DOI:
10.1039/D4NH00093E
(Review Article)
Nanoscale Horiz., 2024, Advance Article
Recent advances in fluorescence and afterglow of CDs in matrices
Received
2nd March 2024
, Accepted 11th April 2024
First published on 12th April 2024
Abstract
Carbon dots (CDs) are novel nanomaterials with dimensions less than 10 nm that have attracted much attention due to their outstanding optical properties. However, the development of solid-state fluorescence and afterglow methods has been relatively slow, although the properties of these materials under liquid conditions have been extensively studied. In recent years, embedding CDs in a matrix has been shown to prevent aggregation quenching and inhibit nonradiative transitions, thus realizing solid-state fluorescence and afterglow, which has greatly broadened the research and application areas of CDs. In terms of hydrogen bonding, ionic bonding, covalent bonding and spatial confinement, the interactions between CDs and matrices can effectively realize and improve the solid-state fluorescence and afterglow effects of CDs. Recent applications of CDs in matrices in optoelectronics, information security, sensing, biotherapeutics and imaging are also summarized. Finally, we summarize the challenges and developments of CDs in matrices.
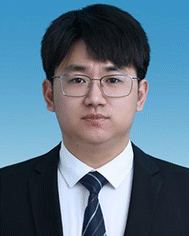 Qiang Fu | Qiang Fu is a lecturer and master tutor at Qufu Normal University, China. He received his Dr degree in 2020 from Beijing Normal University in China. His current research interests focus on the design of optical materials, the synthesis and application of carbon dot-based afterglow materials, analysis of basic mass spectrometry, and electrochemical preparation of nanomaterials. |
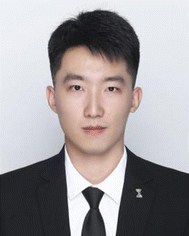 Kangzhi Lu | Kangzhi Lu received his BS degree from the College of Engineering (2021), Qufu Normal University, China. Currently, he is studying for his MS degree at the College of Engineering, Qufu Normal University. His research interests mainly focus on the synthesis and application of carbon dot-based afterglow materials. |
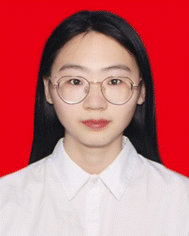 Shouhong Sun | Shouhong Sun received her BS degree from the College of Engineering (2022), Qufu Normal University, China. Currently, she is studying for her MS degree at the College of Engineering, Qufu Normal University. Her research interests mainly focus on the synthesis and application of carbon dot-based afterglow materials. |
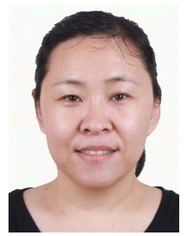 Zhanhua Dong | Zhanhua Dong is an associate professor and master tutor at Qufu Normal University, China. She received her Dr degree from Jiangnan University in China. Her current research interests focus on food packaging and safety, food packaging safety and risk assessment based on artificial intelligence technology. In recent years, she has published more than 10 papers, including 3 SCI indexed papers, numerous EI indexed papers, 1 academic work, and has been granted several invention patents. |
1. Introduction
Carbon dots (CDs) have been shown to possess great potential for applications in optoelectronics,1–3 sensing,4,5 information security,6 and biology7,8 due to their nontoxicity, low cost, superior optical properties and biocompatibility. The optical properties of CDs in solution have been extensively studied and improved.9–11 Currently, the realization of fluorescence and afterglow of solid-state CDs has become a hot research topic because of the limited application of CDs in the solution state. In the solid state, CDs are challenged by aggregation-caused quenching (ACQ) and π–π stacking effects, which may lead to inefficient or quenched fluorescence; additionally, these CDs fail to generate sufficient intersystem crossing (ISC) and are susceptible to external environmental influences, leading to inefficient or quenched afterglow. The research on the realization of solid-state fluorescence and afterglow based on matrix-free CDs is also in progress and has made some progress. Compared with no matrix, the study of matrix binding is also particularly important.12–14 To overcome these problems, new matrix materials or composites can be introduced to improve the stability and fluorescence/afterglow properties of solid-state CDs and to modulate the electronic and energy band structures of CDs through interactions, which in turn affect their fluorescence and afterglow properties.
To achieve solid-state fluorescence and afterglow, one approach is to encapsulate CDs into a matrix with a rigid structure, such as urea/melamine,15 silica,16 boric acid (BA),17 inorganic salts,18 zeolites,19 biomass,20 or polymers.21 The realization and improvement of solid-state fluorescence and afterglow of CDs can be achieved through a combination of hydrogen, ionic and covalent bonding interactions and synergistic effects (Fig. 1). In 2019, blue, yellow, and orange solid-state fluorescent CDs with photoluminescence (PL) quantum yield (PLQY) of 57.9%, 34.3%, and 32.7%, respectively, were prepared by Wu's group using citric acid (CA) and (3-aminopropyl) triethoxysilane (APTES) as raw materials for covalent bond-restricted limiting aggregation quenching.22 In 2020, Shan's group utilized ionic bonding formed by sodium hydroxide (NaOH) as well as spatial confinement to effectively reduce defects and achieve an unprecedented PLQY of 75.9%.23 In 2021, Lei's group combined red CDs with polyvinyl alcohol (PVA) to prepare an excellent agricultural film with antioxidant properties, which effectively promoted photosynthesis in plants and was highly important for agricultural development.24 In 2022, Xie's group prepared high-quality white solid-state fluorescent materials exhibiting ultrabroadband random laser emission in the near-ultraviolet-visible region by in situ hybridization of silane-functionalized CDs with trimethyl 1,3,5-benzenetricarboxylic acid trimethyl ester (Et3BTC).25 In 2023, Yi's group filled graphene quantum dots and precursor solutions containing dithiothreitol (DTT) and borax into inverted opal pores to prepare multidimensional stimulus-responsive solid-state fluorescent materials responsive to pH, temperature, and angle-responsive stimuli as well as exhibiting excellent photothermal conversion.26 In recent years, the combination of CDs and matrices for realizing afterglow emission has been continuously studied. In 2019, Yu's group obtained a strong red afterglow by encapsulating CDs in a matrix capable of determining crystal field splitting with a 6-coordinate Mn center.27 In 2020, utilizing a stable rigid network and a covalent bonding effect, Zheng's group generated multiconstrained blue afterglow CDs with an ultralong lifetime of 5.72 s and a PLQY of 26.36%.28 In 2021, to further explore the relationship between CDs and matrices (covalent bonds), Li's group prepared both green thermally activated delayed fluorescent and near-infrared room temperature phosphorescent (RTP) materials by mixing CDs with cyanuric acid.29 In 2022, Bai's group successfully prepared a white RTP material with 5.8% luminescence efficiency by using urea as a matrix and modulating the synergistic interaction between the carbon core and surface states.30 In 2023, Yuan's group embedded CDs into PVA by utilizing strong hydrogen bonding interactions to achieve ultralong lifetime (2.1 s) as well as afterglow materials that can be activated by low-power white light.31
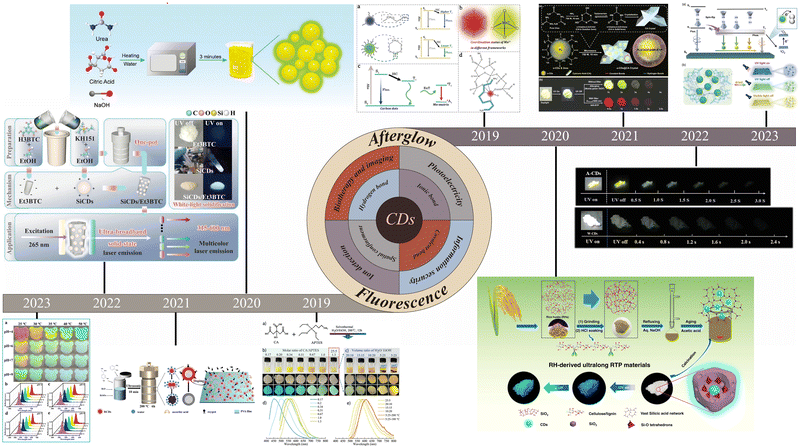 |
| Fig. 1 The development timeline of CDs in a matrix. Reproduced with permission.22–31 Copyright 2019, WILEY-VCH Verlag GmbH & Co. KGaA, Weinheim. Copyright 2020, WILEY-VCH Verlag GmbH & Co. KGaA, Weinheim. Copyright 2021, Elsevier B.V. Copyright 2022, Wiley-VCH GmbH. Copyright 2022, Elsevier B.V. Copyright 2019, Wiley-VCH Verlag GmbH & Co. KGaA, Weinheim. Copyright 2020, Springer Nature. Copyright 2021, Springer. Copyright, 2021 Wiley-VCH GmbH. Copyright 2023, Wiley-VCH GmbH. | |
In this paper, we review how the spatial confinement and synergistic interactions formed by hydrogen bonding, ionic bonding, covalent bonding, and other matrices enable the generation of solid-state fluorescence and afterglow as well as enhance performance. The applications of these technologies in optoelectronics, information security, sensing, and biorelated fields are also presented, summarized, and discussed. Finally, we propose future research directions to further improve the fluorescence and afterglow efficiencies of solid-state CDs and to explore their potential applications in a wider range of fields.
2. CDs combined with a matrix to achieve/improve solid-state fluorescence
2.1. Achieving solid-state fluorescence
CDs have attracted much attention due to their structural tunability, but excessive fluorescence resonance energy transfer or π–π stacking may lead to severe ACQ of CDs in solid-state powders or films, resulting in solid-state CDs with poor fluorescence properties or even loss of fluorescence.32–34 This problem has seriously hindered the development of solid-state CDs for various applications. One effective and simple method for achieving solid-state fluorescence is to introduce CDs into a matrix to restrict molecular motion and avoid ACQ. Different kinds of matrices can restrict the movement of molecules in different ways. The three main areas that can be addressed are hydrogen bonding, covalent bonding, and spatial restriction.
2.1.1. Hydrogen bonds. Hydrogen bonds can form strong interactions, thus restricting the movement of molecules in a matrix to some extent, which in turn affects the luminescence properties of CDs. This limiting effect is due to the properties of the hydrogen bonds themselves, which can produce a relatively stable structure of the CD molecules, thus affecting their photophysical properties. Liu's group reported high-efficiency solid-state fluorescence CDs obtained by a facile one-step microwave method.35 BA not only serves as a boron source to improve the luminescent properties of CDs but also helps to form B–OH functional groups on the surface of CDs. When the H atoms on B–OH are close to the O atoms on B–OH, O–H⋯O, i.e., hydrogen bonding, will form, which ensures that a certain distance is maintained between neighboring nanoparticles and that high-quality solid-state luminescence is achieved (Fig. 2a). The development of luminescent films has provided tremendous support for the application of solid-state fluorescent CDs. However, the preparation of high-quality luminescent films still faces a number of challenges due to the need for suitable matrix materials and complex processes. Gong's team prepared organosilane-grafted CDs (Si-CDs) using a hydrothermal method.36 Si-CDs that can be uniformly dispersed in the PVA matrix were obtained by dehydration condensation reactions and hydrogen bonding between the silicon hydroxyl group of the Si-CDs and the hydroxyl group of PVA. Based on these properties, SiCDs/PVA films with an ultrahigh CD loading (25 wt%) and high transparency can be prepared.
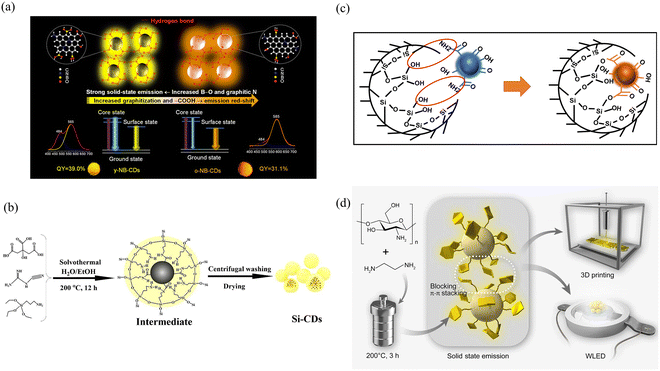 |
| Fig. 2 (a) Schematic illustration of the structure and luminescence mechanism of highly efficient solid-state emission and dual emission of y-NB-CDs and o-NB-CDs. Reproduced with permission.35 Copyright 2021, American Chemical Society. (b) Schematic illustration of the synthesis of Si-CDs by a one-step solvothermal approach. Reproduced with permission.37 Copyright 2021, Elsevier B.V. (c) Internal process of the reaction between encapsulated CDs and NPG. Reproduced with permission.38 Copyright 2021, Elsevier Inc. (d) Preparation of chitosan-based solid state CDs for WLEDs and 3D luminescent printing. Reproduced with permission.40 Copyright 2022, springer. | |
2.1.2. Covalent bonds. When CDs form covalent bonds with molecules in a matrix, the movement of the molecules is more tightly restricted due to the strength of the covalent bonds. The presence of covalent bonds causes the aggregation of CDs in solid powders or films without affecting their fluorescent properties. This phenomenon is due to the stability and persistence of covalent bonds, which allow the CDs to form a relatively stable structure in the solid matrix, thus maintaining their original fluorescent properties. Silicon dioxide is often used as a matrix for achieving solid-state fluorescence due to its stable covalent bonding, good physical properties, and thermal stability. Wang's group synthesized novel solid-state photoluminescence silicon-CDs (Si-CDs) by a solvothermal method using APTES as the source of covalent bonds.37 The Si–O–Si covalent bonding network effectively limits aggregation-induced quenching due to molecular motion and thus emits bright yellow-green fluorescence (Fig. 2b). Yang's group prepared nanoporous glass (NPG) with a silicon covalent bonding network for the first time and embedded CDs in it, successfully obtaining a solid fluorescent material (CDs-in-NPG) with a stable orange color.38 CDs are not only isolated during embedding due to the physical structure of NPG but also well dispersed due to the formation of C–O–Si functional groups. This structure not only effectively alleviates the ACQ problem associated with solid-state CDs but also enhances the luminescence intensity (Fig. 2c). In addition to solid-state fluorescence achieved by fixing CD molecules via covalent bonds, the introduction of a cross-linked covalent polymeric network structure is an efficient method for realizing long wavelength solid-state fluorescence. Qu's group designed a novel type of red/near-infrared-emitting CDs (P-CDs) containing branched polyethyleneimine (b-PEI) with a large number of –NH3 surface functional groups.33 This tactic results in a homogeneous surface state of the P-CDs and prevents the direct connection of neighboring carbon cores, which significantly dampens the nonradiative relaxation caused by the coupled surface states and the π–π interactions between adjacent P-CDs.
2.1.3. Spatial confinement. When CDs are embedded in a matrix, the structure of the matrix restricts the space around the CDs so that the CD molecules are unable to move freely, thus realizing solid-state luminescence. This constraining effect is due to the wrapping effect of the matrix on the CDs as well as the structural properties of the matrix itself, which together restrict the movement of the CD molecules, leading to their luminescent behavior under solid-state conditions. This spatial confinement can be realized by appropriate matrix design, which affects the optical properties of CDs and is a simple and efficient method. Starch, as a biocompatible solid matrix, is considered one of the candidates for realizing solid-state fluorescence due to its simplicity and excellent results. Hu's group successfully prepared multicolored solid fluorescent materials by mixing the prepared CDs with starch and stirring.39 With the CDs confined by starch, the quenching phenomenon caused by aggregation was successfully suppressed. Biomass materials have become one of the matrices for realizing solid-state fluorescence in recent years due to their abundance, easy degradation and excellent restriction properties. Using chitosan and ethylenediamine as raw materials, Ni's group successfully prepared chitosan-based solid CDs (C-SS-CDs) with yellow fluorescence and an ultrahigh PLQY by a one-step hydrothermal method.40 Various experiments have demonstrated that C-SS-CDs retain the basic backbone structure of chitosan and are self-limiting, allowing them to effectively prevent π–π stacking and thus inhibit fluorescence quenching (Fig. 2d). Polymers such as polystyrene (PS), for example, are well suited for the preparation of carbon-dotted solid films due to their low cost, ease of processing, and high transparency. Shan's group prepared ultrahigh-performance yellow luminescent transparent films by mixing pyrrole nitrogen-doped CDs with a PS matrix.41 By dispersing the CDs into the PS matrix, the quenching phenomenon generated by aggregation was avoided. The solid CDs@PS composite film maintained a high PLQY of 84% and a large Stokes shift of 73 nm at 0.05 wt%.Spatial confinement is a simple way to achieve solid-state fluorescence, and many matrices, such as polymers and biomass, among other materials, have been widely used to effectively suppress the fluorescence quenching effect by restricting the molecular motion of CDs. The details are shown in Table 1.
