DOI:
10.1039/D3QM00625E
(Review Article)
Mater. Chem. Front., 2024,
8, 341-353
Coupling electrochemical CO2 reduction with value-added anodic oxidation reactions: progress and challenges
Received
1st June 2023
, Accepted 8th October 2023
First published on 9th October 2023
Abstract
Converting CO2 into chemicals using renewable electricity provides a promising avenue to realize carbon neutrality. Substituting the anodic oxygen evolution reaction by thermodynamically more favourable oxidation reactions with value-added products can significantly lower the cell voltage and enhance the output value of overall CO2 electrolysis. In this review, we summarize the up-to-date breakthroughs in electrochemical systems featuring the CO2 reduction reaction (CO2RR) coupled with various anodic oxidation reactions. Moreover, major challenges with possible solutions and future prospects in this field are pointed out, which are expected to benefit researchers and propel this coupling strategy to industrial applications.
1. Introduction
The global environmental issues and energy crisis are driving us to substitute traditional fossil fuels with green energy sources. Among the various emerging techniques, electrochemically converting CO2 into value-added feedstocks can not only store renewable electricity in chemical bonds but also promote the natural carbon cycle, and therefore holds potential for realizing carbon-neutrality. Currently, great efforts have been devoted to developing efficient catalysts with excellent Faradaic efficiency (FE), high current density, and low overpotential for the electrochemical CO2 reduction reaction (CO2RR).1–4 However, although remarkable catalysts with near-unity FEs and close-to-zero overpotentials have emerged, energy utilization is still far from satisfactory considering the whole electrolysis. For instance, according to the Hess law, the energy consumption for CO2 reduction to CO/HCOOH only takes a small part in the overall CO2 electrolysis where the oxygen evolution reaction (OER) with a theoretical potential of 1.23 V versus reversible hydrogen electrode (vs. RHE) is usually adopted as the anodic reaction.
Cathode:
CO2(g) + H2(g) → CO(g) + H2O(l) |
| (ΔG° = 20.1 kJ mol−1; E0 = −0.106 V) | (1) |
CO2(g) + H2(g) → HCOOH(l) |
| (ΔG° = 33.0 kJ mol−1; E0 = −0.199 V) | (2) |
Anode:
H2O(l) → H2(g) + 1/2O2(g) |
| (ΔG° = 237.1 kJ mol−1; E0 = 1.23 V) | (3) |
Overall:
CO2(g) → CO(g) + 1/2O2(g) |
| (ΔG° = 257.2 kJ mol−1; E0 = 1.34 V) | (4) |
CO2(g) + H2O(l) → HCOOH(g) + 1/2O2(g) |
| (ΔG° = 270.1 kJ mol−1; E0 = 1.43 V) | (5) |
Intuitively, the cathodic reduction of CO
2 to CO and HCOOH only takes 7.81% and 12.2% of the total energy consumption for overall CO
2 electrolysis, respectively, whereas the anodic OER consumes the vast majority of the electricity. In addition, the anodic product O
2 is of little value. To increase the energy utilization efficiency, many alternative reactions have been employed to replace the unsatisfactory OER (
Table 1).
5–9 Briefly, these reactions can be divided into three categories (
Fig. 1). The first category includes reactions thermodynamically more favourable than the OER that can effectively reduce the cell voltage. For example, ammonia, urea and hydrazine oxidation reactions (denoted as the AOR, UOR, and HzOR, respectively) have been substituted for the OER in water splitting owing to their significantly lower theoretical potentials (0.06, 0.37 and −0.33 V
vs. RHE for the AOR, UOR, and HzOR, respectively) to markedly cut down the applied cell voltage.
10–12 Although no value-added products are obtained, these sacrificial agent-like oxidation reactions can be adopted to eliminate pollutants in waste water.
13,14
Table 1 Alternative anodic oxidation reactions for the OER in CO2 overall electrolysis
|
Anodic oxidation reaction |
Redox potential (V vs. RHE) |
OER |
H2O → O2 + 4H+ + 4e− |
1.23 |
Category I |
2NH3 + 6OH− → N2 + 6H2O + 6e− |
0.06 |
CO(NH2)2 + 6OH− → CO2 + N2 + 5H2O + 6e− |
0.07 |
N2H4 + 4OH− → N2 + 4H2O + 4e− |
−0.33 |
Category II |
2Cl− → Cl2 + 2e− |
1.36 |
2H2O → H2O2 + 2H+ + 2e− |
1.76 |
Category III |
CH3OH + 3OH− → HCOO− + 3H2O + 2e− |
0.11 |
C3H8O3 + 11OH− → 3HCOO− + 8H2O + 8e− |
0.14 |
C6H12O6 + 2OH− → C6H12O7 + H2O + 2e− |
0.90 |
C6H6O3 + 6OH− → C6H2O52− + 4H2O + 4e− |
0.30 |
S2− → S + 2e− |
−0.48 |
2HS− + 2OH− → S22− + 2H2O + 2e− |
0.14 |
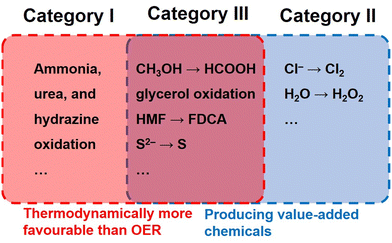 |
| Fig. 1 Different categories of alternative anodic oxidation reactions. | |
Oxidation reactions featuring no superior theoretical potential but value-added products (Category II) have also been employed as the anodic side of full electrolysis. A representative is water oxidation to H2O2 (2H2O → H2O2 + 2H+ + 2e−, E0 = 1.76 V vs. RHE). Albeit this two-electron process requires a higher potential by 0.53 V than that of the OER, the price of H2O2 (∼$560 per ton) is about 14 times as high as that of O2 (∼$40 per ton), making this substitution economically viable.5 As the most attractive substitutions for the OER, selective oxidation of small organic molecules such as alcohols, aldehydes, and amines (Category III) enables simultaneous voltage reduction and value-added chemical production.15–20 The market prices of the raw materials and products for these alternative oxidation reactions are summarized in Table 2.5 Evidently, the added values and the electricity output (added value per electron) for the alternative oxidation reactions are all times higher than that for the OER. In particular, the conversion of 5-hydroxymethylfurfural (HMF) to 2,5-furandicarboxylic acid (FDCA) holds the highest added value of up to $30970 per ton, suggesting its tremendous superiority compared with the OER.
Table 2 Economic comparison between the OER and alternative oxidation reactions
Raw material |
Market price of the raw material ($ per ton) |
Product |
Market price of the product ($ per ton) |
Added value ($ per ton) |
Added value per electron ($ per (ton e)) |
H2O |
0.6 |
O2 |
40 |
39.4 |
9.8 |
H2O |
0.6 |
H2O2 |
560 |
559.4 |
279.7 |
NaCl |
68 |
Cl2 |
822 |
754 |
377 |
CH3OH |
340 |
HCOOH |
970 |
630 |
157.5 |
CH3CH2OH |
610 |
CH3COOH |
680 |
70 |
17.5 |
C3H8O3 (glycerol) |
970 |
C3H6O3 (lactic acid) |
1580 |
610 |
305 |
C5H4O2 (furfural) |
1170 |
C5H4O3 (2-furoic acid) |
6230 |
5060 |
2530 |
C6H6O3 (5-Hydroxymethylfurfural) |
1030 |
C6H4O5 (2,5-Furandicarboxylic acid) |
32000 |
30970 |
5161.7 |
C6H12O6 (glucose) |
315 |
C6H12O7 (gluconic acid) |
644 |
329 |
164.5 |
Currently, increasing efforts are being devoted to coupling the above reactions with the CO2RR to fabricate an energy-efficient full electrolyser. Although several reviews on alternative anodic reactions coupled with the hydrogen evolution reaction (HER) have been reported, the case is much different in CO2 electrolysis considering the electrolyte choice, mass transfer, electrode design, cell structure, etc.21–23 This review starts with a brief introduction of different types of anodic oxidation reactions. Subsequently, we will present up-to-date progress in alternative anodic reactions that have already been or hold the potential to be applied in CO2RR coupling systems, with elucidation of the structure–performance relationship. Finally, we will systematically discuss the challenges and prospects for CO2RR coupling systems regarding the design of electrocatalysts and electrolysers for guidance on propelling this technique towards industrial applications.
2. Recent advances in coupling the CO2RR with alternative anodic reactions
2.1. Category I
N-containing species such as ammonia and urea are major pollutants in industrial and agricultural wastewater, the excessive emission of which will not only lead to the eutrophication of water and destroy the river and lake ecosystems, but also threaten drinking water safety. Classical methods to decompose these pollutants are based on chemical oxidation reactions using toxic and hazardous reagents, which are not preferred given the increasing environmental and economic requirements. Electrochemical oxidation of N-containing pollutants provides appealing routes to remedy the above issues. The pollutants can be transformed directly by current on the electrode surface or indirectly by active radicals in situ generated during electrolysis, rather than hazardous chemicals. However, in spite of their low theoretical potentials, both the AOR and UOR involve 6e−-transfer processes (2NH3 + 6OH− → N2 + 6H2O + 6e−; CO(NH2)2 + 6OH− → CO2 + N2 + 5H2O + 6e−), and thus suffer from sluggish kinetics. Accordingly, developing highly efficient catalysts is essential for the energy-efficient CO2RR-AOR and CO2RR-UOR couple at an industrial current density (>100 mA cm−2).
