DOI:
10.1039/D3MH01926H
(Opinion)
Mater. Horiz., 2024,
11, 1838-1842
Oxovanadium electronics for in-memory, neuromorphic, and quantum computing applications
Received
15th November 2023
, Accepted 5th February 2024
First published on 6th February 2024
Abstract
Vanadium is a critical raw material. In the nearby future, it may, however, become one of the key elements of computer devices based on two-dimensional arrays of spin qubits for quantum information processing or charge- and resistance-based data memory cells for non-volatile in-memory and neuromorphic computing. The research and development (R&D) of vanadium-containing electronic materials and methods for their responsible fabrication underpins the transition to innovative hybrid semiconductors for energy- and resource-efficient memory and information processing technologies. The combination of standard and emerging solid-state semiconductors with stimuli-responsive oxo complexes of vanadium(IV,V) is envisioned to result in electronics with a new room-temperature device nanophysics, and the ability to modulate and control it at the sub-nanometer level. The development of exponential (Boolean) logics based on the oxovanadium-comprising circuitry and crossbar arrays of individual memristive cells for in-memory computing, the implementation of basic synaptic functions via dynamic electrical pulses for neuromorphic computing, and the readout and control of spin networks and interfaces for quantum computing are strategically important future areas of molecular chemistry and applied physics of vanadium.
Need for transformation of current silicon technology
The disruptive transformation of the landscape of modern computer technologies to produce semiconductor microcircuits into energy- and resource-efficient micro- and nanoelectronics with increased functionality (in particular, “More than Moore” devices) requires innovations and bundled efforts at different levels of fundamental research and technology development. This R&D is especially needed due to the growing influence of high power-consuming artificial intelligence (AI) algorithms1 (like e.g. ChatGPT) and the overall negative impact of information and communications technologies (ICT) on the environment and economy.2,3 Research into the spin properties of molecular qubits and charge properties of molecular multi-logic networks, that provide digital (discrete) or analog (continuous) switching functions at room temperature and low potentials on complementary metal oxide semiconductor (CMOS) surfaces as well as surfaces extended by functional two-dimensional (2D) materials,4 is a promising path not only to greener computing, but also to technologies with more natural interfaces. The academic silicon technology roadmap offered by the Japan Society of Applied Physics (JSAP) suggests that the integration of CMOS technology with 1-nm molecular technology might be achieved by 2045.
Responsible electronics, miniaturisation, and natural interfaces (see Si technology roadmap of JSAP), that open access to new added functionality and efficiency of future computer chips, are the main drivers of the Si-based computing technology transformation. The search for innovative materials as active electronic (memristive5) layers on a single chip, inspired in many ways by brain biology (Table 1), is at the heart of highly sought-after transition to green IT.
Table 1 CMOS technology vs. brain biology. A comparison of computer and bioelectronic attributes
|
Insulating material |
Voltage-gated channels to control electrical current |
Memory storage units |
Arithmetic and logic operations |
Technical systems |
SiO2 |
Thin-film field-effect transistor |
Logic gate (e.g., memristor) on a crossbar array |
Arithmetic logic unit |
Biological systems |
DNA |
Cell membrane ion channel |
Synapse in a neural network |
Neuron |
From a chemical perspective, breakthroughs in the eco-friendlier preparation of new functional electron transport and switching compounds and related bioinspired/biomimetic materials can be achieved through automation in the chemistry lab.6 The latter allows among other things for controlled, computer-assisted reactions in continuous flow supported by in-line/online acquisition of data. However, this automation also requires significant improvements in the data- and energy-efficient machine learning models. From an engineering perspective, it is necessary to develop methods for non-destructive, spatially controlled processing of new, synthesised, low-dimensional compounds from a solution or from the gas phase onto scalable device architectures. For example, mass-selective ion soft-landing7 and ink-jet printing8 techniques offer significant opportunities for creating switchable molecularly functionalised surfaces outside of cleanroom conditions.
A look at the landscape of various semiconductor materials currently being developed for CMOS and beyond-CMOS technologies shows that compounds containing vanadium atoms as redox- and spin-active centres have the potential to become important components of next-generation computing devices.