Table 1 Solid-state fluorescence through spatial confinement
|
Precursor |
Matrix |
Color |
Ref. |
1 |
Absolute ethanol |
Epoxy |
Blue, green, red, and white |
42 |
2 |
o-Phenylenediamine (o-PDA) |
Polyvinylpyrrolidone (PVP, K10eK14) |
Red |
43 |
3 |
o-PDA + KCl |
PVA |
Blue, green, yellow, and red |
44 |
4 |
CA + nile blue (NBA) |
PVP |
Blue, yellow, and red |
45 |
5 |
CA + pyrocatechol + o-PDA |
PVP |
Blue |
46 |
6 |
1-Amino-2-naphthol hydrochloride + citric acid CA |
OE-6550A/B silica gel |
White |
47 |
7 |
Polyethylene terephthalate (PET) + pyromellitic anhydride + urea |
Polymethylmethacrylate (PMMA) |
Yellow and white |
48 |
8 |
CA + thiourea |
Epoxy |
Red and white |
49 |
9 |
CA + ethylenediamine (EDA) |
PMMA |
White |
50 |
10 |
Gum tragacanth polysaccharide + triethylenetetramine (TETA) |
Bisphenol A (DGEBA) epoxy resin |
Blue |
51 |
11 |
1,4,7,10-Tetraazacyclododecane + CA |
Silicone |
White |
52 |
12 |
CA + cyanamide |
Polyurethane (TPU) |
White |
53 |
13 |
o-PDA + semicarbazide |
PVA |
White |
54 |
14 |
Urea + phloroglucinol (PG) |
OE6250 A |
Blue, green, yellow, red, and white |
55 |
15 |
Banana peel |
PMMA |
Yellow |
56 |
16 |
Ripe banana peel |
NaCl |
White |
57 |
17 |
o-PDA |
PMMA/silica gel |
Full-color |
58 |
18 |
1,3,5-Benzenetricarboxylic acid + diethylenetriamine/m-phenylenediamine (m-PDA)/o-PDA/p-phenylenediamine (p-PDA) |
Polydimethylsiloxane |
Blue, green, red, and white |
59 |
19 |
o-PDA + ethylorthosilicate |
Starch/hydrogel |
Yellow-green |
60 |
20 |
2,7-Dihydroxynaphthalene + CA |
N-b-Aminoethyl-g-aminopropyl trimethoxysilane |
White |
61 |
2.2. Improving solid-state fluorescence performance
2.2.1. Improving the solid-state fluorescence properties. In the process of realizing solid-state fluorescence, it is very important to correctly select the matching matrix; otherwise, the luminescence properties of the CDs will be seriously affected, resulting in solid-state fluorescence performance. Different matrices have different embedding and limiting effects on CDs, so it is necessary to select the best matrix for specific application scenarios and conduct sufficient experimental verification to ensure the stability and reliability of solid-state fluorescence performance.The PLQY is one of the most important properties of solid-state fluorescence, so improving it has become a developmental challenge. Cao's group achieved bright red solid-state fluorescence by mixing CDs with cellulose films, successfully increasing the PLQY by more than fourfold.62 Characterization revealed that the abundance of oxygen-containing functional groups on the surface of the pristine CDs led to the creation of nonradiative defects, under which the energy was excessively large, resulting in a reduced specific gravity of the radiative transitions and low fluorescence efficiency. When the CDs were combined with cellulose, the abundant hydroxyl groups in cellulose were utilized to react with the oxygen-containing groups on the surface of the CDs and establish a stable chemical bond, which not only eliminated the fluorescence inefficiency caused by the nonradiative recombination of local electron–hole pairs but also restricted the molecular motion and achieved enhanced red solid-state fluorescence (Fig. 3a). In addition to the modulation of the optical properties through the formation of chemical bonds, the modulation of solid-state fluorescence properties through external factors (e.g., temperature and pressure) is a novel approach. This approach provides a more flexible and controllable way to regulate the solid-state optical properties of CDs. Shan's group demonstrated for the first time the realization of piezochromic luminescent materials (CDs@NaOH) by encapsulating CDs in a NaOH matrix.63 Importantly, under low pressure, the distance between the NaOH and the CDs decreases, and the spatial confinement of the NaOH can effectively limit the defects and π–π stacking interactions between the CDs, thereby enhancing the fluorescence intensity (1.6 times) and increasing the PLQY, leading to an increase in fluorescence upon increasing pressure from 1.0 atm to 1.4 GPa (Fig. 3b). However, by further compression, the distance between the embedded CDs becomes shorter, disrupting their conjugated structure and thereby leading to a decrease in fluorescence intensity. When the pressure decreased from 20.0 to 0 GPa, the recovered CDs@NaOH samples showed significantly enhanced fluorescence compared to the initial value.
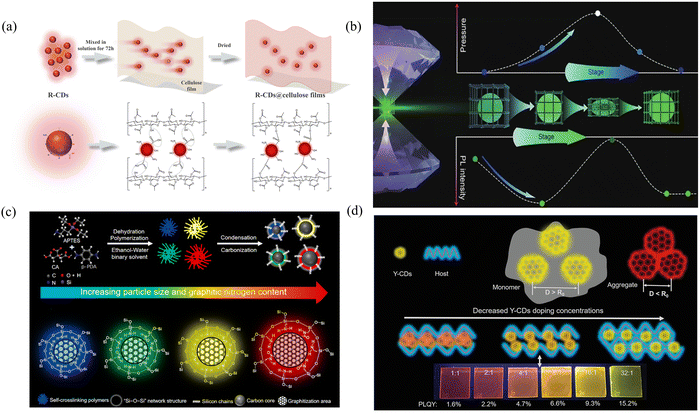 |
| Fig. 3 (a) Schematic diagram of possible chemical bonding and fluorescence mechanism between R-CDs and cellulose films. Reproduced with permission.62 Copyright 2022, American Chemical Society. (b) Schematic illustration of the possible mechanism of fluorescence enhancement and retainability in the CDs@NaOH under pressure. Reproduced with permission.63 Copyright 2022, springer. (c) Schematic illustration of the four selected SiCDs for their formation mechanism and PL mechanism. Reproduced with permission.66 Copyright 2022, Elsevier Ltd. (d) Schematic of host/guest doping systems (PVK/Y-CDs and TFB/Y-CDs). Insets are the corresponding photographs of PVK/Y-CDs thin films under UV light irradiation. Reproduced with permission.69 Copyright 2021, Wiley-VCH GmbH. | |
Stability is an important property of solid-state fluorescent CDs and is highly important for their application. Therefore, how to minimize the influence of external factors and achieve longer luminescence performance has become a hot research topic. Yoon's group polymerized CDs with epoxy monomers to prepare a solid-state luminescent material (CDs/epoxy) with an ultralong stability of more than 80% luminescence intensity after eight weeks of storage under ambient conditions.64 The introduced epoxy monomers can react with amino nitrogen and pyridine nitrogen on the surface of the CDs and cause curing, which restricts the motion of the CDs and inhibits fluorescence quenching through nonradiative transitions and π–π stacking effects. In addition, the epoxy monomer can effectively passivate the surface of the CDs and provide an additional protective layer, thereby enhancing the stability of the CDs. By combining with CDs, resin monomers can increase the stability of CDs, extending their application lifetime in different environments.
2.2.2. Achieving multicolor solid-state fluorescence. Conventional solid-state fluorescent CDs usually emit only a single color and cannot meet the needs of multiple applications. Therefore, one-step synthesis of multicolor solid-state fluorescent CDs has become a popular research topic.Incorporating matrices into synthetic materials and changing the degree of conjugation or the type and number of functional groups of CDs under the protection of the matrix to obtain different energy level structures and thus produce different solid-state fluorescence are among the more common methods used in the present study. Zhan's group prepared solid fluorescent CDs from blue to green by using 3-ureidopropyltriethoxysilane (UPTES) with L-(−)-malic acid and controlling the volume ratio of water to ethanol.65 The Si–O–Si network structure wrapped around the CDs prevents the fluorescence quenching phenomenon caused by molecular motion, whereas the different hydrolysis and condensation degrees of UPTES due to the different volume ratios of water and ethanol lead to different sizes and thus different multicolor solid-state fluorescence. In addition to changing the particle size to tune the solid-state fluorescence wavelength, changing the degree of conjugation and graphitization is also an efficient method. Ding's group synthesized solid-state fluorescent CDs with tunable colors in the presence of APTES by the microwave heating method, changing only the molar ratio of CA and p-PDA.66 Different ratios of CA and p-PDA result in different conjugation domains and graphitization degrees. Graphite N could not only afford off-domain electrons to increase the probability of radiative processes but also enhance the rigidity of aromatic domains to reduce the probability of nonradiative processes (i.e., molecular vibrations). Under the protection of a silicon network (Si–O–Si), the amount of sp2-conjugated structural domains and graphitization increase, and panchromatic solid-state fluorescence in the visible region at 438–633 nm is achieved (Fig. 3c).
The selection of suitable matrix materials and reasonable concentrations in the matrix for solid-state multicolor fluorescence emission through aggregation-induced and Förster resonance energy transfer (FRET) is also an effective method. In a solid-state environment, due to the interaction between the CDs and the matrix, the CD molecules tend to aggregate, where multiple CDs are tightly packed in the matrix. This aggregated state can limit electron transitions between CDs, thus changing their luminescent properties. Moreover, under certain conditions, energy transfer between CDs and the matrix can be realized by FRET, in which the matrix acts as a protector or receiver, absorbing the energy emitted by the CDs and reradiating the luminescence. In Yan's group, green, yellow and orange-red solid fluorescent CDs were synthesized by a solvothermal method using maleic acid (MA) and APTES as the raw materials.67 The presence of Si–C, Si–O and Si–N on the surface of the CDs increases the distance between neighboring CDs, causes direct contact between the luminescent centers and attenuates the direct π–π interactions and excessive FRET between them, thus generating fluorescence in the solid state. By adjusting the APTES/MA ratio, the size and degree of aggregation of the particles change, and the luminescent centers change within the Förster distance (R0), which leads to wavelength changes. Zhan's group prepared solid-state fluorescence materials ranging from blue to orange by controlling the aggregation state between CDs by controlling the ratio of urea to CA and the ratio of APTES to CA.68 The dehydration process between CA and urea occurs to form CDs, while APTES further hydrolyzes and condenses to form polysiloxanes, which begin to encapsulate CDs. Different ratios of CA and urea lead to different chemical behaviors and different degrees of aggregation of CDs of different colors. Polymers have a high degree of transparency and are therefore particularly suitable candidates for the preparation of multicolored films. Chen's group blended yellow CDs (Y-CDs) with a high degree of graphitization with polyvinyl carbazole (PVK) and controlled the ratio between the polymer and the Y-CDs to achieve luminescent films ranging from yellow-green to red.69 Self-sudden extinction is not observed when the Y-CDs are in the sparse solution state (monodisperse state), where the distance between each Y-CD is greater than R0. When the Y-CDs are in the aggregated state (with a distance less than R0), the π-conjugated structural domains overlap each other. The expanded conjugated π-electron system depletes the transition energy, leading to fluorescence burn-off. The introduction of a PVK matrix can spatially separate the Y-CDs and inhibit direct contact. As the doping concentration of the Y-CDs continues to decrease, the distance between the Y-CDs increases, and the degree of aggregation decreases, causing a gradual blueshift of the emission spectrum (Fig. 3d).
3. CDs combined with the matrix to realize/improve afterglow
The main processes that generate afterglow can be divided into two stages: (1) the radiation transition from the lowest singlet state (S1) to the triplet state (T1) through the ISC process and (2) the radiation transition when returning from T1 to the ground state (S0). However, these processes are susceptible to nonradiative transitions induced by internal vibrations and rotations of the molecules, as well as interference from the external environment (e.g., water and oxygen), resulting in the weakening or loss of afterglow properties. The introduction of a matrix can play a key role in overcoming these problems.70,71 Hydrogen bonding, ionic bonding, covalent bonding, or other multiple interactions present in the matrix can effectively inhibit nonradiative transitions, lead to the formation of a stable microenvironment, and prevent the influence of the external environment on afterglow, thereby realizing the generation of afterglow and improving performance.
3.1. Achieving afterglow
3.1.1. Hydrogen bonds. Hydrogen bonding has a strong bonding energy and directionality that allows for the formation of stable structures in molecules. By introducing hydrogen bonds, the position and energy level distribution of excitons can be modulated, which helps to optimize the process of energy transfer. Therefore, the introduction of hydrogen bonds to restrict molecular motion and protect triplet excitons is one of the more common methods for realizing afterglow. Zhao's group prepared three different colors of CDs by a hydrothermal method using glutathione, m-PDA and p-PDA as raw materials and dispersed the CDs into sodium alginate (SA) to produce multicolored afterglow materials.72 The abundant functional groups on the surface of the CDs form a large number of hydrogen bonds with SA, which enhances the rigidity of the whole system, restricts molecular motion, inhibits nonradiative transitions, and results in a stable triplet state. Environmental factors such as water and oxygen can easily deplete triplet excitons and quench afterglow. The existence of a hydrogen bonding network can overcome the above problems and realize afterglow emission in aqueous environments. Yang's group synthesized CDs using methotrexate as a raw material and subsequently introduced them into cyanuric acid in a water environment, successfully generating a hydrogen bond-mediated complex (CA-CDs) that produced afterglow in water.73 A hydrogen bonding network bridge structure is formed between the CDs and the binding water as well as cyanuric acid. This structure can enhance the stability of the whole system by inhibiting molecular motion and stabilizing the C
O bond. In addition, due to the rigid structure of cyanuric acid, the CDs can be effectively protected by dissolved oxygen in water, thus avoiding the quenching effect of oxygen on the CDs (Fig. 4a). Eventually, the CA-CDs, which emit cyan phosphorescence in a room temperature aqueous solution and have a PLQY of 23%, were successfully activated.
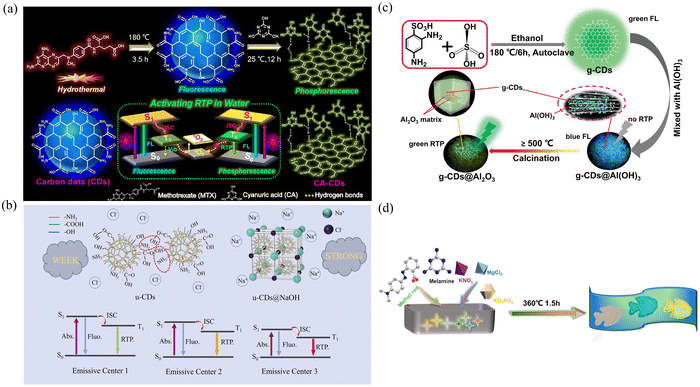 |
| Fig. 4 (a) Synthesis of fluorescent CDs and phosphorescent CA-CDs and the mechanism of activating RTP in water. Reproduced with permission.73 Copyright 2021, Elsevier B.V. (b) The proposed mechanism for the enhanced multicolor RTP emission of u-CDs@NaOH. Reproduced with permission.75 Copyright 2023, Elsevier B.V. (c) Flow chart illustrating the synthesis of g-CDs@Al(OH)3 and g-CDs@Al2O3 composites. Reproduced with permission.77 Copyright 2023, Wiley-VCH GmbH. (d) Schematic diagram of the synthesis of CD-based composite materials. Reproduced with permission.81 Copyright 2023, American Chemical Society. | |
One way to achieve thermally activated delayed fluorescence (TADF) is the formation of hydrogen bonds between the matrix and CDs. Lu's group combined CDs, montmorillonite (MMT) and PVA to prepare green-TADF composite films (CDs-MMT@PVA) with a high PLQY (45.66%) and an ultralong lifetime (30.23 ms).74 The electrostatic attraction and confined space of MMT restrict the movement of CDs to avoid aggregation-caused quenching. At the same time, hydrogen bonds were formed between PVA and CDs-MMT to form a rigid structure with strong interactions, which reduced the non-radiative decay of the triplet excitons and thus activated the TADF of CDs-MMT@PVA films.
3.1.2. Ionic bonds. Compared to hydrogen bonds, ionic bonds are more stable and more sensitive to temperature changes. Thus, ionic bonding has particular applicability in realizing afterglow and stimulating matrices for responsive afterglow materials. Hu's group prepared seeded CDs with CA and urea and modified the seeded CDs with urea, hydrochloric acid (HCl) and NaOH, successfully preparing the multicolor tunable CD materials u-CDs and u-CDs@NaOH.75 For the powder u-CDs, a weak room temperature phosphorescence (RTP) phenomenon was observed. This phenomenon is due to intramolecular hydrogen bonding that inhibits the motion of the molecule, thus stabilizing the triplet exciton. However, due to the relatively weak strength of the hydrogen bond, its ability to stabilize triplet states is limited. In contrast, for the powder u-CDs@NaOH, NaOH was added to the u-CDs. NaOH was used to stabilize the triplet state. The carboxyl groups on the surface of the CDs can effectively ionize with NaOH and create ionic bonds. These ionic bonds produce a stronger rigid network environment that suppresses nonradiative transitions and stabilizes triplet-state excitons, which in turn increases the intensity of the RTP (Fig. 4b).At present, realizing the thermally enhanced, long-life and long-wavelength luminescence of CD-based TADF materials is still a great challenge. Moreover, most TADFs are generated mainly by hydrogen bonding, and their performance currently has difficulty meeting demand. Hu's group introduced CD composites containing hydrogen and covalent bonds into the ionic crystal network and achieved a 250% thermal enhancement from 273 K to 343 K, as well as ultralong-lifetime (109.6 ms) red TADF-emitting (600 nm) CD materials (uCDs@SiO2/NaOH, u-urea).76 In this uCDs@SiO2 system, urea introduces a large number of functional groups (such as –NH2 and C
O/C
N) contributing to (n, π*) that promote ISC, and the covalent and hydrogen bonds formed between the CDs and the matrix inhibit nonradiative transitions, thus promoting delayed fluorescence emission. However, the rate of nonradiative deactivation in this system is too fast, making it difficult to observe the TADF effect. Therefore, after the addition of NaOH, ionic bonds and ionic crystals were introduced to construct a rigid network structure. Under the combined effect of the three chemical forces, the nonradiative transitions were suppressed more effectively, resulting in long-wavelength TADF emission.
3.1.3. Covalent bonds. Compared to hydrogen and ionic bonds, covalent bonds are much stronger and provide greater advantages in terms of the immobilization and stability of luminescent substances. Covalent bonds are formed through the sharing of electrons and are able to firmly connect the luminescent substance to the matrix, which prevents it from destabilizing or breaking during the afterglow process. Covalent bonds are also highly oriented and capable of ordering and stabilizing the internal structure of molecules. This directionality controls the spatial arrangement and intermolecular interactions of luminescent substances, thus affecting their optical properties and afterglow efficiency. This strong connectivity ensures longer luminescence lifetimes and higher PLQY of the luminescent substances. Zhang's group reported the synthesis of a composite material (g-CDs@Al2O3) obtained by mixing and calcining CDs and aluminum hydroxide (Al(OH)3).77 During calcination, Al(OH)3 forms Al2O3 with a compact structure and forms covalent bonds with CDs. Under the effect of covalent bonding, molecular motion and nonradiative transitions are suppressed, and the triplet state is enhanced. G-CDs@Al2O3 emits phosphorescence in water and has good phosphorescence properties under harsh acidic and alkaline conditions (Fig. 4c). The introduction of covalent bonds is an effective way to achieve excellent performance afterglow. Fang's group prepared green RTP CDs with an ultralong lifetime (905.5 ms) and duration (8 s) by a hydrothermal reaction of a carbon source and BA.78 This superior performance occurred because covalent bond (B–O–B) formation successfully restricted the vibration and rotation of the CDs, thus suppressing the nonradiative transitions. In addition, the boron oxide (B2O3) matrix formed by BA is able to effectively protect triplet excitons.The covalently bonded matrix is able to form a homogeneous and tightly packed structure that provides good electron and energy transport channels and helps to prevent triplet excitons from being subjected to nonradiative transitions. Yuan's group prepared composites with dark blue and blue-green TADF properties by microwave heating two different CDs with urea.79 During the dehydration carbonization process, covalent bonds were formed between the CDs and the urea and were encapsulated in g-C3N4. The covalent bond that formed successfully protected the triplet state and promoted ISC, producing a smaller ΔEST and promoting RISC.
