DOI:
10.1039/D4MA00131A
(Review Article)
Mater. Adv., 2024,
5, 5365-5393
Self-healing, injectable chitosan-based hydrogels: structure, properties and biological applications
Received
11th February 2024
, Accepted 10th June 2024
First published on 12th June 2024
Abstract
Conventional biomaterials suffer from mechanical stresses and biochemical degradation, compromising performance and structural integrity. Hydrogels are three-dimensional networks that hold a huge quantity of water but can retain their internal structure due to chemical and physical crosslinking. Unlike self-healing injectable hydrogels, they lack the capacity to repair their inherent structure after damage due to the absence of reversible bonds, but these self-healing injectable hydrogels can withstand and reverse damage accumulation. The recoverability of self-healing injectable hydrogels stems from the presence of reversible chemical bonds within their structure. Schiff base linking involves reversible imine or hydrazone bond formation through reactions between aldehydes or ketones, commonly used in chitosan-based hydrogels. Rheological and morphological characterization help determine the precise structure, mechanical strength and bond types. Moreover, the capacity to hold water in hydrogels can closely mimic the extracellular matrix (ECM). Incorporating various polymers and crosslinkers enhances mechanical strength and biocompatibility. Their porous and hydrophilic nature allows for versatile applications, such as loading living cells, drugs, growth factors, and miRNA, promoting cell proliferation and adhesion. The injectability of these hydrogels facilitates precise administration through narrow syringes, enabling rapid local confinement and reducing off-target side effects. Through 3D bioprinting, the injectability can be proven and through experimental studies conducted in vitro using dissolution tests or in vivo on rodents, the sustained drug release capabilities of these hydrogels can be determined. Chitosan-based self-healing injectable hydrogels possess remarkable properties that find applications in tissue-engineered scaffolds, drug delivery, wound dressings, and cancer treatment.
1. Introduction
Conventional biomaterials like metal, ceramics, polymers and composites possessing higher mechanical strength experience constant mechanical stresses and undergo biochemical degradation within the biological environment. This can compromise their structural integrity and functional performance. Also, they lose the capacity to undergo structural restoration following damage. Hydrogels, being polymers and soft condensed matter, can be intentionally designed to mimic physiological conditions, but the absence of reversible bonds refrains them from recoverability.
Self-healing injectable hydrogels reveal the unique capacity to withstand and reverse the damage that accumulates over time and external factors. The bio-inspired self-healing process is familiar as many parts of our body self-heal on damage: wound healing,1 bone re-modelling,2 liver regeneration,3 neuronal regeneration,4 DNA repair.5,6 In self-healing injectable hydrogels, recoverability arises from the presence of reversible chemical bonds within the hydrogel's structure, allowing it to recover its structural integrity and mechanical properties upon deformation.
Polysaccharides, proteins, and synthetic polymers offer tunable properties to form hydrogels. Chitosan, a polysaccharide is a biopolymer derived from crustaceans, proven to be biocompatible, non-toxic, biodegradable and additionally show the self-healing property on modifying its chemical nature by introducing reversible bonds. Different chemical strategies can be equipped for reversible bonding; however, Schiff base linking is the most commonly used method in chitosan-based self-healing injectable hydrogels, a reaction between aldehydes or ketones to form reversible imine or hydrazone bonds. External conditions such as temperature,7,8 pH,9,10 and thixotropy can trigger the self-healing response and gelling strength in hydrogels.
By incorporating various polymers and corresponding crosslinkers, chitosan having free hydroxyl and amine groups can be engineered to closely resemble the physiological environment in addition to enhanced strength and biocompatibility. Loading living cells, drugs, pro-angiogenic cytokines, growth factors, and miRNA offers versatile applications for cell proliferation and cell adhesion because of its porous and hydrophilic nature. The injectability of these hydrogels can be determined by 3D bioprinting, which transforms the given input CAD file into a printed structure. The concept of additional manufacturing paves the way to higher and sustained drug release, and injectability reduces off-target side effects.11 The minimally invasive administration of self-healing injectable hydrogels through a narrow syringe allows for rapid local confinement of the hydrogel and any incorporated substances.
This review focuses on understanding, designing, and characterizing the inherent behavior of self-healing and injectability in chitosan hydrogels. Chitosan, being insoluble in aqueous phase at neutral conditions, requires structural modifications for its usage in further applications. In addition to structural modifications, derivatives of chitosan often enhance the crosslinking ability and bond reversibility with various other biopolymers. To determine the self-healing and injectability, understanding the structural modification and rheological characterization is significant. Self-healing injectable hydrogel can be locally administered and in desired shapes using 3D bio-printing of hydrogel. Other various experimental tests include characterizing its morphology, and biocompatibility. At a preliminary stage, in vitro tests are conducted to observe cell proliferation, cell adhesion in the self-healing hydrogel matrix using various cell lines in 3D bio-printed hydrogel too. Chitosan based self-healing hydrogels also have the potential to work as drug carriers. The presence of a free amine group imparts an inherent antibacterial property to chitosan.12 Its remarkable properties find application in tissue-engineered scaffolds,13 wound dressings, drug delivery carriers, and cancer treatment. In wound dressing, these hydrogels can effectively conform to irregularly shaped wounds,14 providing an ideal solution through a narrow syringe delivery. All the above-discussed applications will be explained in detail concerning hydrogels and, more prominently, chitosan-based self-healing injectable hydrogels (Fig. 1).
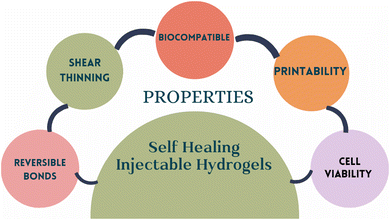 |
| Fig. 1 A mind map representing different properties of self-healing injectable hydrogels, which aid in the potential to be the best alternative for traditional biomaterials. The reversible bonds and shear-thinning nature are the essential properties determining self-healing injectable hydrogels’ nature. | |
2. Self-healing injectable hydrogels
The definition of self-healing has been evolving with technological advancement since the 1990s. Zeroth generation self-healing materials are defined as those that retard, but do not repair mechanical damage. First-generation self-healing materials are defined as those that irreversibly repair but do not restore the damaged matrix, while second-generation materials are based on reversible shape restoration.15 They can go through numerous cycles of stretching and breaking, but they ultimately mend by themselves. As a result of the hydrogel's inevitable breakdown,16 cell would diffuse into the surrounding tissues and eventually die in the severe ischemia environment. Damage developed during this can be repaired automatically thanks to the self-healing capability. Additionally, self-healing hydrogels having the potential to integrate as bulk gels at the target site can be administered without gel fragmentation.11,15,17 Sometimes, these self-healing biomaterials need an external trigger like pH,10,18,19 temperature,8,20,21 UV or chemical healing agents to self-heal. For such cases, chemical healing agents are generally filled in capsules or tubes and released upon breakage of the element.11 However, these self-healing injectable hydrogels lack mechanical strength and biocompatibility issues, which can be addressed by employing stronger interactions between biodegradable substances.
2.1 Design & synthesis
Understanding the design strategies for biomaterials is crucial because self-healing originates at the structural level.17 They are based upon a trade-off between physical and chemical bonding.
The primary design criteria being reversibility and shear thinning facilitate injectability and intrinsic self-healing.11 non-covalent interactions and dynamic covalent interactions are the different chemical bonding strategies. Non-covalent interactions are over a wide range in nature. Due to their adaptability, they can be used in an aqueous environment. Electrostatic interactions: internal attraction forces between cationic and anionic polymers, metal coordination:22via chelation, which involves several ligands sequestering metallic ions, hydrophobic interactions, and hydrogen bonding are the different strategies of non-covalent interactions. Dynamic covalent interactions are the chemical crosslinking of polymers by covalent bonds. This is also the traditional approach for hydrogel fabrication. Click chemistry reactions11,23,24 like Diels–Alder reactions, Schiff-base reactions, and Thiol-Disulfide exchange reactions can be used to design the self-healing injectable hydrogels (Table 1). Diels–Alder reactions involve the [4+2] cyclo-addition of compounds to form a thermally reversible six-membered cycle.25 These disulphide bonds are multi-responsive to pH, light and redox reactions.26 In Schiff-base linkages, the nucleophilic group attack of amines on the aldehydes and ketone groups creates reversible bonds (Fig. 2).
Table 1 Synthesis strategies of chitosan-based hydrogels
Polymers |
Crosslinking strategies |
Ligands or crosslinkers |
Biocompatibility tested against |
Applications |
Brief findings |
Ref. |
Abbreviations: DA-PEG – dibenzaldehyde-modified PEG2000; PF – Furan modified Pectin; maleimide-modified chitosan (CA); CMCS – carboxymethyl chitosan; Ox-HPC – oxidized hydroxypropyl cellulose; N,O-CMC – N,O-carboxymethyl chitosan and OCS – oxidized chondroitin sulfate; OHA – oxidized hyaluronic acid; HTCC N-(2-hydroxypropyl)-3-trimethylammonium chitosan chloride; HTCCMA – N-(2-hydroxypropyl)-3-trimethylammonium chitosan chloride methacrylate; LAP – lithium phenyl-2,4,6-trimethylbenzoylphosphinate; CA – L-arginine conjugated chitosan; CHO-PEG-CHO – benzaldehyde group functionalized poly ethylene glycol pDA-NPs – polydopamine nanoparticles; CA-pDA, as an angiogenic and antibacterial dressing for wound repair. GAMA – gum arabic with multialdehyde groups; SCS – succinic anhydride-modified chitosan; N-Cur – nanocurcumin; OHAH – oxidized hydroxyapatite/alginate hybrids. |
Chitosan–collagen |
Schiff base linkages (imine) |
DA-PEG |
Sprague Dawley (SD) mice model |
Strain sensitive epidermal sensor, wound healing |
Hydrogel has excellent hemostatic ability, multifunctional based on naturally occurring biomass |
19
|
Chitosan and pectin |
Diels–Alder Reaction |
Dil. Aq HCl pH = 5 |
Fibroblast L929 cells |
Drug delivery |
Swelling effects were observed in Water and PBS, pH responsive Fluorouracil release |
27
|
CMCS-OxHPC |
Schiff base linkages |
Ketone bonds are introduced |
— |
Targeted Drug Release |
Selective oxidation of secondary OH groups that terminate substituents of HPC to ketones |
28
|
N,O-CMC and OCS |
Schiff base linkages |
— |
NIH/3T3, HUVECs cells |
Wound Dressing |
The degree of crosslinking, gelation periods, and rheological characteristics of the hydrogel were influenced by varying the mass ratio of N,O-CMC to OCS. Effective reduction in blood loss after surgery |
29
|
Chitosan–dextran |
Schiff base linkages |
Gallic acid |
L929 mouse fibroblast cells, ICR mice model |
Combined radiation & burn injury, diabetic foot ulcer |
Multiple ROS scavenging ability, humid and antibacterial environment, reduction in inflammatory response on the topical wound site. |
30
|
OHA-HTCCMA |
Dual crosslinking: Schiff base, Photo irradiation |
LAP photo-initiator |
Rabbit articular chondrocytes (CP002), primary bone mesenchymal stem cells (BMSC), New Zealand White rabbits |
Osteoarthritis, cartilage regeneration |
The hydrogel promoted cartilage regeneration and prevented cartilage lesions in in vivo systems |
31
|
CA-pDA |
Schiff base linkages |
L-Arg, pDA-NPs, CHO-PEG-CHO |
HSFs, HUVECs, Mouse RAW 264.7 macrophages SD rat model |
Large skin wound repair |
Hydrogel can effectively heal large skin wounds, increasing angiogenesis and minimising scarring. |
32
|
SCS |
Schiff base linkages |
GAMA |
Breast cancer cell line (MCF-7), Human embryonic kidney cell line (HEK-293) |
Anticancer drug delivery |
The hydrogel showed higher pH responsive drug (N-Cur) release at acidic pH. Drug loaded hydrogels inhibited the growth of MCF-7 |
33
|
OHAH-CMCS |
Schiff base linkages |
Hydroxy-apatite nanoparticles |
L929 cells |
Bone repair and regeneration |
HA particles showed strong interface compatibility with the organic matrices, making it a viable material for the application of bone regeneration and repair. |
34
|
Chitosan-cellulose |
Schiff base linkages |
Cellulose nanofibres |
Neural stem cells |
Neural regeneration |
Hydrogel showed excellent space remodeling effect and nutrient transportation |
35
|
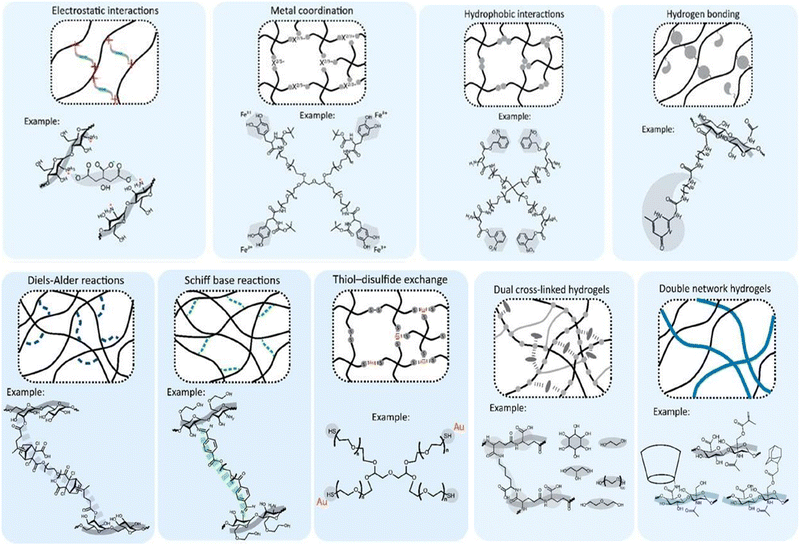 |
| Fig. 2 The chemical strategies for designing self-healing injectable hydrogels, including non-covalent and dynamic covalent bonds. Figure reproduced from Bertsch et al. 202211 open access article under Creative Commons Attribution License with CC-BY-NC-ND. | |
To address the disadvantages of covalent and non-covalent interactions, a new class of introductions were introduced, which includes both covalent and non-covalent interactions. Dual cross-linked hydrogels and double network hydrogels come under this class. Non-covalent interactions are weak and sensitive to specific external triggers like pH or ionic strength. In combination, excellent results were observed where the presence of imine bonds in Schiff base reactions combined with other non-covalent interactions.
The hydrogel network's physical configuration and spatial topology substantially influences the resulting hydrogel system's external and internal characteristics. Different physical strategies to design a hydrogel include monolithic hydrogels, Fibrous hydrogels, colloidal and granular hydrogels, particle cross-linked hydrogels, and particle-filled hydrogels. As the name suggests, mono means one, i.e., hydrogel is made using one polymer. The nature of fibrous hydrogels is to impact mechanical and biological properties. Ruquan Zhang36et al. said Xanthan Gum is a weak hydrogel because of the presence of hydroxyl and carboxyl groups. But on fabricating the same with a fibrous protein like silk fibroin,36 krill protein composite fiber37 the mechanical strength increased substantially. In a similar fashion, to address the issue of biocompatibility, biopolymers like silk fibroin, chitosan, dextran, alginate, cellulose, starch, pullulan, and hyaluronic acid can be used to make self-healing injectable hydrogels improving both biocompatibility and mechanical properties by introducing fibrous, colloidal/granular particulates (Fig. 3).11,38
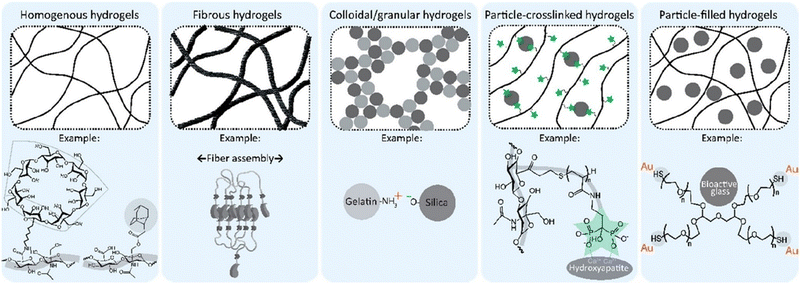 |
| Fig. 3 The overview of physical design strategies of self-healing injectable hydrogels. Monolithic/homogenous hydrogels: impact the network topology of resulting hydrogels; fibrous hydrogels: mechanical stability, colloidal hydrogels: cell ingrowth; particle-crosslinked: mechanical properties and self-healing, particle-filled hydrogels: particles act as fillers to reinforce hydrogels reproduced from Bertsch et al. 2022 ref. 11 open access article under Creative Commons Attribution License with CC-BY-NC-ND. | |
2.1.1 Structure and design strategy of chitosan.
Chitosan is a natural cationic polymer derived from the N-deacetylation of chitin. Chitin can be obtained from fungal mycelia, crab shells, or shrimp shells.39 The other method of production of chitosan is by fermentation processes, which includes alkali treatment to yield chitosan-glucan complexes. Chitosan is composed of N-acetyl glucosamine and glucosamine units. Unlike other polysaccharides, chitosan is highly basic and has been widely used in pharmaceutical and surgical procedures for ophthalmology, wound dressing, tissue regeneration, cancer-treatment, etc. because of its biocompatibility, biodegradability and antimicrobial property. Other than biomedical applications, it can be used in ref. 39. Photography and packaging40 (films), cosmetics,41,42 artificial skin,43 food & nutrition,44,45 application in solid-state batteries,46–48 and to impart wet strength to paper.49
The nucleophilic groups in chitosan facilitate Schiff base reactions, forming reversible bonds. In these reactions, the free amine group in chitosan reacts with aldehyde or ketone groups, creating dynamic and covalent imine or hydrazone bonds. For the progress of the Schiff base reaction, a crosslinker with aldehyde groups is required. Xu Y. et al., suggested four different strategies to generate polysaccharides with aldehyde groups.50 The first strategy is the production of polysaccharides with inbuilt aldehyde through the oxidation of hydroxymethyl groups leading to glycol cleavage with the help of sodium periodate to form aldehyde products. This method of periodate-mediated formation of aldehyde group was utilized in polysaccharides like gum Arabic,51,52 alginate,53–55 chondroitin sulfate,29,56–58 dextran,30,59–61 hyaluronic acid,31,62–65 pectin.66–69 In the second strategy, the polysaccharide is generated so that aldehyde groups are present on the ends of the linear polymers as the crosslinking agent.50,70 Small dialdehyde molecules serve as the best crosslinking agents for this strategy, but their toxicity limits its application.20,70 One of the few biocompatible, water-soluble, and non-toxic polymers is polyethylene glycol or PEG. Hence, dialdehyde derivatives of PEG have been created to address this toxicity issue. Di-benzaldehyde functionalized polyethylene glycol is the widely used crosslinker to form aldehyde groups at the ends of linear polymers.32,70–72 The above crosslinker is formed by the esterification of hydroxyl-terminated poly ethyl glycol with 4-formyl benzoic acid (FBA).33,73,74 The other method strategizes crosslinking with star polymers consisting of an aldehyde group at the end of each arm of the polymer, forming a star-like PEG.75 Multi-aldehyde PEG, multi-armed PEG crosslinkers,76 and star-shaped eight-armed PEG crosslinkers77 create possible ways for Schiff base reactions to increase the crosslinking sites, thereby increasing the gelation time, biodegradability, and self-healing ability. This strategy can be regarded as the appropriate choice to play with as it can create strong self-healing hydrogels.76–78 reveal remarkable self-healing abilities visualized macroscopically and rheologically on the addition of polyethylene glycol(PEG).
The third method utilizes linear polymers as crosslinking agents with many side chains containing aldehyde groups. One main advantage over the previous strategies is that the variation in the concentration of the crosslinker increases both mechanical strength and rate of biodegradation. Chitosan can be blended with other polysaccharides of the same or different family depending on the degree of miscibility and number of polymer chains as another strategy. Alginate,34,55 cellulose,35,38,67,79,80 and gelatin,55 with the help of chemical oxidation, convert the hydroxy groups into aldehyde bonds. This combination of blending of various polysaccharides with chitosan enhances the functional, mechanical, and self-healing ability of chitosan (Fig. 4).