According to the dehydrogenation degree of N intermediates in the N–N coupling step, there are two possible pathways for AOR: (1) the adsorbed ammonia undergoes continuous dehydrogenation steps and the N–N coupling step occurs between two *N species; (2) the N–N coupling step occurs between two incomplete dehydrogenated *NHx species followed by further dehydrogenation of *N2Hy species (x = 1–3 and y = 2–5).24–26 Pt-based catalysts have shown excellent activity in the AOR. The introduction of Ir and Ni into the Pt lattice can decrease the energy barrier of dehydrogenation of *NH2 to *NH and the rate-determining step (RDS) on the Pt surface via elevating the d-band centre for enhanced *NH adsorption, thereby accelerating the reaction kinetics and reducing the overpotential.25,26 Recently, Ni catalysts have been investigated for the cost-effective AOR. Xu et al. reported a bimetallic NiCu catalyst that could achieve 80% removal of ammonia in 500 ppm NH3 solution.27 Almomani et al. reported that an in situ formed Ni(OH)2 layer over NiO endowed the NiO-TiO2 composite catalyst with over 90% removal of N in wastewater with a low ammonia concentration of <100 ppm.28
Although inspiring progress has been made in the AOR, the development of CO2RR-AOR full electrolysis remains immature. Klinkova's group evaluated the CO2RR-AOR couple featuring an Ag/C cathode and Pt/C anode in an H-type cell.29 Compared with the CO2RR-OER, the cell voltage decreased by 0.78 V at a current density (j) of 0.1 mA cm−2 with the addition of 1 M ammonia in the anolyte. However, the improvement shrank to 0.32 V when j was increased to 10 mA cm−2. Besides, the Pt/C catalyst got poisoned easily due to the overstrong adsorption of *N-species, and showed no activity towards the AOR after a four-hour test.
As shown above, in addition to the low solubility of CO2 in aqueous solution (∼34 mM under ambient conditions), the poor mass transfer efficiency over both the anode and the cathode in commonly used H-type cells heavily hindered the electrocatalytic performance of the CO2RR-AOR couple. To overcome the mass transfer limitation, a flow cell with a cathodic gas diffusion electrode (GDE) was fabricated. Both the anolyte and catholyte flow in the flow field immediately remove the products and refresh the electrolyte near the electrode, thereby exposing active sites with a high-concentration of local ammonia and CO2. Moreover, the cathodic GDE helps to construct a three-phase interface composed of electrode-water-CO2, which prominently enhances the contact between the catalyst and CO2 and thus boosts the current density (a more detailed discussion is presented in Section 3.2). Choi et al. successfully coupled the CO2RR with the AOR in a closely packed gas diffusion electrolyser consisting of a Au cathode and a Pt/C anode (Fig. 2a).30 The cell voltages of the CO2RR-OER and CO2RR-AOR were 3.0 and 2.0 V at 10 mA cm−2, respectively, and the disparity increased to 1.5 V at 50 mA cm−2, demonstrating the boosted energy efficiency for the CO2RR-AOR couple in a gas diffusion electrolyser (Fig. 2b). Moreover, the H2/CO ratio of the cathodic product syngas could be optimized by changing the NH3 concentration in the anolyte (Fig. 2c). However, a similar activity decrease was also observed for anodic Pt/C in this work. In addition, it should be pointed out that these reported CO2RR-AOR couples require an extremely high concentration of ammonia to markedly reduce the full cell voltage, whereas the concentration of NH3 (calculated by N mass) in domestic or agricultural effluents is usually below 100 mg L−1, equal to approximately 7 mM NH3, which means that extra NH3 enrichment procedures are demanded for the wastewater before being applied in the CO2RR-AOR couple. Therefore, highly active catalysts that can efficiently convert low concentrations of NH3 with a prolonged lifetime are crucial for the utilization of the CO2RR-AOR technique.
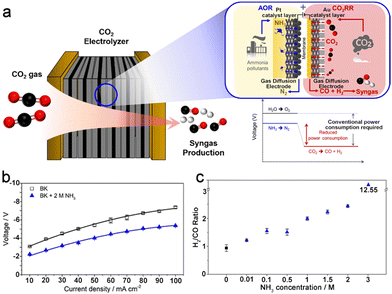 |
| Fig. 2 (a) Schematic illustration of the CO2RR-AOR couple in a gas diffusion electrolyser. (b) Voltages at different current densities in 0.5 M KHCO3 (BK) or 0.5 M KHCO3 + 2 M NH3 (BK + 2 M NH3). (c) H2/CO ratio of syngas as a function of the NH3 concentration at 10 mA cm−2. Reproduced with permission from ref. 30. Copyright 2022, Elsevier Ltd. | |
For the UOR, apart from the dehydrogenation N–N coupling steps, it also involves the breakage of the C–N bond before the formation of N2.31 Jiang et al. found that on O-incorporated NiMoP nanotube arrays, the last dehydrogenation step from *CONHN to *CON2 and subsequent C–N dissociation of *CON2 into *CO and N2 were the slow steps for the UOR, whereas the other steps were all spontaneous.12 In an electrolyte containing 1.0 M KOH + 0.5 M urea, a potential of merely 1.41 V vs. RHE was required to deliver a j value of 100 mA cm−2, 340 mV lower than that of the OER, indicating the improved energy efficiency for the UOR. Besides, the result of long-term electrolysis revealed that the current density slightly dropped from 20 to 17.5 mA cm−2 after 40 h of the UOR, implying the fine stability of the catalyst. Recently, Clark et al. investigated the feasibility of coupling the CO2RR with the UOR using Sn wire and a NiFe film as corresponding electrodes.32 The voltage of the full electrolyser was reduced by the addition of 0.25 M urea in the anolyte, and the FE of the CO2RR was even slightly higher than that of CO2 coupled with the OER.
Hydrazine is also a pollutant in wastewater, and many efforts have been devoted to the development of efficient HzOR catalysts. HzOR involves 4-e− transfer with extremely low theoretical potential (N2H4 + 4OH− → N2 + 4H2O + 4e−; E0 = −0.33 V vs. RHE), which is beneficial to reduce the full cell voltage when employed as the anode reaction. Zhu et al. fabricated a Ni/C hybrid nanosheet array catalyst for efficient HzOR.10 DFT calculation results revealed that the Ni sites in the hybrid catalyst favoured the dehydrogenation of N2H4 to N2, thereby enhancing the HzOR performance. Shi's group reported an unusual HzOR pathway on the CoP/NiCoP heterogenous interface featuring the cleavage of the N–N bond before dehydrogenation with a reduced energy barrier.11 As a result, a potential of merely 0.09 V vs. RHE was required to deliver 200 mA cm−2 current density for HzOR by the optimized catalyst in 1.0 M KOH + 0.1 M N2H4, 1.54 V lower than that for OER by RuO2. However, the CO2RR-HzOR couple has not been reported, to our knowledge. In fact, we can only find limited literature that has reported the successful coupling of the CO2RR with the above water pollutant degradation reactions, whereas the latter have already been employed to couple with the hydrogen evolution reaction (HER). The main reason is that the CO2RR performance is closely influenced by the catalyst activity, gas–liquid–solid interfacial mass transfer, electrolyte types, etc., much more complicated than the case of water splitting. Moreover, the requirement of high concentrations of pollutants to significantly reduce the full cell voltage leads to extra energy-consuming preconcentration of wastewater. Accordingly, further techno-economic analysis is required to evaluate the coupling of the CO2RR with Category I reactions, and developing highly active catalysts that can deal with low concentrations (ppm level) of pollutants may address the issue.
2.2. Category II
The chlorine oxidation reaction (COR) is one of the most crucial chemical industrial techniques as the products Cl2 and ClO− are important feedstocks in the pharmacy, disinfection, and rubber industries. Coupling CO2 reduction to CO with the COR enables simultaneous production of CO, KHCO3, and Cl2 with 100% atom economy theoretically (3CO2 + 2KCl + H2O → CO + 2KHCO3 + Cl2). For example, Guo et al. have successfully assembled a carbon black supported Ni catalyst cathode and commercial dimensional stable anode in a gas diffusion electrolyser for the efficient CO2RR-COR (Fig. 3a).33 High FEs of up to 98.5% and 75.2% were achieved at 250 mA cm−2 for CO2RR-to-CO and COR-to-Cl2, respectively. Lu's group introduced ordered mesoporous structures into cathodic Ni–N–C and anodic Co3O4 catalysts to enhance the activity of the CO2RR-COR couple.34 The improved mass transfer owing to the ordered mesoporous structure effectively boosted the current density. Moreover, the cell voltage was sharply reduced from 4.5 to 2.7 V to achieve 20 mA cm−2 with the substitution of the OER with the COR (Fig. 3b), and the energy efficiency (EE) for the cathode was increased by 40% (Fig. 3c). Qiu's group have evaluated the economy and the net reduction of CO2 for the CO2RR-COR using seawater as the electrolyte, and the results suggested a total cell efficiency for producing CO and Cl2 exceeding 100% without extra CO2 emission (Fig. 3d).35 The above work demonstrates the huge potential and viability of the sCO2RR-COR couple in the industrial process.