3.1.4. Spatial confinement. Spatial confinement using inorganic matrices to suppress molecular motion and nonradiative transitions and protect triplet excitons is an efficient way to realize afterglow. Inorganic matrices have highly ordered structures and are stable and inhibit molecular motion and nonradiative transitions, thereby protecting triplet excitons in afterglow materials. Song's group proposed an efficient molten salt method for the preparation of CD-based RTP materials (CDs@MP) by calcination using inorganic salts as a matrix.80 During the synthesis process, rigid crystalline salt shells can maintain the triplet state of CDs@MP in water and inhibit nonradiative inactivation. In addition, the aggregation of the CDs@MP nanocomposites caused the matrix shells to self-assemble into a network, which further enhanced the rigidity of the shells and prevented intermolecular motion, thus prolonging the RTP lifetime. The realization of afterglow emission in water is an important area for expanding applications in biology and other fields. Because hydrogen bonding easily occurs in water, making it difficult to realize the afterglow effect, the selection of suitable matrices has become a challenge. Inorganic salt matrices are capable of forming rigid networks that insulate water molecules and have attracted much attention in the field. By utilizing an inorganic salt matrix, excitons can be effectively protected, and efficient afterglow emission in water can be achieved, which provides new possibilities for applications in related fields. Zhao's group prepared CDs that emit afterglow in water by using magnesium chloride and potassium nitrate as dopant salts to form a salt shell and potassium nitrate as a salt medium to promote the reaction and by changing the ratio of the precursor melamine.81 The salt shell is composed of magnesium salts with high charge density and has a rigid structure that suppresses the nonradiative transitions of triplet excitons while insulating water molecules (Fig. 4d).As water molecules can easily lead to the bursting of triplet excitons, the afterglow performance of CDs in water is poor, which severely limits the expansion of their applications in the field of bioimaging, among others. By encapsulating CDs in a matrix, the CDs were effectively isolated from an aqueous environment and efficient afterglow emission in water was achieved.82 This approach not only improves the performance of CDs in water, but also provides broader possibilities for their application in aqueous environments. Lou's group successfully constructed near-infrared afterglow-emitting CDs using a photooxidation-induced strategy, which effectively avoided the oxygen burst problem.83 They obtained P-CDs by modifying the surface of the CDs with an amphiphilic block polymer, Pluronic F127, which not only isolated the CDs from the aqueous environment but also slowed the generation of reactive oxygen species and reduced the oxidation rate of the CDs. Ultimately, the luminescence time was prolonged by the transfer of chemical energy to luminescent energy. After the coating treatment, these CDs exhibit an afterglow lifetime of up to 5.9 hours in aqueous solution, which is the highest lifetime of any currently available organic, inorganic or carbon dot-based afterglow emission system.
As shown in Table 2, utilizing the spatial confinement of the matrix as well as chemical forces to achieve efficient afterglow emission from CDs is a relatively efficient and common approach.
Table 2 Afterglow emission through chemical forcing and spatial confinement
|
Precursor |
Matrix |
Chemical bond/forces |
Type |
Emission (nm) |
Color |
Lifetime (ms) |
Ref. |
1 |
Phosphoric acid (PA) + BA + Ethylenediamine (EDA) |
TEOS |
Hydrogen bond |
RTP |
502 |
Green |
1970 |
84 |
2 |
α-Lipoic acid + EDA |
AlPO4-5 Zeolite |
Spatial confinement |
RTP |
516 |
Green |
1400 |
85 |
3 |
Pyridine-2-boronic acid + EDA |
BA |
Spatial confinement |
RTP |
490 |
Green |
539 |
86 |
4 |
Glycine + KNO3 + MgCl2 + NaH2PO4 |
Insoluble phosphate (IP) |
Covalent bond |
RTP |
482, 518 |
Cyan and green |
2010, 1150 |
87 |
5 |
Melamine (MA) + L-cystine |
MA/isophthalic acid |
Hydrogen bond |
RTP |
|
Orang + yellow + green |
|
88 |
6 |
Diethanolamine + PA |
TEOS |
Hydrogen bond + covalent bond |
RTP |
506 |
Green |
1353.9 |
89 |
7 |
P/M/o-PDA |
Urea |
Covalent bond |
RTP |
431–620 |
Full color |
310–2020 |
90 |
8 |
CA + Urea |
BA |
Spatial confinement |
RTP |
503 |
Green |
|
91 |
9 |
TEOS |
TEOS |
Hydrogen bond + covalent bond |
RTP |
505 |
Green |
2190 |
92 |
10 |
Arginine + BA |
BA |
Hydrogen bond + covalent bond |
RTP |
462–623 |
Full color |
492–1106 |
93 |
11 |
Chitosan |
PVA |
Hydrogen bond |
RTP |
|
Green |
200 |
94 |
12 |
L-lysine |
ZnAl2O4 |
Spatial confinement |
RTP |
400, 540 |
Yellow, red |
410, 1050 |
95 |
13 |
Lycorine hydrochloride + BA |
BA |
Covalent bond |
RTP |
520 |
Green |
1760 |
96 |
14 |
5-Amino-1,10-phenanthroline (Aphen) + PVA |
PVA |
Hydrogen bond |
RTP |
518 |
Yellow-green |
103 |
97 |
15 |
Chitosan + NaOH |
PVA |
Hydrogen bond |
RTP |
502 |
Green |
623 |
98 |
16 |
1,3-Bis(3-aminopropyl)-1,1,3,3-tetramethyldisiloxane |
TEOS |
Covalent bond |
DF |
440 |
Blue |
434 |
99 |
17 |
CA + EDA |
Urea |
Hydrogen bond |
RTP |
570, 610 |
Yellow-green + orange |
381, 91 |
100 |
18 |
p-Aminobenzonitrile + metronidazole + PA |
Dicyandiamide |
Hydrogen bond + spatial confinement |
RTP |
511–518 |
Yellow-green |
590–1310 |
101 |
19 |
MA + BA |
BA |
Hydrogen bond + covalent bond |
RTP |
450 |
Green |
1700 |
102 |
20 |
CA + urea/4-aminobenzoic acid |
PVA |
Hydrogen bond |
RTP |
422 |
Blue |
338.4 |
103 |
21 |
ATTES + L-asparaginic acid |
ATTES |
Covalent bond |
RTP |
|
Green |
1300 |
104 |
22 |
Glucose + TEOS |
TEOS |
Covalent bond |
RTP |
460 |
Blue |
1330 |
105 |
23 |
Metformin + PA |
PVA |
Hydrogen bond |
RTP |
496 |
Green |
613.03 |
106 |
3.2. Improved afterglow performance
CDs exhibit different properties when combined with different types of substrates. For instance, the addition of matrices inhibits the occurrence of nonradiative transitions and enhances the lifetime and PLQY of afterglow materials. Hydrogen bonding is weak and reversible in nature and consequently plays a role in realizing the reversibility of afterglow. On the other hand, ionic bonding, which is connected by mutual gravitational forces between positive and negative charges, can change the charge density, thus changing the nature of the afterglow material or giving rise to new properties. Therefore, the rational use of matrices or the combination of multiple types of different matrices is an effective way to improve afterglow properties and to confer new characteristics. This strategy can help to modulate the optical and chemical properties of CDs, increase their adaptability to different application scenarios, and provide a foundation for performance enhancement and innovation of afterglow materials.
3.2.1. Lifetime and PLQY. The introduction of hydrogen or covalent bonds is an effective way to stabilize triplet excitons, suppress nonradiative transitions, and increase the afterglow PLQY and lifetime.107 Triple-state excitons are prone to nonradiative transitions, losing energy and converting it to heat. However, by introducing strong hydrogen or covalent bonds, persistent hydrogen or covalent interactions can be formed within a molecule, which effectively suppresses nonradiative transitions and stabilizes triplet excitons, resulting in improved PLQY and lifetime. Guo's group proposed a strategy to synthesize and prepare polymer CDs (Arg-CPDs) from arginine (Arg) and PA and introduced them into a SiO2 network (Arg-CPDs@SiO2) to substantially increase the lifetime to 543 ms which is more than 3 times greater than that (149 ms) of matrix-free Arg-CPD materials.108 The SiO2 network provides important safeguards for extending the RTP lifetime of Arg-CPDs@SiO2. The formation of a SiO2 network and covalent bonds (C–Si) effectively restricts the vibrations and rotations of C
O/C
N groups, increases their rigidity, promotes the effective filling of ISC with triplet excitons, and suppresses the nonradiative excitations of triplet excitons. Moreover, Arg-CPDs@SiO2 has excellent lifetime properties in both the solid phase and aqueous solution because the protection of the SiO2 network prevents the quenching of light-emitting groups by the external environment. Chen's group successfully developed a versatile strategy for the fabrication of long afterglow materials by embedding biomass-derived CDs into cellulose fibers.109 The formation of intramolecular hydrogen bonds between cellulose and CDs strongly protects against confinement by the triplet exciton, which results in an increase of seven orders of magnitude in the afterglow lifetime (Fig. 5a). Choosing the right size matrix and the degree of cross-linking with the carbon point material is also a way to improve performance. Lu's group prepared CDs with weak afterglow (PLQY = 21.23%, lifetime = 0.13 s) by self-crosslinking and precursor carbonization and formed CDs@PVA (PLQY = 55.49%, lifetime = 0.75 s), CDs@PAM, and CDs@TEOS composites with improved afterglow properties by embedding them in PVA, PAM and TEOS, respectively.110 PVA, PAM, and TEOS are rich in functional groups, such as amino, hydroxyl, and ethoxyl groups, which can improve the spin–orbit coupling from the singlet to the triplet excited states of CDs, resulting in the generation of triplet excitons. The spin–orbit coupling effect of CDs can be enhanced by bonding between the surface groups of CDs surrounding the polymer shell, which can extend the cross-linking network and inhibit the nonradiative decay of the excited state. The hydroxyl group on PVA is bonded to the carboxyl group on the CDs, leaving no additional groups to rotate, thus effectively restricting the spatial structure of the CDs. In contrast, the –CH2– group in the PAM chain allows rotation, so it restricts the motion of CDs relatively little. In addition, TEOS did not directly bind to CDs during the wetting process, so it did not form a cross-linked structure.
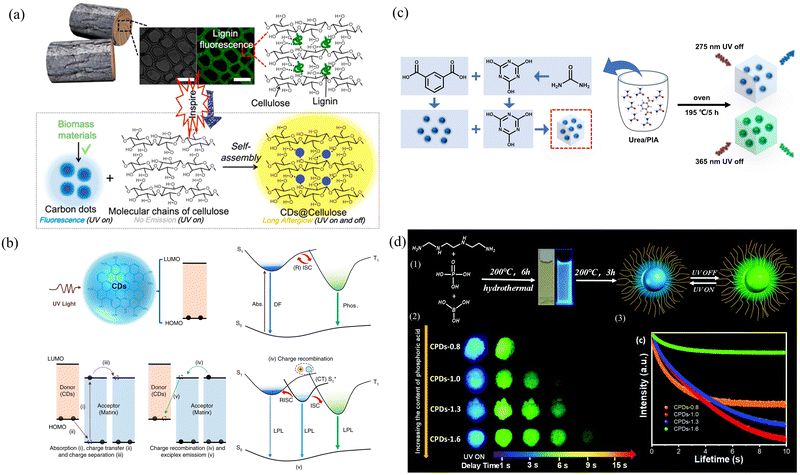 |
| Fig. 5 (a) Design of wood-inspired long afterglow material by embedding CDs into cellulose fibrils. Reproduced with permission.109 Copyright 2020, Elsevier Ltd. (b) Possible mechanisms for achieving long-lived luminescence from CDs. Reproduced with permission.111 Copyright 2022, Springer Nature SharedIt content-sharing initiative. (c) Schematic illustration of the CDs@CA preparation process. Reproduced with permission.112 Copyright 2023, Elsevier B.V. (d) (1) Schematic diagram showing the preparation of CPDs. (2) Phosphorescence of CPD powders. (3) Time-resolved RTP spectra showing the emission peak at 530–532 nm. Reproduced with permission.113 Copyright 2021, Royal Society of Chemistry. | |
To realize ultralong-lifetime afterglow CDs, the realization of efficient charge-separated (CS) states is a viable pathway. However, this process is susceptible to quenching by oxygen and water molecules. Excitons (electron–hole pairs) typically dissipate energy in a nonradiative manner, resulting in short-lived luminescence. However, by designing systems with efficient charge separation, excitons can be separated into separate carriers (electrons and holes), thereby extending the lifetime of the afterglow. However, the use of matrices as a platform for charge separation has been successful. Lin's group presented the first strategy for sustained afterglow emission of more than one hour using m-PDA and cyanuric acid (CA) as raw materials with covalent bonds to limit CDs (m-CDs, m-CDs@CA).111 The m-CDs and cyanuric acid effectively stabilized the triplet state of the m-CDs through the formation of strong covalent bonds, while the crystallized CA was also able to protect the excited state of the m-CDs from quenching by molecular oxygen. The origin of the long-lived fluorescence (LPL) in mCDs@CA was related to the reorganization of the CS state of the heterodimer generated by the m-CDs and CA under UV irradiation (Fig. 5b). When m-CDs and CA are in the CS state under UV irradiation, the donor radical cations (m-CDs) diffuse and segregate toward the acceptor radical anions to form the CS state, in which some of the charge-transferred states of the isomers dissociate into charge-separated states. This charge-separated state can persist for a long time because the separated charge carriers slowly combine, resulting in LPL that can persist for more than two hours. The construction of binary donor–acceptor exciplex systems using CDs has been shown to be an effective method for obtaining organic long persistent luminescence (OLPL). On this basis, Liu's group used isophthalic acid and urea to synthesize color-tunable ultralong afterglow materials (CDs@CA) in one step.112 Varying the degree of separation of the CS states in turn induces a change in the LPL duration (Fig. 5c). The tunability of the LPL color is due to the presence of multiple emission centers in CDs@CA, where certain emission centers dominate the afterglow emission as the excitation wavelength varies, resulting in a different color for the LPL emission.
The confinement effect of the rigid aggregation-induced structure facilitates the blockage of intermolecular motion for efficient ISC and produces long-lived afterglow emission. Wang's group prepared four carbonized polymer CDs (CPDs-0.8, 1.0, 1.3, and 1.6) using a hydrothermal method by varying the ratio of precursors with lifetimes of 0.87 s, 1.31 s, 1.47 s, and 2.43 s, respectively.113 As the PA content increases, compounds containing hydroxyl and PA groups are generated; these compounds can interact with other polymer chains containing hydroxyl and PA groups by hydrogen bonding to form a stable polymeric network backbone (Fig. 5d). In this polymerization network backbone, the CPDs and polymer chains are entangled to form a stable structure that prevents intermolecular movement and enhances ISC. Due to the formation of the CPD polymerization network, the rigidity of the system is improved, which enables the system to maintain the photoexcited state for a longer period of time and extends the lifetime of the afterglow. In addition to its protective role, the matrix binds its own groups to the CDs and promotes ISC, thus increasing the lifetime and PLQY. Bai's group achieved the highest PLQY (50.17%) and longest lifetime (2.03 s) by dissolving and wrapping CDs in urea via the hot-melt method.114 In addition to forming a stable space structure, urea introduces a large number of functional groups in the (n, π*) configuration to the CDs, which can induce strong spin–orbit coupling and greatly increase the ISC rate.
3.2.2. Realization of functional afterglow.
Time-dependent phosphorescence. CDs based on materials with time-dependent phosphorescence color (TDPC) can provide additional time dimensions for higher-level dynamic encryption.115 Combining two or more phosphorescent centers with different colors and decay rates in a single material triggers the TDPC phenomenon. This approach is based on the properties of the phosphorescent centers in the material, which produce different emission spectra under different excitation conditions. This dynamic encryption based on TDPC provides an additional time dimension that enhances the complexity and security of encryption. However, the realization of CD-based TDPCs remains a major challenge. The selection of a suitable matrix and rational chemical bonding or forces are potential ways to realize TDPCs.In general, the excitons generated by CDs located under a single structural constraint have the same transfer path and produce a single emission color.116 However, on the basis of structural constraints, the introduction of another method to open up a new exciton transfer path can realize TDPC emission.117 Lu's group proposed a yellow-to-green TDPC emission strategy by introducing CDs into a structural confinement consisting of NaCl followed by magnesium chloride (MgCl2) as a structural defect.118 Structural constraints activate green phosphorescence from CDs, whereas structural defects activate tunneling-associated yellow phosphorescence. The fact that yellow RTP emits earlier than green RTP is strong evidence that structural defects in the inorganic matrix bind excitons much more weakly than structural constraints. Under structural confinement, the nonradiative transitions are suppressed, and the exciton passes through the ISC and returns from T1 to S0, where it undergoes a radiative transition and produces green phosphorescence. After Mg2+ doping, the number of Na+ lattice defects increases (Fig. 6a). Since the charge of Mg2+ is greater than that of Na+ in the pristine NaCl matrix, the resulting crystal will have a localized excess of positive charge. To maintain the electroneutrality of the whole crystal, Na+ vacancies (VNa′) appear. VNa′ acts as a trap center to stabilize and store triplet excitons, accelerating the radiolysis process and generating yellow phosphorescence through reverse tunneling.
