DOI:
10.1039/D3NR06522G
(Minireview)
Nanoscale, 2024,
16, 10533-10550
Recent advances in atomic cluster synthesis: a perspective from chemical elements
Received
21st December 2023
, Accepted 5th April 2024
First published on 11th April 2024
Abstract
Despite its potential significance, “cluster chemistry” remains a somewhat marginalized topic within the chemistry field. However, atomic clusters with their unusual and unique structures and properties represent a novel material group situated between molecules and nanoparticles or solid matter, judging from both scientific standpoints and historical backgrounds. Surveying an entire material group, including all substances that can be regarded as a cluster, is essential for establishing cluster chemistry as a more prominent chemistry field. This review aims to provide a comprehensive understanding by categorizing, summarizing, and reviewing clusters, focusing on their constituent elements in the periodic table. However, because numerous disparate synthetic processes have been individually developed to date, their straightforward and uniform classification is a challenging task. As such, comprehensively reviewing this field from a chemical composition viewpoint presents significant obstacles. It should be therefore noted that despite adopting a synthetic method-based classification in this review, the discussions presented herein could entail inaccuracies. Nevertheless, this unorthodox viewpoint unfolds a new scientific perspective which accentuates the common ground between different development processes by emphasizing the lack of a definitive border between their synthetic methods and material groups, thus opening new avenues for cementing cluster chemistry as an attractive chemistry field.
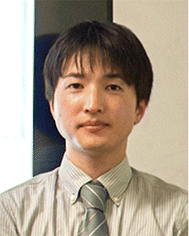 Takamasa Tsukamoto | Takamasa Tsukamoto received his PhD degree in Eng. from Tokyo Metropolitan University in 2015. After being a research fellow of the Japan Society for the Promotion of Science (2014–2016), a visiting researcher at the University of Miami (2014), a doctoral research fellow at the University of Tokyo (2015–2016), and a postdoctoral researcher and an assistant professor at the Tokyo Institute of Technology (2016–2023), he joined the Institute of Industrial Science at the University of Tokyo as a lecturer (2023) and the Japan Science and Technology Agency as a PRESTO researcher (2020–2023). His current research interests include developing organic–inorganic hybrid compounds for cluster synthesis, theoretical studies on clusters involving superatoms, the interpretation of the super-degeneracy phenomenon, and proposing a higher-order periodic table of superatoms. |
1. Introduction
Atomic clusters comprising a few, a few dozen, or hundred atoms, with particle diameters of typically ∼1 nm and reaching up to ∼3 nm fall under an ultrasmall particulate material group (Fig. 1).
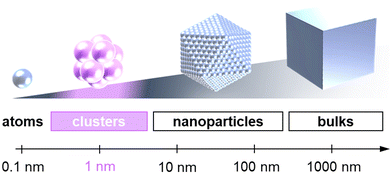 |
| Fig. 1 Classification of materials based on the size scale. | |
Compared to conventional nanoparticles (5–500 nm), their atypical smallness exhibits certain eccentric properties not found in nanoparticles, originating from the remarkable quantum size effect. Regarding general nanoparticles, physical properties and chemical reactivities are determined by their surface structures and geometrical shapes.1–3 On the other hand, their electronic states of clusters resemble more closely those of molecules than bulk materials (including nanoparticles), leading to their unique characteristics.4–8 Such clusters contribute to the manifestation of highly efficient and sophisticated biological functions in nature, such as water oxidation in photosynthesis, electron transfer, nitrogen fixation, and breathing.9–13 Moreover, clusters possess various structural characteristics that are not observed in other chemicals, including the number of constituent atoms, types of constituent elements, elemental ratios, atomic arrangements, and geometrical symmetry. Such a feature affords a great degree of freedom in terms of material design. Furthermore, their steric and electronic structures on a molecular scale offer significant potential for achieving novel properties and functions. Therefore, this sub-nanometer material group has attracted worldwide attention as a candidate for next-generation post-nanotechnology materials.
However, the desired progress in the cluster chemistry field has been hindered by the inherent challenges entailed in synthesizing clusters, as compared to nanoparticles. This issue originates from the critical factors differentiating the properties of nanoparticles and clusters. Particularly, in contrast to nanoparticles, the structures, properties, and stabilities of clusters depend significantly on the number of constituent atoms. Since precise atom manipulation techniques (within 1 nm of the space in cluster particles) are necessary for the synthesis of clusters, applying conventional synthetic methods lacking atomic-level precision is unsuitable for forming such sub-nanosized materials. Therefore, developing new clustering techniques that are independent of these methods is essential. Atomic-level synthesis is significantly influenced by the nature of the constituent elements; however, in many cases, the types of elements that can be treated using a single method tend to be limited. By considering this aspect, synthetic methods dedicated to certain elements or elemental groups have been established, with the regions of the corresponding elements distributed on the periodic table like islands (Fig. 2). Notable developments in cluster chemistry have been achieved using these methods as starting points.
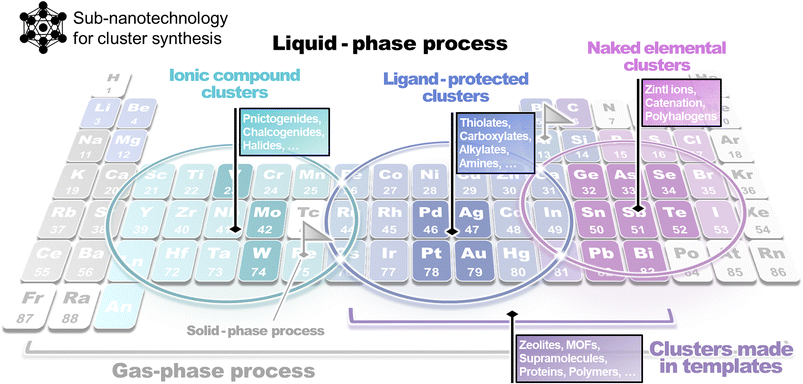 |
| Fig. 2 A rough sketch of a map for the classification of suitable methods for atomic cluster synthesis depending on the chemical element composition. | |
As noted above, synthetic methods for clusters, unlike those for nanoparticles, tend to be inflexible in terms of element selectivity and applicability, despite their high efficacy for a certain region in the periodic table. This limitation has hindered cross-cutting discussions focusing on constituent elements in the topic of cluster synthesis. In this review, with the aim of providing a comprehensive discussion and understanding of the entire field of cluster synthesis, we categorize, summarize, and review these methods by focusing on the constituent elements of clusters in the periodic table.
2. Methods for cluster synthesis
Metal carbonyl clusters composed of single or multiple transition metal elements and carbonyl ligands represent one of the most well-established material groups among chemical clusters. They possess the most fundamental structures in coordination chemistry and serve as precursors in various chemistry fields, typified by organometallic chemistry.14 These clusters are synthesized by common chemical reactions and their reaction formulas are relatively simple. Moreover, borane clusters are also well synthesized mainly by the pyrolysis of diborane, and their atomicity and shape are classified as obeying the Wade–Mingos rule15,16 or the Jemmis mno rule.17
However, in such cases, the degrees of freedom of design and synthesis are reduced and limited from the perspective of expanding the applicable elements. For example, in thermodynamically stable clusters obtained as metal carbonyls, the number of metal atoms, carbonyl ligands, and structures (hapticity) are uniquely determined by the electronic state of the transition metals. Therefore, the introduction of new factors that can be actively added or modified into the synthesis process of clusters is key for obtaining clusters composed of the desired number of atoms and element types. Based on this concept, various synthetic approaches using gas-, liquid-, and solid-phase reactions have been investigated.
3. Gas-phase synthesis
Fullerenes stand as the most well-known clusters synthesized in the gas-phase.18–20 In an arc reactor or through laser vaporization in vacuum, fullerenes, typified by commercially available C60, C70, and C84 are obtained from carbon sources as a mixture and subsequently separated and purified by chromatography.
Endohedral fullerenes in which other elements are constrained in their cages, such as [Li@C60]+, can also be prepared using carbon mixed with metal sources.21,22
Although gas-phase synthesis is also effective in the case of heavier metal elements,23 metal clusters tend to easily undergo aggregation and particle enlargement after production, in contrast to lighter elements. Therefore, techniques for separating clusters from mixtures directly after synthesis are crucial. In particular, gas-phase reaction systems combined with mass-separation units are often employed, leading to numerous reports on metal clusters typified by Nax.24 This method not only effectively yields single elemental clusters such as superatomic [Al13]−,25 stannaspherene [Sn12]2−,26 borospherene [B40]−,27 and tetrahedral Au20,28 but also heteroelemental clusters such as M@Sn1226 and M@Si16.29 On the other hand, stable extraction of clusters produced from the gas phase has recently been investigated, including the soft-landing method, in which clusters are deposited intact on a self-assembled monolayer (SAM) of alkyl groups formed on a substrate.30
As mentioned above, gas-phase preparation techniques offer the merit of involving the use of many elements, including both metal and nonmetal elements, but are fundamentally not conducive to mass production. Therefore, although these methods are significantly effective for analyzing physical properties, including electronic states, they are not suitable for investigations requiring large amounts of clusters, such as chemical reactivity. Additionally, because it is necessary to provide a positive or negative charge to clusters for mass separation, the properties of charged clusters are preferentially observed. The latter can be addressed by establishing a neutralization method for obtaining neutral clusters after mass separation.31
4. Liquid-phase synthesis
Various liquid-phase synthesis methods have been explored due to their practicality. The principal approaches for stabilizing clusters involve methods adopting ligand protection, employing polyoxometalate anions, and applying the Zintl phase. Each method targets specific regions of elements in the periodic table, leading to differences in the properties of the obtained clusters. These disparities often arise from variations in the chemical states of the clusters’ surface or interior. Therefore, given the present circumstances, a suitable synthetic method should be carefully selected that not only considers the employed elements, but that can also induce the desired properties.
4.1. Clusters stabilized by ligand protection
In this method, the clusters are chemically stabilized by the steric and electronic protection effect of the organic ligands, which cover their surface atoms with functional groups. Applicable metal elements vary depending on the functional groups of the ligands. Negatively charged alkylthiolate-type (SR−) ligands typically used for group 11 element clusters (Au, Ag, Cu) are among the most well-investigated representatives of this method.32–36 Alkyl selenide-type analog structures (SeR−) have also been reported.37 The steric structure of ligands modifies the number of constituent atoms and their geometric arrangements, which are often based on highly symmetric core structures.38 In this regard, Au102(SR)44,39 Au144(SR)60,40,41 [Ag180(SR)90(CH3SO3)44]46+,42 and Ag374(SR)113Br2Cl243 stand as the largest well-known clusters with numerous constituent atoms (Fig. 3A). Recent reports have revealed that this method is also applicable to the synthesis of alloy clusters composed of group 11 elements, such as [Au12Ag32(SR)30]4− (ref. 44) (Fig. 3B), despite the composition and arrangement of multiple elements in these clusters tending to be uncontrollable because of the preferential generation of energetically stable clusters. Additionally, an alloying method for doping with other metal elements has been developed by utilizing the stability of ligand-protected Au or Ag clusters.45 This approach enables the use of metals that do not achieve ligand stabilization, such as [M@Au25(SR)18]− (M = Pd, Pt, Cd, Hg)46 and Pd@Ag20(S2PR2)12
47 (S2P(OR)2− = dialkoxyldithiophosphinate). A recently reported anion-templated synthesis method of such clusters (especially for Ag clusters) also provides various geometric structures containing non-metallic elements or anionic species (atomic anion: H−, D−, F−, Cl−, Br−, I−, S2−, Se2−, and Te2−; oxoanion: CO32−, C2O42−, C4O42−, C5O52−, NO3−, AsO43−, SO32−, SO42−, SeO32−, SeO42−, TeO32−, TeO66−, ClO4−, VO43−, CrO42−, MoO42−, and WO42−; polyoxometalate anion: [V10O28]6−, [Mo6O22]8−, [EuW10O36]9−, etc.) in the center of the structure,48 such as [X@Ag8(S2P(OR)2)6]+/0 (X = F, Cl, Br, S).49,50 Other elements in group 11 have also been investigated, such as [Cl@Cu14(S(CH3)2(CH2NH2))12]7+.51 Thiolate-type ligands have also been used to stabilize metal sulfide clusters. For example, group 10 elements (Ni, Pd, Pt) form tiara-shaped cluster complexes, such as [M(SR)2]n (M = Ni, Pd, Pt)52–54 (Fig. 3C), that often encapsulate guest metal ions or molecules in their ring centers (I2, Ag+).55,56 In the case of other group elements typified by Mn, Co, Zn, and Cd, a combination of monoanionic SR− and dianionic S2− provides sulfide clusters including tetrahedral [Mn8Se(SePh)16]2−,57 octahedral [Co8S6(SPh)8]4−,58 tetrahedral [Zn10S4(SPh)16]4−,59 and [Cd54S32(SPh)48(DMF)4]4− (ref. 60) (Fig. 3D).
