DOI:
10.1039/D3NA01125A
(Review Article)
Nanoscale Adv., 2024,
6, 3009-3028
Strategies for enhanced gene delivery to the central nervous system
Received
17th December 2023
, Accepted 12th April 2024
First published on 25th April 2024
Abstract
The delivery of genes to the central nervous system (CNS) has been a persistent challenge due to various biological barriers. The blood–brain barrier (BBB), in particular, hampers the access of systemically injected drugs to parenchymal cells, allowing only a minimal percentage (<1%) to pass through. Recent scientific insights highlight the crucial role of the extracellular space (ECS) in governing drug diffusion. Taking into account advancements in vectors, techniques, and knowledge, the discussion will center on the most notable vectors utilized for gene delivery to the CNS. This review will explore the influence of the ECS – a dynamically regulated barrier-on drug diffusion. Furthermore, we will underscore the significance of employing remote-control technologies to facilitate BBB traversal and modulate the ECS. Given the rapid progress in gene editing, our discussion will also encompass the latest advances focused on delivering therapeutic editing in vivo to the CNS tissue. In the end, a brief summary on the impact of Artificial Intelligence (AI)/Machine Learning (ML), ultrasmall, soft endovascular robots, and high-resolution endovascular cameras on improving the gene delivery to the CNS will be provided.
1. Introduction
Gene therapy holds significant potential for treating CNS diseases, offering innovative approaches to address genetic and acquired neurological disorders. Advances in gene therapy techniques have opened new avenues for the development of targeted and personalized treatments. Gene therapy allows for the precise targeting of specific genes or genetic pathways associated with CNS diseases. This precision can be crucial for addressing the underlying causes of various neurological disorders, including genetic mutations or dysregulation of specific genes. Gene therapy is particularly promising for monogenic disorders, where a single mutated gene is responsible for the disease. By introducing a functional copy of the gene or silencing the mutated gene, gene therapy aims to correct the underlying genetic defect. Gene therapy holds potential for treating neurodegenerative diseases such as Alzheimer's, Parkinson's, and Huntington's disease. Beyond monogenic diseases, gene therapy also holds promise for transforming the pathological diseases in the CNS that impact larger patient populations, such as stroke, spinal cord injury, and tumors (Fig. 1A–C). Strategies involve delivering therapeutic genes to modulate protein expression, enhance neuroprotection, or reduce toxic protein accumulation. Gene therapies also facilitate personalized medicine by tailoring treatments to an individual's genetic profile.
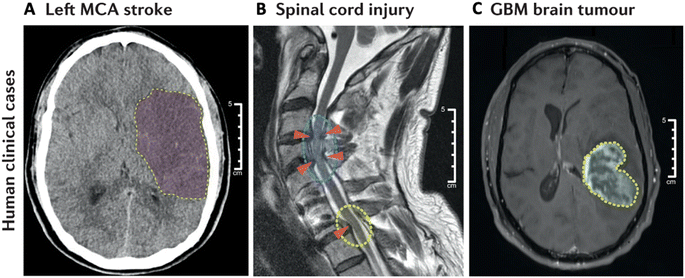 |
| Fig. 1 Representative indications in the CNS. Diagnostic images of (A) middle cerebral artery (MCA) stroke, (B) spinal cord injury (SCI), and (C) glioblastoma multiforme (GBM) brain tumor. Reproduced from ref. 1 with permission from Spring Nature Publishing Group, copyright 2022. | |
Several gene therapies for CNS diseases have reached advanced clinical stages, and some have received regulatory approvals.2 These treatments include Zolgensma for SMN1 spinal muscular atrophy (SMA), Luxturna® for RPE65 inherited retinal dystrophy, and others, marking significant milestones in the field.3 As a novel form of gene therapy, a gene editing system (CRISPR/Cas9, zinc-finger, etc.) allows for precise modification (disrupt, delete, insert, etc.) of genes, opening new possibilities for correcting genetic mutations associated with CNS diseases.4
Despite remarkable progress in gene therapy for CNS diseases, challenges persist, including ensuring safe gene delivery, enhancing the cell specificity, achieving widespread gene distribution, and addressing the need for redosing. Ongoing research and clinical trials continue to refine gene therapy approaches, offering hope for novel and effective treatments across a spectrum of CNS disorders. Gene therapy requires tissue and cell type specific delivery of DNA sequences and/or gene editing machineries (can be RNA format or protein) via biologically or chemically engineered vectors. Advances in gene delivery vectors and techniques are aimed at overcoming the challenge of the blood–brain barrier (BBB)5 and blood-spinal cord barrier (BSCB). Both viral vectors and non-viral nanoparticles have been designed and engineered to enhance BBB/BSCB penetration as demonstrated in many preclinical models.6 Notably, the development of viral vectors, such as adeno-associated viruses (AAVs),7 represents a significant breakthrough due to their ability to efficiently transduce neurons and achieve sustained gene expression.2 We will summarize the challenges by highlighting the key biological barriers that affect the clearance, penetration, diffusion and release of therapeutics in the CNS (Fig. 2).
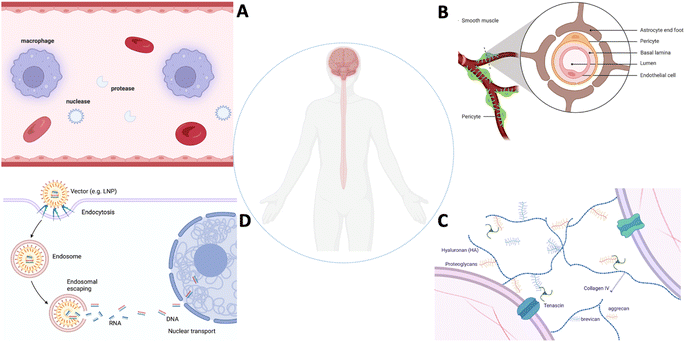 |
| Fig. 2 Biological barriers in the CNS. (A) Systemic barrier, (B) blood–brain barrier, (C) extracellular barrier, and (D) intracellular barrier. | |
Gene delivery to the CNS is a complex and challenging task due to the unique anatomical and biological barriers that protect the brain and spinal cord (Fig. 2A–D). These challenges can significantly impact the effectiveness of therapeutic agents targeting the CNS.8 Some of the key challenges include: (1) systemic clearance: immune cells (macrophages, monocytes, etc.) tend to remove systemically administrated vectors. Endogenous nuclease and protease can cleave nucleic acid payloads (DNA and RNA). The majority of the gene delivery vectors can be cleared quickly, and thus the vectors capable of interacting with the CNS tissue can be limited. (2) BBB/BSCB: the BBB is a highly selective barrier that limits the passage of drugs and other substances from the bloodstream into the brain. It is composed of tightly packed endothelial cells with tight junctions, restricting the entry of large molecules and pathogens. While this barrier is essential for maintaining the brain's homeostasis, it also poses a significant challenge for drug delivery. Similar to the BBB, the BSCB restricts the entry of substances into the spinal cord. It is a barrier that must be considered when designing drug delivery systems for spinal cord-related indications. The BBB/BSCB blocks more than 99% of systemically administered small molecules with a molecular weight <500 Da and nearly 100% of molecules with a molecular weight >500 Da from accessing the CNS parenchyma.9 (3) Efflux transporters: the presence of efflux transporters at the BBB actively pumps drugs out of the brain, reducing their concentration within the CNS. P-glycoprotein is a prominent efflux transporter that plays a role in drug resistance. (4) Biological variability: the variability in individual patient responses to drug treatment, including variations in the BBB/BSCB permeability, genetic background, disease stages and pathology, can complicate drug delivery strategies. (5) Neuroinflammation: in certain CNS disorders, such as neuroinflammatory conditions, the permeability of the BBB/BSCB may be altered, but this can be a double-edged sword. While it may facilitate drug entry, it can also contribute to the progression of the disease. (6) Limited permeability: many drugs, even when they can cross the BBB or BSCB, may not penetrate into the brain or spinal cord tissues effectively inside the complex extracellular microenvironment due to factors such as their size, lipophilicity, and charge. (7) Intracellular uptake: even if a drug successfully crosses the barriers, it may face challenges in reaching the target cells within the CNS. Intracellular uptake can be influenced by various factors, including the drug's chemical properties and the specific cell types involved. (8) Region-specific targeting: achieving precise targeting of drugs to specific regions within the CNS is challenging. Different areas of the brain and spinal cord may have distinct physiological, anatomic, and functional characteristics, and targeting drugs to specific cell types is an ongoing challenge.
The discovery of the CRISPR system and advancements in protein engineering technology have raised hopes for therapeutic gene editing as a novel treatment modality beyond conversional gene addition driven by the episomal expression of the protein coded by a DNA sequence delivered by various vectors in cells (Fig. 3A).10 The emergence of gene editing for therapeutic development induced the need to deliver complicated and multiplex cargo, such as RNA and DNA (Fig. 3B).11 Rational delivery of protein, mRNA, gRNA, and DNA through separate vectors or in a single vector requires complicated design and optimization of the delivery systems. Addressing these challenges necessitates innovative drug delivery approaches, including nanotechnology, prodrugs, and targeted delivery systems. Researchers actively explore strategies to enhance drug delivery to the CNS, aiming to minimize side effects and optimize therapeutic outcomes. The dynamic field of CNS gene delivery continues to evolve as scientific advancements unlock new possibilities for overcoming these complex barriers with novel modalities and new approaches. In this review, we review the advancements in vector technology, BBB/BSCB overcoming approaches, and progress on understanding the impact of the extracellular environment on the gene delivery to the CNS. We will also emphasize the progress in remote-control technology for enhanced delivery to the CNS, as well as the impact and application of cutting-edge technologies, such as AI on the gene delivery to the CNS.
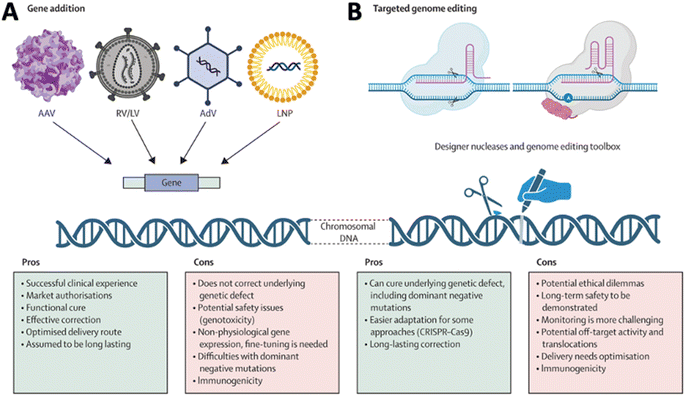 |
| Fig. 3 Comparison of (A) gene addition and (B) targeted genome editing. Reproduced from ref. 10 with permission from Lancet Publishing Group, copyright 2024. | |
2. Gene delivery vectors
2.1 Viral vectors
Viral vectors have been widely used for genetic cargo delivery due to many advantages.12 Among various viral vectors (Table 1), AAV vectors are the most advanced in vivo gene delivery system and have shown significant advancements in delivering genes to various tissues,13 including the CNS.2 AAV-based gene therapy for CNS disorders has progressed due to several key developments.14 Ongoing efforts in capsid engineering have led to the development of novel AAV capsids with improved properties, such as increased transduction efficiency, reduced immunogenicity, and enhanced ability to cross the BBB.15 Researchers have engineered AAV vectors to improve their ability to efficiently deliver genes to target cells with high specificity in the CNS.16 This includes modifications with various molecular engineering approaches to the viral capsid proteins to enhance transduction and improve cell specificity.17 This is crucial for treating various neurological disorders where specific cell populations are affected. The identification and characterization of different AAV serotypes with varying tropisms have expanded the toolkit for therapeutic applications.18 Choosing the appropriate serotype for a specific application allows for better customization of gene delivery strategies. Designing and optimizing promoters for gene expression within the CNS have improved the precision and control of transgene expression.19 This is important for ensuring that therapeutic genes are expressed at appropriate levels in target cells with minimal side effects. Strategies to minimize the immunogenic response to AAV vectors have been explored,20 as this can impact the effectiveness and safety of gene therapy.21 Immune responses can affect the duration of transgene expression and the overall success of treatment.22 The dosage of AAVs in human patents needs to be rationally applied through better understanding the AAV interactions with the human immune system and balanced by the disease conditions in patiens.23 Efforts to expand the cargo capacity of AAV vectors enable the delivery of larger therapeutic genes,24 opening up possibilities for addressing more complex genetic disorders within the CNS.25 The successful translation of AAV-based gene therapies from preclinical studies to clinical trials has demonstrated the feasibility and safety of this approach for treating various CNS disorders, including neurodegenerative diseases and genetic disorders.26 Overall, these advances in AAV technology for CNS gene delivery hold great promise for the development of effective and targeted gene therapies to address a wide range of neurological disorders.