Vanadium as a potential game changer
The unique combination and responsive character of physical state variables (charge, spin, and phase) of vanadium,9 a transition metal [Ar]3d34s2 from group 5 of the periodic table, grants access to advanced electrical, magnetic, optical, thermochromic, and electrochemical properties of its highest valent (V5+) and lower valent (V4+, V3+, and V2+) compounds.10–12 Also, the electronic properties of materials from other chemical classes benefit from the inclusion of vanadium, such as e.g. silicon carbide, where the spin-½ V4+ ions can be implanted as quantum emitters.13 Cesium vanadium antimonide14 (CsV3Sb5) exhibits unconventional physics15 including bulk superconductivity in single crystals due to a two-dimensional (2D) Kagome network of mixed-valent vanadium ions. These properties are of great importance for the emerging era of AI systems, quantum computation and communication, as well as for the electrification of the automotive industry.
Vanadium supply
Vanadium belongs to the fifth list 2023 of critical raw materials for the European Union (EU).16 Its world's largest producers are China (>60%), Russia, and South Africa, which account for approx. 75% of global vanadium supply.17 In view of the growing demand18 for this metal and its compounds, it is important that the European industrial sector considers its “vanadium sovereignty”. One of the strategic projects for domestic supply is the Vanadium Recovery Project in Finland.17
In addition to the current extensive industrial research on vanadium as a battery material,19 growing attention to vanadium is attached to its oxide and oxo compounds for digital and analog computing. According to the ‘Supply chain analysis and material demand forecast in strategic technologies and sectors in the EU – A foresight study’ by the European Commission,20 vanadium(V) pentoxide V2O5 as a processed material can be used in the data transmission networks, where printed circuit boards, optoelectronic components, semiconductors, and sensor chips play a significant role. The production of V2O5 from the vanadinite mineral21 Pb5(VO4)3Cl with naturally occurring deposits, e.g., in Spain or Scotland might provide an interesting source of vanadium for innovative vanadium-based nanomaterials for the EU. It is noteworthy that V2O5 is often a precursor in the wet-chemical synthesis of starting reagents for soluble molecular vanadium oxides, so-called polyoxovanadates. The latter also occur in natural mineral phases.22 Another type of oxovanadium compounds that may have potential applications in some form in computing are vanadyl porphyrins.23 These vanadium(IV) complexes (see, a crystal structure of a synthetic vanadyl petroporphyrin24) were detected e.g. in Venezuela Orinoco heavy crude oil by using Fourier transform-ion cyclotron resonance mass spectrometry25 or could be extracted from oil of the North Buzachi oil-and-gas-bearing region in Kazakhstan.26 Overall, fully-oxidised, mixed-valent, and fully-reduced polyoxovanadates27–29 and macrocyclic vanadyl compounds30–32 are currently being actively studied in the fields of data storage, data processing, and biomedical technologies.33
Oxides and oxo complexes of vanadium
In the area of oxide-based memory materials, vanadium dioxide34 VO2 and trivanadium pentoxide35 V3O5 thin films have been found to show thermally induced insulator-to-metal transition behaviour. This property and the emerging physics have great implication for neuromorphic computing36 with vanadium-based memristors as artificial synapses.
Integrating molecular compounds with discrete energy levels into e.g. memristive in-memory computing approaches37,38 should provide higher levels of precision and spatial control over the transfer of charge and spin through the switching material. This can be expected based on the diverse effects of tailored molecular functionalisation on the physical properties of e.g. two-dimensional (2D) material surfaces.39–44 Transferring the underlying principles of redox-based memristive switching of metal-oxide devices45 to the level of interaction of molecular compounds, containing redox/spin switchable and/or photo inducible metal centres, with bound resistive oxide layers might become a useful lever for modulating electron transport and even for programming memristive characteristics of such electronic and spintronic heterostructures.
Molecular congeners of vanadium oxides46 are mono- and polynuclear oxovanadium complexes. The latter can be divided into two qualified classes with the far-reaching potential for hybrid green IT technologies. Class I is represented by the neutral vanadyl complexes with e.g. dipivaloylmethane,47 phthalocyanine48 (Pc) or tetraphenylporphyrinate ligands.49Fig. 1a illustrates one of these compounds, namely VOPc that is synthesised50 from phthalonitrile and VCl3. The remarkable electronic and magnetic behaviour of I is due to the critical role of the vanadyl group51 VO2+ in a square-pyramidal coordination environment. These complexes exhibit the potential in the form of processable molecular spin qubits with long coherence times for quantum memories.