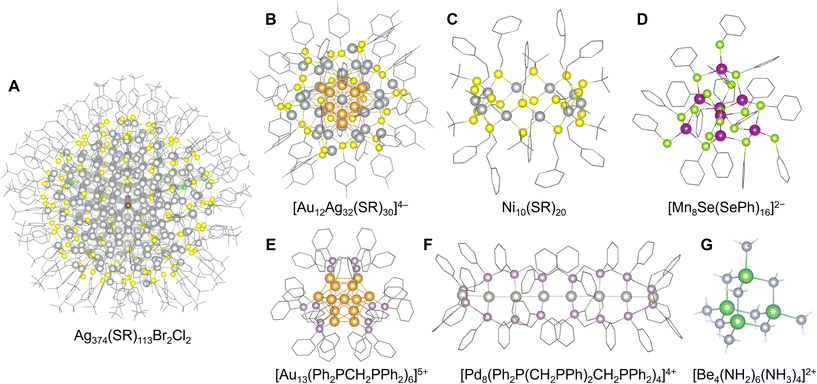 |
| Fig. 3 Crystal structures of: (A) Ag374(SR)113Br2Cl2 (R = 4-tert-butylphenyl),43 Ag: gray, S: yellow, Cl: green, Br: brown, and C: grayish bone; (B) [Au12Ag32(SR)30]4− (R = 4-fluorophenyl),44 Au: orange-yellow, Ag: gray, S: yellow, C: gray bone, and F: pale blue bone; (C) Ni10(SR)20 (R = 2-pyridylethyl),52 Ni: gray, S: yellow, C: grayish bone, and N: pale blue bone; (D) [Mn8Se(SePh)16]2−,56 Mn: violet, Se: yellow-green, C: gray bone; (E) [Au13(Ph2PCH2PPh2)6]5+,66 Au: orange-yellow, P: grayish pink, and C: grayish bone; (F) [Pd8(Ph2P(CH2PPh)2CH2PPh2)4]4+,70 Pt: gray, P: grayish pink, C: grayish bone; and (G) [Be4(NH2)6(NH3)4]2+,75 Be: green, N: pale blue, and H: white. Figures are reproduced from (A) CCDC 1496141, (B) CCDC 953881, (C) CCDC 743607, (D) CCDC 612696, (E) CCDC 1577669, (F) CCDC 1023572, and (G) CCDC 1982295. | |
Among other functional groups used for ligand protection, various neutral, cationic, and anionic ligands have been investigated. Neutral ligands typically include monodentate or multidentate organophosphines (PR3), amines (NR3), and imines (NR(
R)). Analogs with heavier atoms, such as organostibines (SbR3), have recently been reported.61 In the case of phosphines, clusters with group 11 elements, especially gold62–65 (sometimes group 10 elements, such as Ni3(PPh)(PPh2)2(PPh3)366), typified by commercially available [Au3O(PPh3)3]+ and icosahedral [Au13(Ph2PCH2PPh2)6]5+ (ref. 67) are principally explored in the same manner as alkylthiolate (Fig. 3E). In particular, precisely designed multidentate phosphine ligands provide and control unique arrangements of metal atoms in a cluster, such as icosahedron-based [Au20(P(CH2CH2PPh2)3)]4+,68 linear [Au4(Ph2P(CH2PPh)2CH2PPh2)2]4+ (ref. 69) and linear [Pd8(Ph2P(CH2PPh)2CH2PPh2)4]4+ with tetraphosphines70 (Fig. 3F). Anion-templated synthesis is also used for clusters with phosphine ligands such as Cl@Ag12@Ag48(Ph2PCH2PPh2)12.71 Another example is transition-metal chalcogenide clusters with protective ligands, such as Co6Te8(PR3)6.72 The clusters were stabilized by amine or imine ligands: cubic Cu4I4(C5H5N)4 with pyridine ligands,73 rhombic [In4(C10H8N2)6]4+ with bipyridyl ligands,74 and [Be4(NH2)6(NH3)4]2+ with ammonia ligands75 (Fig. 3G).
Anionic ligands, including carboxylate (OCOR−), represent a well-known example, especially for clusters with low atomicity. Examples include dinuclear clusters such as M2(OCOR)4(L)2 (where M = Cr, Mo, Rh, Cu, Bi)76 and trinuclear clusters such as [M3O(OCOR)6(L)3]+ (where M = V, Cr, Fe, Ru, Co, Rh, Ir).77,78 Notably, such clusters are often commercially available. For the formation of these complex clusters, the oxidation number of the transition metal ions is an important factor; for example, dinuclear and trinuclear carboxylate clusters require metal ions with stable valences of +II and +III, respectively. In this regard, square-planar V4(OCOCH3)4(OH)4(H2O)8,77 tetrahedral Zn4O(OCOCH3)6, Zn10O4(OCOCH3)1279 and In37P20(OCOCH2Ph)51
80 have been successfully reported. Alkoxides (OR−), amides (NR2−), and methides (CR3−) have also been used as simple protecting ligands. In particular, research has primarily focused on group 13 and 14 elements (Al, Ga, In, and Sn).81 Examples include [Al77(N(SiMe3)2)20]2− (ref. 82) and Sn15(NR2)6,83 while transition metal elements with structures resembling oxides are often crucial for alkoxides, as seen in compounds like W4(OR)16.84
Organometallic approaches are also effective in stabilizing clusters, with the formation of tetrahedral Li4R4 or octahedral Li6R6 clusters standing as a typical example.85 In particular, cyclopentadienyl-type or pentamethylcyclopentadienyl-type ligands (C5H5− or C5Me5−) have been extensively investigated for the stabilization of clusters composed of various elements. Typically, clusters of group 11, 12, and 13 elements (Cu, Zn, Al, Ga, and In) tend to have structures with large atomicity compared to transition metal elements (Co, Rh, Ir, etc.),86 such as trigonal-bipyramidal Zn9(C5Me5)6
87 and tetrahedral Al4(C5Me5)4
88 (Fig. 4A). Alloy clusters have also been obtained using this method, such as trigonal-bipyramidal Cu3Zn4(C5Me5)5,89 with icosahedral Cu43Al12(C5Me5)12 being one of the largest clusters reported to date90 (Fig. 4B and C). Moreover, some transition metal halides, chalcogenides, and pnictogenides are also stabilized by cyclopentadienyl ligands like pseudo-tridecahedral La9I18(C5Me5)9,91 trigonal-bipyramidal Rh3Se2(C5EtMe4)9,92 and tetrahedral Cr4P4(C5Me5)4
93 or Mn4P4(C5H5)4
94 (Fig. 4D and E). Using this synthetic approach, model clusters mimicking the biochemical functions associated with nitrogen fixation were recently created (e.g., FeMo3S4(C5H4SiR3)3).95 Additionally, the aromatic rings with large π-conjugated systems also serve as a planar protection ligand and often provide sophisticated highly-symmetric clusters such as cuboctahedral [Pd13(C7H7)6]2+ stabilized by cycloheptatrienylium (C7H7+)96 and square-planar [Pd4(C8H8)(C9H9)]+ stabilized by cyclooctatetraene (C8H8) and cyclononatetraenyl (C9H9−)97 (Fig. 5A). Conversely, bridged aromatic ligands can effectively stabilize group 11 elements (Au, Ag, and Cu), yielding star-shaped clusters typified by pentagonal planar Au5(C6H3Me3)5, Cu5(C6H3Me3)5, and square-planar Ag4(C6H3Me3)4 with mesityl anions (C6H2Me3−)98,99 (Fig. 5B). Additionally, tetramesityl diiron Fe2(C6H3Me3)4 has also been reported, albeit not among group 11 elements.100 Recently, by utilizing such bridged aromatic ligands, other atomic arrangements including alloys are obtained, like octahedral [Au4Ag2(C6H4PR2)4]2+.101 In contrast, aromatic ligands with bulky substituents also stabilize unique cluster structures by the steric protection effect, such as adamantane-shaped Al4(PH)6(2,6-C6H4(C6Me3H2)2)4.102
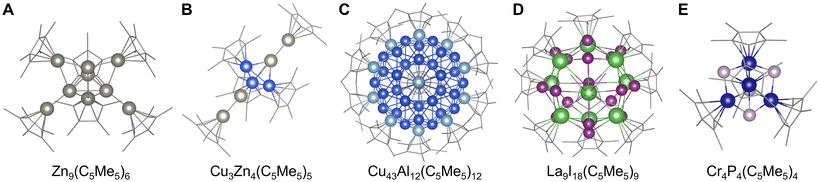 |
| Fig. 4 Crystal structures of (A) Zn9(C5Me5)6.87 (B) Cu3Zn4(C5Me5)5,89 (C) Cu43Al12(C5Me5)12,90 (D) La9I18(C5Me5)9,91 and (E) Cr4P4(C5Me5)4.93 Zn: gray, Cu: blue, Al: light blue, La: green, I: violet, Cr: dark blue, P: grayish pink, and C: grayish bone. Figures are reproduced from (A) CCDC 1434844, (B) CCDC 1854852, (C) CCDC 1845365, (D) CCDC 1992039, and (E) CCDC 984791. | |
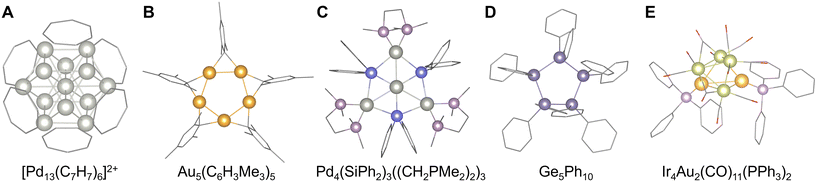 |
| Fig. 5 Crystal structures of (A) [Pd13(C7H7)6]2+,96 (B) Au5(C6H3Me3)5,98 (C) Pd4(SiPh2)3((CH2PMe2)2)3,103 (D) Ge5Ph10,109 and (E) Ir4Au2(CO)11(PPh3)2.118 Pd: gray, Au: orange-yellow, Si: blue-violet, P: grayish pink, Ge: grayish blue-violet, Ir: light green-yellow, C: gray bone, and O: red bone. Figures are reproduced from (A) CCDC 1813458, (B) CCDC 1119882, (C) CCDC 702935, (D) CCDC 1137518, and (E) CCDC 924993. | |
Other organometallic approaches for controlling the number and arrangement of metal atoms in clusters have also been developed. For example, organosilicon or organogermanium ligands containing Si and Ge as metalloid elements yield group 10 metal clusters with unusual geometric structures, such as hexagonal planar Pd4(SiPh2)3((CH2PH2)2)3 and hexagonal-bipyramidal Pd6(GePh2)2(CNC6H3Me2)10
103–106 (Fig. 5C). Otherwise, the organometallic ligands form clusters, such as cyclic (GeMes2)3, (GePh2)4, and (GePh2)5 with Ge–Ge bonds107–109 (Fig. 5D). Organic or organosilicon ligands have also been employed to synthesize clusters comprising nonmetallic C and semimetallic Si. For example, employing a low-temperature photochemical process or a pure organometallic process in a solvent can yield tetrahedral structures such as C4(CR3)4
110 and Si4(SiR3)4.111
In other cases, methods for achieving both the stabilization and alloying of metal clusters using transition metal carbonyl complexes as ligands have been reported, such as octahedral [Ag13Fe8(CO)32]4− (ref. 112) and three pentagonal-antiprismatic [Sb3Rh20(CO)36]3− (ref. 113) complexes. It is worth mentioning that as a technique for clustering certain metals or mixing different metal elements in a cluster, the method using multiple ligands with different functional groups has been investigated, such as Fe8(N[o-H2NC6H4NH(CH2)2]3)2(PMe2Ph)2 with tetraamine and phosphine ligands,114 Ga6(C6Me3H2)4(C3N2Me2Pr2)2 with mesityl and tetraalkylimidazol-2-ylidene ligands,115 [Mg16(C5Me5)8(NEt3)2Br4]2− with cyclopentadienyl and amine ligands,116 Cu10Zn2(C6Me3H2)6(C5Me5)2 with mesityl and cyclopentadienyl ligands,117 Ir4Au2(CO)11(PPh3)2,118 Pt13Au4(CO)10(PPh3)8,119 and Pd145(CO)x(PEt3)30 (x ≈ 60)120 with carbonyl and phosphine ligands (Fig. 5E), and co-crystalized [(AuAg)267(SPhMe2)80]·[(AuAg)45(SPhMe2)27(PPh3)6] with thiolate and phosphine ligands.121 This approach has recently led to the development of model clusters for photosynthetic systems (Mn3Ca clusters with carboxylate and pyridine ligands).122
By surveying ligand protection methods, we can broadly summarize the tendencies of the applicable elements for each method. The most extensively studied elements in ligand protection methods are noble metals (Pd, Pt, Au, and Ag). Following closely are other late transition metal elements (Fe, Co, Rh, Ni, Cu) and post-transition elements (Zn, Cd, Al, Ga, and In). Remarkably, the bond type between the metal atoms directly influences the diversity of the cluster structures. The fact that the most varied cluster structures were found for the metal elements in group 11 could be rationalized by their valence s-electrons forming relatively free bonds and resistance to oxidation. In contrast, other elements tend to be in charged states with the ligands. Although there are relatively few reports on minor elements, a few atoms are often implemented in a cluster by doping the surrounding environment where the stabilization effect of cluster structures composed of major elements is dominant. The types of elements stabilized as a cluster significantly differ by and depend on the functional groups of the organic ligands, which are frequently observed in the case of clusters with single-bond-mediated ligand protection. However, only cyclopentadienyl ligands differ from these ligands and are utilized for applying a broad range of elements by stabilizing clusters with both electrostatic and steric effects. Therefore, such an approach, without obvious single-bond-mediated ligand protection, is expected to be effective for developing versatile synthetic methods from the viewpoint of applicable elements.
Although simple functional groups such as thiolates, carboxylates, and phosphines are used in conventional methods, suitable ligands for this approach are being explored and developed. In particular, new ligands with more complicated structures and compositions containing multidentate ligands afford unique stability to cluster structures.123 Such a method to stabilize a certain geometric structure using custom-designed multidentate ligands is similar to the approach for controlling crystal polymorphs of nanoparticles, in which modification of the surface of a nanoparticle by multidentate ligands induces unusual crystal structures in the whole nanoparticle.124 These reports also indicated that an approach that induces multipoint interactions between a cluster and its ligands is effective for developing versatile synthetic methods.