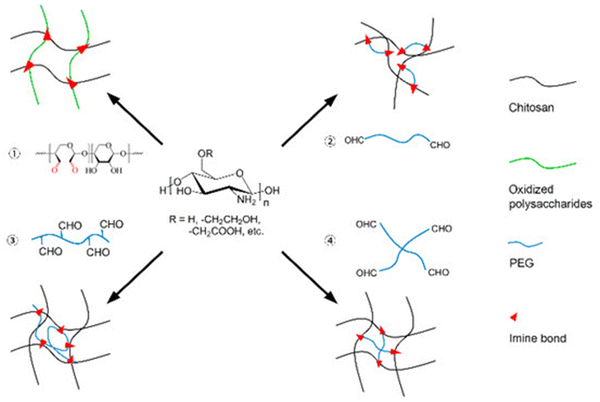 |
| Fig. 4 Representation of four different strategies to introduce aldehyde functional groups into chitosan, enabling the formation of Schiff base linkages. PEG abbreviates to poly-ethylene-glycol Reproduced from ref. 50 (Xu et al., 2018) For articles published under an open-access Creative Common CC BY license. | |
Due to its strong intermolecular hydrogen bonding, chitosan is insoluble and unstable in neutral and high pH solutions, necessitating the use of acids to prepare chitosan solutions. Typically, either citric acid or acetic acid is employed for this purpose; however, it is necessary to perform continuous washing to eliminate any excess acid. The presence of free amine on C2 and free hydroxyl groups on C681 in chitosan is exploited to synthesize its derivatives by methods like methylation, carboxylation, quaternization, sulphation, and phosphorylation,82 making the process much simpler than using acids. The hydroxyl group present on C3 in Fig. 5 has high stearic hindrance, so it cannot react,83 and hence only functional groups of C2 and C6 are readily reactive A clear depiction of the chitosan structure is given in the figure below. The basic idea behind synthesizing its derivatives is to weaken the intermolecular hydrogen bond, making it water-soluble. These derivatives are not only soluble in water but over the whole pH range. They could retain the biological properties of chitosan by improving the chemical and physical properties for various biomedical applications (Fig. 5).
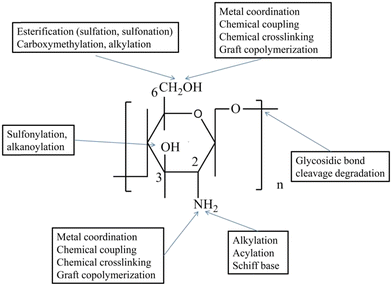 |
| Fig. 5 The processes to synthesize derivatives of chitosan. The hydroxyl groups present on C6 and C3 Reproduced from ref. 83 Wenqian Wang et al. 2020 open-access articles distributed under the terms and conditions of the Creative Commons Attribution (CC BY) license (http://creativecommons.org/licenses/by/4.0/). | |
2.1.2 Chitosan derivatives.
Chitosan oligosaccharides (COS).
Produced via enzymatic or acid hydrolysis of chitosan, they represent truncated chains of the original polysaccharide. This diminutive structure has low molecular weight and shorter chain lengths, readily water soluble, thereby opening vistas to explore its health-promoting attributes, encompassing antioxidant, antitumor, and antimicrobial properties with biodegradation in kidney tissues, plasma, hepatic cells, and urine by lysosomes.84 Its higher water solubility allows for easy absorption of COS in intestinal epithelia thereby blood hence permittable to whole body. COS is proven to show excellent results in wound healing in moist environment due to water solubility.85 The presence of many free amine groups makes it compatible for further structural modifications with both biopolymers and synthetic polymers. Although COS has potential therapeutic applications for various diseases, it is proven that at higher concentrations (70 μg mL−1), COS causes cell death in human lymphocytes. Inadequate animal studies cause a lack of safety and impurity profiles data of COS. It is imperative to recommend thorough safety and chemical investigation when COS is exposed to chemical, enzymatic, or thermal exposure.86–88
Chitosan hydrochloride.
Through a chemical reaction involving chitosan and hydrochloric acid, the formation of chitosan hydrochloride transpires. The ensuing product chitosan hydrochloride, a chitosan derivative salt, evinces heightened water solubility compared to native chitosan, rendering it amenable to application within diverse biomedical contexts.81 Chitosan hydrochloride has mucoadhesive properties, meaning it can adhere to mucosal surfaces like gastrointestinal (GI) tract and respiratory tract where important functions like absorption and excretion occur. Mucoadhesive property is advantageous in drug delivery or in systems requiring prolonged contact with biological tissues.89 Permeability of chitosan hydrochloride is much higher in intestinal epithelial cells when compared to chitosan.90 In addition to biomedical applications, chitosan hydrochloride can be equipped in applications of air filtration membranes,91 coating,92,93 baking,94,95 plant immunity inducer.96,97 This derivative of chitosan is also used as a hydrogel coating in wound dressings exhibited efficient antimicrobial properties.98 At the same concentration levels chitosan hydrochloride has poor antioxidant activity when compared to Chitosan oligosaccharides. Also, its antimicrobial activity varied with the type of target microorganism which was proven by ref. 99.
Carboxymethyl chitosan.
Leveraging the introduction of carboxymethyl groups onto chitosan molecules, carboxymethyl chitosan emerges as a consequence. The replacement of free hydroxyl and amino groups with carboxyl functional groups confers augmented water solubility to chitosan, thus engendering its appropriateness in a plethora of therapeutic and biomedical pursuits, including drug delivery systems and wound healing therapies.29,53,71,74 In addition to Schiff base bonding, the presence of abundant free carboxyl and amino group can confer to form coordination bonds with metal ions like Fe(3+),100,101 Al(3+),101,102 Ag(+).103,104 Carboxymethyl Chitosan has improved antibacterial and antioxidant properties due to the intermolecular non-covalent interactions. It shows antimicrobial activity against E.coli, s.aureus, Candida tropicalis, candida parapsilosis, candida krusei, candida glabrata, B.subtillis.105 The higher number of chelating groups improves the metal ion sorption properties of chitosan. Its moisture retention properties is advantageous for better wound healing, cell adhesion and cell proliferation.
Quaternized chitosan.
It is brought to fruition by grafting quaternary ammonium groups onto the chitosan backbone. Different ways of grafting quaternary ammonium groups include Schiff base reactions,106 addition of glycidyl trimethylammonium chloride (GTMAC)107,108 in hydrophilic chitosan, etherification in alkaline condition, direct dissolution of chitosan in alkaline solvents like LiOH/KOH/urea-aqueous condition at low temperatures.109 Although studies reveal that grafting quaternary ammonium group onto chitosan is tedious and time taking109,110 its chemical modification imparts a heightened propensity for water solubility and accentuates the antimicrobial properties of chitosan. Consequently, quaternized chitosan attains utility in an array of applications, such as antimicrobial agents, drug delivery vehicles, and wound dressings.59,66 Similar to chitosan hydrochloride, quaternized chitosan shows enhanced mucoadhesive properties due to increased solubility and cationic character therefore, it can strongly interact with anionic groups of mucin, thereby binding with the epithelial surfaces111N,N,N trimethyl chitosan (TMC) is the simplest form of quaternized chitosan. Increasing the degree of quaternized groups increases the antioxidant properties.112 In fact, increasing the concentration of Quaternized chitosan tuned and improved the mechanical properties of self-healing injectable hydrogels.107,113,114 Quaternary chitosan revealed applications as a strain sensor effectively adhering to different substrates and contributed efficiently towards electrical conductivity and electrical sensitivity when ions like Fe3+ and Cl− are added. Hence showing applications in human health and motion detection110
Carbamoylated chitosan.
Carbamoyl group (CONH2) is induced onto the chitosan molecule. Various reactions equip carbamoylating agents like isocyanates or carbamoyl chlorides. In addition to enhancing the water solubility and mechanical properties, carbamoylated chitosan serves as the best carrier for drug delivery and cell growth applications due to its non-cytotoxicity115,116 Studies are needed to determine the self-healing nature of this biocompatible injectable chitosan hydrogel. Its applications involve in tissue engineering and 3D printing.
3. Characterization and properties of chitosan-based hydrogels
3.1 Rheological properties
3.1.1 Recoverability.
The synonym of self-healing is regaining its original structure from the damage caused by mechanical and biological stresses. During rheological measurements, various properties like Shear stress, extent of deformation, temperature, and the normal force exerted by the sample influence the viscoelastic properties. The presence of reversible bonds in modified chitosan hydrogel induces a self-healing ability. Recoverability can be determined in two ways. The first one includes cutting the hydrogel into two separate discs (as in Fig. 6(A) and (G)). After a while, these cut pieces join back into their original shape without any visual cracks.117,118 For a much clearer depiction, often researchers dye each part of the hydrogel into a different color using rhodamine52,119 and sometimes methylene blue33,120–122 dyes in general. The second method of determining recoverability involves step-strain measurements using oscillatory or rotational measurements in a rheometer. During the measurement process, the self-healing injectable hydrogel undergoes simultaneous deformation and healing while varying the shear or strain rates applied to it in cycles. The extremities of the extent of deformation are defined by using the strain sweep measurements in a rheometer, which measures the point of yield stress, where the storage modulus (G′) and loss modulus (G′′) meet (precisely, a point where their tangents meet to avoid over-estimation) at a constant frequency of 1 Hz (angular frequency of 10 rad s−1)123,124 or, 6.28 rad s−1 or, 0.1 Hz,125 a point where the hydrogel breaks and from there on the hydrogel no longer can be retrieved back in any means to its original gel state but remains in liquid phase. The step-strain test, also known as alternate-strain or 3iTT (3 interval-time-thixotropy test), is time-dependent and controlled at varied shears beginning with a lower rate. Fig. 6(D) shows the rheological measurement in 3 cycles with varying at 1% strain rate and 500% strain rate.
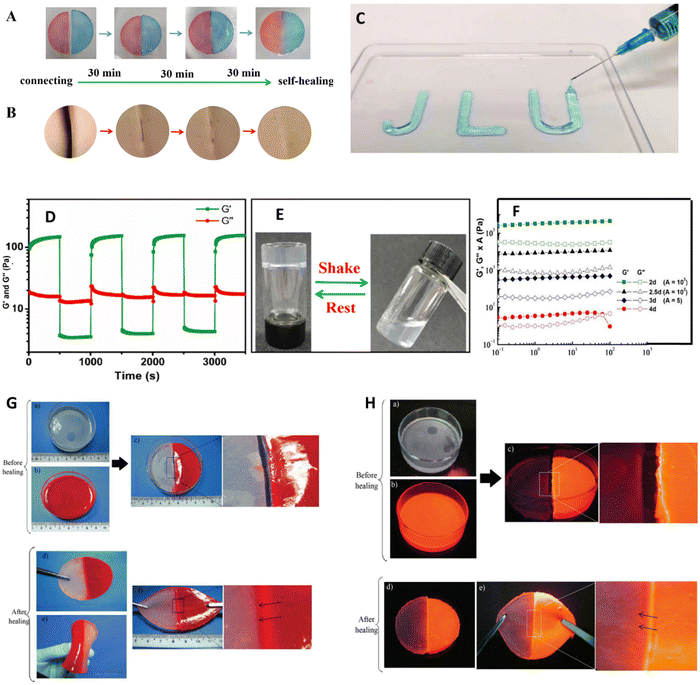 |
| Fig. 6 The rheological properties (A) of the two halves of the gel loaded with methyl orange and methylene blue to determine the self-healing properties after 1.5 h contact time. (B) Microscopic image of (A) revealing its structural recoverability. (C) Injectability of the hydrogel. (D) 3iTT test was performed at 500% and 1% strain rates. (E) inverted test tube test to observe the gelation time physically (F) depicts the curve obtained from the frequency sweep of chitosan and pyridoxal 5-phosphate (P5P) hydrogel loaded with vitamin B6 on diluting at different dilutions. The order of water content is as follows 2d < 2,5d < 3d < 4d; (G) and (H) depicts the visuals of bending and tensile strength of the recovered sample after healing with and without UV. The above images are reproduced from Copyright @ 2023 et al., Yongyan Yang,126 Anda Mihaela Craciun et al. (2022)127 open access article distributed under the terms and conditions of the Creative Commons Attribution (CC BY) license (https://creativecommons.org/licenses/by/4.0/). and Sirajuddin et al. (2014)119 licensed under creative commons (CC BY-NC-ND 3.0). | |
3.1.2 Injectability.
The second word in the title chitosan based self-healing injectable hydrogels, injectability, is an important criterion to be considered. For any hydrogel to be injectable, it has to be a shear-thinning fluid. According to the power law, all thixotropic fluids are non-Newtonian fluids whose flow behaviour index (n) <1 and shear decreases with time. These fluids reveal injectable properties. The basic idea behind self-healing injectable hydrogels is that fluids resist the shear on extrusion and solidify to gels after extrusion. Flow curve measurements aid in determining the viscosity behavior with varying shear rate. A gradual decrease in viscosity on increasing the shear rate depicts a shear thinning fluid i.e. its injectability. A visual test can also be performed by loading the liquid hydrogel into a syringe. Hence injectability and self-healing analysis are two main rheological tests for self-healing injectable hydrogels. Major applications of injectable hydrogels include 3D printing of hydrogels for a minimally invasive local administration.128
3.1.3 Mechanical strength.
Although hydrogels have a huge ability to mimic the extracellular matrix, their mechanical strength is a hindrance. Rheological Measurements, tensile strength, and compressive strength measurements aid in determining their mechanical strength. Frequency sweeps in a rheometer apply an amplitude oscillatory deformation in the linear viscoelastic region over a range of frequencies. This test allows us to determine the equilibrium gel strength, more specifically the storage and loss modulus. In Fig. 6(F), Anda Mihaela Craciun et al. (2022) tested how the G′ and G′′ varied on several dilutions. The modulus values decreased, and the gelation time increased from instantaneous to 30 min on increasing the water content.119,127 The healed hydrogels are also proven strong enough, similar to the undeformed hydrogels on applying tensile and bending forces, according to Fig. 6(G) and (H).
Rheological measurements determine the flow behavior and mechanical strength at oscillatory and rotational stresses. To determine its mechanical strength under tensile and compressive stresses a UTM can be equipped with sample dimensions according to the ASTM standards.129
3.1.4 Pore size & morphology.
Porosity influences the performance and efficacy of chitosan based self-healing injectable hydrogels. It refers to the presence of void spaces or pores within the material, the larger the pore size, the higher the porosity. In self-healing injectable hydrogels, porosity facilitates the swelling, infiltration of cells, and the exchange of nutrients, oxygen, and waste products.130,131 Increased pore size enhances external pH-stimulated self-healing behavior in chitosan hydrogels.78,127 The channels generated in the hydrogel allow host cells migration and proliferation into the damaged tissue132 and eventually swap out the malfunctioned organs. Cell adhesion and cell proliferation rate can be improved.133 High porosity provides a large surface area for drug loading, and the interconnected pores enable the controlled release of therapeutic agents. A suitable porosity influences the overall mechanical strength, elasticity, and stability of the hydrogels.79 Morphology refers to the structure, shape, and arrangement of the hydrogel components. The morphology of self-healing injectable hydrogels influences the structural stability of hydrogel and prevents premature degradation or disintegration of the hydrogel upon injection or during the healing process; it also impacts its interaction with surrounding tissues or substrates. By tailoring the morphology, it is possible to optimize adhesion, integration, and interfacial properties, allowing for better tissue integration and enhanced performance of the hydrogel. Morphology and porosity characterization are done using a scanning electron microscope. The pore diameter of hydrogels can be measured using software like ImageJ. The samples of self-healing injectable hydrogels are freeze-dried and sputter coated with gold126,134 or platinum59 or chromium. In most cases, the pore size decreased with the increase in concentration of the substrate. At the same concentration, the pore size increased by increasing the degree of substitution (DS) (Fig. 7G–J).135 Additionally, Water content present in the chitosan matrix defines and varies the pore size effectively.127
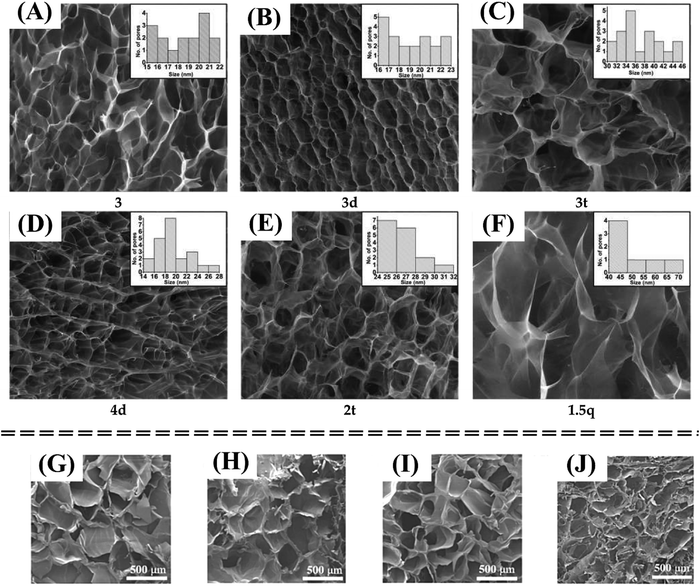 |
| Fig. 7 The porosity is increased with increasing the water quantity in the hydrogel composition from 97.88% to 99.30% reported by Craciun et al. (2022) (A)–(F).127 The variation of pore size is increased with the degree of substitution (DS) reported by Fengjiao Zhang et al. (2023)136 (G)–(J). Figures are reproduced from open access articles. | |
3.1.5 Swelling property.
Freeze-dried samples are weighed and soaked in PBS or DI water inside a vial. After a certain period, the self-healing injectable hydrogel attains an equilibrium that depicts the maximum absorption capacity of the hydrogel. The excess PBS137 or DI water59 is removed with a pipette137 or filter paper,59 and the swollen sample is weighed again. This swelling property is directly related to the porosity discussed above. Higher void space creates pockets for the absorption of PBS or DI Water. The equilibrium swelling ratio can be calculated by the ratio of the weight of media present in the sample to the weight of the dry sample.137 Swelling can support cell proliferation, influences, encapsulation and stiffness. Also, it should be noted that the aqueous media can be changed based on the application of the hydrogel.74 The degree of hydrogel swelling affects the drug release behaviour by regulating the pace at which the penetrant diffuses into the hydrogel matrix as wells as the drug's dissolution and diffusion throughout the swollen hydrogel matrix. Higher polymer concentrations can therefore produce hydrogels with longer-lasting medication release.38
Ws = weight of swollen sample Wd = Weight of dried sample.
