X. Li, S. Li, M. Xu and W. Dai, New J. Chem., 2023, 47, 22495–22507 RSC.
- C. Singh, C. B. Jha, N. Kumar, R. Singh, H. Ojha, S. Upadhyayula, R. Varshney and R. Mathur, J. Mater. Sci., 2022, 57, 18561–18577 CrossRef CAS.
- X. Fu, Z. Yang, T. Deng, J. Chen, Y. Wen, X. Fu, L. Zhou, Z. Zhu and C. Yu, J. Mater. Chem. B, 2020, 8, 1481–1488 RSC.
- S. Zhang, J. Li, L. Yan, Y. You, F. Zhao, J. Cheng, L. Yang, Y. Sun, Q. Chang, R. Liu and Y. Li, Nanomaterials, 2023, 13, 1779 CrossRef CAS PubMed.
- M. Anisi, A. A. Ghoreyshi, E. Mehrvarz and A. Rahimpour, Environ. Sci. Pollut. Res., 2021, 28, 12725–12739 CrossRef CAS PubMed.
- V. Siva, A. Murugan, A. Shameem, S. Thangarasu and S. A. Bahadur, J. Inorg. Organomet. Polym. Mater., 2022, 32, 4707–4714 CrossRef CAS.
- I. Brekalo, W. Yuan, C. Mottillo, Y. Lu, Y. Zhang, J. Casaban, K. T. Holman, S. L. James, F. Duarte, P. A. Williams, K. D. M. Harris and T. Friščić, Chem. Sci., 2020, 11, 2141–2147 RSC.
- W. Lv, Z. Han, S. Dong, Y. Huang, J. Deng, C. Liu, Q. Feng and J. Sun, Nano Res., 2023, 16, 1–10, DOI:10.1007/s12274-023-6213-x.
- S. Feng, X. Zhang, D. Shi and Z. Wang, Front. Chem. Sci. Eng., 2021, 15, 221–237 CrossRef CAS.
- P. Kesharwani, R. Chadar, A. Sheikh, W. Y. Rizg and A. Y. Safhi, Front. Pharmacol., 2022, 12, 800481 CrossRef PubMed.
- D. J. Worm, S. Els-Heindl and A. G. Beck-Sickinger, Pept. Sci., 2020, 112, e24171 CrossRef CAS.
- M. A. Oryani, S. Nosrati, H. Javid, A. Mehri, A. Hashemzadeh and M. Karimi-Shahri, Naunyn-Schmiedeberg's Arch. Pharmacol., 2023, 16, 1–28, DOI:10.1007/s00210-023-02707-y.
- M. Mazloum-Ardakani, N. Shaker-Ardakani and A. Ebadi, J. Cluster Sci., 2023, 34, 1861–1876 CrossRef CAS.
- J. Li, D. Zhu, W. Ma, Y. Yang, G. Wang, X. Wu, K. Wang, Y. Chen, F. Wang, W. Liu and Y. Yuan, Nanoscale, 2020, 12, 17064–17073 RSC.
- Q. Li, R. Feng, Z. Chang, X. Liu, H. Tang and Q. Bai, Front. Bioeng. Biotechnol., 2022, 10, 991949 CrossRef PubMed.
- Y. Zhou, X. Wang, J. Men, M. Jia and C. Liang, J. Radioanal. Nucl. Chem., 2022, 331, 1367–1379 CrossRef CAS.
- M. Yi, X. Zheng, M. Niu, S. Zhu, H. Ge and K. Wu, Mol. Cancer, 2022, 21, 28 CrossRef CAS PubMed.
- L. Shi, S. Chen, L. Yang and Y. Li, J. Hematol. Oncol., 2013, 6, 74 CrossRef PubMed.
- M. Yi, M. Niu, L. Xu, S. Luo and K. Wu, J. Hematol. Oncol., 2021, 14, 10 CrossRef CAS PubMed.
- M. Furuse, H. Kuwabara, N. Ikeda, Y. Hattori, T. Ichikawa, N. Kagawa, K. Kikuta, S. Tamai, M. Nakada, T. Wakabayashi, M. Wanibuchi, T. Kuroiwa, Y. Hirose and S.-I. Miyatake, BMC Cancer, 2020, 20, 277 CrossRef CAS PubMed.
