DOI:
10.1039/D4MA00075G
(Review Article)
Mater. Adv., 2024,
5, 4112-4130
From antigen uptake to immune modulation: the multifaceted potential of peptide nanofibers as vaccine nanocarriers
Received
25th January 2024
, Accepted 16th April 2024
First published on 17th April 2024
Abstract
Spanning from the mitigation of pathogenic diseases via protective immunity to the provision of therapeutic solutions for other debilitating ailments, immune-based interventions have showcased promising and significant outcomes. Peptide nanofibers constructed from self-assembled biocompatible peptide chains have garnered considerable attention. Evaluation of the peptide nanofibers’ capabilities has revealed their aptitude to enhance antigen uptake by antigen-presenting cells (APCs), such as dendritic cells (DCs), and their potential to manifest immune adjuvant-like effects. These results suggest that peptide nanofibers could amplify the efficacy of vaccines administered through diversified approaches and potentially obviate the necessity of co-administering antigens and immune adjuvants to APCs. This review highlights the potential utility of peptide nanofibers as an approach to augment immune responses, with the potential for the effective and safe enhancement of vaccine potency. Furthermore, the opportunistic application of emerging peptide nanofibers to enhance the therapeutic outcomes of recently uncovered immune modulators is also deliberated upon.
1. Introduction
Cancer is recognised as a predominant cause of mortality and poses a significant challenge to enhancement of life expectancy globally.1 In 2020, approximately 19.3 million novel cancer diagnoses and nearly 10.0 million cancer-related fatalities were reported worldwide.2 Neoplasms linked with socio-economic transitions exert considerable strain on the healthcare systems and economic frameworks, particularly in lower to middle income nations.3 Conventional therapeutic approaches, such as radiotherapy and chemotherapy, possess inherent constraints.4–7 For instance, chemotherapy inflicts DNA damage upon cancer cells, impeding their ability to proliferate. However, it also has the potential to harm normal cellular DNA and disrupt cell division, leading to significant health hazards.8 This underscores the necessity of investigating innovative therapeutic strategies to optimise patient prognosis and counteract the expansive global impact of cancer.
The pivotal functions of immune cells stem from their inherent ability to swiftly identify and eliminate a diverse array of threats encompassing pathogenic and neoplastic agent-affected cells.9 In this context, APCs, specifically DCs, emerge as central orchestrators in instigating the commencement of immune reactions.10
Serving as professional APCs, DCs bridge the innate and adaptive immune responses.10 Pattern recognition receptors (PRRs) are a set of receptors that are expressed by APCs such as DCs and play a crucial role in the antigen recognition process.11 PRRs’ interactions with pathogen-associated molecular patterns carried by invading pathogens initiate the process of DC stimulation and maturation.12 Similarly, the immune response can be triggered by PRR signalling mediated via the recognition of damage-associated molecular patterns released by damaged cells or stressed cells.13 In addition, PRR signalling promotes the expression of DCs’ antigen uptake receptors that enable antigen internalisation for subsequent processing and presentation. Hence, the presence of PRR-stimulating molecules in vaccine formulations is crucial for antigen recognition and immune response induction.
Once a tumour cell is pinpointed, APCs engulf and process it, presenting antigens to other immune cells such as CD8+ T lymphocytes (Fig. 1).14 Subsequently, CD8+ T cells transform into specialised cytotoxic T lymphocytes (CTLs) that are capable of selectively eliminating cancer cells. Stimulated CTLs possess the ability to undertake comprehensive surveillance of the entire body to identify cells expressing specific tumour antigens, exclusively targeting cancer cells for annihilation while preserving the integrity of normal cells. The profound safety margin exhibited by this approach, coupled with its efficacy in eliminating both localised and metastasised tumours, has spurred a growing interest by leveraging the body's immune process against cancerous cells. Nonetheless, tumour progression coincides with the emergence of immune suppressive mechanisms that pose substantial impediments to immune cell activity.15 As a result, imperative strategies aimed at bolstering the antitumour immune response have become indispensable to surmount the compromised immune milieu and effectuate the eradication of established neoplastic growths.
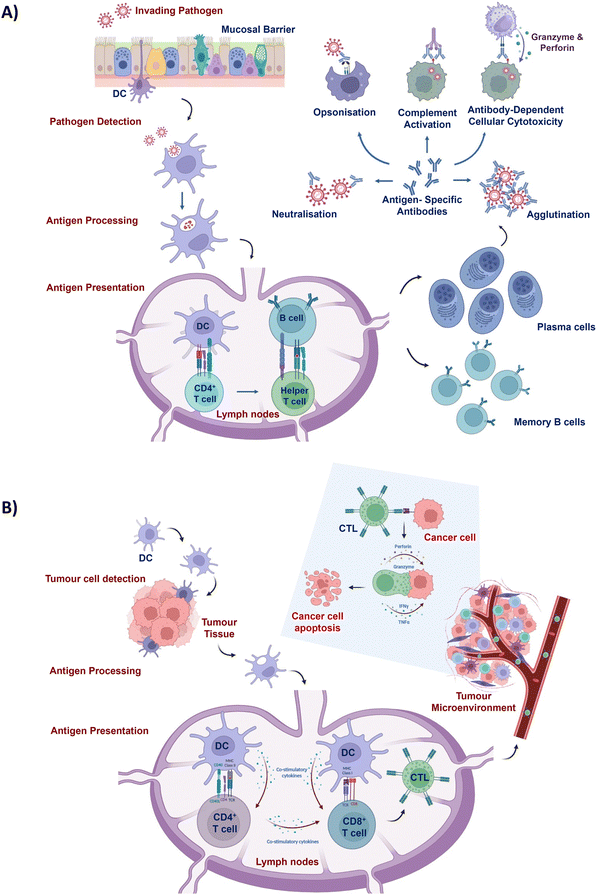 |
| Fig. 1 DC-mediated immune responses. (A) DC-mediated pathogen detection and B cell activation. Following invading pathogen detection and consequent antigen presentation by APCs such as DCs, CD4+ T cells undergo differentiation into CD4+ T-helper cells that provide essential stimulatory signals to B cells. Activated B cells differentiate into plasma cells and memory cells that immediately execute humoral adaptive immune response and provide long-term protection, respectively. Plasma cells produce antigen-specific antibodies that enhance immunogenicity and detection by immune cells. These antibodies neutralise pathogens, enhance phagocytosis by macrophages, and activate the complement system that disrupts pathogen integrity. (B) DC-mediated antitumour immune response induction. DCs actively survey the local environment and engulf the detected tumour cells to present their antigens through the major histocompatibility complex class (MHC) I and II to CD8+ and CD4+ T cells, respectively. Upon activation, CD4+ T cells further stimulate CD8+ T cells that differentiate into tumour-specific cytotoxic T lymphocytes (CTL). Once tumour cells are detected, the stimulated CTLs induce tumour cell killing as part of the antitumour immune response. | |
Immunologically mediated mechanisms hold the potential to confer therapeutic benefits in dealing with proliferating tumour tissue, as well as to establish a defensive barrier against impending infectious agents16,17 (Fig. 1). To amplify the immune response of prophylactic vaccine formulations, incorporation of natural or synthetic immune adjuvants is imperative.18 The effective elicitation of safeguarding immunity against pathogens, such as the emergent severe acute respiratory syndrome coronavirus (SARS-CoV), necessitates the administration of prophylactic vaccines in a profoundly immunogenic state.19 Moreover, the feasibility of administering additional vaccine booster doses is circumscribed by concerns over heightened reactogenicity and inflammatory reactions associated with the administered vaccines.20 Accordingly, there is a call for alternative strategies to augment prophylactic immune responses against infectious diseases.
Multiple research endeavours have explored the application of peptide nanofibers as carriers for nano-vaccines, encompassing a spectrum of investigations involving model antigens, antigens sourced from infectious agents, antigens derived from tumours, and/or immune adjuvants.21–26 Concomitantly, in-depth scrutiny has been dedicated to the examination of physicochemical attributes inherent to these nanofibers, including surface charge and mechanisms underpinning immune enhancement. Previous studies have ascertained the pronounced capacity of nanofibers to notably amplify the uptake of antigens by APCs.27,28 Moreover, these studies have unveiled the potential of nanofibers to assume the role of immune adjuvants upon interaction with APCs. This could offer the prospect of potentially diminishing the necessity for concurrent administration of supplementary immune adjuvants alongside the antigen, while upholding the desired degree of immune response potency.
In the present review, we undertake a critical evaluation of the capabilities exhibited by peptide nanofibers in their role as nanocarriers for vaccines. Furthermore, we carried out a comprehensive assessment of peptide nanofibers’ latent potential to catalyse transformative advancements in the domain of nanovaccinology. This exploration is approached through a multifaceted lens, encompassing innovative inclusion of immune modulators and the exploration of alternate administration routes.
2. Peptide nanofibers
Nanoscale materials have been used in crafting both preventive and remedial vaccine formulations.29,30 Depending on the constituent materials and the methods of synthesis, nanomaterials can manifest as diverse nanostructures, such as nanospheres, nanorods, nanotubes, and nanosheets.31–41 Among these, peptide nanofibers have emerged as prominent nanoarchitectures that arise from the spontaneous arrangement of peptide chains into secondary structures such as α-helical structures, peptide micelles or β-sheets, forming distinct patterns.42,43 The design and evaluation of self-assembled peptide nanofibers have been the subject of prior reviews.44,45 Peptide nanofibers constitute inherently self-assembled nanostructures that leverage the innate capacity of their molecular constituents to engage in spontaneous cohesion through intermolecular interactions (Fig. 2).
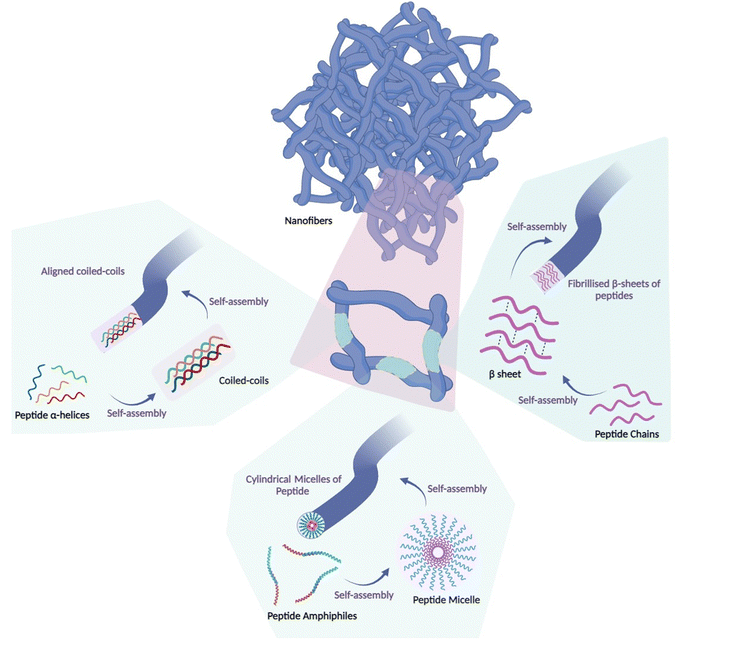 |
| Fig. 2 Peptide nanofiber structures. Peptide nanofibers are self-assembled nanoscale materials formed from spontaneous arrangement of α-helices, peptide micelles or β-sheets into a well-defined fibril pattern, driven by intermolecular interactions such as hydrogen bonding. Peptide chains adopting a secondary α-helical structure depend on the creation of intramolecular hydrogen bonds connecting the carbonyl oxygen and amide hydrogen functional groups along the peptide backbone. Coiled-coils of peptides established by intermolecular hydrogen bonds between neighboring α-helices possess the ability to self-assemble into nanofibers via parallel orientation and sequential stacking. The key step in β-sheet formation is the interaction between peptide chains that align side by side, with the amide and carbonyl groups of adjacent strands forming intermolecular hydrogen bonds. This structural conformation results in highly ordered, stable nanofibers with various potential applications. Peptide amphiphiles are molecular constructs consisting of a hydrophilic peptide sequence tethered to a hydrophobic chain. This structural arrangement provides them with the capacity to self-orient into spherical micelles when placed in aqueous solutions. This self-assembled structure is maintained by intermolecular hydrogen bonding interactions among neighboring amino acids. The resulting micelles serve as nucleation sites for the additional self-assembly of micelles, culminating in the development of a fibrous pattern characterised by dimensions on the order of nanometers in diameter and micrometers in length. | |
2.1. Structures
2.1.1. Coiled-coils.
Peptide chains forming a secondary α-helical structure rely on the formation of intramolecular hydrogen bonds within the peptide backbone to maintain the α-helical configuration.46,47 Driven by an interplay of factors, including amino acid sequence and concentration as well as the applied temperature and pH, α-helical peptides can self-assemble into nanofibers.46 The structural integrity of the self-assembled peptide chains is preserved via the establishment of intermolecular hydrogen bonds between the adjacent chains. These self-assembled helical structures can be described as chains aligned in parallel and stacked atop one another, ultimately forming the nanofiber structure. Altering the pH and temperature of the self-assembly conditions or the amino acid sequence can induce variations in the intensity of intermolecular hydrogen bonding that can impact the structure and stability of the resulting nanofiber structures.48–50 Several additional factors exert significant influence over the self-assembly process. Varying the length of the α-helical peptide chains can adjust the equilibrium between intermolecular and intramolecular interactions, thereby fundamentally impacting the self-assembly dynamics.51 Moreover, the presence of high ionic strength in the assembly conditions can limit the orderly arrangement and peptide nanofiber formation via the induction of electrostatic interactions among the peptide chains.52 The solvent employed also plays a pivotal role by modifying the peptide chains’ hydrophobic interaction, hence affecting the shape of the resulting structures.53 These factors can significantly impact the complex interplay of molecular forces that govern the self-assembly processes and the nanoscale structure formation.