3.1.6 Water retention properties.
Naturally formed hydrogels have much higher water absorption and retention properties when compared to synthetic hydrogels.126 This moisture retention creates an environment conducive to the self-healing process, facilitating the reformation of bonds and structural integrity upon damage. Adequate moisture content helps maintain the structural integrity of the hydrogel matrix and increases the longevity during application. Optimum moisture levels within the hydrogel ensures a suitable environment for cellular activities, wound dressing,129 flame retardants tissue regeneration. Drug delivery systems utilizing chitosan-based hydrogels moisture retention influences the dissolution, rate and pattern of drug release. Proper moisture levels can help control the diffusion or release of therapeutic agents, ensuring optimal dosage and therapeutic efficacy. Pore size, swelling ratio and crosslinking density influence the rate of drug release. Studies show a sustained drug release for higher crosslinking densities as the pore size is decreased and thereby the water absorption and retention.38 Moisture content also affects the long-term stability of hydrogels. Optimizing moisture retention mechanisms, such as cross-linking density or incorporating moisture-retaining additives like glycerol, can enhance stability. Water retention ratio is the percentage of water present in the hydrogel after a certain period of immersion per initial water content in the hydrogel.138 Mathematically, eqn (1) depicts the formula for quantification, Wt denotes the weight of the hydrogel after swelling or deswelling, Wd is the weight of the dry hydrogel and Wi denotes the weight of the initial hydrogel. | 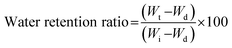 | (1) |
Chitosan owing to its intricate polymeric network has the potential to retain both bound and unbound water molecules. Characterizations like DSC and Raman spectroscopy have been equipped by Mohammad H. Mahaninia et al. 2023 to study the role of water molecules present inside the chitosan polymeric network.139 Jiawei Lu et al. 2023 studied the construction of a bilayer chitosan hydrogel possessing a lasting 7 day water retention rate of 64.91%.129
3.2 Printing
Additive manufacturing (AM), also known as 3D printing (3DP), involves the sequential addition or deposition of materials, either a single material or multiple materials, to construct a three-dimensional (3D) object layer by layer. Injectability, in the context of 3D printing, is a synonymous term for printability, encompassing several crucial aspects such as extrudability, shape fidelity, and filament classification. For 3D printing to be successful with hydrogels, it necessitates fulfilling these aforementioned factors.11 Extrudability delineates the hydrogel's flowability during the printing process. At the same time, filament classification elucidates its shear-thinning property, wherein it experiences a phase transition from a liquid state to a gel-like state. Once the hydrogel attains the desired gel structure, it must retain this configuration to prevent filament fusion, denoted as shape fidelity. Shear-thinning hydrogels exhibit rapid gelation properties, whereas stimulus-responsive hydrogels exhibit slower gelation speeds.140 This extreme gelation kinetics can potentially lead to either clogging of the printing nozzle or the dispersion of printed structures. The former scenario raises concerns about extrudability, whereas the latter compromises the precision of shape fidelity. Thanks to the ingenious implementation of dynamic reversible crosslinking, hydrogels can navigate these challenges. This mechanism enables the hydrogel to flow within the printing extruder under the application of pressure, allowing for smooth extrudability. Subsequently, once the printing process is complete, the hydrogel recovers its structure, ensuring the desired shape fidelity of the printed hydrogel.
3D bioprinting has applications in
(a) Tissue engineering and regenerative medicine in bone, liver, neural, heart valve, cardiac, lung, cartilage, pancreas, and skin
(b) Transplantation and clinics in bone, cartilage, and skin
(c) Pharmaceutics and drug testing
(d) Cancer research.
3D bioprinting is capable of fabricating a diverse range of biomaterials with or without cellular components, utilizing precise and controlled spatial arrangements, ultimately leading to the formation of targeted tissues or organs.141 Three-dimensional printing, also referred to as rapid prototyping (RP), employs computer-generated data such as computer-assisted design (CAD), which can be developed using magnetic resonance imaging (MRI) or computer tomography (CT) and transforms them into molded 3D objects. This translation requires a 3D bioprinter which can run by different mechanisms142 like extrusion-based bioprinting, stereolithography (SLA)-based bioprinting, inkjet bioprinting, and laser-assisting bioprinting. Bioink, a substrate that promotes cell proliferation and cell adhesion, is extruded intricately from the nozzle of the syringe in a pressure-controlled manner onto the printer bed.143 A 3D bio-printed tissue or organ can either undergo incubation in a bioreactor in a laboratory setting in vitro to mature before surgical implantation or can be printed in situ using the animal body itself as a bioreactor.144 But the pressure applied may affect the cell survival rate. Hence as a safer side, cells are seeded after the printing (Fig. 8).
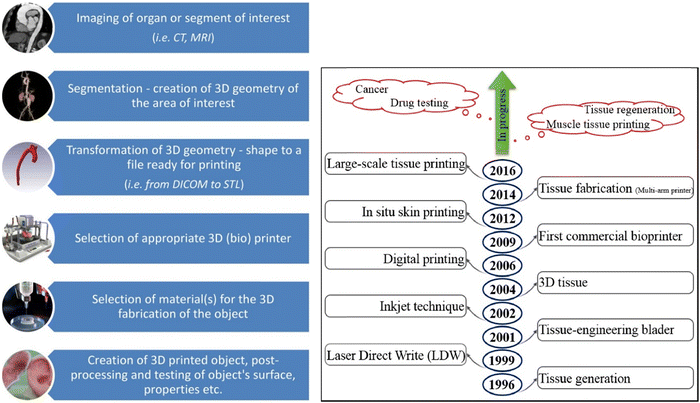 |
| Fig. 8 Schematic flow chart of Organ 3D printing (Lazaridou et al. 2022)145 (A), and the progress in 3D printing concerning various applications (Vanaei et al. 2021)142 (B). Open access Creative Common CC BY license (CC BY-NC-ND 4.0). | |
A quick crosslinking approach is crucial to maintaining the structural fidelity of the printed self-healing injectable hydrogel. Crosslinking methods like thermal crosslinking, pH-induced gelation, photo-responsive, and ionic gelation.
3.2.1 Thermal crosslinking.
Utilizes temperature changes to induce gelation and create a stable network in chitosan-based hydrogels. This can be achieved by incorporating thermosensitive polymers or additives that undergo a sol–gel transition at specific temperatures.146,147
3.2.2 pH-induced gelation.
Takes advantage of the pH sensitivity of chitosan to form a hydrogel structure. By adjusting the pH of the chitosan solution, the chitosan molecules undergo conformational changes and interact to form physical crosslinks.148
3.2.3 Photo-responsive crosslinking.
Involves incorporating light-responsive moieties or photo-initiators into the chitosan-based ink. Upon exposure to specific wavelengths of light,149–151 these moieties or initiators initiate crosslinking reactions, leading to gelation.
3.2.4 Ionic gelation.
Relies on the interaction between chitosan and multivalent ions to form physical crosslinks. By incorporating divalent or trivalent ions, such as calcium or zinc ions, into the chitosan solution, gelation occurs through the ionic interactions between chitosan chains and the ions.148,152 regarded tripolyphosphate and153 glutaraldehyde as the best crosslinkers, which impart high mechanical strength and structural integrity. Their combination could reveal better results but showed poor cell viability.
Chitosan hydrogels have emerged as promising biomaterials in 3D bioprinting due to their biocompatibility, biodegradability, non-immunogenicity, low cost and ability to mimic the extracellular matrix.154 By incorporating bioactive molecules and cells into chitosan-based bioinks, researchers can precisely fabricate complex 3D structures that mimic native tissues, holding significant potential in regenerative medicine for fabricating constructs such as cartilage, skin, and blood vessels.155 Moreover, the tunable mechanical properties of chitosan hydrogels allow for customization according to specific tissue requirements, further enhancing their utility in 3D bioprinting for creating functional tissue substitutes.
Intini C. et al., designed and fabricated 3D printed chitosan-based scaffolds for the treatment of wounds in diabetic rat model.156 After 20 and 35 days of incubation, 3D cell cultures displayed significant qualitative and quantitative in vitro cell growth, verified by neutral red staining, MTT assays, and scanning electron microphotographs. Combining Nhdf and HaCaT cells and filling scaffold holes after 35 days resulted in optimal cell proliferation on 3D scaffolds, forming an early skin-like layer composed of keratinocyte and fibroblast cells. In vivo studies done on streptozotocin-treated diabetic rats also showed enhanced tissue repair quality using 3D printed scaffolds compared to commercial patches and spontaneous healing. In another study conducted by Maturavongsadit P. et al., the use of chitosan and nanocellulose as bioink for 3D printing applications in osteogenic cell differentiation was demonstrated.157 Glycerophosphate, hydroxyethyl cellulose, thermogelling chitosan, and cellulose nanocrystals (CNCs) constituted the bioinks. Impact of CNCs and pre-osteoblast cells (MC3T3-E1) on scaffold mechanics, bioink printability, and rheology was assessed. CNCs and cells (5 million cells per mL) significantly enhanced bioink viscosity and chitosan scaffold mechanical properties post-production. Cell viability remained uncompromised, with bioinks printable within an optimal pressure range (12–20 kPa). In chitosan scaffolds, the inclusion of CNCs enhanced the osteogenesis of MC3T3-E1 cells. This CNCs-incorporated chitosan hydrogel represents a versatile bioink with appeal for 3D bioprinting, facilitating the production of scaffolds for bone tissue engineering and other medical applications.
Moreover, bioinks integrated with cells were successfully bioprinted, demonstrating better cell viability. Hafezi F. et al., developed chitosan based bioink laden with keratinocytes and human dermal fibroblasts cells.158 MTT assay revealed that 93% of the cells in the printed construct were alive after crosslinking, processing, and seven days of exposure to physiological conditions. Li P., and team also developed synovial mesenchymal stem cells (SMSCs) contained chitosan and PCL based hydrogels for the cartilage regeneration.159 This 3D printed chitosan/PCL scaffold provide favourable microenvironment for the proliferation and chondrogenic differentiation of the given SMSCs and encouraged cartilage regeneration, thereby considerably enhancing the healing of cartilage defects.
Some other examples of chitosan-based scaffolds used for 3D bioprinting are summarized in Table 2. Overall, these studies suggest the potential of chitosan-based hydrogels in tissue engineering, drug delivery, and various other medical applications.
Table 2 List of chitosan-based hydrogels used for 3D bioprinting
Chitosan |
Other polymers/ligands/materials |
Preparation method |
Testing of days |
In vitro/in vivo models |
Disease/targeting applications in |
Brief findings |
Ref. |
Abbreviations: PGA: polygamma-glutamic acid, BGP: β-glycerophosphate, HEC: hydroxyethyl cellulose, CNCs: cellulose nanocrystals, PCL: poly(ε-caprolactone), TFNA: tetrahedral framework nucleic acid, SMSCs: synovial mesenchymal stem cells, KC: keratinocytes, HDF: human dermal fibroblasts, GE: genipin, MSCs: human bone marrow mesenchymal stems cells, PVA: poly(vinyl alcohol), LEV: levofloxacin, RHCMA: recombinant human collagen methacrylic anhydride, HUVECs: human umbilical vein endothelial cells, GA: gallic acid. |
Chitosan scaffolds |
Acetic acid, raffinose |
Extrusion |
35 days |
Nhdf and HaCaT cells, Wistar rats |
Wound healing in diabetes condition |
In vitro and in vivo studies suggested 3D printed scaffolds improves the quality of restored tissues |
156
|
Chitosan hydrogel |
Gamma-PGA |
Double extrusion |
14 days |
Human adult fibroblasts |
Biomedical applications |
70% of surviving cells remained in the in the hydrogel with maintained viability over a 14-day incubation period |
160
|
Chitosan |
BGP, HEC and CNCs |
Extrusion |
21 days |
MC3T3-E1 cells |
Osteogenic differentiation |
Cell viability remained uncompromised by the bioinks. CNCs enhanced the osteogenic potential of MC3T3-E1 cells within chitosan scaffolds |
157
|
Chitosan hydrogel |
PCL, TFNA |
Extrusion |
21 days |
SMSCs, Rabbits |
Articular cartilage (AC) injury |
Enhanced cell proliferation, chondrogenesis and promote AC regeneration |
159
|
Chitosan bioink |
GE |
Extrusion |
7 days |
KC and HDF cells |
Skin regeneration |
MTT assay revealed that 93% of the cells in the printed construct were viable |
158
|
Chitosan hydrogel |
Gelatin, BGP, sodium bicarbonate |
— |
— |
MSCs |
3D models and tissue engineering |
The most viable encapsulated mesenchymal stem cells were found in CH2%, and the support bath-assisted bioprinting method |
161
|
Chitosan hydrogel |
MA and tricine |
|
7 days |
MC3T3-E1 cells |
Tissue engineering applications |
When encapsulated in CHTMA-Tricine constructions, MC3T3-E1 pre-osteoblast cells are shown to be cytocompatible and to remain viable for six days |
162
|
Chitosan hydrogel |
PVA, gelatin and LEV |
Physically cross-linking |
24 hours |
NHF cells |
Tissue engineering applications |
Cytotoxicity and cytocompatibility tests confirmed the cytocompatible nature of scaffolds |
163
|
Acidified chitosan |
RHCMA |
Cross-linking |
72 hours |
HUVECs cells |
3D bioprinting and tissue engineering |
In vitro studies confirmed biocompatibility of CS-RHCMA bioinks |
164
|
Chitosan |
GA |
Self-crosslinking |
7 days |
NIH3T3 cells |
Tissue engineering and regenerative medicine |
3D-printed CS-GA bioink scaffolds exhibited good cytocompatibility and biodegradability |
165
|
3.3 Biological characterization
3.3.1 Drug release.
The porosity of the hydrogel makes a path for higher drug release. Depending on the application, a perfectly suitable drug has to be chosen.166 writes that the drug should be selected in a way that improves stiffness, stability, and elastic properties. The selection of release media depends on the environment to be mimicked. Before release tests, the drug loading efficiency percentage must be calculated. The hydrogels were crushed by using a mortar and pestle.21 The powdered hydrogel can be placed in the release media under continuous stirring until maximum release occurs. We can find the loading and encapsulation efficiency. Using the formulae below.
Once the loading and encapsulation efficiencies are determined, the in vitro drug release test can be performed using indirect or direct dissolution methods,167 the loading method must be chosen carefully as it can directly influence the drug release. Direct immersion of hydrogel in buffer solutions38 or Dialysis membrane bags can be used as an indirect method.21 The beakers are placed in a dissolution shaker apparatus at a set rpm and temperature. This continuous agitation of beakers is to keep the sample from aggregating.168 At specific time intervals, aliquots of the sample can be collected, and the same amount of fresh-release media amount of drug release can be calculated using a UV spectrophotometer. The corresponding cumulative drug release can be calculated using a calibration curve.
The porous structure of chitosan hydrogels allows for the sustained release of drugs from the framework. Guaresti et al. designed a pH-responsive Chloramphenicol-loaded chitosan hydrogel and measured the release of the drug.169 They observed sustained drug release from the hydrogel, with the concentration increasing over time. The release process was slower than the swelling, and after 4 hours, the entire drug was released. The degree of cross-linking in the hydrogel and the hydrophilic/hydrophobic interactions will determine both the swelling capacity and the amount and rate of drug release.170
3.3.2
In vivo degradation.
The presence of any foreign body for a while can cause harmful infections leading to cancer and sometimes death too. Hence it is vital to remove it in time as there cannot be a completely biocompatible material. The alternative for removal is in vivo degradation of the material/hydrogel with time.74 has written that biodegradability can be tuned with different types of crosslinking agents, varying the concentration of crosslinkers. Biomaterials must be able to degrade to be safely, easily, and non-invasively removed from the body. With time the subsequent degradation of hydrogel provides space for new formation of tissues. To assess the hydrogel degradability in vivo, the self-healing injectable hydrogel solution was injected into the anesthetized animal body like mice, rats, guinea pigs, and rabbits. In general, ethyl ether50,171–173 is used to anesthetize mice. The animal body can be exposed to UV to construct the gel in situ.38 However, in ref. 172 the formation of transparent hydrogel under the skin was automatic without any additional trigger. In ref. 173, the hydrogel formation was confirmed by hand and observed using a digital camera.
The FDA has approved chitosan as a non-toxic, biocompatible, and biodegradable polymer for use in wound dressings.174 Su F. et al. synthesized carboxymethyl chitosan-crafted polylactide (CMCS-PLA) and carboxymethyl chitosan (CMCS) hydrogel and assessed the biocompatibility and in vivo degradation of this prepared hydrogel.175 The in vitro and in vivo studies demonstrated that the prepared chitosan hydrogels are biocompatible and degrade in vivo after 19 days, proving their promising future as a nanocarrier. A biodegradable carboxymethyl chitosan/oxidative hyaluronic acid hydrogel, synthesized by Xia L. et al., demonstrated intriguing characteristics following intraperitoneal injection.176 Analysis of the biodegradation, distribution, and urine excretion of this synthesized hydrogel revealed rapid drug dispersion to the blood, kidneys, and liver, with minimal distribution to the spleen, lungs, and heart. The majority of the hydrogel was eliminated via urine within 84 hours post-injection, while the remaining portion was excreted as comparatively smaller molecular weight fragments within 36 hours post-injection. Following in vivo degradation, these hydrogels exhibit remarkable characteristics, posing no adverse effects on tissues.177 Their biocompatibility makes them suitable for various biomedical applications. Moreover, studies indicate that chitosan-based hydrogels can potentially enhance cell proliferation and differentiation, thereby offering therapeutic benefits in tissue engineering and regenerative medicine.
3.3.3 Cell viability or cell proliferation.
Cell-based assays are prominently used to screen collections of chemicals to observe the impact of test molecules on cell growth and cytotoxic effects that ultimately result in cell death. Regardless of the cell-based test employed, it is essential to understand how many viable cells are still present after the experiment. The number of cells that are still viable can be determined using a variety of assay techniques.178 The resazurin reduction, protease activity, and tetrazolium reduction assay each measure a different aspect of general metabolism or an enzyme activity as a sign of live cells. To identify live cells, several tetrazolium compounds have been employed.179 The most often used substances are XXT, MTS, MTT, and WST-1.180 These cells along with cellular pH and osmotic pressure controllers, supplements like proteins, vitamins, carbohydrates, amino acids, growth factors, and antibiotics are29 seeded according to cell count in a 96-well plate and allowed for cell culture for a certain period.180 Serums constitute a conventional integration of amino acids, vitamins, carbon sources (e.g., glucose), and inorganic salts. Then the self-healing hydrogel solution is added to the well plates and incubated again.180 The total number of viable cells is calculated by multiplying the number of cells discovered in all four squares by 104 (to get the average cell number per mL), multiplied by 2 (to get the Trypan Blue dilution factor), and the original medium volume of the complete cell suspension. By dividing the total number of labeled cells by the total number of cells and multiplying the result by 100, the percentage of viable cells may be calculated. Cell viability of 80 to 95 percent indicates good cell culture. The other method consists of staining the sample with dyes like calcein-AM (green), Safranine followed by observation under an inverted fluorescence microscope. The cells can be numbered using the ImageJ software. The relative growth rate can be calculated by placing the 96-well plate in a microplate reader at a set absorbance value. The growth rate ratio is also known as the cell proliferation ratio.
In extension to cell culture, chitosan aids cell differentiation; cells acquire specialized structures and functions during development or in response to specific signals. Differentiation allows cells to transform from an undifferentiated state (stem or progenitor cells) into specific cell types with distinct characteristics and functions. Also, they change gene expression, morphology, and functionality to fulfill specific roles in the body. This process is tightly regulated and influenced by various factors, including growth factors, signaling molecules, and environmental cues. Cell differentiation is a fundamental process involving embryonic development, tissue regeneration, and the maintenance of tissue homeostasis.181 used chitosan graphene oxide composite hydrogels for the differentiation of neuroblastoma cells.
Modi U. et al., developed a 3D tumoroid model using chemically modified dextran-chitosan hydrogel. This 3D tumoroid model exhibits physiologically similar migration, proliferation, and invasive potential.182 Another chitosan hydrogel synthesized by Kwon J. S. et al. provides a 3D substrate for the cell proliferation, attachment, and differentiation of rat muscle-derived stem cells (rMDSCs) in the presence of valproic acid (VA).183 The literature has demonstrated that modified chitosan-based hydrogels promote cell proliferation and differentiation of Human retinal pigmented epithelial-1 (RPE-1) cells,184 osteoblasts,185 neural progenitor cells (NSCs),186 rat muscle-derived stem cells (rMDSCs),183 fibroblasts,187 and human adipose-derived stem cells (hADSCs).188
3.3.4 Blood compatibility.
Blood compatibility refers to a material's ability to interact with blood without causing adverse reactions. Assessing this is crucial for hydrogels, which are extensively used in biomedical applications. Methods for evaluating blood compatibility include hemolysis assays, platelet adhesion and activation tests, coagulation assays, complement activation assays, and in vivo studies.189 These assessments measure the hydrogel's impact on red blood cells, platelets, blood clotting, immune response, and overall physiological compatibility, ensuring its safety for use within the circulatory system and other blood-contacting applications. As discussed, chitosan stands as an FDA-approved biocompatible polymer, with numerous studies consistently demonstrating its non-toxic and biocompatible nature upon interaction with blood components. Su F. et al., assessed the biocompatibility of a hydrogel prepared from carboxymethyl chitosan polylactide (CMCS-PLA) and CMCS using the hemolysis test.175 Samples of CMCS and CMCS-PLA did not induce hemolysis in the blood system. It is widely acknowledged that if the Hemolysis Rate (HR) value is less than 5%, biomaterials can be considered suitable for biological applications. The HR values for CMCS and CMCS-PLA were found to be 1.4% and 1.7%, respectively, indicating that both materials possess exceptional anti-hemolysis qualities. Overall, literature suggests that chitosan-based hydrogels are biocompatible and suitable for biomedical applications.190
3.3.5 Antibacterial activity191.
Antibacterial agents fight against infectious diseases, and hence to evaluate it, a small volume of Gram-positive (Staphylococcus aureus) and Gram-negative bacteria (Escherichia coli) is added into the 24-well microplate consisting of the hydrogel solution. The suspension is evenly scattered on an agar plate and incubated at 37 °C for a day or so (18–24 h),135 the number of colonies formed is counted, and the kill percentage can be calculated using the formula below.