- E. M. Palsson-McDermott, L. Dyck, Z. Zasłona, D. Menon, A. F. McGettrick, K. H. G. Mills and L. A. O’Neill, Front. Immunol., 2017, 8, 1300 CrossRef PubMed.
- M. Zuazo, H. Arasanz, A. Bocanegra, G. Fernandez, L. Chocarro, R. Vera, G. Kochan and D. Escors, Front. Immunol., 2020, 11, 586907 CrossRef CAS PubMed.
- I. N. Ivashko and J. M. Kolesar, Am. J. Health-Syst. Pharm., 2016, 73, 193–201 CrossRef CAS PubMed.
- L. Chocarro de Erauso, M. Zuazo, H. Arasanz, A. Bocanegra, C. Hernandez, G. Fernandez, M. J. Garcia-Granda, E. Blanco, R. Vera, G. Kochan and D. Escors, Front. Pharmacol., 2020, 11, 441 CrossRef PubMed.
- K. X. Lin, A. C. Istl, D. Quan, A. Skaro, E. Tang and X. Zheng, Cancer Immunol. Immunother., 2023, 72, 3875–3893 CrossRef CAS PubMed.
- C. Cheng, L. Zhuge, X. Xiao, S. Luan and Y. Yuan, Front. Oncol., 2022, 12, 955163 CrossRef CAS PubMed.
- H. Xie, X. Liu, Z. Huang, L. Xu, R. Bai, F. He, M. Wang, L. Han, Z. Bao, Y. Wu, C. Xie and Y. Gong, Cancers, 2022, 14, 3935 CrossRef CAS PubMed.
- S. Wang, L. Ouyang, G. Deng, Z. Deng and S. Wang, RSC Adv., 2020, 10, 31012–31021 RSC.
- S. Saha and A. Mishra, Biomater. Sci., 2023, 11, 481–488 RSC.
- Q. Lei, D. Wang, K. Sun, L. Wang and Y. Zhang, Front. Cell Dev. Biol., 2020, 8, 672 CrossRef PubMed.
- A. Puccini, F. Battaglin, M. L. Iaia, H.-J. Lenz and M. E. Salem, Immunother. Cancer, 2020, 8, e000404 CrossRef PubMed.
- R. Bazak, M. Houri, S. El Achy, S. Kamel and T. Refaat, J. Cancer Res. Clin. Oncol., 2015, 141, 769–784 CrossRef CAS PubMed.
- M. F. Attia, N. Anton, J. Wallyn, Z. Omran and T. F. Vandamme, J. Pharm. Pharmacol., 2019, 71, 1185–1198 CrossRef CAS PubMed.
- Q. Zhang, G. Kuang, W. Li, J. Wang, H. Ren and Y. Zhao, Nano-Micro Lett., 2023, 15, 44 CrossRef CAS PubMed.
- B. Murugan, S. Sagadevan, I. Fatimah, W.-C. Oh, M. A. M. Hossain and M. R. Johan, Nanotechnol. Rev., 2021, 10, 933–953 CrossRef CAS.
- J. Lin, L. Huang, H. Ou, A. Chen, R. Xiang and Z. Liu, RSC Adv., 2021, 11, 21414–21425 RSC.
- M. Uz, S. Alsoy Altinkaya and S. K. Mallapragada, J. Mater. Res., 2017, 32, 2930–2953 CrossRef CAS.
- Y. Xia, S. Fu, Q. Ma, Y. Liu and N. Zhang, Nano-Micro Lett., 2023, 15, 145 CrossRef CAS PubMed.
- G. Liu, L. Yang, G. Chen, F. Xu, F. Yang, H. Yu, L. Li, X. Dong, J. Han, C. Cao, J. Qi, J. Su, X. Xu, X. Li and B. Li, Front. Pharmacol., 2021, 12, 735446 CrossRef CAS PubMed.
- X. Cheng, Q. Xie and Y. Sun, Front. Bioeng. Biotechnol., 2023, 11, 1177151 CrossRef PubMed.