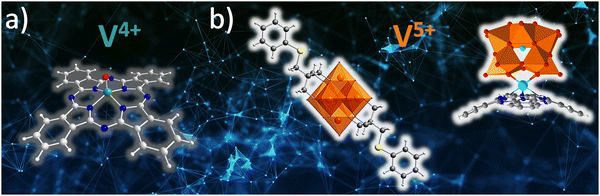 |
| Fig. 1 Selected examples of micro-spectroscopically investigated spin- and redox-active oxovanadium structures. The structures are supported by organic ligands providing a stable interface with the substrate surface and determined by single-crystal X-ray diffraction: (a) sublimable VOPc compound with a V4+ centre and (b) solution-processable [V6O13{(OCH2)3C-R}2]2− (R is an organic or organometallic moiety) and [HV12O32Cl(LnPc)]3− (Ln is a lanthanide; Pc is phthalocyanine) with V5+ centres. Counter cations of the anionic compounds are not shown. Colour code: V4+ = green, V5+ = orange, O = red, S = yellow, Cl = pale green, N = blue, C = grey, Ln = turquoise. | |
The vanadyl VO2+ can be found in the mineral cavansite Ca(VO)Si4O10·4H2O, but also in synthetic semimetal-functionalised polyoxovanadates27 such as e.g. the [V12As8O40(HCO2)]5− anion52 with V4+8V5+4 centres from class II. This spherically shaped, arsenato-substituted polyoxovanadate has been regarded as a quantum cellular automata.53,54
In addition to fully-reduced and mixed-valent derivatives, class II contains fully-oxidised polyoxovanadate anions, charged balanced e.g. by quaternary alkyl ammonium or alkali metal cations. Their high-performance representatives (Fig. 1b and c), the bis(trisalkoxo)-ligated Lindqvist-type hexavanadates55 with octahedrally coordinated vanadium(V) centres and the tubular dodecavanadate cages composed of square-pyramidal VO55− groups (one lacunary position is covalently blocked by a lanthanide (Ln) phthalocyanine moiety56) have emerged to be promising polyoxometalate (POM) candidates for molecular charge- and resistance-based memories.57,58 These ion species show unprecedented physical multi-state switching at the level of single molecules59,60 as well as thin films61 in response to an external potential-induced electrical field of a scanning tunneling microscope (STM) tip. As soluble compounds, these polyoxovanadates can be processed from solution to the gas phase62 and onto surfaces. Thus, their synthesis could be scaled up by automating reactions in a continuous flow that follows a low thermal budget approach in the preparation of switching materials with improved chemical and waste management. Materials reinforced with such POM structures promise to open up a revolutionary way to store digital information in wafer-scale hybrid devices, including neuromorphic devices based on ink-jet printed filaments.63
The molecular candidates from classes I and II also provide great opportunities for the realisation of brain-inspired molecularly empowered classic and quantum logic gates for information processing,64e.g. by the on-surface implementation of low-/high-potential counterpart switches with substantially different band gaps, spin qubit arrays, and/or by tuning the molecular conductance through counter cations in the case of polyoxoanionic ground-state structures. Their integration as spin qubits, electrical or opto-electrical switches, and/or conductance modulating agents at the level of single molecules or nano-machined thin films onto CMOS compatible material systems clearly paves the way for wafer-scale bottom-up neuromorphic electronics and spintronics. This rapidly developing field of materials can be seen as part of molecular cybernetics, i.e. the integration of molecular charge- and spin-based electronics and computational cybernetics. However, the technologically crucial transition from fundamental classical or spin-polarised STM-based model studies to real-world electrical contacting of I and II remains the biggest challenge of research and development at the interface of molecular inorganic chemistry, condensed matter solid physics, and device engineering.
Polyoxovanadates: from single molecules to layers – what's next?
Recently, a technologically important departure from experiments with single molecules65 to the solution-processed formation and multi-state switching of monolayers of polyoxovanadates61 has been realised. The latter can be modified into structured patterns by using STM nanomachining. However, there remain a number of important unresolved issues at the level of single molecules and thin films, in particular multi-state on/off reversibility and hysteresis behaviour of polyoxovanadate compounds, that need to be addressed in further research.