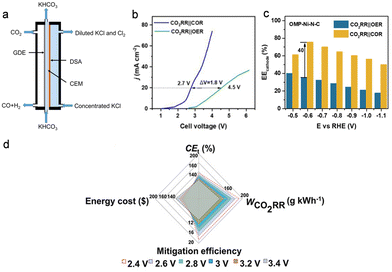 |
| Fig. 3 (a) Schematic illustration of the flow cell for the CO2RR-COR couple. Reproduced with permission from ref. 33. Copyright 2020, Elsevier Ltd. (b) Linear sweep voltammetry curves and (c) cathode energy efficiencies for the CO2RR coupled with the COR or OER. Reproduced with permission from ref. 34. Copyright 2022, Elsevier Ltd. (d) CO2 emission and economy evaluation via total cell efficiency, mitigation efficiency, the weight of CO2 reduced per kWh electricity consumption, and energy cost on the basis of per kWh electricity consumption for the CO2RR-COR couple in seawater. Reproduced with permission from ref. 35. Copyright 2021, John Wiley & Sons, Inc. | |
H2O oxidation to H2O2 rather than O2 is attracting increasing interest. Although the theoretical potential for H2O2 formation (1.76 V vs. RHE) is higher than that of O2 (1.23 V vs. RHE), 2-e− transfer of the H2O2 evolution reaction makes it more dynamically favoured than the OER. However, no work has been reported focused on coupling the CO2RR with the H2O2 evolution reaction to date. The Wang group found a CO2/carbonate mediated electrochemical H2O oxidation for high-performance H2O2 production.36 The carbonate ions could steer the 4-e− pathway to 2-e− through carbonate radicals and percarbonate intermediates. Consequently, an industrial scale current density of up to 1.3 A cm−2 was achieved with an H2O2 FE of 70% in the K2CO3 electrolyte. This inspiring result indicates the possibility of coupling the CO2RR with H2O oxidation to H2O2 in the anion exchange membrane (AEM) electrolyser where in situ formed CO32− in the cathode can migrate to the anode to help boost the formation of H2O2.
2.3. Category III
Methanol is a main product in methane conversion and an important building block for the chemical industry. Selective oxidation of methanol to formic acid (formate) is desired given the large disparity in prices of methanol (∼$200 per ton) and formic acid (∼$600 per ton). Wei et al. fabricated CuO nanosheets on Cu foam for the efficient methanol oxidation reaction (MOR) (Fig. 4a).16 A high FEformate of up to 95% was achieved for the MOR, and the potential was decreased by 250 mV to achieve a current density of 10 mA cm−2 compared with that for the OER. Besides, mesoporous SnO2 on carbon cloth was prepared for the efficient CO2RR with 81% FEformate. For the CO2RR-MOR couple, it required only 0.93 V to deliver a current density of 10 mA cm−2, which was 500 mV lower than that for the CO2RR-OER. Another work by Cao et al. presented a CO2RR-MOR full electrolyser equipped with Bi- and Ni-metal–organic framework-derived catalysts as the respective cathode and anode.17 A current density of 27 mA cm−2 was achieved at a cell voltage of 3.0 V with a FEformate of ∼100% at both electrodes (Fig. 4b). Lan's group successfully integrated metalloporphyrin (Ni) and Ni8 clusters in PCN-601 as a bifunctional electrocatalyst for the CO2RR-MOR couple.37 The Ni site in metalloporphyrin served as the active centre for the CO2RR to CO, while the Ni8 cluster acted as the site for the MOR to formate. Consequently, high FECO and FEformate values of over 90% were obtained at 2.2 V with a current density of 7 mA cm−2. Nevertheless, the current densities above are too low to meet industrial requirements. Recently, our group developed a bifunctional CuSn alloy electrocatalyst with a hierarchical structure for the CO2RR-MOR couple at a high current density.15 In an H-type cell, the CO2RR-MOR couple delivered a current density as high as 125 mA cm−2 with near-unity FEformate at both sides, corresponding to a formate production rate of up to 3313 μmol h−1 cm−2. Such a strategy contributed to a reduction in energy consumption by 40% for formate synthesis. Moreover, in situ Raman spectroscopic results revealed that the CH3OH adsorbed on the catalyst surface via an O atom followed by a series of dehydrogenation steps to form an *OCH intermediate. Subsequently, *OCHO was generated through the addition of an O atom from the OH group to *OCH and then desorbed from the CuSn alloy surface for formate formation (Fig. 4c and d). This reaction pathway was also verified on partially pyrolyzed Ni–organic frameworks.18 The in situ formed NiOOH sites were conducive to O–H activation and thus promote the oxidation of methanol to formate. In a zero-gap membrane electrode assembly (MEA), the CO2RR-MOR couple exhibited an excellent performance of ∼500 mA cm−2 at 2.2 V with over 90% FEs for both CO2RR-to-CO and MOR-to-formate, ranking top among the up-to-date CO2RR-MOR systems (Fig. 4e and f).
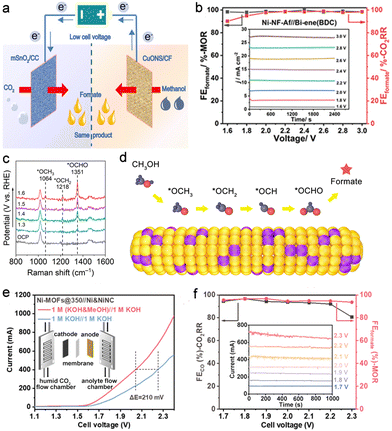 |
| Fig. 4 (a) Schematic illustration of the CO2RR-MOR couple. Reproduced with permission from ref. 16. Copyright 2020, John Wiley & Sons, Inc. (b) Cathodic and anodic FEformate of the CO2RR-MOR couple using Bi- and Ni-MOF-derived materials as corresponding electrodes. Reproduced with permission from ref. 17. Copyright 2021, John Wiley & Sons, Inc. (c) In situ Raman spectra and the (d) proposed reaction pathway for the MOR on CuSn alloy. Reproduced with permission from ref. 15. Copyright 2022, Elsevier Ltd. (e) Polarization curves (inset shows the MEA scheme) and (f) voltage-dependent FE (inset shows the corresponding i-t curves) for the CO2RR-MOR in an MEA electrolyser. Reproduced with permission from ref. 18. Copyright 2023, American Chemical Society. | |
Electrochemical upgrade of biomass-derived materials such as glycerol, furfurals and glucose into useful chemicals is thermodynamically more favourable than the OER owing to the –CHO and –OH groups. Glycerol is a massive byproduct of the transesterification process for biodiesel and soap production, and it is profitable to upgrade glycerol into other chemicals through the glycerol oxidation reaction (GOR). Verma et al. performed a techno-economic analysis of cradle-to-gate CO2 emissions of the overall CO2RR process using grid electricity, and the result indicated that only HCOOH could be produced in a carbon-neutral or -negative manner when the OER was employed as the anodic reaction.38 As a step further, they found that using the GOR on Pt black at the anode lowered the onset potential for the overall CO2 electrolysis by 0.85 V, contributing to an energy consumption reduction of 53%, which drastically reduced the operating costs and carbon footprint of CO2 electrolysis and thereby made the production of CO, C2H4, and CH3CH2OH become carbon-neutral, strongly evidencing the economic viability of the CO2RR-GOR couple. However, there are various products for the GOR such as glyceraldehyde, glycerate, lactate, glycolate, formate, and carbonate, resulting in poor selectivity. A flow cell consisting of a Bi/C cathode and Pt/C anode has been reported for coupling the CO2RR with the GOR.39 While a high formate concentration of 18 g L−1 was obtained by the cathodic CO2RR with over 80% FE at 200 mA cm−2, a low FE of ∼20% was delivered for formate by the anodic GOR owing to the severe overoxidation evidenced by the high FE of up to 40% for the carbonate product. Wang et al. constructed an F, N co-doped carbon nanosheet-supported Ni single atom cathode and carbon cloth-supported CoSe2 anode to couple the CO2RR with the GOR (Fig. 5a).40 A long-term continuous electrolysis of 400 h was achieved at 100 mA cm−2 with both the cathodic FECO and anodic FEformate kept over 90%. Apart from formate, another useful chemical glyceraldehyde was selectively generated at the anode of the CO2RR-GOR by Reisner's group.41 They reported a hybrid assembly based on a (2,2,6,6-tetramethylpiperidin-1-yl)oxyl electrocatalyst modified with a silatrane-anchor for selective glyceraldehyde production from glycerol with an FE of up to 83%; meanwhile, syngas was produced at the cathode using cobalt phthalocyanine on carbon nanotubes as the catalyst (Fig. 5b).