- H. Chen, L. Wang, X. Zeng, H. Schwarz, H. S. Nanda, X. Peng and Y. Zhou, Front. Cell Dev. Biol., 2021, 9, 751079 CrossRef PubMed.
- E. Kianfar, J. Supercond. Novel Magn., 2021, 34, 1709–1735 CrossRef CAS.
- H. Katsumi, S. Yamashita, M. Morishita and A. Yamamoto, Chem. Pharm. Bull., 2020, 68, 560–566 CrossRef CAS PubMed.
- T. Fukuta and K. Kogure, Chem. Pharm. Bull., 2022, 70, 334–340 CrossRef CAS PubMed.
- G. Bahlakeh, R. Rahbarghazi, D. Mohammadnejad, A. Abedelahi and M. Karimipour, Cell Biosci., 2021, 11, 181 CrossRef CAS PubMed.
- A. Zeb, M. Gul, T.-T.-L. Nguyen and H.-J. Maeng, J. Pharm. Invest., 2022, 52, 683–724 CrossRef CAS.
- N. Baig, I. Kammakakam and W. Falath, Mater. Adv., 2021, 2, 1821–1871 RSC.
- M. Kim, S. Osone, T. Kim, H. Higashi and T. Seto, KONA Powder Part. J., 2017, 34, 80–90 CrossRef CAS.
- H. Tabasi, M. T. H. Mosavian, M. Darroudi, M. Khazaei, A. Hashemzadeh and Z. Sabouri, J. Porous Mater., 2022, 29, 1817–1828 CrossRef CAS.
- A. Hashemzadeh, G. P. Drummen, A. Avan, M. Darroudi, M. Khazaei, R. Khajavian, A. Rangrazi and M. Mirzaei, J. Mater. Chem. B, 2021, 9, 3967–3982 RSC.
- A. Hashemzadeh, F. Asgharzadeh, A. Yaghoubi, S. E. Nazari, S. M. Hassanian, A. Avan, M. Rezaei, S. Soleimanpour and M. Khazaei, J. Trace Elem. Miner., 2022, 2, 100012 CrossRef.
- A. Hashemzadeh, F. Amerizadeh, F. Asgharzadeh, G. P. Drummen, S. M. Hassanian, M. Landarani, A. Avan, Z. Sabouri, M. Darroudi and M. Khazaei, J. Cluster Sci., 2022, 33, 2345–2361 CrossRef CAS.
- A. Hashemzadeh, F. Amerizadeh, F. Asgharzadeh, M. Darroudi, A. Avan, S. M. Hassanian, M. Landarani and M. Khazaei, Toxicol. Appl. Pharmacol., 2021, 423, 115573 CrossRef CAS PubMed.
- F. Asgharzadeh, A. Hashemzadeh, A. Yaghoubi, A. Avan, S. E. Nazari, S. Soleimanpour, S. M. Hassanian, G. A. Ferns, F. Rahmani and M. Khazaei, J. Drug Delivery Sci. Technol., 2021, 61, 102133 CrossRef CAS.
- M. N. Jabbour, C. Y. Massad and F. I. Boulos, Breast Cancer Res. Treat., 2012, 135, 29–37 CrossRef CAS PubMed.
- S. Ramón y Cajal, M. Sesé, C. Capdevila, T. Aasen, L. De Mattos-Arruda, S. J. Diaz-Cano, J. Hernández-Losa and J. Castellví, J. Mol. Med., 2020, 98, 161–177 CrossRef PubMed.
- T. Liu, H. Choi, R. Zhou and I.-W. Chen, PLoS One, 2014, 9, e103576 CrossRef PubMed.
- L. Liu, T. K. Hitchens, Q. Ye, Y. Wu, B. Barbe, D. E. Prior, W. F. Li, F.-C. Yeh, L. M. Foley, D. J. Bain and C. Ho, Biochim. Biophys. Acta, 2013, 1830, 3447–3453 CrossRef CAS PubMed.
- J. L. Vahle, Toxicol. Pathol., 2018, 46, 1013–1019 CrossRef CAS PubMed.