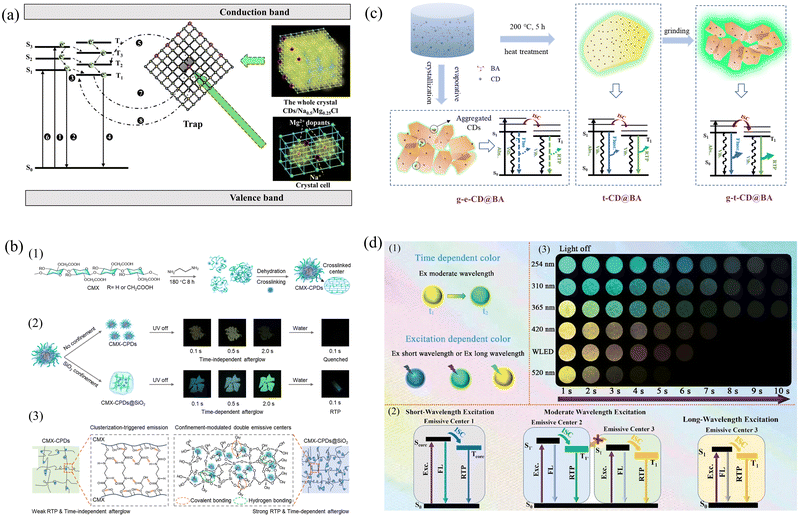 |
| Fig. 6 (a) A schematic representation of the persistent luminescence mechanism. Reproduced with permission.118 Copyright 2023, Wiley-VCH GmbH. (b) (1) Schematic of the design strategy and hierarchical core–shell structure of the CMX-CPDs. (2) Digital photographs of the RTP performance of CMX-CPDs and TDPC of CMX-CPDs@SiO2. (3) Schematic illustration of the CTE mechanism of CMX-CPDs. Reproduced with permission.120 Copyright 2023, American Chemical Society. (c) Schematic of the proposed phosphorescence mechanism of CD@BA composites. Reproduced with permission.126 Copyright 2021, Tianjin University and John Wiley & Sons Australia, Ltd. (d) Schematic of the proposed phosphorescence mechanism and design strategy of Si-CDs@SiO2 dynamic RTP material. (1) Schematic of the time-dependent and excitation-dependent Si-CDs@SiO2. (2) Possible mechanism for multicolor and dynamic color-changing phosphorescence. (3) Photographs of the Si-CDs@SiO2-550 powder under irradiation by lamps of WLED and various wavelengths (i.e., 254, 310, 365, 420, and 520 nm) exhibiting different delay times after the corresponding irradiation being switched off.129 Copyright 2023, Wiley-VCH GmbH. | |
CDs have fluorescence properties. In the presence of a substrate, CDs produce an afterglow phenomenon, and the duration of this afterglow is very long. If the decay rates of the fluorescence and afterglow of the CDs can be reasonably adjusted and the two kinds of emission can be activated, TDPC can be obtained. In Hu's group, CD porous Al2O3 composites (CDs@Al2O3) with TDPC properties were prepared by pyrolysis using the Al-based metal–organic skeleton NH2-MIL-101(Al) as the raw material.119 During the pyrolysis process, the metal element Al in the MOF was oxidized to Al2O3, but the overall structure of NH2MIL-101(Al) was not totally destroyed. The reconstructed MOF formed porous Al2O3, and the CDs were embedded into the interstices and pores of the Al2O3 matrix. Porous Al2O3 offered several confinement effects for the CDs through rigid networks, strong spatial confinement and stable covalent bonds, which inhibited the intramolecular vibrations of the CDs, reduced the nonradiative transitions, and ultimately resulted in high brightness and a long RTP. The core and surface states of the CDs have two emission centers, and both emission centers are activated simultaneously under 280 nm irradiation. Electrons return from T1 to S0 to produce fluorescence. The ISC occurs between T1 and S1, and the radiative transition from T1 to S0 permits the emission of blue phosphorescence at 400 nm. Similarly, a similar electron transfer is produced by the surface state with green phosphorescence at 500 nm. The combination of blue (96 ms) and green (911 ms) phosphorescence, which have different lifetimes, creates the magic of TDPC. Polymer CDs with cluster-departing TDPC were prepared by Peng's group using xylan as the raw material.120 With heteroatom doping and the spatial confinement of the SiO2 matrix, simultaneous activation of the low-energy emission centers of the surface oxygen functional groups and the high-energy emission centers of the crosslinked core-nitrogen-related functional groups under excitation was achieved, and high-performance TDPC emission from blue (425.6 ms) to green (1506 ms) was realized through different decay rates (Fig. 6b).
Stimulation-responsive afterglow. A dynamic stimulus response afterglow material is a material with leading-edge technology that can produce corresponding afterglow responses in response to different stimuli.121,122 This technology has a wide range of applications in the field of anticounterfeiting. To realize the effectiveness of stimulus-responsive afterglow materials, one can consider using a matrix with suitable chemical bonds or forces (e.g., ionic bonds or hydrogen bonds). This matrix can react specifically with the stimulus source to produce the desirable afterglow effect. By adjusting the structure and properties of the matrix, a sensitive response to different stimuli can be achieved.Humidity and temperature are two of the most common external stimuli, and the stimulus response afterglow underlying these stimuli can be perfectly realized through the breaking and formation of hydrogen or ionic bonds in the matrix. When stimulated by high humidity environments, dynamic stimulus-responsive afterglow materials absorb water, which alters the number and structure of hydrogen bonds in the matrix. This alteration affects the fluorescence intensity and afterglow time of the material, which in turn produces a response. Similarly, when stimulated by high temperatures, dynamic stimulus-responsive afterglow materials undergo thermotropic deformation, resulting in the breaking or formation of ionic bonds in the matrix, which affects the fluorescence intensity and afterglow time of the material. Xu's group proposed a strategy based on boron-doped CDs (BCQDs) with a paper to produce bright reversible RTP under hydrothermal stimulation.123 Due to the low electronegativity of boron and the fact that BA itself can form borate esters, it is unable to form hydrogen bonds with the matrix. Successive stimulation with water and heat destroyed the hydrogen and borate bonds of the BCQDs themselves and succeeded in allowing the BCQDs to form a strong hydrogen bonding network with the hydroxyl groups in the paper. By removing the effects of water and oxygen quenching by heating, a bright RTP effect was realized. Ionic bonding is more sensitive to temperature, so the introduction of ionic bonding can effectively improve the sensitivity and effectiveness of the response to temperature. Bi's group proposed a strategy to combine fluorine (F)-rich doped CDs (FCDs) with carboxy methyl cellulose sodium (CMCNa) with water solubility to prepare optical materials (FCDs-CMCNa) with special temperature sensitivity.124 In the FCDs-CMCNa system, F, which is highly electronegative, easily forms hydrogen bonds and ionic bonds with Na+. As the temperature decreases, the hydrogen bonding force increases, the ionic bonding force decreases, and the two combinations work together to realize a variation in the luminescence intensity with a change in temperature.
In addition to changes in chemical bonding and forces, the physical properties of the matrices themselves are nonnegligible factors in realizing the stimulus response. Factors such as the structure, morphology and surface properties of the substrate play important roles in the response to external stimuli and directly affect the performance of dynamic stimulus-responsive afterglow materials. By carefully designing and regulating these properties of the matrices, a more efficient and sensitive stimulus response can be achieved, providing additional possibilities for the application of these materials in sensing, optoelectronics and other fields. Jia's group prepared ultralong RTP films (CD/PVP) with reversible photoactivation and thermal activation by mixing luminescent CDs with PVP with excellent oxygen permeability.125 Triple-state oxygen (3O2) can quench RTP emission. Triple-state PSs can react with 3O2 under photoexcitation to produce single-linear oxygen (1O2) through a triplet–triplet energy transfer process to produce RTP. However, the penetration of 3O2 can be accelerated by heat treatment, leading to RTP quenching. The above factors are synthesized to achieve a photoactivated and thermally inactivated active state response to RTP emission. Generally, the matrix reacts with CDs to form a rigid crystal structure. However, the loading capacity of the crystal is poor, resulting in a weak luminescence peak. Therefore, improving the loading capacity is difficult for improving the afterglow intensity. Qu's group prepared an efficient RTP material through pressure-induced mixing of CDs and BA via an annealing method.126 The RTP is enhanced by grinding-induced fracture and fragmentation of crystals, which promotes the transition of high-energy amorphous states to low-energy crystalline states, maximizes the homogeneous dispersion of CDs into crystals, and enhances the suppression of nonradiative transitions. Thus, this material possesses composite RTP properties enhanced by the pressure stimulus response (Fig. 6c).
Photo-stimuli responsive afterglow materials have important applications in the field of information security due to their advantages of remote manipulation and non-contact controllability. Through the selection of matrices with excellent oxygen permeability properties for combination with CDs, the transition between 3O2 and 1O2 can be controlled, resulting in photo-stimuli responsive afterglow. Sun's group successfully prepared CDs@Gelatin films with spatially precise photodynamic phosphorescence by combining CDs with porous gelatin.127 According to the research data, the generation of dynamic phosphorescence is closely related to the special micro- and nanoporous structures of gelatin formed during water evaporation. Under the effect of light, triple-state excitons generated by CDs embedded in a porous gelatin matrix can deplete 3O2 and thus activate phosphorescence. When oxygen again entered the porous CDs@Gelatin membrane, the phosphorescence of the material disappeared.
Excitation wavelength-dependent afterglow. Excitation wavelength-dependent afterglow materials are important for achieving multicolor afterglow. By combining CDs with multiple emission centers in the matrix and stabilizing and promoting ISC through interactions such as hydrogen, ionic and covalent bonds, excitation-dependent afterglows are ultimately achieved, which results in various color effects.128 Zheng's group formed composites of CDs (Si-CDs@SiO2) with excitation-dependent properties by mixing 1-[3-(trimethoxysilyl) propyl]urea (TMSPU) and an aqueous solution of sodium citrate and introducing SiO2 and NaOH.129 The surface of Si-CDs is rich in a large number of carbonyl and amino functional groups, which not only effectively form intermolecular hydrogen bonds with SiO2, but also construct a stable covalent bonding network between SiO2. When three emission centers (415 nm, 495 nm, and 580 nm) exist on the surface of Si-CDs and form a stable structure with the matrix, their nonradiative transitions are restricted, resulting in CD composites with excitation-dependent afterglow (Fig. 6d).
3.2.3. Achieving multicolor afterglow. Current developments based on monochromatic afterglow cannot meet demand, and the synthesis of high-performance CD materials with multicolor afterglow emission has become a research hotspot. We introduce several strategies to realize multicolor afterglow emission based on the matrix.
Modulation of the size of the CDs and the surface functional groups. Modulation of the conjugate size of the CDs and the type of surface functional groups by changing the synthesis conditions (temperature and time) allows modulation of the different energy levels, resulting in multicolor afterglow emission.130 By adjusting the synthesis temperature and time, the dimensional size and morphology of the CDs can be controlled, thus directly affecting the energy band structure and energy level distribution of the CDs and thus realizing multicolor emission. Second, by changing the synthesis conditions, the type and number of functional groups on the surface of CDs, such as carboxyl groups, amino groups, and hydroxyl groups, can be regulated to affect the energy level structure of CDs and realize multicolor emission. In Hu's group, 1,8-naphthimide (NA), which contains a large amount of oxygen and nitrogen with a tendency to undergo an n–π* transition, was chosen as a precursor, and panchromatic RTP emitting materials from 619 to 376 nm were prepared by varying the calcination temperature with Al2O3 as a matrix.131 The high spin–orbit coupling and high intramolecular charge transfer efficiency due to the large conjugated structure of NA and the imine structure that enhances the push–pull effect of electrons, coupled with the formation of a hard and rigid structure of the Al2O3 matrix to limit nonradiative transitions, resulted in efficient afterglow emission (Fig. 7a). However, with increasing calcination temperature, the conjugated structure of the CDs decreases due to pyrolysis, leading to an increase in the energy gap and a blueshift of the RTP wavelength. Controlling the temperature range promotes the synthesis of precursors and thus a redshift of the wavelength. Qiu's group synthesized tunable panchromatic long-lived RTP CDs in the range of 466–638 nm in one step by controlling the ratio of CA to BA as well as the temperature.132 When the B2O3 polycrystalline network is confined within three-dimensional space, the nonradiative transitions are effectively suppressed, and the triplet states are stabilized, laying the foundation for the realization of full-color RTP CDs. With increasing amounts of citric acid and temperature, the size of the CDs continues to increase, the oxidation degree continues to increase, and the energy gap continues to decrease, thus realizing full-color RTP emission from blue to red (Fig. 7b).
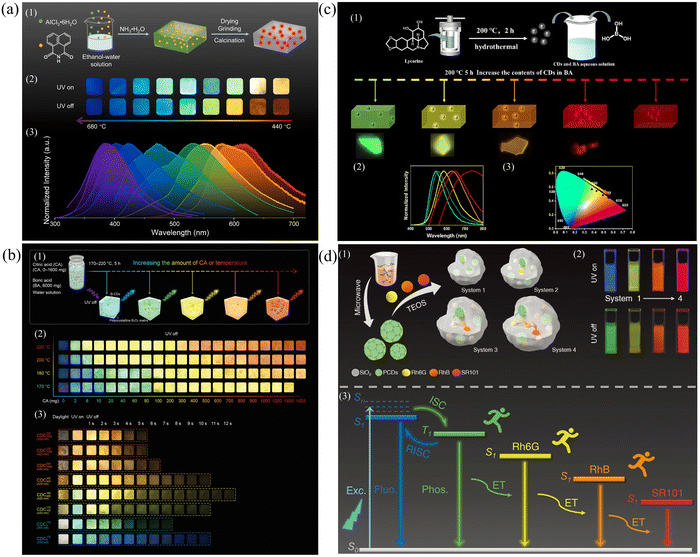 |
| Fig. 7 (a) (1) Schematic representation of procedures for the preparation of CDs@Al2O3. (2) and (3) Images and normalized afterglow spectra of the CDs@Al2O3 powders. Reproduced with permission.131 Copyright 2023, Elsevier B.V. (b) (1) Schematic representation of the fabrication process of B-CD composites with tunable full-color ultralong RTP. (2) Optical images of B-CD composites under different reaction conditions. (3) Optical images of CDCs. Reproduced with permission.132 Copyright 2022, Wiley-VCH GmbH. (c) (1) Schematic illustration for the preparation of the CDs@BA composites. (2) and (3) Phosphorescence spectra and CIE 1931 coordinates of CDs@BA composites. Reproduced with permission.140 Copyright 2023, Elsevier B.V. (d) (1) Schematic illustration of procedures for the preparation of PCDs and systems 1–4. (2) The fluorescence and afterglow images in water. (3) Proposed possible afterglow mechanism. Reproduced with permission.142 Copyright 2022, Wiley-VCH GmbH. | |
Matrix modulation. Matrices can restrict molecular motion and inhibit nonradiative transitions, while also increasing the ISC. Therefore, introducing multicolor fluorescent CDs into a suitable matrix achieves or accelerates the ISC rate, thus realizing multicolor afterglow emission. As a common matrix, PAM itself can form a rigid protective environment to inhibit the vibration and rotation of luminescent centers such as the C
O orbital of CDs; second, the amide carbonyl group (C
N) in PAM has a lone electron pair, which can promote the n–π* transition to promote the coupling of spin orbitals while generating a large number of energy levels, greatly reducing the ΔEST.133 Finally, the hydrogen bonds formed in PAM can inhibit nonradiative transitions, which can promote the generation of afterglow. Chen's group and Yu's group introduced multicolor CDs into PAM, and both achieved multicolor RTP emission via hydrogen and amide bonds, respectively.134,135 The use of SiO2-based matrices enables multicolor afterglow emission in aqueous solutions, greatly broadening the range of applications. Lu's group hydrolyzed TEOS in situ to form covalent bonds to wrap multicolor carbon points by pyrolysis, and the resulting multicolor RTP still maintained good performance in the water phase.136 TEOS forms a large number of covalent bonds (Si–O–C and Si–C) during hydrolysis, inhibiting the rotation and vibration of the CD luminescence center, and forms a tight silicon shell that can isolate oxygen and water, providing a rigid network, thus realizing multicolor RTP at 465–680 nm in water with a lifetime of up to 2.11 s.
Aggregation-induced emission (AIE). The unique flexible structure of AIE fluorophores allows them to consume excited-state energy through nonradiative decay in the dispersed state. However, once fluorophores form an aggregated state, their free rotation is limited by steric hindrance, thereby impeding nonradiative decay and enhancing emission.137 Therefore, the combination of AIE-based CDs with a matrix or the preparation of composites with AIE CDs is a novel strategy for realizing multicolor afterglow emission. Using dithiosalicylic acid (DTSA) with disulfide bonds and urea as the raw materials and only one set of precursors, Li's group proposed a strategy for the rapid preparation of color-tunable AIE CDs and embedding them in PVA to achieve multicolor delayed fluorescence (DF) by changing the filling ratio.138 Modulating the degree of aggregation of CDs through the three-dimensional space of the matrix to change the interactions between electrons is an effective strategy for achieving efficient multicolor afterglow emission in one step. In Shan's group, citric acid was used as the carbon source, CDs were obtained in NaOH by a microwave-assisted in situ carbonation condensation process, and the ratio of NaOH was changed to produce multicolor RTP CDs with adjustable wavelengths ranging from 483 to 635 nm and adjustable lifetimes ranging from 58 to 389 ms.139 In this system, the ionization of the carboxyl groups in the CDs and NaOH results in the formation of a large number of ionic bonds and a rigid crystal structure that suppresses nonradiative transitions and promotes ISC. However, as the ratio of NaOH decreases, the CDs agglomerate to produce large clusters, which generate π–π stacking and nonradiative energy transitions with each other, which effectively decreases the ΔEST between T1 and S0 and results in a redshift of the wavelength. Qu's group obtained the first tunable RTP carbon dot material from the green to near-infrared regions by adjusting the degree of aggregation of CDs in BA.140 During the synthesis process, the surface of the CDs is uniformly altered by BA passivation, which effectively prevents the quenching phenomenon due to aggregation. The three-dimensional rigid network formed by BA can effectively restrict the motion of molecules and inhibit nonradiative transitions, which lays the foundation for realizing multicolor reversible phosphorescence emission (Fig. 7c). As the degree of aggregation of the CDs increases, the distance between the CDs decreases, causing a large number of electronic interactions, and the energy level gradually becomes broader and splits into low-energy aggregation states, resulting in a reduction in the ΔEST, thus realizing tunable phosphorescence emission (from 530 to 555, 585, 625, 645 and 750 nm) from the green color to the near-infrared region.