Table 1 Summary of gene delivery vectors
|
AAV |
Adenovirus |
Lentivirus |
LNP |
PNP |
VLP |
Exosome |
3D dimension |
25 nm |
80–100 nm |
80–130 nm |
50–200 nm |
30–200 nm |
20–200 nm |
30–150 nm |
Cargo type |
DNA(ss) |
DNA(ds) |
RNA |
RNA or DNA |
RNA or DNA |
RNA or DNA |
RNA or DNA |
Packaging size |
4.6 kb |
36 kb |
9 kb |
Varies |
Varies |
Varies |
Varies |
Cell specificity |
Capsid dependent |
Capsid dependent |
Capsid dependent |
Ligand dependent |
Ligand dependent |
Ligand dependent |
Ligand dependent |
Immunogenicity |
Low–moderate |
Moderate |
Moderate |
Low |
Low |
Low |
Low |
Genome integration |
<1% |
Moderate |
Moderate |
Unknow |
Unknow |
Unknow |
Unknow |
AAV vectors are widely used for delivering CRISPR/Cas9 gene-editing tools in vivo due to their ability to efficiently transduce cells and their powerful capability of delivery DNA to the cell nucleus.27 However, limitations associated with AAVs for CRISPR/Cas9 delivery still required effort to overcome: limited cargo capacity: AAV vectors have a restricted packaging capacity, limiting the size of the genetic cargo they can carry. This constraint poses challenges when delivering large CRISPR/Cas9 components, such as long guide RNAs or Cas variants with additional functionalities. Immune response: AAV vectors can induce immune responses in the host. Immune reactions can lead to clearance of the viral vector and reduce the effectiveness of CRISPR/Cas9 delivery. Pre-existing immunity to AAVs, which can be present in a significant portion of the population due to natural exposure, can reduce the efficacy. Size constraints of promoters: the size constraints of AAV vectors also apply to the regulatory elements, such as promoters, which control the expression of Cas9 and guide RNAs. Selecting appropriate promoters that fit within the limited cargo capacity while maintaining efficient expression is a challenge. Off-target effects: AAV-mediated CRISPR/Cas9 delivery may have off-target effects, where the durable expression of the Cas9 enzyme may induce unintended genetic modifications. Optimization of the CRISPR/Cas9 system and delivery strategy is necessary to minimize off-target effects. Tropism and specificity: AAV vectors exhibit tissue tropism, showing preferences for certain cell types or tissues. Achieving precise targeting of specific cell populations can be challenging, and the vector may not efficiently transduce the desired cells within a complex tissue environment. In the CNS, achieving 100% targeting specificity to major cell types, including neurons, astrocytes, and glial cells, is still impossible. Leaked gene and protein expression in untargeted cells has been broadly observed. Integration risk into the genome: AAV vectors predominantly exist as episomes in the host cell. A low level of integration (<1%) into the host genome facilitated by the spontaneous chromosome breakage has been reported,28 which could help increase the durability of the AAV gene therapy as reported recently;29 however, the clear understanding of the impact of the insertional event requires more effort to investigate at both molecular and functional levels.30
AAVs are a promising gene delivery platform, but clinical trials continue to highlight a relatively narrow therapeutic window. Effective clinical translation is confounded by differences in AAV biology across animal species. It is still challenging to maintain the tissue tropism transduction efficiency in primates. Sequentially evolving AAV capsid libraries in mice, pigs and macaques may offer a valid approach for tackling the challenge. A study has demonstrated organ-specific targeting of AAV capsids following intravenous delivery.31 Using a Cre-transgenic-based screening platform and sequential engineering of AAV-PHP.eB between AA452 and AA460 of VP3, researchers identified capsid variants enriched in the brain, avoiding the liver in C57BL/6J mice. This targeting specificity extends to marmosets, allowing effective, non-invasive gene delivery to the marmoset brain after intravenous administration. This progress in crossing the BBB with neuronal precision in rodents and non-human primates opens new avenues for both basic research and therapeutic applications not achievable with naturally occurring serotypes.32
Recent observations have shown that the administration of AAV vectors at high doses can cause local or widespread neurotoxicity in the CNS and PNS, and the long-term consequences of this for human therapies targeting nervous tissue remain to be determined. Recent studies in non-human primates that were administered recombinant adeno-associated viruses (rAAVs) have shown lesions in the dorsal root ganglia (DRG) of unknown pathogenesis. This study further supports that DRG toxicity is associated with transgene overexpression in DRGs, with particular sensitivity at the lumbar and lumbosacral levels.33 Monitoring sensory neuropathies in the human central nervous system and high-dose IV clinical studies seem prudent to determine the functional consequences of DRG pathology.34 Guo and colleagues investigated the mechanisms by which AAVs mediate neurotoxicity following intraparenchymal injection into a mouse brain.35 Their observation that high doses of AAVs can cause localized disruption of the BBB, which allows an influx of blood and serum factors into brain parenchyma, provides important mechanistic insights into our understanding of how neurotoxicity at the site of injection is mediated by infiltrating lymphocytes. A recent study demonstrated that thrombotic microangiopathy (TMA) associated with AAV gene therapy is antibody dependent (classical pathway) and amplified by the alternative complement pathway. Optimal time points and interventions need to be identified to allow for management of immune mediated events of systemic gene therapy.36 Researchers are actively working on addressing these limitations through various strategies, including the development of novel AAV variants with improved properties, optimizing delivery protocols, and exploring alternative delivery methods to enhance the efficiency and safety of CRISPR/Cas9-mediated genome editing.
2.2 Non-viral vectors
Advancements in non-viral technologies for gene delivery to the CNS exhibit promising potential in mitigating constraints associated with viral vectors.37 Key innovations encompass the utilization of nanoparticles, including lipid nanoparticles (LNPs) and polymeric nanoparticles (PNPs), exosomes, and virus-like particles (VLPs), designed to encapsulate and shield gene payloads (Table 1). These nanocarriers exhibit the capacity to augment cellular uptake and enable controlled release of genetic payloads within the CNS.38 LNPs, specifically, have garnered attention because of their adept encapsulation and delivery of nucleic acids.39 Research suggests that LNPs can be tailored to traverse the BBB and achieve targeted delivery to specific CNS cells in preclinical models,40,41 presenting a non-viral alternative for gene therapy. Enhanced side-dependent LNP penetration to the brain parenchyma has been suggested in pathohistological conditions in preclinical models, such as brain injury where BBB integrity is interrupted.42 Synthetic polymers, exemplified by poly (lactic-co-glycolic acid) (PLGA), can form nanoparticles tailored for gene delivery, with modifications in composition aimed at improving biocompatibility and stability, and the controlled release of genetic payloads within the CNS.43 Exosomes, small extracellular vesicles, have been investigated as innate carriers for genetic material, with modified exosomes loaded with therapeutic genes directed to target cells within the CNS.44 Injectable hydrogels have emerged as non-viral carriers for gene delivery, forming three-dimensional networks that facilitate sustained release of therapeutic genes, thereby allowing for prolonged exposure and improved distribution within the CNS.45 The integration of ultrasound technology, in conjunction with microbubbles or nanoparticles, has demonstrated the capacity to enhance the permeability of the BBB, facilitating gene delivery to specific regions within the CNS.46 This non-invasive approach exhibits promise in preclinical studies. Non-viral gene delivery through intrathecal administration, involving the direct injection of therapeutic genes into cerebrospinal fluid, facilitates widespread distribution of genetic material within the CNS. These non-viral technologies confer potential advantages such as reduced immunogenicity, tunable release kinetics, and versatility in cargo delivery. Ongoing research endeavors are directed towards optimizing these methodologies for clinical applications, thereby offering alternative strategies for gene therapy in CNS disorders.38
2.2.1 LNPs.
LNPs have witnessed substantial progress in the realm of CNS drug delivery.47 These nano-sized lipid carriers show significant potential for efficiently transporting therapeutic agents to the CNS.48 Key advancements in the utilization of lipid nanoparticles for CNS applications include: enhanced BBB permeability: LNPs have been intricately engineered to augment the delivery of drugs across the BBB in small animals.6 Modifications in LNP composition and surface characteristics facilitate their passage through the BBB, predominantly through receptor-mediated transport. Suitability for nucleic acid-based therapeutics: LNPs are particularly well-suited for delivering nucleic acid-based therapeutics, such as RNA and DNA, to the CNS.49 This feature is particularly relevant for gene therapy applications aimed at treating genetic disorders or modulating gene expression in neurological diseases. Intranasal drug delivery investigations: LNPs have been explored for intranasal drug delivery to the CNS. Intranasal administration offers a non-invasive route to bypass the BBB, allowing direct targeting of the brain.50 This has the potential to improve drug access to regions affected by neurological disorders. Tailoring LNP properties: researchers are customizing LNP properties, including size, surface charge, and lipid composition, to optimize their performance for CNS applications.50 This tailored approach aims to enhance drug encapsulation, stability, and delivery efficiency. Clinical progression: some LNP-based formulations have advanced to clinical trials with an established safety profile.51,52 These trials evaluate the safety and efficacy of LNPs in human subjects, representing a pivotal step toward potential clinical applications in the CNS. The advancements in LNP technology for CNS drug delivery offer promise in addressing the challenges associated with treating neurological disorders. Ongoing research endeavors seek to further refine LNP formulations, broaden their therapeutic applications, and translate these developments into clinically viable treatments.
LNP delivery systems present notable advantages in the context of CRISPR/Cas9 gene-editing applications:52 large cargo capacity: LNPs can accommodate substantial cargo sizes, enabling the delivery of sizable CRISPR/Cas9 components like long guide RNAs or modified Cas9 variants, along with a DNA template in one packet,53 which may pose challenges with alternative delivery methods such as viral vectors. Low immunogenicity: LNPs generally provoke a lower immune response compared to viral vectors, reducing the risk of host immune reactions that could impede CRISPR/Cas9 delivery and effectiveness.54 Low pre-existing immunity: LNPs are less likely to encounter pre-existing immunity in the host population compared to certain viral vectors, decreasing the likelihood of immune responses that might hinder the delivery efficiency of CRISPR/Cas9 components. Targeted delivery: LNPs can be tailored to target specific cell types or tissues by modifying their surface properties, allowing versatile applications across diverse cell populations within complex tissue environments.55,56 Customizable and easily synthesized: LNPs are relatively easy to synthesize with microfluidics and can be readily modified to incorporating the target elements.57 This versatility facilitates the development of customized LNPs for specific CRISPR/Cas9 applications, offering researchers the ability to tailor their properties as needed. Genomic safety: unlike certain viral vectors, LNPs delivers RNA cargo that does not integrate into the host genome,58 reducing the risk of insertional mutagenesis and enhancing the safety profile of LNP-mediated CRISPR/Cas9 delivery.
Despite these advantages, challenges and limitations persist in LNP delivery systems for CRISPR/Cas9 gene editing: systemic distribution challenges: achieving uniform distribution of LNPs and CRISPR/Cas9 components throughout the target tissue or organ when administered systemically poses a challenge, potentially leading to suboptimal gene-editing outcomes.40 Liver is the primary organ that sinks the majority of LNPs upon systemic administration.51In vivo delivery efficiency can vary among different tissues and organs.59 Inefficient delivery to specific cell types: designing LNPs for precise cellular targeting demands a profound understanding of the target tissue, cell-type specific receptor expression and cross interaction pattern, and sophisticated surface modifications to enhance cell-specific delivery.60 Immunogenicity and toxicity: LNPs may induce immune responses or exhibit toxicity,61 particularly when administered systemically, potentially affecting the safety and efficacy of CRISPR/Cas9 delivery. Limited in vivo stability: LNPs may face challenges in maintaining stability in vivo, especially during circulation in the bloodstream, impacting the efficiency of CRISPR/Cas9 delivery. Limited escaping efficiency from endosomes: only a fraction of <2% LNPs can release the cargo from endosomes with the best performing lipid composition and the most optimal formulation.62,63 Overcoming barriers such as the extracellular matrix and cellular uptake challenges is essential for improving the overall success of LNP-mediated CRISPR/Cas9 delivery in vivo. Researchers are actively addressing these challenges through ongoing studies focused on optimizing LNP formulations, improving targeting strategies, and enhancing the overall safety and efficacy of LNP-mediated CRISPR/Cas9 gene editing, contributing to the advancement of CRISPR/Cas9-based therapeutic interventions.64
2.2.2 PNPs.
PNPs have demonstrated significant progress in drug delivery to the CNS, particularly in overcoming challenges posed by the BBB and enabling targeted therapy.64 Notable PNP advantages include: enhanced BBB penetration: similar to LNPs, some PNPs can be chemically tailored to enhance drug penetration across the BBB. Surface modifications, including ligands interacting with specific BBB receptors, facilitate targeted and efficient drug delivery to the CNS.65 Tunability and customization: PNPs offer a high degree of tunability. Researchers can customize their size, surface charge, and composition to optimize drug encapsulation, release kinetics, and biodistribution, tailoring them for specific CNS applications.66 Biocompatible and biodegradable polymers: advances in polymer chemistry have resulted in the development of biocompatible and biodegradable polymers for nanoparticle construction. These polymers minimize toxicity concerns and enable controlled release of therapeutic agents within the optimal therapeutic window in the CNS.67 Sustained release: PNPs can be engineered to provide sustained release of therapeutic agents, extending drug availability in the CNS and reducing the need for frequent administrations, particularly beneficial for chronic conditions. Hydrogel-based polymer nanoparticles have garnered attention for CNS drug delivery for achieving sustainable and controlled release of drugs. They can form hydrogels in situ, offering sustained drug release and improved residence time at the target site.68 Intranasal drug delivery: PNPs are explored for intranasal drug delivery to the CNS, providing a non-invasive route to bypass the BBB.69 Polymers enhance drug stability and absorption, improving access to the brain, but this approach may require a more frequent and complicated dosing plan. For rapid and aggressive diseases, the efficacy can be very limited. Active targeting with ligands: PNPs can be functionalized with ligands for active targeting,70 for example, glucose transporter-1 (GLUT1), with high expression on the endothelial cells of the BBB, and facilitate transport of the drugs to the CNS tissue via transcytosis. Multifunctionality: advances in PNP design enable the incorporation of multiple functions within a single carrier. Responsive PNP can alter their properties in response to environmental cues, allowing for triggered drug release and enhancing precision in therapeutic agent delivery to the brain.71 Ongoing research aims to refine nanoparticle formulations, enhance their clinical applicability, and broaden their use in treating various neurological conditions, contributing to continuous innovation in drug delivery to the CNS.
In the context of gene editing in the CNS, PNPs exhibit unique and significant potential with customizable properties, including size, surface charge and functional modification.72 Advances have extended the applications of PNP in gene editing for many neurological conditions in preclinical models. The prospect of multifunctionality and ongoing research endeavors seeks to optimize formulations, addressing challenges such as off-target effects, cell-type specificity, and immunogenicity.
2.2.3 VLPs.
VLPs are synthetic particles engineered to replicate the structural features of viruses while lacking infectious genetic material, ensuring their safety for therapeutic applications.64 VLPs can be precisely engineered for targeted delivery to specific cells or tissues within the CNS. Their ability to mimic virus entry mechanisms enables efficient internalization and release of payloads, including drugs or nucleic acids, into the intracellular space. This characteristic enhances their ability to traverse biological barriers, including the BBB. Engineered VLPs can aid the transport of therapeutic agents across the BBB.73 Surface modifications, such as the incorporation of glycoprotein, peptides or antibodies, improve the interaction of VLPs with the BBB, enhancing their ability to traverse this protective barrier. Ongoing research endeavors continue to explore the full potential of VLPs in CNS drug delivery.