- N. Bano, C. Ehlinger, T.-Y. Yang, M. Swanson and S. Allen, AAPS J., 2022, 24, 93 CrossRef CAS PubMed.
- A. N. Khan, A. Chowdhury, A. Karulkar, A. K. Jaiswal, A. Banik, S. Asija and R. Purwar, Front. Immunol., 2022, 13, 886546 CrossRef CAS PubMed.
- T. Lu, S. Ji, W. Jin, Q. Yang, Q. Luo and T.-L. Ren, Sensors, 2023, 23, 2991 CrossRef CAS PubMed.
- J. K. Patra, G. Das, L. F. Fraceto, E. V. R. Campos, M. D. P. Rodriguez-Torres, L. S. Acosta-Torres, L. A. Diaz-Torres, R. Grillo, M. K. Swamy, S. Sharma, S. Habtemariam and H.-S. Shin, J. Nanobiotechnol., 2018, 16, 71 CrossRef PubMed.
- S. Saallah and I. W. Lenggoro, KONA Powder Part. J., 2018, 35, 89–111 CrossRef CAS.
- N. Zahin, R. Anwar, D. Tewari, M. T. Kabir, A. Sajid, B. Mathew, M. S. Uddin, L. Aleya and M. M. Abdel-Daim, Environ. Sci. Pollut. Res., 2020, 27, 19151–19168 CrossRef CAS PubMed.
- M. Loizidou and A. M. Seifalian, Br. J. Surg., 2010, 97, 463–465 CrossRef CAS PubMed.
- R. D. Handy and B. J. Shaw, Health, Risk Soc., 2007, 9, 125–144 CrossRef.
- A. Rejeb, J. G. Keogh and K. Rejeb, J. Data, Inf. Manag., 2022, 4, 33–47 CrossRef.
- M. Färber and A. Jatowt, Int. J. Dig. Lib., 2020, 21, 375–405 CrossRef.
- A. Kennedy, M. A. Robinson, C. Hinze, E. Waters, C. Williams, N. Halliday, S. Dovedi and D. M. Sansom, EMBO J., 2023, 42, e111556 CrossRef CAS PubMed.
- D. M. Lussier, J. L. Johnson, P. Hingorani and J. N. Blattman, Immunother. Cancer, 2015, 3, 21 CrossRef PubMed.
- N. R. Latimer, A. Towse and C. Henshall, Expert Rev. Pharm. Outcomes Res., 2021, 21, 331–333 Search PubMed.
- A. Xiong, W. Li, X. Li, Y. Fan, Z. Ma, J. Fang, Q. Xie, W. Zhuang, M. Kang, J. Wang, T. Xu, M. Xu, L. Zhi, Q. Liu, N. Wang and C. Zhou, Eur. J. Cancer, 2023, 190 Search PubMed.
- Q. Zuo, T. Li, L. Huang, Z. Liu and W. Xue, Biomater. Sci., 2023, 11, 5025–5045 RSC.
- S. Zhou, Q. Shang, J. Ji and Y. Luan, ACS Appl. Mater. Interfaces, 2021, 13, 47407–47417 CrossRef CAS PubMed.
- Y. Zhao, X. Xiao, M. Zou, B. Ding, H. Xiao, M. Wang, F. Jiang, Z. Cheng, P. Ma and J. Lin, Adv. Mater., 2021, 33, 2006363 CrossRef CAS PubMed.
- X. Zhao, H. Cheng, Q. Wang, W. Nie, Y. Yang, X. Yang, K. Zhang, J. Shi and J. Liu, ACS Nano, 2023, 17, 13746–13759 CrossRef CAS PubMed.
- H. Xie, X. Liu, Z. Huang, L. Xu, R. Bai, F. He, M. Wang, L. Han, Z. Bao, Y. Wu, C. Xie and Y. Gong, Cancers, 2022, 14, 3935 CrossRef CAS PubMed.
- L. Ma, J. Zhou, Q. Wu, G. Luo, M. Zhao, G. Zhong, Y. Zheng, X. Meng, S. Cheng and Y. Zhang, Biomaterials, 2023, 301, 122236 CrossRef CAS PubMed.