4.2. Clusters stabilized as ionic compounds
Clusters solely stabilized without protecting ligands have also been reported. They are generally obtained as ionic compounds that undergo electronic stabilization by bonding with pnictogens, chalcogens, halides, and metals with lower electronegativity. Polyoxometalate (POM) clusters are well-known materials. These clusters are almost negatively charged metal oxides and tend to be composed of metal atoms with high oxidation numbers.125–127 In many cases, group 5 and 6 elements (V, Nb, Ta, Mo, and W) in the +V or +VI state prefer a six-coordination state. Some species such as paramolybdate [Mo7O24]6−, decatungstate [W10O32]4−, metatungstate [W12O40]8−, paratungstate [W12O42]12−, molybdenum blue reagents [Mo154O462H14(H2O)70]14− and [Mo152O457H14(H2O)68]16− are commercially available.128 [HxMo368O1032(H2O)240(SO4)48]48− was one of the largest POM clusters129 (Fig. 6A). Similarly, group 3 superheavy elements (U, Np, Pu, and partially Am) in the +V or +VI state also form POM structures, such as [U60O240(OH)60]60− with fullerene topologies130–132 (Fig. 6B). The atomicity and arrangement of POM clusters can often be tuned by introducing other elements as the central core. For example, 4-coordinating species mainly in group 13, 14, and 15 elements (B, Al, Ga, C, Si, Ge, P, and As), such as PO4−, SiO42−, and AlO43− species, form unique structures typified by tungstophosphate [PMo12O40]3− called Keggin-type structures.125,126 Fe, Co, Cu, and Zn in the +II or +III state, and S and Se in the +VI state have also been reported as tetrahedral centers. In the case of 6-coordinating species of elements (Cr, Mn, Fe, Co, Rh, Ir, Ni, Pd, Pt, Cu, Zn, Al, Ga, Sb, Te, I), more planar Anderson–Evans-type POM clusters are constructed by a central EO6 unit that is often in highly oxidized states, such as [CoMo6O18(OH)6]6− with Co(+III), [PtW6O24]8− with Pt(+IV), and [IMo6O24]8− with I(+VII).124,133 Moreover, icosahedrally 12-coordinating EO12 central units containing group 3 and 4 heavy elements (Ce, Th, U, Np, and Zr in the +IV or +V state) provide unique Dexter–Silverton-type structures such as pyritohedral [CeMo12O42]8−.134 By introducing two or more core units, POMs lead to the construction of more complicated structures called Wells–Dawson-type or Preyssler–Pope–Jeannin-type structures.125 Additionally, multiple POMs form sandwich-type or capsule-type structures intercalating group 1, 2, and 3 elements and low valent transition metals as a cation,125,135 and they are also found in natural minerals, typified by the [Zn2Mn2(H2O)2(FeW9O34)2]12− cluster in ophirite.136 By applying this unique property that partially incorporates other elements in or between POMs, it is possible to synthesize rarer, larger and more complicated structures, such as tetrapod-shaped [Ti4Cl(OH)12(P2W15Ti3O62)4]45− where Ti atoms are sandwiched by four POM cluster units,137 wheel-shaped [Cu20Cl(OH)24(H2O)12(P8W48O184)]25− where Cu atoms are encapsulated in a circular POM cluster host,138 and a tetrahedral layered superstructure of [La10Ni48W140Sb16P12O568(OH)24(H2O)20]86−.139 Recently, it was reported that large capsule-like POM clusters can also incorporate other small metal clusters into their centers, such as [Ag27@(Si2W18O66)3]31−.140
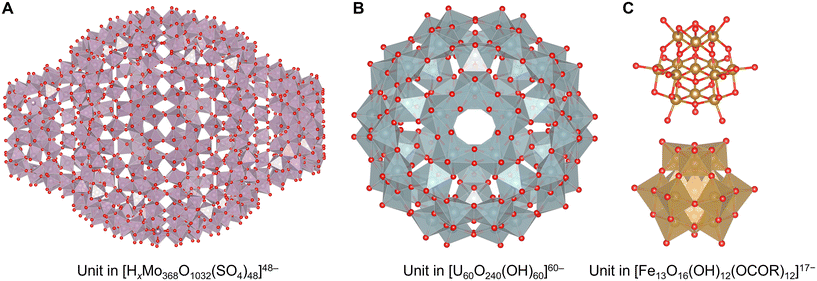 |
| Fig. 6 Crystal structures of (A) a unit in [HxMo368O1032(H2O)240(SO4)48]48− with a structure showing molybdenum oxide polyhedra,129 (B) a unit in [U60O240(OH)60]60− with a structure showing uranium oxide polyhedra,130 and (C) a unit in [Fe13O16(OH)12(OCOR)12]17− with a structure showing iron oxide polyhedra (below).142 Mo: reddish gray polyhedron, O: red, U: bluish gray polyhedron, Fe: brown, and O: red. Figures are reproduced from (A) CCDC 1727624, (B) CCDC 1732644, and (C) CCDC 1525080. | |
With the exception of V, Mo, and W, advanced synthesis techniques have been developed. In particular, the polycationic Keggin-type structures of aluminum [Al30O8(OH)56(H2O)24]18+ containing 6-coordinating AlO6 sites and central 4-coordinating AlO4 sites are well known.141 However, in such cases, co-adoption of the concept of ligand protection in these clusters is also effective in synthesizing POMs composed of minor elements, such as Keggin-type [Fe13O16(OH)12(OCOR)12]17− containing an Fe(+II) center and Fe(+III) shells with carboxylate ligands142 (Fig. 6C), Keggin-type [Mn13O6(OH)2(OMe)4L6]4+ containing a Mn(+III) center and Mn(+IV) shells with alkoxylate and imine ligands (L = 2,6-bis[N-(2-hydroxyethyl)iminomethyl]-4-methylphenol),143 Ti17O24(OiPr)20 with alkoxylate ligands,144 and octahedral [(BuSn)12O14(OH)6]2+ with alkyl ligands.145 In contrast, a few POMs composed of only four-coordinating units are reported including [Mn39O55]26− (ref. 146) (Fig. 7A). Interestingly, the anion-templated synthesis described in the section on ligand-protected noble metal clusters is also effective for the synthesis of POM clusters. The geometries and charges of the template anion provide clusters with various sophisticated core–shell structures, such as [(SCN)@HV22O54]6−, [(CH3COO)@H2V22O54]7−,147 [Cl@Eu15(OH)20]24+,148 and [(SO4)@As4Mo6V7O39]4−.149 Even electronically neutral chemical species, such as solvent molecules, often serve as templates. For example, water and acetonitrile molecules compose [(H2O)@V18O42]12− (ref. 150) and [(CH3CN)@V12O32]4−,151 respectively.
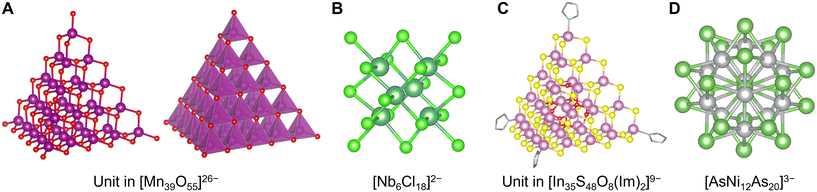 |
| Fig. 7 Crystal structures of (A) a unit in [Mn39O55]26− with a structure showing manganese oxide polyhedra (right),146 (B) [Nb6Cl18]2−,152 (C) a unit in [In35S48O8(Im)2]9−,153 and (D) [AsNi12As20]3−.155 Mn: reddish violet, O: red, Nb: bluish green, Cl: light green, In: pale magenta, S: yellow, Ni: gray, As: green, C: grayish bone, and N: light blue bone. Figures are reproduced from (A) CCDC 1728352, (B) CCDC 654117, (C) CCDC 1862551, and (D) CCDC 206301. | |
Except for the stabilization of clusters, such as the oxides mentioned above, other pnictogenides, chalcogenides, and halides can induce such stabilization. Polyhalometalate clusters, especially of early transition metals (Zr, Hf, Nb, Ta, Mo, W, Re), are well-known, such as [Nb6Cl18]2− (ref. 152) (Fig. 7B). The compound clusters also often have larger layered structures, such as tetrahedral [In35S48O8(Im)2]9−,153 tetrahedral [Sn10O4S20]8−,154 icosahedral [AsNi12As20]3−,155 and tetrahedral Sc4C10Sc20I30
156 (Fig. 7C and D). Additionally, it has been reported that the cation-templated synthesis of some chalcogenide clusters provides rare geometric structures, such as [(NH4)@Pd2S28]4− (ref. 157) and [Na2@Fe18S30]8−.158
In summary, in the case of clusters obtained as ionic compounds, the oxide clusters of the early transition metals (V, Mo, and W) are the most investigated, and the runner-up is another base metal element with a relatively high valence (Al, Ti, Mn, Fe, and U). Halide clusters of early transition metals (Zr, Nb, Mo, Hf, Ta, W, and Re) are the main compounds used in this field. Similar to ligand-protected clusters, the introduction of minor elements is achieved by doping a few atoms into the cluster structure composed of major elements. Particularly for POM clusters, it appears that metal elements in remarkably high oxidation states play an important role in diversifying their steric structures. One factor is that metals with high oxidation states maintain the charge balance of the entire cluster structure by reducing the negative charge on their own metalate units. If the metalate anion is represented as {MnO6}(12−n)−, its total negative charge is smaller when oxidation state n is higher. On the other hand, because the stability of these clusters is significantly associated with the affinity of the metal elements for pnictogens, chalcogens, and halogens, clusters composed of noble metals have rarely been reported. As represented by POMs, these clusters often have unique symmetric structures, whereas in the case of different metal elements, these metal oxide units tend to undergo atomic-level phase separation in a cluster and do not form true complex oxides with a uniform atomic co-arrangement. This suggests that the variety of ionic compound clusters can be expanded by improving the synthetic schemes.
4.3. Naked elementary clusters
In contrast, naked clusters composed only of icosagens, tetrels, pnictogens, chalcogens, and halogens (groups 13, 14, 15, 16, and 17) are also well known. Although white phosphorus (tetrahedral P4) is the most famous example, it is generally an ionic species called Zintl clusters or Zintl ions that is stabilized in crystals.159 In particular, there are many reports of Zintl clusters of group 13, 14, 15, and 16 metal elements with various polyhedral structures, such as icosahedral [Tl13]10−,160 trigonal-bypyramidal [Sn5]2−,161 bicapped-square-antiprismatic [Pb10]2−,162 square antiprismatic [Bi8]2+,163 square-planar [Te4]2+, and barrel-shaped [Te8]2+ (ref. 164) (Fig. 8A–C). In other cases, examples of clusters made of group 11, 12, and 17 elements are slightly known like icosahedral [Ag13]4+ (ref. 165) or polyhalogen cations and anions typified by rectangular [Cl4]+ (ref. 166) and trigonal-pyramidal [I7]− (ref. 167) (Fig. 8D and E). Similar to other methods, Zintl clusters also form molecular alloys (intermetalloids) of such elements including [BiIn8Bi12]3−/5− (ref. 168) and [K2Zn20Bi16]6−,169 or clusters incorporating a few other transition metal elements170 including [Sn9Pt2(PPh3)]2−, [Sn9Ni2(CO)]3− (ref. 171) and [Th@Bi12]4− (ref. 172) with and without protecting ligands, respectively (Fig. 8F and G).
 |
| Fig. 8 Crystal structures of (A) [Pb10]2−,162 (B) [Bi8]2+,163 (C) [Te8]2+,164 (D) [Ag13]4+,165 (E) [Cl4]+,166 (F) [K2Zn20Bi16]6−,169 and (G) [Th@Bi12]4−.172 Pb: black, Bi: purple, Te: grayish yellow, Ag: pale gray, Cl: light green, K: violet, Zn: gray, and Th: bluish green. Figures are reproduced from (A) CCDC 288640, (B) CCDC 1728864, (C) CCDC 1471867, (D) CCDC 1728248, (E) CCDC 1726993, (F) CCDC 1969162, and (G) CCDC 1983072. | |
As mentioned above, these clusters are mainly composed of post-transition elements with many valence electrons, and in many cases, they are positively or negatively charged structures. This tendency is rationalized by the thermodynamic stability based on the electronic configurations unique to clusters typified by superatoms.6–8,25,32,36,47,90 These clusters generally have relatively rigid structures with large bond angles of up to 90° originating from p-electrons and the ability to contain a few atoms of other group elements in electronically stabilized skeletons. In particular, the endohedral structures typified by stannaspherenes mentioned in the gas-phase synthesis section enable the incorporation of other groups of elements without ligands. It should be noted that the accurate choice of counter ions is an important factor in the stable design of these clusters because most clusters are obtained only in the crystal form.
4.4. Clusters obtained by nanospace-assisted template synthesis
The third method utilizes nanospace as reaction fields for cluster synthesis. Because the aforementioned synthetic methods depend on the thermodynamic stability of the cluster structure, only certain clusters with energetically suitable atomicity, atomic arrangement, and composition ratios were obtained. However, in this method, the reaction field is confined within a nanosized space using various approaches, and unique clusters in metastable states that are not found using other methods are forcibly obtained. The pores of zeolites serve as typical capsules for cluster synthesis, such as tetrahedral [Ag4(H2O)4]2+ in their cages.173–177 Metal–organic frameworks (MOFs) are also used for nanosized cages, and templated synthesis of various clusters, including Pt quasi-linear [Pd4]2+,178 tetrahedral Ir4,179 Pt12±x,180 and triple-decker trigonal [Au3L3-Ag-Au3L3-Ag-Au3L3]2+,181 has been reported. Recently, MOFs have been used to cluster carbon as a nonmetal element through selective template synthesis of polyacenes using reactants.182 Flexible supramolecular capsules realize the synthesis of such metastable clusters, including some allotropes of nonmetal elements, such as phosphorus P4
183 and sulfur S6 or S12,184 where the catenation behavior is controlled. In other cases, cages of crystalline proteins and nanospaces of macromolecules have been used as templates and protectors for clusters.185,186 In such cases, because the precision of cluster synthesis depends on the operation of the atomic assembly, it is expected that only one method will allow us to use a broad range of elements by adopting the same chemical principle for the assembly. Indeed, the atom hybridization method utilizing multimetallic multinuclear complexes of dendrimers as macromolecular templates for cluster synthesis affords the highest degrees of freedom in the design of multielemental clusters.187–191 Because it adopts the coordination behavior of guests for metal assembly based on the simple principle of acid–base chemistry, all elements can be treated under the same conditions during cluster synthesis. This approach led to the first synthesis of multimetallic clusters containing five or six metal elements like GaInAu3Bi2Sn6.187
In contrast to other liquid-phase synthesis methods, template synthesis provides clusters with relatively metastable structures, which are also regarded as intermediates in the process producing more stable clusters. Capsules need to be individually designed for each chemical element, while this approach, which reduces the direct influence originating from the properties of the elements, enables the use of a broad range of elements. Alternatively, in this method, the interaction between the capsules and precursor compounds, such as electrostatic interactions, acid–base reactions (including coordination), and hydrophobic interactions, is a key factor for accumulating atoms. However, it is important to stabilize such clusters in order to hold them in capsules or fix them on/in support materials. Moreover, in many cases, it is difficult to conduct the usual identification analyses, such as single-crystal X-ray diffraction and direct observation by STEM, SPM, and MS, which are often effective.