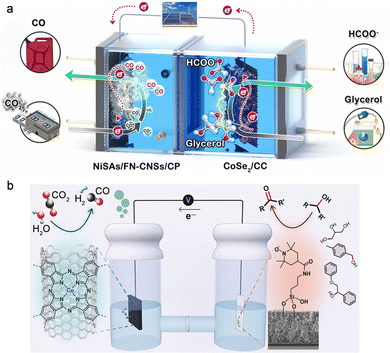 |
| Fig. 5 (a) Schematic illustration of the electrolyser featuring CO2RR-to-CO coupled with GOR-to-formate. Reproduced with permission from ref. 40. Copyright 2022, Elsevier Ltd. (b) Coproduction of syngas and glyceraldehyde using the CO2RR-GOR couple. Reproduced with permission from ref. 41. Copyright 2020, John Wiley & Sons, Inc. | |
The electrochemical 5-hydroxymethylfurfural (HMF) oxidation reaction (HMFOR) has attracted increasing attention.42–45 Composed of a –CHO and a –CH2OH with a furan ring, HMF can be oxidized to 2,5-diformylfuran (DFF) via initial –CH2OH oxidation, or 5-hydroxymethyl-2-furancarboxylic acid (HMFCA) via initial –CHO oxidation. Subsequently, the DFF and HMFCA can be further oxidized to 2-formyl-5-furancarboxylic acid (FFCA), and finally to 2,5-furandicarboxylic acid (FDCA) (Fig. 6). The choice of the DFF-pathway or HMFCA-pathway for the oxidation of HMF to FDCA greatly depends on the pH of the electrolyte.46 Generally, the HMFOR undergoes the HMFCA-path in a strong alkaline electrolyte (pH > 13) due to the preferential adsorption of the aldehyde group on the catalyst, whereas the DFF-pathway is favoured in the electrolyte with pH < 13 where the adsorption of the alcohol group becomes thermodynamically more favourable.46 To further investigate the HMFOR pathway, Wang's group developed a vibrational spectroscopy technique using in situ sum frequency generation to monitor the interfacial process during the HMFOR.47 A signal related to HMFCA emerged and grew stronger as the electrolysis time was prolonged, which validated the HMFCA-pathway for the HMFOR. To simultaneously promote the oxidation of –CH2OH and –CHO, Lu et al. introduced Ni into the tetrahedral catalytic sites of cobalt spinel oxides.48 The Ni and Co sites served as active centres for –CH2OH and –CHO adsorption, respectively, thus enhancing the HMFOR with a high yield of 92.4% and FE of 90.3% for FDCA.
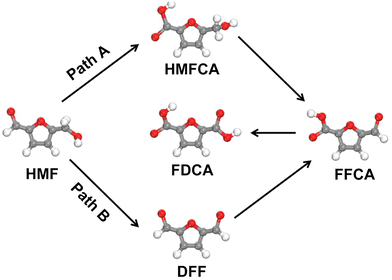 |
| Fig. 6 Reaction pathways for the HMFOR. | |
With the remarkable progress made in the HMFOR, however, few works about the CO2RR-HMFOR couple have been reported so far. Recently, Han's group carried out the coupling of the HMFOR with the CO2RR (Fig. 7a and b).19 The full electrolyser featuring PdOx/ZIF-8 as the cathode and PdO as the anode only required an onset cell voltage of 1.06 V for the CO2RR-HMFOR couple, 0.71 V lower than that of the CO2RR-OER couple (Fig. 7c). Different from previous studies for the HMFOR, an acidic anolyte containing H2SO4 (0.5 M)/CH3CN (6.0 M)/H2O was employed, and the products were mainly maleic acid and formic acid, indicating that a strong acidic environment may lead to an unconventional reaction pathway involving C–C breaking and furan ring opening for the HMFOR. Notably, the organic acid yield reached 84.3% for the HMFOR, including 60.0% formic acid and 24.3% maleic acid, accompanied by a FECO of 97.0% for the CO2RR at 103.5 mA cm−2 (Fig. 7d and e).
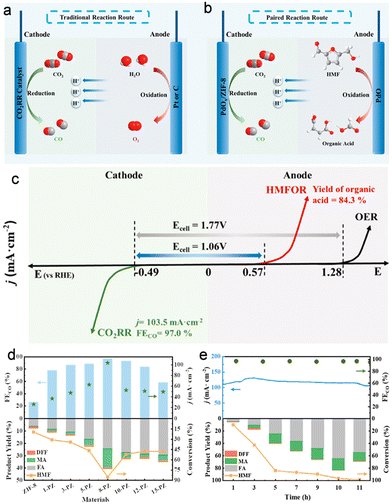 |
| Fig. 7 Overview of (a) CO2RR-OER and (b) CO2RR-HMFOR routes. (c) Comparison between the CO2RR-OER and the CO2RR-HMFOR. (d) CO2RR and HMFOR performances with different anodic catalysts. (e) Long-term electrolysis results of the CO2RR and HMFOR. Reproduced with permission from ref. 19. Copyright 2022, American Chemical Society. | |
There are also some other oxidation reactions that have already been or have the potential to be coupled with the CO2RR. Sulfur production through the sulfide oxidation reaction (SOR) is an appealing candidate for the anodic reaction in overall CO2 electrolysis by virtue of its low potential (0.14 V vs. RHE). Besides, the ingredient H2S/S2− widely exists in industrial exhaust/effluent. Therefore, coupling the CO2RR with the SOR is also of environmental value. Liu's group recently carried out a co-electrolysis system consisting of Bi for CO2RR-to-formate, and S, Cu-codoped cobalt hydroxide for SOR-to-sulfur, which showed a pronounced reduction in energy consumption.49 To avoid the deposition of S on the anode, an alkaline electrolyte (1 M KOH + 1 M K2S) was employed to generate soluble Sx2− from the direct SOR. As a result, the substitution of the OER with the SOR markedly lowered the cell voltage from 3.43 to 2.10 V at 100 mA cm−2, with near-unity faradaic efficiencies for both sides. Economic evaluation based on the cell operation conditions and the prices of cathodic and anodic products revealed a gross margin of $0.35 kW h−1 for the CO2RR-SOR, about 2 times as high as that for the CO2RR-OER ($0.18 kW h−1), further demonstrating the economic viability of the former. Electricity-driven partial oxidation of methane is also a promising anodic reaction of overall CO2 electrolysis.50,51 Lu et al. developed an efficient solid oxide electrolyser for the coupling of CO2 reduction with CH4 oxidation to produce syngas.52 However, the high operating temperature (1073 K) for O2− transport led to extra energy input. Given the insoluble CH4, the construction of a high-quality gas–liquid–solid interface using gas diffusion electrodes will favour the efficient coupling of the CO2RR with CH4 oxidation. In addition to the aforementioned small molecule oxidation, electrochemical upcycling of plastic waste53 or reforming of raw biomass such as lignin54 and chitin55 are also competing substitutions for the OER. In particular, electrochemical conversions of lignin and chitin have already been successfully employed as the anodic reaction for energy-saving hydrogen evolution systems, demonstrating the possibility for CO2 electrolysis.55,56 However, achieving high selectivity for the target product remains a huge challenge due to the complicated structures and decomposition pathways for these macromolecules. Moreover, in addition to the electrolysis device design, comprehensive techno-economic analysis covering not only the design of electrolysis devices but also the up- and down-stream supply chain is urgently demanded for guidance on the development of industrial-scale plants.
3. Challenges in coupling the CO2RR with alternative anodic reactions
3.1. Trade-off between conversion and current density
To reduce the cell voltage and enhance the current density for overall CO2 electrolysis, a high-concentration substrate is usually required on the anodic side. However, the concentration of the substrate decreases as the electrolysis time is prolonged, which leads to a continuous drop in current density. Besides, the faradaic efficiencies of these alternative anodic reactions may also decrease due to the low reactant concentration. To address this issue, one common way is to refresh the anolyte with a high-concentration substrate.15 However, such a strategy heavily restricts the conversion of the substrate, and brings extra procedures for the separation of products and the unreacted substrate. A more feasible solution is to develop efficient catalysts that are able to selectively convert the substrates at low concentrations. Wang's group fabricated a catalyst featuring dispersed Ru atoms in a Cu nanowire matrix towards efficient NO3− reduction to NH3.57 An industrial-scale current density of up to 1 A cm−2 was achieved with an NH3 FE of 93% at a low nitrate concentration of typical industrial wastewater (2000 ppm). More importantly, 99% of the nitrate could be converted to NH3 with the FE maintained over 90% at 400 mA cm−2, with the concentration of the nitrate residue as low as 50 ppm. Although this work is focused on the cathodic process, we can learn from it that active sites with a high density (dispersed Ru atoms) and porous structure with a high surface area (hollow Cu nanowire matrix) endow the catalyst with enhanced performance at a low substrate concentration. Besides, increasing the local substrate concentration near the electrode surface with functional groups may also help to maintain the faradaic efficiency and current density when the substrate concentration is reduced. For example, amine groups anchored on the electrode surface can capture dilute CO2 and thus enhance the FE for the CO2RR.58,59
3.2. Electrolyser design
In addition to electrocatalysts, electrolysers with different architectures also influence the performance of overall CO2 electrolysis.60,61 H-type cells are widely employed in various electrochemical reactions due to their simple and cost-effective setup. However, the low CO2 solubility in aqueous solution (∼34 mM under ambient conditions) and the slow CO2 diffusion limit the mass transfer efficiency over the electrode, leading to inferior current density. Moreover, the excessive supply of H2O and insufficient supply of CO2 over the immersed electrode are unfavoured for the sCO2RR to multi-carbon products (denoted as C2+, i.e., C2H4 and C2H5OH) as high concentrations of CO2 or CO intermediates are preferred for the C–C coupling process.62–64 Besides, the long distance between the anode and the cathode inevitably results in a pronounced voltage drop due to the high resistance.