FRET. According to FRET theory, the spectral overlap integral of the donor–acceptor system is proportional to the energy transfer probability, which implies that multicolor afterglow emission can be achieved by predesigning suitable energy donors and acceptors. Yu's group successfully realized tunable afterglows from blue to red with lifetimes ranging from 232 to 1500 ms by using CDs@zeolite with excellent afterglow lifetimes and tunable afterglow as energy donors and selecting quantum dots with different tunable wavelengths as energy acceptors.141 In this luminescent system, quantum dots can absorb blue or green high-energy photons from CDs@zeolite composites and release them into the material as low-energy photons. Most of the current FRET-based multicolor afterglows are in the solid state, and one energy transfer occurs, which severely limits the range of afterglow wavelength variation. Therefore, realizing multiple energy transfers has become a research difficulty. Hu's group reported a strategy to encapsulate green afterglow CDs into SiO2 matrices that ensures sufficient spectral overlap between donor emission and acceptor absorption for cascade resonance energy transfer of afterglow-emitting nanoparticles.142 A silica shell was formed during the hydrolysis of TEOS, which provided a rigid environment, inhibited nonradiative transitions, and blocked the quenching of water and oxygen, which provided a basis for the realization of multicolor afterglow in water. Moreover, to ensure sufficient spectral overlap between donor emission and acceptor absorption, rhodamine 6G was chosen as the energy acceptor for the green afterglow CDs to form system 1, rhodamine B was used as the energy acceptor for system 1 to form system 2, and sulfanilamide 101 was used as the energy acceptor of system 2 to form system 3 (Fig. 7d). The distance between the donor and the acceptor should be less than approximately 10 nm; thus, restriction of the silica shell can effectively ensure that the distance between the donor and acceptor decreases, eliminating efficient energy transfer. In summary, the emission of a long afterglow with a wavelength tunable in water from 510 to 610 nm is realized by the effect of energy transfer.
4. Application
Due to the special properties of a matrix, CDs exhibit solid-state fluorescence and afterglow characteristics, which endow them with great potential for application in the fields of optoelectronics, anticounterfeiting, sensing, bioimaging and therapeutics.
4.1. Photoelectric field
The composites of CDs combined with a matrix as the luminescent part in photovoltaic devices are particularly suitable for various applications of photovoltaic energy conversion, such as light-emitting solar concentrators (LSCs) and light-emitting diodes (LEDs), and play a significant role in advancing the field of photovoltaics. This composite material effectively improved the photoelectric conversion efficiency due to its excellent luminescence performance. Moreover, their tunable wavelength characteristics make it possible to prepare colorful LEDs, thus meeting the needs of different application scenarios. In addition, its excellent chemical and optical stability ensures the stability and long life of photovoltaic devices, reducing the occurrence of problems such as light decay and color drift.
LSCs are large-area solar collectors coupled with small-area solar cells for efficient solar-to-electricity conversion. Therefore, the preparation of high-efficiency LSCs is an effective method for alleviating energy shortages. Zhao's group prepared efficient LSCs by combining CDs prepared with citric acid and urea as precursors with PVP.143 The prepared CDs had an ultrahigh PLQY (∼65%) and a large Stokes shift (0.53 eV). When coupled with PVA, large LSCs (15 × 15 × 0.5 cm3) can be formed that exhibit an external light efficiency of 2.2%. Moreover, after coupling with silicon solar cells, the LSC exhibited an excellent power conversion efficiency (60 mW cm−2, uncharacterized spectrum) under natural light. Circularly polarized light is a special kind of polarized light whose electric field vector rotates in space in a circular trajectory, and the application of its optical devices is highly important. The preparation of high-performance photodetectors by combining matrices with circularly polarized light and CDs is an efficient strategy. Jelinek's group combined chiral anthraquinone-polydiacetylene with circular light polarization selectivity and CDs with excellent properties to prepare photodetectors with rapid photoresponsivity, high fidelity and redox reversibility.144 This capacitive multispectral photodetector exhibited significant sensitivity and wavelength selectivity in the spectral interval in the wavelength range of 350 to 650 nm (Fig. 8a).
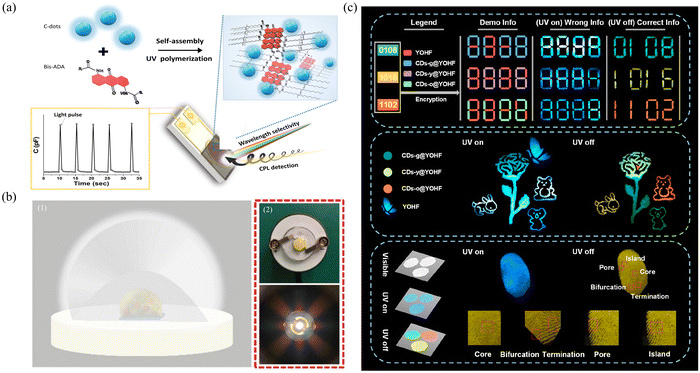 |
| Fig. 8 (a) Formation of the polymerized-bis-anthraquinone-diacetylene (polymerized-Bis-ADA)/C-dot film on the surface of the interdigitated electrode (IDE); wavelength-dependent and polarization-dependent capacitive signals are induced by illumination. Reproduced with permission.144 Copyright 2022, Wiley-VCH GmbH. (b) Application of WLED. (1) Schematic diagram of the manufacture of WLEDs. (2) Schematic diagram of the actual finished WLED.145 Copyright 2023, Wiley-VCH GmbH. (c) Images of information encryption, dual-mode color-switching anticounterfeiting, and fingerprint recognition application. Reproduced with permission.148 Copyright 2022, American Chemical Society. | |
LEDs play an important role in a wide range of applications in the field of photovoltaics due to their advantages of high efficiency and rich colors. CDs, as promising material candidates with bright, wavelength-tunable characteristics, can be applied in LED phosphors. Wu's group prepared high-efficiency LEDs by dispersing CDs in a 3-glycidyloxypropyltrimethoxysilane (KH-560) matrix.145 An excellent yellow LED with a color temperature of 2619 K, a color rendering index of 70.1, and a Commission Internationale de I’Elcairage coordinate (CIE) of (0.503, 0.475) was prepared from the yellow phosphor. A white LED (WLED) with a color temperature of 3935, a color rendering index of 84.2, and a CIE of (0.364, 0.312) was prepared by combining the obtained yellow phosphor with a commercial blue (450 nm) chip. Gong's group prepared silane-modified CD composites with an ultrahigh PLQY (70.7%), an excellent phosphorescence lifetime (0.45 s) and an ultralong afterglow duration (15 s) using BA as a matrix.146 High-performance WLEDs were successfully prepared by combining RTP powder with commercial powder, and have excellent luminescence efficiency and wavelength tunability (Fig. 8b). The WLED has a color rendering index of 93.6, a correlation color temperature of 3805 K, and a CIE of (0.39, 0.39) and simultaneously achieves a luminescence efficiency of 66.89 lm W−1, which are much greater than reported for other WLED performances.
4.2. Information security
With the rapid development of science and technology, incidents of information fraud have become increasingly frequent, causing serious damage to the interest of society and individuals. Therefore, we must attach great importance to the development of the information security field. Composites of CDs with excellent optical properties combined with matrices show great potential for development in the fields of fingerprint identification and information storage. Such composites have dynamic afterglow properties and are able to display different information, thus playing an important role in advanced anti-counterfeiting. In summary, their composites provide important technical support for building a safe and reliable information society in many fields, such as information security, identity authentication, data encryption, and biometrics.
Deng's group combined RTP CDs with a lifetime of 1.23 s with chiral helical polyacetylene (PSA) and PVA to prepare circularly polarized fluorescent (CPF) and RTP (CPRTP) composites that were highly effective.147 The combination of RTP emission from CDs and white film emission is utilized to create a multi-information display. When excited by UV, “sun” emits white light, and “tree” emits blue fluorescence. When the UV light is turned off, the “sun” and “tree” still emit green afterglow.
The use of multicolored afterglow materials as a tool in the field of information security enables covert encryption and authentication of data. By designing luminescent materials with different wavelengths and according to specific decoding rules, data can be encoded and transmitted in the form of multicolor afterglow. The original information content can be restored only when appropriate decoding techniques and equipment are used, thus improving the security and confidentiality of the information. Zhang's multicolor CDs were confined to Y(OH)xF3−x (YOHF) matrices to form multicolor afterglow materials for multicolor information security applications.148 Four different wavelength afterglow materials were combined under ultraviolet irradiation and shutdown conditions, and different combinations of digital arrangements and picture color transformations were used to achieve information confidentiality and dual-mode anticounterfeiting (Fig. 8c). It has a fast response time in fingerprint identification and has great potential in this field. Stimulus-responsive materials can emit different colors of light in response to changes in external stimuli. By utilizing this property, multichannel transmission can be achieved via message encryption, thereby providing a higher level of protection. Tan's group prepared thermally stimulated color-changing afterglow materials via the conversion of sodium bicarbonate (NaHCO3) in sodium carbonate (Na2CO3) through heat treatment, resulting in the reduction of surface defects and nonradiative transitions.149 Advanced anticounterfeiting is achieved by skillfully combining afterglow materials to form anticounterfeiting combinations that can be heat-treated differently under the same UV irradiation and shutoff conditions. This method enables the production of different patterns before and after, greatly enhancing product security and anticounterfeiting capabilities.
4.3. Ion detection
In addition to restricting molecular motion to realize solid-state fluorescence and afterglow effects, the matrix can also be used as a platform for ion detection. By rationally designing the structure and composition of the matrix, highly sensitive detection of specific ions can be achieved.150,151 The utilization of such matrix platforms not only expands the application scope of matrices but also provides a feasible solution for ion detection. Su's group prepared enzyme sensors and dye sensors using amino acids and polymer precursors synthesized by doping CDs into an agarose hydrogel matrix.152 By encapsulating the enzyme in this matrix, better enzyme kinetics and stability can be achieved. This matrix also provided a stable colorimetric response for the five sensors, thus improving the accuracy of the entire sensor array. The experimental results showed that the sensor array was clinically relevant for all five wound markers with high accuracy (recoveries between 91.5% and 113.1%). The application of this matrix provides a reliable solution for the detection of wound markers (Fig. 9a). With the advantages of high sensitivity, wide detection range, reversibility and high stability, afterglow materials provide a reliable and efficient solution for ion detection. Xu's group encapsulated phosphorescent CDs in a SiO2 matrix as a donor, the detected substance Hg2+ responded to rhodamine derivatives as an acceptor, and sensitive Hg2+ detection was achieved by energy transfer between the Hg2+-departure donor and the acceptor.153 The probe exhibited good linearity with respect to the Hg2+ concentration, and its phosphorescence intensity was proportional to the Hg2+ concentration (Y = 0.0892X + 0.2456, R2 = 0.998, 0.5–10 μM). Its detection limit is 58 nM, which provides high sensitivity. In addition, the probe has good specificity for Hg2+ and can accurately detect Hg2+ in the presence of other interferences (Fig. 9b). The unique feature of this probe is that the addition of Hg2+ changes the probe from colorless to pink during daylight, a change that can be observed with the naked eye. In contrast, under UV excitation and extinction conditions, long-lived orange phosphorescence can be observed only in the probe in the presence of Hg2+. This property makes the probe more reliable and visible for the detection of Hg2+ under specific conditions.
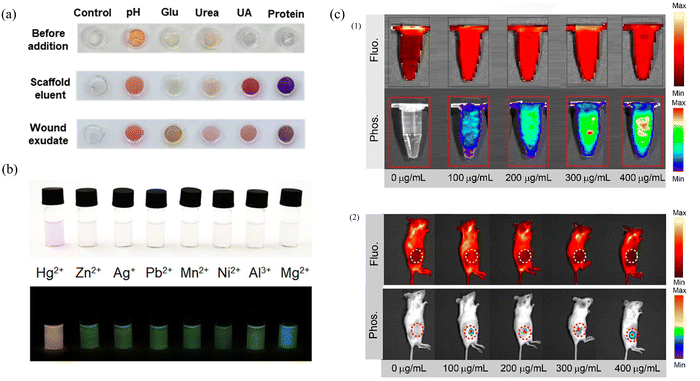 |
| Fig. 9 (a) Color changes of sensors from left to right, control, pH, glucose, urea, uric acid (UA) and protein before and after adding wound fluids. Reproduced with permission.152 Copyright 2023, American Chemical Society. (b) Photographs of the probe in the presence of various metal ions (10 μM) under sunlight (top panel) and 365 nm excitation off (bottom panel). Reproduced with permission.153 Copyright 2023, American Chemical Society. (c) (1) In vitro fluorescence and afterglow images of the CNDs-RhB@silica with different concentrations (1.5 mL). (2) In vivo fluorescence and phosphorescence images of mice with the subcutaneous implantation of different concentrations of CNDs-RhB@silica. Reproduced with permission.156 Copyright 2021, American Chemical Society. | |
4.4. Biotherapeutics and imaging
CDs are excellent optical materials with tunable wavelengths and are particularly suitable for biotherapeutic and imaging applications. However, due to their susceptibility to environmental influences, which can lead to the degradation of photoemission properties, the use of biocompatible matrices to protect CDs and ensure their stability and reliability for applications in biological systems is an important strategy. Wang's group proposed a strategy for reacting prepared CDs with cellulose nanocrystals to use as a phototherapeutic agent and cross-linking agent during hydrogel matrix formation, which satisfies both photothermal therapy (PTT) and photodynamic therapy (PDT) conditions.154 The CDs exhibited an ultrahigh photothermal conversion efficiency (77.6%) and had a very high single-linear quantum yield of 0.37 under single 660 nm LED irradiation. The treated tissues and organs maintained a normal cellular morphology, demonstrating that the composites are nontoxic. In vitro experiments on B16F10 and HeLa cells as well as in vivo experiments on tumor-bearing nude mice demonstrated that the injectable hydrogel has good biocompatibility, biosafety, and efficient tumor killing potency.
Long wavelength luminescent materials have better tissue penetration ability and can be imaged at deeper tissue levels, thus enabling observation and analysis of deep tissues. This approach is important for studying the structural, functional and pathological changes in biological tissues. Li's group confined CDs to mesoporous silica nanoparticles (MSNs) with low biotoxicity and utilized the n–π interactions between the host and guest to achieve deep red emission in both the solid and liquid states.155 The composites were injected into HeLa cells, and the morphology of the cells was more evident under UV light irradiation as indicated by the red fluorescence. Through FRET, Shan's group generated a red afterglow material (CNDs-RhB@silica) that lasts for 8 s in the liquid state, as observed by the naked eye, by utilizing the confining effect of the silica matrix.156 CNDs-RhB@silica was injected into live rats as an afterglow agent, and significant imaging information was obtained in their bodies. The afterglow intensity increased as the concentration increased from 0 to 400 μg mL−1, indicating that CNDs-RhB@silica has the advantages of low background noise and a high signal-to-noise ratio (Fig. 9c). Under the same signal acquisition conditions, the tissue background emission intensity was 0.702 × 105 ps−1 cm−2 sr−1; when the concentration of CNDs-RhB@silica was 200 μg mL−1, the signal-to-noise ratio of afterglow in vivo imaging was as high as 80.1, which indicated that the system had excellent performance and excellent imaging performance.
5. Summary and outlook
We successfully realized solid-state fluorescence and afterglow effects by thoroughly investigating the role of CDs in a matrix. To realize solid-state fluorescence, we adopted a strategy to limit the ACQ and π–π stacking effects of the CDs. By controlling these effects, we were able to obtain stable fluorescence emission in the solid state. In addition, to realize the afterglow effect, we restricted the motion of the molecules in the matrix and actively promoted ISC. This strategy effectively suppresses nonradiative transitions, resulting in long-lasting afterglow emission from CDs in the matrix. We explored pathways to realize and improve solid-state fluorescence and afterglow effects from the perspective of multiple chemical forces. Forces such as hydrogen, ionic, and covalent bonds play an important role in this process. By skillfully modulating these forces, we can realize the synergistic effect of single or multiple chemical forces to further enhance the functional performance of solid-state fluorescence and afterglow. The detailed elaboration of these mechanisms provides us with an important foundation for the preparation of various materials for various applications. In addition, we endow CDs with different properties based on their different matrix characteristics, thereby expanding their applications in the fields of optoelectronics, information security, sensing, and biotherapeutics and imaging. These applications include but are not limited to the fields of highly efficient optoelectronic devices, secure cryptography, precision sensors, and high-resolution bioimaging. We hope that these research results will provide important references and guidance for the development of a wide range of applications and promote the widespread use of CDs in various fields.
However, there are still many challenges in solid-state fluorescence and afterglow studies based on the binding of fluorescent quantum dots (CDs) to matrices.
1. Sample preparation difficulties: there are a number of difficulties in sample preparation. The selection and preparation of suitable matrix materials, as well as CDs that can interact well with the matrix, is not easy. The method used in the preparation process, as well as the doping ratio between the two and the purification method, will have a significant impact on the optical properties and reliability of the composites. The binding of CDs with different kinds of functional groups and conjugated structures of different sizes to the matrices affects, among other things, their energy transport and electron transition. Therefore, we need to explore and make use of adequate characterization techniques to study the structural features of CDs and matrices in depth, including the crystal structure, electronic structure and other aspects. Through an in-depth understanding of their internal structure, we can provide a basis for the preparation of high-quality samples.