5. Solid-phase synthesis
Several methods have been reported for the synthesis of clusters in the solid phase. The mechanochemical synthesis of general nanoparticles has already been established, but that of clusters remains limited.192 For instance, Keggin-type POM of aluminum [Al13O4(OH)24(H2O)12]7+ can be obtained by a mechanochemical reaction between the [Al(H2O)6]Cl3 and (NH4)2CO3 reactants.193 Dimensional reduction of solid-state precursors is effective for the precise synthesis of clusters. Some metal chalcogenide or halide clusters typified by [Re6S6Cl8]2− are stoichiometrically synthesized by dimensional reduction of an extended solid-state structure.194,195 As an ultimate physical method, a technique for the mechanical synthesis of clusters by directly manipulating atoms using scanning probe microscopy was recently developed. Indeed, metal clusters such as Au12, Ag12, and Au5Pb have been synthesized directly on Si surfaces.196
As mentioned previously, the advantages of synthetic methods involving solid-phase processes are extremely limited in terms of the selectability of elements. However, bottom–up synthesis involving mechanochemical reactions and top–down synthesis involving dimensional reduction are significantly more effective for mass production than liquid-phase synthesis. This suggests that expanding the applicable elements in solid-phase synthesis will contribute to promoting clusters as more general and valuable materials in the future.
6. Conclusions and perspectives
As discussed above, the optimal method for synthesizing clusters varies significantly depending on the chemical element involved. Consequently, research on cluster synthesis has been pursued individually, with each method serving as a starting point. Notably, in liquid-phase processes, doping other group elements using the stable structures and atomic-level space obtained by each method has proven highly effective. Recently, these methods have been increasingly interconnected based on this approach.
However, according to the classification of clusters based on chemical elements, it is evident that there is no accurate border between cluster structures, even though each adopts a different synthetic method. For example, certain representatives among ligand-protected clusters and ionic compound clusters, namely [Be4(NH2)6(NH3)4]2+,75 [Zn10S4(SR)16]4−,59 Zn10O4(OCOR)12,79 In37P20(OCOCH2Ph)51,80 [Mn13O6(OH)2(OMe)4L6]4+,143 and [In35S48O8(Im)2]9−,153 partially contain both their two structural factors for cluster stabilization. [Sn9Pt2(PPh3)]2− (ref. 171) can be regarded as a cluster undergoing stabilizing effects by both ligand-protection and Zintl anions. Interestingly, the clusters developed using different methods often exhibit similar structures with reversed atomic arrangements and elemental ratios. Some of the simplest examples are [Co8S6(SPh)8]4− (ref. 58) and Co6Te8(PR3)6,72 where the transition metal and chalcogen atoms occupy reversed positions in the same symmetric structure. Moreover, [Sb3Rh20(CO)36]3− reported as a ligand-protected cluster with a few free post-transition metal atoms113 and [Sn9Ni2(CO)]3− reported as a Zintl cluster with a few ligand-protected transition metal atoms171 have a complementary relationship (Fig. 9A). Additionally, the [Mo6O22@Ag58S2(SPhtBu)36(OCOCF3)10] cluster obtained by the anion-template synthesis of ligand-protected clusters48,197 and the [Ag27@(Si2W18O66)3]31− clusters obtained by the expansion of the synthesis method of POMs140 also have complementary structures. In their skeletons, where the [Mo6O22]8− POM unit was encapsulated in a ligand-protected Ag58 cluster and the [Ag27]17+ unit was encapsulated in three open-Dawson-type POMs [Si2W18O66]16−, the core and shell structures were completely inverted (Fig. 9B). Ag6@(MoO4)7@Ag56(MoO4)2(SiPr)28(p-SO3PhMe)14 with a nested structure was recently synthesized.198 This similarity and reversibility of the cluster structures indicate that the area of elements covered by each synthetic method has been extended widely enough to be close to each other in recent investigations. This suggests that a more flexible cluster design can be realized in the future by improving synthetic techniques.
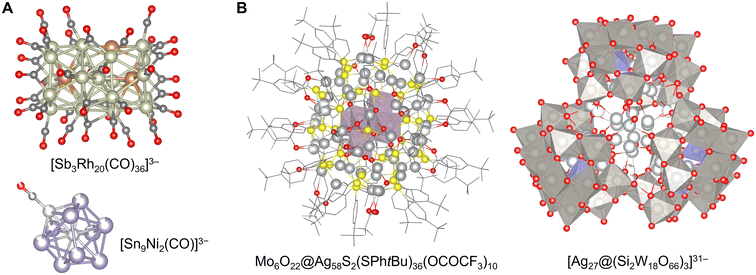 |
| Fig. 9 (A) Crystal structures of [Sb3Rh20(CO)36]3− (ref. 113) and [Sn9Ni2(CO)]3−,171 C: gray, O: red, Ni: light gray, Rh: grayish green, Sn: pale purple, and Sb: brown. (B) Crystal structures of Mo6O22@Ag58S2(SPhtBu)36(OCOCF3)10 showing molybdenum oxide polyhedra197 and [Ag27@(Si2W18O66)3]31− showing silicon oxide and tungsten oxide polyhedra.140 C: grayish bone, O: red, Si: pale blue polyhedron, S: yellow, Mo: reddish gray polyhedron, Ag: pale gray, and W: gray polyhedron. Part A reproduced from CCDC 1960490 and 185824. Part B reproduced from CCDC 1050793 and 1949947. | |
However, there are uninvestigated clusters for which precise synthetic methods that allow mass production, such as multimetallic clusters, have not yet been established. In light of the tendencies mentioned above, blending these individually developed synthetic methods is expected to make a breakthrough in revealing as-yet-unknown clusters. Although such an attempt has already been made, for example, the combination of ligand protection and nanospace-assisted synthesis methods,199 reports are still rare.
The electronic analogy between the clusters obtained using different methods suggests the possibility of new cluster structures by isomorphic substitution. For example, the ligand-protected [M(SR)2]n (M(II) = Ni, Pd, Pt) in section 4.1 might provide as-yet-unknown tiara-type bimetallic clusters, such as [M(SR)2]n[M'S2]x (M(III) = Mn, Fe, In), by doping based on the concept of chalcogenide clusters. Borane and Zintl clusters often have very similar geometric structures according to the Wade–Mingos rule,15,16 such as square pyramidal B5H9
200 and [In5]9− (ref. 201) or bicapped-square-antiprismatic [B10H10]2− (ref. 202) and [Pb10]2−.162 As some transition-metal elements doped with ligands have been reported,171,203 mutual feedback from each chemistry associated with the synthetic methods will become an effective approach for designing new cluster structures. Cation- or anion-templated methods will also contribute to the creation of such hybridized clusters because they are reported in both ligand-protected clusters and ionic compound clusters. Although conventional clusters obtained by ion-templated synthesis are composed of relatively simple anions as core structures, undeveloped ionic species of metals or metal oxides allow expandability in their structures. For example, by tracing these methods, the templated synthesis of clusters adopting uninvestigated ions, such as Ni2+, Zn2+, VO2+, RuO4−, and [Pt(OH)6]2−, may contribute to creating clusters with new combinations of elements. Additionally, the secondary chemical treatment of these clusters, inspired by nanospace-assisted synthesis, is expected to add new lineups, even in Zintl clusters. Unrevealed pure metal clusters typified by such Zintl clusters will be obtained by the reduction of ionic compound clusters with diverse structures typified by POMs as precursors.
In contrast to clusters, synthesis methods for nanoparticles cover a broad range of chemical elements and chemical fields.1 Therefore, hybridizing conventional synthesis methods for nanoparticles and clusters is expected to contribute significantly and provide new guidelines for cluster synthesis. As described in the sections on ligand-protected clusters, modifying the cluster structures with multidentate ligands is a strong candidate.123,124 The effectiveness of such an approach following the methods for nanoparticles might ultimately be extended to bulk materials containing clusters as partial structures, such as clathrate compounds, gas hydrates, or minerals204–208 and cluster units in metallic glasses or quasi-crystals.209–213
In conclusion, a comprehensive categorization of clusters based on their constituent chemical elements allowed us to understand which method covered which elements and revealed the common points of the structures synthesized by different methods. It is suggested that blending chemical concepts for stabilizing clusters by combining these methods or introducing them into nanoparticles will enable the synthesis of as-yet-unknown clusters not obtained by conventional techniques, affording us many bridgeheads for the next stage of developing cluster fields. Finally, the one-size-fits-all synthetic method constructed using such an approach is a new strategy to promote this cluster chemistry to a more central and larger chemistry field in the future, leading to the creation of next-generation functional materials, such as quantum-sized catalysts.
Author contributions
T. T. composed the entire manuscript.
Conflicts of interest
There are no conflicts of interest to declare.
Acknowledgements
The author acknowledges grants from JST PRESTO Grant Number JPMJPR20AA, JSPS KAKENHI Grant Number JP22H01904, the Advanced Technology Institute, the Yoshinori Ohsumi Fund for Fundamental Research, the JGC-Saneyoshi Scholarship Foundation, the Toshiaki Ogasawara Memorial Foundation, the Iwatani Naoji Foundation, the Inamori Foundation, Mizuho Foundation for the Promotion of Sciences, Iketani Science and Technology Foundation, and the Special Fund of Institute of Industrial Science, University of Tokyo. Drawings of the crystal structures of the clusters were reproduced using VESTA214 according to the Cambridge Crystallographic Data Centre (CCDC) database.
References
-
M. Naito, T. Yokoyama, K. Hosokawa and K. Nogi, Nanoparticle Technology Handbook, Elsevier, 3rd edn, 2018 Search PubMed
.
- C. Burda, X. Chen, R. Narayanan and M. A. El-Sayed, Chemistry and properties of nanocrystals of different shapes, Chem. Rev., 2005, 105, 1025–1102 CrossRef CAS PubMed
.
- K. D. Gilroy, A. Ruditskiy, H.-C. Peng, D. Qin and Y. Xia, Bimetallic nanocrystals: syntheses, properties, and applications, Chem. Rev., 2016, 116, 10414–10472 CrossRef CAS PubMed
.
- M. Arndt, O. Nairz, J. Vos-Andreae, C. Keller, G. van der Zouw and A. Zeilinger, Wave–particle duality of C60 molecules, Nature, 1999, 401, 680–682 CrossRef CAS PubMed
.
- M. Haruta, When gold is not noble: catalysis by nanoparticles, Chem. Rec., 2003, 3, 75–87 CrossRef CAS PubMed
.
- T. Tsukamoto, N. Haruta, T. Kambe, A. Kuzume and K. Yamamoto, Periodicity of molecular clusters based on symmetry-adapted orbital model, Nat. Commun., 2019, 10, 3727 CrossRef PubMed
.
- N. Haruta, T. Tsukamoto, A. Kuzume, T. Kambe and K. Yamamoto, Nanomaterials design for super-degenerate electronic state beyond the limit of geometrical symmetry, Nat. Commun., 2018, 9, 3758 CrossRef PubMed
.
- T. Tsukamoto, T. Kambe, T. Imaoka and K. Yamamoto, Modern cluster design based on experiment and theory, Nat. Rev. Chem., 2021, 5, 338–347 CrossRef CAS PubMed
.
- J. Yano and V. Yachandra, Mn4Ca cluster in photosynthesis: where and how water is oxidized to dioxygen, Chem. Rev., 2014, 114, 4175–4205 CrossRef CAS PubMed
.
- D. C. Johnson, D. R. Dean, A. D. Smith and M. K. Johnson, Structure, function, and formation of biological iron–sulfur clusters, Annu. Rev. Biochem., 2005, 74, 247–281 CrossRef CAS PubMed
.
- C. V. Stappen, L. Decamps, G. E. Cutsail III, R. Bjornsson, J. T. Henthorn, J. A. Birrell and S. DeBeer, The Spectroscopy of Nitrogenases, Chem. Rev., 2020, 120, 5005–5081 CrossRef PubMed
.
- Z. Gai, A. Matsuno, K. Kato, S. Kato, M. R. I. Khan, T. Shimizu, T. Yoshioka, Y. Kato, H. Kishimura, G. Kanno, Y. Miyabe, T. Terada, Y. Tanaka and M. Yao, Crystal structure of the 3.8-MDa respiratory supermolecule hemocyanin at 3.0 A° resolution, Structure, 2015, 23, 2204–2212 CrossRef CAS PubMed
.
- H. Komori and Y. Higuchi, Structural insights into the O2 reduction mechanism of multicopper oxidase, J. Biochem., 2015, 158, 293–298 CrossRef CAS PubMed
.
-
P. J. Dyson and J. S. McIndoe, Transition metal carbonyl cluster chemistry, CRC Press, 1st edn, 2000, vol. 2, Advanced chemistry texts Search PubMed
.
- K. Wade, Structural and bonding patterns in cluster chemistry, Adv. Inorg. Chem. Radiochem., 1976, 18, 1–66 CrossRef CAS
.
- D. M. P. Mingos, Polyhedral skeletal electron pair approach, Acc. Chem. Res., 1984, 17, 311–319 CrossRef CAS
.
- E. D. Jemmis and E. G. Jayasree, Analogies between boron and carbon, Acc. Chem. Res., 2003, 36, 816–824 CrossRef CAS PubMed
.
- H. W. Kroto, J. R. Heath, S. C. O'Brien, R. F. Curl and R. E. Smalley, C60: Buckminsterfullerene, Nature, 1985, 318, 162–163 CrossRef CAS
.
- S. Liu, Y.-J. Lu, M. M. Kappes and J. A. Ibers, The structure of the C60 molecule: X-ray crystal structure determination of a twin at 110K, Science, 1991, 254, 408–410 CrossRef CAS PubMed
.
- A. Astefanei, O. Núñez and M. T. Galceran, Characterisation and determination of fullerenes: A critical review, Anal. Chim. Acta, 2015, 882, 1–21 CrossRef CAS PubMed
.
- S. Guha and K. Nakamoto, Electronic structures and spectral properties of endohedral fullerenes, Coord. Chem. Rev., 2005, 249, 1111–1132 CrossRef CAS
.
- A. A. Popov, S. Yang and L. Dunsch, Endohedral fullerenes, Chem. Rev., 2013, 113, 5989–6113 CrossRef CAS PubMed
.
- M. D. Morse, Clusters of transition-metal atoms, Chem. Rev., 1986, 86, 1049–1109 CrossRef CAS
.