2.1.2. Cylindrical micelles.
Peptide amphiphiles consist of a hydrophilic peptide sequence linked to a hydrophobic chain capable of spontaneous assembly into supramolecular self-assorted nanostructures such as spherical micelles.54 In aqueous conditions, the genesis of peptide micelles arises from the hydrophobic effect, which facilitates the aggregation of the hydrophobic tails at the core site, leaving the hydrophilic peptide sequences exposed to the surrounding aqueous solvent.55 Subsequently, the transition of these spherical micelles into nanofibers is steered by intermolecular hydrogen bonding among the amino acids. The formed micelles undergo self-organisation and serve as nuclei that allow the growth and extension of nanofibers measuring nanometers in diameter and several micrometers in length.56,57 The size and length of the resulting nanofibers can be influenced by the intensity of the intermolecular hydrogen bonding among the adjacent spherical micelles.56,57 In addition, the peptide amphiphiles’ self-assembly process can be controlled by other variables such as the contained amino acid sequence, hydrophobic domain size, hydrophilicity of incorporated spacers, applied temperature, and solvent polarity.58,59 These factors can dramatically affect the formation as well as the properties of the micelle-based peptide nanofibers.
2.1.3. Fibrillised β-sheets.
Peptide β-sheets are morphologically characterised by the presence of peptide chains arranged either parallelly or antiparallelly that are structurally stabilised by hydrogen bonding between the amino acid residues.60–62 With the aid of intermolecular hydrogen bonds, spontaneous alignment and aggregation of these β-sheet structures form the foundation for the construction of fibrillised peptide chains.60,61,63 Additionally, hydrophobic interactions exert their influence by facilitating the cohesion of nonpolar segments within peptides, thereby contributing to the overall process of self-assembly.64 The equilibrium between hydrogen bonding and hydrophobic interactions is instrumental in maintaining the structures of the fibrillised β-sheets and nanofibers. The assembly of self-assembling peptides into these fibrillised β-sheets and nanofibers can be highly impacted by several critical factors such as the contained amino acid sequence, solvent polarity, pH, temperature and added salt concentration.62,65 The amino acid sequence can alter the peptide chains’ tendency to form β-sheets. The applied temperature can further modulate the assembly kinetics that shape the self-assorted structures. Furthermore, the used salt concentration can also alter the peptide solubility and the subsequent ability to self-aggregate. Additionally, the pH of the self-assembly conditions can exert a substantial impact by altering the amino acids’ degree of ionisation and, consequently, the molecular interactions. These variables have the potential to modulate the mode and strength of the interchain interactions, thereby orchestrating the distinctive attributes of the resultant peptide nanofibers. To this end, solvent conditions act as a critical determinant, either enhancing or reducing peptide chain interactions that constitute the driving force of the self-assembly process.
2.2. Influence of peptide nanofibers’ properties on immune modulation
Understanding the influence of peptide nanofibers’ properties on immune modulation is crucial for optimising vaccine design and immune-based therapies. In this section, we provide a comprehensive exploration of the multifaceted relationship between the peptide nanofibers’ morphological and physicochemical properties and their impact on immune modulation.
2.2.1. Morphological properties.
The effects of peptide chains’ morphological features on the ensuing immune response were previously assessed. Fries et al. studied the impact of the structural characteristics of nanofiber-forming peptides on the magnitude of the evoked immune response, using the fibre-forming peptide Coil29.66 The results showed that mice subjected to immunisation with short Coil29, featuring terminal hydrophilic residue capping and OVA257–264 linkage, demonstrated a heightened CD8+ T cell response in comparison to the long uncapped variant. Furthermore, the longer nanofiber exhibited a more efficacious internalisation by APCs in vitro.
Previous studies had also shed light on the influence of the self-assembly process on the peptide carrier's immune modulation capabilities. Rudra et al. showed that mice subjected to subcutaneous immunisation using free OVA323–339 or OVA323–339 linked to nanofibers comprised of the β-sheet-forming SGSG-Q11 peptide combined with CFA manifested a comparable and robust OVA-specific IgG response that endured for a span of 52 weeks.67 Furthermore, parallel in vivo investigations revealed commensurate CD4+ T cell proliferation. Notably, OVA323–339-specific IgG detection witnessed a reduction in T cell receptor-knockout mice, implying an imperative role of CD4+ T cells in its initiation. On the other hand, mice immunised with the OVA323–339-loaded non-fibril-forming P3-Q11 peptide showed a reduced IgG response in contrast to those receiving the OVA323–339-incorporated fibril-forming Q11 peptide, underscoring the dependence of immune response elicitation on the formation of peptide β-sheet secondary structure. Remarkably, mice subjected to immunisation with OVA323–339 covalently tethered to KFE8 (an alternate nanofiber) exhibited an elevated magnitude of specific IgG responses compared to those receiving separate injections of unlinked OVA323–339 and KFE8.
In a more advanced study, Wu et al. assessed the inherent immunogenicity of two distinct self-assembled peptide nano-carriers using the loaded OVA as a model antigen.68 In the conducted comparative studies, α-helix-based nanofibers (Coil29) induced higher levels of OVA-specific IgG in murine subjects than β-sheet-based (Q11) nanofibers. The study highlighted that Coil29 nanofibers were more readily internalised by DCs from immunised mice, than Q11 nanofibers. Moreover, Coil29 nanofibers were more effective in the costimulatory molecules CD80 and CD86 expression upregulation on DCs as well as antibody response induction. It was suggested that the T cell epitope inherently contained in the Coil29 nanofibers’ structure contributed to their observed increased immunogenicity. These findings propose a promising strategy for enhancing the immune responses by incorporating specific T cell assistance within the self-assembling units of fibrillar peptide materials.
These studies set the stage for in-depth mechanistic investigations. Exploring how altered nanofiber characteristics impact cellular internalisation, endosomal escape, and antigen presentation could provide crucial insights into the immune response mechanisms, enabling more efficient vaccine designs. A thorough analysis of these studies underscores the prospective merits conferred to vaccine formulations through the integration of peptide nanofibers as adept cargo carriers.
2.2.2. Physicochemical properties.
Understanding the effect of peptide nanofibers’ physicochemical properties on immune response elicitation has sparked considerable attention. Wen et al. explored the immune responses triggered by OVA323–339 complexed with β sheet-forming Q11 peptide-based nanofibers that were characterised by diverse charges.69 The induction of immune response via subcutaneous immunisation of mice using positively charged nanofibers-OVA323–339 resulted in elevated levels of OVA-specific IgG, surpassing those observed with negatively charged nanofibers-OVA323–339. Correspondingly, the peak levels of IFN-γ and IL-4 were recorded within ex vivo stimulated lymphocytes from mice treated with positively charged nanofibers-OVA323–339. Additionally, the uptake of positively charged nanofibers-OVA323–339 by APCs was superior, as evidenced by both in vitro and in vivo assessments.
In a separate study, the immunogenic potential of nanofibers constructed using the self-assembled positively charged Nap-GDFDFDYDK (YK) peptide or the negatively charged Nap-GDFDFDYDE (YE) peptide was investigated by Yang et al.70 Mice subcutaneously injected with nanofibers exhibited an increase in OVA-specific IgG levels. Notably the YK-conjugated OVA induced a more pronounced IgG response than YE-OVA. In vitro assays using FITC-OVA revealed that nanofibers enhanced the internalisation of OVA by DCs, where the uptake of positively charged YK-OVA by DCs surpassed that of negatively charged YE-OVA.
The impact of nanofiber's hydrophobicity on the immune response induced using the β-sheet-forming peptide sequence FVIFLD was also studied.71 The investigation revealed that the in vitro immune response, triggered by a covalently attached OVA257–264 antigen, was more potent when associated with a short rather than long oligo-ethylene glycol chain. The authors suggested in another study that long hydrophilic chains incorporated into the peptide nanofiber structure could limit the hydrophobic interaction with the APCs’ cell membrane.72 These studies investigated the effects of peptide nanofiber components on cellular interactions, aiming to bridge the gaps between materials science and immune modulation.
3. Peptide nanofibers as a vaccine carrier
3.1. Antigen and/or immune adjuvant loading approaches
The inherent characteristics of amino acids in the peptide chain can facilitate covalent bonding such as disulfide bridges or non-covalent interactions via hydrophobic interactions or hydrogen bonding through various means.73–76 For example, non-covalent interactions can be prompted by adjusting the peptide-surrounding factors such as pH or temperature. The structural attributes and physicochemical characteristics inherent to peptide nanofibers are subject to multiple influences, including the amino acid sequence delineating the peptide chains and the specific conditions governing their self-assembly. The distinctive configuration and chemical attributes exhibited by peptide nanofibers facilitate the entrenchment of antigens and/or immune adjuvants in a manner that offers versatility (Fig. 3). This is manifested in the potential for antigens and/or immune adjuvants to be integrated through non-covalent interactions with the molecular domains of the peptide nanofibers. Moreover, the functional moieties inherent in the amino acid constituents that underpin the nanofiber architecture offer the prospect of covalent incorporation of antigenic fragments and/or immune adjuvants. An integral advantage of peptide nanofibers resides in their amenability to accommodate and dispense numerous copies of the antigen and/or immune adjuvant, which could ultimately elevate intracellular concentrations and augment the effectiveness of the resultant immune response.22,77
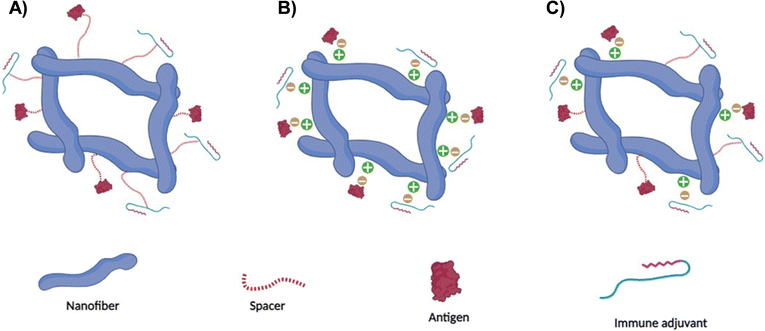 |
| Fig. 3 Antigen and/or immune adjuvant incorporation onto peptide nanofibers. (A) Covalent conjugation. This approach relies on the covalent interaction between the chemical functional groups contained in the peptide chains along with the antigen and/or immune adjuvant. (B) Non-covalent conjugation. This strategy utilises the electrostatic or hydrophobic interaction to facilitate loading of the antigen and/or immune adjuvant onto nanofiber chains. (C) Hybrid binding. The versatile peptide nanofiber surface could be harnessed in the concurrent incorporation of the antigen and/or immune adjuvant via both covalent and non-covalent conjugation methods. | |
Notably, the nanoscale dimensions characterising peptide nanofibers hold the potential to expedite lymphatic drainage, thereby facilitating accessibility to APCs.78 Furthermore, the compositional makeup of peptide nanofibers, which undergo degradation into their constituent amino acids, underscores their inherent biocompatibility.79
3.2. Validation of vaccine deliverability using model antigens
The potential of peptide-based nanofibers as a vaccine carrier, with a focus on model antigens such as the epitope derived from ovalbumin (OVA), has been previously explored (Table 1). Using nanofibers constructed using a fibrillised β-sheet forming peptide composed of the SGSG (spacer)-linked QQKFQFQFEQQ peptide (Q11, fibril forming or assembly domain), Rudra et al. conducted a study in which CD4+ T-cell epitope OVA323–339 was employed as a model antigen.80 Through an in vivo evaluation, it was elucidated that mice administered with nanofiber-OVA323–339via subcutaneous injection demonstrated OVA-specific immunoglobulin G (IgG) levels akin to those exhibited by mice immunised with a blend of OVA323–339 and complete Freund's adjuvant (CFA). Furthermore, mice immunised with nanofiber-OVA323–339 displayed heightened OVA-specific immunoglobulin M (IgM) levels in comparison to their counterparts vaccinated with the OVA323–339 and CFA amalgamation.