Compared with H-type cells, flow cells enable efficient mass-transfer for industrial-scale current density owing to the high-quality catalyst-electrolyte-gas tri-phase interface and the continuous electrolyte/gas flow (Fig. 8a).65 Different from H-type cells, a gas diffusion electrode consisting of a hydrophobic gas diffusion layer (GDL) and catalyst layer is required. Gaseous CO2 can efficiently diffuse across the GDL to the catalyst layer to meet the catholyte, thereby forming the above-mentioned tri-phase interface for the enhanced CO2RR. The flowing anolyte also promotes the removal of products and attached bubbles and facilitates mass transfer near the anode, thereby enhancing the anodic reaction rate. However, flow cells also show drawbacks. The hydrophobic surface of the GDE gradually becomes hydrophilic during high-current electrolysis, which leads to catholyte flooding and gas feed blocking. Besides, the commonly used carbon paper to fabricate GDL is broken easily in the electrolyte/gas flow due to its weak mechanical strength. To alleviate these issues, Dinh et al. reported a polytetrafluoroethylene (PTFE)/Cu/carbon nanoparticles/graphite composite GDE which could run for 150 h with the C2H4 FE maintained over 60%.64 In sharp contrast, the C2H4 FE rapidly dropped to 10% within 1 h for the conventional carbon paper/Cu GDE. The robust PTFE with a hydrophobic surface effectively prevented flooding, and the carbon nanoparticles and graphite layer stabilized the Cu catalyst surface.
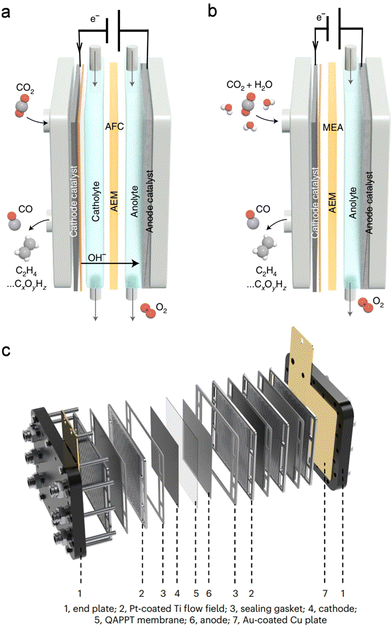 |
| Fig. 8 Schematic of (a) an alkaline electrolyte flow cell (AFC) and (b) an MEA electrolyser. Reproduced with permission from ref. 65. Copyright 2022, Springer Nature. (c) Scale-up electrolyser stack with four 10 × 10 cm2 MEAs. Reproduced with permission from ref. 67. Copyright 2023, Springer Nature. | |
A catholyte-free MEA electrolyser provides a solution to prevent the flooding issue (Fig. 8b).65,66 Moreover, the closely packed cathode–membrane–anode structure of the MEA minimizes the electricity resistance for enhanced energy efficiency. Zhou et al. reported that in a flow cell, the CO2RR-MOR required 2.74 V to deliver a current density of 100 mA cm−2, whereas a high current density approaching 500 mA cm−2 was achieved at merely 2.4 V in an MEA electrolyser.18 This is especially important for a scale-up stack where several MEAs are integrated. An electrolyser stack with four 10 × 10 cm2 MEAs was successfully constructed for scale-up CO2 electrolysis by Bao's group (Fig. 8c).67 Impressively, a current of 60 A was achieved with a C2+ FE of over 80% at a cell voltage of ∼13.5 V, and the current could be further increased to 150 A with nearly 90% C2+ FE at a cell voltage less than 11 V when fed with CO. Such a scale-up device provides an appealing way for practical utilization of CO2 electrolysis, and further improvements are required to couple the CO2RR with various value-added anodic reactions in the future.
In addition to the electrolyser architecture, the electrolyte also plays a pivotal role in electrolysis. An alkaline solution is employed in many CO2RR cases owing to the low ohmic resistance and effective suppression of the HER. However, severe CO2 loss (75–95%) occurs under these conditions due to the rapid reaction of CO2 with alkali to form carbonate and bicarbonate (Fig. 9), and the regeneration of the alkaline electrolyte accounts for 60–75% of total energy consumption.65 Moreover, CO2RR products such as formate can diffuse across the anion exchange membrane to the anodic part, leading to difficulties in product collection and separation. Therefore, the acidic CO2RR seems attractive. Unfortunately, most of the alternative anodic reactions such as CH3OH, glycerol, and HMF oxidation are kinetically favourable in alkaline electrolytes. To deal with the above issues, a bipolar membrane was adopted.17 Nevertheless, the sluggish water dissociation into H+ and OH− inside the bipolar membrane as well as the increased membrane thickness leads to extra voltage loss compared with an AEM or cation exchange membrane (CEM). In addition, the higher expense of the bipolar membrane also hinders its application.
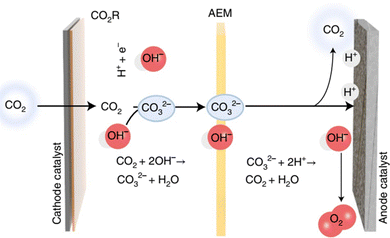 |
| Fig. 9 CO2 loss via carbonate formation and crossover to the anodic side under alkaline conditions. Reproduced with permission from ref. 65. Copyright 2022, Springer Nature. | |
3.3. Development of an electrocatalyst under acidic conditions
As mentioned above, overall CO2 electrolysis in acidic electrolytes can address the issue of CO2 loss for improved energy efficiency. Although performing the CO2RR under acidic conditions is preferred, and indeed improved performances were obtained for the acidic CO2RR by controlling the catalyst surface microenvironments,68–72 few catalysts have shown promising activity for the alternative anodic reactions in acidic anolyte. In fact, the hydroxyl group (*OH) plays a pivotal role in the dehydrogenation processes of the anodic oxidation reactions, the formation of which is hindered due to the acidic conditions.73 Moreover, operation at the positive potential in an acidic solution will cause rapid decomposition of the non-noble metal anode, resulting in poor stability. A recent work presented an electrocatalyst featuring MnO2 nanosheets for the acidic GOR.74 A long-term steady electrolysis of over 800 h was achieved at 10 mA cm−2 in 0.2 M glycerol + 0.005 M H2SO4. However, both formate and CO2 were obtained with close FEs (∼50% for each), indicating the easy overoxidation of glycerol under acidic conditions. Therefore, developing highly acid-tolerant electrocatalysts with enhanced activity and selectivity remains a great challenge, and demands further investigations.
3.4. Selectivity control for target products
It is quite challenging to control the selectivity of certain products for anodic organic oxidations. For instance, in alcohol oxidations, the C–OH group easily gets oxidized to –COOH rather than –CHO. Therefore, partial oxidation of alcohol to aldehyde is difficult. It becomes more challenging to selectively produce intermediate chemicals when there are more than one reductive group in the reactant molecule. For the HMFOR, owing to the coexistence of –CHO and –CH2OH groups in an HMF molecule, the oxidation of HMF to FDCA can proceed via HMFCA or DFF (Fig. 6). However, given the widely-used alkaline electrolyte for the HMFOR in the CO2RR-HMFOR couple, the HMFCA pathway is usually preferred and thus it is difficult to obtain DFF in such a coupling system.46 Besides, C–C bond breaking may occur under harsh conditions for multi-carbon molecules, increasing the variety of products.19 Moreover, overoxidation of organic molecules to CO2 was also observed at high potentials/current densities. For example, quite an amount of CO2 can be generated during the MOR on Pt and IrO2 catalysts at ∼100 mA cm−2.15 Simultaneously achieving high current density and selectivity in these alternative organic oxidation reactions remains challenging. As for the cathodic side, although most works show no significant change in the selectivity of the CO2RR when the CO2RR is coupled with alternative anodic oxidation reactions, sometimes the organic molecules may cross the ion-exchange membrane to the cathodic side and influence the CO2RR process.
4. Conclusions and perspectives
Coupling CO2RR to various anodic oxidation reactions with value-added products provides a novel avenue for enhancing the economic output of overall CO2 electrolysis. In this review, a series of oxidation reactions have been discussed as competing alternatives to the OER with reference to the latest advances. We have also pointed out the major challenges in achieving efficient overall CO2 electrolysis with alternative anodic reactions. Despite the pronounced advancement made in the coupling strategy, this field is still nascent.