- W. D. Knight, K. Clemenger, W. A. de Heer, W. A. Saunders, M. Y. Chou and M. L. Cohen, Electronic shell structure and abundances of sodium clusters, Phys. Rev. Lett., 1984, 52, 2141–2143 CrossRef CAS
.
- D. E. Bergeron, A. W. Castleman, T. Morisato and S. N. Khanna, Formation of Al13I−: evidence for the superhalogen character of Al13, Science, 2004, 304, 84–87 CrossRef CAS PubMed
.
- L.-F. Cui, X. Huang, L.-M. Wang, J. Li and L.-S. Wang, Endohedral stannaspherenes M@Sn12−: a rich class of stable molecular cage clusters, Angew. Chem., Int. Ed., 2007, 46, 742–745 CrossRef CAS PubMed
.
- H.-J. Zhai, Y.-F. Zhao, W.-L. Li, Q. Chen, H. Bai, H.-S. Hu, Z. A. Piazza, W.-J. Tian, H.-G. Lu, Y.-B. Wu, Y.-W. Mu, G.-F. Wei, Z.-P. Liu, J. Li, S.-D. Li and L.-S. Wang, Observation of an all-boron fullerene, Nat. Chem., 2014, 6, 727–731 CrossRef CAS PubMed
.
- J. Li, X. Li, H.-J. Zhai and L.-S. Wang, Au20: a tetrahedral cluster, Science, 2003, 299, 864–867 CrossRef CAS PubMed
.
- K. Koyasu, M. Akutsu, M. Mitsui and A. Nakajima, Selective formation of MSi16 (M = Sc, Ti, and V), J. Am. Chem. Soc., 2005, 127, 4998–4999 CrossRef CAS PubMed
.
- M. Mitsui, S. Nagaoka, T. Matsumoto and A. Nakajima, Soft-landing isolation of vanadium–benzene sandwich clusters on a room- temperature substrate using n-alkanethiolate self- assembled monolayer matrixes, J. Am. Chem. Soc., 2006, 110, 2968–2971 CAS
.
- C. A. Schalley, G. Hornung, D. Schröder and H. Schwarz, Mass spectrometric approaches to the reactivity of transient neutrals, Chem. Soc. Rev., 1998, 27, 91–104 RSC
.
- S. Takano, S. Hasegawa, M. Suyama and T. Tsukuda, Hydride doping of chemically modified gold-based superatoms, Acc. Chem. Res., 2018, 51, 3074–3083 CrossRef CAS PubMed
.
- R. Jin, C. Zeng, M. Zhou and Y. Chen, Atomically precise colloidal metal nanoclusters and nanoparticles: fundamentals and opportunities, Chem. Rev., 2016, 116, 10346–10413 CrossRef CAS PubMed
.
- I. Chakraborty and T. Pradeep, Atomically precise clusters of noble metals: emerging link between atoms and nanoparticles, Chem. Rev., 2017, 117, 8208–8271 CrossRef CAS PubMed
.
- Q. Yao, T. Chen, X. Yuan and J. Xie, Toward total synthesis of thiolate- protected metal nanoclusters, Acc. Chem. Res., 2018, 51, 1338–1348 CrossRef CAS PubMed
.
- S. Sharma, K. K. Chakrahari, J.-Y. Saillard and C. W. Liu, Structurally precise dichalcogenolateprotected copper and silver superatomic nanoclusters and their alloys, Acc. Chem. Res., 2018, 51, 2475–2483 CrossRef CAS PubMed
.
- Y. Negishi, W. Kurashige and U. Kamimura, Isolation and structural characterization of an octaneselenolate-protected Au25 cluster, Langmuir, 2011, 27, 12289–12292 CrossRef CAS PubMed
.
- M. G. Taylor and G. Mpourmpakis, Thermodynamic stability of ligand-protected metal nanoclusters, Nat. Commun., 2017, 8, 15988 CrossRef CAS PubMed
.
- P. D. Jadzinsky, G. Calero, C. J. Ackerson, D. A. Bushnell and R. D. Kornberg, Structure of a thiol monolayer-protected gold nanoparticle at 1.1 Å resolution, Science, 2007, 318, 430–433 CrossRef CAS PubMed
.
- H. Qian and R. Jin, Controlling nanoparticles with atomic precision: The case of Au144(SCH2CH2Ph)60, Nano Lett., 2009, 9, 4083–4087 CrossRef CAS PubMed
.
- H.-C. Weissker, H. Escobar, V. D. Thanthirige, K. Kwak, D. Lee, G. Ramakrishna, R. L. Whetten and X. López-Lozano, Information on quantum states pervades the visible spectrum of the ubiquitous Au144(SR)60 gold nanocluster, Nat. Commun., 2014, 5, 3785 CrossRef PubMed
.
- Z. Wang, H.-F. g. Su, Y.-Z. Tan, S. Schein, S.-C. Lin, W. Liu, S.-A. Wang, W.-G. Wang, C.-H. Tung, D. Sun and L.-S. Zheng, Assembly of silver Trigons into a buckyball-like Ag180 nanocage, Proc. Natl. Acad. Sci. U. S. A., 2017, 114, 12132–12137 CrossRef CAS PubMed
.
- H. Yang, Y. Wang, X. Chen, X. Zhao, L. Gu, H. Huang, J. Yan, C. Xu, G. Li, J. Wu, A. J. Edwards, B. Dittrich, Z. Tang, D. Wang, L. Lehtovaara, H. Häkkinen and N. Zheng, Plasmonic twinned silver nanoparticles with molecular precision, Nat. Commun., 2016, 7, 12809 CrossRef CAS PubMed
.
- H. Yang, Y. Wang, H. Huang, L. Gell, L. Lehtovaara, S. Malola, H. Häkkinen and N. Zheng, All-thiol-stabilized Ag44 and Au12Ag32 nanoparticles with single-crystal structures, Nat. Commun., 2013, 4, 2422 CrossRef PubMed
.
- S. Hossain, Y. Niihori, L. V. Nair, B. Kumar, W. Kurashige and Y. Negishi, Alloy clusters: Precise synthesis and mixing effects, Acc. Chem. Res., 2018, 51, 3114–3124 CrossRef CAS PubMed
.
- W. Fei, S. Antonello, T. Dainese, A. Dolmella, M. Lahtinen, K. Rissanen, A. Venzo and F. Maran, Metal doping of Au25(SR)18− clusters: Insights and hindsights, J. Am. Chem. Soc., 2019, 141, 16033–16045 CrossRef CAS PubMed
.
- S. K. Barik, C.-Y. Chen, T.-H. Chiu, Y.-R. Ni, F. Gam, I. Chantrenne, S. Kahlal, J.-Y. Saillard and C. W. Liu, Surface modifications of eight-electron palladium silver superatomic alloys, Commun. Chem., 2022, 5, 151 CrossRef PubMed
.
- Y. Horita, M. Ishimi and Y. Negishi, Anion-templated silver nanoclusters: precise synthesis and geometric structure, Sci. Technol. Adv. Mater., 2023, 24, 2203832 CrossRef PubMed
.
- C. W. Liu, H.-W. Chang, C.-S. Fang, B. Sarkar and J.-C. Wang, Anion-templated syntheses of octanuclear silverclusters from a silver dithiophosphate chain, Chem. Commun., 2010, 46, 4571–4573 RSC
.
- J.-H. Liao, H.-W. Chang, Y.-J. Li, C.-S. Fang, B. Sarkar, W. E. van Zyl and C. W. Liu, Anion templating from a silver(I) dithiophosphate 1D polymer forming discrete cationic and neutral octa- and decanuclear silver(I) clusters, Dalton Trans., 2014, 43, 12380–12389 RSC
.
- H. J. Schugar, C. Ou, J. A. Thich, J. A. Potenza, R. A. Lalancette and W. Furey Jr., Molecular structure and copper(II)-mercaptide charge-transfer spectra of a novel Cu14[SC(CH3)2CH2NH2]12Cl cluster, J. Am. Chem. Soc., 1976, 98, 3047–3048 CrossRef CAS PubMed
.
- C. Zhang, T. Matsumoto, M. Samoc, S. Petrie, S. Meng, T. C. Corkery, R. Stranger, J. Zhang, M. G. Humphrey and K. Tatsumi, Dodecanuclear-ellipse and decanuclear-wheel nickel(II) thiolato clusters with efficient femtosecond nonlinear absorption, Angew. Chem., Int. Ed., 2010, 49, 4209–4212 CrossRef CAS PubMed
.
- J. Chen, L. Liu, L. Weng, Y. Lin, L. Liao, C. Wang, J. Yang and Z. Wu, Synthesis and properties evolution of a family of tiara-like phenylethanethiolated palladium nanoclusters, Sci. Rep., 2015, 5, 16628 CrossRef CAS PubMed
.
- T. Imaoka, Y. Akanuma, N. Haruta, S. Tsuchiya, K. Ishihara, T. Okayasu, W.-J. Chun, M. Takahashi and K. Yamamoto, Platinum clusters with precise numbers of atoms for preparative-scale catalysis, Nat. Commun., 2017, 8, 688 CrossRef PubMed
.
- Y. Yamashina, Y. Kataoka and Y. Ura, Inclusion of an iodine molecule in a tiara-like octanuclear palladium thiolate complex, Eur. J. Inorg. Chem., 2014, 2014, 4073–4078 CrossRef CAS
.
- Y. Akanuma, T. Imaoka, H. Sato and K. Yamamoto, Silver in the center enhances room-temperature phosphorescence of a platinum sub-nanocluster by 18 times, Angew. Chem., Int. Ed., 2021, 60, 4551–4554 CrossRef CAS PubMed
.
- A. Eichhöfer, P. T. Wood, R. N. Viswanath and R. A. Mole, Synthesis, structure and physical properties of the manganese(II) selenide/selenolate cluster complexes [Mn32Se14(SePh)36(PnPr3)4] and [Na(benzene-15-crown-5)(C4H8O)2]2[Mn8Se(SePh)16], Chem. Commun., 2008, 1596–1598 RSC
.
- G. Christou, K. S. Hagen and R. H. Holm, Synthesis, structure, and properties of [Co8S6(SC6H5)8]4− containing an octanuclear Co8S6 rhombic dodecahedron related to that of cobalt pentlandite, J. Am. Chem. Soc., 1982, 104, 1744–1745 CrossRef CAS
.
- I. G. Dance, A. Choy and M. L. Scudder, Syntheses, properties, and molecular and crystal structures of (Me4N)4[E4M10(SPh)16] (E = sulfur or selenium; M = zinc or cadmium): molecular supertetrahedral fragments of the cubic metal chalcogenide lattice, J. Am. Chem. Soc., 1984, 106, 6285–6295 CrossRef CAS
.
- T. I. Levchenko, B. E. G. Lucier, J. F. Corrigan and Y. Huang, Crystalline superlattices of nanoscopic CdS molecular clusters: An x-ray crystallography and 111Cd SSNMR spectroscopy study, Inorg. Chem., 2018, 57, 204–217 CrossRef CAS PubMed
.
- K. K. Singh, A. Bhattacharyya, S. Havenridge, M. Ghabin, H. Ausmann, M. A. Siegler, C. M. Aikens and A. Das, A first glance into mixed phosphine–stibine moieties as protecting ligands for gold clusters, Nanoscale, 2023, 15, 6934–6940 RSC
.
- R. H. Adnan, J. M. L. Madridejos, A. S. Alotabi, G. F. Metha and G. G. Andersson, A review of state of the art in phosphine ligated gold clusters and application in catalysis, Adv. Sci., 2022, 9, 2105692 CrossRef CAS PubMed
.
- C. A. McCandler, J. C. Dahl and K. A. Persson, Phosphine-stabilized hidden ground states in gold clusters investigated via a Aun(PH3)m database, ACS Nano, 2023, 17, 1012–1021 CrossRef CAS PubMed
.
- S. Liu, M. S. Eberhart, J. R. Norton, X. Yin, M. C. Neary and D. W. Paley, Cationic copper hydride clusters arising from oxidation of (Ph3P)6Cu6H6, J. Am. Chem. Soc., 2017, 139, 7685–7688 CrossRef CAS PubMed
.
- T. Nakajima, K. Nakamae, Y. Ura and T. Tanase, Multinuclear copper hydride complexes supported by polyphosphine ligands, Eur. J. Inorg. Chem., 2020, 2020, 2211–2226 CrossRef CAS
.
- A. J. Touchton, G. Wu and T. W. Hayton, Generation of a Ni3 phosphinidene cluster from the Ni(0) synthon, Ni(η3-CPh3)2, Organometallics, 2020, 39, 1360–1365 CrossRef CAS
.
- S.-S. Zhang, L. Feng, R. D. Senanayake, C. M. Aikens, X.-P. Wang, Q.-Q. Zhao, C.-H. Tung and D. Sun, Diphosphine-protected ultrasmall gold nanoclusters: opened icosahedral Au13 and heart-shaped Au8 clusters, Chem. Sci., 2018, 9, 1251–1258 RSC
.
- J. Chen, Q.-F. Zhang, P. G. Williard and L.-S. Wang, Synthesis and Structure Determination of a New Au20 Nanocluster Protected by Tripodal Tetraphosphine Ligands, Inorg. Chem., 2014, 53, 3932–3934 CrossRef CAS PubMed
.
- T. Tanase, R. Otaki, T. Nishida, H. Takenaka, Y. Takemura, B. Kure, T. Nakajima, Y. Kitagawa and T. Tsubomura, Strongly luminous tetranuclear gold(I) complexes supported by tetraphosphine ligands, meso- or rac-Bis[(diphenylphosphinomethyl)phenylphosphino]methane, Chem. – Eur. J., 2014, 20, 1577–1596 CrossRef CAS PubMed
.
- K. Nakamae, Y. Takemura, B. Kure, T. Nakajima, Y. Kitagawa and T. Tanase, Self-alignment of low-valent octanuclear palladium atoms, Angew. Chem., Int. Ed., 2015, 54, 1016–1021 CrossRef CAS PubMed
.
- A. Zavras, A. Mravak, M. Bužančić, J. M. White, V. Bonačić-Koutecký and R. A. J. O'Hair, Structure of the ligated Ag60 nanoparticle [{Cl@Ag12}@Ag48(dppm)12] (where dppm=bis(diphenylphosphino)methane), Chin. J. Chem. Phys., 2019, 32, 182–186 CrossRef CAS
.