The other method is the zone of inhibition. The bacterial suspensions alone are spread on the Luria-Bertani agar plates and some amount of hydrogel samples were placed on the surfaces.192 The same can be performed by making a hydrogel + bacterial suspension beforehand. After prolonged incubation,193 a circular region will be created where the bacteria cannot grow around the samples; this was considered the inhibition zone. The zone of inhibition can be obtained using vernier calipers.14 observed the morphology of bacteria in the hydrogel using a Scanning electron microscope after incubating for a period of 5 h. They found that the hydrogel with S. aureus cells revealed a wrinkled, ruptured, and damaged membrane structure and morphology, but the hydrogel with loaded E. coli did not significantly affect the cell morphology.
Chitosan-based injectable, self-repairing hydrogels exhibit remarkable antimicrobial efficacy, particularly against drug-resistant bacteria, owing to their multifaceted antimicrobial mechanisms.194 These hydrogels possess inherent cationic properties, enabling electrostatic interactions with negatively charged bacterial cell membranes, thereby disrupting membrane integrity and inducing leakage of cellular contents.195 Additionally, chitosan-based hydrogels can interfere with bacterial cell wall synthesis, inhibit microbial enzyme activity, and disrupt microbial biofilm formation, further augmenting their antimicrobial potency.196 Moreover, the injectable and self-repairing nature of these hydrogels allows for targeted delivery and sustained release of antimicrobial agents, ensuring prolonged therapeutic efficacy against recalcitrant bacterial strains.197 Pengpeng Deng et al., developed injectable, self-healing chitosan hydrogels modified with adenine for use in wound healing applications. The hydrogels shown remarkable antibacterial properties against drug-resistant bacteria, fungus, and Gram-positive and Gram-negative bacteria. Furthermore, these designed hydrogels significantly improved wound healing and decrease the infiltration of inflammatory cells in skin defect model.198 In another study, Farasati Far B. et al., developed gelatin corss-linked chitosan-based hydrogels and antibacterial efficiency was examined against Gram-positive and Gram-negative bacteria.199 These antibacterial studies demonstrated that prepared chitosan hydrogels significantly inhibit bacterial growth and reduce the MIC and MBC values against E. coli and S. aureus. The biocompatibility of the hydrogels was also confirmed by the MTT assay and demonstrated antibacterial effects of chitosan-based hydrogels. Overall, chitosan-based hydrogels could be a promising avenue for use in antibacterial applications.
4. Biological applications of chitosan-based hydrogels
Chitosan-based hydrogels offer promising avenues in biological applications due to their biocompatibility and bioactivity. These hydrogels find utility in tissue engineering, wound healing, and drug delivery systems, providing a versatile platform for controlled release and cell interaction, thus advancing therapeutic interventions in regenerative medicine. Table 3 summarize the different biological applications of Chitosan hydrogels.
Table 3 Different biological applications of chitosan hydrogels
Type of application |
Type of chitosan – substrate used |
Main findings |
Ref. |
Tissue regeneration |
N-Carboxyethyl chitosan |
Electroactivity and conductivity |
200
|
Carboxymethyl chitosan |
(1) Cytocompatibility |
34 and 201–203
|
(2) Wet-tissue adhesion |
Antimicrobial properties |
|
Wound dressing |
Quaternized chitosan |
(1) Can be applied on irregular and motion wounds |
59 and 204
|
(2) Tissue adhesion |
Acetic acid modified chitosan |
(1) Nontoxic |
69, 134 and 205 |
(2) Blood absorption & hemostatic capability |
Adenine-modified chitosan (AC) |
Hemostatic effect, reduced inflammation, regeneration of collagen |
135
|
Chitosan Hydrochloride |
Anti-inflammation, skin repair |
81
|
PEG-Modified Chitosan |
Hemostatic effect, Tissue adhesion |
19
|
Carboxymethyl chitosan |
(1) Enhances angiogenesis, collagen secretion |
206 and 207
|
(2) Tissue adhesive |
|
Drug delivery |
Orotic acid modified chitosan |
Stimulus responsiveness, injectability, conductivity |
126
|
|
Gastrointestinal |
Carboxymethyl chitosan |
Cell migration, wet tissue adhesive drug delivery |
201
|
|
Cell carriers |
N-Carboxyethyl chitosan |
Cell delivery carrier for cell therapy for skeletal muscle repair |
200 and 208
|
Chitosan Hydrochloride |
RPC-based transplantation therapy |
209
|
Proliferation & differentiation of RPC |
PEG-modified chitosan |
Cell therapy & cardiac tissue repair |
210
|
Carbamoylated chitosan |
Improved cell viability, adhesion, growth and cell differentiation, |
115 and 116
|
|
Cancer treatment |
Acetic acid modified chitosan |
Superior mechanical strength |
134
|
N,O carboxymethyl chitosan |
Prolonged release of anticancer agents at the tumoral site |
74
|
4.1 Tissue regeneration
Self-healing injectable hydrogels have emerged as an appealing technique for tissue regeneration in various medical applications. These hydrogels are composed of cross-linked polymers that can absorb huge quantities of water, giving them a gel-like consistency similar to extracellular matrix (ECM). They possess the unique ability to autonomously repair themselves upon damage, making them particularly advantageous for tissue engineering and regenerative medicine.
4.2 Cardiac tissue
Myocardial infarction is the world's largest reason for heart failure. Cardiac injuries are irreversible, and heart transplantation was the only solution till the invention of self-healing injectable hydrogels, but modified chitosan with self-healing injectable properties could reduce the infarct size, protect the transplanted cells and improve cardiac function211 due to their ability to conjugate with various bioactive molecules.212
4.3 Cartilage tissue
Cartilage is a complex and vascular tissue with limited self-healing capacity. Injectable hydrogels can be loaded with chondrogenic cells, growth factors, and extracellular matrix components and then injected into the damaged cartilage site to provide a supportive environment for cell growth and tissue formation, while its self-healing properties ensure the integrity of the scaffold, promoting the regeneration of functional cartilage.34
4.4 Bone regeneration
chitosan-based self-healing injectable hydrogels can mimic the natural extracellular matrix, providing structural support and an ideal environment for bone regeneration in bone defect repair caused by trauma, disease, or surgical procedures.
4.5 Bone defect repair
Chitosan-based self-healing injectable hydrogels have been used to repair bone defects caused by trauma, disease, or surgical procedures. These hydrogels can be injected directly into the defect site, promoting cell proliferation, angiogenesis, and mineralization, leading to bone regeneration.203 Chitosan-based injectable hydrogels can treat osteoarthritis, a degenerative joint disease213 Periodontitis is an inflammatory disease caused by infection, destroying soft gum tissues and subsequent bone loss. Carboxymethyl chitosan (CMCS), which possesses properties of wet-tissue adhesion, excellent antibacterial capabilities, and cytocompatibility, can be utilized in this context. Additionally, CMCS holds the potential for drug delivery to wet tissues in oral diseases.201 Other than the above applications, it can be used in nerve regeneration which is a gradual process involving the disconnection of nerve sheaths and underlying neurons. To expedite healing and regeneration, external assistance is required.202 In this study, a conductive hydrogel was introduced into the cavity of a carboxymethyl chitosan conduit prepared via electrodeposition. The application of the hydrogel demonstrated the promotion of sciatic nerve regeneration in an in vivo setting. Skeletal muscle possesses the intrinsic regenerative capacity, enabling it to undergo regeneration in response to minor injuries, as well as more severe conditions such as myopathies, prolonged denervation, significant traumatic injury, ischemia, and aggressive tumor ablation.200 These clinical conditions can result in substantial muscle loss.
4.6 Wound healing
The ability to be administered in a minimally invasive manner allows the hydrogel formulation prepared as a liquid can be easily injected into the wound site. Once it comes in contact with physiological conditions, the liquid transforms into a gel, forming a physical barrier that protects the wound from external contaminants and provides a moist environment for wound healing so that secondary infections do not occur. Chitosan has vast applications in wound dressing because of its hemostatic capability, antibacterial, antioxidant properties, and tissue adhesion. The uniform porous structure of chitosan-based hydrogels is beneficial in absorbing and storing excess exudate from the wound area and ensures oxygen permeability for wound healing. Its conductive nature can be exploited to monitor the real-time physical condition of the patient reducing the risk of delaying the disease.18 The combined motion of polymer chains and the dynamic nature of Schiff bases within the hydrogels, particularly at the fracture surface, facilitate the re-crosslinking of free functional groups. This unique characteristic grants the hydrogels rapid self-healing capability. Consequently, the morphology and mechanical properties of the hydrogels can effectively recover following damage, showcasing their potential to withstand internal and external forces at the site of injury.
Chitosan has gained attention in wound healing due to its unique properties such as antimicrobial activity, haemostatic effect, and promotion of tissue regeneration.214 When formulated into hydrogels, chitosan exhibits enhanced properties, making it an attractive material for various wound types.215
4.6.1 Diabetic wounds.
Diabetic wounds pose a significant challenge in wound management due to impaired healing processes.216 Chitosan-based hydrogels offer a promising solution by providing a scaffold for cell proliferation and angiogenesis. that chitosan hydrogel loaded with growth factors (such as EGF, Epidermal Growth Factor) accelerated healing in diabetic foot ulcers by promoting cell proliferation and collagen deposition, along with exhibiting antibacterial effects.217
4.6.2 Acute wounds.
Acute wounds resulting from traumatic injuries require rapid and effective treatment to prevent infection and facilitate healing.218 Chitosan-based hydrogels have been utilized as wound dressings due to their haemostatic properties and ability to adhere to the wound bed. Research by Zhang Y. et al., and Guo S. et al., demonstrated the efficacy of chitosan-based hydrogels in promoting haemostasis and accelerating wound closure in acute trauma cases, skin injury, rapid hemostasis and hemorrhage.219,220
4.6.3 Ulcerated wounds.
Chronic ulcerated wounds, such as pressure ulcers and venous ulcers, present persistent challenges in clinical management.221 Chitosan-based hydrogels offer a multifaceted approach to ulcer healing by reducing inflammation, promoting granulation tissue formation, and preventing bacterial colonization.222 An in vivo study conducted by J. H. et al., on rabbits and pigs demonstrated significant improvements in gastrointestinal ulcer healing rates with the application of chitosan hydrogel containing EGF compared to control groups.223 The EGF-chitosan hydrogels were applied in two models of rabbits and pigs: the acetic acid-induced gastric ulcer model (AAU) and the mucosal resection-induced gastric ulcer model (MRU). The ulcer size decreased up to 2.3 times within 3 days and 5.4 times within a single day in the AAU and MRU models, respectively, after ulceration implying the chitosan hydrogels as a promising candidate for the treatment of ulcer wounds.
4.6.4 Sports wounds.
Athletes frequently encounter abrasions, lacerations, and other skin injuries during sports activities. Chitosan-based hydrogels provide an ideal wound dressing solution for sports-related wounds due to their flexibility, antimicrobial properties, and promotion of tissue regeneration.224 Djekic, L. et al., developed the chitosan-based hydrogels for the skin wounds leading to sustained release of ibuprofen.225
4.6.5 Burn wounds.
Burn injuries often result in extensive tissue damage and impaired wound healing. Chitosan-based hydrogels offer several advantages in burn wound management, including cooling effects, moisture retention, pain reduction, infection prevention, and promotion of epithelialization.226,227 Yang C. et al., prepared carboxymethylated chitosan hydrogel cross-linked with thione groups and loaded with curcumin (Cur@CMCTS-Tk).228 In the full-thickness skin burn defect rat model, this hydrogel loaded with curcumin effectively accelerated the wound healing process and demonstrated good regenerative properties, such as the formation of hair follicles, promotion of new blood vessel formation, and highly ordered collagen fiber arrangement (Fig. 9). Overall, because the produced hydrogel can scavenge excess ROS, it may be applied to wound healing and tissue regeneration.
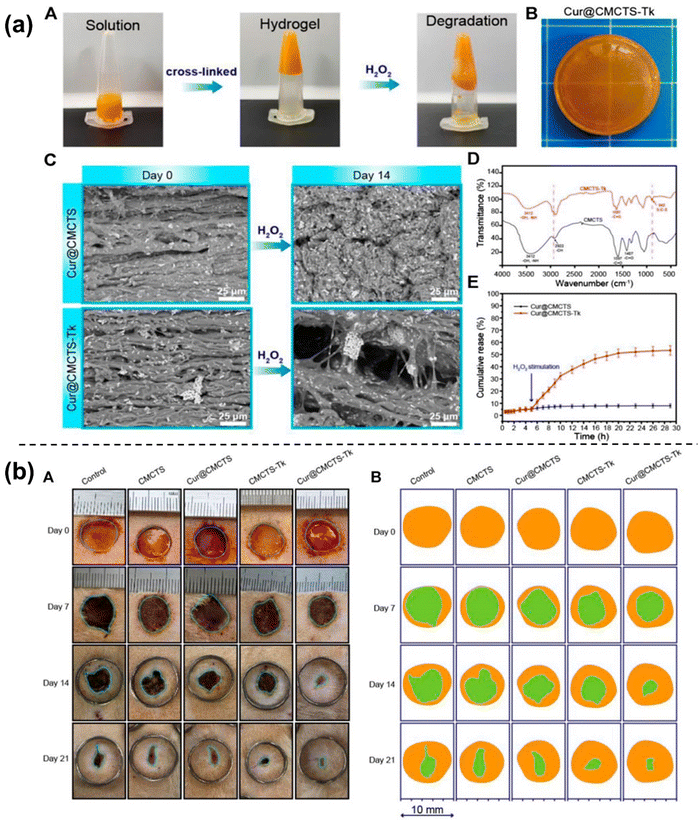 |
| Fig. 9 Characterization including SEM analysis, FTIR and release study of prepared Cur@CMCTS-Tk hydrogel (a). Digital photographs of the wounds on the dorsum of rats treated with Cur@CMCTS-Tk hydrogel for up to 21 days (b). Figures reproduced from Front. Pharmacol. [ref. 18], Open access, Copyright © 2021, Frontiers. | |
4.7 Drug delivery
Hydrogels can be induced into the human body through different routes such as oral, transdermal, ocular,64 rectal, vaginal,229 and subcutaneous.230 Oral delivery can treat the stomach, intestine, i.e., Intraperitoneal, oral cavity, and colon.231 Chitosan hydrogels can undergo significant swelling and degradation in aqueous environments. This can result in a loss of structural integrity over time, leading to decreased mechanical properties and potential release of encapsulated substances. The degree of swelling and degradation depends on factors such as chitosan concentration, degree of acetylation, pH of the surrounding environment, and the nature of encapsulated molecules.
pH sensitivity and thermal, photothermal responsive techniques are smart drug delivery applications.126 Yongyan Yang et al., 2023 synthesized a pH-responsive hydrogel with orotic acid-modified chitosan and 2,6 diaminopurine (OACS-DAP). When formed in an acidic solution, the hydrogel was in a solid state but at a pH above 4, led to a phase shift from solid to liquid or semi-liquid state. This physical change was reversible in nature depicting the self-healing ability of chitosan. Often pH-responsive drug release is induced in applications or gastrointestinal and Periodontal Diseases.201 Additionally, self-healing hydrogels can be combined with other technologies, such as nanoparticles232,233 or microneedles,234,235 to further enhance drug delivery capabilities.153 Marapureddy et al. 2022 induced nanosheets of graphene oxide to improve the mechanical strength and to obtain a pH-responsive sustained drug release. In addition to hydrogels153 could fabricate chitosan films using the same. These hybrid systems can provide additional functionalities, such as improved drug loading capacity, enhanced targeting, or sustained release.
4.8 Cell carriers
Self-healing and injectable hydrogels offer distinct advantages compared to conventional injectable hydrogels for cell delivery: (1) mitigation of cell loss risks during injection: Self-healing and injectable hydrogels minimize the potential risks associated with cell loss during the injection process. They provide mechanical protection to the delivered cells, safeguarding them from shear damage during injection.121 (2) Assurance of cell morphology and functionality: These hydrogels enable the confirmation of cell morphologies and functionalities within the three-dimensional (3D) microenvironment. This allows for quality control of loaded cells before transplantation, ensuring optimal cellular characteristics for successful outcomes.236 (3) Facilitation of rapid mechanical recovery: Self-healing and injectable hydrogels can rapidly recover from mechanical damage. This property preserves the intrinsic functionalities of the hydrogel and extends the service life of implanted cell-loaded hydrogels. Maintaining the hydrogel's structural integrity ensures the sustained functionality and longevity of the encapsulated cells.237
5. Conclusions, drawbacks & prospectives
Self-healing injectable hydrogels exhibit the unique ability to withstand and reverse the damage that accumulates over time and external factors. They can be intentionally designed to mimic physiological conditions, but the absence of reversible bonds refrains them from recoverability. In a biopolymer like chitosan, introducing Schiff base linkages create reversible imine bonds, which can revert back the damage both macroscopically and microscopically. The insoluble nature of chitosan was addressed using different modifications, be it with periodate oxidation or poly-ethyl-glycol, a non-toxic, water-soluble polymer, or the usage of any chitosan derivatives. To determine the properties various instruments and experiments equipped in all the tests, chitosan could reveal its recoverability, printability, biocompatibility, and mechanical strength. As an application to these properties, chitosan was used in tissue-engineered scaffolds, wound dressings, drug delivery, and cancer treatment.
Chitosan can be used as a carrier for drugs, proteins, antigens, and genes, but the complication in insolubility requires harsh and long synthesis procedures to be followed. Modification of chitosan enhances the solubility and mechanical performance in neutral and alkaline solutions but might lower its inherent properties like biocompatibility and degradation. In fact, it is better to combine biodegradable substances as the slightest non-biodegradability in vivo has a sure chance of infection, cancer, and sometimes death too. They often need to be combined with strength-inducing substances because of their low strength. This limits its combination with various components, although it's compatible. Traditional hypodermic injections can cause pain and discomfort. The inherent property of the above-discussed hydrogels is injectability, but using hydrogel-forming microneedles (HFMs) provides a minimally invasive approach through transdermal layers eliminating the need for injection equipment.
It's worth noting that the specific drawbacks of chitosan-based hydrogels can be mitigated or overcome to some extent through modifications, such as blending with other polymers, incorporation of cross-linking agents, reinforcement with fibers,36,238 or optimization of processing conditions. These modifications can improve mechanical properties, control gelation behavior, and enhance biocompatibility.
The Prospects of self-healing injectable hydrogels are promising and hold significant potential for various applications in the medicinal field. The tunable nature of self-healing injectable hydrogels allows for customization based on patient-specific needs. By tailoring the properties of these hydrogels, such as mechanical strength, degradation rate, and bioactive molecule release, they can be optimized for individual patients or specific medical conditions, supporting the concept of personalized medicine by encapsulating different drugs and therapeutics. Their potential to revolutionize minimally invasive procedures makes them both patient and doctor friendly. (1) Inculcating the conductive nature of chitosan-based self-healing injectable hydrogels can aid in biosensing applications. (2) Recently, chitosan-based injectable microneedles were invented whose application was safe and easy. It requires a gentle pressure or patching onto the skin without any specialized equipment or medical professionals. In the same route, other polysaccharides can be designed for various and better applications.
Conflicts of interest
The authors declare no conflict of interest.