2. Interactions based on the binding of CDs to matrices to achieve and improve solid-state fluorescence and afterglow are diverse and poorly defined. Such interactions often involve intramolecular charge transfer, electron transition, and molecular bonding or molecular force interactions. The spatial constraints and interactions arising from the hydrogen, ionic, and covalent bonds formed between CDs and substrates are also not well understood. Therefore, the interactions and synergies between them need to be elaborated and accounted for with the help of more sophisticated characterization techniques. For example, infrared spectroscopy can be used to obtain information about functional groups in molecules, and X-ray diffraction can be used to determine the arrangement of molecules with each other and thus identify possible interactions. Chemical shifts and coupling constants are observed using nuclear magnetic resonance spectroscopy to infer the type and strength of the interactions. The effect of intermolecular interactions on optical properties was modeled using calculations such as density functional theory. A comprehensive understanding of the mechanisms of hydrogen bonding, ionic bonding and covalent bonding during solid-state fluorescence and afterglow generation and their spatial constraints and synergistic interactions through the above or other characterization methods is highly important for further improving their optical properties.
3. The optical properties are not outstanding. The combination of CDs with matrices based on CDs is a complex photophysical process to achieve and improve solid-state fluorescence and afterglow, including the formation of excited states and excited state transitions. These processes are affected by the nature of the material itself as well as by external conditions. When inappropriate matrices are chemically bonded to CDs, they may alter the functional groups on the surface of the CDs, which in turn affects the electronic structure and leads to a decrease in the optical properties (e.g., PLQY, luminescence intensity, lifetime, and luminescence wavelength). Therefore, it is necessary to understand their interactions to achieve precise modulation to improve the luminescence performance. For example, excessive defects on the surface of CDs lead to a reduced lifetime and PLQY. Therefore, selecting suitable matrices that utilize their internal functional groups to bind to defects in CDs and eliminate excessive defects can help to increase the lifetime and PLQY, thus improving the luminescence performance. The triplet excitons of CDs are susceptible to external environments, which leads to diminished or quenched afterglow performance. Therefore, these problems can be avoided by selecting appropriate matrices with excellent water and oxygen barrier properties. Hydrogen, ionic and covalent bonds as well as synergistic interactions between the matrices and the CDs can stabilize the CDs and ensure the efficient conduction of electrons, thus improving the optical performance. The matrix not only protects the CDs, but also modifies them by chemical reactions to further improve their optical performance. Therefore, it is crucial to select and prepare appropriate matrices to combine with CDs to improve luminescence performance according to specific needs.
4. CDs bonded to matrices show promising applications in achieving and improving solid-state fluorescence and afterglow. Hydrogen, ionic and covalent bonds formed between CDs and matrices can stabilize CDs, effectively preventing them from aggregating due to motion and vibrations, thus reducing energy loss. At the same time, the matrix isolates CDs from the outside world, effectively preventing interference from the external environment (e.g., water, oxygen, etc.), further improving the luminescence stability, which is necessary for the realization of application. When the hydrogen, ionic and covalent bonds formed between CDs and matrices are stimulated by external conditions, they will break and regenerate, thus triggering the disappearance and generation of solid-state fluorescence, a property that can be applied to the field of ion detection. By adjusting the concentration ratio of CDs to matrices, the energy transfer efficiency can be altered, and multicolor emission can be achieved, opening up new possibilities for optoelectronic devices. External stimuli leading to changes in the interaction between CDs and matrices are key for achieving stimulus-responsive afterglow, for example, by adjusting factors such as temperature and acid–base conditions to disrupt or generate interactions, which affects the rate of excitation of ISC and thus modulates the performance of afterglow. In addition, the properties of the matrix itself, such as water and oxygen resistance, confer different stimulus responses to the composites, such as humidity and light stimulus responses. Properties such as hydrophilicity, lipophilicity, or hydrophobicity of the matrices enable excellent afterglow in water, providing new possibilities for in vivo imaging. Therefore, how to optimize and regulate single or multiple interaction forces (to achieve single or multiple chemical bond formation and to achieve chemical bond breakage or regeneration in response to external stimuli) to prepare luminescent materials with single or multiple responsiveness is one of the most important strategies to broaden their application areas.
Conflicts of interest
The authors declare no competing financial interest.
Acknowledgements
We gratefully acknowledge the Youth Talent Program Startup Foundation of Qufu Normal University (No. 602601), and the Natural Science Foundation of Rizhao (No. RZ2021ZR37).
References
- A. Panigrahi, R. K. Behera, L. Mishra, S. Kumar, P. Dubey, S. Dutta and M. K. Sarangi, Exploring optoelectronic properties of undoped and amine-doped carbon dots: Impact of surface functionalization and pH, Carbon, 2023, 206, 114–123 CrossRef CAS.
- B. Zhao, H. Ma, H. Jia, M. Zheng, K. Xu, R. Yu, S. Qu and Z. Tan, Triphenylamine-Derived Solid-State Emissive Carbon Dots for Multicolor High-Efficiency Electroluminescent Light-Emitting Diodes, Angew. Chem., Int. Ed., 2023, 62, e202301651 CrossRef CAS PubMed.
- L. Yang, F. Yuan, L. Sui, Y. Zhang, Y. Li, X. Li, Z. Tan and L. Fan, Carbon Quantum Dots with Near-Unity Quantum Yield Bandgap Emission for Electroluminescent Light-Emitting Diodes, Angew. Chem., Int. Ed., 2023, 62, e202218568 CrossRef PubMed.
- L. Yang, W. Hu, F. Pei, Z. Liu, J. Wang, Z. Tong, X. Mu, B. Du, M. Xia, F. Wang and B. Liu, A ratiometric fluorescence imprinted sensor based on N-CDs and metal-organic frameworks for visual smart detection of malathion, Food Chem., 2024, 438, 138068 CrossRef CAS PubMed.
- C. Cao and W. Guo, Carbon dots-based fluorescent probe for the detection of imidacloprid residue in leafy vegetables, Food Chem., 2024, 435, 137578 CrossRef CAS PubMed.
- K. Zhao, F. Liu, H. Sun, P. Xia, J. Qu, C. Lu, S. Zong, R. Zhang, S. Xu and C. Wang, A Novel Ion Species- and Ion Concentration-Dependent Anti-Counterfeiting Based on Ratiometric Fluorescence Sensing of CDs@MOF-Nanofibrous Films, Small, 2023, 2305211 Search PubMed.
- H. Liu, Y. Chen, L. Mo, F. Long, Y. Wang, Z. Guo, C. Hu, Z. Liu and H. Chen, Afterglow Photodynamic Therapy Based on Carbon Dots Embedded Silica Nanoparticles for Nondestructive Teeth Whitening, ACS Nano, 2023, 17, 21195–21205 CrossRef.
- T. Zhang, Q. Cheng, J. H. Lei, B. Wang, Y. Chang, Y. Liu, G. Xing, C. Deng, Z. Tang and S. Qu, Constructing Oxygen-Related Defects in Carbon Nanodots with Janus Optical Properties: Noninvasive NIR Fluorescent Imaging and Effective Photocatalytic Therapy, Adv. Mater., 2023, 35, 2302705 CrossRef CAS PubMed.
- Y. Li, C. Liu, H. Sun, M. Chen, D. Hou, Y. Zheng, H. Xie, B. Zhou and X. Lin, Formation and Band Gap Tuning Mechanism of Multicolor Emissive Carbon Dots from m-Hydroxybenzaldehyde, Adv. Sci., 2023, 10, 2300543 CrossRef CAS PubMed.
- H. Sun, P. Xia, H. Shao, R. Zhang, C. Lu, S. Xu and C. Wang, Heating-free synthesis of red emissive carbon dots through separated processes of polymerization and carbonization, J. Colloid Interface Sci., 2023, 646, 932–939 CrossRef CAS PubMed.
- Q. Fu, S. Sun, K. Lu, N. Li and Z. Dong, Boron-doped carbon dots: Doping strategies, performance effects, and applications, Chinese Chem. Lett., 2023, 109136 Search PubMed.
- J. Xu, Q. Liang, Z. Li, V. Y. Osipov, Y. Lin, B. Ge, Q. Xu, J. Zhu and H. Bi, Rational Synthesis of Solid-State Ultraviolet B Emitting Carbon Dots via Acetic Acid-Promoted Fractions of sp3 Bonding Strategy, Adv. Mater., 2022, 34, 2200011 CrossRef CAS PubMed.
- J. Xu, C. Dong, H. Ding and H.-H. Bi, Synthesis, Luminescence Mechanism and Applications of Carbon Dots with Afterglow, Chin. J. Lumin., 2020, 41, 1567–1578 CAS.
- T. Zhang, X. Wu, L. Liu, Y. Yang, J. Zhuang, Z. Li and H. Bi, Supramolecular Surface Engineering of Carbon Dots enables Matrix-free Room Temperature Phosphorescence, Chin. J. Chem., 2023, 41(18), 2330–2336 CrossRef CAS.
- Y. Zheng, Z. Wang, J. Liu, Y. Zhang, L. Gao, C. Wang, X. Zheng, Q. Zhou, Y. Yang, Y. Li, H. Tang, L. Qu, Y. Zhao and C. Yang, Long-Lived Room Temperature Phosphorescence Crystals with Green Light Excitation, ACS Appl. Mater. Interfaces, 2022, 14, 15706–15715 CrossRef CAS PubMed.
- Y. Liu, X. Kang, Y. Xu, Y. Li, S. Wang, C. Wang, W. Hu, R. Wang and J. Liu, Modulating the Carbonization Degree of Carbon Dots for Multicolor Afterglow Emission, ACS Appl. Mater. Interfaces, 2022, 14, 22363–22371 CrossRef CAS PubMed.
- S. Cui, B. Wang, Y. Zan, Z. Shen, S. Liu, W. Fang, X. Yan, Y. Li and L. Chen, Colorful, time-dependent carbon dot-based afterglow with ultralong lifetime, Chem. Eng. J., 2022, 431, 133373 CrossRef CAS.
- Y. Jie, Y. Gao, G. Yang, P. Xi, F. Li, J. Zhang, D. Wang, Z. Fan, J. Yan, P. Dai and J. Fang, Yellow-Emissive Carbon Dots with Long-Lifetime Room-Temperature Phosphorescence for Information Encryption and Bioimaging, ACS Appl. Nano Mater., 2023, 6, 20431–20439 CrossRef CAS.
- J. Wen, Z. Zeng, B. Wang, J. Hong, Y. Chen, J. Zhang, J. Li and J. Jiang, Modulating hydrothermal condition to achieve carbon dots-zeolite composites with multicolor afterglow, Nano Res., 2023, 16, 7761–7769 CrossRef CAS.
- W. Xu, Q. Han, C. Ji, F. Zeng, X. Zhang, J. Deng, C. Shi and Z. Peng, Solid-State, Hectogram-Scale Preparation of Red Carbon Dots as Phosphor for Energy-Transfer-Induced High-Quality White LEDs with CRI of 97, Small, 2023, 2304123 CrossRef CAS PubMed.
- G. Guo, T. Li, Y. Wang, H. Hu, H. Xing, S. Tang, S. Gao, X. Leng and D. Chen, Aggregation-induced bimodal excitation of nitrogen-doped carbon dots for ratiometric sensing of new coccine and solid-state multicolor lighting, J. Colloid Interface Sci., 2023, 645, 96–106 CrossRef CAS PubMed.
- Y. Zhan, B. Shang, M. Chen and L. Wu, One-Step Synthesis of Silica-Coated Carbon Dots with Controllable Solid-State Fluorescence for White Light-Emitting Diodes, Small, 2019, 15, 1901161 CrossRef PubMed.
- J.-Y. Wei, Q. Lou, J.-H. Zang, Z.-Y. Liu, Y.-L. Ye, C.-L. Shen, W.-B. Zhao, L. Dong and C.-X. Shan, Scalable Synthesis of Green Fluorescent Carbon Dot Powders with Unprecedented Efficiency, Adv. Optical Mater., 2020, 1901938 CrossRef CAS.
- D. Li, E. Kou, W. Li, H. Zhang, X. Zhang, J. Zhuang, Y. Liu, C. Hu, Y. Zheng, Q. Yang and B. Lei, Oxidation-induced quenching mechanism of ultrabright red carbon dots and application in antioxidant RCDs/PVA film, Chem. Eng. J., 2021, 425, 131653 CrossRef CAS.
- J. Wang, S. Zhang, Y. Li, C. Wu, W. Zhang, H. Zhang, Z. Xie and S. Zhou, Ultra-Broadband Random Laser and White-Light Emissive Carbon Dots/Crystal In-Situ Hybrids, Small, 2022, 18, 2203152 CrossRef CAS PubMed.
- J. Yan, G. Pan, W. Lin, Z. Tang, J. Zhang, J. Li, W. Li, X. Lin, H. Luo and G. Yi, Multi-responsive graphene quantum dots hybrid self-healing structural color hydrogel for information encoding and encryption, Chem. Eng. J., 2023, 451, 138922 CrossRef CAS.
- B. Wang, Y. Yu, H. Zhang, Y. Xuan, G. Chen, W. Ma, J. Li and J. Yu, Carbon Dots in a Matrix: Energy-Transfer-Enhanced Room-Temperature Red Phosphorescence, Angew. Chem., Int. Ed., 2019, 131, 18614–18619 CrossRef.
- Y. Sun, S. Liu, L. Sun, S. Wu, G. Hu, X. Pang, A. T. Smith, C. Hu, S. Zeng, W. Wang, Y. Liu and M. Zheng, Ultralong lifetime and efficient room temperature phosphorescent carbon dots through multi-confinement structure design, Nat. Commun., 2020, 11, 5591 CrossRef CAS PubMed.
- Y. Wang, K. Jiang, J. Du, L. Zheng, Y. Li, Z. Li and H. Lin, Green and Near-Infrared Dual-Mode Afterglow of Carbon Dots and Their Applications for Confidential Information Readout, Nano-Micro Lett., 2021, 13, 198 CrossRef PubMed.
- J. Zhu, J. Hu, Q. Hu, X. Zhang, E. V. Ushakova, K. Liu, S. Wang, X. Chen, C. Shan, A. L. Rogach and X. Bai, White Light Afterglow in Carbon Dots Achieved via Synergy between the Room-Temperature Phosphorescence and the Delayed Fluorescence, Small, 2022, 18, 2105415 CrossRef CAS PubMed.
- B. Xu, Y. Jia, H. Ning, Q. Teng, C. Li, X. Fang, J. Li, H. Zhou, X. Meng, Z. Gao, X. Wang, Z. Wang and F. Yuan, Visible Light-Activated Ultralong-Lived Triplet Excitons of Carbon Dots for White-Light Manipulated Anti-Counterfeiting, Small, 2023, 2304958 Search PubMed.
- J. Guo, Y. Lu, A.-Q. Xie, G. Li, Z.-B. Liang, C.-F. Wang, X. Yang and S. Chen, Yellow-Emissive Carbon Dots with High Solid-State Photoluminescence, Adv. Funct. Mater., 2022, 32, 2110393 CrossRef CAS.
- Y. Qu, X. Bai, D. Li, X. Zhang, C. Liang, W. Zheng and S. Qu, Solution-processable carbon dots with efficient solid-state red/near-infrared emission, J. Colloid Interface Sci., 2022, 613, 547–553 CrossRef CAS PubMed.
- Q. Fu, K. Zhang, K. Lu, N. Li, S. Sun and Z. Dong, Recent advances in solid-state fluorescent of red carbon dots: A comprehensive review, J. Alloys Compd., 2024, 971, 172688 CrossRef CAS.
- J. Wang, Q. Li, J. Zheng, Y. Yang, X. Liu and B. Xu, N, B-Codoping Induces High-Efficiency Solid-State Fluorescence and Dual Emission of Yellow/Orange Carbon Dots, ACS Sustainable Chem. Eng., 2021, 9, 2224–2236 CrossRef CAS.
- J. Li, J. Chen, X. Zhao, A. Vomiero and X. Gong, High-loading of organosilane-grafted carbon dots in high-performance luminescent solar concentrators with ultrahigh transparency, Nano Energy, 2023, 115, 108674 CrossRef CAS.
- J. Wu, W. Xin, Y. Wu, Y. Zhan, J. Li, J. Wang, S. Huang and X. Wang, Solid-state photoluminescent silicone-carbon dots/dendrimer composites for highly efficient luminescent solar concentrators, Chem. Eng. J., 2021, 422, 130158 CrossRef CAS.
- Y. Ma, N. Zhang and L. Yang, Long-wavelength emissive solid-state carbon dots in nanoporous glass with excellent thermal stability, J. Colloid Interface Sci., 2021, 599, 686–693 CrossRef CAS.
- R. Dai, X. Chen, N. Ouyang and Y. Hu, A pH-controlled synthetic route to violet, green, and orange fluorescent carbon dots for multicolor light-emitting diodes, Chem. Eng. J., 2022, 431, 134172 CrossRef CAS.
- J. Ni, X. Huang, Y. Bai, B. Zhao, Y. Han, S. Han, T. Xu, C. Si and C. Zhang, Resistance to aggregation-caused quenching: chitosan-based solid carbon dots for white light-emitting diode and 3D printing, Adv. Compos. Hybrid Mater., 2022, 5, 1865–1875 CrossRef CAS.
- Q. Lou, Q. Ni, C. Niu, J. Wei, Z. Zhang, W. Shen, C. Shen, C. Qin, G. Zheng, K. Liu, J. Zang, L. Dong and C.-X. Shan, Carbon Nanodots with Nearly Unity Fluorescent Efficiency Realized via Localized Excitons, Adv. Sci., 2022, 9, 2203622 CrossRef CAS.
- B. Wang, H. Song, Z. Tang, B. Yang and S. Lu, Ethanol-derived white emissive carbon dots: the formation process investigation and multi-color/white LEDs preparation, Nano Res., 2022, 15, 942–949 CrossRef CAS.