- M. L. Steigerwald, T. Siegrist and S. M. Stuczynski, Octatelluridohexakis(triethylphosphine)hexacobalt and a connection between Chevrel clusters and the NiAs structure, Inorg. Chem., 1991, 30, 2256–2257 CrossRef CAS
.
- P. C. Ford and A. Vogler, Photochemical and photophysical properties of tetranuclear and hexanuclear clusters of metals with d10 and s2 electronic configurations, Acc. Chem. Res., 1993, 26, 220–226 CrossRef CAS
.
- M. R. Lichtenthaler, F. Stahl, D. Kratzert, L. Heidinger, E. Schleicher, J. Hamann, D. Himmel, S. Weber and I. Krossing, Cationic cluster formation versus disproportionation of low-valent indium and gallium complexes of 2,2′-bipyridine, Nat. Commun., 2015, 6, 8288 CrossRef CAS PubMed
.
- M. Müller, A. J. Karttunen and M. R. Buchner, Speciation of Be2+ in acidic liquid ammonia and formation of tetra- and octanuclear beryllium amido clusters, Chem. Sci., 2020, 11, 5415–5422 RSC
.
- W. Frank, V. Reiland and G. J. Reiβ, [Bi2(O2CCF3)4]·C6Me6 - Arene adduct of a reduced main group element carboxylate with paddle wheel structure, Angew. Chem., Int. Ed., 1998, 37, 2984–2985 CrossRef CAS
.
- F. H. Fry, B. A. Dougan, N. McCann, C. J. Ziegler and N. E. Brasch, Characterization of novel vanadium(III)/acetate clusters formed in aqueous solution, Inorg. Chem., 2005, 44, 5197–5199 CrossRef CAS PubMed
.
- J. K. Beattie, J. A. Klepetko, A. F. Masters and P. Turner, The chemistry of cobalt acetate. VIII. New members of the family of oxo-centred trimers, [Co3(μ3-O)(μ-O2CCH3)5−p(μ-OR)pL5]2+ (R=H, alkyl, L=ligand, p=0–4). The preparation and characterisation of the trimeric tetrakis(μ-acetato)-(μ-hydroxo)-μ3-oxo-pentakis(pyridine)-tri-cobalt(III) hexafluorophosphate, [Co3(μ3-O)(μ-O2CCH3)4(μ-OH)(C5H5N)5][PF6]2, and the preparation and crystal structure of the trimeric tris(μ-acetato)-(μ-hydroxo)-(μ-methoxo)-μ3-oxo-pentakis(pyridine)-tri-cobalt(III) hexafluorophosphate·methanol·water solvate [Co3(μ3-O)(μ-O2CCH3)3(μ-OH)(μ-OCH3)(C5H5N)5][PF6]2·CH3OH·0.25H2O, Polyhedron, 2003, 22, 947–965 CrossRef CAS
.
- L. Spanhel, Colloidal ZnO nanostructures and functional coatings: A survey, J. Sol-Gel Sci. Technol., 2006, 39, 7–24 CrossRef CAS
.
- D. C. Gary, S. E. Flowers, W. Kaminsky, A. Petrone, X. Li and B. M. Cossairt, Single-crystal and electronic structure of a 1.3 nm indium phosphide nanocluster, J. Am. Chem. Soc., 2016, 138, 1510–1513 CrossRef CAS PubMed
.
- A. Schnepf and H. Schnökel, Metalloid aluminum and gallium clusters: Element modifications on the molecular scale?, Angew. Chem., Int. Ed., 2002, 41, 3532–3552 CrossRef CAS PubMed
.
- A. Ecker, E. Weckert and H. Schnöckel, Synthesis and structural characterization of an AI77 cluster, Nature, 1997, 387, 379–381 CrossRef CAS
.
- M. Brynda, R. Herber, P. B. Hitchcock, M. F. Lappert, I. Nowik, P. P. Power, A. V. Protchenko, A. Růžička and J. Steiner, Higher-nuclearity group 14 metalloid clusters: [Sn9{Sn(NRR’)}6], Angew. Chem., Int. Ed., 2006, 45, 4333–4337 CrossRef CAS PubMed
.
- M. H. Chisholm, J. C. Huffman, C. C. Kirkpatrick, J. Leonelli and K. Folting, Metal alkoxides - models for metal oxides. 1. Preparations and structures of hexadecaalkoxytetratungsten compounds, W4(OR)16, where R = Me and Et, and octaoxotetraisopropoxytetrapyridinotetramolybdenum, Mo4(O)4(μ-O)2(μ3-O)2(O-i-Pr)2(μ-O-i-Pr)2(py)4, J. Am. Chem. Soc., 1981, 103, 6093–6099 CrossRef CAS
.
-
C. Elschenbroich, Organometallics, Wiley-VCH Verlag GmbH & Co. KGaA, Weinheim, 5th edn, 2016 Search PubMed
.
- H. Wadepohl and S. Gebert, (Cyclopentadienyl) metal cluster complexes of the group 9 transition metals, Coord. Chem. Rev., 1995, 143, 535–609 CrossRef CAS
.
- H. Banh, K. Dilchert, C. Schulz, C. Gemel, R. W. Seidel, R. Gautier, S. Kahlal, J.-Y. Saillard and R. A. Fischer, Atom-precise organometallic zinc clusters, Angew. Chem., Int. Ed., 2016, 55, 3285–3289 CrossRef CAS PubMed
.
- H. Schnöckel, Structures and properties of metalloid Al and Ga clusters open our eyes to the diversity and complexity of fundamental chemical and physical processes during formation and dissolution of metals, Chem. Rev., 2010, 110, 4125–4153 CrossRef PubMed
.
- H. Banh, J. Hornung, T. Kratz, C. Gemel, A. Pöthig, F. Gam, S. Kahlal, J.-Y. Saillard and R. A. Fischer, Embryonic brass: pseudo two electron Cu/Zn clusters, Chem. Sci., 2018, 9, 8906–8913 RSC
.
- J. Weßing, C. Ganesamoorthy, S. Kahlal, R. Marchal, C. Gemel, O. Cador, A. C. H. Da Silva, J. L. F. Da Silva, J.-Y. Saillard and R. A. Fischer, The mackay-type cluster [Cu43Al12](Cp*)12: Open-shell 67-electron superatom with emerging metal-like electronic structure, Angew. Chem., Int. Ed., 2018, 57, 14630–14634 CrossRef PubMed
.
- D. A. Buschmann, H. M. Dietrich, D. Schneider, V. M. Birkelbach, C. Stuhl, K. W. Törnroos, C. Maichle-Mössmer and R. Anwander, Nanoscale organolanthanum clusters: Nuclearity-directing role of cyclopentadienyl and halogenido ligands, Chem. – Eur. J., 2020, 26, 10834–10840 CrossRef CAS PubMed
.
- M. N. Sokolov and P. A. Abramov, Chalcogenide clusters of groups 8–10 noble metals, Coord. Chem. Rev., 2012, 256, 1972–1991 CrossRef CAS
.
- S. Reisinger, M. Bodensteiner, E. M. Pineda, J. J. W. McDouall, M. Scheer and R. A. Layfield, Addition of pnictogen atoms to chromium(II): synthesis, structure and magnetic properties of a chromium(IV) phosphide and a chromium(III) arsenide, Chem. Sci., 2014, 5, 2443–2448 RSC
.
- S. Heinl, K. Kiefer, G. Balázs, C. Wickleder and M. Scheer, The synthesis of the heterocubane cluster [{CpMn}4(μ3-P)4] as a tetrahedral shaped starting material for the formation of polymeric coordination compounds, Chem. Commun., 2015, 51, 13474–13477 RSC
.
- Y. Ohki, K. Munakata, Y. Matsuoka, R. Hara, M. Kachi, K. Uchida, M. Tada, R. E. Cramer, W. M. C. Sameera, T. Takayama, Y. Sakai, S. Kuriyama, Y. Nishibayashi and K. Tanifuji, Nitrogen reduction by the Fe sites of synthetic [Mo3S4Fe] cubes, Nature, 2022, 607, 86–90 CrossRef CAS PubMed
.
- T. Murahashi, R. Inoue, K. Usui and S. Ogoshi, Square tetrapalladium sheet sandwich complexes: cyclononatetraenyl as a versatile face-capping ligand, J. Am. Chem. Soc., 2009, 131, 9888–9889 CrossRef CAS PubMed
.
- M. Teramoto, K. Iwata, H. Yamaura, K. Kurashima, K. Miyazawa, Y. Kurashige, K. Yamamoto and T. Murahashi, Three-dimensional sandwich nanocubes composed of 13-atom palladium core and hexakis-carbocycle shell, J. Am. Chem. Soc., 2018, 140, 12682–12686 CrossRef CAS PubMed
.
- S. Gambarotta, C. Floriani, A. Chiesi-Villa and C. Guastini, A homoleptic arylgold(I) complex: Synthesis and structure of pentanuclear mesitylgold(I), J. Chem. Soc., Chem. Commun., 1983, 1304–1306 RSC
.
- E. M. Meyer, S. Gambarotta, C. Floriani, A. Chiesi-Villa and C. Guastini, Polynuclear aryl derivatives of group 11 Metals: Synthesis, solid state-solution structural relationship, and reactivity with phosphines, Organometallics, 1989, 8, 1067–1079 CrossRef CAS
.
- H. Müller, W. Seidel and H. Görls, Zur chemie des dimesityleisens: VI. Die struktur von tetramesityldieisen, J. Organomet. Chem., 1993, 445, 133–136 CrossRef
.
- X.-L. Pei, A. Pereira, E. S. Smirnova and A. M. Echavarren, Small gold(I) and gold(I)–silver(I) clusters by C−Si auration, Chem. – Eur. J., 2020, 26, 7309–7313 CrossRef CAS PubMed
.
- C. E. Melton, J. W. Dube, P. J. Ragogna, J. C. Fettinger and P. P. Power, Synthesis and characterization of primary aluminum parent amides and phosphides, Organometallics, 2014, 33, 329–337 CrossRef CAS
.
- Y. Sunada, K. Yamaguchi and K. Suzuki, “Template synthesis” of discrete metal clusters with two- or three-dimensional architectures, Coord. Chem. Rev., 2022, 469, 214673 CrossRef CAS
.
- T. Yamada, A. Mawatari, M. Tanabe, K. Osakada and T. Tanase, Planar tetranuclear and dumbbell-shaped octanuclear palladium complexes with bridging silylene ligands, Angew. Chem., Int. Ed., 2009, 48, 568–571 CrossRef CAS PubMed
.
- T. Koizumi, K. Tanaka, Y. Tsuchido, M. Tanabe, T. Ide and K. Osakada, Bimolecular fusion of [Pd3(μ-CN-C6H3Me2-2,6)3(CN-C6H3Me2-2,6)3] induced by Ph2GeH2: formation of the redox-active Pd6Ge2 complex, Dalton Trans., 2019, 48, 7541–7545 RSC
.
- Y. Umehara, R. Usui, Y. Wada and Y. Sunada, Dinuclear and tetranuclear group 10 metal complexes constructed from linear tetrasilane comprising both Si-H and Si-Si moieties, Commun. Chem., 2023, 6, 93 CrossRef CAS PubMed
.
- T. Tsumuraya, Y. Kabe and W. Ando, Synthesis and thermal decomposition, J. Organomet. Chem., 1994, 482, 131–138 CrossRef CAS
.
- W. P. Neumann and K. Kühlein, Organogermaniumverbindungen, i über diphenylgermanium. octaphenyl-cyclotetragerman, decaphenyl-cyclopentagerman und dodecaphenyl-cyclohexagerman, Justus Liebigs Ann. Chem., 1965, 683, 1–11 CrossRef CAS
.
- L. Roß and M. Dräger, Phases of Decaphenylcyclopentagermane (Ph2Ge)5, Z. Naturforsch., B: J. Chem. Sci., 2014, 38, 665–673 CrossRef
.
- G. Maier, S. Pfriem, U. Schäfer and R. Matusch, Tetra-tert-butyltetrahedrane, Angew. Chem., Int. Ed. Engl., 1978, 17, 520–521 CrossRef
.
- N. Wiberg, C. M. M. Finger and K. Polborn, Tetrakis(tri-tert-butylsilyl)-tetrahedro-tetrasilane (t,Bu3Si)4Si4: The first molecular silicon compound with a Si4 tetrahedron, Angew. Chem., Int. Ed. Engl., 1993, 32, 1054–1056 CrossRef
.
- V. G. Albano, L. Grossi, G. Longoni, M. Monari, S. Mulley and A. Sironi, Synthesis and characterization of the paramagnetic [Ag13Fe8(CO)32]4− tetraanion: A cuboctahedral Ag13 cluster stabilized by Fe(CO)4 groups behaving as four-electron donors, J. Am. Chem. Soc., 1992, 114, 5708–5713 CrossRef CAS
.
- C. Femoni, T. Funaioli, M. C. Iapalucci, S. Ruggieri and S. Zacchini, Rh–Sb nanoclusters: synthesis, structure, and electrochemical studies of the atomically precise [Rh20Sb3(CO)36]3− and [Rh21Sb2(CO)38]5− carbonyl compounds, Inorg. Chem., 2020, 59, 4300–4310 CrossRef CAS PubMed
.
- R. H. Sánchez, A. M. Willis, S.-L. Zheng and T. A. Betley, Synthesis of well-defined bicapped octahedral iron clusters [(trenL)2Fe8(PMe2Ph)2]n (n=0, −1), Angew. Chem., Int. Ed., 2015, 54, 12009–12013 CrossRef PubMed
.
- R. Wolf and W. Uhl, Main-group-metal clusters stabilized by N-heterocyclic carbenes, Angew. Chem., Int. Ed., 2009, 48, 6774–6776 CrossRef CAS PubMed
.
- T. Kruczyński, F. Henke, M. Neumaier, K. H. Bowen and H. Schnöckel, Many Mg–Mg bonds form the core of the Mg16Cp*8Br4K cluster anion: the key to a reassessment of the Grignard reagent (GR) formation process?, Chem. Sci., 2016, 7, 1543–1547 RSC
.
- M. Schütz, M. Muhr, K. Freitag, C. Gemel, S. Kahlal, J.-Y. Saillard, A. C. H. Da Silva, J. L. F. Da Silva, T. F. Fässler and A. Roland, Fischer, Contrasting Structure and Bonding of a Copper-Rich and a Zinc-Rich Intermetalloid Cu/Zn Cluster, Inorg. Chem., 2020, 59, 9077–9085 CrossRef PubMed
.