VLPs show significant promise in the field of gene editing in the CNS. In gene editing, engineered VLPs offer a versatile platform for packaging and delivering therapeutic ribonucleoprotein complexes (RNPs), such as base editors and Cas9 nuclease. By combining the strengths of viral and non-viral delivery strategies, this innovative approach has resulted in significant advancements.74 Through meticulous engineering of VLP architecture, in a recent study, new generation VLPs have been achieved capable of packaging base editor RNPs at a factor of 16-fold more compared to earlier designs based on previously reported VLPs. These VLPs exhibit remarkable efficiency in base editing across various cell types, with minimal off-target effects. Moreover, they demonstrate a 4.7-fold enhancement in Cas9 nuclease-mediated indel formation compared to previously reported Cas9-VLPs. Remarkably, single injections of VLPs into mice have led to efficient base editing of target genes in multiple organs, including the brain. These findings underscore the potential of VLPs as a promising platform for transiently delivering gene editing agents in vivo, offering therapeutically relevant efficiencies while mitigating the risk of off-target editing or DNA integration. Furthermore, the versatility of this platform extends beyond gene editing applications, holding promise for the efficient delivery of other proteins and RNPs in vivo, paving the way for safer and more effective treatment strategies for various genetic disorders and diseases. From the therapeutic development perspective, large scale manufacture at high quality would need to be optimized to fulfill the promise in clinical applications.
2.2.4 Exosomes.
Exosomes, small extracellular vesicles released by cells, play a pivotal role in mediating gene delivery to the CNS.44 These nanosized vesicles inherently carry genetic material, specifically RNA, making them a prospective conduit for therapeutic gene delivery. The customization of exosomes through genetic engineering allows for the encapsulation of therapeutic genes, enabling targeted delivery to specific cells within the CNS.75 Engineered exosomes exhibit notable potential in facilitating precise and targeted gene delivery, overcoming inherent challenges such as the BBB. Ongoing scientific study seeks to harness the inherent qualities of exosomes as a natural and efficient modality for gene delivery in the context of treating neurological disorders. In the following context, we will summarize the advantages and challenges of exosomes in CNS delivery.
Advantages of exosomes in CNS gene delivery: innate carrier properties: exosomes manifest as naturally occurring extracellular vesicles, providing an inherently biocompatible and biodegradable platform for gene delivery. Stability in biological fluids: exosomes demonstrate robust stability in bodily fluids, ensuring the preservation of encapsulated genetic material during transit. Targeted delivery mechanisms: engineered exosomes can be meticulously designed to target specific cell types within the CNS,75,76 thereby facilitating precise and efficient gene delivery. BBB penetration: exosomes hold promise in surmounting the blood–brain barrier, presenting an avenue for direct therapeutic gene delivery to the CNS. Attenuated immunogenic response: the utilization of exosomes derived from the patient's own cells mitigates the risk of immune reactions, thereby enhancing the safety profile of gene delivery. Facilitation of intercellular communication: exosomes naturally engage in intercellular communication, potentially enhancing the integration of therapeutic genes into target cells.
Challenges in exosomes for CNS gene delivery: inherent cargo limitations: exosomes are constrained by limited cargo capacity, imposing restrictions on the amount of genetic material deliverable in a single vesicle. Complexities in large-scale production: the scalability of exosome production for therapeutic purposes poses substantial challenges, thereby restricting their widespread clinical applicability.77 Variable loading efficiency: the efficiency of loading therapeutic genes into exosomes exhibits variability, exerting an influence on the overall efficacy of gene delivery. Technical hurdles in genetic engineering: the genetic modification of exosomes for specific gene delivery applications presents technical challenges that can impact the reliability and consistency of the engineered vesicles. Inter-donor variability: exosomes derived from distinct donors may exhibit compositional variability,77 potentially influencing their performance in the context of gene delivery.
Engineered exosomes can be loaded with gene-editing tools and directed to specific cells,76 offering a promising avenue for precise and targeted gene editing. However, further improvements are needed to optimize gene editing efficiency and cell specificity.78 At present, two primary methodologies are employed for loading EVs: direct loading of purified EVs and cell-based loading. In direct loading, EVs are loaded after purification, while in cell-based loading, loading occurs either passively via CRISPR/Cas9 overexpression or actively through interactions involving proteins or RNA. Challenges are encountered with both approaches, including the need for large-scale purification, efficient cargo loading, optimal gene editing efficiency, precise cell targeting, and comprehensive assessment of collateral effects. Direct loading methods may result in cargo aggregation, whereas cell-based approaches face hurdles such as low EV yield and limited endosomal escape. To address these challenges, solutions such as the utilization of viral proteins and the development of EV hybrids are being explored. These innovations aim to enhance loading efficiency, improve targeting specificity, and mitigate adverse effects associated with EV-based gene editing approaches.
Overall, while exosomes hold considerable promise for gene delivery in the CNS, addressing challenges related to cargo capacity, production scalability, and the precision of engineering is imperative for their successful translation into clinical applications. Current scientific endeavors are directed toward optimizing exosome-based gene delivery systems and surmounting these limitations.
2.2.5 Future directions of non-viral vector development.
Non-viral gene delivery technologies for the CNS exhibit promising advancements, including LNPs, PNPs, exosomes, and VLPs. LNPs show potential for targeted delivery to the CNS, especially in gene addition and gene editing. PNPs offer tunability, sustained release, and enhanced penetration of the BBB. VLPs, engineered without infectious genetic cargo, hold promise for targeted drug delivery in the CNS. Exosomes, natural carriers, play a crucial role in gene delivery, with potential benefits such as biocompatibility and stability. Other inorganize nanoparticle based delivery systems enabling control of editing events with physical approaches are also of high interest.79
Challenges include cargo limitations and production complexities, but ongoing research aims to optimize these non-viral approaches for clinical use in treating CNS disorders.80 The initial investigations into non-viral vectors revealed limited efficacy in the CNS tissue. However, subsequent research endeavors have focused on refining these vectors by exploring diverse vector materials and employing various functionalization methods. The primary objective is to optimize specific attributes, including augmented transcytosis across the BBB, enhanced perfusion within the brain region, heightened cellular uptake, proficient endosomal escape in neural cells, and successful nuclear transport of genetic material subsequent to intracellular delivery.
A combination of strategies is being implemented to enhance the performance of non-viral vectors, culminating in the development of multi-functional vectors. These vectors have exhibited success in numerous pre-clinical applications for the treatment of conditions such as Parkinson's disease, brain cancers, and cellular reprogramming for neuron replacement. Although further refinement in the design of these multi-functional non-viral vectors for neural applications is imperative, substantial groundwork has been laid. This underscores a promising trajectory in the advancement of non-viral vectors within the realm of neural therapeutics, presenting potential solutions for an array of neurological disorders.
When considering whether to use a viral or non-viral vector for gene delivery, it's important to recognize that there is no universal solution. The selection of an appropriate vector requires careful consideration and a balance of multiple factors (see Table 1 for the basic features of different vectors). These factors include weighing the risks against the benefits, the specific therapeutic approach, the size and type of genetic cargo being delivered, the targeted tissue and cell type, the need for durability and persistence of gene expression, and the characteristics of the patient population. Viral vectors, such as adenoviruses or lentiviruses, offer high transduction efficiency and long-term gene expression but may raise safety concerns such as immunogenicity or potential insertional mutagenesis. On the other hand, non-viral vectors, like nanoparticles, are often safer and more versatile but may have lower transduction efficiency and a shorter duration of gene expression. The choice between viral and non-viral vectors should be guided by a comprehensive assessment of these factors, considering the specific requirements of the gene therapy application and the patient population being targeted. It's essential to weigh the benefits of efficient gene delivery against the potential risks and limitations associated with each vector system, ensuring the optimal balance for achieving therapeutic goals while minimizing adverse effects.
3. Overcoming biological barriers
3.1 Crossing or bypassing the BBB and BSCB
3.1.1 The challenge of the BBB/BSCB.
The BBB serves as a selective and formidable impediment that demarcates the circulatory system from the neural tissues of the brain and spinal cord. It assumes a pivotal role in upholding CNS homeostasis and shielding it from putatively deleterious agents. The BBB is constituted by specialized endothelial cells ensconcing cerebral and spinal vasculature, featuring an exceptionally compact arrangement devoid of intercellular fenestrations. Notably, these endothelial cells are interconnected by intricate tight junctions, specialized protein complexes that confer a robust physical barrier. These junctions act to restrict molecular transit between adjacent cells, thereby circumscribing the transfer of substances from the bloodstream into the cerebral milieu. Enveloping the vasculature, astrocytes, a subtype of glial cells, extend foot processes that confer supplementary structural support to the BBB. These processes intricately modulate molecular interchange between the blood and the brain. Exteriorly situated pericytes further contribute to BBB integrity by participating in the regulation of vascular perfusion, thereby influencing barrier permeability.
The BBB functions as a guardian, precluding ingress of diverse entities, including toxins, pathogens, and large molecular species, into the cerebral compartment. This custodial function is integral to the preservation of the requisite microenvironment conducive to optimal neuronal functionality. Essential nutrients, ions, and water ingress are meticulously orchestrated, while the egress of potentially injurious entities is obstructed, thereby preserving the chemical constancy of the CNS. The BBB additionally confers immune privilege upon the CNS by restricting the admittance of immune effectors and antibodies, mitigating the risk of inflammatory responses. This immune-protection, while beneficial, poses therapeutic challenges in the context of neuroinflammatory pathologies.
While selectively permeable to imperative molecules such as oxygen, glucose, and amino acids, the BBB rigorously regulates the ingress of other substances. This selectivity, though pivotal for neurophysiological homeostasis, complicates the delivery of therapeutic agents to the CNS. Numerous pharmacotherapeutic agents, particularly those designated for neurological disorders, encounter impediments in traversing the BBB. A nuanced comprehension of the BBB is hence imperative for researchers and clinicians engaged in drug delivery, neuropharmacology, and neurological disorder interventions. Strategies aimed at circumventing or selectively permeating the BBB are actively under investigation to optimize treatments for CNS afflictions.
The BSCB, analogous in principle to the BBB, assumes a crucial role in maintaining spinal cord homeostasis and shielding it from potentially detrimental substances.81 Comprising endothelial cell lining spinal cord vasculature, the BSCB similarly features tight junctions, acting as a physical impediment to the majority of molecular species and cellular elements within the bloodstream. Pericytes and astrocytes contribute to BSCB regulation, akin to their roles in the BBB.
The BSCB orchestrates the selective permeability of substances, safeguarding the spinal cord's microenvironment. Analogous challenges to therapeutic agent delivery exist due to the BSCB's selective permeability, necessitating innovative strategies for overcoming these hindrances for treating the diseases and disorders affecting the spinal cord. A comprehensive grasp of the concept and significance of the BSCB is imperative for scientific and clinical endeavors directed towards spinal cord-related conditions and interventions. Efforts are ongoing to devise targeted drug delivery strategies to the spinal cord while upholding the integrity of the BSCB.82
Overcoming the BSCB has been a significant challenge in developing therapies for spinal cord disorders.82 However, researchers have made notable progress in strategies to enhance drug and gene delivery to the spinal cord. They explore the use of nanoparticles like liposomes and polymeric nanoparticles to carry drugs or genetic materials.83 These nanoparticles can be designed and engineered to improve drug delivery by exploiting transport mechanisms or bypassing the BSCB. Strategies involving receptor-mediated transcytosis (RMT) use natural transport mechanisms to move drugs or genes across the BSCB, employing specific ligands targeting endothelial cell receptors.
3.1.2 Molecular trafficking and transport across the BBB/BSCB.
We highlight the primary pathways for molecular trafficking across the BBB/BSCB and the endothelial cell interactions with the perivascular end-feet of astrocytes (Fig. 4A–G).84 Unlike peripheral endothelial cells, BBB endothelial cells feature tight junctions comprising occludin and claudins, tethered by intracellular scaffolding proteins (Zonula occludens), exhibit low pinocytotic activity, and lack fenestrations. This unique architecture rigorously regulates nutrient delivery to the brain via luminal receptors and transporters. Small molecules (molecular weight < 500 Da), facilitated by solute carrier (SLC) family transporters like LAT1 and MCT1, occurs for hexoses, monocarboxylic acids, fatty acids, and amino acids diffuse along concentration gradients. Larger molecules, including proteins (e.g., transferrin and insulin) and low-density lipoproteins (LDL), are transported through receptor-mediated pathways (RMTs) or nonspecific adsorptive-mediated transcytosis (AMT). This involves endocytic vesicle formation initiated by receptor or membrane interactions, with subsequent trafficking pathways ranging from lysosomal degradation to basolateral release. Lipophilic molecule diffusion is impeded by ATP-binding cassette transporters such as P-gp, BCRP, or MRPs. Additionally, BBB endothelial cells express enzymes for degrading harmful xenobiotics or waste products. Regulation of the receptor, transporter, enzyme, and tight junction expression is dynamic, responding to brain demands and mediated through interactions among BBB endothelial cells and neural and mural cell types like brain pericytes, astrocytes, neurons, oligodendrocytes, and microglial cells, collectively constituting the neurovascular unit (NVU).