- C. Ji, Q. Han, Y. Zhou, J. Wu, W. Shi, L. Gao, R. M. Leblanc and Z. Peng, Phenylenediamine-derived near infrared carbon dots: The kilogram-scale preparation, formation process, photoluminescence tuning mechanism and application as red phosphors, Carbon, 2022, 192, 198–208 CrossRef CAS.
- H. Ding, X.-X. Zhou, Z.-H. Zhang, T.-P. Zhao, J.-S. Wei and H.-M. Xiong, Large scale synthesis of full-color emissive carbon dots from a single carbon source by a solvent-free method, Nano Res., 2022, 15, 3548–3555 CrossRef CAS.
- Z. Sun, F. Yan, J. Xu, H. Zhang and L. Chen, Solvent-controlled synthesis strategy of multicolor emission carbon dots and its applications in sensing and light-emitting devices, Nano Res., 2022, 15, 414–422 CrossRef CAS.
- M. Cao, X. Zhao and X. Gong, Ionic Liquid-Assisted Fast Synthesis of Carbon Dots with Strong Fluorescence and Their Tunable Multicolor Emission, Small, 2022, 18, 2106683 CrossRef CAS PubMed.
- H. Guo, Z. Liu, X. Shen and L. Wang, One-Pot Synthesis of Orange Emissive Carbon Quantum Dots for All-Type High Color Rendering Index White Light-Emitting Diodes, ACS Sustainable Chem. Eng., 2022, 10, 8289–8296 CrossRef CAS.
- G. Ma, R. Wang, M. Zhang, Z. Dong, A. Zhang, M. Qu, L. Gao, Y. Wei and J. Wei, Solvothermal preparation of nitrogen-doped carbon dots with PET waste as precursor and their application in LEDs and water detection, Spectrochim. Acta, Part A, 2023, 289, 122178 CrossRef CAS.
- X. Yang, L. Sui, B. Wang, Y. Zhang, Z. Tang, B. Yang and S. Lu, Red-emitting, self-oxidizing carbon dots for the preparation of white LEDs with super-high color rendering index, Sci. China: Chem., 2021, 64, 1547–1553 CrossRef CAS.
- I. A. Olivares-Torres, J. Alvarado-Rivera, R. Guzmán-Zamudio, R. A. Iñiguez-Palomares and M. E. Álvarez-Ramos, Adjustable emission of carbon dots and rhodamine B embedded in organic-inorganic hybrid gels for solid-state light devices, J. Sol-Gel Sci. Technol., 2023, 106, 186–198 CrossRef CAS.
- F. Salehtabar and M. Ghaemy, Preparation of strongly photoluminescent nanocomposite from DGEBA epoxy resin and highly fluorescent nitrogen-doped carbon dots, Polym. Bull., 2023, 80, 3247–3264 CrossRef CAS.
- H.-J. Wang, W.-Y. Hou, Y.-W. Hao, W.-S. Jiang, H.-L. Chen and Q.-Q. Zhang, Novel yellow solid-state fluorescent-emitting carbon dots with high quantum yield for white light-emitting diodes, Spectrochim. Acta, Part A, 2021, 250, 119340 CrossRef CAS PubMed.
- J. Guo, Y. Chen, P. Zhang, G. Li, X. Yang, C. Wang and S. Chen, Easy-to-perform organic-solvent-free synthesis of carbon dots with strong green photoluminescence, Chinese Chem. Lett., 2023, 108481 Search PubMed.
- P. Varatharajan, I. B. S. Banu, M. H. Mamat and N. Vasimalai, Hydrothermal synthesis of orange fluorescent carbon dots and their application in fabrication of warm WLEDs and fluorescent ink, Physica B, 2023, 654, 414703 CrossRef CAS.
- J. Wang, J. Zheng, Y. Yang, X. Liu, J. Qiu and Y. Tian, Tunable full-color solid-state fluorescent carbon dots for light emitting diodes, Carbon, 2022, 190, 22–31 CrossRef CAS.
- S. Prakash, S. Sahu, B. Patra and A. K. Mishra, Understanding the aggregation of excitation wavelength independent emission of amphiphilic carbon dots for bioimaging and organic acid sensing, Spectrochim. Acta, Part A, 2023, 290, 122257 CrossRef CAS.
- S. Prakash, S. Sahu, S. Bhattacharya, P. B. Bisht and A. K. Mishra, Carbon Dot−NaCl Crystals for White-Light Generation and Fabry-Perot Lasing, Chem. - Asian J., 2021, 16, 783–792 CrossRef CAS PubMed.
- L. Wang, W. Li, L. Yin, Y. Liu, H. Guo, J. Lai, Y. Han, G. Li, M. Li, J. Zhang, R. Vajtai, P. M. Ajayan and M. Wu, Full-color fluorescent carbon quantum dots, Sci. Adv., 2020, 6, eabb6772 CrossRef CAS PubMed.
- Y.-J. Tong, L.-D. Yu, Y. Huang, Y. Li, N. Li, Q. Fu, Y.-X. Ye, F. Zhu, J. Pawliszyn, J. Xu and G. Ouyang, High-quality full-color carbon quantum dots synthesized under an unprecedentedly mild condition, iScience, 2022, 25, 104421 CrossRef CAS PubMed.
- Z. Sun, W. Zhou, J. Luo, J. Fan, Z.-C. Wu, H. Zhu, J. Huang and X. Zhang, High-efficient and pH-sensitive orange luminescence from silicon-doped carbon dots for information encryption and bio-imaging, J. Colloid Interface Sci., 2022, 607, 16–23 CrossRef CAS PubMed.
- X. Liu, L. Yan, J. Zheng, Y. Yang, X. Liu and B. Xu, Highly optically and thermally stable carbon dots enabled by thermal annealing for laser illumination, J. Mater. Chem. C, 2023, 11, 3562–3570 RSC.
- Y. Chen, G. Xiong, L. Zhu, J. Huang, X. Chen, Y. Chen and M. Cao, Enhanced Fluorescence and Environmental Stability of Red-Emissive Carbon Dots via Chemical Bonding with Cellulose Films, ACS Omega, 2022, 7, 6834–6842 CrossRef CAS PubMed.
- Q. Lou, X. Yang, K. Liu, Z. Ding, J. Qin, Y. Li, C. Lv, Y. Shang, Y. Zhang, Z. Zhang, J. Zang, L. Dong and C.-X. Shan, Pressure-induced photoluminescence enhancement and ambient retention in confined carbon dots, Nano Res., 2022, 15, 2545–2551 CrossRef CAS.
- U. Lee, E. Heo, T.-H. Le, H. Lee, S. Kim, S. Le, H. Jo and H. Yoon, Carbon dots for epoxy curing: Anti-forgery patterns with long-term luminescent stability, Chem. Eng. J., 2021, 405, 126988 CrossRef CAS.
- X. Cao, J. Chen, Y. Chen, X. Jiang, W. Fan, H. Ma, Z. Sun and Y. Zhan, Simple synthesis of carbon dots/organosilicon composites with tunable solid-state emission and size for accurate latent fingerprint identification, J. Mater. Chem. C, 2024, 12, 187–196 RSC.
- G. Hu, Y. Wang, S. Zhang, H. Ding, Z. Zhou, J. Wei, X. Li and H. Xiong, Rational synthesis of silane-functionalized carbon dots with high-efficiency full-color solid-state fluorescence for light emitting diodes, Carbon, 2023, 203, 1–10 CrossRef CAS.
- F. Yan, H. Zhang, J. Xu, Y. Wu, Y. Zang and J. Sun, Color Emission Carbon Dots with Quench-ResixAstant Solid-State Fluorescence for Light-Emitting Diodes, ACS Sustainable Chem. Eng., 2021, 9, 3901–3908 CrossRef CAS.
- Y. Wu, L. Zhao, X. Cao, Y. Zhang, X. Jiang, Z. Sun and Y. Zhan, Bright and multicolor emissive carbon dots/organosilicon composite for highly efficient tandem luminescent solar concentrators, Carbon, 2023, 207, 77–85 CrossRef CAS.
- Z. Wang, N. Jiang, M. Liu, R. Zhang, F. Huang and D. Chen, Bright Electroluminescent White-Light-Emitting Diodes Based on Carbon Dots with Tunable Correlated Color Temperature Enabled by Aggregation, Small, 2021, 17, 2104551 CrossRef CAS PubMed.
- X. Liu, W. Liu, K. Zuo, J. Zheng, M. Wang and X. Liu, High Color Stability Blue-to-Violet Room Temperature Phosphorescent Carbon Dot Composites with Ultralong Lifetime for Information Encryption, ACS Sustainable Chem. Eng., 2023, 11, 1809–1819 CrossRef CAS.
- C. Zheng, S. Tao, Y. Liu, C. Kang and B. Yang, Achieving blue water-dispersed room-temperature phosphorescence of carbonized polymer dots through nano-compositing with mesoporous silica, Chinese Chem. Lett., 2022, 33, 4213–4218 CrossRef CAS.
- H. Zhang, L. Sun, X. Guo, J. Xu, X. Zhao and Y. Xia, Multicolor fluorescent/room temperature phosphorescent carbon dot composites for information encryption and anti-counterfeiting, Appl. Surf. Sci., 2023, 613, 155945 CrossRef CAS.
- Q. Su, L. Gan and X. Yang, Achieving room temperature phosphorescence in aqueous phase through rigidifying the triplet state and information encryption, Appl. Surf. Sci., 2021, 566, 150726 CrossRef CAS.
- F. F. Peng, R. X. Wang, X. R. Wang, W. Y. Shi and C. Lu, Thermally activated delayed fluorescence from confined carbon dots activated by the triple synergy effect for advanced information encryption, J. Mater. Chem. C, 2023, 31, 10712–10721 RSC.
- C. Li, X. Zhao, C. Li, J. Hu, J. Zhu, Q. Lou, N. Chen, Z. Song, X. Chen and G. Pan, Efficient color-tunable room temperature phosphorescence through confining carbon dots in ionic crystal, J. Alloys Compd., 2023, 948, 169674 CrossRef CAS.
- Q. Lou, N. Chen, J. Zhu, K. Liu, C. Li, Y. Zhu, W. Xu, X. Chen, Z. Song, C. Liang, C.-X. Shan and J. Hu, Thermally Enhanced and Long Lifetime Red TADF Carbon Dots via Multi-Confinement and Phosphorescence Assisted Energy Transfer, Adv. Mater., 2023, 35, 2211858 CrossRef CAS PubMed.
- D. Lu, K. Lu, H.-T. Wen, Z. Wei, A. Bianco, G.-G. Wang and H. Zhang, Synthesis of Visible Light Excitable Carbon Dot Phosphor-Al2O3 Hybrids for Anti-Counterfeiting and Information Encryption, Small, 2023, 19, 2207046 CrossRef CAS PubMed.
- Y. Jie, D. Wang, W. Li, P. Dai, Y. Gao, F. Li, J. Li and J. Fang, Green Room-Temperature Phosphorescent Carbon Dots with a Lifetime of around 1 s, ACS Appl. Opt. Mater., 2023, 1, 1026–1032 CrossRef CAS.
- W. Sun, X. Bao, H. Wang, Z. Liu, Z. Tian, H. Li and X. Yuan, Temperature response TADF/RTP dual mode afterglow of carbon dots and flake C3N4 composites for information encryption and advanced lighting, Appl. Surf. Sci., 2023, 642, 158655 CrossRef.
- C. Wang, Y. Chen, Y. Xu, G. Ran, Y. He and Q. Song, Aggregation-Induced Room-Temperature Phosphorescence Obtained from Water-Dispersible Carbon Dot-Based Composite Materials, ACS Appl. Mater. Interfaces, 2020, 12, 10791–10800 CrossRef CAS PubMed.
- X. Guo, H. Zhang, L. Fu, K. Jian and X. Zhao, Carbon Dot-Based Room-Temperature
Phosphorescent Materials for Information Encryption, ACS Appl. Nano Mater., 2023, 6, 15597–15605 CrossRef CAS.
- K. Shao, H. Zhang, Q. Ling, W. Xie, D. Gu, Y. Teng, X. Yuan, S. Ye and Z. Pan, Developing single silane-derived delayed fluorescent carbon dots as donors for energy transfer-based aqueous-phase multicolor afterglow application, J. Mater. Chem. C, 2023, 11, 10341–10350 RSC.
- G. S. Zheng, C. L. Shen and C. Y. Niu, et al., Photooxidation triggered ultralong afterglow in carbon nanodots. Photooxidation triggered ultralong afterglow in carbon nanodots, Nat. Commun., 2024, 15, 2365 CrossRef CAS PubMed.
- L. Chen, S. Zhao, Y. Wang, S. Yu, Y. Yang and X. Liu, Long-lived room-temperature phosphorescent complex of B, N, P co-doped carbon dots and silica for afterglow imaging, Sens. Actuators, B, 2023, 390, 133946 CrossRef CAS.
- X. Yu, K. Liu, H. Zhang, B. Wang, W. Ma, J. Li and J. Yu, Carbon Dots-in-EuAPO-5 Zeolite: Triple-Emission for Multilevel Luminescence Anti-Counterfeiting, Small, 2021, 17, 2103374 CrossRef CAS PubMed.
- X. Wang, J. Zeng, S. Xie, L. Tao and X. Sun, One-step synthesis of N, B-doped carbon dots and their multifunctional applications in the detection of tin ions and gallic acid and information encryption, New J. Chem., 2023, 47, 9879–9886 RSC.
- Z. Chen, X. Liang, D. He, M. Hu and L. Wen, The synthesis and application of an excitation-dependent ultra-long lifetime room temperature phosphorescence carbon dot composite, New J. Chem., 2023, 47, 12688–12696 RSC.
- J. Qu, X. Zhang, S. Zhang, Z. Wang, Y. Yu, H. Ding, Z. Tang, X. Heng, R. Wang and S. Jing, A facile co-crystallization approach to fabricate two-component carbon dot composites showing time-dependent evolutive room temperature phosphorescence colors, Nanoscale Adv., 2021, 3, 5053–5061 RSC.
- Y. Zhu, Z. Feng, Z. Yan and X. Yang, Promoting the transfer of phosphorescence from the solid state to aqueous phase and establishing the universal real-time detection based on the smartphone imaging, Sens. Actuators, B, 2022, 371, 132529 CrossRef CAS.
- W. Wang, Q. Chang, L. Li, J.-A. Li, D. Yue and S. Su, Carbon dots with full-color-tunable room-temperature phosphorescence for photo-stimulated responsive application, J. Lumin., 2023, 263, 120017 CrossRef CAS.
- Y. Wu, X. Chen, P. Guo, E. Xue, B. Tian, K. Zheng, J. Liang and W. Wu, In situ synthesis of polychromic up/down-conversion carbon dots/YF3 composites for information encryption and security, J. Lumin., 2023, 263120013 Search PubMed.
- C. Hao, Y. Bai, Z. Chen, F. Geng, J. Qin, T. Zhou and F. Feng, Ultralong lifetime room-temperature phosphorescence in aqueous medium from silica confined polymer carbon dots for autoluminescence-free bioimaging and multilevel information encryption, Dyes Pigm., 2022, 197, 109890 CrossRef CAS.
- X. Wang, S. Wang, Y. Huang, L. Huang, J. Sun and Z. Lin, Full-color Persistent Room-temperature Phosphorescence from Carbon Dot Composites Based on a Single Nonaromatic Carbon Source, Chem. - Asian J., 2023, 18, e202201027 CrossRef CAS PubMed.
- Y. Ni, P. Zhou, Q. Jiang, Q. Zhang, X. Huang and Y. Jing, Room-temperature phosphorescence based on chitosan carbon dots for trace water detection in organic solvents and anti-counterfeiting application, Dyes Pigm., 2022, 197, 109923 CrossRef CAS.
- Z. Song, Y. Liu, X. Lin, Z. Zhou, X. Zhang, J. Zhuang, B. Lei and C. Hu, Multiemissive Room-Temperature Phosphorescent Carbon Dots@ZnAl2O4 Composites by Inorganic Defect Triplet-State Energy Transfer, ACS Appl. Mater. Interfaces, 2021, 13, 34705–34713 CrossRef CAS PubMed.
- Q. Li, Y. Li, S. Meng, J. Yang, Y. Qin, J. Tan and S. Qu, Achieving 46% efficient white-light emissive carbon dot-based materials by enhancing phosphorescence for single-component white-light-emitting diodes, J. Mater. Chem. C, 2021, 9, 6796–6801 RSC.
- F. Zhao, T. Zhang, Q. Liu and C. Lü, Aphen-derived N-doped white-emitting carbon dots with room temperature phosphorescence for versatile applications, Sens. Actuators, B, 2020, 304, 127344 CrossRef CAS.
- Q. Wu, L. Wang, Y. Yan, S. Li, S. Yu, J. Wang and L. Huang, Chitosan-Derived Carbon Dots with Room-Temperature Phosphorescence and Energy Storage Enhancement Properties, ACS Sustainable Chem. Eng., 2022, 10, 3027–3036 CrossRef CAS.
- K. Shao, H. Zhang, Q. Ling, W. Xie, D. Gu, Y. Teng, X. Yuan, S. Ye and Z. Pan, Developing single silane-derived delayed fluorescent carbon dots as donors for energy transfer-based aqueous-phase multicolor afterglow application, J. Mater. Chem. C, 2023, 11, 10341–10350 RSC.
- C. Li, H. Liang, S. Bai, J. Zhu, Z. Chen, G. Yang and Y. Zhu, Efficient color-tunable room temperature phosphorescence through carbon dot confinement in urea crystals, J. Lumin., 2023, 254, 119497 CrossRef CAS.
- M. Fu, L. Lin, X. Wang and X. Yang, Hydrogen bonds and space restriction promoting long-lived room-temperature phosphorescence and its application for white light-emitting diodes, J. Colloid Interface Sci., 2023, 639, 78–86 CrossRef CAS PubMed.
- S. Han, G. Lian, X. Zhang, Z. Cao, Q. Wang, D. Cui and C.-P. Wong, Boron nitride dots In-situ embedded in a B2O3 matrix with the long lifetime Room-Temperature phosphorescence in dry and wet states, Chem. Eng. J., 2021, 417, 129175 CrossRef CAS.