Acknowledgements
We thank all the members of DB and PT labs for useful discussions and feedback. ME thanks MoE GoI for PhD fellowship. R. S. acknowledges the Science and Engineering Research Board (SERB), Government of India, for financial support through the National Post-Doctoral Fellowship (NPDF). DB and PT thank SERB GoI for Ramanujan fellowship and Core research grant.
References
- G. C. Gurtner, S. Werner, Y. Barrandon and M. T. Longaker, Wound Repair and Regeneration, Nature, 2008, 314–321, DOI:10.1038/nature07039.
- ’ S. L. Teitelbaum and F. P. Ross, Genetic Regulation of Osteoclast Development and Function, Nat. Rev. Genet., 2003, 4(8), 638–649 CrossRef CAS PubMed.
- G. K. Michalopoulos, Liver Regeneration, J. Cell. Physiol., 2007, 286–300, DOI:10.1002/jcp.21172.
- M. H. Tuszynski and O. Steward, Concepts and Methods for the Study of Axonal Regeneration in the CNS, Neuron, 2012, 777–791, DOI:10.1016/j.neuron.2012.05.006.
- V. Morya, S. Walia, B. B. Mandal, C. Ghoroi and D. Bhatia, Functional DNA Based Hydrogels: Development, Properties and Biological Applications, ACS Biomater. Sci. Eng., 2020, 6021–6035, DOI:10.1021/acsbiomaterials.0c01125.
- Y. Dong, C. Yao, Y. Zhu, L. Yang, D. Luo and D. Yang, Dna Functional Materials Assembled from Branched Dna: Design, Synthesis, and Applications, Chem. Rev., 2020, 9420–9481, DOI:10.1021/acs.chemrev.0c00294.
- J. Y. Lai and A. C. Hsieh, A Gelatin-g-Poly(N-Isopropylacrylamide) Biodegradable in Situ Gelling Delivery System for the Intracameral Administration of Pilocarpine, Biomaterials, 2012, 33(7), 2372–2387, DOI:10.1016/J.BIOMATERIALS.2011.11.085.
- X. D. Xu, B. Wang, Z. C. Wang, S. X. Cheng, X. Z. Zhang and R. X. Zhuo, Fabrication of Fast Responsive, Thermosensitive Poly(N-Isopropylacrylamide) Hydrogels by Using Diethyl Ether as Precipitation Agent, J. Biomed. Mater. Res., Part A, 2008, 86(4), 1023–1032, DOI:10.1002/jbm.a.31695.
- G. Deng, F. Li, H. Yu, F. Liu, C. Liu, W. Sun, H. Jiang and Y. Chen, Dynamic Hydrogels with an Environmental Adaptive Self-Healing Ability and Dual Responsive Sol–Gel Transitions, ACS Macro Lett., 2012, 1(2), 275–279, DOI:10.1021/mz200195n.
- M. V. Risbud, A. A. Hardikar, S. V. Bhat and R. R. Bhonde, PH-Sensitive Freeze-Dried Chitosan–Polyvinyl Pyrrolidone Hydrogels as Controlled Release System for Antibiotic Delivery, J. Controlled Release, 2000, 68(1), 23–30, DOI:10.1016/S0168-3659(00)00208-X.
- P. Bertsch, M. Diba, D. J. Mooney and S. C. G. Leeuwenburgh, Self-Healing Injectable Hydrogels for Tissue Regeneration, Chem. Rev., 2022, 123(2), 834–873, DOI:10.1021/acs.chemrev.2c00179.
- R. Chen, Y. Hao, S. Francesco, X. Mao and W.-C. Huang, A Chitosan-Based Antibacterial Hydrogel with Injectable and Self-Healing Capabilities, Mar. Life Sci. Technol., 2023, 6(1), 115–125, DOI:10.1007/s42995-023-00211-z.
- K.-C. Cheng, C.-F. Huang, Y. Wei and S. Hsu, Novel Chitosan–Cellulose Nanofiber Self-Healing Hydrogels to Correlate Self-Healing Properties of Hydrogels with Neural Regeneration Effects, NPG Asia Mater., 2019, 11(1), 25, DOI:10.1038/s41427-019-0124-z.
- H. Cui, B. Cui, H. Chen, X. Geng, X. Geng, Z. Li, S. Cao, J. Shen and J. Li, A Chitosan-Based Self-Healing Hydrogel for Accelerating Infected Wound Healing, Biomater. Sci., 2023, 11, 4226–4237, 10.1039/d3bm00061c.
- A. B. W. Brochu, S. L. Craig and W. M. Reichert, Self-Healing Biomaterials, J. Biomed. Mater. Res., Part A, 2011, 96A(2), 492–506, DOI:10.1002/jbm.a.32987.
- F. Ding, H. Li, Y. Du and X. Shi, Recent Advances in Chitosan-Based Self-Healing Materials, Res. Chem. Intermed., 2018, 44(8), 4827–4840, DOI:10.1007/s11164-018-3339-7.
- Y. Tu, N. Chen, C. Li, H. Liu, R. Zhu, S. Chen, Q. Xiao, J. Liu, S. Ramakrishna and L. He, Advances in Injectable Self-Healing Biomedical Hydrogels, Acta Biomater., 2019, 1–20, DOI:10.1016/j.actbio.2019.03.057.
- L. Fan, Z. He, X. Peng, J. Xie, F. Su, D.-X. Wei, Y. Zheng and D. Yao, Injectable, Intrinsically Antibacterial Conductive Hydrogels with Self-Healing and PH Stimulus Responsiveness for Epidermal Sensors and Wound Healing, ACS Appl. Mater. Interfaces, 2021, 13(45), 53541–53552, DOI:10.1021/acsami.1c14216.
- C. Ding, M. Tian, R. Feng, Y. Dang and M. Zhang, Novel Self-Healing Hydrogel with Injectable, PH-Responsive, Strain-Sensitive, Promoting Wound-Healing, and Hemostatic Properties Based on Collagen and Chitosan, ACS Biomater. Sci. Eng., 2020, 6(7), 3855–3867, DOI:10.1021/acsbiomaterials.0c00588.
- G. Jiang, J. Sun and F. Ding, PEG-g-Chitosan Thermosensitive Hydrogel for Implant Drug Delivery: Cytotoxicity, in Vivo Degradation and Drug Release, J. Biomater. Sci., Polym. Ed., 2014, 25(3), 241–256, DOI:10.1080/09205063.2013.851542.
- A. Ahsan, M. Asim Farooq and A. Parveen, Thermosensitive Chitosan-Based Injectable Hydrogel as an Efficient Anticancer Drug Carrier, ACS Omega, 2020, 5(32), 20450–20460, DOI:10.1021/acsomega.0c02548.
- E. Khare, N. Holten-Andersen and M. J. Buehler, Transition-Metal Coordinate Bonds for Bioinspired Macromolecules with Tunable Mechanical Properties, Nat. Rev. Mater., 2021, 6(5), 421–436 CrossRef CAS.
- J. Xu, Y. Liu and S. H. Hsu, Hydrogels Based on Schiff Base Linkages for Biomedical Applications, Molecules, 2019, 24(16), 3005, DOI:10.3390/molecules24163005.
- D. M. Beaupre and R. G. Weiss, Thiol-and Disulfide-Based Stimulus-Responsive Soft Materials and Self-Assembling Systems, Molecules, 2021, 26(11), 3332, DOI:10.3390/molecules26113332.
- S. M. Morozova, Recent Advances in Hydrogels via Diels–Alder Crosslinking: Design and Applications, Gels, 2023, 9(2), 102, DOI:10.3390/gels9020102.
- G. Deng, F. Li, H. Yu, F. Liu, C. Liu, W. Sun, H. Jiang and Y. Chen, Dynamic Hydrogels with an Environmental Adaptive Self-Healing Ability and Dual Responsive Sol–Gel Transitions, ACS Macro Lett., 2012, 1(2), 275–279, DOI:10.1021/mz200195n.
- D. Q. Li, S. Y. Wang, Y. J. Meng, Z. W. Guo, M. M. Cheng and J. Li, Fabrication of Self-Healing Pectin/Chitosan Hybrid Hydrogel via Diels-Alder Reactions for Drug Delivery with High Swelling Property, PH-Responsiveness, and Cytocompatibility, Carbohydr. Polym., 2021, 268, 118244, DOI:10.1016/J.CARBPOL.2021.118244.
- Y. Zhou, Z. Zhai, Y. Yao, J. C. Stant, S. L. Landrum, M. J. Bortner, C. E. Frazier and K. J. Edgar, Oxidized Hydroxypropyl Cellulose/Carboxymethyl Chitosan Hydrogels Permit PH-Responsive, Targeted Drug Release, Carbohydr. Polym., 2023, 300, 120213, DOI:10.1016/j.carbpol.2022.120213.
- H. Li, F. Cheng, X. Wei, X. Yi, S. Tang, Z. Wang, Y. S. Zhang, J. He and Y. Huang, Injectable, Self-Healing, Antibacterial, and Hemostatic N,O-Carboxymethyl Chitosan/Oxidized Chondroitin Sulfate Composite Hydrogel for Wound Dressing, Mater. Sci. Eng., C, 2021, 118, 111324, DOI:10.1016/j.msec.2020.111324.
- J. Shen, W. Jiao, Z. Chen, C. Wang, X. Song, L. Ma, Z. Tang, W. Yan, H. Xie, B. Yuan, C. Wang, J. Dai, Y. Sun, L. Du and Y. Jin, Injectable Multifunctional Chitosan/Dextran-Based Hydrogel Accelerates Wound Healing in Combined Radiation and Burn Injury, Carbohydr. Polym., 2023, 316, 121024, DOI:10.1016/J.CARBPOL.2023.121024.
- H. Qiu, J. Deng, R. Wei, X. Wu, S. Chen, Y. Yang, C. Gong, L. Cui, Z. Si, Y. Zhu, R. Wang and D. Xiong, A Lubricant and Adhesive Hydrogel Cross-Linked from Hyaluronic Acid and Chitosan for Articular Cartilage Regeneration, Int. J. Biol. Macromol., 2023, 243, 125249, DOI:10.1016/J.IJBIOMAC.2023.125249.
- Z. Ling, Z. Chen, J. Deng, Y. Wang, B. Yuan, X. Yang, H. Lin, J. Cao, X. Zhu and X. Zhang, A Novel Self-Healing Polydopamine-Functionalized Chitosan-Arginine Hydrogel with Enhanced Angiogenic and Antibacterial Activities for Accelerating Skin Wound Healing, Chem. Eng. J., 2021, 420, 130302, DOI:10.1016/J.CEJ.2021.130302.
- A. H. Pandit, N. Mazumdar, K. Imtiyaz, M. M. Alam Rizvi and S. Ahmad, Self-Healing and Injectable Hydrogels for Anticancer Drug Delivery: A Study with Multialdehyde Gum Arabic and Succinic Anhydride Chitosan, ACS Appl. Bio Mater., 2020, 3(12), 8460–8470, DOI:10.1021/acsabm.0c00835.
- L. Ma, W. Su, Y. Ran, X. Ma, Z. Yi, G. Chen, X. Chen, Z. Deng, Q. Tong, X. Wang and X. Li, Synthesis and Characterization of Injectable Self-Healing Hydrogels Based on Oxidized Alginate-Hybrid-Hydroxyapatite Nanoparticles and Carboxymethyl Chitosan, Int. J. Biol. Macromol., 2020, 165, 1164–1174, DOI:10.1016/J.IJBIOMAC.2020.10.004.
- K.-C. Cheng, C.-F. Huang, Y. Wei and S. Hsu, Novel Chitosan–Cellulose Nanofiber Self-Healing Hydrogels to Correlate Self-Healing Properties of Hydrogels with Neural Regeneration Effects, NPG Asia Mater., 2019, 11(1), 25, DOI:10.1038/s41427-019-0124-z.
- R. Zhang, Y. Tao, Q. Xu, N. Liu, P. Chen, Y. Zhou and Z. Bai, Rheological and Ion-Conductive Properties of Injectable and Self-Healing Hydrogels Based on Xanthan Gum and Silk Fibroin, Int. J. Biol. Macromol., 2020, 144, 473–482, DOI:10.1016/j.ijbiomac.2019.12.132.
- Z. Li, J. Guo, F. Guan, J. Yin, Q. Yang, S. Zhang, J. Tian, Y. Zhang, M. Ding and W. Wang, Oxidized Sodium Alginate Cross-Linked Calcium Alginate/Antarctic Krill Protein Composite Fiber for Improving Strength and Water Resistance, Colloids Surf., A, 2023, 656, 130317, DOI:10.1016/J.COLSURFA.2022.130317.
- Y. Zhou, Z. Zhai, Y. Yao, J. C. Stant, S. L. Landrum, M. J. Bortner, C. E. Frazier and K. J. Edgar, Oxidized Hydroxypropyl Cellulose/Carboxymethyl Chitosan Hydrogels Permit PH-Responsive, Targeted Drug Release, Carbohydr. Polym., 2023, 300, 120213, DOI:10.1016/j.carbpol.2022.120213.
- M. N. V. Ravi Kumar, A Review of Chitin and Chitosan Applications, React. Funct. Polym., 2000, 46(1), 1–27, DOI:10.1016/S1381-5148(00)00038-9.
- F. S. Kittur, K. R. Kumar and R. N. Tharanathan, Functional Packaging Properties of Chitosan Films, Z. Lebensm.-Unters. -Forsch. A, 1998, 206(1), 44–47, DOI:10.1007/s002170050211.
- C. Augustin and O. Damour, Pharmacotoxicological Applications of an Equivalent Dermis: Three Measurements of Cytotoxicity, Cell Biol. Toxicol., 1995, 11(3–4), 167–171, DOI:10.1007/BF00756519.
- C. Augustin, V. Frei, E. Perrier, A. Huc and O. Damour, A Skin Equivalent Model for Cosmetological Trials: An in Vitro Efficacy Study of a New Biopeptide, Skin Pharmacol. Physiol., 1997, 10(2), 63–70, DOI:10.1159/000211470.
- Y.-G. Lu, J.-J. Wu and T.-Y. Zhu, Establishment of Composite Chitosan Artificial Skin, Chin. J. Clin. Rehabil., 2002, 6(4), 518–519 Search PubMed.
- E. Agulló, M. S. Rodríguez, V. Ramos and L. Albertengo, Present and Future Role of Chitin and Chitosan in Food, Macromol. Biosci., 2003, 3(10), 521–530, DOI:10.1002/mabi.200300010.
- T. Chandy and C. P. Sharma, Chitosan-as a Biomaterial, Biomater., Artif. Cells, Artif. Organs, 1990, 18(1), 1–24, DOI:10.3109/10731199009117286.
- T. Winie, S. R. Majid, A. S. A. Khiar and A. K. Arof, Ionic Conductivity of Chitosan Membranes and Application for Electrochemical Devices, Polym. Adv. Technol., 2006, 17(7–8), 523–527, DOI:10.1002/pat.744.
-
S. B. da Silva; G. L. Batista and C. K. Santin, Chitosan for Sensors and Electrochemical Applications, Chitin and Chitosan, Wiley, 2019, pp. 461–476 DOI:10.1002/9781119450467.ch18.
- A. Wibowo, R. F. Indrawan, L. A. T. Wulan Asri, S. S. Rahardi and B. S. Purwasasmita, The Influence of Chitosan Concentration on Morphology and Conductivity of Lithium Aluminium Titanate Phosphate for Solid Electrolytes of Lithium-Ion Battery Application, IOP Conf. Ser.: Mater. Sci. Eng., 2019, 509, 012021, DOI:10.1088/1757-899X/509/1/012021.
- S. Habibie, M. Hamzah, M. Anggaravidya and E. Kalembang, The Effect of Chitosan on Physical and Mechanical Properties of Paper, J. Chem. Eng. Mater. Sci., 2016, 7(1), 1–10, DOI:10.5897/JCEMS2015.0235.
- Y. Xu, Y. Li, Q. Chen, L. Fu, L. Tao and Y. Wei, Injectable and Self-Healing Chitosan Hydrogel Based on Imine Bonds: Design and Therapeutic Applications, Int. J. Mol. Sci., 2018, 19(8), 2198, DOI:10.3390/ijms19082198.
- Y. Wang, L. Tong, Y. Zheng, S. Pang, J. Sha, L. Li and G. Zhao, Hydrogels with Self-Healing Ability, Excellent Mechanical Properties and Biocompatibility Prepared from Oxidized Gum Arabic, Eur. Polym. J., 2019, 117, 363–371, DOI:10.1016/J.EURPOLYMJ.2019.05.033.
- A. H. Pandit, N. Mazumdar, K. Imtiyaz, M. M. Alam Rizvi and S. Ahmad, Self-Healing and Injectable Hydrogels for Anticancer Drug Delivery: A Study with Multialdehyde Gum Arabic and Succinic Anhydride Chitosan, ACS Appl. Bio Mater., 2020, 3(12), 8460–8470, DOI:10.1021/acsabm.0c00835.
- Y. Wu, L. Yuan, N. A. Sheng, Z. Q. Gu, W. H. Feng, H. Y. Yin, Y. Morsi and X. M. Mo, A Soft Tissue Adhesive Based on Aldehyde-Sodium Alginate and Amino-Carboxymethyl Chitosan Preparation through the Schiff Reaction, Front. Mater. Sci., 2017, 11(3), 215–222, DOI:10.1007/s11706-017-0392-x.
- W. Xu, K. Liu, T. Li, W. Zhang, Y. Dong, J. Lv, W. Wang, J. Sun, M. Li, M. Wang, Z. Zhao and Y. Liang, An in Situ Hydrogel Based on Carboxymethyl Chitosan and Sodium Alginate Dialdehyde for Corneal Wound Healing after Alkali Burn, J. Biomed. Mater. Res., Part A, 2019, 107(4), 742–754, DOI:10.1002/jbm.a.36589.
- Z. Naghizadeh, A. Karkhaneh and A. Khojasteh, Self-Crosslinking Effect of Chitosan and Gelatin on Alginate Based Hydrogels: Injectable in Situ Forming Scaffolds, Mater. Sci. Eng., C, 2018, 89, 256–264, DOI:10.1016/J.MSEC.2018.04.018.
- F. Z. Yuan, H. F. Wang, J. Guan, J. N. Fu, M. Yang, J. Y. Zhang, Y. R. Chen, X. Wang and J. K. Yu, Fabrication of Injectable Chitosan-Chondroitin Sulfate Hydrogel Embedding Kartogenin-Loaded Microspheres as an Ultrasound-Triggered Drug Delivery System for Cartilage Tissue Engineering, Pharmaceutics, 2021, 13(9), 1487, DOI:10.3390/pharmaceutics13091487.
- S. Lü, C. Gao, X. Xu, X. Bai, H. Duan, N. Gao, C. Feng, Y. Xiong and M. Liu, Injectable and Self-Healing Carbohydrate-Based Hydrogel for Cell Encapsulation, ACS Appl. Mater. Interfaces, 2015, 7(23), 13029–13037, DOI:10.1021/acsami.5b03143.
- S. Wu, Z. Zhang, R. Xu, S. Wei, F. Xiong, W. Cui, B. Li, Y. Xue, H. Xuan and H. Yuan, A Spray-Filming, Tissue-Adhesive, and Bioactive Polysaccharide Self-Healing Hydrogel for Skin Regeneration, Mater. Des., 2022, 217, 110669, DOI:10.1016/J.MATDES.2022.110669.
- L. Nie, Q. Wei, M. Sun, P. Ding, L. Wang, Y. Sun, X. Ding, O. V. Okoro, G. Jiang and A. Shavandi, Injectable, Self-Healing, Transparent, and Antibacterial Hydrogels Based on Chitosan and Dextran for Wound Dressings, Int. J. Biol. Macromol., 2023, 233, 123494, DOI:10.1016/j.ijbiomac.2023.123494.
- B. Balakrishnan, D. Soman, U. Payanam, A. Laurent, D. Labarre and A. Jayakrishnan, A Novel Injectable Tissue Adhesive Based on Oxidized Dextran and Chitosan, Acta Biomater., 2017, 53, 343–354, DOI:10.1016/J.ACTBIO.2017.01.065.