The combination of polyoxovanadates with the family of existing, technically suitable switching materials (e.g., metal oxides,66 metal alloys as phase change materials,67 2D transition metal chalcogenides,68 and MXenes69), that are currently being exploited or developed for digital and analog computing, represents another challenging task. The combination of both classes I and II together in one hybrid switching material system might offer an interesting approach to exponential logics. Moreover, due to their rich molecular magnetism, the possibilities of chemical design70 of reduced and mixed-valent polyoxovanadates as potential spin qubits merit further investigation in the field of spintronics and quantum information. The role of countercations71 in the electron transport and switching properties of devices with embedded POM functionalities is not yet fully understood, so that further mechanistic studies are needed.
Concluding remarks
Key points:
– Vanadium is a critical raw material.
– Vanadium oxides have characteristics important for quantum and memristive materials.
– VO2 thin films exhibit thermally induced insulator-to-metal transition.
– V2O5 is often used in the synthesis of molecular oxovanadium(IV,V) complexes.
– Vanadyl fragment is an important object of research in quantum information processing.
– Polyoxovanadates behave as electrically accessible multi-state switches at room temperature, currently exhibiting retention times ranging from a few ms to approximately 300 ms.
– Electrical contacting and integration of oxovanadium(IV,V) thin films at the wafer scale is one of the biggest challenges towards functional molecular electronics and molecular memristive computing.
Further research and development should be aimed at (i) the computer-assisted, controlled wet-chemical synthesis, (ii) surface engineering of large-area ‘oxovanadium switch–electrode surface’ interactions, (iii) solution-processed molecular printing of macro-/micro-/nano-objects, and (iv) their electrical measurements by a macroscopic external voltage source. In this endeavor, one important challenge is the tailoring of oxovanadium(IV,V) compound ligands to achieve chemically and thermally stable, surface-selective structures and their stimuli-responsive arrays, as well as to enhance interface effects on the physical properties of the switching materials in contact with technical electrode contacts. These studies will ultimately pave the way for the determination of important device parameters with integrated oxovanadium electronics such as endurance, retention times, uniformity, statistical variability, and electrical and mechanical reliability.
Conflicts of interest
There are no conflicts to declare.
Acknowledgements
This work was supported by the Leibniz Association through the Leibniz Collaborative Excellence funding program (iMolKit).
References
- A. A. Conklin and S. Kumar, Nat. Electron., 2023, 6, 464–466 CrossRef
.
- E. Williams, Nature, 2011, 479, 354–358 CrossRef CAS PubMed
.
- X. Zhang and C. Wie, Resour., Conserv. Recycl., 2022, 185, 106477 CrossRef
.
- M. C. Lemme, D. Akinwande, C. Huyghebaert and C. Stampfer, Nat. Commun., 2022, 13, 1392 CrossRef CAS PubMed
.
- M. Lanza, A. Sebastian, W. D. Lu, M. Le Gallo, M.-F. Chang, D. Akinwande, F. M. Puglisi, H. N. Alshareef, M. Liu and J. B. Roldan, Science, 2022, 376, eabj9979 CrossRef CAS PubMed
.
- M. Abolhasani and E. Kumacheva, Nat. Synth., 2023, 2, 483–492 CrossRef
.
- H. Y. Samayoa-Oviedo, K.-A. Behrend, S. Kawa, H. Knorke, P. Su, M. E. Belov, G. Anderson, J. Warneke and J. Laskin, Analyt. Chem., 2021, 93, 14489–14496 CrossRef CAS PubMed
.
- A. Matavž and B. Malič, J. Sol-Gel Sci. Technol., 2018, 87, 1–21 CrossRef
.
- J. P. Gustafsson, Appl. Geochem., 2019, 102, 1–125 CrossRef CAS
.
- C.-J. Yu, M. J. Graham, J. M. Zadrozny, J. Niklas, M. D. Krzyaniak, M. R. Wasielewski, O. G. Poluektov and D. E. Freedman, J. Am. Chem. Soc., 2016, 138, 14678–14685 CrossRef CAS PubMed
.
- M. Dorn, J. Kalmbach, P. Boden, A. Päpcke, S. Gómez, C. Förster, F. Kuczelinis, L. M. Carrella, L. A. Büldt, N. H. Bings, E. Rentschler, S. Lochbrunner, L. González, M. Gerhards, M. Seitz and K. Heinze, J. Am. Chem. Soc., 2020, 142, 7947–7955 CrossRef CAS PubMed
.
- M. Dorn, D. Hunger, C. Förster, R. Naumann, J. van Slageren and K. Heinze, Chem. – Eur. J., 2023, 29, e202202898 CrossRef CAS PubMed
.