- Q. Fan, J. Zuo, H. Tian, C. Huang, E. C. Nice, Z. Shi and Q. Kong, J. Exp. Clin. Cancer Res., 2022, 41, 1–7 CrossRef PubMed.
- B. Ding, H. Chen, J. Tan, Q. Meng, P. Zheng, P. Ma and J. Lin, Angew. Chem., Int. Ed., 2023, 62 Search PubMed.
- H. N. Abdelhamid, Curr. Med. Chem., 2021, 28, 7023–7075 CAS.
- Y. Wang, H. Wang, Y. Song, M. Lv, Y. Mao, H. Song, Y. Wang, G. Nie, X. Liu, J. Cui and X. Zou, J. Nanobiotechnol., 2022, 20, 96 CrossRef CAS PubMed.
- C. Cui, H. Qian, J. Wang, J. Kang, W. Ma, Y. Nian, Z. Sun and H. Weng, Int. J. Biol. Macromol., 2023, 247, 125692 CrossRef CAS PubMed.
- N. M. Ratnam, S. C. Frederico, J. A. Gonzalez and M. R. Gilbert, Neuro-Oncol. Adv., 2021, 3, vdaa161 CrossRef PubMed.
- X. Hu, J. Guo, J. Shi, D. Li, X. Li and W. Zhao, BMC Pulm. Med., 2023, 23, 223 CrossRef CAS PubMed.
- J. Zhang, D. Liu, J. Liu, Y. Han, H. Xu, X. Leng, D. Kong and L. Liu, Biomater. Sci., 2020, 8, 4757–4766 RSC.
- L. Yu, Y. Wang, S. Shao, M. Yang, H. Niu, Q. Yu and X. Wang, Tumori, 2015, 101, 549–554 CrossRef CAS PubMed.
- N. Skafi, M. Fayyad-Kazan and B. Badran, Gene, 2020, 754, 144888 CrossRef CAS PubMed.
- S. A. Shukla, P. Bachireddy, B. Schilling, C. Galonska, Q. Zhan, C. Bango, R. Langer, P. C. Lee, D. Gusenleitner, D. B. Keskin, M. Babadi, A. Mohammad, A. Gnirke, K. Clement, Z. J. Cartun, E. M. Van Allen, D. Miao, Y. Huang, A. Snyder, T. Merghoub, J. D. Wolchok, L. A. Garraway, A. Meissner, J. S. Weber, N. Hacohen, D. Neuberg, P. R. Potts, G. F. Murphy, C. G. Lian, D. Schadendorf, F. S. Hodi and C. J. Wu, Cell, 2018, 173, 624–633 CrossRef CAS PubMed.
- Y. Kondo, T. Ohno, N. Nishii, K. Harada, H. Yagita and M. Azuma, Oral Oncol., 2016, 57, 54–60 CrossRef CAS PubMed.
- K. Kim, S. Park, S. Y. Park, G. Kim, S. M. Park, J. W. Cho, D. H. Kim, Y. M. Park, Y. W. Koh, H. R. Kim, S. J. Ha and I. Lee, Genome Med., 2020, 12, 1–6 CrossRef PubMed.
- V. D. Fedorov, M. Themeli and M. Sadelain, Sci. Transl. Med., 2013, 5, 215ra172 Search PubMed.
- J. Duraiswamy, R. Turrini, A. Minasyan, D. Barras, I. Crespo, A. J. Grimm, J. Casado, R. Genolet, F. Benedetti, A. Wicky, K. Ioannidou, W. Castro, C. Neal, A. Moriot, S. Renaud-Tissot, V. Anstett, N. Fahr, J. L. Tanyi, M. A. Eiva, C. A. Jacobson, K. T. Montone, M. C. W. Westergaard, I. M. Svane, L. E. Kandalaft, M. Delorenzi, P. K. Sorger, A. Färkkilä, O. Michielin, V. Zoete, S. J. Carmona, P. G. Foukas, D. J. Powell, S. Rusakiewicz, M. A. Doucey, D. Dangaj Laniti and G. Coukos, Cancer Cell, 2021, 39, 1623–1642 CrossRef CAS PubMed.