The design of efficient electrocatalysts requires an in-depth understanding of the mechanisms, which largely relies on advanced characterization studies and theoretical simulations.75 Recently, in situ infrared spectroscopy, X-ray photoelectron spectroscopy (XPS), X-ray absorption fine structure spectroscopy and other techniques have been adopted in many works, providing useful local information about reaction intermediates. However, given the complex microenvironment over the catalyst's surface during the reaction, detecting the evolution of active sites and intermediates under working conditions using operando characterization is crucial to uncover the true reaction pathways, which is still difficult owing to the harsh conditions such as high vacuum for XPS and the severe decay and disturbance of signals by the electrolyte for infrared spectroscopy.76,77 The design of electrolysers that are compatible to various operando and time-resolved characterization studies is in urgent demand.
As for theoretical simulation, the accuracy of the model directly influences the calculation result, which is, however, extremely challenging in heterogeneous electrocatalysis considering the interfacial diversity. The structures of active sites are not uniform over the catalyst surface. Moreover, the active sites may evolve during catalysis, making it more difficult to obtain accurate surface structures for the simulation. Besides, the electrolyte near the electrode should also be taken into account in the simulation. The ion concentration and solvation effect may influence the adsorption of intermediates, thus leading to different calculation results. Obviously, combining advanced calculation methods with operando characterization holds the key to deciphering the in-depth reaction mechanisms, and therefore benefits the development of high-performance electrocatalysts.
Beyond the catalysts, the overall design of the whole electrolysis system is also crucial, which has not received enough attention currently. For electrolysers, the flow fields for both the anode and cathode should be specially designed to achieve efficient mass transfer. Besides, the feeding rates for CO2 and anodic reactants should be well adjusted to optimize the total conversion rates. In addition, for industrial applications, the feeding, collecting, and recycling systems should match with each other for steady operation. A well-established overall CO2 electrolysis system with comprehensive technoeconomic evaluations requires scientists from various backgrounds including chemistry, materials science, physics, engineering, economics, etc. It is expected that continuous efforts will propel this technique to industrial application in the near future.
Author contributions
Y. L. and T. L. supervised the project. Y. L. wrote the manuscript.
Conflicts of interest
There are no conflicts to declare.
Acknowledgements
This work was supported by the National Key R & D Program of China (2017YFA0700104), the National Natural Science Foundation of China (21905204 and 21931007), and the 111 Project of China (D17003).
References
- C. Chen, J. F. Khosrowabadi Kotyk and S. W. Sheehan, Progress toward commercial application of electrochemical carbon dioxide reduction, Chem, 2018, 4, 2571–2586 CAS.
- R. Francke, B. Schille and M. Roemelt, Homogeneously catalyzed electroreduction of carbon dioxide—methods, mechanisms, and catalysts, Chem. Rev., 2018, 118, 4631–4701 CrossRef CAS PubMed.
- P. Zhu, C. Xia, C.-Y. Liu, K. Jiang, G. Gao, X. Zhang, Y. Xia, Y. Lei, H. N. Alshareef, T. P. Senftle and H. Wang, Direct and continuous generation of pure acetic acid solutions via electrocatalytic carbon monoxide reduction, Proc. Natl. Acad. Sci. U. S. A., 2021, 118, e2010868118 CrossRef CAS PubMed.
- J. Gu, C.-S. Hsu, L. Bai, H. M. Chen and X. Hu, Atomically dispersed Fe3+ sites catalyze efficient CO2 electroreduction to CO, Science, 2019, 364, 1091–1094 CrossRef CAS PubMed.
- J. Na, B. Seo, J. Kim, C. W. Lee, H. Lee, Y. J. Hwang, B. K. Min, D. K. Lee, H.-S. Oh and U. Lee, General technoeconomic analysis for electrochemical coproduction coupling carbon dioxide reduction with organic oxidation, Nat. Commun., 2019, 10, 5193 CrossRef.
- M.-Y. Qi, M. Conte, M. Anpo, Z.-R. Tang and Y.-J. Xu, Cooperative coupling of oxidative organic synthesis and hydrogen production over semiconductor-based photocatalysts, Chem. Rev., 2021, 121, 13051–13085 CrossRef CAS.
- L. Yuan, M.-Y. Qi, Z.-R. Tang and Y.-J. Xu, Coupling strategy for CO2 valorization integrated with organic synthesis by heterogeneous photocatalysis, Angew. Chem., Int. Ed., 2021, 60, 21150–21172 CrossRef CAS PubMed.
- M.-Y. Qi, M. Conte, Z.-R. Tang and Y.-J. Xu, Engineering semiconductor quantum dots for selectivity switch on high-performance heterogeneous coupling photosynthesis, ACS Nano, 2022, 16, 17444–17453 CrossRef CAS PubMed.
- F.-K. Shang, Y.-H. Li, M.-Y. Qi, Z.-R. Tang and Y.-J. Xu, Photocatalytic materials for sustainable chemistry via cooperative photoredox catalysis, Catal. Today, 2023, 410, 85–101 CrossRef CAS.
- Y. Zhu, J. Zhang, Q. Qian, Y. Li, Z. Li, Y. Liu, C. Xiao, G. Zhang and Y. Xie, Dual nanoislands on Ni/C hybrid nanosheet activate superior hydrazine oxidation-assisted high-efficiency H2 production, Angew. Chem., Int. Ed., 2022, 61, e202113082 CrossRef CAS.
- L. Zhu, J. Huang, G. Meng, T. Wu, C. Chen, H. Tian, Y. Chen, F. Kong, Z. Chang, X. Cui and J. Shi, Active site recovery and N-N bond breakage during hydrazine oxidation boosting the electrochemical hydrogen production, Nat. Commun., 2023, 14, 1997 CrossRef CAS PubMed.
- H. Jiang, M. Sun, S. Wu, B. Huang, C.-S. Lee and W. Zhang, Oxygen-incorporated NiMoP nanotube arrays as efficient bifunctional electrocatalysts for urea-assisted energy-saving hydrogen production in alkaline electrolyte, Adv. Funct. Mater., 2021, 31, 2104951 CrossRef CAS.
- S.-S. Liu, Q.-J. Xing, Y. Chen, M. Zhu, X.-H. Jiang, S.-H. Wu, W. Dai and J.-P. Zou, Photoelectrochemical degradation of organic pollutants using BiOBr anode coupled with simultaneous CO2 reduction to liquid fuels via CuO cathode, ACS Sustainable Chem. Eng., 2019, 7, 1250–1259 CrossRef CAS.
- Q. Wang, C. Zhu, C. Wu and H. Yu, Direct synthesis of bismuth nanosheets on a gas diffusion layer as a high-performance cathode for a coupled electrochemical system capable of electroreduction of CO2 to formate with simultaneous degradation of organic pollutants, Electrochim. Acta, 2019, 319, 138–147 CrossRef CAS.
- Y. Li, C.-Z. Huo, H.-J. Wang, Z.-X. Ye, P.-P. Luo, X.-X. Cao and T.-B. Lu, Coupling CO2 reduction with CH3OH oxidation for efficient electrosynthesis of formate on hierarchical bifunctional CuSn alloy, Nano Energy, 2022, 98, 107277 CrossRef CAS.
- X. Wei, Y. Li, L. Chen and J. Shi, Formic acid electro-synthesis by concurrent cathodic CO2 reduction and anodic CH3OH oxidation, Angew. Chem., Int. Ed., 2021, 60, 3148–3155 CrossRef CAS.
- C. Cao, D.-D. Ma, J. Jia, Q. Xu, X.-T. Wu and Q.-L. Zhu, Divergent paths, same goal: a pair-electrosynthesis tactic for cost-efficient and exclusive formate production by metal–organic-framework-derived 2D electrocatalysts, Adv. Mater., 2021, 33, 2008631 CrossRef CAS.
- Y. Zhou, Z. Wang, W. Fang, R. Qi, Z. Wang, C. Xia, K. Lei, B. You, X. Yang, Y. Liu, W. Guo, Y. Su, S. Ding and B. Y. Xia, Modulating O–H activation of methanol oxidation on nickel-organic frameworks for overall CO2 electrolysis, ACS Catal., 2023, 13, 2039–2046 CrossRef CAS.
- J. Bi, Q. Zhu, W. Guo, P. Li, S. Jia, J. Liu, J. Ma, J. Zhang, Z. Liu and B. Han, Simultaneous CO2 reduction and 5-hydroxymethylfurfural oxidation to value-added products by electrocatalysis, ACS Sustainable Chem. Eng., 2022, 10, 8043–8050 CrossRef CAS.
- Y. Liang, W. Zhou, Y. Shi, C. Liu and B. Zhang, Unveiling in situ evolved In/In2O3−x heterostructure as the active phase of In2O3 toward efficient electroreduction of CO2 to formate, Sci. Bull., 2020, 65, 1547–1554 CrossRef CAS.
- T. Wang, X. Cao and L. Jiao, Progress in hydrogen production coupled with electrochemical oxidation of small molecules, Angew. Chem., Int. Ed., 2022, 61, e202213328 CrossRef CAS PubMed.