Table 1 Model antigens delivered via peptide nanofibers
Nanofiber |
Antigen |
Immune adjuvant |
Ref. |
Abbreviations: OVA, ovalbumin; OVA257–264, class I-restricted peptide epitope of ovalbumin; OVA323–339, class II-restricted peptide epitope of ovalbumin; GFP, green fluorescent protein; CpG, cytidine-phosphate-guanosine-containing oligodeoxynucleotides; SGSG, spacer; Z1, RVQVRVQVRVQV; Q11, QQKFQFQFEQQ. Non-covalently conjugated. Covalently conjugated. |
VVAGKK |
OVAa |
— |
21
|
Lauryl-VVAGKK-biotin |
OVAa |
CpGa |
22
|
SGSG – Z1 |
OVA257–264b |
— |
81
|
Q11 |
GFP or cutinase enzymeb |
— |
76
|
SGSG – Q11 |
OVA323–339b |
— |
80
|
GGAAY – Q11 |
OVA257–264b |
— |
82
|
p-Nitrophenyl phosphonate-Q11 |
GFPb |
— |
83
|
Chen et al. presented findings wherein the impact of OVA323–339 linked to nanofibers formed using fibrillised β-sheet-forming SGSG-Q11 on J774.1 macrophage viability was examined through in vitro incubation at concentrations spanning from 0.007 to 0.7 mg ml−1 over a duration of 4 hours.77 Notably, the administration of nanofiber-OVA323–339 to mice did not elicit localised inflammatory responses, which were conversely observed with alum-adjuvanted OVA323–339. Subsequent to intraperitoneal administration to mice, nanofibers were shown to heighten the uptake of OVA323–339 by DCs and macrophages, as discerned through flow cytometric analysis conducted 20 hours post-administration. Comparative analysis with OVA323–339 alone unveiled that subcutaneous injection of nanofiber-OVA323–339 into mice substantially bolstered the differentiation of CD4+ T cells, evinced by elevated expressions of PD-1 and CXCR5. Additionally, nanofiber-OVA323–339 exhibited a marked escalation in the production of interferon-gamma (IFN-γ) and interleukin-4 (IL-4) upon ex vivo stimulation of lymphocytes. It was also observed that immunisation involving alum-adjuvanted nanofiber-OVA323–339 demonstrated enhanced efficacy in inducing T cell differentiation and cytokine production. Furthermore, immunisation with nanofiber-OVA323–339 resulted in an augmented percentage of differentiated B cells specific to OVA323–339 (GL-7+ and FAS+ cells), along with elevated OVA323–339-specific IgG titers, in comparison to vaccination utilising alum-adjuvanted OVA323–339.77 The non-inflammatory nature of peptide nanofibers could render them a promising candidate not only for prophylactic or therapeutic vaccines but also for immune therapies targeting autoimmune disorders, where excessive inflammation can worsen the symptoms.
In another study, Zhang et al. presented the CD8+ T-cell epitope (OVA257–264) to DCs using peptide nanofibers formulated using the β-sheet forming RVQVRVQVRVQV peptide (Z1)-conjugated SGSG spacer.81 The nanofiber-OVA257–264 demonstrated a discernible augmentation in the presentation of OVA257–264 by DCs to CD8+ T cells in vitro. Murine subcutaneous immunisation with nanofiber-OVA257–264, as well as OVA257–264 alone, yielded commensurate in vivo T-cell proliferation outcomes.
Furthermore, Chesson et al. studied the covalent conjugation of OVA257–264 with Q11-based nanofibers.82 The implementation of nanofiber-OVA257–264 instigated heightened proliferation of OVA-specific CD8+ T cells in mice, as evidenced by flow cytometric analysis of lymphocytes within lymph nodes subsequent to footpad immunisation. This effect was notably superior to the administration of OVA257–264 alone or its co-administration with incomplete Freund's adjuvant. This discernment was substantiated by the observation of mice attaining protection against the infectious OVA-expressing influenza PR8 virus subsequent to vaccination with nanofiber-OVA257–264, in contradistinction to the outcomes achieved by mice subjected to exclusive OVA257–264 vaccination or its combination with incomplete Freund's solution.82
Hudalla et al. achieved successful conjugation of green fluorescent protein (GFP) to β sheet forming p-nitrophenyl phosphonate-linked Q11, leading to the formation of nanofibers that elicited an augmented antibody response targeting GFP within murine subjects.83 Furthermore, the same research team demonstrated the capability of these nanofibers to enhance the production of antibodies specific to GFP or cutinase subsequent to subcutaneous administration in mice.76 The administration of nanofibers physically mixed with GFP yielded a significantly diminished specific antibody response compared to treatment involving nanofiber-bound GFP. Through the utilisation of an alternative amphiphilic peptide sequence, specifically VVAGKK, nanofibers were successfully conjugated with OVA using a biotin–streptavidin interaction mechanism, resulting in the induction of a CD8+ T cell response that was specific to OVA within murine subjects.21
The non-covalent conjugation of OVA and CpG (an immune adjuvant) to lauryl and biotin-modified amphiphilic VVAGKK peptides by Tohumeken et al. unveiled the capacity of CpG-nanofiber-OVA constructs to augment the uptake of CpG by DCs.22 Subsequent outcomes included the elicitation of cytokine production, an upregulated expression of CD40 and CD86 co-stimulatory molecules, and an enhancement in antigen presentation by murine splenocytes during in vitro assessments. Furthermore, administration of the nanofiber construct led to a heightened production of OVA-specific IgG after subcutaneous immunisation with CpG-nanofiber-OVA in mice. Following ex vivo restimulation of splenocytes with OVA after immunisation with CpG-nanofiber-OVA constructs, a notable augmentation in cellular proliferation and an increased production of IFN-γ became evident. Moreover, the CpG-nanofiber-OVA entities exhibited the ability to enhance IFN-γ production within CD8+ T cells upon ex vivo re-stimulation with OVA. The non-covalent attachment of antigens to the nanofiber system could offer modularity and versatility. This flexibility can be advantageous in creating vaccines for various diseases, including emerging infectious diseases, as it enables the rapid development and adaptation of vaccines to changing pathogenic threats.
The research studies presented in this section highlight the potential and versatility of peptide-based nanofibers as potent vectors for antigen delivery and immune modulation. The investigations discussed consistently demonstrated the ability of these nanofiber constructs to effectively enhance the immune responses triggered by various antigenic epitopes. The utilisation of different peptide sequences, such as Q11 and VVAGKK, in conjunction with model antigens including OVA and GFP, revealed the capacity of nanofibers to stimulate robust and targeted antibody and T-cell responses. Moreover, the integration of immune adjuvants such as CpG further amplified the immunogenicity of the nanofiber constructs, facilitating enhanced antigen presentation, cytokine production, and cellular proliferation.
3.3. Applications
3.3.1. Infectious disease vaccines.
The delivery capabilities inherent in peptide nanofibers with respect to antigens derived from infectious agents were previously investigated within more complex and demanding vaccination strategies (Table 2). For example, FLIVIGSIIGPGGDGPGGD (H9e) amphiphile-based nanofibers synthesised from a combination of an elastic segment of spider silk and a trans-membrane segment of the human muscle L-type calcium channel were examined for their capacity to facilitate the delivery of the killed H1N1 swine influenza virus.84 The results showed that mice subjected to immunisation with the nanofiber-H1N1 virus or those administered an oil-based adjuvant-mixed H1N1 virus displayed comparable levels of H1N1-specific IgG1 antibodies.
Table 2 Vaccines against infectious diseases delivered via peptide nanofibers
Nanofiber-forming peptide |
Antigen [conjugation] |
Immune adjuvant |
Ref. |
Abbreviations: H9e, FLIVIGSIIGPGGDGPGGD; KFE8, FKFEFKFE; EAK16-II, AEAEAKAKAEAEAKAK; GGAAY, spacer; Ag85B, mycobacterium tuberculosis-derived CD4+ T cell epitope; E214, Staphylococcus aureus B cell epitope; HSV gB peptide, herpes simplex virus-derived CD8+ T cell epitope; rHBsAg, recombinant hepatitis B virus surface antigen; SL9, HIV-1 CTL epitope; (NANP)3, malaria peptide epitope; R848, resiquimod. Covalently conjugated. Non-covalently conjugated. |
KFE8 |
Ag85Ba |
— |
23
|
Q11 |
HIV envelope-derived-antigen gp120a |
Squalene,b R848b and CpGb |
24
|
SGSG-Q11 |
(NANP)3a |
— |
86
|
SGSG-Q11 |
PADRE (CD4+ T cell epitope)a and E214a |
— |
87
|
SGSG-Q11 |
TNF4-23 (B cell epitope)a and PADRE (CD4+ T cell epitope)a or vaccinia I1L7-21 (CD4+ T cell epitope)a |
— |
89
|
GGAAY-KFE8 |
Ag85Ba |
— |
88
|
H9e |
Killed H1N1 swine influenza virusb |
— |
84
|
H9e |
PRRSVb |
— |
85
|
Nap-GFFY-OMe |
DNA encoding the HIV-1 envelope protein gp 145b |
— |
90
|
GGAAY-KFE8 |
HSV gB peptidea |
CpGb or gardiquimodb |
92
|
(RADA)4 oligopeptide |
rHBsAgb |
CpGb |
73
|
SGSG – EAK16-II |
SL9a |
R848b |
91
|
Harnessing the H9e peptide amphiphiles, Li et al. studied the use of peptide nanofibers as an adjuvant to enhance the efficacy of the modified live porcine reproductive and respiratory syndrome virus (PRRSV).85 The implementation of the nanofiber formulation exhibited a capacity to prolong the duration of PRRSV circulation, a phenomenon substantiated through the detection of viral load in pig serum via polymerase chain reaction subsequent to intramuscular vaccination. Notably, the nanofiber-PRRSV vaccine demonstrated the ability to elicit a state of protective immunity against both the VR-2332 and MN184A strains of PRRSV. This was evidenced by a discernible reduction in the circulating viral load. In stark contrast, vaccination involving PRRSV alone conferred protection solely against VR-2332 infection. Remarkably, administration of the nanofiber-PRRSV vaccine yielded notably heightened proportions of memory helper T cells (CD4+ CD8+) alongside correspondingly reduced proportions of regulatory T cells (CD4+CD25+FoxP3+), as compared to vaccination employing PRRSV alone.85
In a study by Rudra et al., the immunogenicity of the malaria peptide epitope (NANP)3 when conjugated to the self-assembling fibrillised-β sheet-forming peptide Q11 was assessed in a murine model.86 Their findings indicated that when mice were immunised with equivalent quantities of Q11 devoid of the (NANP)3 epitope, no antibody response was observed, even after a booster dose, underscoring the essential role of the epitope in antibody generation. Further, the research highlighted that the initiation of an antigen-specific antibody response in mice immunised subcutaneously necessitated the involvement of T cells and the activation of the myeloid differentiation primary response gene 88 (MyD88), without the need for TLR2 or TLR5 interactions. Importantly, when mice were exposed to malaria sporozoites and subsequently administered a booster of the vaccine containing (NANP)3-Q11 nanofibers, there was a pronounced presence of antigen-specific antibodies, an observation not seen in unboosted mice.86 While the study focuses on the (NANP)3 epitope from Plasmodium falciparum circumsporozoite that could possess significantly different immunogenicity in humans, the ability to co-assemble different epitope-bearing peptides opens avenues for developing multi-epitope vaccines. This approach could enhance the immune system's ability to target multiple strains or variants of a pathogen, ultimately leading to increased effectiveness against diseases with high antigenic variability.
In a different study, Pompano et al. established a covalent linkage between PADRE (a CD4+ T cell epitope) and E214 (a B cell epitope derived from Staphylococcus aureus) with the fibrillised-β sheet-forming SGSG-Q11.87 Mice that underwent subcutaneous vaccination with the co-assembled PADRE-Q11 nanofiber showed a significant elevation in the CD4+ PADRE+ CD44+ T cell count within the lymph nodes, in contrast to those vaccinated with PADRE alone. Remarkably, mice administered the nanofiber-conjugated PADRE and E214 demonstrated an E214-specific IgG titer comparable to that seen in mice vaccinated with a combination of E214 linked to a diphtheria toxin carrier and alum. The results also illustrated the potential importance of epitope content modulation in influencing the magnitude and nature of the immune response.87 Utilising modular self-assembly to incorporate B- and T-cell epitopes into vaccines offers a promising avenue for the construction of efficient vaccines. By precisely tailoring immune responses, this approach could lead to the development of vaccines capable of eliciting appropriate and protective immune responses against a wide range of diseases.
Rudra et al. synthesised a conjugate that incorporated the GGAAY spacer and the β sheet-forming FKFEFKFE (KFE8) peptide, which was covalently linked with the CD4+ T cell epitope derived from Mycobacterium tuberculosis (Ag85B). The study sought to elucidate the fundamental mechanisms governing the initiation of the immune response.88 Notably, inhibiting autophagy within APCs resulted in a significant reduction in the enhanced CD4+ or CD8+ T cell responses induced by nanofibers in vitro. Furthermore, inhibition of proteasomal activity compromised the capacity of macrophages treated with nanofibers and antigens to effectively elicit stimulation of CD8+ T cells. A recent study illustrated the ability of Ag85B delivered using KFE8-based β sheet-forming peptide nanofibers to significantly augment the CD4+ T cell response in Bacillus Calmette-Guérin (BCG)-primed mice following intratracheal administration, suggesting the suitability of the peptide nanofibers for efficient pulmonary vaccination.23 Current models highlight the need for multivalent tuberculosis vaccines capable of eliciting immune protection from both CD4+ and CD8+ T cells, along with functional B cells and other immune cell populations. Incorporating these components into a vaccine formulation poses a challenge. The KFE8 nanofiber construct provides a modular vaccine platform capable of accommodating additional immunogenic Mycobacterium tuberculosis-derived antigens and adjuvants. This modularity offers an opportunity to customise the vaccine composition for optimal immune responses against Mycobacterium tuberculosis. Mora-Solano and colleagues have demonstrated that the administration of β sheet-based nanofiber-conjugated TNF4-23 (a B cell epitope) alongside either PADRE (a CD4+ T cell epitope) or vaccinia I1L7-21 (a CD4+ T cell epitope) to mice led to the induction of antigen-specific IgG production and cytokine release from ex vivo stimulated lymphocytes.89 Furthermore, mice that received nanofiber-based vaccinations and subsequently challenged with LPS exhibited reduced signs of inflammation, as evidenced by mitigated hypothermic responses and prolonged survival durations. The ability to elicit protective antibody responses without triggering strong T-cell responses that could lead to autoimmune reactions represents a significant advantage. These approaches pave the way for developing immune therapies that minimise adverse effects and ensure patient safety.