- L. Zhai, X.-M. Ren and Q. Xu, Carbogenic π-conjugated domains as the origin of afterglow emissions in carbon dot-based organic composite films, Mater. Chem. Front., 2021, 5, 4272–4279 RSC.
- T. Chao, J. Wang, X. Dong, J. Ren, H. Zhang, R. Song and Z. Xie, Defects and Structural Limitation-Induced Carbon Dots-Silica Hybrid Materials with Ultralong Room Temperature Phosphorescence, J. Phys. Chem. Lett., 2022, 13, 9558–9563 CrossRef CAS PubMed.
- H. Cheng, S. Chen, M. Li, Y. Lu, H. Chen, X. Fang, H. Qiu, W. Wang, C. Jiang and Y. Zheng, Ultra-stable dual-color phosphorescence Carbon-Dot@Silica material for advanced anti-counterfeiting, Dyes Pigm., 2023, 208, 110827 CrossRef.
- Z. Feng, J. Wang, X. Chen, J. Liu, Y. Zhu and X. Yang, Employing metformin-directed carbon dots with room-temperature phosphorescent towards the dual-channel detection of L-tryptophan, Colloids Surf., B, 2022, 210, 112236 CrossRef CAS PubMed.
- Z. Zhou, Z. Song, J. Liu, B. Lei, J. Zhuang, X. Zhang, Y. Liu and C. Hu, Energy Transfer Mediated Enhancement of Room-Temperature Phosphorescence of Carbon Dots Embedded in Matrixes, Adv. Opt. Mater., 2022, 10, 2100704 CrossRef CAS.
- Y. Zhang, Q. Long, Y. Ding, C. Huang and M. Guo, Carbonized polymer dots-based composites with solution and solid-state room temperature phosphorescence for white light-emitting diodes, Carbon, 2024, 217, 118607 CrossRef CAS.
- P. Wang, D. Zheng, S. Liu, M. Luo, J. Li, S. Shen, S. Li, L. Zhun and Z. Chen, Producing long afterglow by cellulose confinement effect: A wood-inspired design for sustainable phosphorescent materials, Carbon, 2021, 171, 946–952 CrossRef CAS.
- B. Wang, Z. Sun, J. Yu, G. I. N. Waterhouse, S. Lu and B. Yang, Cross-linking enhanced room-temperature phosphorescence of carbon dots, SmartMat, 2022, 3, 337–348 CrossRef CAS.
- K. Jiang, Y. Wang, C. Lin, L. Zheng, J. Du, Y. Zhuang, R. Xie, Z. Li and H. Lin, Enabling robust and hour-level organic long persistent luminescence from carbon dots by covalent fixation, Light: Sci. Appl., 2022, 11, 80 CrossRef CAS PubMed.
- Y. Di, W. Liu, S. Shi, T. Wu, M. Wang and X. Liu, One-step synthesis of color-tunable carbon dots-based organic long persistent luminescence materials, Chem. Eng. J., 2024, 479, 147589 CrossRef CAS.
- J. Shen, B. Xu, Z. Wang, J. Zhang, W. Zhang, Z. Gao, X. Wang, C. Zhu and X. Meng, Aggregation-induced room temperature phosphorescent carbonized polymer dots with wide-range tunable lifetimes for optical multiplexing, J. Mater. Chem. C, 2021, 9, 6781–6788 RSC.
- Z. Song, Y. Shang, Q. Lou, J. Zhu, J. Hu, W. Xu, C. Li, X. Chen, K. Liu, C.-X. Shan and X. Bai, A Molecular Engineering Strategy for Achieving Blue Phosphorescent Carbon Dots with Outstanding Efficiency above 50%, Adv. Mater., 2023, 35, 2207970 CrossRef CAS PubMed.
- B. Lü, Q. Gao, P. Li, J. Rao, Z. Lv, M. Shi, Y. Hu, X. Hao, G. Chen, M. Yin and F. Peng, Natural ultralong hemicelluloses phosphorescence, Cell Rep. Phys. Sci., 2022, 3, 101015 CrossRef.
- Z. Wang, L. Qu, L. Gao, X. Zheng, Y. Zheng, Y. Zhu, J. Xia, Y. Zhang, C. Wang, Y. Li and C. Yang, Regulation of Irradiation-Dependent Long-Lived Room Temperature Phosphorescence by Controlling Molecular Structures of Chromophores and Matrix, Adv. Opt. Mater., 2022, 10, 2200481 CrossRef CAS.
- W. Shi, J. Yao, L. Bai and C. Lu, Defect-Stabilized Triplet State Excitons: Toward Ultralong Organic Room-Temperature Phosphorescence, Adv. Funct. Mater., 2018, 28, 1804961 CrossRef.
- W. Shi, R. Wang, J. Liu, F. Peng, R. Tian and C. Lu, Time-dependent Phosphorescence Color of Carbon Dots in Binary Salt Matrices through Activations by Structural Confinement and Defects for Dynamic Information Encryption, Angew. Chem., Int. Ed., 2023, 62, e202303063 CrossRef CAS PubMed.
- L. Zhang, X. Chen and Y. Hu, Pyrolysis of Al-Based Metal-Organic Frameworks to Carbon Dot-Porous Al2O3 Composites with Time-Dependent Phosphorescence Colors for Advanced Information Encryption, Small, 2023, 2305185 Search PubMed.
- M. Shi, Q. Gao, J. Rao, Z. Lv, M. Chen, G. Chen, J. Bian, J. Ren, B. Lü and F. Peng, Confinement-Modulated Clusterization-Triggered Time-Dependent Phosphorescence Color from Xylan-Carbonized Polymer Dots, J. Am. Chem. Soc., 2023, 146, 1294–1304 CrossRef PubMed.
- Z. Han, P. Li, Y. Deng and H. Li, Reversible and color-variable afterglow luminescence of carbon dots triggered by water for multi-level encryption and decryption, Chem. Eng. J., 2021, 415, 128999 CrossRef CAS.
- Q. Gao, M. Shi, Z. Lü, Q. Zhao, G. Chen, J. Bian, H. Qi, J. Ren, B. Lü and F. Peng, Large-Scale Preparation for Multicolor Stimulus-Responsive Room-Temperature Phosphorescence Paper via Cellulose Heterogeneous Reaction, Adv. Mater., 2023, 35, 2305126 CrossRef CAS PubMed.
- L. Yang, Q. Zhang, Y. Huang, C. Luo, Z. Quan, H. Li, S. Sun and Y. Xu, A sequential dual-lock strategy for generation of room-temperature phosphorescence of boron doped carbon dots for dynamic anti-counterfeiting, J. Colloid Interface Sci., 2023, 632, 129–139 CrossRef CAS PubMed.
- M. Xu, C. Dong, J. Xu, S. Rehman, Q. Wang, V. Osipov, K. Jiang, J. Wang and H. Bi, Fluorinated carbon dots/carboxyl methyl cellulose sodium composite with a temperature-sensitive fluorescence/phosphorescence applicable for anti-counterfeiting marking, Carbon, 2022, 189, 459–466 CrossRef CAS.
- Y. Liu, X. Huang, Z. Niu, D. Wang, H. Gou, Q. Liao, K. Xi, Z. An and X. Jia, Photo-induced ultralong phosphorescence of carbon dots for thermally sensitive dynamic patterning, Chem. Sci., 2021, 12, 8199–8206 RSC.
- Q. Li, Z. Zhao, S. Meng, Y. Li, Y. Zhao, B. Zhang, Z. Tang, J. Tam and S. Qu, Ultra-strong phosphorescence with 48% quantum yield from grinding treated thermal annealed carbon dots and boric acid composite, SmartMat, 2022, 3, 260–268 CrossRef CAS.
- R. Li, Y. Wang, Q. Li and G. Sun, Photo-stimuli responsive phosphorescence from carbon dots in porous gelatin, J. Lumin., 2023, 257, 119725 CrossRef CAS.
- J. Gao, K. Zhang, S. Huang, L. Tang, L. Chen, R. He, M. Li and W. Shen, Composite Carbon Dot Materials with Long Room Temperature Phosphorescence in Solid and Liquid Environments for Encryption Technologies and Biological Detection, ACS Appl. Nano Mater., 2024, 7, 6109–6119 CrossRef CAS.
- J. Chen, J. Tan, P. Liang, C. Wu, Z. Hou, K. Shen, B. Lei, C. Hu, X. Zhang, J. Zhuang, L. Sun, Y. Liu and M. Zheng, Dynamic Room Temperature Phosphorescence of Silane-Functionalized Carbon Dots Confining within Silica for Anti-Counterfeiting Applications, Small, 2023, 2306323 Search PubMed.
- Y. Jie, Y. Gao, D. Wang, F. Li, R. Chen, Y. Feng, W. Li and J. Fang, Modulating multi-color room temperature phosphorescence emission for carbon dot composites with ultralong lifetime, New J. Chem., 2023, 47, 16659–16665 RSC.
- J. Liu, Y. Luo, Z. Ran, F. Wang, M. Sun, Y. Luo, J. Zhuang, X. Zhang, B. Lei, Y. Liu and C. Hu, Calcination temperature tuning of RTP and TADF with wide range of emission color from carbon dots confined in Al2O3, Chem. Eng. J., 2023, 474, 145597 CrossRef CAS.
- Y. Ding, X. Wang, M. Tang and H. Qiu, Tailored Fabrication of Carbon Dot Composites with Full-Color Ultralong Room-Temperature Phosphorescence for Multidimensional Encryption, Adv. Sci., 2021, 9, 2103833 CrossRef PubMed.
- P. Long, Y. Feng, C. Cao, Y. Li, J. Han, S. Li, C. Peng, Z. Li and W. Feng, Self-Protective Room-Temperature Phosphorescence of Fluorine and Nitrogen Codoped Carbon Dots, Adv. Funct. Mater., 2018, 28, 1800791 CrossRef.
- H. Wang, L. Zhou, H.-M. Yu, X.-D. Tang, C. Xing, G. Nie, H. Akafzade, S.-Y. Wang and W. Chen, Exploration of Room-Temperature Phosphorescence and New Mechanism on Carbon Dots in a Polyacrylamide Platform and their Applications for Anti-Counterfeiting and Information Encryption, Adv. Opt. Mater., 2022, 10, 2200678 CrossRef CAS.
- H. Wang, B. Shi, H. Yu, S. Yang, G. Nie, S. Wang and W. Chen, Visible light-excited full-color phosphorescent material realized by carbon dots dispersed in polyacrylamide and applied to anti-counterfeiting, Mater. Today Adv., 2023, 20, 100429 CrossRef CAS.
- Y. Zhang, M. Li and S. Lu, Rational Design of Covalent Bond Engineered Encapsulation Structure toward Efficient, Long-Lived Multicolored Phosphorescent Carbon Dots, Small, 2023, 19, 2206080 CrossRef CAS PubMed.
- J. Zhang, B. He, Y. Hu, P. Alam, H. Zhang, J. W. Y. Lam and B. Tang, Stimuli-Responsive AIEgens, Adv. Mater., 2021, 33, 2008071 CrossRef CAS PubMed.
- Y. Wang, S. Zhou, S. Pan, X. Sun, J. Zhou and H. Li, Color-Tunable Carbon Dots with Aggregation-Induced Emission Constructed by FRET between Surface Luminescence Centers, Adv. Opt. Mater., 2023, 12, 2301486 CrossRef.
- Z.-Z. Ding, C.-L. Shen, J.-F. Han, G.-S. Zheng, Q.-C. Ni, R.-W. Song, K.-K. Liu, J.-H. Zang, L. Dong, Q. Lou and C.-X. Shan, In Situ Confining Citric Acid-Derived Carbon Dots for Full-Color Room-Temperature Phosphorescence, Small, 2023, 19, 2205916 CrossRef CAS PubMed.
- Q. Li, D. Cheng, H. Gu, D. Yang, Y. Li, S. Meng, Y. Zhao, Z. Tang, Y. Zhang, J. Tan and S. Qu, Aggregation-induced color fine-tunable carbon dot phosphorescence covering from green to near-infrared for advanced information encryption, Chem. Eng. J., 2023, 462, 142339 CrossRef CAS.
- X. Yu, K. Liu, B. Wang, H. Zhang, Y. Qi and J. Yu, Time-Dependent Polychrome Stereoscopic Luminescence Triggered by Resonance Energy Transfer between Carbon Dots-in-Zeolite Composites and Fluorescence Quantum Dots, Adv. Mater., 2023, 35, 2208735 CrossRef CAS PubMed.
- L. Mo, H. Liu, Z. Liu, X. Xu, B. Lei, J. Zhuang, Y. Liu and C. Hu, Cascade Resonance Energy Transfer for the Construction of Nanoparticles with Multicolor Long Afterglow in Aqueous Solutions for Information Encryption and Bioimaging, Adv. Opt. Mater., 2022, 10, 2102666 CrossRef CAS.
- H. Zhao, G. Liu, S. You, F. V. A. Camargo, M. Zavelani-Rossi, X. Wang, C. Sun, B. Liu, Y. Zhang, G. Han, A. Vomiero and X. Gong, Gram-scale synthesis of carbon quantum dots with a large Stokes shift for the fabrication of eco-friendly and high-efficiency luminescent solar concentrators, Energy Environ. Sci., 2021, 14, 396–406 RSC.
- N. Shauloff, R. Bisht, Y. Turkulets, R. Manikandan, A. Morag, A. Lehrer, J. H. Baraban, L. Shalish and R. Jelinek, Multispectral and Circular Polarization-Sensitive Carbon Dot-Polydiacetylene Capacitive Photodetector, Small, 2023, 19, 2206519 CrossRef CAS PubMed.
- X. Gong, W. Li, Y. Wu, X. Li, Y. Ma, H. Wang and M. Wu, A rational strategy for directly synthesizing strongly yellow solid-state fluorescent-emitting carbon nanodots composite microparticles by structure regulation, Mater. Today Adv., 2023, 20, 100428 CrossRef CAS.
- J. Li, X. Zhao and X. Gong, Rational Design of Dual-Mode Emitting Carbon Dots to Exploit the Synergistic Effect of Matrices for High-Efficiency WLEDs and Anti-Counterfeiting, Adv. Opt. Mater., 2023, 2302297 Search PubMed.
- S. Ma, H. Ma, K. Yang, Z. A. Tan, B. Zhao and J. Deng, Intense circularly polarized fluorescence and room-temperature phosphorescence in carbon dots/chiral helical polymer composite films, ACS Nano, 2023, 17, 6912–6921 CrossRef CAS PubMed.
- P. Liang, Y. Zheng, X. Zhang, H. Wei, X. Xu, X. Yang, H. Lin, C. Hu, X. Zhang, B. Lei, W.-Y. Wong, Y. Liu and J. Zhuang, Carbon Dots in Hydroxy Fluorides: Achieving Multicolor Long-Wavelength Room-Temperature Phosphorescence and Excellent Stability via Crystal Confinement, Nano Lett., 2022, 22, 5127–5136 CrossRef CAS PubMed.
- Q. Li, S. Meng, Y. Li, D. Cheng, H. Gu, Z. Zhao, Z. Tang, J. Tan and S. Qu, Surface ionization-induced tunable dynamic phosphorescence colors from carbon dots on paper for dynamic multimode encryption, Carbon, 2022, 195, 191–198 CrossRef CAS.
- S. Chen, X. Li, M. Bai, S. Q. Shi, J. T. Aladejana, J. Cao and J. Li, Oyster-inspired carbon dots-functionalized silica and dialdehyde chitosan to fabricate a soy protein adhesive with high strength, mildew resistance, and long-term water resistance, Carbohydr. Polym., 2023, 319, 121093 CrossRef CAS PubMed.
- X. Chen, Z. Song, B. Yuan, X. Li, S. Li, T. T. Nguyen, M. Guo and Z. Guo, Fluorescent carbon dots crosslinked cellulose Nanofibril/Chitosan interpenetrating hydrogel system for sensitive detection and efficient adsorption of Cu(II) and Cr(VI), Chem. Eng. J., 2022, 430, 133154 CrossRef CAS.
- X. Zheng, Y. Zhong, H. Chu, Y. Yu, Y. Zhang, J. S. Chin, D. L. Becker, X. Su and X. J. Loh, Carbon Dot-Doped Hydrogel Sensor Array for Multiplexed Colorimetric Detection of Wound Healing, ACS. Appl. Mater. Interfaces, 2023, 15, 17675–17687 CrossRef CAS PubMed.
- T. Li, X. Li, Y. Zheng, P. Zhu, C. Zhang, K. Zhang and J.-J. Xu, Phosphorescent Carbon Dots as Long-Lived Donors to Develop an Energy Transfer-Based Sensing Platform, Anal. Chem., 2023, 95, 2445–2451 CrossRef CAS PubMed.
- T. Chen, T. Yao, H. Peng, A. K. Whittaker, Y. Li, S. Zhu and Z. Wang, An Injectable Hydrogel for Simultaneous Photothermal Therapy and Photodynamic Therapy with Ultrahigh Efficiency Based on Carbon Dots and Modified Cellulose Nanocrystals, Adv. Funct. Mater., 2021, 31, 2106079 CrossRef CAS.
- H. Zhang, Q. Li, S. Wang, X. Yu, B. Wang, G. Chen, L. Ren, J. Li, M. Jin and J. Yu, Confining carbon dots in amino-functionalized mesoporous silica: n → π* interaction triggered deep-red solid-state fluorescence, Nano Res., 2023, 16, 4170–4177 CrossRef CAS.
- Y. Liang, Q. Cao, K.-K. Liu, X.-Y. Peng, L.-Z. Sui, S.-P. Wang, S.-Y. Song, X.-Y. Wu, W.-B. Zhao, Y. Deng, Q. Lou, L. Dong and C.-X. Shan, Phosphorescent Carbon-Nanodots-Assisted Förster Resonant Energy Transfer for Achieving Red Afterglow in an Aqueous Solution, ACS Nano, 2021, 15, 16242–16254 CrossRef CAS PubMed.
Footnote |
† These authors contributed equally. |
|
This journal is © The Royal Society of Chemistry 2024 |