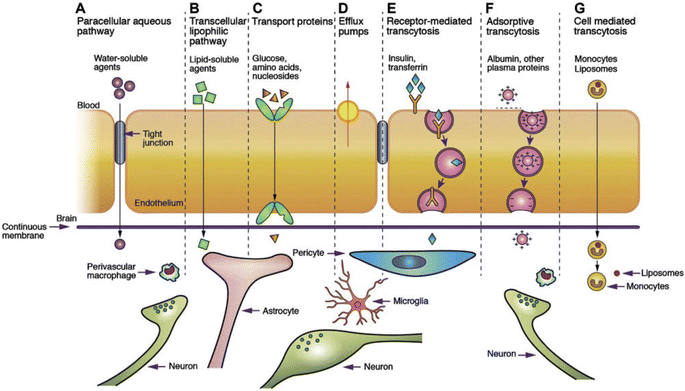 |
| Fig. 4 Transport mechanism through the BBB/BSCB.85. (A) Under normal conditions, tight junctions between endothelial cells greatly restrict the passage of water-soluble compounds, including polar drugs. (B) However, the extensive surface area of endothelial lipid membranes provides an efficient diffusive pathway for lipid-soluble agents. (C) Endothelial cells host transport proteins (carriers) facilitating the transport of glucose, amino acids, purine bases, nucleosides, choline, and other substances. Some transporters, like P-glycoprotein, rely on energy and function as efflux transporters. Notably, azidothymidine (AZT) is depicted as an example. (D) BBB/BSCB active drug efflux transporters of the ATP-binding cassette gene family are increasingly recognized as important determinants of drug distribution to, and elimination from, the CNS. (E) Specific receptor-mediated endocytosis and transcytosis mechanisms facilitate the uptake of certain proteins such as insulin and transferrin. (F) While native plasma proteins like albumin have poor transport across the BBB, cationization can enhance their uptake through adsorptive-mediated endocytosis and transcytosis. Overall, drug delivery across the brain endothelium predominantly relies on pathways B to F, with most CNS drugs entering via route B. (G) The route involves monocytes, macrophages and other immune cells and can be used for any drugs or drug incorporated liposomes or nanoparticles.86 Reproduced from ref. 86 with permission from Korean Society of Biomaterials, copyright 2019. | |
Intrathecal administration (IT) delivers therapeutic agents directly into the cerebrospinal fluid around the spinal cord and has been widely used in the both preclinic and clinical circumstance, partially bypassing the BSCB for more direct access of the spinal cord.87 Focused ultrasound (FUS), combined with sound wave responsive microbubbles, shows promise in temporarily disrupting the BSCB in preclinical animal models,88,89 and validation and optimization on human patients are ongoing. This technique creates localized transient openings, can be performed with transvertebral ultrasound,90 enhancing the delivery of drugs or genes to focal lesion the spinal cord.91 Osmotic agents, similar to those used for the BBB, are being explored to induce temporary disruptions in the BSCB, improving drug penetration.92
Various biochemical modulators, including vasoactive substances, are investigated for their potential to temporarily alter tight junctions within the BSCB, enhancing permeability for therapeutic agents.93 Advances in gene therapy, using viral vectors or non-viral methods, aim to introduce therapeutic genes directly into the spinal cord, improving the efficiency and precision of gene delivery.
In many neuroinflammatory and neurodegenerative conditions where the BSCB may be compromised, researchers explore leveraging this altered integrity to enhance drug delivery.94 Ongoing research aims to optimize and combine these strategies, providing new possibilities for treating spinal cord disorders by effectively overcoming the challenges posed by the BSCB.
The route of administration (ROA, Fig. 5A–E), including IT, convection-enhanced delivery (CED), RMT, and remote-controlled modulation of the BBB/BSCB using ultrasound, light, and a magnetic field, has a significant impact on the drug penetration and distribution in the CNS, is discussed herein.
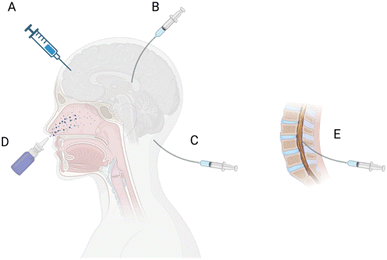 |
| Fig. 5 Route of administration (ROA) to the CNS. (A) Direct injection to the brain tissue, (B) intraventricular, (C) intracisternal magna, (D) intranasal, and (E) intrathecal injection. | |
3.1.3 IT.
IT involves the direct administration of substances into the cerebrospinal fluid (CSF) within the spinal canal. This method circumvents the BBB, particularly suitable for delivering to the spinal cord. The IT method allows for higher drug concentrations at the target spinal tissue area compared to systemic administration. By minimizing systemic circulation, IT administration may reduce the risk of systemic side effects associated with certain drugs. IT often results in a quicker onset of action, providing rapid and effective delivery. Commonly employed in pain management, these injections offer localized relief for conditions like chronic pain, cancer-related pain, or neuropathic pain.95 However, the nature of the procedure carries inherent risks, including the potential for infections and neurological damage.96 Precision and expertise are crucial to minimize these risks. ITs are primarily indicated for conditions necessitating direct access to the spinal canal, limiting their application to diseases dominantly affecting the spinal cord, with much less drug distribution in the brain tissue. Patient suitability, comfort with invasive treatments, and the availability of skilled healthcare providers may influence the accessibility of this treatment option. Additionally, as with any drug administration, there is a risk of allergic reactions, requiring vigilant monitoring for adverse events.97 Despite these considerations, ITs remain a valuable intervention for targeted treatment in certain neurological and pain management scenarios.
In the realm of gene delivery, IT has established itself as a targeted procedure wherein vectors carrying therapeutic genes are introduced directly into the spinal canal. The cerebrospinal fluid acts as a conduit, facilitating the distribution of genetic material throughout the brain and spinal cord. This approach holds promise for addressing conditions characterized by deficient or aberrant gene expression, offering potential treatment options for disorders like spinal muscular atrophy and ALS.98 Ongoing research endeavors aim to enhance the safety and efficacy of intrathecal gene delivery, paving the way for innovative treatments in various neurological contexts.99
3.1.4 CED.
CED represents a method of delivering therapeutic agents to the CNS by directly infusing them into the brain or spinal cord under positive pressure. Advances in CED have significantly improved precision and efficacy in delivering drugs to specific areas within the CNS.100 Utilizing advanced imaging techniques like magnetic resonance imaging (MRI) and real-time stereotactic navigation systems enables precise targeting of specific brain regions or tumors, minimizing damage to surrounding tissues.101 Progress in catheter design and infusion technologies has resulted in enhanced catheter systems, improving the distribution and dispersion of therapeutic agents within the target area.102 Real-time monitoring during CED procedures allows continuous assessment of drug distribution within the CNS, employing techniques such as microdialysis and imaging modalities to monitor drug concentration and spread in real-time. Researchers are developing specialized drug formulations optimized for CED, including drugs with enhanced diffusion characteristics and stability. CED offers a direct route for delivering therapeutic agents to the CNS, circumventing the challenges posed by the BBB. This is particularly advantageous for drugs facing hurdles in crossing the BBB when administered systemically. CED facilitates the delivery of combination therapies, including multiple drugs or a combination of drugs and other treatment modalities, enhancing the potential for synergistic effects and improved therapeutic outcomes.
Advances in gene therapy have been integrated into CED approaches, involving the delivery of therapeutic genes directly to target tissues within the CNS. CED has transitioned from preclinical studies to clinical trials for various neurological disorders, such as brain tumors and neurodegenerative diseases.101 CED has been applied and tested in several clinical trials, for delivering AAVs carrying the Brain-Derived Neurotrophic Factor (BDNF) gene into the entorhinal cortex of patients with Alzheimer's disease (AD) and Mild Cognitive Impairment (MCI), examining whether the BDNF prevents neuronal loss and facilitates building new synapses, thereby potentially improving memory in these patients. Recent studies have investigated the safety and efficacy of delivery of a viral vector expressing AADC (AAV2-hAADC) to the midbrain in children with aromatic L-amino acid decarboxylase (AADC) deficiency.103 Direct bilateral infusion of AAV2-hAADC has been proven to be safe and well-tolerated and achieved a target coverage of 98% and 70% of the bilateral substantia nigra (SN) and ventral tegmental area (VTA), respectively (total infusion volume: 80 μL per hemisphere) in 2 dose cohorts: 1.3 × 1011 vg (n = 3) and 4.2 × 1011 vg (n = 4). Dopamine metabolism increasing was observed in all patients and FDOPA uptake enhancement was indicated within both the midbrain and the striatum. Oculogyric crisis (OGC) was resolved completely in 6 of 7 patients by Month 3 post-surgery. Twelve (12) months post-surgery, 6/7 subjects gained normal head control and 4/7 could sit without assistance. At 18 months, 2 subjects could walk with 2-hand support. These results suggested that midbrain gene delivery in children with AADC deficiency via CED is feasible and safe and leads to clinical improvements in symptoms and motor function. Clinical trials of CED of the AAV Encoding Glial Cell Line-Derived Neurotrophic Factor (AAV2-GDNF) in patients with advanced Parkinson's Disease (NCT01621581) and Multiple System Atrophy-Parkinsonian Type (MSA-P, NCT04680065) have been initialized. These trials assess the safety and efficacy of CED in human subjects, opening avenues for treating many diseases in the CNS. Ongoing research focuses on optimizing infusion parameters for maximizing drug distribution while minimizing adverse effects, exploring controlled infusion systems, and developing adaptive algorithms for personalized treatment. These advancements collectively contribute to the refinement and broader application of CED for drug delivery in the CNS, offering promising avenues for the treatment of neurological conditions.
Enhancing the permeability of the BBB is a critical focus of research aimed at facilitating drug and gene delivery to the CNS. Technological advancements in this field seek to overcome the natural barrier restricting the passage of substances from the bloodstream to the brain. Various methodologies have been explored to enhance BBB permeability. Focused ultrasound involves the targeted application of ultrasound waves to specific brain regions, inducing transient disruptions in the BBB when combined with microbubbles. Controlled microwave-induced hyperthermia can induce temporary changes in BBB integrity.104 Osmotic agents, like mannitol, can induce hyperosmolarity, leading to transient BBB disruption.105 Chemical agents, including vasoactive substances and certain peptides, can modulate tight junctions between endothelial cells of the BBB.
3.1.5 RMT.
Nanoparticles, such as liposomes or polymeric nanoparticles, can be engineered to encapsulate drugs or genetic material and pass through the BBB via the RMT transport pathway.106 For instance, a new BBB transport vehicle targeting the CD98 heavy chain (CD98hc or SLC3A2) of heterodimeric amino acid transporters (TVCD98hc) has been reported recently.107 The pharmacokinetic and biodistribution properties of a CD98hc antibody transport vehicle (ATVCD98hc) have been evaluated in humanized CD98hc knock-in mice and cynomolgus monkeys. Compared to the transferrin receptor targeted BBB crossing platform, peripherally administered ATVCD98hc has shown much slower and more prolonged kinetic properties. A similar platform has been proven to be valid for targeted crossing of bispecific antibodies to the brain in preclinical animal models.108,109 Bispecific antibody shuttles that engage CD98hc efficiently transport protein cargoes into the brain and lead to much longer-lived brain retention of proteins than TfR-1 shuttles. Functionalization of the gene delivery vectors with engineered targeting elements to increase the engagement with receptors and transcytosis will be highly valuable taking advantage of RMT. For example, exosomes, natural extracellular vesicles, have been explored for carrying therapeutic cargo across the BBB via RMT. RMT can be adapted for enhancing the CNS access via the intranasal route.110 RMT is a widely tested endogenous transport system, offering a targeted approach for delivering drugs or genes across the BBB. This approach can be limited by the expression of receptors in non-CNS tissue. Continued research aims to optimize techniques for safely and effectively enhancing BBB permeability, enabling the development of more specifically targeted and efficient treatments for CNS disorders.
3.1.6 Ultrasound-mediated delivery.
A remote-controlled technique has been reported for successful opening of the BBB and targeted delivery of adeno-associated virus serotype 9 vectors to specific brain regions implicated in Parkinson's disease.111 This was achieved using low-intensity FUS in adult macaque monkeys. The barrier openings were well-tolerated, showing no abnormal signals in magnetic resonance imaging. Neuronal green fluorescent protein expression occurred specifically in regions where the BBB was opened. Similar safe BBB openings were demonstrated in three patients with Parkinson's disease. In both patients and one monkey, the openings were followed by 18F-Choline uptake in the putamen and midbrain regions based on positron emission tomography. This indicates focal and cellular binding of molecules that typically cannot enter the brain parenchyma. Recent advancements in FUD mediated delivery of antiamyloid antibodies (aducanumab) in patients with Alzheimer's disease reported reduced cerebral amyloid-beta (Aβ) load.112 The reduction in the level of Aβ was significantly greater in regions treated with FUS than in the homologous regions in the contralateral hemisphere without FUD. These results encourage further improvement and validation of the FUS for delivering various therapeutics, including AAVs and LNPs carrying nucleic acid cargoes in human. The less-invasive nature of this method could facilitate targeted viral or non-viral vector delivery for gene therapy and enable early and repeated interventions for neurodegenerative disorders. FUS has also been applied as a safe and effective gene editing technique used to transiently open the BBB, allowing intravenously delivered CRISPR/Cas9 machinery to reach the brain.113 Gene editing in the brain faces challenges due to the restricted transport imposed by the BBB. Existing approaches often involve local injection to bypass the BBB, but this method is invasive and not suitable for treating certain delicate brain regions.
3.1.7 Light-mediated delivery.
Optical-controlled technology for delivering genes to the CNS has been an area of active research. The concept involves using light-responsive materials to control the release of genetic material, providing spatiotemporal precision in gene therapy. Researchers have developed nanoparticles that respond to specific wavelengths of light. These nanoparticles can encapsulate genetic material and release it upon exposure to light. This approach allows for precise control over the timing and location of gene delivery within the CNS. Optogenetics is a technique that uses light to control cells that have been genetically modified to express light-sensitive proteins. In the context of gene delivery, optogenetic approaches can be employed to control the expression of therapeutic genes in specific neurons or cell types within the CNS. Hydrogels are three-dimensional networks that can hold and release genetic material. Light-responsive hydrogels have been designed to release genes in response to specific light cues. These hydrogels can be injected into the CNS to provide controlled and localized gene delivery. Viral vectors, such as AAVs, can be engineered to respond to light. This engineering allows for the activation or deactivation of the viral vector's ability to deliver genes using precise light stimulation. For example, researchers have developed an innovative solution by engineering an AAV vector system, termed OptoAAV, capable of transferring genetic information into native target cells upon exposure to cell-compatible red light.114 The OptoAAV system allows for adjustable and spatially resolved gene transfer, reaching single-cell resolution, and is compatible with various cell lines and primary cells. Furthermore, the sequential application of multiple OptoAAVs enables spatially resolved transduction with different transgenes. The versatility of this approach extends its applicability to other viral vector classes, holding promise for advancements in both basic and applied genetic research. Nanomaterials, like gold nanoparticles or carbon nanotubes, with photothermal properties can absorb light and convert it into heat. This localized heat generation can trigger the release of genes from carriers, providing a light-controlled release mechanism. Near-infrared light has better tissue penetration capabilities. Utilizing near-infrared light-responsive materials can enhance the feasibility of optical-controlled gene delivery to deeper structures within the CNS. Optical-controlled gene delivery systems often incorporate in vivo imaging techniques, allowing researchers to monitor and control gene expression in real-time within the living organism. These optically controlled technologies offer the advantage of non-invasiveness, precise control, and the ability to target specific regions within the CNS.