- G. Wolfowicz, C. P. Anderson, B. Diler, O. G. Poluektov, F. J. Heremans and D. D. Awschalom, Sci. Adv., 2020, 6, eaaz1192 CrossRef CAS PubMed
.
- B. R. Ortiz, L. C. Gomes, J. R. Morey, M. Winiarski, M. Bordelon, J. S. Mangum, I. W. H. Oswald, J. A. Rodriguez-Rivera, J. R. Neilson, S. D. Wilson, E. Ertekin, T. M. McQueen and E. S. Toberer, Phys. Rev. Mater., 2019, 3, 094407 CrossRef CAS
.
- B. R. Ortiz, S. M. L. Teicher, Y. Hu, J. L. Zuo, P. M. Sarte, E. C. Schueller, A. M. M. Abeykoon, M. J. Krogstad, S. Rosenkranz, R. Osborn, R. Seshadri, L. Balents, J. He and S. D. Wilson, Phys. Rev. Lett., 2020, 125, 247002 CrossRef CAS PubMed
.
- Proposal for a REGULATION OF THE EUROPEAN PARLIAMENT AND OF THE COUNCIL establishing a framework for ensuring a secure and sustainable supply of critical raw materials and amending Regulations (EU) 168/2013, (EU) 2018/858, 2018/1724 and (EU) 2019/1020. COM/2023/160 final.
- EIT RawMaterials, ‘Win-Win for Europe: Mineral processing facility in Finland set to increase vanadium production for Europe’, 2022.
- M. Petranikova, A. H. Tkaczyk, A. Bartl, A. Amato, V. Lapkovskis and C. Tunsu, Waste Manage., 2020, 113, 521–544 CrossRef CAS PubMed
.
- A. Aluko and A. Knight, IEEE Access, 2023, 11, 13773–13793 Search PubMed
.
-
S. Carrara, S. Bobba, D. Blagoeva, P. Alves Dias, A. Cavalli, K. Georgitzikis, M. Grohol, A. Itul, T. Kuzov, C. Latunussa, L. Lyons, G. Malano, T. Maury, Á. Prior Arce, J. Somers, T. Telsnig, C. Veeh, D. Wittmer, C. Black, D. Pennington and M. Christou, Publications Office of the European Union, Luxembourg, 2023, JRC132889. DOI:10.2760/386650.
- T. L. Gerke, K. G. Scheckel and M. R. Schock, Environ. Sci. Technol., 2009, 43, 4412–4418 CrossRef CAS PubMed
.
- K. Y. Monakhov, Nat. Sci., 2024, e20230020 CrossRef
.
- D. Mannikko and S. Stoll, Energy Fuels, 2019, 33, 4237–4243 CrossRef CAS
.
- G. L. Pakhomov, A. I. Koptyaev, P. A. Yunin, N. V. Somov, A. S. Semeikin, E. D. Rychikhina and P. A. Stuzhin, ChemistrySelect, 2023, 8, e202303271 CrossRef CAS
.
- X. Zhao, Q. Shi, M. R. Gray and C. Xu, Sci. Rep., 2014, 4, 5373 CrossRef CAS PubMed
.
- Z. K. Myltykbaeva, A. V. Anisimov, A. B. Seisembekova and M. B. Smaiyl, Theor. Found. Chem. Eng., 2022, 56, 928–933 CrossRef
.
- K. Y. Monakhov, W. Bensch and P. Kögerler, Chem. Soc. Rev., 2015, 44, 8443–8483 RSC
.
- N. I. Gumerova and A. Rompel, Nat. Rev. Chem., 2018, 2, 0112 CrossRef CAS
.
- M. Aureliano, N. I. Gumerova, G. Sciortino, E. Garribba, A. Rompel and D. C. Crans, Coord. Chem. Rev., 2021, 447, 214143 CrossRef CAS
.
- Y. Zhang, T. Learmonth, S. Wang, A. Y. Matsuura, J. Downes, L. Plucinski, S. Bernardis, C. O'Donnell and K. E. Smith, J. Mater. Chem., 2007, 17, 1276–1283 RSC
.
- F. Pan, H. Tian, X. Qian, L. Huang, Y. Geng and D. Yan, Org. Electron., 2011, 12, 1358–1363 CrossRef CAS
.