- G. Liu, Z. Shi, T. Kuriger, L. R. Hanton, J. Simpson, S. C. Moratti, B. H. Robinson, T. Athanasiadis, R. Valentine, P. J. Wormald and S. Robinson, Synthesis and Characterization of Chitosan/Dextran-Based Hydrogels for Surgical Use, Macromol. Symp., 2009, 279(1), 151–157, DOI:10.1002/masy.200950523.
- Z. Zhou, X. Zhang, L. Xu, H. Lu, Y. Chen, C. Wu and P. Hu, A Self-Healing Hydrogel Based on Crosslinked Hyaluronic Acid and Chitosan to Facilitate Diabetic Wound Healing, Int. J. Biol. Macromol., 2022, 220, 326–336, DOI:10.1016/J.IJBIOMAC.2022.08.076.
- H. Weng, W. Jia, M. Li and Z. Chen, New Injectable Chitosan-Hyaluronic Acid Based Hydrogels for Hemostasis and Wound Healing, Carbohydr. Polym., 2022, 294, 119767, DOI:10.1016/J.CARBPOL.2022.119767.
- S. Wang, J. Chi, Z. Jiang, H. Hu, C. Yang, W. Liu and B. Han, A Self-Healing and Injectable Hydrogel Based on Water-Soluble Chitosan and Hyaluronic Acid for Vitreous Substitute, Carbohydr. Polym., 2021, 256, 117519, DOI:10.1016/J.CARBPOL.2020.117519.
- S. Maiz-Fernández, L. Pérez-Álvarez, U. Silván, J. L. Vilas-Vilela and S. Lanceros-Méndez, Dynamic and Self-Healable Chitosan/Hyaluronic Acid-Based In Situ-Forming Hydrogels, Gels, 2022, 8(8), 477, DOI:10.3390/gels8080477.
- M. Chanmontri, A. E. Swilem, A. L. Mutch, L. Grøndahl and O. Suwantong, Physicochemical and in Vitro Biological Evaluation of an Injectable Self-Healing Quaternized Chitosan/Oxidized Pectin Hydrogel for Potential Use as a Wound Dressing Material, Int. J. Biol. Macromol., 2023, 242, 124984, DOI:10.1016/J.IJBIOMAC.2023.124984.
- A. A. Bazghaleh, M. A. Dogolsar and J. Barzin, Preparation and Characterization of Oxidized Pectin/N-Succinyl Chitosan/Graphene Oxide Hydrogels, Cellulose, 2023, 30(4), 2165–2179, DOI:10.1007/s10570-022-05015-5.
- D. Q. Li, S. Y. Wang, Y. J. Meng, J. F. Li and J. Li, An Injectable, Self-Healing Hydrogel System from Oxidized Pectin/Chitosan/γ-Fe2O3, Int. J. Biol. Macromol., 2020, 164, 4566–4574, DOI:10.1016/J.IJBIOMAC.2020.09.072.
- A. A. Bazghaleh, M. A. Dogolsar and J. Barzin, Development of an Injectable Self-Healing Hydrogel Based on N-Succinyl Chitosan/ Oxidized Pectin for Biomedical Applications, J. Polym. Res., 2022, 29(5), 165, DOI:10.1007/s10965-022-02983-x.
- Y. Zhang, L. Tao, S. Li and Y. Wei, Synthesis of Multiresponsive and Dynamic Chitosan-Based Hydrogels for Controlled Release of Bioactive Molecules, Biomacromolecules, 2011, 12(8), 2894–2901, DOI:10.1021/bm200423f.
- X. Zhang, B. Tan, Y. Wu, M. Zhang, X. Xie and J. Liao, An Injectable, Self-Healing Carboxymethylated Chitosan Hydrogel with Mild Photothermal Stimulation for Wound Healing, Carbohydr. Polym., 2022, 293, 119722, DOI:10.1016/J.CARBPOL.2022.119722.
- I. Natalie, J. Balitaan, W.-J. Luo, Y.-W. Su, C.-Y. Yu, T.-Y. Wu, C.-A. Chang, H.-W. Jia, S.-R. Lin, C.-D. Hsiao and J.-M. Yeh, Healing Wounds Efficiently with Biomimetic Soft Matter: Injectable Self-Healing Neutral Glycol Chitosan/Dibenzaldehyde-Terminated Poly(Ethylene Glycol) Hydrogel with Inherent Antibacterial Properties, ACS Appl. Bio Mater., 2023, 6(2), 552–565, DOI:10.1021/acsabm.2c00859.
- L. Cao, B. Cao, C. Lu, G. Wang, L. Yu and J. Ding, An Injectable Hydrogel Formed by in Situ Cross-Linking of Glycol Chitosan and Multi-Benzaldehyde Functionalized PEG Analogues for Cartilage Tissue Engineering, J. Mater. Chem. B, 2015, 3(7), 1268–1280, 10.1039/c4tb01705f.
- A. Hussain Pandit, S. Nisar, K. Imtiyaz, M. Nadeem, N. Mazumdar, M. Moshahid Alam Rizvi and S. Ahmad, Injectable, Self-Healing, and Biocompatible N,O-Carboxymethyl Chitosan/Multialdehyde Guar Gum Hydrogels for Sustained Anticancer Drug Delivery, Biomacromolecules, 2021, 22(9), 3731–3745, DOI:10.1021/acs.biomac.1c00537.
- C. Li, T. Jiang, C. Zhou, A. Jiang, C. Lu, G. Yang, J. Nie, F. Wang, X. Yang and Z. Chen, Injectable Self-Healing Chitosan-Based POSS-PEG Hybrid Hydrogel as Wound Dressing to Promote Diabetic Wound Healing, Carbohydr. Polym., 2023, 299, 120198, DOI:10.1016/J.CARBPOL.2022.120198.
- J. Lu, Y. Chen, M. Ding, X. Fan, J. Hu, Y. Chen, J. Li, Z. Li and W. Liu, A 4arm-PEG Macromolecule Crosslinked Chitosan Hydrogels as Antibacterial Wound Dressing, Carbohydr. Polym., 2022, 277, 118871, DOI:10.1016/J.CARBPOL.2021.118871.
- C. Li, T. Jiang, C. Zhou, A. Jiang, C. Lu, G. Yang, J. Nie, F. Wang, X. Yang and Z. Chen, Injectable Self-Healing Chitosan-Based POSS-PEG Hybrid Hydrogel as Wound Dressing to Promote Diabetic Wound Healing, Carbohydr. Polym., 2023, 299, 120198, DOI:10.1016/J.CARBPOL.2022.120198.
- J. Qu, X. Zhao, P. X. Ma and B. Guo, PH-Responsive Self-Healing Injectable Hydrogel Based on N-Carboxyethyl Chitosan for Hepatocellular Carcinoma Therapy, Acta Biomater., 2017, 58, 168–180, DOI:10.1016/j.actbio.2017.06.001.
- S. Alven and B. A. Aderibigbe, Chitosan and Cellulose-Based Hydrogels for Wound Management, Int. J. Mol. Sci., 2020, 1–30, DOI:10.3390/ijms21249656.
- W. Huang, Y. Wang, Z. Huang, X. Wang, L. Chen, Y. Zhang and L. Zhang, On-Demand Dissolvable Self-Healing Hydrogel Based on Carboxymethyl Chitosan and Cellulose Nanocrystal for Deep Partial Thickness Burn Wound Healing, ACS Appl. Mater. Interfaces, 2018, 10(48), 41076–41088, DOI:10.1021/acsami.8b14526.
- S. Liu, N. Jiang, Y. Chi, Q. Peng, G. Dai, L. Qian, K. Xu, W. Zhong and W. Yue, Injectable and Self-Healing Hydrogel Based on Chitosan-Tannic Acid and Oxidized Hyaluronic Acid for Wound Healing, ACS Biomater. Sci. Eng., 2022, 8(9), 3754–3764, DOI:10.1021/acsbiomaterials.2c00321.
- Shahid-ul-Islam and B. S. Butola, Recent Advances in Chitosan Polysaccharide and Its Derivatives in Antimicrobial Modification of Textile Materials, Int. J. Biol. Macromol., 2019, 905–912, DOI:10.1016/j.ijbiomac.2018.10.102.
- W. Wang, Q. Meng, Q. Li, J. Liu, M. Zhou, Z. Jin and K. Zhao, Chitosan Derivatives and Their Application in Biomedicine, Int. J. Mol. Sci., 2020, 21(2), 487, DOI:10.3390/ijms21020487.
- M. Naveed, L. Phil, M. Sohail, M. Hasnat, M. M. F. A. Baig, A. U. Ihsan, M. Shumzaid, M. U. Kakar, T. Mehmood Khan, M. D. Akabar, M. I. Hussain and Q. G. Zhou, Chitosan Oligosaccharide (COS): An Overview, Int. J. Biol. Macromol., 2019, 129, 827–843, DOI:10.1016/J.IJBIOMAC.2019.01.192.
- X. Lv, Y. Liu, S. Song, C. Tong, X. Shi, Y. Zhao, J. Zhang and M. Hou, Influence of Chitosan Oligosaccharide on the Gelling and Wound Healing Properties of Injectable Hydrogels Based on Carboxymethyl Chitosan/Alginate Polyelectrolyte Complexes, Carbohydr. Polym., 2019, 205, 312–321, DOI:10.1016/J.CARBPOL.2018.10.067.
- H. Jafari, H. Alimoradi, C. Delporte, K. V. Bernaerts, R. Heidari, D. Podstawczyk, S. V. Niknezhad and A. Shavandi, An Injectable, Self-Healing, 3D Printable, Double Network Co-Enzymatically Crosslinked Hydrogel Using Marine Poly- and Oligo-Saccharides for Wound Healing Application, Appl. Mater. Today, 2022, 29, 101581, DOI:10.1016/J.APMT.2022.101581.
- L. Tang, Z. Zhang, S. Lei, J. Zhou, Y. Liu, X. Yu, J. Wang, D. Wan, J. Shi and S. Wang, A Temperature and PH Dual-Responsive Injectable Self-Healing Hydrogel Prepared by Chitosan Oligosaccharide and Aldehyde Hyaluronic Acid for Promoting Diabetic Foot Ulcer Healing, Int. J. Biol. Macromol., 2023, 253, 127213, DOI:10.1016/J.IJBIOMAC.2023.127213.
- C. Muanprasat and V. Chatsudthipong, Chitosan Oligosaccharide: Biological Activities and Potential Therapeutic Applications, Pharmacol. Ther., 2017, 170, 80–97, DOI:10.1016/J.PHARMTHERA.2016.10.013.
- H. Li, M. Li, P. Liu, K. Wang, H. Fang, J. Yin, D. Zhu, Q. Yang, J. Gao, Q. Ke, H. Yu, Y. Guo, Y. Gao and C. Zhang, A Multifunctional Substance P-Conjugated Chitosan Hydrochloride Hydrogel Accelerates Full-Thickness Wound Healing by Enhancing Synchronized Vascularization, Extracellular Matrix Deposition, and Nerve Regeneration, Biomater. Sci., 2021, 9(11), 4199–4210, 10.1039/D1BM00357G.
- A. F. Kotzé, H. L. Lueßen, A. G. De Boer, J. C. Verhoef and H. E. Junginger, Chitosan for Enhanced Intestinal Permeability: Prospects for Derivatives Soluble in Neutral and Basic Environments, Eur. J. Pharm. Sci., 1999, 7(2), 145–151, DOI:10.1016/S0928-0987(98)00016-5.
- R. Shen, Z. Shao, R. Chen, Q. Wang, Z. Gui, Y. Qi, W. Song, Y. Liu and G. Zheng, Fully Bio-Based Zein/Chitosan Hydrochloride/Phloretin Bimodal Fibrous Membrane for High-Performance and Antibacterial Air Filtration Based on Green Electrospinning, Sep. Purif. Technol., 2024, 341, 126893, DOI:10.1016/J.SEPPUR.2024.126893.
- A. S. Araújo, G. S. D. Lima, I. D. S. Nunes, J. C. R. D. O. F. D. Aguiar, D. M. D. A. F. Navarro, N. F. C. B. Melo, N. S. S. Magalhães, R. França, R. D. S. F. Carvalho and T. C. M. Stamford, Chitosan Hydrochloride-Gum Arabic-Passion Fruit Seed Oil Nanoparticle Edible Coating to Control Fungal Infection and Maintain Quality Parameters of Strawberries, Food Control, 2024, 161, 110360, DOI:10.1016/J.FOODCONT.2024.110360.
- C. Chen, S. Zhang, X. Cheng, Y. Ren, Y. Qian, C. Zhang, M. Chen, N. Sun and H. Liu, Reducing Cherry Rain-Cracking: Enhanced Wetting and Barrier Properties of Chitosan Hydrochloride-Based Coating with Dual Nanoparticles, Int. J. Biol. Macromol., 2024, 268, 131660, DOI:10.1016/J.IJBIOMAC.2024.131660.
- X. Dou, Y. Hao, Y. Sun, P. Yang, L. Liu, Y. He, Y. Shi, C. Yang and F. Chen, A Novel Baking Additive: Preparation, Characterization, and Application of Chitosan Hydrochloride/Carboxymethyl Starch Sodium Nano-Gel for Wheat Bread, Food Hydrocolloids, 2024, 148, 109459, DOI:10.1016/J.FOODHYD.2023.109459.
- H. Tao, X. H. Fang, P. Chen, B. Q. Yang, R. Feng and B. Zhang, Casein/Butyrylated Dextrin Nanoparticles and Chitosan Stabilized Bilayer Emulsions as Fat Substitutes in Sponge Cakes, Food Chem., 2024, 448, 139043, DOI:10.1016/J.FOODCHEM.2024.139043.
- C. K. Kavroumatzi, P. Matziarli, M. Chatzidimopoulos, A. Boutsika, D. I. Tsitsigiannis, E. Paplomatas and A. Zambounis, Control of Peach Leaf Curl with Foliar Applications of Plant Immunity Inducers and Insights in Elicitation of Defense Responses against Taphrina Deformans, J. Fungi, 2024, 10(5), 325, DOI:10.3390/jof10050325.
- S. Francesconi, B. Steiner, H. Buerstmayr, M. Lemmens, M. Sulyok and G. M. Balestra, Chitosan Hydrochloride Decreases Fusarium Graminearum Growth and Virulence and Boosts Growth, Development and Systemic Acquired Resistance in Two Durum Wheat Genotypes, Molecules, 2020, 25(20), 4752, DOI:10.3390/molecules25204752.
- M. Straccia, G. d’Ayala, I. Romano, A. Oliva and P. Laurienzo, Alginate Hydrogels Coated with Chitosan for Wound Dressing, Mar. Drugs, 2015, 13(5), 2890–2908, DOI:10.3390/md13052890.
- S. M. Makau, M. Moumni, L. Landi, D. Pirozzi, F. Sannino and G. Romanazzi, In Vitro Evaluation of Chitosan Hydrochloride and COS (Chito-Oligosaccharides)-OGA (Oligo-Galacturonides) on Phytopathogenic Fungi and Escherichia Coli, Horticulturae, 2023, 9(12), 1275, DOI:10.3390/horticulturae9121275.
- T. Zhou, H. Zhou, F. Wang, P. Zhang, J. Shang and L. Shi, An Injectable Carboxymethyl Chitosan Hydrogel Scaffold Formed via Coordination Bond for Antibacterial and Osteogenesis in Osteomyelitis, Carbohydr. Polym., 2024, 324, 121466, DOI:10.1016/J.CARBPOL.2023.121466.
- J. Cao, P. Wu, Q. Cheng, C. He, Y. Chen and J. Zhou, Ultrafast Fabrication of Self-Healing and Injectable Carboxymethyl Chitosan Hydrogel Dressing for Wound Healing, ACS Appl. Mater. Interfaces, 2021, 13(20), 24095–24105, DOI:10.1021/acsami.1c02089.
- L. Shi, P. Ding, Y. Wang, Y. Zhang, D. Ossipov and J. Hilborn, Self-Healing Polymeric Hydrogel Formed by Metal–Ligand Coordination Assembly: Design, Fabrication, and Biomedical Applications, Macromol. Rapid Commun., 2019, 40(7), e1800837, DOI:10.1002/marc.201800837.
- M. Pandian, V. Selvaprithviraj, A. Pradeep and J. Rangasamy, In-Situ Silver Nanoparticles Incorporated N, O-Carboxymethyl Chitosan Based Adhesive, Self-Healing, Conductive, Antibacterial and Anti-Biofilm Hydrogel, Int. J. Biol. Macromol., 2021, 188, 501–511, DOI:10.1016/J.IJBIOMAC.2021.08.040.
- J. Yang, Y. Chen, L. Zhao, Z. Feng, K. Peng, A. Wei, Y. Wang, Z. Tong and B. Cheng, Preparation of a Chitosan/Carboxymethyl Chitosan/AgNPs Polyelectrolyte Composite Physical Hydrogel with Self-Healing Ability, Antibacterial Properties, and Good Biosafety Simultaneously, and Its Application as a Wound Dressing, Composites, Part B, 2020, 197, 108139, DOI:10.1016/J.COMPOSITESB.2020.108139.
- Z. Shariatinia, Carboxymethyl Chitosan: Properties and Biomedical Applications, Int. J. Biol. Macromol., 2018, 120, 1406–1419, DOI:10.1016/J.IJBIOMAC.2018.09.131.
- J. Cao, G. He, X. Ning, X. Chen, L. Fan, M. Yang, Y. Yin and W. Cai, Preparation and Properties of O-Chitosan Quaternary Ammonium Salt/Polyvinyl Alcohol/Graphene Oxide Dual Self-Healing Hydrogel, Carbohydr. Polym., 2022, 287, 119318, DOI:10.1016/J.CARBPOL.2022.119318.
- H. Cui, B. Cui, H. Chen, X. Geng, X. Geng, Z. Li, S. Cao, J. Shen and J. Li, A Chitosan-Based Self-Healing Hydrogel for Accelerating Infected Wound Healing, Biomater. Sci., 2023, 11(12), 4226–4237, 10.1039/D3BM00061C.
- S. Guo, Y. Ren, R. Chang, Y. He, D. Zhang, F. Guan and M. Yao, Injectable Self-Healing Adhesive Chitosan Hydrogel with Antioxidative, Antibacterial, and Hemostatic Activities for Rapid Hemostasis and Skin Wound Healing, ACS Appl. Mater. Interfaces, 2022, 14(30), 34455–34469, DOI:10.1021/acsami.2c08870.
- J. You, S. Xie, J. Cao, H. Ge, M. Xu, L. Zhang and J. Zhou, Quaternized Chitosan/Poly(Acrylic Acid) Polyelectrolyte Complex Hydrogels with Tough, Self-Recovery, and Tunable Mechanical Properties, Macromolecules, 2016, 49(3), 1049–1059, DOI:10.1021/acs.macromol.5b02231.
- S. Devadarshini Sahoo, T. K. Vasudha, V. Muthuvijayan and E. Prasad, Chitosan-Based Self-Healable and Adhesive Hydrogels for Flexible Strain Sensor Application, ACS Appl. Polym. Mater., 2022, 4(12), 9176–9185, DOI:10.1021/acsapm.2c01488.
- M. Yadav, B. Kaushik, G. K. Rao, C. M. Srivastava and D. Vaya, Advances and Challenges in the Use of Chitosan and Its Derivatives in Biomedical Fields: A Review, Carbohydr. Polym. Technol. Appl., 2023, 5, 100323, DOI:10.1016/J.CARPTA.2023.100323.
- F. Luan, L. Wei, J. Zhang, W. Tan, Y. Chen, F. Dong, Q. Li and Z. Guo, Preparation and Characterization of Quaternized Chitosan Derivatives and Assessment of Their Antioxidant Activity, Molecules, 2018, 23(3), 516, DOI:10.3390/molecules23030516.
- W. Guo, X. Gao, X. Ding, P. Ding, Y. Han, Q. Guo, Y. Ma, O. V. Okoro, Y. Sun, G. Jiang, M. Mirzaei, A. Shavandi and L. Nie, Self-Adhesive and Self-Healing Hydrogel Dressings Based on Quaternary Ammonium Chitosan and Host-Guest Interacted Silk Fibroin, Colloids Surf., A, 2024, 684, 133145, DOI:10.1016/J.COLSURFA.2024.133145.