A latest study introduced an optical technology to address the challenge of overcoming the BSCB for targeted molecular delivery to the spinal cord.115 A novel approach involves the transient modulation of BSCB permeability for localized molecular (peptide and gene vectors) delivery with exceptional spatial precision (Fig. 6A–C). This method employs optical stimulation of vasculature-targeted nanoparticles, enabling the delivery of molecules impermeable to the BSCB without inducing significant glial activation or disrupting animal locomotor behavior. The study demonstrates the efficacy by minimally invasive light delivery into the spinal cord using an optical fiber, achieving successful BSCB permeability modulation in the lumbar region. This technique allows the delivery of bombesin, a centrally acting itch-inducing peptide, into the spinal cord, resulting in a rapid and transient increase in itching behaviors in mice. The minimally invasive optical approach, devoid of genetic modifications, holds promise for delivering various biologics into the spinal cord for behavior modulation and potential therapeutic applications. The study addresses the formidable challenge of bypassing the BSCB for targeted therapeutic delivery. The introduced optical approach, characterized by its minimally invasive nature and precise spatial resolution, overcomes limitations associated with prior technologies. The method induces behavior modulation, such as itch, without necessitating genetic modifications, showcasing its potential for delivering biologics like peptides into the spinal cord. Notably, a similar technique has been used for delivering AAVs and LNPs to the brain in mice with high spatial precision,116 highlighting the potential for delivery cargoes of gene therapy and gene editing.
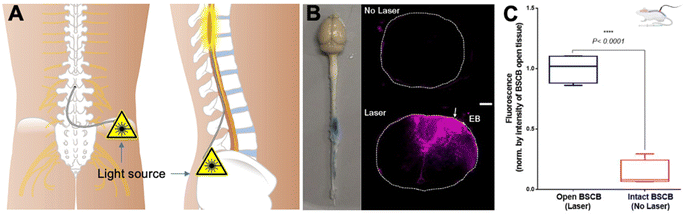 |
| Fig. 6 Optical modulation of the BSCB for local delivery of the therapeutics to the spinal cord. (A) Scheme of the IT access to the spinal cord with an optical fiber, (B) images of the mouse CNS tissue, and fluorescence – Evans blue in the spinal cord tissues with and without laser modulation, and (C) quantitative fluorescence indicating the enhanced Evans blue penetration and distribution in the lesion with laser modulation. Reproduced from ref. 115 with permission from author, copyright 2022. | |
3.1.8 Magnet-mediated delivery.
In light of the BBB's inherent limitations in facilitating the penetration of conventional drugs, a recent study explored the viability of utilizing magnetic nanoparticles (MNPs) as an enhancer and potential drug carriers for enhanced BBB permeation in the presence of an external static magnetic field (SMF).117 The focus is on the development and validation of a physiologically based pharmacokinetic (PBPK) model for intraperitoneal (IP) administered superparamagnetic iron oxide nanoparticles coated with gold and conjugated with poly(ethylene glycol) (SPIO-Au-PEG NPs) in murine subjects. The primary objective of the investigation is to quantitatively assess the influence of the SMF on the in vivo dynamics of SPIO-Au-PEG NPs concerning their traversal across the BBB, administration of SPIO-Au-PEG NPs via IP in murine models, formulation and validation of a PBPK model to comprehensively capture the pharmacokinetic behavior, determination of BBB permeabilities under differing conditions (with and without SMF) and computational simulation of NP concentration in cerebral blood and brain tissue utilizing advection-diffusion equations, numerically solved via COMSOL Multiphysics. The integration of brain permeability parameters into the PBPK model substantially improved its predictive accuracy, exhibiting a commendable correlation with in vivo experimental outcomes. The distribution coefficient from blood to brain demonstrated a modest enhancement under SMF exposure. The incorporation of insulin into SPIO-Au-PEG NPs (SPIO-Au-PEG-insulin) manifested an augmentation in brain bioavailability, with a further incremental improvement under SMF stimulation. This investigation establishes the robustness of the PBPK model, validated through in vivo experiments, elucidating the promise of SPIO-Au-PEG NPs, particularly when augmented with insulin, for non-invasive targeted drug delivery to the brain. The findings set a firm groundwork for prospective research endeavors in the realm of enhanced therapeutic strategies for cerebral applications through the adept utilization of magnetic nanoparticles and external magnetic fields.
The study seeks to enhance the efficacy of magnetic stimulation in facilitating drug permeation through the BBB. The existing impediment involves the limited dimensions of the magnetic core, adversely affecting magnetic targeting precision. In response, the research proposes the development of a biodegradable hydrogel micro-swimmer incorporating superparamagnetic iron oxide-gold nanoparticles (SPIO-AuNPs). This micro-swimmer is anticipated to exhibit heightened magnetic responsiveness, mitigating size-related constraints and optimizing targeting efficiency for enhanced BBB penetration. Subsequent investigations will systematically design the micro-swimmer to address size-related limitations and refine an advanced physiologically based pharmacokinetic (PBPK) model to assess its efficiency. The overarching objective is to deepen our comprehension of the intricate interplay between magnetic responsiveness, particle size, and BBB permeation, thereby paving the way for refined therapeutic strategies in neuropharmacology (Table 2).
Table 2 Summary of the modalities for enhancing gene delivery to the CNS
|
Optical |
Acoustic |
Magnetic |
Chemical |
Biological |
BBB opening |
✓ |
✓ |
Possible |
✓ |
Pathway/mechanism dependent |
ECS modulation |
Possible |
✓ |
Possible |
Possible |
Possible |
Depth |
mm |
cm |
10 cm |
ROA dependent |
ROA dependent |
Resolution |
μm–mm |
mm–cm |
Focus technology to be developed |
ROA dependent |
ROA dependent |
Volume |
Scanning method dependent |
Scanning method dependent |
To be demonstrated |
ROA dependent |
ROA dependent |
Shape control |
✓ |
✓ |
Possible |
NA |
NA |
Enhancer |
Light-absorbing nanoparticle |
Gap-filled microbubble |
Large magnetic nanoparticle |
Possible |
Possible |
Invasiveness |
Tissue region dependent |
Low |
Non |
To be determined |
To be determined |
Remote control |
✓ |
✓ |
✓ |
NA |
NA |
Clinical relevance |
✓ |
✓ |
✓ |
Low |
✓ |
3.2 Enhancing vector diffusion in the extracellular environment
3.2.1 ECS as the diffusion barriers in the CNS.
Effective gene delivery to the CNS relies on the diffusion of vectors through the extracellular space (ECS).118 This holds true for drugs administered directly into the CNS tissue and those crossing the BBB/BSCB after systemic administration. The diffusion of substances within the CNS is dynamically influenced by various properties of the brain microenvironment (Fig. 2), including ECS volume fraction, geometry, width, tortuosity, local viscosity, and interactions with cell surfaces, extracellular matrix, and interstitial fluid components.119 The ECS properties play a crucial role in governing the distribution of nanoparticles, and viral vectors within the CNS.
The ECS within the CNS constitutes the fluid-filled intercellular regions among neurons and glial cells, predominantly occupied by cerebrospinal fluid (CSF) and various substances.120 This microenvironment is pivotal for sustaining an equilibrium conducive to optimal neural functionality. Facilitating the transport of neurotransmitters, hormones, and nutrients between blood vessels and cells, the ECS regulates ion and nutrient concentrations, concurrently participating in waste removal through CSF circulation for the preservation of a conducive cellular milieu. A nuanced comprehension of ECS dynamics is imperative for deciphering CNS information processing intricacies, as alterations may potentially impact neuronal function and contribute to neurological disorders.
In the realm of gene delivery and CNS-targeted medication efficacy, the ECS operates as a discernible diffusion barrier impeding vectors' access to target cells.121 Complex interactions within the ECS involving cellular constituents, notably glial cells and neurons, exert influence over vector uptake, metabolism, and response dynamics.122 Glial cells, in particular, demonstrate a modulatory role in drug effects and distribution.123 Furthermore, the ECS contributes significantly to the clearance of vectors from the brain, implicating enzymes and transporters both within the ECS and intracellularly.124 It is noteworthy that ECS characteristics exhibit spatial and temporal variability across different brain regions, exerting consequential effects on the treatment distribution and duration.118 This intricate landscape underscores the imperative for an exhaustive understanding of ECS intricacies in the development of effective gene delivery strategies tailored for neurological conditions.125
3.2.2 Unveiling structure and function of ECS.
Recent advancements in unraveling the intricacies of the ECS within the CNS have significantly enriched our comprehension of the intricate microenvironment enwrapping neurons and glial cells. Noteworthy progress encompasses the utilization of molecular imaging probes, facilitating the precise tracking of distinct molecules within the ECS, including nanoparticles, neurotransmitters and ions.121 Cutting-edge imaging methodologies such super-resolution microscopy, single particle tracking, two-photon microscopy and magnetic resonance imaging have empowered real-time, high-resolution visualization of dynamic ECS processes.126 Single-particle tracking of individual fluorescent single-wall carbon nanotubes (SWCNTs) in living samples elucidates that the ECS nanoscale organization127 and surrounding synapses exhibit distinctive nanoscale morphology and internal diffusivity properties.128 The ECS in the juxta-synaptic region undergoes alterations in diffusion parameters in response to neuronal activity. This observation sheds light on the spatial characteristics of the ECS around synapses that impact the local diffusion and uptake of substances including therapeutics by active neurons.129 Super-resolution microscopy (SUSHI) allows for viewing the local ECS dynamic changes during activity and function-dependent cell migration.130
Electrophysiological approaches employing ion-selective microelectrodes have unveiled nuanced changes in ion concentrations, providing valuable insights into neurotransmission dynamics.131 Cerebral microdialysis offers a continuous surveillance mechanism for ECS substances, shedding light on neurotransmitter release and metabolism.132 Mathematical modeling, incorporating parameters like diffusion, convection, and cellular uptake, has offered a theoretical framework for understanding molecule movement within the ECS.133 The recent revelation of the glymphatic system has notably enhanced our comprehension of fluid dynamics in the CNS.134 Deeper insights into neurovascular coupling mechanisms135 and the pivotal role of astrocytes in ECS homeostasis136 contribute to a holistic understanding. The implications extend to neurological disorders, with ECS dysregulation implicated in conditions such as epilepsy and neurodegenerative diseases. These scientific strides unveil potential therapeutic targets for interventions in neurological pathologies.
A profound comprehension of ECS properties and precise measurement techniques is imperative for advancing gene delivery strategies within the challenging CNS environment. Ongoing research is continually refining our understanding of the dynamic and intricate ECS microenvironment, with a particular focus on emerging technologies.137 Mathematical models have been crafted to simulate and predict drug diffusion within the intricate structures of the brain and spinal cord, incorporating factors such as tissue composition, flow dynamics, and molecular properties.138 These models enhance our insights into the spatiotemporal aspects of drug distribution. Research on cerebral and spinal cord microcirculation has advanced our understanding of blood flow patterns and their impact on drug transport, considering factors like vessel density and architecture influencing drug diffusion.135 In parallel, new methods for quantitatively measuring drug diffusion in the ECS are under development,125 encompassing modeling delivery across species, drug screening, assessing methods to alter drug distribution, and recognizing changes in drug distribution in CNS diseases. Collectively, these advancements contribute to a more holistic understanding of drug diffusion in the brain and spinal cord, fostering the development of targeted and effective treatments for neurological disorders.
3.2.3 Enhancing diffusion by modulating the ECS.
Ultrasound has emerged as a promising technique for enhancing gene diffusion within the CNS by expanding the interstitial space and enhancing the flow transport.139 This non-invasive method involves the application of FUS waves to targeted brain regions, inducing mechanical vibrations and temporarily widening the ECS (Table 2). The expansion of the interstitial space facilitates improved penetration and distribution of therapeutic vectors, overcoming diffusion barriers.140
A study employed electron microscopy-based ultrastructural analysis and high-resolution tracking of non-adhesive nanoparticles to investigate changes in the extracellular and perivascular spaces of the brain following a non-destructive pulsed ultrasound regimen known to alter diffusivity in other tissues.141 Rat brain neocortical slices underwent sham treatment or pulsed low-intensity ultrasound treatment for 5 minutes at 1 MHz. Transmission electron microscopy revealed intact cells and blood vessels, along with evidence of enlarged spaces, particularly adjacent to blood vessels, in ultrasound-treated brain slices. Additionally, ultrasound significantly increased the diffusion rate of 100 nm, 200 nm, and 500 nm nanoparticles injected into the brain slices, while 2000 nm particles were unaffected. In ultrasound-treated slices, 91.6% of the 100 nm particles, 20.7% of the 200 nm particles, 13.8% of the 500 nm particles, and 0% of the 2000 nm particles exhibited diffusive motion. These findings demonstrate that pulsed ultrasound can have meaningful structural effects on the brain extracellular and perivascular spaces without evidence of tissue disruption.
Delivering systemically administered gene therapies to brain tumors faces considerable challenges due to the presence of the BBB in the tumor along with hindrances posed by the adhesive and nanoporous tumor ECS. FUS-mediated BBB opening induces a substantial increase in interstitial tumor flow, playing a critical role in enhancing the transport of brain penetrating nanoparticles carrying therapeutics through the tumor tissue. A recent study introduced a platform approach utilizing MRI-guided FUS and MBs for transfecting brain tumors.142 This involved targeting the delivery of systemically administered nanoparticle gene vectors across the BBB. The MRI-based transport analysis revealed that FUS-mediated BBB opening doubled the mean interstitial flow velocity magnitude, with “per voxel” flow directions changing by an average of ∼70° to 80°. Moreover, FUS-mediated BBB opening significantly increased the dispersion of directly injected nanoparticles through tumor tissue by over 100%. This approach holds potential for enhancing the effectiveness of gene delivery to the CNS, offering a non-surgical and precise method for therapeutic intervention.
The glymphatic system serves as a perivascular fluid transport mechanism for waste clearance in the brain.134 It relies on the pulsation of the arterial wall, driven by the cardiac cycle, to create a perivascular pumping effect.120 A recent study explored the mechanical manipulation of glymphatic transport using FUS sonication of circulating MBs.143 The study employed intranasal administration of fluorescently labeled albumin as a fluid tracer and FUS sonication at a deep brain target (thalamus) in the presence of intravenously injected MBs. Traditional intracisternal magna injection was used for comparison. Confocal microscopy of optically cleared brain tissue demonstrated that FUS sonication enhanced the transport of the fluorescently labeled albumin tracer in the perivascular space (PVS) along microvessels, particularly arterioles. The study provided evidence of FUS-enhanced penetration of the albumin tracer from the PVS into the interstitial space, revealing that ultrasound, when combined with circulating MBs, could mechanically enhance glymphatic transport in the brain.