Peptide nanofibers were also previously studied as delivery vehicles for HIV-1 vaccines. Tian et al. conducted an investigation in which mice were vaccinated using intramuscular, intradermal, or subcutaneous injection with nanofibers conjugated to DNA encoding the helical HIV-1 envelope protein gp145.90 This approach resulted in a more robust IgG response, alongside heightened production of IFN-γ and IL-4 by restimulated lymphocytes, in comparison to administering HIV-DNA alone. In vitro incubation of nanofiber-conjugated HIV-DNA with T cells, B cells, or macrophages for a duration of 72 hours did not exert an adverse influence on cellular viability. Importantly, the histological analysis of muscles, epidermis, and dermis following nanofiber administration showed no evident pathological features.
In order to improve vaccine efficacy, Ding et al. integrated the HIV-1 CTL epitope SL9 with the TLR7 and TLR8 agonist R848 (Resiquimod) using a β sheet-forming peptide nanofiber-based delivery approach.91 The incubation of nanofibers carrying SL9 and R848 with peripheral blood monocyte-derived DCs showed the highest propensity for triggering the production of immune-stimulatory cytokines by CD8+ T cells, sourced from individuals with HIV infection. Additionally, nanofibers demonstrated the capacity to facilitate the expansion of antigen-specific CD8+ T cells subsequent to subcutaneous vaccination in murine subjects. Understanding the durability of these responses and the long-term effectiveness of the vaccine could provide insights into the longevity of the elicited CTL responses. Using fibrillised-β sheet-forming Q11-based peptide nanofibers conjugated to the HIV envelope-derived-antigen gp120, Chen et al. illustrated the capacity of a single dose of the nanofiber vaccine to enhance the capacity of the induced antibodies to bind to the heterologous HIV envelope antigen in rabbits.24
Rudra et al. studied the use of the GGAAY spacer-linked and fibrillised-β sheet-forming KFE8 peptide to develop a nanofiber-based vaccine formulation targeting herpes simplex virus (HSV).92 The results showed that subcutaneous administration of the nanofiber-conjugated HSV-derived CD8+ T cell epitope (HSV gB peptide) combined with CpG or Gardiquimod (TLR7 agonist) to mice induced an antigen-specific CTL response in an in vivo setting. Subsequent ex vivo re-stimulation of lymphocytes extracted from the immunised mice revealed significant production of IFN-γ. Using a β sheet-forming (RADA)4 oligopeptide that self-assembles into nanofibers, Grenfell and colleagues demonstrated a novel approach where nanofibers facilitated the delivery of the recombinant hepatitis B virus surface antigen (rHBsAg) in conjugation with CpG.73 This method yielded elevated levels of rHBsAg-specific IgG and IgM when compared to an alum-based combination of rHBsAg and CpG, following subcutaneous administration in murine subjects.
Collectively, the findings presented in this diverse array of studies demonstrated the multifaceted potential of peptide nanofibers as versatile vehicles for antigen delivery and immune modulation. Through their capacity to effectively facilitate antigen transport and presentation, these nanofibers showed their utility across a spectrum of infectious disease models. From influenza and porcine viral infections to malaria, tuberculosis, HIV and herpes simplex virus, the results consistently highlighted the ability of peptide nanofibers to enhance immune responses, evoke antigen-specific antibody production, and promote cell-mediated immunity. Furthermore, the strategic incorporation of various adjuvants and epitopes into nanofiber formulations has unveiled novel avenues for fine-tuning immune outcomes. These studies presented the peptide nanofibers as a promising platform for next-generation vaccine development, offering a tailored approach to combatting a diverse range of infectious agents. Nevertheless, despite the encouraging outcomes observed in murine studies, transitioning to human clinical trials represents a complex challenge. Several variables, such as pre-existing immunity, age and health status, may significantly influence the vaccine's efficacy in human subjects. Addressing these factors requires careful consideration to ensure the successful translation of research findings into practical and effective immunisation strategies.
3.3.2. Cancer vaccines.
The ability of peptide nanofibers to enhance antitumour immune responses has gained substantial attention (Table 3). Subcutaneous administration of coiled coil-forming QARILEADAEILR-AYARILEAHAEILRAQ (Coil29) peptide-based nanofibers incorporating PEPvIII (B cell epitope), Trp2 (melanoma-derived T cell epitope) and Td (tetanus-derived CD4+ T cell epitope) dramatically retarded the growth of subcutaneous B16vII tumours in C57BL/6NHsd mice.25 Song et al. developed a trivalent cancer vaccine using fibrillised-β sheet-forming FEFEFKFK peptide-based nanofibers incorporating various antigenic epitopes, namely glycoprotein 100209-217, tyrosinase369–377 and melanoma-derived MART-126-35.26 The trivalent nanofiber vaccine augmented the CD8+ T cell response and significantly delayed the growth of subcutaneously inoculated B16 cells in C57BL/6J mice. The ability to design vaccines tailored to specific epitopes represents a gateway to personalised cancer immunotherapies. Customising treatments based on individual tumour profiles could significantly improve the effectiveness of immunotherapeutic interventions.
Table 3 Anticancer vaccines delivered via peptide nanofibers
Nanofiber-forming peptide |
Antigen |
Immune adjuvant |
Ref. |
Q11, QQKFQFQFEQQ; Coil29, QARILEADAEILRAYARILEAHAEILRAQ; MAX1, VKVKVKVKVPPTKVKVKVKV; MAX8, VKVKVKVKVPPTKVEVKVKV; HLT2, VLTKVKTKVPPTKVEVKVLV. Covalently conjugated. Non-covalently conjugated. |
FEFEFKFK |
Glycoprotein 100209-217,a tyrosinase369–377a and melanoma-derived MART-126-35a |
— |
26
|
Coil29 |
Melanoma-derived T cell epitope,a B cell epitopea and tetanus-derived CD4+ T cell epitopea |
— |
25
|
VVAGKS |
Melanoma-derived glycosphingolipidb |
Mannosea |
27
|
Naphthylacetic acid-GFFYK |
MUC1 glycopeptidea |
— |
93
|
MAX1, MAX8 or HLT2 |
Plasmid vector encoding for melanoma-specific tumour antigen gp100b |
HMGN1b |
94
|
SGSG-Q11 |
Glycosylated MUC1-derived B cell epitope (MUC1 VNTR)a |
— |
95
|
Q11 |
Human papillomavirus oncoprotein E7(44–62)a |
— |
96
|
Naphthylacetic acid-D- or L-GFFY-methyl amide |
OVAb |
— |
28
|
Using the nanofibers constructed from amphiphilic peptide VVAGKS, Gunay et al. showed that nanofiber-mediated delivery of melanoma-derived glycosphingolipids and mannose exhibited efficient uptake by DCs that stimulated maturation signal initiation in vitro.27 While DC activation is crucial, the complexity of immune responses in vivo poses challenges. Understanding the broader immune reactions elicited by these carriers, including potential regulatory mechanisms, is essential for predicting their overall therapeutic impact accurately. Mice subcutaneously immunised with MUC1 glycopeptides non-covalently mixed or covalently linked with nanofibers constructed using the β sheet-forming naphthylacetic acid-GFFYK peptide displayed heightened production of antigen-specific IgG, IgM, and immunostimulatory cytokines in comparison to counterparts immunised solely with MUC1 glycopeptides.93 However, the covalently linked nanofiber-MUC1 glycopeptide formulation demonstrated superior potency compared to the noncovalent mixture. The antisera derived from mice immunised with covalent nanofiber-MUC1 glycopeptides exhibited the most pronounced level of complement-dependent cytotoxicity upon incubation with MCF-7 cells.
Amphiphilic peptide-based nanofibers were also used as carriers for the plasmid vector encoding a melanoma-specific tumour antigen and the immune adjuvant HMGN1-gp100.94 Under physiological conditions, the nanofiber formulations exhibited sustained DNA retention of approximately 90% over a period of 14 days. Following subcutaneous administration, the co-delivery of DNA with the peptide MAX8 (VKVKVKVKVDPPTKV
VKVKV-NH2) or HLT2 (V![[L with combining low line]](https://www.rsc.org/images/entities/char_004c_0332.gif)
KVK
KVDPPTKV
VKV
V-NH2)-based nanofibers resulted in enhanced ex vivo lymphocyte proliferation, an effect not observed with DNA injection. However, administration of DNA-conjugated MAX1(VKVKVKVKVDPPTKVKVKVKV-NH2)-based nanofibers did not show this response. Importantly, lymphocytes and splenocytes harvested from mice subjected to HLT2-DNA injections demonstrated no detectable cytotoxicity towards co-cultured B16F1 melanoma cells during in vitro assessments. In another study, fibrillised β sheet-forming Q11 peptide-contained nanofibers conjugated with glycosylated MUC1-derived B-cell epitope (MUC1 VNTR) induced a pronounced specific IgG response in murine subjects.95 The antibodies generated through this process demonstrated the capacity to selectively target MUC1-expressing MCF-7 breast cancer cells, leading to the induction of complement-dependent cytotoxicity.
The subcutaneous immunisation of mice with OVA-conjugated nanofibers formed using the D- or L-naphthylacetic acid-linked GFFY β sheet-forming peptide significantly enhanced the OVA-specific IgG response.28 Based on FITC-OVA and flow cytometry, the D or L nanofiber-OVA formulations were found to exhibit elevated uptake of OVA by DCs under in vitro conditions. Notably, the D-nanofiber-OVA formulation showed a more significant cytosolic accumulation of FITC-OVA when compared to L-nanofiber-OVA. Furthermore, the release kinetics of D-nanofiber-OVA at a lysosomal pH of 4.5 surpassed that of L-nanofiber-OVA, highlighting the ability of the D-nanofiber to facilitate antigen escape into the cytosol. These findings suggested that variations in optical properties could significantly impact the vaccine-mediated adjuvanticity. Thus, finding bioactive sequences that maintain this potency is crucial for the nanofiber's effectiveness as a vaccine adjuvant. Enhanced and sustained retention of the introduced fluorescent OVA at lymph nodes, both proximal and distal to the subcutaneous injection site, was notably observed with the D-nanofiber variant. After administration of the D-nanofiber-OVA vaccine to mice, significant increases in cell proliferation and IFN-γ production were observed upon ex vivo stimulation of splenocytes. Specifically, the D-nanofiber-OVA formulation was more effective in suppressing OVA-expressing melanoma B16 cells within a subcutaneous tumour model, leading to improved mouse survival rates compared to either OVA or L-nanofiber-OVA vaccination approaches. To assess the induction of protective immunity, mice were first administered with E.G7 lymphoma cell-incorporated nanofibers and later challenged with the E.G7 tumour cells after 28 days. Remarkably, D-nanofiber-bound E.G7 tumour antigens significantly slowed tumour growth.
Li et al. assessed the efficacy of a vaccine formulation comprising a covalent linked peptide from the human papillomavirus oncoprotein E7(44–62) with β sheet-forming Q11 peptide-contained nanofibers.96 Mice immunised with Q11-E744-62 exhibited a pronounced CD8+ T cell response specific to the antigen, leading to inhibited growth of subcutaneous human papilloma virus oncoprotein-expressing epithelial TC-1 cells. In a related study, mice vaccinated with Q11-E744-62 via intravaginal or intranasal routes displayed significant delay in genital TC-1 tumour growth.97
The studies discussed collectively highlight the promising potential of peptide nanofibers as dynamic tools for inducing and modulating immune responses against tumours. These studies showed the capacity of peptide nanofibers to effectively deliver tumour-specific antigens, adjuvants, and immune modulatory molecules, thereby directing immune reactions that result in tumour growth retardation and prolonged survival outcomes. Moreover, the immunisation strategies involving nanofiber-mediated formulations have demonstrated significant efficiency in inducing antigen-specific immune responses, marked by heightened production of immune effectors such as antigen-specific antibodies and cytokines. Further investigations into the optimisation of nanofiber formulations, delivery methodologies, and combination therapies hold great potential for advancing these nanofiber-mediated cancer immunisation strategies towards clinical application.
3.3.3. Development of needle-free vaccination approaches.
In light of the promising results from previous studies demonstrating the efficacy of nanofiber-based vaccines in eliciting immune responses, this section explores the utilisation of peptide nanofibers in needle-free immunisation strategies. Needle-free vaccination, via intranasal or sublingual routes, for example, offers a range of advantages compared to traditional injection-based vaccines.98 The user-friendly nature of needle-free vaccine administration, coupled with its elimination of the need for specialised skills or training, presents a viable option for self-administration. This attribute proves particularly advantageous for global vaccination initiatives, as it reduces the demand for healthcare personnel and facilitates the implementation of contingency strategies and isolation protocols during instances of pandemic outbreaks. Furthermore, the painless nature of needle-free vaccination, as opposed to conventional injection methods, is a compelling feature that can potentially boost compliance with multi-dose vaccination regimes. Notably, needle-free approaches not only mitigate the risks associated with needle accidents and needlestick injuries, thereby substantially diminishing the potential for blood-borne transmissions, but also contribute to enhanced patient comfort and safety.