- T. Wang, X. Ren, Y. Bai, L. Liu and G. Wu, Adhesive and Tough Hydrogels Promoted by Quaternary Chitosan for Strain Sensor, Carbohydr. Polym., 2021, 254, 117298, DOI:10.1016/J.CARBPOL.2020.117298.
- S. G. Marapureddy, P. Hivare, S. Kumar, S. Gupta and P. Thareja, Carbamoylated Chitosan Hydrogels with Improved Viscoelastic Properties and Stability for Potential 3D Cell Culture Applications, Biomed. Mater., 2021, 16(4) DOI:10.1088/1748-605X/abf88c.
- R. Gupta, S. Swarupa, C. Mayya, D. Bhatia and P. Thareja, Graphene Oxide–Carbamoylated Chitosan Hydrogels with Tunable Mechanical Properties for Biological Applications, ACS Appl. Bio Mater., 2023, 6(2), 578–590, DOI:10.1021/acsabm.2c00885.
- Y. Zhong, F. Seidi, Y. Wang, L. Zheng, Y. Jin and H. Xiao, Injectable Chitosan Hydrogels Tailored with Antibacterial and Antioxidant Dual Functions for Regenerative Wound Healing, Carbohydr. Polym., 2022, 298, 120103, DOI:10.1016/j.carbpol.2022.120103.
- H. Li, F. Cheng, X. Wei, X. Yi, S. Tang, Z. Wang, Y. S. Zhang, J. He and Y. Huang, Injectable, Self-Healing, Antibacterial, and Hemostatic N,O-Carboxymethyl Chitosan/Oxidized Chondroitin Sulfate Composite Hydrogel for Wound Dressing, Mater. Sci. Eng., C, 2021, 118, 111324, DOI:10.1016/J.MSEC.2020.111324.
- N. A. Sirajuddin, M. S. M. Jamil and M. A. S. M. Lazim, Effect of Cross-Link Density and the Healing Efficiency of Self-Healing Poly(2-Hydroxyethyl Methacrylate) Hydrogel, e-Polym., 2014, 14(4), 289–294, DOI:10.1515/epoly-2014-0036.
- Z. Wei and S. Gerecht, A Self-Healing Hydrogel as an Injectable Instructive Carrier for Cellular Morphogenesis, Biomaterials, 2018, 185, 86–96, DOI:10.1016/j.biomaterials.2018.09.003.
- J. Wei, F. Wan, P. Zhang, Z. Zeng, H. Ping, J. Xie, Z. Zou, W. Wang, H. Xie, Z. Shen, L. Lei and Z. Fu, Bioprocess-Inspired Synthesis of Printable, Self-Healing Mineral Hydrogels for Rapidly Responsive, Wearable Ionic Skin, Chem. Eng. J., 2021, 424, 130549, DOI:10.1016/j.cej.2021.130549.
- P. Sun, S. Ren, F. Liu, A. Wu, N. Sun, L. Shi and L. Zheng, Smart Low Molecular Weight Hydrogels with Dynamic Covalent Skeletons, Soft Matter, 2018, 14(32), 6678–6683, 10.1039/c8sm01482e.
-
Amplitude sweeps: Anton paar wiki
.
-
Determining the Linear Viscoelastic Region in Oscillatory Measurements
.
- Y. Zhong, F. Seidi, Y. Wang, L. Zheng, Y. Jin and H. Xiao, Injectable Chitosan Hydrogels Tailored with Antibacterial and Antioxidant Dual Functions for Regenerative Wound Healing, Carbohydr. Polym., 2022, 298, 120103, DOI:10.1016/j.carbpol.2022.120103.
- Y. Yang, G. Feng, J. Wang, R. Zhang, S. Zhong, J. Wang and X. Cui, Injectable Chitosan-Based Self-Healing Supramolecular Hydrogels with Temperature and PH Dual-Responsivenesses, Int. J. Biol. Macromol., 2023, 227, 1038–1047, DOI:10.1016/J.IJBIOMAC.2022.11.279.
- A. M. Craciun, S. Morariu and L. Marin, Self-Healing Chitosan Hydrogels: Preparation and Rheological Characterization, Polymers, 2022, 14(13), 2570, DOI:10.3390/polym14132570.
- H. Herrada-Manchón, M. A. Fernández and E. Aguilar, Essential Guide to Hydrogel Rheology in Extrusion 3D Printing: How to Measure It and Why It Matters?, Gels, 2023, 9(7), 517, DOI:10.3390/gels9070517.
- J. Lu, X. Fan, J. Hu, J. Li, J. Rong, W. Wang, Y. Chen, W. Liu, J. Chen and Y. Chen, Construction and Function of Robust and Moist Bilayer Chitosan-Based Hydrogel Wound Dressing, Mater. Des., 2023, 226, 111604, DOI:10.1016/J.MATDES.2023.111604.
- S. M. Samani, F. Ahmadi, Z. Oveisi and Z. Amoozgar, Chitosan Based Hydrogels: Characteristics and Pharmaceutical Applications, Res. Pharm. Sci., 2015, 10, 1–16 Search PubMed.
- L. Ma, W. Su, Y. Ran, X. Ma, Z. Yi, G. Chen, X. Chen, Z. Deng, Q. Tong, X. Wang and X. Li, Synthesis and Characterization of Injectable Self-Healing Hydrogels Based on Oxidized Alginate-Hybrid-Hydroxyapatite Nanoparticles and Carboxymethyl Chitosan, Int. J. Biol. Macromol., 2020, 165, 1164–1174, DOI:10.1016/J.IJBIOMAC.2020.10.004.
- G. Fletes-Vargas, H. Espinosa-Andrews, J. M. Cervantes-Uc, I. Limón-Rocha, G. Luna-Bárcenas, M. Vázquez-Lepe, N. Morales-Hernández, J. A. Jiménez-Ávalos, D. G. Mejía-Torres, P. Ramos-Martínez and R. Rodríguez-Rodríguez, Porous Chitosan Hydrogels Produced by Physical Crosslinking: Physicochemical, Structural, and Cytotoxic Properties, Polymers, 2023, 15(9), 2203, DOI:10.3390/polym15092203.
- E. Khor and L. Y. Lim, Implantable Applications of Chitin and Chitosan, Biomaterials, 2003, 24(13), 2339–2349, DOI:10.1016/S0142-9612(03)00026-7.
- F. Azadikhah and A. R. Karimi, Injectable Photosensitizing Supramolecular Hydrogels: A Robust Physically Cross-Linked System Based on Polyvinyl Alcohol/Chitosan/Tannic Acid with Self-Healing and Antioxidant Properties, React. Funct. Polym., 2022, 173, 105212, DOI:10.1016/J.REACTFUNCTPOLYM.2022.105212.
- P. Deng, L. Yao, J. Chen, Z. Tang and J. Zhou, Chitosan-Based Hydrogels with Injectable, Self-Healing and Antibacterial Properties for Wound Healing, Carbohydr. Polym., 2022, 276, 118718, DOI:10.1016/J.CARBPOL.2021.118718.
- F. Zhang, S. Zhang, R. Lin, S. Cui, X. Jing and S. Coseri, High Mechanical and Self-Healing Carboxymethyl Chitosan-Hyaluronic Acid Hybrid Hydrogel via Multiple Dynamic Covalent Bonds for Drug Delivery, Eur. Polym. J., 2023, 197, 112342 CrossRef CAS.
- J. Chen, B. L. B. Nichols, A. M. Norris, C. E. Frazier and K. J. Edgar, All-Polysaccharide, Self-Healing Injectable Hydrogels Based on Chitosan and Oxidized Hydroxypropyl Polysaccharides, Biomacromolecules, 2020, 21(10), 4261–4272, DOI:10.1021/acs.biomac.0c01046.
- Y.-T. Lan, Q.-P. Cheng, J. Xu, S.-H. Lin, J.-M. Lin and S. Hsu, Gelation and the Self-Healing Behavior of the Chitosan–Catechol Hydrogel, Polymers, 2022, 14(21), 4614, DOI:10.3390/polym14214614.
- M. H. Mahaninia, Z. Wang, A. Rajabi-Abhari and N. Yan, Self-Healing, Flame-Retardant, and Antimicrobial Chitosan-Based Dynamic Covalent Hydrogels, Int. J. Biol. Macromol., 2023, 252, 126422, DOI:10.1016/J.IJBIOMAC.2023.126422.
- S. Maiz-Fernández, N. Barroso, L. Pérez-Álvarez, U. Silván, J. L. Vilas-Vilela and S. Lanceros-Mendez, 3D Printable Self-Healing Hyaluronic Acid/Chitosan Polycomplex Hydrogels with Drug Release Capability, Int. J. Biol. Macromol., 2021, 188, 820–832, DOI:10.1016/J.IJBIOMAC.2021.08.022.
- T. G. Papaioannou, D. Manolesou, E. Dimakakos, G. Tsoucalas, M. Vavuranakis and D. Tousoulis, 3D Bioprinting Methods and Techniques: Applications on Artificial Blood Vessel Fabrication, Acta Cardiol. Sin., 2019, 35(3), 284–289, DOI:10.6515/ACS.201905_35(3).20181115A.
- S. Vanaei, M. S. Parizi, S. Vanaei, F. Salemizadehparizi and H. R. Vanaei, An Overview on Materials and Techniques in 3D Bioprinting Toward Biomedical Application, Eng. Regen., 2021, 2, 1–18, DOI:10.1016/J.ENGREG.2020.12.001.
- N. Hong, G. H. Yang, J. H. Lee and G. H. Kim, 3D Bioprinting and Its in Vivo Applications, J. Biomed. Mater. Res., Part B, 2018, 444–459, DOI:10.1002/jbm.b.33826.
- R. Chang, J. Nam and W. Sun, Effects of Dispensing Pressure and Nozzle Diameter on Cell Survival from Solid Freeform Fabrication-Based Direct Cell Writing, Tissue Eng., Part A, 2008, 14(1), 41–48, DOI:10.1089/ten.a.2007.0004.
- M. Lazaridou, D. N. Bikiaris and D. A. Lamprou, 3D Bioprinted Chitosan-Based Hydrogel Scaffolds in Tissue Engineering and Localised Drug Delivery, Pharmaceutics, 2022, 14(9), 1978, DOI:10.3390/pharmaceutics14091978.
- M. Taghizadeh, A. Taghizadeh, M. K. Yazdi, P. Zarrintaj, F. J. Stadler, J. D. Ramsey, S. Habibzadeh, S. Hosseini Rad, G. Naderi, M. R. Saeb, M. Mozafari and U. S. Schubert, Chitosan-Based Inks for 3D Printing and Bioprinting, Green Chem., 2022, 62–101, 10.1039/d1gc01799c.
- F. Pati, J. Jang, D. H. Ha, S. Won Kim, J. W. Rhie, J. H. Shim, D. H. Kim and D. W. Cho, Printing Three-Dimensional Tissue Analogues with Decellularized Extracellular Matrix Bioink, Nat. Commun., 2014, 5, 3935, DOI:10.1038/ncomms4935.
- S. Liu, H. Zhang, T. Ahlfeld, D. Kilian, Y. Liu, M. Gelinsky and Q. Hu, Evaluation of Different Crosslinking Methods in Altering the Properties of Extrusion-Printed Chitosan-Based Multi-Material Hydrogel Composites, Bio-Des. Manuf., 2023, 6(2), 150–173, DOI:10.1007/s42242-022-00194-3.
- Q. Liu, N. Ji, L. Xiong and Q. Sun, Rapid Gelling, Self-Healing, and Fluorescence-Responsive Chitosan Hydrogels Formed by Dynamic Covalent Crosslinking, Carbohydr. Polym., 2020, 246, 116586, DOI:10.1016/J.CARBPOL.2020.116586.
- C. M. Valmikinathan, V. J. Mukhatyar, A. Jain, L. Karumbaiah, M. Dasari and R. V. Bellamkonda, Photocrosslinkable Chitosan Based Hydrogels for Neural Tissue Engineering, Soft Matter, 2012, 8(6), 1964–1976, 10.1039/c1sm06629c.
- Y. Liu, C. W. Wong, S. W. Chang and S. H. Hsu, An Injectable, Self-Healing Phenol-Functionalized Chitosan Hydrogel with Fast Gelling Property and Visible Light-Crosslinking Capability for 3D Printing, Acta Biomater., 2021, 122, 211–219, DOI:10.1016/J.ACTBIO.2020.12.051.
- T. Fischetti, N. Celikkin, N. Contessi Negrini, S. Farè and W. Swieszkowski, Tripolyphosphate-Crosslinked Chitosan/Gelatin Biocomposite Ink for 3D Printing of Uniaxial Scaffolds, Front. Bioeng. Biotechnol., 2020, 8, 400, DOI:10.3389/fbioe.2020.00400.
- S. G. Marapureddy and P. Thareja, Synergistic Effect of Chemical Crosslinking and Addition of Graphene-Oxide in Chitosan—Hydrogels, Films, and Drug Delivery, Mater. Today Commun., 2022, 31, 103430, DOI:10.1016/J.MTCOMM.2022.103430.
- M. Rajabi, M. McConnell, J. Cabral and M. A. Ali, Chitosan Hydrogels in 3D Printing for Biomedical Applications, Carbohydr. Polym., 2021, 260, 117768 CrossRef CAS PubMed.
- M. Taghizadeh, A. Taghizadeh, M. K. Yazdi, P. Zarrintaj, F. J. Stadler, J. D. Ramsey, S. Habibzadeh, S. H. Rad, G. Naderi and M. R. Saeb, Chitosan-Based Inks for 3D Printing and Bioprinting, Green Chem., 2022, 24(1), 62–101 RSC.
- C. Intini, L. Elviri, J. Cabral, S. Mros, C. Bergonzi, A. Bianchera, L. Flammini, P. Govoni, E. Barocelli and R. Bettini, 3D-Printed Chitosan-Based Scaffolds: An in Vitro Study of Human Skin Cell Growth and an in-Vivo Wound Healing Evaluation in Experimental Diabetes in Rats, Carbohydr. Polym., 2018, 199, 593–602 CrossRef CAS PubMed.
- P. Maturavongsadit, L. K. Narayanan, P. Chansoria, R. Shirwaiker and S. R. Benhabbour, Cell-Laden Nanocellulose/Chitosan-Based Bioinks for 3D Bioprinting and Enhanced Osteogenic Cell Differentiation, ACS Appl. Bio Mater., 2021, 4(3), 2342–2353 CrossRef CAS PubMed.
- F. Hafezi, S. Shorter, A. G. Tabriz, A. Hurt, V. Elmes, J. Boateng and D. Douroumis, Bioprinting and Preliminary Testing of Highly Reproducible Novel Bioink for Potential Skin Regeneration, Pharmaceutics, 2020, 12(6), 550 CrossRef CAS PubMed.
- P. Li, L. Fu, Z. Liao, Y. Peng, C. Ning, C. Gao, D. Zhang, X. Sui, Y. Lin and S. Liu, Chitosan Hydrogel/3D-Printed Poly(E-caprolactone) Hybrid Scaffold Containing Synovial Mesenchymal Stem Cells for Cartilage Regeneration Based on Tetrahedral Framework Nucleic Acid Recruitment, Biomaterials, 2021, 278, 121131 CrossRef CAS PubMed.
- S. Pisani, R. Dorati, F. Scocozza, C. Mariotti, E. Chiesa, G. Bruni, I. Genta, F. Auricchio, M. Conti and B. Conti, Preliminary Investigation on a New Natural Based Poly(Gamma-glutamic Acid)/Chitosan Bioink, J. Biomed. Mater. Res., Part B, 2020, 108(7), 2718–2732 CrossRef CAS PubMed.
- M. Rahimnejad, A. Adoungotchodo, N. R. Demarquette and S. Lerouge, FRESH Bioprinting of Biodegradable Chitosan Thermosensitive Hydrogels, Bioprinting, 2022, 27, e00209 CrossRef.
- P. M. S. Ouro, D. C. S. Costa, A. J. R. Amaral and J. F. Mano, A Supramolecular Injectable Methacryloyl Chitosan-Tricine-Based Hydrogel with 3D Printing Potential for Tissue Engineering Applications, Macromol. Biosci., 2024, 24(1), 2300058 CrossRef CAS PubMed.
- I. Koumentakou, M. J. Noordam, A. Michopoulou, Z. Terzopoulou and D. N. Bikiaris, 3D-Printed Chitosan-Based Hydrogels Loaded with Levofloxacin for Tissue Engineering Applications, Biomacromolecules, 2023, 24(9), 4019–4032 CrossRef CAS PubMed.
- Y. Yang, Z. Wang, Y. Xu, J. Xia, Z. Xu, S. Zhu and M. Jin, Preparation of Chitosan/Recombinant Human Collagen-Based Photo-Responsive Bioinks for 3d Bioprinting, Gels, 2022, 8(5), 314 CrossRef CAS PubMed.
- M. A. Gwak, S. J. Lee, D. Lee, S. A. Park and W. H. Park, Highly Gallol-Substituted, Rapidly Self-Crosslinkable, and Robust Chitosan Hydrogel for 3D Bioprinting, Int. J. Biol. Macromol., 2023, 227, 493–504 CrossRef CAS PubMed.
- H. Hamedi, S. Moradi, S. M. Hudson and A. E. Tonelli, Chitosan Based Hydrogels and Their Applications for Drug Delivery in Wound Dressings: A Review, Carbohydr. Polym., 2018, 445–460, DOI:10.1016/j.carbpol.2018.06.114.
- E. Wolska and M. Szymańska, Comparison of the In Vitro Drug Release Methods for the Selection of Test Conditions to Characterize Solid Lipid Microparticles, Pharmaceutics, 2023, 15(2), 511, DOI:10.3390/pharmaceutics15020511.
- Y. Herdiana, N. Wathoni, S. Shamsuddin and M. Muchtaridi, Drug Release Study of the Chitosan-Based Nanoparticles, Heliyon, 2021, 8(1), e08674, DOI:10.1016/j.heliyon.2021.e08674.
- O. Guaresti, C. García–Astrain, T. Palomares, A. Alonso–Varona, A. Eceiza and N. Gabilondo, Synthesis and Characterization of a Biocompatible Chitosan–Based Hydrogel Cross–Linked via ‘Click’Chemistry for Controlled Drug Release, Int. J. Biol. Macromol., 2017, 102, 1–9 CrossRef CAS PubMed.
- N. Bhattarai, J. Gunn and M. Zhang, Chitosan-Based Hydrogels for Controlled, Localized Drug Delivery, Adv. Drug Delivery Rev., 2010, 62(1), 83–99 CrossRef CAS PubMed.
- P. Li, H. Zong, G. Li, Z. Shi, X. Yu, K. Zhang, P. Xia, S. Yan and J. Yin, Building a Poly(Amino Acid)/Chitosan-Based Self-Healing Hydrogel via Host–Guest Interaction for Cartilage Regeneration, ACS Biomater. Sci. Eng., 2023, 9(8), 4855–4866, DOI:10.1021/acsbiomaterials.2c01547.
- L. Cao, B. Cao, C. Lu, G. Wang, L. Yu and J. Ding, An Injectable Hydrogel Formed by in Situ Cross-Linking of Glycol Chitosan and Multi-Benzaldehyde Functionalized PEG Analogues for Cartilage Tissue Engineering, J. Mater. Chem. B, 2015, 3(7), 1268–1280, 10.1039/c4tb01705f.
- J. Shen, R. Chang, L. Chang, Y. Wang, K. Deng, D. Wang and J. Qin, Light Emitting CMC-CHO Based Self-Healing Hydrogel with Injectability for in Vivo Wound Repairing Applications, Carbohydr. Polym., 2022, 281, 119052, DOI:10.1016/J.CARBPOL.2021.119052.
- T. Kean and M. Thanou, Biodegradation, Biodistribution and Toxicity of Chitosan, Adv. Drug Delivery Rev., 2010, 62(1), 3–11 CrossRef CAS PubMed.