4. Summary and prospects
4.1 Incorporation of artificial intelligence (AI) and machine learning (ML) for vector discovery
To date, the challenge of achieving safe and effective gene delivery to the CNS still necessitates the integration of novel molecular and engineering approaches. A notable advancement in this pursuit involves the incorporation of AI and ML144 in the discovery of AAV vectors, revolutionizing gene delivery.144 AI algorithms systematically analyze extensive datasets, considering parameters such as viral tropism, transduction efficiency, and safety, to predict optimal AAV vectors tailored for specific gene delivery tasks.145 This analytical process guides the design of AAV vectors with augmented targeting capabilities, thereby enhancing precision in gene delivery. AI extends its impact to the innovation of novel AAV capsids, elevating vector stability and fortifying immune system evasion strategies. Additionally, AI optimizes therapeutic gene expression by scrutinizing genetic sequences, accelerating drug development through early success prediction and resource efficiency. The implementation of AI in personalized AAV therapies leverages patient-specific data analysis to optimize therapeutic efficacy while mitigating potential adverse effects.
Concurrently, AI's integration in the discovery of LNPs and PNPs is reshaping the landscape of RNA therapeutic drug delivery.146 AI expedites the optimization of formulations by dissecting lipid and polymer compositions, augmenting stability and efficiency.147 Predicting physicochemical properties aids in tailoring LNPs and PNPs for superior circulation and enhanced cellular uptake. AI enhances targeting precision by parsing through biological data, models drug release kinetics for nuanced control and sustained release, and predicts biological interactions for optimized safety and performance. Early success prediction through AI streamlines the drug development trajectory, and the individualized dimension of AI-driven analysis of patient-specific data tailored formulations to personalized treatment strategies. In summation, the integration of AI significantly contributes to the optimization of drug delivery systems, holding immense promise for the advancement of more effective and personalized therapeutics.
4.2 Ultrasmall, ultrasoft endovascular catheter for localized gene delivery
Recent progress in ultrasmall and flexible endovascular catheters as well as surgical robots may also be incorporated to enhance the gene delivery to the CNS.148 For example, an endovascular micro-robotic toolkit with a cross-sectional area that is orders of magnitude smaller than the smallest catheter currently available has been shown to improve state-of-the-art practices with potential for localized gene delivery in the CNS as it enhances the reachability driven by the blood fluid.149 Recently, Dreyfus et al. have reported on a dexterous helical magnetic robot and used it to improve the navigation of catheters in tortuous blood vessels.150 The robotic device enabled successful endovascular navigation from the aorta to millimeter-sized cranial arteries in vivo, demonstrating its potential for accessing blood vessels with complex architecture in the brain and potentially extended applications in drug and gene delivery. An ultrasmall endovascular endoscope can also assist neurosurgeons with therapeutic interventions by releasing drugs, including gene vectors to the local lesion affected by the disease in the CNS.151
4.2.1 Closing remarking.
To summarize, rapid advancements in AI/MI and endovascular robots have been made to access tissue in the CNS guided by big database and powerful algorithms to increase precision. Remote stimulation and control technologies have shown early success in facilitating the gene delivery by overcoming the BBB/BSCB and enhancing the vector diffusion in the extracellular space. In future, integrated tools combined with novel therapeutic modalities will be capable of treating CNS diseases at ultralow therapeutic doses through directly addressing genetic causes of the diseases under guiding or controlling by remote technologies, such as ultrasound, light and magnetic fields.
Conflicts of interest
There are no conflicts to declare.
References
- E. Nance, S. H. Pun, R. Saigal and D. L. Sellers, Nat. Rev. Mater., 2022, 7, 314–331 CrossRef CAS.
- L. Kang, S. Jin, J. Wang, Z. Lv, C. Xin, C. Tan, M. Zhao, L. Wang and J. Liu, J. Controlled Release, 2023, 355, 458–473 CrossRef CAS PubMed.
- J. R. Mendell, S. Al-Zaidy, R. Shell, W. D. Arnold, L. R. Rodino-Klapac, T. W. Prior, L. Lowes, L. Alfano, K. Berry, K. Church, J. T. Kissel, S. Nagendran, J. L'Italien, D. M. Sproule, C. Wells, J. A. Cardenas, M. D. Heitzer, A. Kaspar, S. Corcoran, L. Braun, S. Likhite, C. Miranda, K. Meyer, K. D. Foust, A. H. M. Burghes and B. K. Kaspar, N. Engl. J. Med., 2017, 377, 1713–1722 CrossRef CAS PubMed.
- A. Cota-Coronado, N. F. Díaz-Martínez, E. Padilla-Camberos and N. E. Díaz-Martínez, Front. Mol. Neurosci., 2019, 12, 110 CrossRef CAS PubMed.
- R. Daneman and A. Prat, Cold Spring Harbor Perspect. Biol., 2015, 7, a020412 CrossRef PubMed.
- W. M. Pardridge, Trends Mol. Med., 2023, 29, 343–353 CrossRef CAS PubMed.
- C. Li and R. J. Samulski, Nat. Rev. Genet., 2020, 21, 255–272 CrossRef CAS PubMed.
- G. C. Terstappen, A. H. Meyer, R. D. Bell and W. Zhang, Nat. Rev. Drug Discovery, 2021, 20, 362–383 CrossRef CAS PubMed.
- W. M. Pardridge, Drug Discovery Today, 2002, 7, 5–7 CrossRef.
- A. Schambach, C. J. Buchholz, R. Torres-Ruiz, K. Cichutek, M. Morgan, I. Trapani and H. Büning, Lancet, 2023, 403(10426), 568–582 CrossRef.
- V. Madigan, F. Zhang and J. E. Dahlman, Nat. Rev. Drug Discovery, 2023, 22, 875–894 CrossRef CAS PubMed.
- B. L. Davidson and X. O. Breakefield, Nat. Rev. Neurosci., 2003, 4, 353–364 CrossRef CAS PubMed.
- D. Wang, P. W. L. Tai and G. Gao, Nat. Rev. Drug Discovery, 2019, 18, 358–378 CrossRef CAS PubMed.
- A. Pupo, A. Fernández, S. H. Low, A. François, L. Suárez-Amarán and R. J. Samulski, Mol. Ther., 2022, 30, 3515–3541 CrossRef CAS.
- H. Kawabata, A. Konno, Y. Matsuzaki and H. Hirai, Mol. Ther.–Methods Clin. Dev., 2023, 29, 81–92 CrossRef CAS PubMed.
- T. J. Gonzalez, A. Mitchell-Dick, L. O. Blondel, M. M. Fanous, J. A. Hull, D. K. Oh, S. Moller-Tank, R. M. Castellanos Rivera, J. A. Piedrahita and A. Asokan, Nat. Protoc., 2023, 18, 3413–3459 CrossRef CAS.
- S. J. O’Carroll, W. H. Cook and D. Young, Front. Mol. Neurosci., 2021, 13, 618020 CrossRef.
- Z. Wu, A. Asokan and R. J. Samulski, Mol. Ther., 2006, 14, 316–327 CrossRef CAS PubMed.
- B. Nieuwenhuis, B. Haenzi, S. Hilton, A. Carnicer-Lombarte, B. Hobo, J. Verhaagen and J. W. Fawcett, Gene Ther., 2021, 28, 56–74 CrossRef CAS.
- Y. K. Chan, S. K. Wang, C. J. Chu, D. A. Copland, A. J. Letizia, H. Costa Verdera, J. J. Chiang, M. Sethi, M. K. Wang, W. J. Neidermyer, Y. Chan, E. T. Lim, A. R. Graveline, M. Sanchez, R. F. Boyd, T. S. Vihtelic, R. G. C. O. Inciong, J. M. Slain, P. J. Alphonse, Y. Xue, L. R. Robinson-McCarthy, J. M. Tam, M. H. Jabbar, B. Sahu, J. F. Adeniran, M. Muhuri, P. W. L. Tai, J. Xie, T. B. Krause, A. Vernet, M. Pezone, R. Xiao, T. Liu, W. Wang, H. J. Kaplan, G. Gao, A. D. Dick, F. Mingozzi, M. A. McCall, C. L. Cepko and G. M. Church, Sci. Transl. Med., 2021, 13, eabd3438 CrossRef CAS PubMed.
- M. Muhuri, Y. Maeda, H. Ma, S. Ram, K. A. Fitzgerald, P. W. L. Tai and G. Gao, J. Clin. Invest., 2021, 131(1), e143780 CrossRef CAS PubMed.
- H. C. Verdera, K. Kuranda and F. Mingozzi, Mol. Ther., 2020, 28, 723–746 CrossRef CAS.
- A. Lek, B. Wong, A. Keeler, M. Blackwood, K. Ma, S. Huang, K. Sylvia, A. R. Batista, R. Artinian, D. Kokoski, S. Parajuli, J. Putra, C. K. Carreon, H. Lidov, K. Woodman, S. Pajusalu, J. M. Spinazzola, T. Gallagher, J. LaRovere, D. Balderson, L. Black, K. Sutton, R. Horgan, M. Lek and T. Flotte, N. Engl. J. Med., 2023, 389, 1203–1210 CrossRef CAS PubMed.
- L. Marrone, P. M. Marchi and M. Azzouz, Expet Opin. Biol. Ther., 2022, 22, 1163–1176 CrossRef CAS PubMed.
- Z. Wu, H. Yang and P. Colosi, Mol. Ther., 2010, 18, 80–86 CrossRef CAS PubMed.
- T. W. Leong, A. Pal, Q. Cai, Z. Gao, X. Li, L. Bleris, H. N. Hayenga and Z. Qin, J. Controlled Release, 2023, 357, 511–530 CrossRef CAS PubMed.
- A. J. Boender, M. Boon, H. E. Albers, S. R. Eck, B. A. Fricker, A. M. Kelly, J. E. LeDoux, S. C. Motta, P. Shrestha, J. H. Taylor, B. C. Trainor, R. Triana-Del Rio and L. J. Young, Sci. Adv., 2023, 9, eadf4950 CrossRef CAS.
- D. G. Miller, L. M. Petek and D. W. Russell, Nat. Genet., 2004, 36, 767–773 CrossRef CAS.
- J. A. Greig, K. M. Martins, C. Breton, R. J. Lamontagne, Y. Zhu, Z. He, J. White, J.-X. Zhu, J. A. Chichester, Q. Zheng, Z. Zhang, P. Bell, L. Wang and J. M. Wilson, Nat. Biotechnol., 2023 DOI:10.1038/s41587-023-01974-7.
- K. S. Hanlon, B. P. Kleinstiver, S. P. Garcia, M. P. Zaborowski, A. Volak, S. E. Spirig, A. Muller, A. A. Sousa, S. Q. Tsai, N. E. Bengtsson, C. Lööv, M. Ingelsson, J. S. Chamberlain, D. P. Corey, M. J. Aryee, J. K. Joung, X. O. Breakefield, C. A. Maguire and B. György, Nat. Commun., 2019, 10, 4439 CrossRef PubMed.
- T. J. Gonzalez, K. E. Simon, L. O. Blondel, M. M. Fanous, A. L. Roger, M. S. Maysonet, G. W. Devlin, T. J. Smith, D. K. Oh, L. P. Havlik, R. M. Castellanos Rivera, J. A. Piedrahita, M. K. ElMallah, C. A. Gersbach and A. Asokan, Nat. Commun., 2022, 13, 5947 CrossRef CAS.
- D. Goertsen, N. C. Flytzanis, N. Goeden, M. R. Chuapoco, A. Cummins, Y. Chen, Y. Fan, Q. Zhang, J. Sharma, Y. Duan, L. Wang, G. Feng, Y. Chen, N. Y. Ip, J. Pickel and V. Gradinaru, Nat. Neurosci., 2022, 25, 106–115 CrossRef CAS PubMed.
- N. Buss, L. Lanigan, J. Zeller, D. Cissell, M. Metea, E. Adams, M. Higgins, K. H. Kim, E. Budzynski, L. Yang, Y. Liu, M. Butt, O. Danos and M. Fiscella, Mol. Ther.–Methods Clin. Dev., 2022, 24, 342–354 CrossRef CAS PubMed.
- J. Hordeaux, E. L. Buza, C. Dyer, T. Goode, T. W. Mitchell, L. Richman, N. Denton, C. Hinderer, N. Katz, R. Schmid, R. Miller, G. R. Choudhury, M. Horiuchi, K. Nambiar, H. Yan, M. Li and J. M. Wilson, Hum. Gene Ther., 2020, 31, 808–818 CrossRef CAS PubMed.
- Y. Guo, J. Chen, W. Ji, L. Xu, Y. Xie, S. He, C. Lai, K. Hou, Z. Li, G. Chen and Z. Wu, Mol. Ther.–Methods Clin. Dev., 2023, 31, 101105 CrossRef.
- S. M. Salabarria, M. Corti, K. E. Coleman, M. B. Wichman, J. A. Berthy, P. D’Souza, C. J. Tifft, R. W. Herzog, M. E. Elder, L. R. Shoemaker, C. Leon-Astudillo, F. Tavakkoli, D. H. Kirn, J. D. Schwartz and B. J. Byrne, J. Clin. Invest., 2024, 134(1), e173510 CrossRef PubMed.
- J.-K. Y. Tan, D. L. Sellers, B. Pham, S. H. Pun and P. J. Horner, Front. Mol. Neurosci., 2016, 9 DOI:10.3389/fnmol.2016.00108.
- J. Yang, K. M. Luly and J. J. Green, WIRES Nanomed Nanobi., 2023, 15, e1853 CrossRef CAS PubMed.
- R. Xiong, G. Ling, Y. Zhang, J. Guan and P. Zhang, Brain-X, 2023, 1, e7 CrossRef.