- J. A. Marin-Acevedo, B. Dholaria, A. E. Soyano, K. L. Knutson, S. Chumsri and Y. Lou, J. Hematol. Oncol., 2018, 11, 39 CrossRef PubMed.
- Ritu, P. Chandra and A. Das, Clin. Exp. Med., 2023, 23, 4297–4322 CrossRef CAS PubMed.
- N. R. Latimer, D. Pollard, A. Towse, C. Henshall, L. Sansom, R. L. Ward, A. Bruce and C. Deakin, BMC Health Serv. Res., 2021, 21, 412 CrossRef.
- B. J. Schneider, J. Naidoo, B. D. Santomasso, C. Lacchetti, S. Adkins, M. Anadkat, M. B. Atkins, K. J. Brassil, J. M. Caterino and I. Chau, J. Clin. Oncol., 2021, 39, 4073–4126 CrossRef CAS PubMed.
- E. P. Darnell, M. J. Mooradian, E. N. Baruch, M. Yilmaz and K. L. Reynolds, Curr. Oncol. Rep., 2020, 22, 39 CrossRef PubMed.
- Y. Zhang, Y. Yang, J. Shi and L. Wang, Nanoscale, 2021, 13, 16571–16588 RSC.
- Y. Ma, J. Xue, Y. Zhao, Y. Zhang, Y. Huang, Y. Yang, W. Fang, Y. Guo, Q. Li, X. Ge, J. Sun, B. Zhang, Y. Zhang, J. Xiao, L. Zhang and H. Zhao, Immunother. Cancer, 2023, 11 Search PubMed.
- M. Li, C. Wu, Y. Liu, R. Zhang, Q. Yang, Z. Shi, W. Sun and X. Hu, Diabetes, Metab. Syndr. Obes.: Targets Ther., 2022, 15, 1253–1260 CrossRef PubMed.
- M. Ding, C. Deng, X. Liu, S. Jiang, Y. Gao, D. Fan, Y. Zhou, J. He and C. Liu, Front. Immunol., 2023, 14, 1132692 CrossRef CAS PubMed.
- Z. Li, H. Cai, Z. Li, L. Ren, X. Ma, H. Zhu, Q. Gong, H. Zhang, Z. Gu and K. Luo, Bioactive Mater., 2023, 21, 299–312 CrossRef CAS PubMed.
- Y. Shen, Y. Zou, B. Bie, C. Dong and Y. Lv, Acta Biomater., 2023, 157, 578–592 CrossRef CAS PubMed.
- N. Jannatun, Y. Zhang, B. Wu, Q. Song, G. Cao, W. Luo, F. Yuan, Q. Li, Y. Zeng, G. Zhang, G. Wang and Y. Li, ACS Appl. Nano Mater., 2023, 6, 4379–4389 CrossRef CAS.
- G. Chen, B. Yu, C. Lu, H. Zhang, Y. Shen and H. Cong, CrystEngComm, 2018, 20, 7486–7491 RSC.
- N. M. Phan, T. L. Nguyen and J. Kim, Tissue Eng. Regener. Med., 2022, 19, 237–252 CrossRef CAS PubMed.
- Z. Li, Q. Wang, X. Huang, M. Yang, S. Zhou, Z. Li, Z. Fang, Y. Tang, Q. Chen, H. Hou, L. Li, F. Fei, Q. Wang, Y. Wu and A. Gong, Front. Nutr., 2023, 10, 1113739 CrossRef PubMed.
- F. L. Qi, M. F. Wang, B. Z. Li, Z. F. Lu, G. J. Nie and S. P. Li, Acta Pharmacol. Sin., 2020, 41, 895–901 CrossRef CAS PubMed.
- Q. Cheng, F. Gao, W. Y. Yu, M. Z. Zou, X. L. Ding, M. J. Li, S. X. Cheng and X. Z. Zhang, Adv. Funct. Mater., 2020, 30, 2000335 CrossRef CAS.
|
This journal is © The Royal Society of Chemistry 2024 |