- I. Pozo, Z. Huang, F. Lombardi, D. I. Alexandropoulos, F. Kong, M. Slota, I. Tkach, M. Bennati, J.-R. Deng, W. Stawski, P. N. Horton, S. J. Coles, W. K. Myers, L. Bogani and H. L. Anderson, Chemistry, 2024, 10, 299–316 CrossRef CAS
.
- K. Monakhov, Bunsenmagazin, 2024, 1, 32–35 Search PubMed
.
- X. Gao, C. M. M. Rosário and H. Hilgenkamp, AIP Adv., 2022, 12, 015218 CrossRef CAS
.
- S. K. Das, S. K. Nandi, C. V. Marquez, A. Rúa, M. Uenuma, E. Puyoo, S. K. Nath, D. Albertini, N. Baboux, T. Lu, Y. Liu, T. Haeger, R. Heiderhoff, T. Riedl, T. Ratcliff and R. G. Elliman, Adv. Mater., 2023, 35, 2208477 CrossRef CAS PubMed
.
- W. Yi, K. K. Tsang, S. K. Lam, X. Bai, J. A. Crowell and E. A. Flores, Nat. Commun., 2018, 9, 4661 CrossRef PubMed
.
- A. Sebastian, M. LeGallo, R. Khaddam-Aljameh and E. Eleftheriou, Nat. Nanotechnol., 2020, 15, 529–544 CrossRef CAS PubMed
.
- H. Bao, H. Zhou, J. Li, H. Pei, J. Tian, L. Yang, S. Ren, S. Tong, Y. Li, Y. He, J. Chen, Y. Cai, H. Wu, Q. Liu, Q. Wan and X. Miao, Front. Optoelectron., 2022, 15, 23 CrossRef PubMed
.
- M.-A. Stoeckel, M. Gobbi, T. Leydecker, Y. Wang, M. Eredia, S. Bonacchi, R. Verucchi, M. Timpel, M. V. Nardi, E. Orgiu and P. Samorì, ACS Nano, 2019, 13, 11613–11622 CrossRef CAS PubMed
.
- L. Daukiya, J. Seibel and S. De Feyter, Adv. Phys.: X, 2019, 4, 1625723 CAS
.
- Y. Zhao, M. Gobbi, L. E. Hueso and P. Samorì, Chem. Rev., 2022, 122, 50–131 CrossRef CAS PubMed
.
- S. Aftab, M. Z. Iqbal and M. W. Iqbal, Adv. Mater. Interfaces, 2022, 9, 2201219 CrossRef CAS
.
- J. Muñoz, Adv. Mater., 2023, 2305546 CrossRef PubMed
.
- J. P. Guerrero-Felipe, A. M. Valencia and C. Cocchi, J. Phys. Chem. C, 2023, 127, 23926–23934 CrossRef CAS
.
-
C. Bäumer and R. Dittmann, in Metal Oxides, Metal Oxide-Based Thin Film Structures ed. N. Pryds and V. Esposito, Elsevier, 2018, pp. 489–522 Search PubMed
.
- P. Hu, P. Hu, T. D. Vu, M. Li, S. Wang, Y. Ke, X. Zeng, L. Mai and Y. Long, Chem. Rev., 2023, 123, 4353–4415 CrossRef CAS PubMed
.
- L. Tesi, E. Lucaccini, I. Cimatti, M. Perfetti, M. Mannini, M. Atzori, E. Morra, M. Chiesa, A. Caneschi, L. Sorace and R. Sessoli, Chem. Sci., 2016, 7, 2074–2083 RSC
.
- L. Malavolti, M. Briganti, M. Hänze, G. Serrano, I. Cimatti, G. McMurtrie, E. Otero, P. Ohresser, F. Totti, M. Mannini, R. Sessoli and S. Loth, Nano Lett., 2018, 18, 7955–7961 CrossRef CAS PubMed
.
- E. Garlatti, A. Albino, S. Chicco, V. H. A. Nguyen, F. Santanni, L. Paolasini, C. Mazzoli, R. Caciuffo, F. Totti, P. Santini, R. Sessoli, A. Lunghi and S. Carretta, Nat. Commun., 2023, 14, 1653 CrossRef CAS PubMed
.
-
N. B. Mckeown, in Phthalocyanine Materials: Synthesis, Structure and Function, ed. B. Dunn, Cambridge University Press, Cambridge, UK, 1998 Search PubMed
.