- J. Jack, W. Zhu, J. L. Avalos, J. Gong and Z. J. Ren, Anode co-valorization for scalable and sustainable electrolysis, Green Chem., 2021, 23, 7917–7936 RSC.
- L. Chen and J. Shi, Co-electrolysis toward value-added chemicals, Sci. China Mater., 2022, 65, 1–9 CrossRef CAS.
- S. A. Lee, M. G. Lee and H. W. Jang, Catalysts for electrochemical ammonia oxidation: Trend, challenge, and promise, Sci. China Mater., 2022, 65, 3334–3352 CrossRef.
- K. Siddharth, Y. Hong, X. Qin, H. J. Lee, Y. T. Chan, S. Zhu, G. Chen, S.-I. Choi and M. Shao, Surface engineering in improving activity of Pt nanocubes for ammonia electrooxidation reaction, Appl. Catal., B, 2020, 269, 118821 CrossRef CAS.
- M. J. Trenerry, C. M. Wallen, T. R. Brown, S. V. Park and J. F. Berry, Spontaneous N2 formation by a diruthenium complex enables electrocatalytic and aerobic oxidation of ammonia, Nat. Chem., 2021, 13, 1221–1227 CrossRef CAS PubMed.
- W. Xu, D. Du, R. Lan, J. Humphreys, D. N. Miller, M. Walker, Z. Wu, J. T. S. Irvine and S. Tao, Electrodeposited NiCu bimetal on carbon paper as stable non-noble anode for efficient electrooxidation of ammonia, Appl. Catal., B, 2018, 237, 1101–1109 CrossRef CAS.
- F. Almomani, R. Bhosale, M. Khraisheh, A. Kumar and M. Tawalbeh, Electrochemical oxidation of ammonia on nickel oxide nanoparticles, Int. J. Hydrogen Energy, 2020, 45, 10398–10408 CrossRef CAS.
- X. V. Medvedeva, J. J. Medvedev, S. W. Tatarchuk, R. M. Choueiri and A. Klinkova, Sustainable at both ends: electrochemical CO2 utilization paired with electrochemical treatment of nitrogenous waste, Green Chem., 2020, 22, 4456–4462 RSC.
- M. Choi, J. W. Kim, S. Chung, Y. Lee, S. Bong and J. Lee, Syngas production for Fischer-Tropsch process via co-electrolytic processes of CO2 reduction and NH3 oxidation, Chem. Eng. J., 2022, 430, 132563 CrossRef CAS.
- X. Wang, J.-P. Li, Y. Duan, J. Li, H. Wang, X. Yang and M. Gong, Electrochemical urea oxidation in different environment: From mechanism to devices, ChemCatChem, 2022, 14, e202101906 CrossRef CAS.
- R. Clark, A. Moore, M. MacInnis and E. Bertin, Investigation of urea oxidation as a potential anode reaction during CO2 electrolysis, J. Appl. Electrochem., 2021, 51, 1583–1590 CrossRef CAS.
- J.-H. Guo and W.-Y. Sun, Integrating nickel-nitrogen doped carbon catalyzed CO2 electroreduction with chlor-alkali process for CO, Cl2 and KHCO3 production with enhanced techno-economics, Appl. Catal., B, 2020, 275, 119154 CrossRef CAS.
- R. Ge, L.-Y. Dong, X. Hu, Y.-T. Wu, L. He, G.-P. Hao and A.-H. Lu, Intensified coupled electrolysis of CO2 and brine over electrocatalysts with ordered mesoporous transport channels, Chem. Eng. J., 2022, 438, 135500 CrossRef CAS.
- X. Tan, C. Yu, X. Song, C. Zhao, S. Cui, H. Xu, J. Chang, W. Guo, Z. Wang, Y. Xie and J. Qiu, Toward an understanding of the enhanced CO2 electroreduction in NaCl electrolyte over CoPc molecule-implanted graphitic carbon nitride catalyst, Adv. Energy Mater., 2021, 11, 2100075 CrossRef CAS.
- L. Fan, X. Bai, C. Xia, X. Zhang, X. Zhao, Y. Xia, Z.-Y. Wu, Y. Lu, Y. Liu and H. Wang, CO2/carbonate-mediated electrochemical water oxidation to hydrogen peroxide, Nat. Commun., 2022, 13, 2668 CrossRef CAS PubMed.
- S.-N. Sun, L.-Z. Dong, J.-R. Li, J.-W. Shi, J. Liu, Y.-R. Wang, Q. Huang and Y.-Q. Lan, Redox-active crystalline coordination catalyst for hybrid electrocatalytic methanol oxidation and CO2 reduction, Angew. Chem., Int. Ed., 2022, 61, e202207282 CrossRef CAS PubMed.
- S. Verma, S. Lu and P. J. A. Kenis, Co-electrolysis of CO2 and glycerol as a pathway to carbon chemicals with improved technoeconomics due to low electricity consumption, Nat. Energy, 2019, 4, 466–474 CrossRef CAS.
- K. Fernández-Caso, A. Peña-Rodríguez, J. Solla-Gullón, V. Montiel, G. Díaz-Sainz, M. Alvarez-Guerra and A. Irabien, Continuous carbon dioxide electroreduction to formate coupled with the single-pass glycerol oxidation to high value-added products, J. CO2 Util., 2023, 70, 102431 CrossRef.
- G. Wang, J. Chen, K. Li, J. Huang, Y. Huang, Y. Liu, X. Hu, B. Zhao, L. Yi, T. W. Jones and Z. Wen, Cost-effective and durable electrocatalysts for Co-electrolysis of CO2 conversion and glycerol upgrading, Nano Energy, 2022, 92, 106751 CrossRef CAS.
- M. A. Bajada, S. Roy, J. Warnan, K. Abdiaziz, A. Wagner, M. M. Roessler and E. Reisner, A precious-metal-free hybrid electrolyzer for alcohol oxidation coupled to CO2-to-syngas conversion, Angew. Chem., Int. Ed., 2020, 59, 15633–15641 CrossRef CAS PubMed.
- G. Yang, Y. Jiao, H. Yan, Y. Xie, A. Wu, X. Dong, D. Guo, C. Tian and H. Fu, Interfacial engineering of MoO2-FeP heterojunction for highly efficient hydrogen evolution coupled with biomass electrooxidation, Adv. Mater., 2020, 32, 2000455 CrossRef CAS PubMed.
- P. Prabhu, Y. Wan and J.-M. Lee, Electrochemical conversion of biomass derived products into high-value chemicals, Matter, 2020, 3, 1162–1177 CrossRef.
- K. Gu, D. Wang, C. Xie, T. Wang, G. Huang, Y. Liu, Y. Zou, L. Tao and S. Wang, Defect-rich high-entropy oxide nanosheets for efficient 5-hydroxymethylfurfural electrooxidation, Angew. Chem., Int. Ed., 2021, 60, 20253–20258 CrossRef CAS PubMed.
- R. Ge, Y. Wang, Z. Li, M. Xu, S.-M. Xu, H. Zhou, K. Ji, F. Chen, J. Zhou and H. Duan, Selective electrooxidation of biomass-derived alcohols to aldehydes in a neutral medium: Promoted water dissociation over a nickel-oxide-supported ruthenium single-atom catalyst, Angew. Chem., Int. Ed., 2022, 61, e202200211 CrossRef CAS PubMed.
- Y. Yang and T. Mu, Electrochemical oxidation of biomass derived 5-hydroxymethylfurfural (HMF): pathway, mechanism, catalysts and coupling reactions, Green Chem., 2021, 23, 4228–4254 RSC.
- N. Zhang, Y. Zou, L. Tao, W. Chen, L. Zhou, Z. Liu, B. Zhou, G. Huang, H. Lin and S. Wang, Electrochemical oxidation of 5-hydroxymethylfurfural on nickel nitride/carbon nanosheets: Reaction pathway determined by in situ sum frequency generation vibrational spectroscopy, Angew. Chem., Int. Ed., 2019, 58, 15895–15903 CrossRef CAS.
- Y. Lu, T. Liu, Y.-C. Huang, L. Zhou, Y. Li, W. Chen, L. Yang, B. Zhou, Y. Wu, Z. Kong, Z. Huang, Y. Li, C.-L. Dong, S. Wang and Y. Zou, Integrated catalytic sites for highly efficient electrochemical oxidation of the aldehyde and hydroxyl groups in 5-hydroxymethylfurfural, ACS Catal., 2022, 12, 4242–4251 CrossRef CAS.
- K. Yang, N. Zhang, J. Yang, Z. Xu, J. Yan, D. Li and S. Liu, Synergistic marriage of CO2 reduction and sulfide oxidation towards a sustainable co-electrolysis process, Appl. Catal., B, 2023, 332, 122718 CrossRef CAS.
- J. C. Fornaciari, D. Primc, K. Kawashima, B. R. Wygant, S. Verma, L. Spanu, C. B. Mullins, A. T. Bell and A. Z. Weber, A perspective on the electrochemical oxidation of methane to methanol in membrane electrode assemblies, ACS Energy Lett., 2020, 5, 2954–2963 CrossRef CAS.