- R. D. Adams, M. Chen and X. Yang, Iridium–gold cluster compounds: Syntheses, structures, and an unusual ligand-induced skeletal rearrangement, Organometallics, 2012, 31, 3588–3598 CrossRef CAS
.
- N. de Silva and L. F. Dahl, Synthesis and structural analysis of the first nanosized platinum−gold carbonyl/phosphine cluster, Pt13[Au2(PPh3)2]2(CO)10(PPh3)4, containing a Pt-centered [Ph3PAu−AuPPh3]-capped icosahedral Pt12 cage, Inorg. Chem., 2005, 44(26), 9604–9606 CrossRef CAS PubMed
.
- E. G. Mednikov, M. C. Jewell and L. F. Dahl, Nanosized (μ12-Pt)Pd164(-xPtx(CO)72(PPh3)20 (x ≈ 7) containing Pt-centered four-shell 165-atom Pd−Pt core with unprecedented intershell bridging carbonyl ligands: Comparative analysis of icosahedral shell-growth patterns with geometrically related Pd145(CO)x(PEt3)30 (x ≈ 60) containing capped three-shell Pd145 core, J. Am. Chem. Soc., 2007, 129, 11619–11630 CrossRef CAS PubMed
.
- J. Yan, S. Malola, C. Hu, J. Peng, B. Dittrich, B. K. Teo, H. Häkkinen, L. Zheng and N. Zheng, Co-crystallization of atomically precise metal nanoparticles driven by magic atomic and electronic shells, Nat. Commun., 2018, 9, 3357 CrossRef PubMed
.
- J. S. Kanady, E. Y. Tsui, M. W. Day and T. Agapie, A synthetic model of the Mn3Ca subsite of the oxygen-evolving complex in photosystem II, Science, 2011, 333, 733–736 CrossRef CAS PubMed
.
- M. Kato, T. Fukui, H. Sato, Y. Shoji and T. Fukushima, Capturing the trajectory of metal-ion-cluster formation: Stepwise accumulation of Zn(II) ions in a robust coordination space formed by a rigid tridentate carboxylate ligand, Inorg. Chem., 2022, 8, 3649–3654 CrossRef PubMed
.
- N. Araki, K. Kusada, S. Yoshioka, T. Sugiyama, T. Ina and H. Kitagawa, Observation of the formation processes of hexagonal close-packed and face-centered cubic Ru nanoparticles, Chem. Lett., 2019, 48, 1062–1064 CrossRef CAS
.
- H. J. Lunk and H. Hartl, The fascinating polyoxometalates, ChemTexts, 2021, 7, 26 CrossRef CAS
.
- A. V. Anyushin, A. Kondinski and T. N. Parac-Vogt, Hybrid polyoxometalates as post-functionalization platforms: from fundamentals to emerging applications, Chem. Soc. Rev., 2020, 49, 382–432 RSC
.
- N. I. Gumerova and A. Rompel, Polyoxometalates in solution: speciation under spotlight, Chem. Soc. Rev., 2020, 49, 7568–7601 RSC
.
- A. Müller, S. K. Das, V. P. Fedin, E. Krickemeyer, C. Beugholt, H. Bögge, M. Schmidtmann and B. Hauptfleisch, Rapid and simple isolation of the crystalline molybdenum-blue compounds with discrete and linked nanosized ring-shaped anions: Na15[MoVI126MoV28O462H14(H2O)70]0.5[MoVI124MoV28O457H14(H2O)68]0.5·ca.400 H2O and Na22[MoVI118MoV28O442H14(H2O)58]·ca. 250 H2O, Z. Anorg. Allg. Chem., 1999, 625, 1187–1192 CrossRef
.
- A. Müller, B. Botar, S. K. Das, H. Bögge, M. Schmidtmann and A. Merca, On the complex hedgehog-shaped cluster species containing 368 Mo atoms: simple preparation method, new spectral details and information about the unique formation, Polyhedron, 2004, 23, 2381–2385 CrossRef
.
- P. C. Burns, K.-A. Kubatko, G. Sigmon, B. J. Fryer, J. E. Gagnon, M. R. Antonio and L. Soderholm, Actinyl peroxide nanospheres, Angew. Chem., Int. Ed., 2005, 44, 2135–2139 CrossRef CAS PubMed
.
- G. E. Sigmon, D. K. Unruh, J. Ling, B. Weaver, M. Ward, L. Pressprich, A. Simonetti and P. C. Burns, Symmetry versus minimal pentagonal adjacencies in uranium-based polyoxometalate fullerene topologies, Angew. Chem., Int. Ed., 2009, 48, 2737–2740 CrossRef CAS PubMed
.
- H. Zhang, A. Li, K. Li, Z. Wang, X. Xu, Y. Wang, M. V. Sheridan, H.-S. Hu, C. Xu, E. V. Alekseev, Z. Zhang, P. Yan, K. Cao, Z. Chai, T. E. Albrecht-Schönzart and S. Wang, Ultrafiltration separation of Am(VI)-polyoxometalate from lanthanides, Nature, 2023, 616, 482–487 CrossRef CAS PubMed
.
- A. Blazevic and A. Rompel, The Anderson–Evans polyoxometalate: From inorganic building blocks via hybrid organic–inorganic structures to tomorrows “Bio-POM”, Coord. Chem. Rev., 2016, 307, 42–64 CrossRef CAS
.
- D. D. Dexter and J. V. Silverton, A new structural type for heteropoly anions. The crystal structure of (NH4)2H6(CeMo12O42)·12H2O, J. Am. Chem. Soc., 1968, 90, 3589–3590 CrossRef CAS
.
- C. Boskovic, Rare earth polyoxometalates, Acc. Chem. Res., 2017, 50, 2205–2214 CrossRef CAS PubMed
.
- S. V. Krivovichev, Polyoxometalate clusters in minerals: review and complexity analysis, Acta Crystallogr., Sect. B: Struct. Sci., Cryst. Eng. Mater., 2020, 76, 618–629 CrossRef CAS PubMed
.
- Y. Sakai, K. Yoza, C. N. Kato and K. Nomiya, Tetrameric, trititanium(IV)-substituted polyoxotungstates with α-Dawson substructure as soluble metal-oxide analogues: Molecular structure of the giant “Tetrapod” [(α-1,2,3-P2W15Ti3O62)4{μ3-Ti(OH)3}4Cl]45−, Chem. – Eur. J., 2003, 9, 4077–4083 CrossRef CAS PubMed
.
- S. S. Mal and U. Kortz, The wheel-shaped Cu20 tungstophosphate [Cu20Cl(OH)24(H2O)12(P8W48O184)]25− ion, Angew. Chem., Int. Ed., 2005, 44, 3777–3780 CrossRef CAS PubMed
.
- S.-R. Li, H.-Y. Wang, H.-F. Su, H.-J. Chen, M.-H. Du, L.-S. Long, X.-J. Kong and L.-S. Zheng, A giant 3d-4f polyoxometalate super-tetrahedron with high proton conductivity, Small Methods, 2021, 5, 2000777 CrossRef CAS PubMed
.
- K. Yonesato, H. Ito, H. Itakura, D. Yokogawa, T. Kikuchi, N. Mizuno, K. Yamaguchi and K. Suzuki, Controlled assembly synthesis of atomically precise ultrastable silver nanoclusters with polyoxometalates, J. Am. Chem. Soc., 2019, 141, 19550–19554 CrossRef CAS PubMed
.
- W. H. Casey, Large aqueous aluminum hydroxide molecules, Chem. Rev., 2006, 106, 1–16 CrossRef CAS PubMed
.
- O. Sadeghi, L. N. Zakharov and M. Nyman, Aqueous formation and manipulation of the iron-oxo Keggin ion, Science, 2015, 347, 1359–1362 CrossRef CAS PubMed
.
- G. N. Newton, S. Yamashita, K. Hasumi, J. Matsuno, N. Yoshida, M. Nihei, T. Shiga, M. Nakano, H. Nojiri, W. Wernsdorfer and H. Oshio, Redox-controlled magnetic {Mn13} Keggin systems, Angew. Chem., Int. Ed., 2011, 50, 5716–5720 CrossRef CAS PubMed
.
- C. Liu, J. Hu, F. Zhu, J. Zhan, L. Du, C.-H. Tung and Y. Wang, Functionalization of titanium oxide cluster Ti17O24(OiC3H7)20 with catechols: Structures and ligand-exchange reactivities, Chem. – Eur. J., 2019, 25, 14843–14849 CrossRef CAS PubMed
.
- C. Eychenne-Baron, F. Ribot and C. Sanchez, New synthesis of the nanobuilding block {(BuSn)12O14(OH)6}2+ and exchange properties of {(BuSn)12O14(OH)6}(O3SC6H4CH3)2, J. Organomet. Chem., 1998, 567, 137–142 CrossRef CAS
.
- A. Möller, P. Amann, V. Kataev and N. Schittner, The first T5-supertetrahedron in oxide chemistry: Na26Mn39O55, Z. Anorg. Allg. Chem., 2004, 630, 890–894 CrossRef
.
- A. Müller, D.-C. R. Rohlfing, E. Krickemeyer and H. Bögge, Control of the linkage of inorganic fragments of V–O compounds: From cluster shells as carcerands via cluster aggregates to solid-state structures, Angew. Chem., Int. Ed. Engl., 1993, 32, 909–912 CrossRef
.
- R. Wang, H. D. Selby, H. Liu, M. D. Carducci, T. Jin, Z. Zheng, J. W. Anthis and R. J. Staples, Halide-templated assembly of polynuclear lanthanide-hydroxo complexes, Inorg. Chem., 2002, 41, 278–286 CrossRef CAS PubMed
.
- A. Müller, E. Krickemeyer, S. Dillinger, H. Bögge and A. Stammler, [As4Mo6V7O39(SO4)]4−: A species with an unusual structure and a model for the different host-guest properties of poly-vanadates and -molybdates, J. Chem. Soc., Chem. Commun., 1994, 2539–2540 RSC
.
- G. K. Johnson and E. O. Schlemper, Existence and structure of the molecular ion 18-vanadate(IV), J. Am. Chem. Soc., 1978, 100, 3645–3646 CrossRef CAS
.
- V. W. Day, W. G. Klemperer and O. M. Yaghi, Synthesis and characterization of a soluble oxide inclusion complex, [CH3CN⊂(V12O324−)], J. Am. Chem. Soc., 1989, 111, 5959–5961 CrossRef CAS
.
- A. Flemming, A. Hoppe and M. Köckerling, Synthesis and structures of new niobium cluster compounds with pyridinium cations: (PyrH)2[Nb6Cl18]·EtOH (Pyr: pyridine, Et: ethyl) and the cubic modification of (PyrH)2[Nb6Cl18], J. Solid State Chem., 2008, 181, 2660–2665 CrossRef CAS
.
- H. Yang, J. Zhang, M. Luo, W. Wang, H. Lin, Y. Li, D. Li, P. Feng and T. Wu, The largest supertetrahedral oxychalcogenide nanocluster and its unique assembly, J. Am. Chem. Soc., 2018, 140, 11189–11192 CrossRef CAS PubMed
.
- T. Kaib, M. Kapitein and S. Dehnen, Synthesis and crystal structure of [Li8(H2O)29][Sn10O4S20]·2H2O. Z, Anorg. Allg. Chem., 2011, 637, 1683–1686 CrossRef CAS
.
- M. J. Moses, J. C. Fettinger and B. W. Eichhorn, Interpenetrating As20 fullerene and Ni12 icosahedra in the onion-skin [As@Ni12@As20]3− ion, Science, 2003, 300, 778–780 CrossRef CAS PubMed
.
- L. Jongen, A.-V. Mudring and G. Meyer, The molecular solid Sc24C10I30 : A truncated, hollow T4 supertetrahedron of iodine filled with a T3 supertetrahedron of scandium that encapsulates the adamantoid cluster Sc4C10, Angew. Chem., Int. Ed., 2006, 45, 1886–1889 CrossRef CAS PubMed
.
- A. Müller, K. Schmitz, E. Krickemeyer, M. Penk and H. Bögge, [Pd2S28]4− : A 30-membered cage containing an entrapped cation, Angew. Chem., Int. Ed. Engl., 1986, 25, 453–454 CrossRef
.
- J. F. You, B. S. Snyder, G. C. Papaefthymiou and R. H. Holm, On the molecular solid-state boundary. A cyclic iron-sulfur cluster of nuclearity eighteen: synthesis, structure, and properties, J. Am. Chem. Soc., 1990, 112, 1067–1076 CrossRef CAS
.
- S. Scharfe, F. Kraus, S. Stegmaier, A. Schier and T. F. Fässler, Zintl ions, cage compounds, and intermetalloid clusters of group 14 and group 15 elements, Angew. Chem., Int. Ed., 2011, 50, 3630–3670 CrossRef CAS PubMed
.
- Z.-C. Dong and J. D. Corbett, Unusual
icosahedral cluster compounds: Open-shell Na4A6Tl13 (A = K, Rb, Cs) and the metallic Zintl phase Na3K8Tln (How does chemistry work in solids?), J. Am. Chem. Soc., 1995, 117, 6447–6455 CrossRef CAS
.
- M. Somer, W. Carrillo-Cabrera, E.-M. Peters, K. Peters, M. Kaupp and H. G. von Schnering, The [Sn5]2− cluster compound [K-(2,2,2-crypt)]2Sn5 − synthesis, crystal structure, raman spectrum, and hierarchical relationship to CaIn2, Z. Anorg. Allg. Chem., 1999, 625, 37–42 CrossRef CAS
.
- A. Spiekermann, S. D. Hoffmann and T. F. Fässler, The Zintl ion [Pb10]2−: A rare example of a homoatomic closo cluster, Angew. Chem., Int. Ed., 2006, 45, 3459–3462 CrossRef CAS PubMed
.
- M. Lindsjö, A. Fischer and L. Kloo, Improvements of and Insights into the Isolation of Bismuth Polycations from Benzene Solution – Single-Crystal Structure Determinations of Bi8[GaCl4]2 and Bi5[GaCl4]3, Eur. J. Inorg. Chem., 2005, 2005, 670–675 CrossRef
.