Previous studies demonstrated that the intranasal administration of Q11-based nanofibers, covalently linked to an epitope derived from the influenza A/PR/8/34 virus, elicited specific CD8+ T cell responses and provided protective immunity in C57BL/6 mice.99 Kelly et al. conducted a study exploring a potential vaccination strategy using nanofiber-based vaccines delivered through an alternative route of administration.100 Mice that were given a vaccine consisting of cholera toxin and a protein fragment linked to an OVA peptide sublingually exhibited elevated levels of OVA-specific IgG in their bloodstream over a duration of 72 weeks, in contrast to unvaccinated mice. Interestingly, the use of fluorescently labelled nanofibers, detectable subsequent to sublingual administration in mice, indicated an extended sublingual retention period attributed to the presence of polyethylene glycol within their structural composition. To further explore the needle-free vaccination approach, Kelly et al. employed nona-arginine-assembled Q11 peptide nanofibers complexed with a cyclic dinucleotide for sublingual delivery to mice.101In vivo assessments underscored the capacity of the peptide nanofibers to enhance the mucosal immune adjuvanticity of the cyclic dinucleotide, as evidenced by upregulation of CD80 and CD86 levels, crucial costimulatory molecules governing antigen presentation. In a separate study by the same research cohort, sublingual administration of peptide nanofibers loaded with a B-cell epitope from uropathogenic Escherichia coli to mice demonstrated significant protection against urinary tract infection.102 Earlier investigations have also demonstrated that intranasal or vaginal administration of Q11 peptide nanofiber-delivered human papilloma virus-derived epitopes in mice elicited an antitumour immune response, leading to delayed growth of genital tumours.97
These encouraging findings highlight the prospective utility of peptide nanofibers as a promising carrier for needle-free vaccination strategies via mucosal immunisation that could offer more accessible and effective vaccines (Fig. 4).
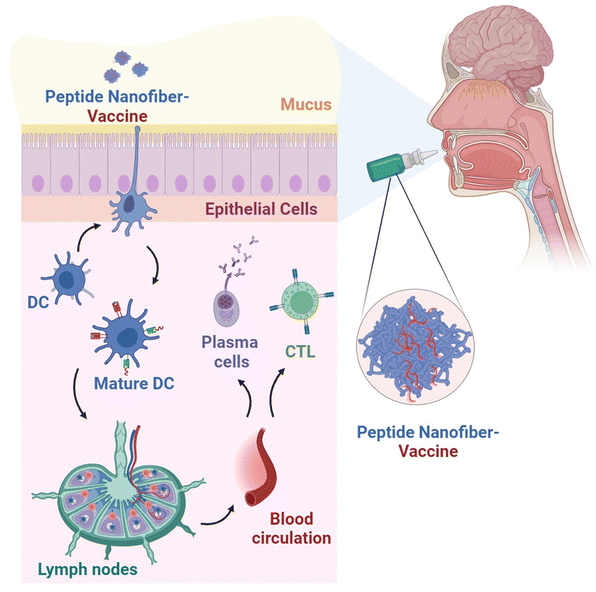 |
| Fig. 4 Peptide nanofiber-mediated mucosal non-invasive vaccination approach. The induction of mucosal immunity that can establish a defensive barrier at the mucosal surface against invading pathogens or develop antitumour immune response against mucosal cancer could be attained through needle-free vaccination routes such as the nasal, sublingual or oral routes. Following the detection and uptake of administered vaccines by the DC at the mucosal tissues, mature DCs migrate to the mucosal lymph nodes to stimulate the resident B-cells and T-cells. The antigen-specific plasma cells and CTLs subsequently migrate to the mucosal sites to establish humoral and cell-mediated immunity, respectively. | |
4. Future perspective
Building upon the foundations laid by the previously discussed studies, this section offers a forward-looking exploration of promising avenues that could enhance the effectiveness of peptide nanofiber-based vaccines.
4.1. Comparative studies and hybrid systems for enhanced vaccine delivery
The diverse capabilities of various nanocarriers pose an important question about how they compare with peptide nanofibers. To this end, the initiation of comparative studies to evaluate how material and morphological characteristics of nanosized carriers influence the potency of incorporated vaccines could provide valuable insights into optimising therapeutic effectiveness. Furthermore, the results of these comparative studies could facilitate the development of a hybrid nanocarrier system that could, for instance, leverage the efficient cell internalisation properties of one type of nanocarrier alongside the vaccine-loading capacity and self-adjuvanticity of another.
Several tubular and spherical nanocarriers showed the capacity to improve the potency of a loaded vaccine.29 Examples for spherical nanoparticles include the cationic chitosan biopolymer-based nanocarriers that were previously utilised as an efficient mucoadhesive vaccine delivery system.103–105 Niosomes, another spherical non-ionic surfactant-based nanomaterial, have also showed a remarkable ability to enhance the delivery and the pharmacological action of the loaded cargo.32,106 Previous studies demonstrated the niosomal ability, as a vaccine delivery vector, to intensify the immune response against the delivered antigens derived from infectious agents or tumour cells.107,108 The efficient deliverability of inorganic material-based nanoparticles such as gold nanoparticles has also promoted their utilisation in vaccine delivery.109 Additionally, tubular nanostructures such as carbon nanotubes previously showed significant enhancement in immune response potency as vaccine carriers.110,111 While spherical and tubular carriers may possess high loading capacity and efficient cell internalisation capacity, respectively, peptide nanofiber-mediated adjuvanticity holds the potential to facilitate the development of nanocarrier-based vaccines capable of eliciting immune responses without relying on additional adjuvants.74 The conductance of comparative studies aimed at identifying the influence of these aforementioned nanocarriers’ attributes on immune response potency could guide future research endeavors towards nanocarriers with favorable properties. This could also facilitate the construction of hybrid systems that harness the full potential of existing nanocarriers to address pressing challenges.
4.2. Exploitation in mRNA-based vaccine delivery
Harnessing a diverse array of antigens expressed by cancer cells or infectious agents, as opposed to a limited selection, can potentially amplify the therapeutic or preventive outcomes of vaccination. This approach could counteract immune evasion mechanisms, often driven by the downregulation of specific antigens.112 Providing the APCs with a comprehensive repertoire of antigens, covering both known and unidentified entities, may promote the generation of a wide range of cytotoxic T cells specific to different antigens. The construction of such a vaccine entails capturing a wide spectrum of antigens through the isolation of either DNA or mRNA.113 A significant challenge in DNA-based vaccines pertains to their requisite delivery to the nuclei of APCs for optimal functionality.114 Consequently, mRNA-based vaccines, which only require cytosolic delivery, present a potentially more potent approach for vaccination.
To date, the application of peptide nanofibers for the delivery of mRNA-based vaccines incorporating antigens derived from infectious agents or cancer cells remains unreported. However, prior investigations have concentrated on exploring the potential utility of peptide nanofibers in gene delivery. Leveraging the capacity of peptide nanofibers as gene delivery carriers in the development of mRNA-based vaccines could potentially help overcome challenges associated with traditional vaccines. In light of this, Mazza et al. utilised the palmitoyl-conjugated GGGAAAKRK peptide in the delivery of BCL2 siRNA, a modulator of apoptosis.115 Their findings indicated that nanofibers significantly augmented the internalisation of fluorescently labelled siNEG-A546 by human neuronal SH-SY5Y cells. Moreover, administration of nanofiber-siRNA in rats resulted in notably diminished BCL2 expression levels, surpassing those induced by naked siRNA injections. In a separate study, Zhang et al. used a peptide containing four arginine residues, suitably modified with palmitic acid and tetraphenylethene, to facilitate the efficient delivery of plasmid DNA encoding enhanced green fluorescent protein (EGFP-N1).75 The resulting nanofiber complexes effectively transduced HeLa, HepG2, NIH 3T3, and stem cells after 24 and 48 hours of in vitro incubation. These investigations highlight the suitability of peptide nanotubes as promising candidates for the development of potent mRNA-based vaccines.
4.3. Disruption of tumour-mediated immune suppression
The immune regulatory receptor programmed cell death protein 1 (PD-1), expressed by T cells, has shown elevated expression in T cells that are specific for tumour antigens.116–118 Its ligand PD-1L is expressed not only by cancer cells but also APCs. The interaction between PD-1 and PD-L1 has been substantively elucidated as a mechanism that dampens T cell functionality, particularly by curbing their proliferative potential and tempering cytokine production. Notably, blocking this interaction with specific antibodies against either PD-1 or PD-1L can restore T cell activities.117–119 Accordingly, anti-PD-1 antibodies such as nivolumab and pembrolizumab have successfully attained clinical approval for the treatment of malignant melanoma due to their proven efficacy.120,121 Concurrently, various clinical trials are evaluating alternative anti-PD-1 or anti-PD-1L antibodies.122 Previously reported studies utilised nanoparticles in disruption of the PD-1/PD-1L interaction through the application of either specific antibodies123–125 or siRNA.126,127 However, this strategy raises safety concerns due to potential emergence of autoimmune disorders, given that it blocks a regulatory pathway governing T cell activity.128,129 Additionally, the efficacy of therapeutic approaches centered on targeting the PD-1/PD-L1 axis is susceptible to decline due to the eventual emergence of acquired therapeutic resistance.130
Emerging investigations have brought to light a fresh array of co-inhibitory receptors such as T-cell immunoglobulin mucin-3 (Tim-3) and lymphocyte-activation gene-3 (Lag-3), which contribute to the immune suppressive mechanisms. These receptors are expressed in regulatory T cells, CD8+ T cells, and natural killer (NK) cells.131 In the preclinical settings, previous studies aimed at evaluating the effects of disrupting these receptors on antitumour immune responses demonstrated promising results, encompassing both effectiveness and tolerability.132,133 This encouraging preclinical evidence has set the stage for further clinical investigations. To this end, the prospect of development of a nanofiber-based immune therapeutic approach targeting these emerging co-inhibitory receptors represents an exciting opportunity. The rationale of this approach is rooted in its potential to harness the nuances of these novel receptors, thus holding promise as a pathway to augmenting immune therapeutic strategies.
The recent advancements in immune modulatory strategies could offer promising avenues for therapeutic interventions. Nevertheless, a significant gap remains in the development of precise delivery vectors to maximise the efficacy of these approaches. The full therapeutic potential of these immune modulators could be effectively achieved through their integration into cutting-edge nanoscale materials, with peptide nanofibers standing out as promising candidates for this purpose.
5. Conclusions
Immunologically mediated prophylactic and therapeutic strategies have attracted significant attention. The quest for safe and efficacious methods to regulate and amplify immune modulatory responses has catalysed the development and application of innovative nanomaterials, including peptide nanofibers. Beyond their deliverability attributes, peptide nanofibers exhibit unique immune adjuvanticity, potentially positioning them as optimal candidates for vaccine delivery. Nonetheless, the proposed immune therapeutic use of peptide nanofibers remains a rich domain of research, mandating thorough investigation to fully elucidate their potential merits. Future research endeavours should prioritise the optimisation of nanofiber synthesis to ensure biocompatibility, stability, and enhanced delivery efficiency. A crucial element of this pursuit is the rigorous evaluation of the clinical efficacy of peptide nanofibers, which demands the design and execution of robust clinical trials assessing therapeutic outcomes and monitoring potential toxicities. To tailor these materials for specific therapeutic applications, a deeper understanding of the molecular interactions between peptide nanofibers and immune cells is pivotal. Integrating computational modelling with experimental studies can provide valuable insights into designing nanofibers with enhanced immune modulatory properties. Furthermore, the development of multifunctional peptide nanofibers, capable of simultaneously delivering antigens, adjuvants, and immune modulators, holds promise for enhancing the efficacy of therapeutic interventions. This combinatorial approach can potentiate immune responses while minimising potential side effects. The incorporation of bio-sensing or imaging modalities into peptide nanofibers enables real-time monitoring of their distribution, degradation, and interaction with immune cells in vivo, offering valuable feedback for dose adjustments and therapeutic efficacy evaluations. In addition, while preliminary findings on peptide nanofibers are promising, upscaling production poses a significant challenge in the transition from bench to bedside. Addressing this challenge involves investigating scalable synthesis methods, optimising purification protocols, and ensuring batch-to-batch consistency, all of which are critical components of this endeavour. Moreover, exploring the potential synergy of peptide nanofibers with other immune modulatory agents opens up exciting avenues for combinatorial therapeutic strategies, potentially leading to therapies with augmented efficacy.
Author contributions
Hatem A. F. M. Hassan, conceptualisation, investigation, visualisation, writing – original draft, and writing – review and editing. Mohamed Haider investigation, writing – original draft, and writing – review and editing. Sherif Ashraf Fahmy, investigation, writing – original draft, and writing – review and editing.
Conflicts of interest
There are no conflicts to declare.
Acknowledgements
This work was supported by the University of Sharjah targeted research project grant # 2201110363 and competitive research grant # 2101110252 to Mohamed Haider. Sincere thanks are extended to Dr Wafa Aljamal for initial feedback on an earlier draft. The graphical abstract and Fig. 1–4 were created using BioRender.com.
References
- F. Bray, M. Laversanne, E. Weiderpass and I. Soerjomataram, The ever-increasing importance of cancer as a leading cause of premature death worldwide, Cancer, 2021, 127(16), 3029–3030 CrossRef PubMed.
- H. Sung, J. Ferlay, R. L. Siegel, M. Laversanne, I. Soerjomataram, A. Jemal and F. Bray, Global Cancer Statistics 2020: GLOBOCAN Estimates of Incidence and Mortality Worldwide for 36 Cancers in 185 Countries, Ca-Cancer J. Clin., 2021, 71(3), 209–249 CrossRef PubMed.