- M. Atzori, E. Morra, L. Tesi, A. Albino, M. Chiesa, L. Sorace and R. Sessoli, J. Am. Chem. Soc., 2016, 138, 11234–11244 CrossRef CAS PubMed
.
- D. Gatteschi, B. S. Tsukerblat, A. L. Barra, L. C. Brunel, A. Müller and J. Döring, Inorg. Chem., 1993, 32, 2114–2117 CrossRef CAS
.
- A. Palii, B. Tsukerblat, J. M. Clemente-Juan and E. Coronado, J. Phys. Chem. C, 2016, 120, 16994–17005 CrossRef CAS
.
- B. Tsukerblat, A. Palii, J. M. Clemente-Juan and E. Coronado, Int. Rev. Phys. Chem., 2020, 39, 217–265 Search PubMed
.
- E. Vogelsberg, M. Moors, A. S. Sorokina, D. A. Ryndyk, S. Schmitz, J. S. Freitag, A. V. Subbotina, T. Heine, B. Abel and K. Y. Monakhov, Chem. Mater., 2023, 35, 5447–5457 CrossRef CAS
.
- I. Werner, J. Griebel, A. Masip-Sánchez, X. López, K. Załęski, P. Kozłowski, A. Kahnt, M. Boerner, Z. Warneke, J. Warneke and K. Y. Monakhov, Inorg. Chem., 2023, 62, 3761–3775 CrossRef CAS PubMed
.
- M. Moors, J. Warneke, X. López, C. de Graaf, B. Abel and K. Y. Monakhov, Acc. Chem. Res., 2021, 54, 3377–3389 CrossRef CAS PubMed
.
- S. Soni, I. Werner, M. Aidi, M. Moors, C. L. Mthembu, M. Zharnikov, R. W. A. Havenith, K. Y. Monakhov and R. C. Chiechi, ACS Appl. Nano Mater., 2023, 6, 22643–22650 CrossRef CAS
.
- O. Linnenberg, M. Moors, A. Notario-Estévez, X. López, C. de Graaf, S. Peter, C. Baeumer, R. Waser and K. Y. Monakhov, J. Am. Chem. Soc., 2018, 140, 16635–16640 CrossRef CAS PubMed
.
-
K. Y. Monakhov, M. Moors, E. Vogelsberg, J. Lorenz, J. Warneke and F. Yang, 2023 IEEE International Interconnect Technology Conference (IITC) and IEEE Materials for Advanced Metallization Conference (MAM)(IITC/MAM), Dresden, Germany, 2023, pp. 1–3.
- M. Moors, I. Werner, J. Bauer, J. Lorenz and K. Y. Monakhov, Nanoscale Horiz., 2024, 9, 233–237 RSC
.
- F. Yang, M. Moors, D. A. Hoang, S. Schmitz, M. Rohdenburg, H. Knorke, A. Charvat, X.-B. Wang, K. Y. Monakhov and J. Warneke, ACS Appl. Nano Mater., 2022, 5, 14216–14220 CrossRef CAS
.
- B. Salonikidou, A. Mehonic, Y. Takeda, S. Tokito, J. England and R. A. Sporea, Adv. Eng. Mater., 2022, 24, 2200439 CrossRef CAS
.
- R. Sessoli, Natl. Sci. Rev., 2021, 8, nwaa267 CrossRef PubMed
.
- S. K. Petrovskii, M. Moors, S. Schmitz, E. V. Grachova and K. Y. Monakhov, Chem. Commun., 2023, 59, 9517–9520 RSC
.
- D. Ielmini, Semicond. Sci. Technol., 2016, 31, 063002 CrossRef
.
- M. Le Gallo and A. Sebastian, J. Phys. D: Appl. Phys., 2020, 53, 213002 CrossRef CAS
.
- K. C. Kwon, J. H. Baek, K. Hong, S. Y. Kim and H. W. Jang, Nano-Micro Lett., 2022, 14, 58 CrossRef CAS PubMed
.
- S. Ling, C. Zhang, C. Ma, Y. Li and Q. Zhang, Adv. Funct. Mater., 2023, 33, 2208320 CrossRef CAS
.
- E. Coronado, Nat. Rev. Mater., 2020, 5, 87–104 CrossRef
.
- C. Huez, S. Renaudineau, F. Volatron, A. Proust and D. Vuillaume, Nanoscale, 2023, 15, 10634–10641 RSC
.
|
This journal is © The Royal Society of Chemistry 2024 |