- R. Palanki, S. K. Bose, A. Dave, B. M. White, C. Berkowitz, V. Luks, F. Yaqoob, E. Han, K. L. Swingle, P. Menon, E. Hodgson, A. Biswas, M. M. Billingsley, L. Li, F. Yiping, M. Carpenter, A. Trokhan, J. Yeo, N. Johana, T. Y. Wan, M.-G. Alameh, F. C. Bennett, P. B. Storm, R. Jain, J. Chan, D. Weissman, M. J. Mitchell and W. H. Peranteau, ACS Nano, 2023, 17, 13594–13610 CrossRef CAS PubMed.
- M. Amiri, S. Jafari, M. Kurd, H. Mohamadpour, M. Khayati, F. Ghobadinezhad, O. Tavallaei, H. Derakhshankhah, S. Sadegh Malvajerd and Z. Izadi, ACS Chem. Neurosci., 2021, 12, 4475–4490 CrossRef CAS PubMed.
- I. Khalin, N. Adarsh, M. Schifferer, A. Wehn, B. Groschup, T. Misgeld, A. Klymchenko and N. Plesnila, Small, 2022, 18, 2200302 CrossRef CAS.
- M. Pinto, V. Silva, S. Barreiro, R. Silva, F. Remião, F. Borges and C. Fernandes, Ageing Res. Rev., 2022, 79, 101658 CrossRef CAS.
- M. J. Haney, N. L. Klyachko, Y. Zhao, R. Gupta, E. G. Plotnikova, Z. He, T. Patel, A. Piroyan, M. Sokolsky, A. V. Kabanov and E. V. Batrakova, J. Controlled Release, 2015, 207, 18–30 CrossRef CAS.
- E. Bellotti, A. L. Schilling, S. R. Little and P. Decuzzi, J. Controlled Release, 2021, 329, 16–35 CrossRef CAS PubMed.
- A. Carpentier, M. Canney, A. Vignot, V. Reina, K. Beccaria, C. Horodyckid, C. Karachi, D. Leclercq, C. Lafon, J.-Y. Chapelon, L. Capelle, P. Cornu, M. Sanson, K. Hoang-Xuan, J.-Y. Delattre and A. Idbaih, Sci. Transl. Med., 2016, 8, 343re342 Search PubMed.
- P. Khare, S. X. Edgecomb, C. M. Hamadani, E. E. L. Tanner and D. S. Manickam, Adv. Drug Delivery Rev., 2023, 197, 114861 CrossRef CAS PubMed.
- A. Mwema, G. G. Muccioli and A. des Rieux, J. Controlled Release, 2023, 364, 435–457 CrossRef CAS PubMed.
- J. Tuma, Y.-J. Chen, M. G. Collins, A. Paul, J. Li, H. Han, R. Sharma, N. Murthy and H. Y. Lee, Biochemistry, 2023, 62(24), 3533–3547 CrossRef CAS PubMed.
- T. Kurano, T. Kanazawa, A. Ooba, Y. Masuyama, N. Maruhana, M. Yamada, S. Iioka, H. Ibaraki, Y. Kosuge, H. Kondo and T. Suzuki, J. Controlled Release, 2022, 344, 225–234 CrossRef CAS PubMed.
- D. Witzigmann, J. A. Kulkarni, J. Leung, S. Chen, P. R. Cullis and R. van der Meel, Adv. Drug Delivery Rev., 2020, 159, 344–363 CrossRef CAS PubMed.
- J. D. Gillmore, E. Gane, J. Taubel, J. Kao, M. Fontana, M. L. Maitland, J. Seitzer, D. O'Connell, K. R. Walsh, K. Wood, J. Phillips, Y. Xu, A. Amaral, A. P. Boyd, J. E. Cehelsky, M. D. McKee, A. Schiermeier, O. Harari, A. Murphy, C. A. Kyratsous, B. Zambrowicz, R. Soltys, D. E. Gutstein, J. Leonard, L. Sepp-Lorenzino and D. Lebwohl, N. Engl. J. Med., 2021, 385, 493–502 CrossRef CAS PubMed.
- L. Farbiak, Q. Cheng, T. Wei, E. Álvarez-Benedicto, L. T. Johnson, S. Lee and D. J. Siegwart, Adv. Mater., 2021, 33, 2006619 CrossRef CAS PubMed.
- Y. Lee, M. Jeong, J. Park, H. Jung and H. Lee, Exp. Mol. Med., 2023, 55, 2085–2096 CrossRef CAS PubMed.
- J. G. Rurik, I. Tombácz, A. Yadegari, P. O. Méndez Fernández, S. V. Shewale, L. Li, T. Kimura, O. Y. Soliman, T. E. Papp, Y. K. Tam, B. L. Mui, S. M. Albelda, E. Puré, C. H. June, H. Aghajanian, D. Weissman, H. Parhiz and J. A. Epstein, Science, 2022, 375, 91–96 CrossRef CAS PubMed.
- L. Breda, T. E. Papp, M. P. Triebwasser, A. Yadegari, M. T. Fedorky, N. Tanaka, O. Abdulmalik, G. Pavani, Y. Wang, S. A. Grupp, S. T. Chou, H. Ni, B. L. Mui, Y. K. Tam, D. Weissman, S. Rivella and H. Parhiz, Science, 2023, 381, 436–443 CrossRef CAS PubMed.
- G. Prakash, A. Shokr, N. Willemen, S. M. Bashir, S. R. Shin and S. Hassan, Adv. Drug Delivery Rev., 2022, 184, 114197 CrossRef CAS PubMed.
- R. Tenchov, R. Bird, A. E. Curtze and Q. Zhou, ACS Nano, 2021, 15, 16982–17015 CrossRef CAS PubMed.
- T. Nakamura, Y. Sato, Y. Yamada, M. M. Abd Elwakil, S. Kimura, M. A. Younis and H. Harashima, Adv. Drug Delivery Rev., 2022, 188, 114417 CrossRef CAS PubMed.
- S. Priya, V. M. Desai and G. Singhvi, ACS Omega, 2023, 8, 74–86 CrossRef CAS PubMed.
- S. Ndeupen, Z. Qin, S. Jacobsen, A. Bouteau, H. Estanbouli and B. Z. Igyártó, iScience, 2021, 24, 103479 CrossRef CAS PubMed.
- S. F. Dowdy, Nat. Biotechnol., 2017, 35, 222–229 CrossRef CAS PubMed.
- L. Zheng, S. R. Bandara, Z. Tan and C. Leal, Proc. Natl. Acad. Sci. U. S. A., 2023, 120, e2301067120 CrossRef CAS PubMed.
- A. Raguram, S. Banskota and D. R. Liu, Cell, 2022, 185, 2806–2827 CrossRef CAS PubMed.
- W. Zhang, A. Mehta, Z. Tong, L. Esser and N. H. Voelcker, Adv. Sci., 2021, 8, 2003937 CrossRef CAS PubMed.
- A. Joseph, G. M. Simo, T. Gao, N. Alhindi, N. Xu, D. J. Graham, L. J. Gamble and E. Nance, Biomaterials, 2021, 277, 121086 CrossRef CAS PubMed.
- M. Peviani, U. Capasso Palmiero, F. Cecere, R. Milazzo, D. Moscatelli and A. Biffi, Biomaterials, 2019, 209, 25–40 CrossRef CAS PubMed.
- Q. Li, X. Shao, X. Dai, Q. Guo, B. Yuan, Y. Liu and W. Jiang, NPG Asia Mater., 2022, 14, 14 CrossRef.
- N. Rabiee, S. Ahmadi, R. Afshari, S. Khalaji, M. Rabiee, M. Bagherzadeh, Y. Fatahi, R. Dinarvand, M. Tahriri, L. Tayebi, M. R. Hamblin and T. J. Webster, Adv. Ther., 2021, 4, 2000076 CrossRef CAS.
- Y. Anraku, H. Kuwahara, Y. Fukusato, A. Mizoguchi, T. Ishii, K. Nitta, Y. Matsumoto, K. Toh, K. Miyata, S. Uchida, K. Nishina, K. Osada, K. Itaka, N. Nishiyama, H. Mizusawa, T. Yamasoba, T. Yokota and K. Kataoka, Nat. Commun., 2017, 8, 1001 CrossRef CAS PubMed.
- H. Wu, B. Peng, F. S. Mohammed, X. Gao, Z. Qin, K. N. Sheth, J. Zhou and Z. Jiang, Small, 2022, 18, 2107126 CrossRef CAS PubMed.
- Y. Rui, D. R. Wilson, J. Choi, M. Varanasi, K. Sanders, J. Karlsson, M. Lim and J. J. Green, Sci. Adv., 2019, 5, eaay3255 CrossRef CAS PubMed.
- D. Ye, T. Zimmermann, V. Demina, S. Sotnikov, C. L. Ried, H. Rahn, M. Stapf, C. Untucht, M. Rohe, G. C. Terstappen, K. Wicke, M. Mezler, H. Manninga and A. H. Meyer, Nanoscale Adv., 2021, 3, 2488–2500 RSC.
- S. Banskota, A. Raguram, S. Suh, S. W. Du, J. R. Davis, E. H. Choi, X. Wang, S. C. Nielsen, G. A. Newby, P. B. Randolph, M. J. Osborn, K. Musunuru, K. Palczewski and D. R. Liu, Cell, 2022, 185, 250–265 CrossRef CAS PubMed.
- Y. Liang, L. Duan, J. Lu and J. Xia, Theranostics, 2021, 11, 3183–3195 CrossRef CAS PubMed.
-
S. Dubey, Z. Chen, A. Talis, A. Molotkov, A. Ali, A. Mintz and F. Momen-Heravi, bioRxiv, 2023, preprint, DOI:10.1101/2023.06.09.542202.
- K. Adlerz, D. Patel, J. Rowley, K. Ng and T. Ahsan, Stem Cell Res., 2020, 48, 101978 CrossRef CAS PubMed.
- A. H. Berggreen, J. L. Petersen, L. Lin, K. Benabdellah and Y. Luo, J. Extracell. Biol., 2023, 2, e111 CrossRef CAS.
- Y. Pan, J. Yang, X. Luan, X. Liu, X. Li, J. Yang, T. Huang, L. Sun, Y. Wang, Y. Lin and Y. Song, Sci. Adv., 2019, 5, eaav7199 CrossRef CAS PubMed.
- A. Tasset, A. Bellamkonda, W. Wang, I. Pyatnitskiy, D. Ward, N. Peppas and H. Wang, Nanoscale, 2022, 14, 3698–3719 RSC.
- V. Bartanusz, D. Jezova, B. Alajajian and M. Digicaylioglu, Ann. Neurol., 2011, 70, 194–206 CrossRef PubMed.
- P. Yin, W. Liang, B. Han, Y. Yang, D. Sun, X. Qu, Y. Hai and D. Luo, Small Methods, 2024, 8, 2301173 CrossRef PubMed.
- F. Wu, P. Wang, X. Wei, Y. Yang, A. Al Mamun, X. Zhang, Y. Zhu, T. Mo, H. Zhang, C. Jiang, J. Hu and J. Xiao, Mater. Today Bio, 2023, 18, 100546 CrossRef CAS PubMed.
- M. D. Sweeney, Z. Zhao, A. Montagne, A. R. Nelson and B. V. Zlokovic, Physiol. Rev., 2019, 99, 21–78 CrossRef CAS PubMed.
- N. J. Abbott, L. Rönnbäck and E. Hansson, Nat. Rev. Neurosci., 2006, 7, 41–53 CrossRef CAS PubMed.
- D. Chenthamara, S. Subrmaniam, S. Ramakrishnan, S. Krishnaswamy, M. Essa, F.-H. Lin and M. Qoronfleh, Biomater. Res., 2019, 23, 20 CrossRef CAS PubMed.
- M. J. Fowler, J. D. Cotter, B. E. Knight, E. M. Sevick-Muraca, D. I. Sandberg and R. W. Sirianni, Adv. Drug Delivery Rev., 2020, 165–166, 77–95 CrossRef CAS PubMed.
- S.-M. P. Fletcher, M. Choi, R. Ramesh and M. A. O'Reilly, Ultrasound Med. Biol., 2021, 47, 1747–1760 CrossRef PubMed.
- A.-S. Montero, F. Bielle, L. Goldwirt, A. Lalot, G. Bouchoux, M. Canney, F. Belin, K. Beccaria, P.-F. Pradat, F. Salachas, S. Boillée, C. Lobsiger, C. Lafon, J.-Y. Chapelon and A. Carpentier, Ultrasound Med. Biol., 2019, 45, 2417–2426 CrossRef PubMed.
- S. P. Fletcher, M. Choi, N. Ogrodnik and M. A. O'Reilly, Theranostics, 2020, 10, 7758–7774 CrossRef CAS PubMed.
- A. H. Payne, G. W. Hawryluk, Y. Anzai, H. Odéen, M. A. Ostlie, E. C. Reichert, A. J. Stump, S. Minoshima and D. J. Cross, Neural Regener. Res., 2017, 12, 2045–2049 CrossRef PubMed.
- S. I. Rapoport, Cell. Mol. Neurobiol., 2000, 20, 217–230 CrossRef CAS PubMed.
- K. L. Black, Adv. Drug Delivery Rev., 1995, 15, 37–52 CrossRef CAS PubMed.
- C. Y. Lee, W. H. Chooi, S. Y. Ng and S. Y. Chew, Bioeng. Transl. Med., 2023, 8, e10389 CrossRef CAS PubMed.
- S. Jain, M. Malinowski, P. Chopra, V. Varshney and T. R. Deer, Expert Opin. Drug Delivery, 2019, 16, 815–822 CrossRef PubMed.
- E. M. Delhaas and F. J. P. M. Huygen, BJA Educ., 2020, 20, 51–57 CrossRef CAS PubMed.
- V. Goel, Y. Yang, S. Kanwar, R. K. Banik, A. M. Patwardhan, M. Ibrahim, E. Sivanesan and H. Shankar, Neuromodulation, 2021, 24, 1181–1189 CrossRef PubMed.
- K. Bey, C. Ciron, L. Dubreil, J. Deniaud, M. Ledevin, J. Cristini, V. Blouin, P. Aubourg and M. A. Colle, Gene Ther., 2017, 24, 325–332 CrossRef CAS PubMed.
- A. Ledeboer, B. M. Jekich, E. M. Sloane, J. H. Mahoney, S. J. Langer, E. D. Milligan, D. Martin, S. F. Maier, K. W. Johnson, L. A. Leinwand, R. A. Chavez and L. R. Watkins, Brain, Behav., Immun., 2007, 21, 686–698 CrossRef CAS PubMed.