- S. C. Shah, V. Kayamba, R. M. Peek and D. Heimburger, Cancer Control in Low- and Middle-Income Countries: Is It Time to Consider Screening?, J. Glob. Oncol., 2019,(5), 1–8 Search PubMed.
- J. Artym and M. Zimecki, Colostrum Proteins in Protection against Therapy-Induced Injuries in Cancer Chemo- and Radiotherapy: A Comprehensive Review, Biomedicines, 2023, 11(1), 114 CrossRef CAS PubMed.
- M. Haider, K. Z. Zaki, M. R. El Hamshary, Z. Hussain, G. Orive and H. O. Ibrahim, Polymeric nanocarriers: A promising tool for early diagnosis and efficient treatment of colorectal cancer, J. Adv. Res., 2022, 39, 237–255 CrossRef CAS PubMed.
- M. Haider, A. Elsherbeny, V. Pittalà, V. Consoli, M. A. Alghamdi, Z. Hussain, G. Khoder and K. Greish, Nanomedicine Strategies for Management of Drug Resistance in Lung Cancer, Int. J. Mol. Sci., 2022, 23(3), 1853 CrossRef CAS PubMed.
- K. S. Mallikarjun, R. W. Eldaya, M. M. Miller-Thomas, H. L. Orlowski and M. S. Parsons, Good Gone Bad: Complications of Chemotherapy, Immunotherapy, and Radiotherapy on the CNS, Curr. Probl. Diagn. Radiol., 2024, 53(1), 133–149 CrossRef PubMed.
- B. A. Chabner and T. G. Roberts, Jr., Timeline: Chemotherapy and the war on cancer, Nat. Rev. Cancer, 2005, 5(1), 65–72 CrossRef CAS PubMed.
- J. Couzin-Frankel, Cancer Immunotherapy, Science, 2013, 342(6165), 1432–1433 CrossRef CAS PubMed.
- S. K. Wculek, F. J. Cueto, A. M. Mujal, I. Melero, M. F. Krummel and D. Sancho, Dendritic cells in cancer immunology and immunotherapy, Nat. Rev. Immunol., 2020, 20(1), 7–24 CrossRef CAS PubMed.
- T. Kawai and S. Akira, The role of pattern-recognition receptors in innate immunity: update on Toll-like receptors, Nat. Immunol., 2010, 11(5), 373–384 CrossRef CAS PubMed.
- P. Broz and D. M. Monack, Newly described pattern recognition receptors team up against intracellular pathogens, Nat. Rev. Immunol., 2013, 13(8), 551–565 CrossRef CAS PubMed.
- T. Gong, L. Liu, W. Jiang and R. Zhou, DAMP-sensing receptors in sterile inflammation and inflammatory diseases, Nat. Rev. Immunol., 2020, 20(2), 95–112 CrossRef CAS PubMed.
- I. Heras-Murillo, I. Adán-Barrientos, M. Galán, S. K. Wculek and D. Sancho, Dendritic cells as orchestrators of anticancer immunity and immunotherapy, Nat. Rev. Clin. Oncol., 2024, 21(4), 257–277 CrossRef PubMed.
- Y. Togashi, K. Shitara and H. Nishikawa, Regulatory T cells in cancer immunosuppression – implications for anticancer therapy, Nat. Rev. Clin. Oncol., 2019, 16(6), 356–371 CrossRef CAS PubMed.
- J. L. Gerberding and B. F. Haynes, Vaccine Innovations – Past and Future, N. Engl. J. Med., 2021, 384(5), 393–396 CrossRef PubMed.
- A. D. Waldman, J. M. Fritz and M. J. Lenardo, A guide to cancer immunotherapy: from T cell basic science to clinical practice, Nat. Rev. Immunol., 2020, 20(11), 651–668 CrossRef CAS PubMed.
- B. Pulendran, P. S. Arunachalam and D. T. O’Hagan, Emerging concepts in the science of vaccine adjuvants, Nat. Rev. Drug Discovery, 2021, 20(6), 454–475 CrossRef CAS PubMed.
- J. L. Excler, M. Saville, S. Berkley and J. H. Kim, Vaccine development for emerging
infectious diseases, Nat. Med., 2021, 27(4), 591–600 CrossRef CAS PubMed.
- C. Herve, B. Laupeze, G. Del Giudice, A. M. Didierlaurent and F. Tavares Da Silva, The how's and what's of vaccine reactogenicity, npj Vaccines, 2019, 4, 39 CrossRef PubMed.
- M. B. Demircan, S. Tohumeken, N. Gunduz, M. A. Khalily, T. Tekinay, M. O. Guler and A. B. Tekinay, Biotin Functionalized Self-Assembled Peptide Nanofiber as an Adjuvant for Immunomodulatory Response, Biotechnol. J., 2020, 15(12), e2000100 CrossRef PubMed.
- S. Tohumeken, N. Gunduz, M. B. Demircan, G. Gunay, A. E. Topal, M. A. Khalily, T. Tekinay, A. Dana, M. O. Guler and A. B. Tekinay, A Modular Antigen Presenting Peptide/Oligonucleotide Nanostructure Platform for Inducing Potent Immune Response, Adv. Biosyst., 2017, 1(5), 1700015 CrossRef PubMed.
- M. A. Files, K. F. Naqvi, T. B. Saito, T. M. Clover, J. S. Rudra and J. J. Endsley, Self-adjuvanting nanovaccines boost lung-resident CD4(+) T cell immune responses in BCG-primed mice, npj Vaccines, 2022, 7(1), 48 CrossRef CAS PubMed.
- J. L. Chen, C. N. Fries, S. J. Berendam, N. S. Rodgers, E. F. Roe, Y. Wu, S. H. Li, R. Jain, B. Watts, J. Eudailey, R. Barfield, C. Chan, M. A. Moody, K. O. Saunders, J. Pollara, S. R. Permar, J. H. Collier and G. G. Fouda, Self-assembling peptide nanofiber HIV vaccine elicits robust vaccine-induced antibody functions and modulates Fc glycosylation, Sci. Adv., 2022, 8(38), eabq0273 CrossRef CAS PubMed.
- Y. Wu, H. Wen, Z. J. Bernstein, K. M. Hainline, T. S. Blakney, K. L. Congdon, D. J. Snyder, J. H. Sampson, L. Sanchez-Perez and J. H. Collier, Multiepitope supramolecular peptide nanofibers eliciting coordinated humoral and cellular antitumor immune responses, Sci. Adv., 2022, 8(29), eabm7833 CrossRef CAS PubMed.
- H. Song, Q. Su, Y. Nie, C. Zhang, P. Huang, S. Shi, Q. Liu and W. Wang, Supramolecular assembly of a trivalent peptide hydrogel vaccine for cancer immunotherapy, Acta Biomater., 2023, 158, 535–546 CrossRef CAS PubMed.
- G. Gunay, M. Sardan Ekiz, X. Ferhati, B. Richichi, C. Nativi, A. B. Tekinay and M. O. Guler, Antigenic GM3 Lactone Mimetic Molecule Integrated Mannosylated Glycopeptide Nanofibers for the Activation and Maturation of Dendritic Cells, ACS Appl. Mater. Interfaces, 2017, 9(19), 16035–16042 CrossRef CAS PubMed.
- Z. Luo, Q. Wu, C. Yang, H. Wang, T. He, Y. Wang, Z. Wang, H. Chen, X. Li, C. Gong and Z. Yang, A Powerful CD8+ T-Cell Stimulating D-Tetra-Peptide Hydrogel as a Very Promising Vaccine Adjuvant, Adv. Mater., 2017, 29(5), 1601776 CrossRef PubMed.
- C. N. Fries, E. J. Curvino, J. L. Chen, S. R. Permar, G. G. Fouda and J. H. Collier, Advances in nanomaterial vaccine strategies to address infectious diseases impacting global health, Nat. Nanotechnol., 2021, 16(4), 1–14 CrossRef CAS PubMed.
- M. Saxena, S. H. van der Burg, C. J. M. Melief and N. Bhardwaj, Therapeutic cancer vaccines, Nat. Rev. Cancer, 2021, 21(6), 360–378 CrossRef CAS PubMed.
- A. H. AbuBakr, H. Hassan, A. Abdalla, O. M. Khowessah and G. A. Abdelbary, Therapeutic potential of cationic bilosomes in the treatment of carrageenan-induced rat arthritis via fluticasone propionate gel, Int. J. Pharm., 2023, 635, 122776 CrossRef CAS PubMed.
- A. R. Al Jayoush, H. A. F. M. Hassan, H. Asiri, M. Jafar, R. Saeed, R. Harati and M. Haider, Niosomes for nose-to-brain delivery: A non-invasive versatile carrier system for drug delivery in neurodegenerative diseases, J. Drug Delivery Sci. Technol., 2023, 89, 105007 CrossRef CAS.
- S. A. Fahmy, A. Ramzy, A. M. Sawy, M. Nabil, M. Z. Gad, M. El-Shazly, M. A. M. Aboul-Soud and H.M.E.-S. Azzazy, Ozonated Olive Oil: Enhanced Cutaneous Delivery via Niosomal Nanovesicles for Melanoma Treatment, Antioxidants, 2022, 11(7), 1318 CrossRef CAS PubMed.
- H. M. E.-S. Azzazy, A. M. Sawy, A. Abdelnaser, M. R. Meselhy, T. Shoeib and S. A. Fahmy, Peganum harmala Alkaloids and Tannic Acid Encapsulated in PAMAM Dendrimers: Improved Anticancer Activities as Compared to Doxorubicin, ACS Appl. Polym. Mater., 2022, 4(10), 7228–7239 CrossRef CAS.
- R. A. Youness, A. M. Al-Mahallawi, F. H. Mahmoud, H. Atta, M. Braoudaki and S. A. Fahmy, Oral Delivery of Psoralidin by Mucoadhesive Surface-Modified Bilosomes Showed Boosted Apoptotic and Necrotic Effects against Breast and Lung Cancer Cells, Polymers, 2023, 15(6), 1464 CrossRef CAS PubMed.
- N. K. Sedky, N. M. Abdel-Kader, M. Y. Issa, M. M. M. Abdelhady, S. N. Shamma, U. Bakowsky and S. A. Fahmy, Co-Delivery of Ylang Ylang Oil of Cananga odorata and Oxaliplatin Using Intelligent pH-Sensitive Lipid-Based Nanovesicles for the Effective Treatment of Triple-Negative Breast Cancer, Int. J. Mol. Sci., 2023, 24(9), 8392 CrossRef CAS PubMed.
- Q. Sun, J. Wu, L. Jin, L. Hong, F. Wang, Z. Mao and M. Wu, Cancer cell membrane-coated gold nanorods for photothermal therapy and radiotherapy on oral squamous cancer, J. Mater. Chem. B, 2020, 8(32), 7253–7263 RSC.
- S. Eltahir, R. Al Homsi, J. Jagal, I. S. Ahmed and M. Haider, Graphene Oxide/Chitosan Injectable Composite Hydrogel for Controlled Release of Doxorubicin: An Approach for Enhanced Intratumoral Delivery, Nanomaterials, 2022, 12(23), 4261 CrossRef CAS PubMed.
- H. Hassan, S. S. Diebold, L. A. Smyth, A. A. Walters, G. Lombardi and K. T. Al-Jamal, Application of carbon nanotubes in cancer vaccines: Achievements, challenges and chances, J. Controlled Release, 2019, 297, 79–90 CrossRef CAS PubMed.
- N. K. Sedky, N. K. Mahdy, N. M. Abdel-kader, M. M. M. Abdelhady, M. Maged, A. L. Allam, M. Y. Alfaifi, S. N. Shamma, H. A. F. M. Hassan and S. A. Fahmy, Facile sonochemically-assisted bioengineering of titanium dioxide nanoparticles and deciphering their potential in treating breast and lung cancers: biological, molecular, and computational-based investigations, RSC Adv., 2024, 14(12), 8583–8601 RSC.
- S. A. Fahmy, A. Dawoud, Y. A. Zeinelabdeen, C. J. Kiriacos, K. A. Daniel, O. Eltahtawy, M. M. Abdelhalim, M. Braoudaki and R. A. Youness, Molecular Engines, Therapeutic Targets, and Challenges in Pediatric Brain Tumors: A Special Emphasis on Hydrogen Sulfide and RNA-Based Nano-Delivery, Cancers, 2022, 14(21), 5244 CrossRef CAS PubMed.
- S. Eskandari, T. Guerin, I. Toth and R. J. Stephenson, Recent advances in self-assembled peptides: Implications for targeted drug delivery and vaccine engineering, Adv. Drug Delivery Rev., 2017, 110–111, 169–187 CrossRef CAS PubMed.
- W. Zhang, X. Yu, Y. Li, Z. Su, K. D. Jandt and G. Wei, Protein-mimetic peptide nanofibers: Motif design, self-assembly synthesis, and sequence-specific biomedical applications, Prog. Polym. Sci., 2018, 80, 94–124 CrossRef CAS.
- F. Gelain, Z. Luo, M. Rioult and S. Zhang, Self-assembling peptide scaffolds in the clinic, npj Regener. Med., 2021, 6(1), 9 CrossRef CAS PubMed.
- F. Gelain, Z. Luo and S. Zhang, Self-Assembling Peptide EAK16 and RADA16 Nanofiber Scaffold Hydrogel, Chem. Rev., 2020, 120(24), 13434–13460 CrossRef CAS PubMed.
- Y. Wu and J. H. Collier, α-Helical coiled-coil peptide materials for biomedical applications, Wiley Interdiscip. Rev.: Nanomed. Nanobiotechnol., 2017, 9(2), e1424 Search PubMed.