- F. Su, Y. Wang, X. Liu, X. Shen, X. Zhang, Q. Xing, L. Wang and Y. Chen, Biocompatibility and in Vivo Degradation of Chitosan Based Hydrogels as Potential Drug Carrier, J. Biomater. Sci., Polym. Ed., 2018, 29(13), 1515–1528 CrossRef CAS PubMed.
- L. Xia, S. Wang, Z. Jiang, J. Chi, S. Yu, H. Li, Y. Zhang, L. Li, C. Zhou and W. Liu, Hemostatic Performance of Chitosan-Based Hydrogel and Its Study on Biodistribution and Biodegradability in Rats, Carbohydr. Polym., 2021, 264, 117965 CrossRef CAS PubMed.
- V. Pertici, C. Pin-Barre, C. Rivera, C. Pellegrino, J. Laurin, D. Gigmes and T. Trimaille, Degradable and Injectable Hydrogel for Drug Delivery in Soft Tissues, Biomacromolecules, 2018, 20(1), 149–163 CrossRef PubMed.
-
T. L. Riss; R. A. Moravec and A. L. Niles, Cell Viability Assays, 2013 Search PubMed.
- B. Tyliszczak, A. Drabczyk, S. Kudłacik-Kramarczyk, K. Bialik-Wąs and A. Sobczak-Kupiec, In Vitro Cytotoxicity of Hydrogels Based on Chitosan and Modified with Gold Nanoparticles, J. Polym. Res., 2017, 24(10), 153, DOI:10.1007/s10965-017-1315-3.
-
C. P. Segeritz and L. Vallier, Cell Culture: Growing Cells as Model Systems In Vitro, Basic Science Methods for Clinical Researchers, Elsevier Inc., 2017, pp. 151–172 DOI:10.1016/B978-0-12-803077-6.00009-6.
- S. G. Marapureddy, P. Hivare, A. Sharma, J. Chakraborty, S. Ghosh, S. Gupta and P. Thareja, Rheology and Direct Write Printing of Chitosan - Graphene Oxide Nanocomposite Hydrogels for Differentiation of Neuroblastoma Cells, Carbohydr. Polym., 2021, 269, 118254, DOI:10.1016/J.CARBPOL.2021.118254.
- U. Modi, D. Kedaria and R. Vasita, Differential Migration and Proliferation Potential of the Hydrogel Aided 3D Tumoroid, Macromol. Biosci., 2022, 22(11), 2200196 CrossRef CAS PubMed.
- J. S. Kwon, G. H. Kim, S. M. Yoon, H. W. Seo, J. H. Kim, B. H. Min and M. S. Kim, Chitosan-Based Hydrogels to Induce Neuronal Differentiation of Rat Muscle-Derived Stem Cells, Int. J. Biol. Macromol., 2012, 51(5), 974–979 CrossRef CAS PubMed.
- R. Gupta, S. Swarupa, C. Mayya, D. Bhatia and P. Thareja, Graphene Oxide–Carbamoylated Chitosan Hydrogels with Tunable Mechanical Properties for Biological Applications, ACS Appl. Bio Mater., 2023, 6(2), 578–590 CrossRef CAS PubMed.
- I.-H. Liu, S.-H. Chang and H.-Y. Lin, Chitosan-Based Hydrogel Tissue Scaffolds Made by 3D Plotting Promotes Osteoblast Proliferation and Mineralization, Biomed. Mater., 2015, 10(3), 035004 CrossRef PubMed.
- C. M. Valmikinathan, V. J. Mukhatyar, A. Jain, L. Karumbaiah, M. Dasari and R. V. Bellamkonda, Photocrosslinkable Chitosan Based Hydrogels for Neural Tissue Engineering, Soft Matter, 2012, 8(6), 1964–1976 RSC.
- Y. Hao, W. Zhao, H. Zhang, W. Zheng and Q. Zhou, Carboxymethyl Chitosan-Based Hydrogels Containing Fibroblast Growth Factors for Triggering Diabetic Wound Healing, Carbohydr. Polym., 2022, 287, 119336 CrossRef CAS PubMed.
- T. Debnath, S. Ghosh, U. S. Potlapuvu, L. Kona, S. R. Kamaraju, S. Sarkar, S. Gaddam and L. K. Chelluri, Proliferation and Differentiation Potential of Human Adipose-Derived Stem Cells Grown on Chitosan Hydrogel, PLoS One, 2015, 10(3), e0120803 CrossRef PubMed.
- P. Urbán, N. J. Liptrott and S. Bremer, Overview of the Blood Compatibility of Nanomedicines: A Trend Analysis of in Vitro and in Vivo Studies, Wiley Interdiscip. Rev.: Nanomed. Nanobiotechnol., 2019, 11(3), e1546 Search PubMed.
- H. Y. Zhou, Y. P. Zhang, W. F. Zhang and X. G. Chen, Biocompatibility and Characteristics of Injectable Chitosan-Based Thermosensitive Hydrogel for Drug Delivery, Carbohydr. Polym., 2011, 83(4), 1643–1651 CrossRef CAS.
- S. Li, S. Dong, W. Xu, S. Tu, L. Yan, C. Zhao, J. Ding and X. Chen, Antibacterial Hydrogels, Adv. Sci., 2018, 5, 1700527, DOI:10.1002/advs.201700527.
- W. Han, C. Chen, K. Yang, H. Wang, H. Xia, Y. Zhao, Y. Teng, G. Feng and Y. M. Chen, Hyaluronic Acid and Chitosan-Based Injectable and Self-Healing Hydrogel with Inherent Antibacterial and Antioxidant Bioactivities, Int. J. Biol. Macromol., 2023, 227, 373–383, DOI:10.1016/J.IJBIOMAC.2022.12.037.
- X. Wang, R. Song, M. Johnson, A. Sigen, Z. He, I. Lara-Sáez, Q. Xu, W. Wang, C. Milne and X. Wang, An Injectable Chitosan-Based Self-Healable Hydrogel System as an Antibacterial Wound Dressing, Materials, 2021, 14(20), 5956, DOI:10.3390/ma14205956.
- Y. Li, Y. Han, H. Li, X. Niu, D. Zhang and K. Wang, Antimicrobial Hydrogels: Potential Materials for Medical Application, Small, 2024, 20(5), 2304047 CrossRef CAS PubMed.
- Y. Wang, Z. Wang, W. Lu and Y. Hu, Review on Chitosan-Based Antibacterial Hydrogels: Preparation, Mechanisms, and Applications, Int. J. Biol. Macromol., 2023, 128080 Search PubMed.
- D. Yan, Y. Li, Y. Liu, N. Li, X. Zhang and C. Yan, Antimicrobial Properties of Chitosan and Chitosan Derivatives in the Treatment of Enteric Infections, Molecules, 2021, 26(23), 7136 CrossRef CAS PubMed.
- Y. Ou and M. Tian, Advances in Multifunctional Chitosan-Based Self-Healing Hydrogels for Biomedical Applications, J. Mater. Chem. B, 2021, 9(38), 7955–7971 RSC.
- P. Deng, L. Yao, J. Chen, Z. Tang and J. Zhou, Chitosan-Based Hydrogels with Injectable, Self-Healing and Antibacterial Properties for Wound Healing, Carbohydr. Polym., 2022, 276, 118718 CrossRef CAS PubMed.
- B. Farasati Far, M. R. Naimi-Jamal, M. Jahanbakhshi, A. Hadizadeh, S. Dehghan and S. Hadizadeh, Enhanced Antibacterial Activity of Porous Chitosan-Based Hydrogels Crosslinked with Gelatin and Metal Ions, Sci. Rep., 2024, 14(1), 7505 CrossRef CAS PubMed.
- B. Guo, J. Qu, X. Zhao and M. Zhang, Degradable Conductive Self-Healing Hydrogels Based on Dextran-Graft-Tetraaniline and N-Carboxyethyl Chitosan as Injectable Carriers for Myoblast Cell Therapy and Muscle Regeneration, Acta Biomater., 2019, 84, 180–193, DOI:10.1016/j.actbio.2018.12.008.
- X. Lin, J. Lv, D. Wang and K. Liu, Injectable Adhesive Carboxymethyl Chitosan-Based Hydrogels with Self-Mending and Antimicrobial Features for the Potential Management of Periodontal Diseases, RSC Adv., 2023, 13(18), 11903–11911, 10.1039/d3ra00904a.
- ’ P. Deng, F. Chen, H. Zhang, Y. Chen and J. Zhou, Multifunctional Double-Layer Composite Hydrogel Conduit Based on Chitosan for Peripheral Nerve Repairing, Adv. Healthcare Mater., 2022, 11(13), e2200115 CrossRef PubMed.
- M. Chen, H. Tan, W. Xu, Z. Wang, J. Zhang, S. Li, T. Zhou, J. li and X. Niu, A Self-Healing, Magnetic and Injectable Biopolymer Hydrogel Generated by Dual Cross-Linking for Drug Delivery and Bone Repair, Acta Biomater., 2022, 153, 159–177, DOI:10.1016/J.ACTBIO.2022.09.036.
- S. Guo, Y. Ren, R. Chang, Y. He, D. Zhang, F. Guan and M. Yao, Injectable Self-Healing Adhesive Chitosan Hydrogel with Antioxidative, Antibacterial, and Hemostatic Activities for Rapid Hemostasis and Skin Wound Healing, ACS Appl. Mater. Interfaces, 2022, 14(30), 34455–34469, DOI:10.1021/acsami.2c08870.
- Z. Zhu, K. Zhang, Y. Xian, G. He, Z. Pan, H. Wang, C. Zhang and D. Wu, A Choline Phosphoryl-Conjugated Chitosan/Oxidized Dextran Injectable Self-Healing Hydrogel for Improved Hemostatic Efficacy, Biomacromolecules, 2022, 24(2), 690–703, DOI:10.1021/acs.biomac.2c01143.
- B. Kong, R. Liu, Y. Cheng, X. Cai, J. Liu, D. Zhang, H. Tan and Y. Zhao, Natural Biopolymers Derived Hydrogels with Injectable, Self-Healing, and Tissue Adhesive Abilities for Wound Healing, Nano Res., 2023, 16(2), 2798–2807, DOI:10.1007/s12274-022-4936-8.
- R. Wang, L. Liu, X. He, Z. Xia, Z. Zhao, Z. Xi, J. Yu and J. Wang, Dynamic Crosslinked Injectable Mussel-Inspired Hydrogels with Adhesive, Self-Healing, and Biodegradation Properties, Polymers, 2023, 15(8), 1876, DOI:10.3390/polym15081876.
- Z. Wei, J. Zhao, Y. M. Chen, P. Zhang and Q. Zhang, Self-Healing Polysaccharide-Based Hydrogels as Injectable Carriers for Neural Stem Cells, Sci. Rep., 2016, 6, 37841, DOI:10.1038/srep37841.
- F. Jiang, Z. Tang, Y. Zhang, Y. Ju, H. Gao, N. Sun, F. Liu, P. Gu and W. Zhang, Enhanced Proliferation and Differentiation of Retinal Progenitor Cells through a Self-Healing Injectable Hydrogel, Biomater. Sci., 2019, 7(6), 2335–2347, 10.1039/c8bm01579a.
- R. Dong, X. Zhao, B. Guo and P. X. Ma, Self-Healing Conductive Injectable Hydrogels with Antibacterial Activity as Cell Delivery Carrier for Cardiac Cell Therapy, ACS Appl. Mater. Interfaces, 2016, 8(27), 17138–17150, DOI:10.1021/acsami.6b04911.
- H. Wang, J. Shi, Y. Wang, Y. Yin, L. Wang, J. Liu, Z. Liu, C. Duan, P. Zhu and C. Wang, Promotion of Cardiac Differentiation of Brown Adipose Derived Stem Cells by Chitosan Hydrogel for Repair after Myocardial Infarction, Biomaterials, 2014, 35(13), 3986–3998, DOI:10.1016/J.BIOMATERIALS.2014.01.021.
- A. Hasan, A. Khattab, M. A. Islam, K. A. Hweij, J. Zeitouny, R. Waters, M. Sayegh, M. M. Hossain and A. Paul, Injectable Hydrogels for Cardiac Tissue Repair after Myocardial Infarction, Adv. Sci., 2015, 2(11), 1500122, DOI:10.1002/advs.201500122.
- J. Tao and Y. Zhang, Injectable Chitosan-Based Thermosensitive Hydrogel/Nanoparticle-Loaded System for Local Delivery of Vancomycin in the Treatment of Osteomyelitis, Int. J. Nanomed., 2020, 15, 5855–5871 CrossRef CAS PubMed.
- A. Moeini, P. Pedram, P. Makvandi, M. Malinconico and G. G. d’Ayala, Wound Healing and Antimicrobial Effect of Active Secondary Metabolites in Chitosan-Based Wound Dressings: A Review, Carbohydr. Polym., 2020, 233, 115839 CrossRef CAS PubMed.
- S. Alven and B. A. Aderibigbe, Chitosan and Cellulose-Based Hydrogels for Wound Management, Int. J. Mol. Sci., 2020, 21(24), 9656 CrossRef CAS PubMed.
- G. FrykbergRobert, Challenges in the Treatment of Chronic Wounds, Adv. Wound Care, 2015, 4(9), 560–582 CrossRef CAS PubMed.
- Y.-H. Lee, Y.-L. Hong and T.-L. Wu, Novel Silver and Nanoparticle-Encapsulated Growth Factor Co-Loaded Chitosan Composite Hydrogel with Sustained Antimicrobility and Promoted Biological Properties for Diabetic Wound Healing, Mater. Sci. Eng., C, 2021, 118, 111385 CrossRef CAS PubMed.
- K. Raziyeva, Y. Kim, Z. Zharkinbekov, K. Kassymbek, S. Jimi and A. Saparov, Immunology of Acute and Chronic Wound Healing, Biomolecules, 2021, 11(5), 700 CrossRef CAS PubMed.
- Y. Zhang, Y. Wang, L. Chen, J. Zheng, X. Fan, X. Xu, G. Zhou, N. Ullah and X. Feng, An Injectable Antibacterial Chitosan-Based Cryogel with High Absorbency and Rapid Shape Recovery for Noncompressible Hemorrhage and Wound Healing, Biomaterials, 2022, 285, 121546 CrossRef CAS PubMed.
- S. Guo, Y. Ren, R. Chang, Y. He, D. Zhang, F. Guan and M. Yao, Injectable Self-Healing Adhesive Chitosan Hydrogel with Antioxidative, Antibacterial, and Hemostatic Activities for Rapid Hemostasis and Skin Wound Healing, ACS Appl. Mater. Interfaces, 2022, 14(30), 34455–34469 CrossRef CAS PubMed.
- P. J. Franks, J. Barker, M. Collier, G. Gethin, E. Haesler, A. Jawien, S. Laeuchli, G. Mosti, S. Probst and C. Weller, Management of Patients with Venous Leg Ulcers: Challenges and Current Best Practice, J. Wound Care, 2016, 25(Sup6), S1–S67 CrossRef PubMed.
- K. C. Maita, F. R. Avila, R. A. Torres-Guzman, J. P. Garcia, A. S. Eldaly, L. Palmieri, O. S. Emam, O. Ho and A. J. Forte, Local Anti-Inflammatory Effect and Immunomodulatory Activity of Chitosan-Based Dressing in Skin Wound Healing: A Systematic Review, J. Clin. Transl. Res., 2022, 8(6), 488 CAS.
- J. H. Maeng, B. W. Bang, E. Lee, J. Kim, H. G. Kim, D. H. Lee and S.-G. Yang, Endoscopic Application of EGF-Chitosan Hydrogel for Precipitated Healing of GI Peptic Ulcers and Mucosectomy-Induced Ulcers, J. Mater. Sci.: Mater. Med., 2014, 25, 573–582 CrossRef CAS PubMed.
- M. Hafezi, S. Nouri Khorasani, M. Zare, R. Esmaeely Neisiany and P. Davoodi, Advanced Hydrogels for Cartilage Tissue Engineering: Recent Progress and Future Directions, Polymers, 2021, 13(23), 4199 CrossRef CAS PubMed.
- L. Djekic, M. Martinović, A. Ćirić and J. Fraj, Composite Chitosan Hydrogels as Advanced Wound Dressings with Sustained Ibuprofen Release and Suitable Application Characteristics, Pharm. Dev. Technol., 2020, 25(3), 332–339 CrossRef CAS PubMed.
- W. Shu, Y. Wang, X. Zhang, C. Li, H. Le and F. Chang, Functional Hydrogel Dressings for Treatment of Burn Wounds, Front. Bioeng. Biotechnol., 2021, 9, 788461 CrossRef PubMed.
- J. J. He, C. McCarthy and G. Camci-Unal, Development of Hydrogel-based Sprayable Wound Dressings for Second-and Third-degree Burns, Adv. NanoBiomed Res., 2021, 1(6), 2100004 CrossRef CAS.
- C. Yang, Y. Chen, H. Huang, S. Fan, C. Yang, L. Wang, W. Li, W. Niu and J. Liao, ROS-Eliminating Carboxymethyl Chitosan Hydrogel to Enhance Burn Wound-Healing Efficacy, Front. Pharmacol., 2021, 12, 679580 CrossRef CAS PubMed.
- Rectal and vaginal drug delivery.
-
W. Nafo, Hydrogel Biomaterials for Drug Delivery: Mechanisms, Design, and Drugs, Intech Open, 2022 Search PubMed.
-
S. M. Samani; F. Ahmadi; Z. Oveisi and Z. Amoozgar, Chitosan Based Hydrogels: Characteristics and Pharmaceutical Applications, 2015, vol. 10 Search PubMed.
- E. S. Ko, C. Kim, Y. Choi and K. Y. Lee, 3D Printing of Self-Healing Ferrogel Prepared from Glycol Chitosan, Oxidized Hyaluronate, and Iron Oxide Nanoparticles, Carbohydr. Polym., 2020, 245, 116496, DOI:10.1016/J.CARBPOL.2020.116496.
- X. Chen, M. Fan, H. Tan, B. Ren, G. Yuan, Y. Jia, J. Li, D. Xiong, X. Xing, X. Niu and X. Hu, Magnetic and Self-Healing Chitosan-Alginate Hydrogel Encapsulated Gelatin Microspheres via Covalent Cross-Linking for Drug Delivery, Mater. Sci. Eng., C, 2019, 101, 619–629, DOI:10.1016/J.MSEC.2019.04.012.
- A. Chandrasekharan, Y. J. Hwang, K. Y. Seong, S. Park, S. Kim and S. Y. Yang, Acid-Treated Water-Soluble Chitosan Suitable for Microneedle-Assisted Intracutaneous Drug Delivery, Pharmaceutics, 2019, 11(5), 209, DOI:10.3390/pharmaceutics11050209.
- S. Gorantla, N. Dabholkar, S. Sharma, V. K. Rapalli, A. Alexander and G. Singhvi, Chitosan-Based Microneedles as a Potential Platform for Drug Delivery through the Skin: Trends and Regulatory Aspects, Int. J. Biol. Macromol., 2021, 184, 438–453, DOI:10.1016/J.IJBIOMAC.2021.06.059.
- Y. L. Chiu, S. C. Chen, C. J. Su, C. W. Hsiao, Y. M. Chen, H. L. Chen and H. W. Sung, PH-Triggered Injectable Hydrogels Prepared from Aqueous N-Palmitoyl Chitosan: In Vitro Characteristics and in Vivo Biocompatibility, Biomaterials, 2009, 30(28), 4877–4888, DOI:10.1016/J.BIOMATERIALS.2009.05.052.
- M. Guvendiren, H. D. Lu and J. A. Burdick, Shear-Thinning Hydrogels for Biomedical Applications, Soft Matter, 2012, 260–272, 10.1039/c1sm06513k.
- W. Qiu, H. Han, M. Li, N. Li, Q. Wang, X. Qin, X. Wang, J. Yu, Y. zhou, Y. Li, F. Li and D. Wu, Nanofibers Reinforced Injectable Hydrogel with Self-Healing, Antibacterial, and Hemostatic Properties for Chronic Wound Healing, J. Colloid Interface Sci., 2021, 596, 312–323, DOI:10.1016/J.JCIS.2021.02.107.
|
This journal is © The Royal Society of Chemistry 2024 |