- R. R. Lonser, M. Sarntinoranont, P. F. Morrison and E. H. Oldfield, J. Neurosurg., 2015, 122, 697–706 Search PubMed.
- M. T. Rocco, A. S. Akhter, D. J. Ehrlich, G. C. Scott, C. Lungu, V. Munjal, A. Aquino, R. R. Lonser, M. S. Fiandaca, M. Hallett, J. D. Heiss and K. S. Bankiewicz, Mol. Ther., 2022, 30, 3632–3638 CrossRef CAS PubMed.
- R. M. Richardson, K. S. Bankiewicz, C. W. Christine, A. D. V. Laar, R. E. Gross, R. Lonser, S. A. Factor, S. K. Kostyk, A. P. Kells, B. Ravina and P. S. Larson, J. Neurol. Neurosurg. Psychiatry., 2020, 91, 1210–1218 CrossRef PubMed.
- T. S. Pearson, N. Gupta, W. San Sebastian, J. Imamura-Ching, A. Viehoever, A. Grijalvo-Perez, A. J. Fay, N. Seth, S. M. Lundy, Y. Seo, M. Pampaloni, K. Hyland, E. Smith, G. de Oliveira Barbosa, J. C. Heathcock, A. Minnema, R. Lonser, J. B. Elder, J. Leonard, P. Larson and K. S. Bankiewicz, Nat. Commun., 2021, 12, 4251 CrossRef CAS PubMed.
- E. Moriyama, M. Salcman and R. D. Broadwell, Surg. Neurol., 1991, 35, 177–182 CrossRef CAS PubMed.
- C. Chu, A. Jablonska, Y. Gao, X. Lan, W. G. Lesniak, Y. Liang, G. Liu, S. Li, T. Magnus, M. Pearl, M. Janowski and P. Walczak, Nat. Protoc., 2022, 17, 76–94 CrossRef CAS PubMed.
- T. Okuyama, Y. Eto, N. Sakai, K. Nakamura, T. Yamamoto, M. Yamaoka, T. Ikeda, S. So, K. Tanizawa, H. Sonoda and Y. Sato, Mol. Ther., 2021, 29, 671–679 CrossRef CAS PubMed.
- K. S. Chew, R. C. Wells, A. Moshkforoush, D. Chan, K. J. Lechtenberg, H. L. Tran, J. Chow, D. J. Kim, Y. Robles-Colmenares, D. B. Srivastava, R. K. Tong, M. Tong, K. Xa, A. Yang, Y. Zhou, P. Akkapeddi, L. Annamalai, K. Bajc, M. Blanchette, G. M. Cherf, T. K. Earr, A. Gill, D. Huynh, D. Joy, K. N. Knight, D. Lac, A. W.-S. Leung, K. W. Lexa, N. P. D. Liau, I. Becerra, M. Malfavon, J. McInnes, H. N. Nguyen, E. I. Lozano, M. E. Pizzo, E. Roche, P. Sacayon, M. E. K. Calvert, R. Daneman, M. S. Dennis, J. Duque, K. Gadkar, J. W. Lewcock, C. S. Mahon, R. Meisner, H. Solanoy, R. G. Thorne, R. J. Watts, Y. J. Y. Zuchero and M. S. Kariolis, Nat. Commun., 2023, 14, 5053 CrossRef CAS PubMed.
- G. Pornnoppadol, L. G. Bond, M. J. Lucas, J. M. Zupancic, Y.-H. Kuo, B. Zhang, C. F. Greineder and P. M. Tessier, Cell Chem. Biol., 2024, 31(2), 361–372 CrossRef CAS PubMed.
- W. M. Pardridge, Front. Drug Deliv., 2023, 3, 1227816 CrossRef PubMed.
- L.-A. Keller, O. Merkel and A. Popp, Drug Delivery Transl. Res., 2022, 12, 735–757 CrossRef PubMed.
- J. Blesa, J. A. Pineda-Pardo, K.-i. Inoue, C. Gasca-Salas, T. Balzano, N. L.-G. Del Rey, A. Reinares-Sebastián, N. Esteban-García, R. Rodríguez-Rojas, R. Márquez, M. Ciorraga, M. del Álamo, L. García-Cañamaque, S. Ruiz de Aguiar, I. Rachmilevitch, I. Trigo-Damas, M. Takada and J. A. Obeso, Sci. Adv., 2023, 9, eadf4888 CrossRef CAS PubMed.
- A. R. Rezai, P.-F. D'Haese, V. Finomore, J. Carpenter, M. Ranjan, K. Wilhelmsen, R. I. Mehta, P. Wang, U. Najib, C. Vieira Ligo Teixeira, T. Arsiwala, A. Tarabishy, P. Tirumalai, D. O. Claassen, S. Hodder and M. W. Haut, N. Engl. J. Med., 2024, 390, 55–62 CrossRef CAS PubMed.
- Y.-H. Lao, R. Ji, J. K. Zhou, K. J. Snow, N. Kwon, E. Saville, S. He, S. Chauhan, C.-W. Chi, M. S. Datta, H. Zhang, C. H. Quek, S. S. Cai, M. Li, Y. Gaitan, L. Bechtel, S.-Y. Wu, C. M. Lutz, R. Tomer, S. A. Murray, A. Chavez, E. E. Konofagou and K. W. Leong, Proc. Natl. Acad. Sci. U. S. A., 2023, 120, e2302910120 CrossRef CAS PubMed.
- M. Hörner, C. Jerez-Longres, A. Hudek, S. Hook, O. S. Yousefi, W. W. A. Schamel, C. Hörner, M. D. Zurbriggen, H. Ye, H. J. Wagner and W. Weber, Sci. Adv., 2021, 7, eabf0797 CrossRef PubMed.
-
Z. Gao, E. T. David, T. W. Leong, X. Li, Q. Cai, J. Mwirigi, M. Giannotta, E. Dejana, J. Wiggins, S. Krishnagiri, R. M. Bachoo, T. J. Price and Z. Qin, bioRxiv, 2022, preprint, DOI:10.1101/2022.05.20.492752.
- X. Li, V. Vemireddy, Q. Cai, H. Xiong, P. Kang, X. Li, M. Giannotta, H. N. Hayenga, E. Pan, S. R. Sirsi, C. Mateo, D. Kleinfeld, C. Greene, M. Campbell, E. Dejana, R. Bachoo and Z. Qin, Nano Lett., 2021, 21, 9805–9815 CrossRef CAS PubMed.
- J. Chen, M. Yuan, C. A. Madison, S. Eitan and Y. Wang, J. Controlled Release, 2022, 345, 557–571 CrossRef CAS PubMed.
- E. Syková and C. Nicholson, Physiol. Rev., 2008, 88, 1277–1340 CrossRef PubMed.
- D. J. Wolak and R. G. Thorne, Mol. Pharm., 2013, 10, 1492–1504 CrossRef CAS PubMed.
- M. K. Rasmussen, H. Mestre and M. Nedergaard, Physiol. Rev., 2022, 102, 1025–1151 CrossRef CAS PubMed.
- E. A. Nance, G. F. Woodworth, K. A. Sailor, T.-Y. Shih, Q. Xu, G. Swaminathan, D. Xiang, C. Eberhart and J. Hanes, Sci. Transl. Med., 2012, 4, 149ra119 Search PubMed.
- C. Nicholson, Phys. Today, 2022, 75, 26–32 CrossRef CAS.
- P. T. Nguyen, L. C. Dorman, S. Pan, I. D. Vainchtein, R. T. Han, H. Nakao-Inoue, S. E. Taloma, J. J. Barron, A. B. Molofsky, M. A. Kheirbek and A. V. Molofsky, Cell, 2020, 182, 388–403 CrossRef CAS PubMed.
- J. M. Tarasoff-Conway, R. O. Carare, R. S. Osorio, L. Glodzik, T. Butler, E. Fieremans, L. Axel, H. Rusinek, C. Nicholson, B. V. Zlokovic, B. Frangione, K. Blennow, J. Ménard, H. Zetterberg, T. Wisniewski and M. J. de Leon, Nat. Rev. Neurol., 2015, 11, 457–470 CrossRef CAS PubMed.
- Z.-G. Wang, L. Wang, D. C. Lamb, H.-J. Chen, Y. Hu, H.-P. Wang, D.-W. Pang and S.-L. Liu, Nano Lett., 2021, 21, 642–650 CrossRef CAS PubMed.
- F. N. Soria, C. Miguelez, O. Peñagarikano and J. Tønnesen, Front. Neurosci., 2020, 14 DOI:10.3389/fnins.2020.570750.
- A. G. Godin, J. A. Varela, Z. Gao, N. Danné, J. P. Dupuis, B. Lounis, L. Groc and L. Cognet, Nat. Nanotechnol., 2017, 12, 238–243 CrossRef CAS PubMed.
- C. Paviolo, J. S. Ferreira, A. Lee, D. Hunter, I. Calaresu, S. Nandi, L. Groc and L. Cognet, Nano Lett., 2022, 22, 6849–6856 CrossRef CAS PubMed.
- J. Tønnesen, S. Hrabětová and F. N. Soria, Neurobiol. Dis., 2023, 177, 105981 CrossRef PubMed.
- J. Tønnesen, V. V. G. K. Inavalli and U. V. Nägerl, Cell, 2018, 172, 1108–1121 CrossRef PubMed.
- C. Nicholson, J. Neurosci. Methods, 1993, 48, 199–213 CrossRef CAS PubMed.
- M. P. G. Bellettieri, M. Anderloni, V. Rass, P. Kindl, K. Donadello, F. S. Taccone, R. Helbok and E. Gouvea Bogossian, Clin. Neurol. Neurosurg., 2023, 234, 108011 CrossRef PubMed.
- C. Nicholson, J. R. Soc., Interface, 2023, 20, 20230223 CrossRef PubMed.
- N. A. Jessen, A. S. F. Munk, I. Lundgaard and M. Nedergaard, Neurochem. Res., 2015, 40, 2583–2599 CrossRef CAS PubMed.
- S. Holstein-Rønsbo, Y. Gan, M. J. Giannetto, M. K. Rasmussen, B. Sigurdsson, F. R. M. Beinlich, L. Rose, V. Untiet, L. M. Hablitz, D. H. Kelley and M. Nedergaard, Nat. Neurosci., 2023, 26, 1042–1053 CrossRef PubMed.
-
M. J. Sætra, A. J. Ellingsrud and M. E. Rognes, bioRxiv, 2023, preprint, DOI:10.1101/2023.03.06.531247.
- C. Nicholson and S. Hrabětová, Biophys. J., 2017, 113, 2133–2142 CrossRef CAS PubMed.
- L. Tao and C. Nicholson, J. Theor. Biol., 2004, 229, 59–68 CrossRef CAS PubMed.
- M. Aryal, M. M. Azadian, A. R. Hart, N. Macedo, Q. Zhou, E. L. Rosenthal and R. D. Airan, J. Controlled Release, 2022, 349, 434–442 CrossRef CAS PubMed.
- Y. Liu, S. Paliwal, K. S. Bankiewicz, J. R. Bringas, G. Heart, S. Mitragotri and M. R. Prausnitz, AAPS PharmSciTech, 2010, 11, 1005–1017 CrossRef PubMed.
- D. S. Hersh, B. A. Nguyen, J. G. Dancy, A. R. Adapa, J. A. Winkles, G. F. Woodworth, A. J. Kim and V. Frenkel, Brain Res., 2016, 1646, 543–550 CrossRef CAS PubMed.
- C. T. Curley, B. P. Mead, K. Negron, N. Kim, W. J. Garrison, G. W. Miller, K. M. Kingsmore, E. A. Thim, J. Song, J. M. Munson, A. L. Klibanov, J. S. Suk, J. Hanes and R. J. Price, Sci. Adv., 2020, 6, eaay1344 CrossRef CAS PubMed.
- D. Ye, S. Chen, Y. Liu, C. Weixel, Z. Hu, J. Yuan and H. Chen, Proc. Natl. Acad. Sci. U. S. A., 2023, 120, e2212933120 CrossRef CAS PubMed.
- D. H. Bryant, A. Bashir, S. Sinai, N. K. Jain, P. J. Ogden, P. F. Riley, G. M. Church, L. J. Colwell and E. D. Kelsic, Nat. Biotechnol., 2021, 39, 691–696 CrossRef CAS PubMed.
- P. J. Ogden, E. D. Kelsic, S. Sinai and G. M. Church, Science, 2019, 366, 1139–1143 CrossRef CAS PubMed.
- A. Hasanzadeh, M. R. Hamblin, J. Kiani, H. Noori, J. M. Hardie, M. Karimi and H. Shafiee, Nano Today, 2022, 47, 101665 CrossRef CAS PubMed.
-
Y. Xu, S. Ma, H. Cui, J. Chen, S. Xu, K. Wang, A. Varley, R. X. Z. Lu, B. Wang and B. Li, bioRxiv, 2023, preprint, DOI:10.1101/2023.06.01.543345.
- T. Gopesh, J. H. Wen, D. Santiago-Dieppa, B. Yan, J. S. Pannell, A. Khalessi, A. Norbash and J. Friend, Sci. Robot., 2021, 6, eabf0601 CrossRef PubMed.
- L. Pancaldi, P. Dirix, A. Fanelli, A. M. Lima, N. Stergiopulos, P. J. Mosimann, D. Ghezzi and M. S. Sakar, Nat. Commun., 2020, 11, 6356 CrossRef CAS PubMed.
- R. Dreyfus, Q. Boehler, S. Lyttle, P. Gruber, J. Lussi, C. Chautems, S. Gervasoni, J. Berberat, D. Seibold, N. Ochsenbein-Kölble, M. Reinehr, M. Weisskopf, L. Remonda and B. J. Nelson, Sci. Robot., 2024, 9, eadh0298 CrossRef CAS PubMed.
- L. E. Savastano, Q. Zhou, A. Smith, K. Vega, C. Murga-Zamalloa, D. Gordon, J. McHugh, L. Zhao, M. M. Wang, A. Pandey, B. G. Thompson, J. Xu, J. Zhang, Y. E. Chen, E. J. Seibel and T. D. Wang, Nat. Biomed. Eng., 2017, 1, 0023 CrossRef CAS PubMed.
|
This journal is © The Royal Society of Chemistry 2024 |