- J. Li, Y. Zhao, P. Zhou, X. Hu, D. Wang, S. M. King, S. E. Rogers, J. Wang, J. R. Lu and H. Xu, Ordered Nanofibers Fabricated from Hierarchical Self-Assembling Processes of Designed α-Helical Peptides, Small, 2020, 16(45), 2003945 CrossRef CAS PubMed.
- V. Castelletto, J. Seitsonen, J. Ruokolainen and I. W. Hamley, Alpha helical surfactant-like peptides self-assemble into pH-dependent nanostructures, Soft Matter, 2021, 17(11), 3096–3104 RSC.
- S. Zhang, Fabrication of novel biomaterials through molecular self-assembly, Nat. Biotechnol., 2003, 21(10), 1171–1178 CrossRef CAS PubMed.
- N. L. Fletcher, C. V. Lockett, A. F. Dexter and A. pH-responsive, coiled-coil peptide hydrogel, Soft Matter, 2011, 7(21), 10210–10218 RSC.
- M. Meleties, P. Katyal, B. Lin, D. Britton and J. K. Montclare, Self-assembly of stimuli-responsive coiled-coil fibrous hydrogels, Soft Matter, 2021, 17(26), 6470–6476 RSC.
- D.-i Kim, S.-h Han, H. Park, S. Choi, M. Kaur, E. Hwang, S.-j Han, J.-y Ryu, H.-K. Cheong, R. P. Barnwal and Y.-b Lim, Pseudo-Isolated α-Helix Platform for the Recognition of Deep and Narrow Targets, J. Am. Chem. Soc., 2022, 144(34), 15519–15528 CrossRef CAS PubMed.
- C. Minelli, J. X. Liew, M. Muthu and H. Andresen, Coiled coil peptide-functionalized surfaces for reversible molecular binding, Soft Matter, 2013, 9(20), 5119–5124 RSC.
- X. Le, T. Gao, L. Wang, F. Wei, C. Chen and Y. Zhao, Self-Assembly of Short Amphiphilic Peptides and Their Biomedical Applications, Curr. Pharm. Des., 2022, 28(44), 3546–3562 CrossRef CAS PubMed.
- C. J. C. Edwards-Gayle and I. W. Hamley, Self-assembly of bioactive peptides, peptide conjugates, and peptide mimetic materials, Org. Biomol. Chem., 2017, 15(28), 5867–5876 RSC.
- C. J. C. Edwards-Gayle and I. W. Hamley, Self-assembly of bioactive peptides, peptide conjugates, and peptide mimetic materials, Org. Biomol. Chem., 2017, 15(28), 5867–5876 RSC.
- C. Zhao, H. Chen, F. Wang and X. Zhang, Amphiphilic self-assembly peptides: Rational strategies to design and delivery for drugs in biomedical applications, Colloids Surf., B, 2021, 208, 112040 CrossRef CAS PubMed.
- Z. Liu, X. Tang, F. Feng, J. Xu, C. Wu, G. Dai, W. Yue, W. Zhong and K. Xu, Molecular design of peptide amphiphiles for controlled self-assembly and drug release, J. Mater. Chem. B, 2021, 9(15), 3326–3334 RSC.
- A. Dasgupta and D. Das, Designer Peptide Amphiphiles: Self-Assembly to Applications, Langmuir, 2019, 35(33), 10704–10724 CrossRef CAS PubMed.
-
Y.-C. Lee and J.-Y. Moon, Bionanotechnology: Biological Self-Assembly, Introduction to Bionanotechnology, Springer, Singapore, 2020, pp. 79–92 Search PubMed.
- S. Kim, J. H. Kim, J. S. Lee and C. B. Park, Beta-Sheet-Forming, Self-Assembled Peptide Nanomaterials towards Optical, Energy, and Healthcare Applications, Small, 2015, 11(30), 3623–3640 CrossRef CAS PubMed.
- Z. Yu, Z. Cai, Q. Chen, M. Liu, L. Ye, J. Ren, W. Liao and S. Liu, Engineering beta-sheet peptide assemblies for biomedical applications, Biomater. Sci., 2016, 4(3), 365–374 RSC.
- D. M. Raymond and B. L. Nilsson, Multicomponent peptide assemblies, Chem. Soc. Rev., 2018, 47(10), 3659–3720 RSC.
- S. Chen, Y. Wu, F. Lortie, J. Bernard, W. H. Binder and J. Zhu, Hydrogen-Bonds-Mediated Nanomedicine: Design, Synthesis, and Applications, Macromol. Rapid Commun., 2022, 43(18), e2200168 CrossRef PubMed.
- K. M. Wong, A. S. Robang, A. H. Lint, Y. Wang, X. Dong, X. Xiao, D. T. Seroski, R. Liu, Q. Shao, G. A. Hudalla, C. K. Hall and A. K. Paravastu, Engineering beta-Sheet Peptide Coassemblies for Biomaterial Applications, J. Phys. Chem. B, 2021, 125(50), 13599–13609 CrossRef CAS PubMed.
- C. N. Fries, Y. Wu, S. H. Kelly, M. Wolf, N. L. Votaw, S. Zauscher and J. H. Collier, Controlled Lengthwise Assembly of Helical Peptide Nanofibers to Modulate CD8(+) T-Cell Responses, Adv. Mater., 2020, 32(39), e2003310 CrossRef PubMed.
- J. S. Rudra, T. Sun, K. C. Bird, M. D. Daniels, J. Z. Gasiorowski, A. S. Chong and J. H. Collier, Modulating adaptive immune responses to peptide self-assemblies, ACS Nano, 2012, 6(2), 1557–1564 CrossRef CAS PubMed.
- Y. Wu, S. H. Kelly, L. Sanchez-Perez, J. H. Sampson and J. H. Collier, Comparative study of alpha-helical and beta-sheet self-assembled peptide nanofiber vaccine platforms: influence of integrated T-cell epitopes, Biomater. Sci., 2020, 8(12), 3522–3535 RSC.
- Y. Wen, A. Waltman, H. Han and J. H. Collier, Switching the Immunogenicity of Peptide Assemblies Using Surface Properties, ACS Nano, 2016, 10(10), 9274–9286 CrossRef CAS PubMed.
- C. Yang, F. Shi, C. Li, Y. Wang, L. Wang and Z. Yang, Single Dose of Protein Vaccine with Peptide Nanofibers As Adjuvants Elicits Long-Lasting Antibody Titer, ACS Biomater. Sci. Eng., 2018, 4(6), 2000–2006 CrossRef CAS PubMed.
- T. Waku, S. Nishigaki, Y. Kitagawa, S. Koeda, K. Kawabata, S. Kunugi, A. Kobori and N. Tanaka, Effect of the Hydrophilic-Hydrophobic Balance of Antigen-Loaded Peptide Nanofibers on Their Cellular Uptake, Cellular Toxicity, and Immune Stimulatory Properties, Int. J. Mol. Sci., 2019, 20(15), 3781 CrossRef CAS PubMed.
- T. Waku, A. Kasai, A. Kobori and N. Tanaka, Investigation on the Interactions between Self-Assembled beta-Sheet Peptide Nanofibers and Model Cell Membranes, Int. J. Mol. Sci., 2020, 21(24), 9518 CrossRef CAS PubMed.
- R. F. Q. Grenfell, L. M. Shollenberger, E. F. Samli and D. A. Harn, Vaccine Self-Assembling Immune Matrix Is a New Delivery Platform That Enhances Immune Responses to Recombinant HBsAg in Mice, Clin. Vaccine Immunol., 2015, 22(3), 336–343 CrossRef CAS PubMed.
- Y. Si, Y. Wen, J. Chen, R. R. Pompano, H. Han, J. H. Collier and A. S. Chong, MyD88 in antigen-presenting cells is not required for CD4+ T-cell responses during peptide nanofiber vaccination, MedChemComm, 2018, 9(1), 138–148 RSC.
- C. Zhang, T. Zhang, S. Jin, X. Xue, X. Yang, N. Gong, J. Zhang, P. C. Wang, J. H. Tian, J. Xing and X. J. Liang, Virus-Inspired Self-Assembled Nanofibers with Aggregation-Induced Emission for Highly Efficient and Visible Gene Delivery, ACS Appl. Mater. Interfaces, 2017, 9(5), 4425–4432 CrossRef CAS PubMed.
- G. A. Hudalla, T. Sun, J. Z. Gasiorowski, H. Han, Y. F. Tian, A. S. Chong and J. H. Collier, Gradated assembly of multiple proteins into supramolecular nanomaterials, Nat. Mater., 2014, 13, 829 CrossRef CAS PubMed.
- J. Chen, R. R. Pompano, F. W. Santiago, L. Maillat, R. Sciammas, T. Sun, H. Han, D. J. Topham, A. S. Chong and J. H. Collier, The use of self-adjuvanting nanofiber vaccines to elicit high-affinity B cell responses to peptide antigens without inflammation, Biomaterials, 2013, 34(34), 8776–8785 CrossRef CAS PubMed.
- Y. Wen and J. H. Collier, Supramolecular peptide vaccines: tuning adaptive immunity, Curr. Opin. Immunol., 2015, 35, 73–79 CrossRef CAS PubMed.
- A. N. Moore and J. D. Hartgerink, Self-Assembling Multidomain Peptide Nanofibers for Delivery of Bioactive Molecules and Tissue Regeneration, Acc. Chem. Res., 2017, 50(4), 714–722 CrossRef CAS PubMed.
- J. S. Rudra, Y. F. Tian, J. P. Jung and J. H. Collier, A self-assembling peptide acting as an immune adjuvant, Proc. Natl. Acad. Sci. U. S. A., 2010, 107(2), 622–627 CrossRef CAS PubMed.
- H. Zhang, J. Park, Y. Jiang and K. A. Woodrow, Rational design of charged peptides that self-assemble into robust nanofibers as immune-functional scaffolds, Acta Biomater., 2017, 55, 183–193 CrossRef CAS PubMed.
- C. B. Chesson, E. J. Huelsmann, A. T. Lacek, F. J. Kohlhapp, M. F. Webb, A. Nabatiyan, A. Zloza and J. S. Rudra, Antigenic peptide nanofibers elicit adjuvant-free CD8+ T cell responses, Vaccine, 2014, 32(10), 1174–1180 CrossRef CAS PubMed.
- G. A. Hudalla, J. A. Modica, Y. F. Tian, J. S. Rudra, A. S. Chong, T. Sun, M. Mrksich and J. H. Collier, A self-adjuvanting supramolecular vaccine carrying a folded protein antigen, Adv. Healthcare Mater., 2013, 2(8), 1114–1119 CrossRef CAS PubMed.
- H. Huang, J. Shi, J. Laskin, Z. Liu, D. S. McVey and X. S. Sun, Design of a shear-thinning recoverable peptide hydrogel from native sequences and application for influenza H1N1 vaccine adjuvant, Soft Matter, 2011, 7(19), 8905–8912 RSC.
- X. Li, A. Galliher-Beckley, H. Huang, X. Sun and J. Shi, Peptide nanofiber hydrogel adjuvanted live virus vaccine enhances cross-protective immunity to porcine reproductive and respiratory syndrome virus, Vaccine, 2013, 31(41), 4508–4515 CrossRef CAS PubMed.
- J. S. Rudra, S. Mishra, A. S. Chong, R. A. Mitchell, E. H. Nardin, V. Nussenzweig and J. H. Collier, Self-assembled peptide nanofibers raising durable antibody responses against a malaria epitope, Biomaterials, 2012, 33(27), 6476–6484 CrossRef CAS PubMed.
- R. R. Pompano, J. Chen, E. A. Verbus, H. Han, A. Fridman, T. McNeely, J. H. Collier and A. S. Chong, Titrating T-Cell Epitopes within Self-Assembled Vaccines Optimizes CD4+ Helper T Cell and Antibody Outputs, Adv. Healthcare Mater., 2014, 3(11), 1898–1908 CrossRef CAS PubMed.
- J. S. Rudra, A. Khan, T. M. Clover, J. J. Endsley, A. Zloza, J. Wang and C. Jagannath, Supramolecular Peptide Nanofibers Engage Mechanisms of Autophagy in Antigen-Presenting Cells, ACS Omega, 2017, 2(12), 9136–9143 CrossRef CAS PubMed.
- C. Mora-Solano, Y. Wen, H. Han, J. Chen, A. S. Chong, M. L. Miller, R. R. Pompano and J. H. Collier, Active immunotherapy for TNF-mediated inflammation using self-assembled peptide nanofibers, Biomaterials, 2017, 149, 1–11 CrossRef CAS PubMed.
- Y. Tian, H. Wang, Y. Liu, L. Mao, W. Chen, Z. Zhu, W. Liu, W. Zheng, Y. Zhao, D. Kong, Z. Yang, W. Zhang, Y. Shao and X. Jiang, A Peptide-Based Nanofibrous Hydrogel as a Promising DNA Nanovector for Optimizing the Efficacy of HIV Vaccine, Nano Lett., 2014, 14(3), 1439–1445 CrossRef CAS PubMed.
- Y. Ding, J. Liu, S. Lu, J. Igweze, W. Xu, D. Kuang, C. Zealey, D. Liu, A. Gregor, A. Bozorgzad, L. Zhang, E. Yue, S. Mujib, M. Ostrowski and P. Chen, Self-assembling peptide for co-delivery of HIV-1 CD8+ T cells epitope and Toll-like receptor 7/8 agonists R848 to induce maturation of monocyte derived dendritic cell and augment polyfunctional cytotoxic T lymphocyte (CTL) response, J. Controlled Release, 2016, 236, 22–30 CrossRef CAS PubMed.