- C. Schulz, J. Daniels, T. Bredow and J. Beck, The electrochemical synthesis of polycationic clusters, Angew. Chem., Int. Ed., 2016, 55, 1173–1177 CrossRef CAS PubMed
.
- S. Ahlert, W. Klein, O. Jepsen, O. Gunnarsson, O. K. Andersen and M. Jansen, Ag13OsO6: A silver oxide with interconnected icosahedral Ag134+ clusters and dispersed [OsO6]4− octahedra, Angew. Chem., Int. Ed., 2003, 42, 4322–4325 CrossRef CAS PubMed
.
- S. Seidel and K. Seppelt, The Cl4+ Ion, Angew. Chem., Int. Ed., 2000, 39, 3923–3925 CrossRef CAS PubMed
.
- P. H. Svensson and L. Kloo, Synthesis, structure, and bonding in polyiodide and metal iodide-iodine systems, Chem. Rev., 2003, 103, 1649–1684 CrossRef CAS PubMed
.
- N. V. Tkachenko, X.-W. Zhang, L. Qiao, C.-C. Shu, D. Steglenko, A. MuÇoz-Castro, Z.-M. Sun and A. I. Boldyrev, Spherical aromaticity of all-metal [Bi@In8Bi12]3−/5− clusters, Chem. – Eur. J., 2020, 26, 2073–2079 CrossRef CAS PubMed
.
- A. R. Eulenstein, Y. J. Franzke, P. Bügel, W. Massa, F. Weigend and S. Dehnen, Stabilizing a metalloid {Zn12} unit within a polymetallide environment in [K2Zn20Bi16]6−, Nat. Commun., 2020, 11, 5122 CrossRef CAS PubMed
.
- R. J. Wilson, N. Lichtenberger, B. Weinert and S. Dehnen, Intermetalloid and heterometallic clusters combining p-block (semi)metals with d- or f-block metals, Chem. Rev., 2019, 119, 8506–8554 CrossRef CAS PubMed
.
- B. Kesanli, J. Fettinger, D. R. Gardner and B. Eichhorn, The [Sn9Pt2(PPh3)]2− and [Sn9Ni2(CO)]3− complexes: Two markedly different Sn9M2L transition metal Zintl ion clusters and their dynamic behaviour, J. Am. Chem. Soc., 2002, 124, 4779–4786 CrossRef CAS PubMed
.
- A. R. Eulenstein, Y. J. Franzke, N. Lichtenberger, R. J. Wilson, H. L. Deubner, F. Kraus, R. Clérac, F. Weigend and S. Dehnen, Substantial π-aromaticity in the anionic heavy-metal cluster [Th@Bi12]4−, Nat. Chem., 2021, 13, 149–155 CrossRef CAS PubMed
.
- M. Quintanilla and L. M. Liz-Marzán, Caged clusters shine brighter, Science, 2018, 361, 645 CrossRef CAS PubMed
.
- E. Coutiño-Gonzalez, W. Baekelant, J. A. Steele, C. W. Kim, M. B. J. Roeffaers and J. Hofkens, Silver clusters in zeolites: from self- assembly to ground- breaking luminescent properties, Acc. Chem. Res., 2017, 50, 2353–2361 CrossRef PubMed
.
- M. Choi, Z. Wu and E. Iglesia, Mercaptosilane-assisted synthesis of metal clusters within zeolites and catalytic consequences of encapsulation, J. Am. Chem. Soc., 2010, 132, 9129–9137 CrossRef CAS PubMed
.
- N. Wang, Q. Sun, R. Bai, X. Li, G. Guo and J. Yu, In situ confinement of ultrasmall
Pd clusters within nanosized silicalite-1 zeolite for highly efficient catalysis of hydrogen generation, J. Am. Chem. Soc., 2016, 138, 7484–7487 CrossRef CAS PubMed
.
- L. Liu, Generation of subnanometric platinum with high stability during transformation of a 2D zeolite into 3D, Nat. Mater., 2017, 16, 132–138 CrossRef CAS PubMed
.
- F. R. Fortea-Pérez, M. Mon, J. Ferrando-Soria, M. Boronat, A. Leyva-Pérez, A. Corma, J. M. Herrera, D. Osadchii, J. Gascon, D. Armentano and E. Pardo, The MOF-driven synthesis of supported palladium clusters with catalytic activity for carbene-mediated chemistry, Nat. Mater., 2017, 16, 760–766 CrossRef PubMed
.
- D. Yang, C. A. Gaggioli, E. Conley, M. Babucci, L. Gagliardi and B. C. Gates, Synthesis and characterization of tetrairidium clusters in the metal organic framework UiO-67: Catalyst for ethylene hydrogenation, J. Catal., 2020, 382, 165–172 CrossRef CAS
.
- K. Kratzl, T. Kratky, S. Günther, O. Tomanec, R. Zbořil, J. Michalička, J. M. Macak, M. Cokoja and R. A. Fischer, Generation and stabilization of small platinum clusters Pt12±x inside a metal−organic framework, J. Am. Chem. Soc., 2019, 141, 13962–13969 CrossRef CAS PubMed
.
- T. Osuga, T. Murase and M. Fujita, Triple-decker Au3−Ag–Au3−Ag–Au3 ion cluster enclosed in a self-assembled cage, Angew. Chem., Int. Ed., 2012, 51, 12199–12201 CrossRef CAS PubMed
.
- T. Kitao, T. Miura, R. Nakayama, Y. Tsutsui, Y. S. Chan, H. Hayashi, H. Yamada, S. Seki, T. Hitosugi and T. Uemura, Synthesis of polyacene by using a metal–organic framework, Nat. Synth., 2023, 2, 848–854 CrossRef
.
- P. Mal, B. Breiner, K. Rissanen and J. R. Nitschke, White phosphorus is air-stable within a self-assembled tetrahedral capsule, Science, 2009, 324, 1697–1699 CrossRef CAS PubMed
.
- S. Matsuno, M. Yamashina, Y. Sei, M. Akita, A. Kuzume, K. Yamamoto and M. Yoshizawa, Exact mass analysis of sulfur clusters upon encapsulation by a polyaromatic capsular matrix, Nat. Commun., 2017, 8, 749 CrossRef PubMed
.
- B. Maity, S. Abe and T. Ueno, Observation of gold subnanocluster nucleation within a crystalline protein cage, Nat. Commun., 2017, 8, 14820 CrossRef CAS PubMed
.
- R. M. Crooks, M. Zhao, L. Sun, V. Chechik and L. K. Yeung, Dendrimer-encapsulated metal nanoparticles: synthesis, characterization, and applications to catalysis, Acc. Chem. Res., 2001, 34, 181–190 CrossRef CAS PubMed
.
- T. Tsukamoto, T. Kambe, A. Nakao, T. Imaoka and K. Yamamoto, Atom-hybridization for synthesis of polymetallic clusters, Nat. Commun., 2018, 9, 3873 CrossRef PubMed
.
- T. Tsukamoto, T. Imaoka and K. Yamamoto, Unique functions and applications of rigid dendrimers featuring radial aromatic chains, Acc. Chem. Res., 2021, 54, 4486–4497 CrossRef CAS PubMed
.
- T. Tsukamoto, K. Tomozawa, T. Moriai, N. Yoshida, T. Kambe and K. Yamamoto, Highly accurate synthesis of quasi-sub-nanoparticles by dendron-assembled supramolecular templates, Angew. Chem., Int. Ed., 2022, 61, e202114353 CrossRef CAS PubMed
.
- T. Tsukamoto, A. Kuzume, M. Nagasaka, T. Kambe and K. Yamamoto, Quantum materials exploration by sequential screening technique of heteroatomicity, J. Am. Chem. Soc., 2020, 142, 19078–19084 CrossRef CAS PubMed
.
- T. Moriai, T. Tsukamoto, M. Tanabe, T. Kambe and K. Yamamoto, Selective hydroperoxygenation of olefin realized by coinage multimetallic 1-nanometer catalyst, Angew. Chem., Int. Ed., 2020, 59, 23051–23055 CrossRef CAS PubMed
.
- A. Murugadoss, N. Kai and H. Sakurai, Synthesis of bimetallic gold–silver alloy nanoclusters by simple mortar grinding, Nanoscale, 2012, 4, 1280–1282 RSC
.
- P. Billik and B. Horváth, Mechanochemical synthesis of the [Al13O4(OH)24(H2O)12]7+ Keggin ion, Inorg. Chem. Commun., 2008, 11, 1125–1127 CrossRef CAS
.
- J.-C. Gabriel, K. Boubekeur and P. Batail, Molecular hexanuclear clusters in the system rhenium-sulfur-chlorine: solid state synthesis, solution chemistry, and redox properties, Inorg. Chem., 1993, 32, 2894–2900 CrossRef CAS
.
- E. G. Tulsky and J. R. Long, Dimensional reduction: a practical formalism for manipulating solid structures, Chem. Mater., 2001, 13, 1149–1166 CrossRef CAS
.
- Y. Sugimoto, A. Yurtsever, N. Hirayama, M. Abe and S. Morita, Mechanical gate control for atom-by-atom cluster assembly with scanning probe microscopy, Nat. Commun., 2014, 5, 4360 CrossRef CAS PubMed
.
- X.-Y. Li, Y.-Z. Tan, K. Yu, X.-P. Wang, Y.-Q. Zhao, D. Sun and L.-S. Zheng, Atom-Precise polyoxometalate–Ag2S core–shell nanoparticles, Chem. – Asian J., 2015, 10, 1295–1298 CrossRef CAS PubMed
.
- Z. Wang, H.-F. Su, M. Kurmoo, C.-H. Tung, D. Sun and L.-S. Zheng, Trapping an octahedral Ag6 kernel in a seven-fold symmetric Ag56 nanowheel, Nat. Commun., 2018, 9, 2094 CrossRef PubMed
.
- H. Muramatsu, T. Kambe, T. Tsukamoto, T. Imaoka and K. Yamamoto, Controlled synthesis of Au25 superatom using a dendrimer template, Molecules, 2022, 27, 3398 CrossRef CAS PubMed
.
- H. J. Hrostowski and R. J. Myers, The microwave spectra, structure, and dipole moment of stable pentaborane, J. Chem. Phys., 1954, 22, 262–265 CrossRef CAS
.
- J.-T. Zhao and J. D. Corbett, Square pyramidal clusters in La3In5 and Y3In5. La3In5 as a metallic Zintl phase, Inorg. Chem., 1995, 34, 378–383 CrossRef CAS
.
- V. M. Retivov, E. Y. Matveev, M. V. Lisovskiy, G. A. Razgonyaeva, L. I. Ochertyanova, K. Y. Zhizhin and N. T. Kuznetsov, Nucleophilic substitution in closo-decaborate [B10H10]2− in the presence of carbocations, Russ. Chem. Bull., 2010, 59, 550–555 CrossRef CAS
.
- I. J. Mavunkal, B. C. Noll, R. Meijboom, A. Muller and T. P. Fehlner, Novel Approach to Multimetal Metallaborane Clusters. Synthesis of Hypoelectronic nido-Cp*3IrRu2B5H9 from the Reaction of arachno-Cp*IrB3H9 with nido-(Cp*RuH)2B3H7, Organometallics, 2006, 25, 2906–2907 CrossRef CAS
.
- R. Ludwig, Water: from clusters to the bulk, Angew. Chem., Int. Ed., 2001, 40, 1808–1827 CrossRef CAS PubMed
.
- A. Hassanpouryouzband, E. Joonaki, M. Vasheghani Farahani, S. Takeya, C. Ruppel, J. Yang, N. J. English, J. M. Schicks, K. Edlmann, H. Mehrabian, Z. M. Aman and B. Tohidi, Gas hydrates in sustainable chemistry, Chem. Soc. Rev., 2020, 49, 5225–5309 RSC
.
- A. Falenty, T. C. Hansen and W. F. Kuhs, Formation and properties of ice XVI obtained by emptying a type sII clathrate hydrate, Nature, 2014, 516, 231–233 CrossRef CAS PubMed
.
- K. Momma, T. Ikeda, K. Nishikubo, N. Takahashi, C. Honma, M. Takada, Y. Furukawa, T. Nagase and Y. Kudoh, New silica clathrate minerals that are isostructural with natural gas hydrates, Nat. Commun., 2011, 2, 196 CrossRef PubMed
.
- A. Y. Likhacheva, S. V. Goryainov, Y. V. Seryotkin, K. D. Litasov and K. Momma, Raman spectroscopy of chibaite, natural MTN silica clathrate, at high pressure up to 8 GPa, Microporous Mesoporous Mater., 2016, 224, 100–106 CrossRef CAS
.
- A. Hirata, L. J. Kang, T. Fujita, B. Klumov, K. Matsue, M. Kotani, A. R. Yavari and M. W. Chen, Geometric frustration of icosahedron in metallic glasses, Science, 2013, 341, 376–379 CrossRef CAS PubMed
.
- V. Y. Shevchenko, V. A. Blatov and G. D. Ilyushin, Cluster self-organization of intermetallic systems: New three-layer cluster precursor K136 = 0@Zn12@32(Mg20Zn12)@92(Zr12Zn80) and a new two-layer cluster precursor K30 = 0@Zn6@Zn24 in the crystal structure of Zr6Mg20Zn128-cP,154, Glass Phys. Chem., 2020, 46, 455–461 CrossRef CAS
.
- D. Shechtman, I. Blech, D. Gratias and J. W. Cahn, Metallic phase with long-range orientational order and no translational symmetry, Phys. Rev. Lett., 1984, 53, 1951–1953 CrossRef CAS
.
- D. Levine and P. J. Steinhardt, Quasicrystals: a new class of ordered structures, Phys. Rev. Lett., 1984, 53, 2477–2480 CrossRef CAS
.
- H. Takakura, C. P. Gómez, A. Yamamoto, M. De Boissieu and A. P. Tsai, Atomic structure of the binary icosahedral Yb–Cd quasicrystal, Nat. Mater., 2007, 6, 58–63 CrossRef CAS PubMed
.
- K. Momma and F. Izumi, VESTA 3 for three-dimensional visualization of crystal, volumetric and morphology data, J. Appl. Crystallogr., 2011, 44, 1272–1276 CrossRef CAS
.
|
This journal is © The Royal Society of Chemistry 2024 |