- E. Sargeant, A. Kolodziej, C. S. Le Duff and P. Rodriguez, Electrochemical conversion of CO2 and CH4 at subzero temperatures, ACS Catal., 2020, 10, 7464–7474 CrossRef CAS.
- J. Lu, C. Zhu, C. Pan, W. Lin, J. P. Lemmon, F. Chen, C. Li and K. Xie, Highly efficient electrochemical reforming of CH4/CO2 in a solid oxide electrolyser, Sci. Adv., 2018, 4, eaar5110 Search PubMed.
- T. Tan, W. Wang, K. Zhang, Z. Zhan, W. Deng, Q. Zhang and Y. Wang, Upcycling plastic wastes into value-added products by heterogeneous catalysis, ChemSusChem, 2022, 15, e202200522 CrossRef CAS PubMed.
- X. Du, H. Zhang, K. P. Sullivan, P. Gogoi and Y. Deng, Electrochemical lignin conversion, ChemSusChem, 2020, 13, 4318–4343 CrossRef CAS PubMed.
- H. Zhao, D. Lu, J. Wang, W. Tu, D. Wu, S. W. Koh, P. Gao, Z. J. Xu, S. Deng, Y. Zhou, B. You and H. Li, Raw biomass electroreforming coupled to green hydrogen generation, Nat. Commun., 2021, 12, 2008 CrossRef CAS PubMed.
- X. Du, W. Liu, Z. Zhang, A. Mulyadi, A. Brittain, J. Gong and Y. Deng, Low-energy catalytic electrolysis for simultaneous hydrogen evolution and lignin depolymerization, ChemSusChem, 2017, 10, 847–854 CrossRef CAS PubMed.
- F.-Y. Chen, Z.-Y. Wu, S. Gupta, D. J. Rivera, S. V. Lambeets, S. Pecaut, J. Y. T. Kim, P. Zhu, Y. Z. Finfrock, D. M. Meira, G. King, G. Gao, W. Xu, D. A. Cullen, H. Zhou, Y. Han, D. E. Perea, C. L. Muhich and H. Wang, Efficient conversion of low-concentration nitrate sources into ammonia on a Ru-dispersed Cu nanowire electrocatalyst, Nat. Nanotechnol., 2022, 17, 759–767 CrossRef CAS.
- G. Lee, Y. C. Li, J.-Y. Kim, T. Peng, D.-H. Nam, A. Sedighian Rasouli, F. Li, M. Luo, A. H. Ip, Y.-C. Joo and E. H. Sargent, Electrochemical upgrade of CO2 from amine capture solution, Nat. Energy, 2021, 6, 46–53 CrossRef CAS.
- I. Sullivan, A. Goryachev, I. A. Digdaya, X. Li, H. A. Atwater, D. A. Vermaas and C. Xiang, Coupling electrochemical CO2 conversion with CO2 capture, Nat. Catal., 2021, 4, 952–958 CrossRef CAS.
- D. M. Weekes, D. A. Salvatore, A. Reyes, A. Huang and C. P. Berlinguette, Electrolytic CO2 reduction in a flow cell, Acc. Chem. Res., 2018, 51, 910–918 CrossRef CAS PubMed.
- S. Liang, N. Altaf, L. Huang, Y. Gao and Q. Wang, Electrolytic cell design for electrochemical CO2 reduction, J. CO2 Util., 2020, 35, 90–105 CrossRef CAS.
- J. Li, G. Chen, Y. Zhu, Z. Liang, A. Pei, C.-L. Wu, H. Wang, H. R. Lee, K. Liu, S. Chu and Y. Cui, Efficient electrocatalytic CO2 reduction on a three-phase interface, Nat. Catal., 2018, 1, 592–600 CrossRef CAS.
- T.-T. Zhuang, Z.-Q. Liang, A. Seifitokaldani, Y. Li, P. De Luna, T. Burdyny, F. Che, F. Meng, Y. Min, R. Quintero-Bermudez, C. T. Dinh, Y. Pang, M. Zhong, B. Zhang, J. Li, P.-N. Chen, X.-L. Zheng, H. Liang, W.-N. Ge, B.-J. Ye, D. Sinton, S.-H. Yu and E. H. Sargent, Steering post-C–C coupling selectivity enables high efficiency electroreduction of carbon dioxide to multi-carbon alcohols, Nat. Catal., 2018, 1, 421–428 CrossRef CAS.
- C.-T. Dinh, T. Burdyny, M. G. Kibria, A. Seifitokaldani, C. M. Gabardo, F. P. García de Arquer, A. Kiani, J. P. Edwards, P. De Luna, O. S. Bushuyev, C. Zou, R. Quintero-Bermudez, Y. Pang, D. Sinton and E. H. Sargent, CO2 electroreduction to ethylene via hydroxide-mediated copper catalysis at an abrupt interface, Science, 2018, 360, 783–787 CrossRef CAS.
- A. Ozden, F. P. García de Arquer, J. E. Huang, J. Wicks, J. Sisler, R. K. Miao, C. P. O’Brien, G. Lee, X. Wang, A. H. Ip, E. H. Sargent and D. Sinton, Carbon-efficient carbon dioxide electrolysers, Nat. Sustainable, 2022, 5, 563–573 CrossRef.
- T. Zheng, K. Jiang, N. Ta, Y. Hu, J. Zeng, J. Liu and H. Wang, Large-scale and highly selective CO2 electrocatalytic reduction on nickel single-atom catalyst, Joule, 2019, 3, 265–278 CrossRef CAS.
- P. Wei, D. Gao, T. Liu, H. Li, J. Sang, C. Wang, R. Cai, G. Wang and X. Bao, Coverage-driven selectivity switch from ethylene to acetate in high-rate CO2/CO electrolysis, Nat. Nanotechnol., 2023, 18, 299–306 CrossRef CAS PubMed.
- M. C. O. Monteiro, M. F. Philips, K. J. P. Schouten and M. T. M. Koper, Efficiency and selectivity of CO2 reduction to CO on gold gas diffusion electrodes in acidic media, Nat. Commun., 2021, 12, 4943 CrossRef CAS PubMed.
- Y. Xie, P. Ou, X. Wang, Z. Xu, Y. C. Li, Z. Wang, J. E. Huang, J. Wicks, C. McCallum, N. Wang, Y. Wang, T. Chen, B. T. W. Lo, D. Sinton, J. C. Yu, Y. Wang and E. H. Sargent, High carbon utilization in CO2 reduction to multi-carbon products in acidic media, Nat. Catal., 2022, 5, 564–570 CrossRef CAS.
- J. Gu, S. Liu, W. Ni, W. Ren, S. Haussener and X. Hu, Modulating electric field distribution by alkali cations for CO2 electroreduction in strongly acidic medium, Nat. Catal., 2022, 5, 268–276 CrossRef CAS.
- J. E. Huang, F. Li, A. Ozden, A. Sedighian Rasouli, F. P. García de Arquer, S. Liu, S. Zhang, M. Luo, X. Wang, Y. Lum, Y. Xu, K. Bertens, R. K. Miao, C.-T. Dinh, D. Sinton and E. H. Sargent, CO2 electrolysis to multicarbon products in strong acid, Science, 2021, 372, 1074–1078 CrossRef CAS PubMed.
- Y. Zhao, L. Hao, A. Ozden, S. Liu, R. K. Miao, P. Ou, T. Alkayyali, S. Zhang, J. Ning, Y. Liang, Y. Xu, M. Fan, Y. Chen, J. E. Huang, K. Xie, J. Zhang, C. P. O’Brien, F. Li, E. H. Sargent and D. Sinton, Conversion of CO2 to multicarbon products in strong acid by controlling the catalyst microenvironment, Nat. Synth., 2023, 2, 403–412 CrossRef.
- T. Iwasita, Electrocatalysis of methanol oxidation, Electrochim. Acta, 2002, 47, 3663–3674 CrossRef CAS.
- Y. Li, X. Wei, S. Han, L. Chen and J. Shi, MnO2 electrocatalysts coordinating alcohol oxidation for ultra-durable hydrogen and chemical productions in acidic solutions, Angew. Chem., Int. Ed., 2021, 60, 21464–21472 CrossRef CAS.
- L. Li, Y. Sun and Y. Xie, Catalysts design for CO2 electroreduction, Sci. China: Chem., 2022, 65, 425–427 CrossRef CAS.
- Q. Liu, X.-G. Zhang, Z.-Y. Du, C.-J. Zou, H.-Y. Chen, Y. Zhao, J.-C. Dong, P.-P. Fang and J.-F. Li, Converting CO2 to ethanol on Ag nanowires with high selectivity investigated by operando Raman spectroscopy, Sci. China: Chem., 2023, 66, 259–265 CrossRef CAS.
- X. Song, L. Xu, X. Sun and B. Han, In situ/operando characterization techniques for electrochemical CO2 reduction, Sci. China Chem., 2023, 66, 315–323 CrossRef CAS.
|
This journal is © the Partner Organisations 2024 |