- J. S. Rudra, B. N. Banasik and G. N. Milligan, A combined carrier-adjuvant system of peptide nanofibers and toll-like receptor agonists potentiates robust CD8+ T cell responses, Vaccine, 2018, 36(4), 438–441 CrossRef CAS PubMed.
- Y. Liu, Y. Wang, F. Yu, Z. Zhang, Z. Yang, W. Zhang, P. G. Wang and W. Zhao, Potentiating the immune response of MUC1-based antitumor vaccines using a peptide-based nanovector as a promising vaccine adjuvant, Chem. Commun., 2017, 53(68), 9486–9489 RSC.
- S. H. Medina, S. Li, O. M. Howard, M. Dunlap, A. Trivett, J. P. Schneider and J. J. Oppenheim, Enhanced immunostimulatory effects of DNA-encapsulated peptide hydrogels, Biomaterials, 2015, 53, 545–553 CrossRef CAS PubMed.
- Z. H. Huang, L. Shi, J. W. Ma, Z. Y. Sun, H. Cai, Y. X. Chen, Y. F. Zhao and Y. M. Li, A totally synthetic, self-assembling, adjuvant-free MUC1 glycopeptide vaccine for cancer therapy, J. Am. Chem. Soc., 2012, 134(21), 8730–8733 CrossRef CAS PubMed.
- S. Li, Q. Zhang, H. Bai, W. Huang, C. Shu, C. Ye, W. Sun and Y. Ma, Self-Assembled Nanofibers Elicit Potent HPV16 E7-Specific Cellular Immunity And Abolish Established TC-1 Graft Tumor, Int. J. Nanomed., 2019, 14, 8209–8219 CrossRef CAS PubMed.
- S. Li, W. Zhu, C. Ye, W. Sun, H. Xie, X. Yang, Q. Zhang and Y. Ma, Local mucosal immunization of self-assembled nanofibers elicits robust antitumor effects in an orthotopic model of mouse genital tumors, Nanoscale, 2020, 12(5), 3076–3089 RSC.
- M. M. Levine, Can needle-free administration of vaccines become the norm in global immunization?, Nat. Med., 2003, 9(1), 99–103 CrossRef CAS PubMed.
- Y. Si, Y. Wen, S. H. Kelly, A. S. Chong and J. H. Collier, Intranasal delivery of adjuvant-free peptide nanofibers elicits resident CD8(+) T cell responses, J. Controlled Release, 2018, 282, 120–130 CrossRef CAS PubMed.
- S. H. Kelly, Y. Wu, A. K. Varadhan, E. J. Curvino, A. S. Chong and J. H. Collier, Enabling sublingual peptide immunization with molecular self-assemblies, Biomaterials, 2020, 241, 119903 CrossRef CAS PubMed.
- S. H. Kelly, B. J. Cossette, A. K. Varadhan, Y. Wu and J. H. Collier, Titrating Polyarginine into Nanofibers Enhances Cyclic-Dinucleotide Adjuvanticity in Vitro and after Sublingual Immunization, ACS Biomater. Sci. Eng., 2021, 7(5), 1876–1888 CrossRef CAS PubMed.
- S. H. Kelly, N. L. Votaw, B. J. Cossette, Y. Wu, S. Shetty, L. S. Shores, L. A. Issah and J. H. Collier, A sublingual nanofiber vaccine to prevent urinary tract infections, Sci. Adv., 2022, 8(47), eabq4120 CrossRef CAS PubMed.
- X. Gong, Y. Gao, J. Shu, C. Zhang and K. Zhao, Chitosan-Based Nanomaterial as Immune Adjuvant and Delivery Carrier for Vaccines, Vaccines, 2022, 10(11), 1906 CrossRef CAS PubMed.
- X. Gao, N. Liu, Z. Wang, J. Gao, H. Zhang, M. Li, Y. Du, X. Gao and A. Zheng, Development and Optimization of Chitosan Nanoparticle-Based Intranasal Vaccine Carrier, Molecules, 2021, 27(1), 204 CrossRef PubMed.
- H. Hassan, A. I. Ali, E. M. ElDesawy and A. H. ElShafeey, Pharmacokinetic and Pharmacodynamic Evaluation of Gemifloxacin Chitosan Nanoparticles As an Antibacterial Ocular Dosage Form, J. Pharm. Sci., 2022, 111(5), 1497–1508 CrossRef CAS PubMed.
- S. G. Abonashey, H. A. F. M. Hassan, M. A. Shalaby, A. G. Fouad, E. Mobarez and H. A. El-Banna, Formulation, pharmacokinetics, and antibacterial activity of florfenicol-loaded niosome, Drug Delivery Transl. Res., 2024, 14(4), 1077–1092 CrossRef CAS PubMed.
- M. A. Obeid, T. Teeravatcharoenchai, D. Connell, K. Niwasabutra, M. Hussain, K. Carter and V. A. Ferro, Examination of the effect of niosome preparation methods in encapsulating model antigens on the vesicle characteristics and their ability to induce immune responses, J. Liposome Res., 2021, 31(2), 195–202 CrossRef CAS PubMed.
- S. Fallarini, F. Papi, F. Licciardi, F. Natali, G. Lombardi, F. Maestrelli and C. Nativi, Niosomes as Biocompatible Scaffolds for the Multivalent Presentation of Tumor-Associated Antigens (TACAs) to the Immune System, Bioconjugate Chem., 2023, 34(1), 181–192 CrossRef CAS PubMed.
- R. Mateu Ferrando, L. Lay and L. Polito, Gold nanoparticle-based platforms for vaccine development, Drug Discovery Today: Technol., 2020, 38, 57–67 CrossRef PubMed.
- H. A. Hassan, L. Smyth, N. Rubio, K. Ratnasothy, J. T. Wang, S. S. Bansal, H. D. Summers, S. S. Diebold, G. Lombardi and K. T. Al-Jamal, Carbon nanotubes’ surface chemistry determines their potency as vaccine nanocarriers in vitro and in vivo, J. Controlled Release, 2016, 225, 205–216 CrossRef CAS PubMed.
- H. A. F. M. Hassan, L. Smyth, J. T. W. Wang, P. M. Costa, K. Ratnasothy, S. S. Diebold, G. Lombardi and K. T. Al-Jamal, Dual stimulation of antigen presenting cells using carbon nanotube-based vaccine delivery system for cancer immunotherapy, Biomaterials, 2016, 104, 310–322 CrossRef CAS PubMed.
- Z. Hu, P. A. Ott and C. J. Wu, Towards personalized, tumour-specific, therapeutic vaccines for cancer, Nat. Rev. Immunol., 2018, 18(3), 168–182 CrossRef CAS PubMed.
- N. Pardi, M. J. Hogan, F. W. Porter and D. Weissman, mRNA vaccines – a new era in vaccinology, Nat. Rev. Drug Discovery, 2018, 17(4), 261–279 CrossRef CAS PubMed.
- R. S. Riley, C. H. June, R. Langer and M. J. Mitchell, Delivery technologies for cancer immunotherapy, Nat. Rev. Drug Discovery, 2019, 18(3), 175–196 CrossRef CAS PubMed.
- M. Mazza, M. Hadjidemetriou, I. de Lazaro, C. Bussy and K. Kostarelos, Peptide nanofiber complexes with siRNA for deep brain gene silencing by stereotactic neurosurgery, ACS Nano, 2015, 9(2), 1137–1149 CrossRef CAS PubMed.
- M. E. Keir, L. M. Francisco and A. H. Sharpe, PD-1 and its ligands in T-cell immunity, Curr. Opin. Immunol., 2007, 19(3), 309–314 CrossRef CAS PubMed.
- D. M. Pardoll, The blockade of immune checkpoints in cancer immunotherapy, Nat. Rev. Cancer, 2012, 12(4), 252–264 CrossRef CAS PubMed.
- L. T. Nguyen and P. S. Ohashi, Clinical blockade of PD1 and LAG3--potential mechanisms of action, Nat. Rev. Immunol., 2015, 15(1), 45–56 CrossRef CAS PubMed.
- A. Swaika, W. A. Hammond and R. W. Joseph, Current state of anti-PD-L1 and anti-PD-1 agents in cancer therapy, Mol. Immunol., 2015, 67(2 Pt A), 4–17 CrossRef CAS PubMed.
- J. Gong, A. Chehrazi-Raffle, S. Reddi and R. Salgia, Development of PD-1 and PD-L1 inhibitors as a form of cancer immunotherapy: a comprehensive review of registration trials and future considerations, J. Immunother. Cancer, 2018, 6(1), 8 CrossRef PubMed.
- F. F. Gellrich, M. Schmitz, S. Beissert and F. Meier, Anti-PD-1 and Novel Combinations in the Treatment of Melanoma-An Update, J. Clin. Med., 2020, 9(1), 223 CrossRef CAS PubMed.
- R. M. Webster, The immune checkpoint inhibitors: where are we now?, Nat. Rev. Drug Discovery, 2014, 13(12), 883–884 CrossRef CAS PubMed.
- R. Meir, K. Shamalov, T. Sadan, M. Motiei, G. Yaari, C. J. Cohen and R. Popovtzer, Fast Image-Guided Stratification Using Anti-Programmed Death Ligand 1 Gold Nanoparticles for Cancer Immunotherapy, ACS Nano, 2017, 11(11), 11127–11134 CrossRef CAS PubMed.
- Y. Fan, R. Kuai, Y. Xu, L. J. Ochyl, D. J. Irvine and J. J. Moon, Immunogenic Cell Death Amplified by Co-localized Adjuvant Delivery for Cancer Immunotherapy, Nano Lett., 2017, 17(12), 7387–7393 CrossRef CAS PubMed.
- G. Ma, N. Kostevsek, I. Monaco, A. Ruiz, B. Markelc, C. C. L. Cheung, S. Hudoklin, M. E. Kreft, H. Hassan, M. Barker, J. Conyard, C. Hall, S. Meech, A. G. Mayes, I. Sersa, M. Cemazar, K. Markovic, J. Scancar, M. C. Franchini and W. T. Al-Jamal, PD1 blockade potentiates the therapeutic efficacy of photothermally-activated and MRI-guided low temperature-sensitive magnetoliposomes, J. Controlled Release, 2021, 332, 419–433 CrossRef CAS PubMed.
- J. Dolina, S.-S. Sung, T. Novobrantseva and Y. Hahn,
In vivo delivery of lipidoid nanoparticles containing PD-L1 siRNA enhances NK and CD8+ T cell-mediated hepatic antiviral immunity (168.6), J. Immunol., 2012, 188(1 Suppl.), 168.6 CrossRef.
- W. Hobo, T. I. Novobrantseva, H. Fredrix, J. Wong, S. Milstein, H. Epstein-Barash, J. Liu, N. Schaap, R. van der Voort and H. Dolstra, Improving dendritic cell vaccine immunogenicity by silencing PD-1 ligands using siRNA-lipid nanoparticles combined with antigen mRNA electroporation, Cancer Immunol. Immunother., 2013, 62(2), 285–297 CrossRef CAS PubMed.
- Y. C. Kong and J. C. Flynn, Opportunistic Autoimmune Disorders Potentiated by Immune-Checkpoint Inhibitors Anti-CTLA-4 and Anti-PD-1, Front. Immunol., 2014, 5, 206 Search PubMed.
- A. Pedoeem, I. Azoulay-Alfaguter, M. Strazza, G. J. Silverman and A. Mor, Programmed death-1 pathway in cancer and autoimmunity, Clin. Immunol., 2014, 153(1), 145–152 CrossRef CAS PubMed.
- S. Koyama, E. A. Akbay, Y. Y. Li, G. S. Herter-Sprie, K. A. Buczkowski, W. G. Richards, L. Gandhi, A. J. Redig, S. J. Rodig, H. Asahina, R. E. Jones, M. M. Kulkarni, M. Kuraguchi, S. Palakurthi, P. E. Fecci, B. E. Johnson, P. A. Janne, J. A. Engelman, S. P. Gangadharan, D. B. Costa, G. J. Freeman, R. Bueno, F. S. Hodi, G. Dranoff, K.-K. Wong and P. S. Hammerman, Adaptive resistance to therapeutic PD-1 blockade is associated with upregulation of alternative immune checkpoints, Nat. Commun., 2016, 7(1), 10501 CrossRef CAS PubMed.
- D. Romero, Immunotherapy: PD-1 says goodbye, TIM-3 says hello, Nat. Rev. Clin. Oncol., 2016, 13(4), 202–203 Search PubMed.
- M. Kozlowski, D. Borzyszkowska and A. Cymbaluk-Ploska, The Role of TIM-3 and LAG-3 in the Microenvironment and Immunotherapy of Ovarian Cancer, Biomedicines, 2022, 10(11), 2826 CrossRef CAS PubMed.
- N. Sauer, N. Janicka, W. Szlasa, B. Skinderowicz, K. Kołodzińska, W. Dwernicka, M. Oślizło, J. Kulbacka, V. Novickij and K. Karłowicz-Bodalska, TIM-3 as a promising target for cancer immunotherapy in a wide
range of tumors, Cancer Immunol. Immunother., 2023, 72(11), 3405–3425 CrossRef CAS PubMed.
|
This journal is © The Royal Society of Chemistry 2024 |