DOI:
10.1039/D3MA00822C
(Review Article)
Mater. Adv., 2024,
5, 30-50
Metal–organic frameworks for next-generation energy storage devices; a systematic review
Received
9th October 2023
, Accepted 17th November 2023
First published on 17th November 2023
Abstract
The future of renewable energy and sustainable transportation depends on advanced energy storage technologies. However, the capacity, durability, and safety issues associated with traditional technologies are often problematic. The rapidly developing field of metal–organic frameworks (MOFs) as essential components for the development of new energy storage technologies is investigated in this study. MOFs, which include technologies like batteries, supercapacitors, and fuel cells, provide fascinating platforms for energy storage due to their distinctive structures and configurable porosities. This review describes the underlying engineering, highlights the performance advancements, and examines the difficulties still associated with the application of MOFs. It highlights the value of ongoing study and the potential for MOFs to alter our energy landscape. Overall, this study provides in-depth knowledge of MOFs in terms of energy storage potential and recent developments making them a crucial resource for academics and engineers for providing a foundation with more sustainable energy in the future.
1 Introduction
Energy, in all of its appearances, is the driving force behind all life on earth and the many activities that keep it functioning.1 For decades, the search for efficient, sustainable, and reliable energy storage devices has been a key focus in the scientific community.2 The field of energy storage has been a focal point of research in recent years due to the increasing demand for renewable and sustainable energy sources.3 Metal–organic frameworks (MOFs) are a distinct class of porous materials and in the past few years, have drawn a lot of interest from the scientific community because of their exceptional characteristics and numerous potential applications.4 These crystalline compounds, which are made up of metal ions or clusters coupled to organic ligands, have outstanding surface areas, a high degree of structural diversity, and adjustable porosity, making them the perfect choice for a variety of applications.5
Numerous methods have been discovered for modifying the structural and functional features of MOFs, which have been the target of research.6 These synthesis techniques, such as solvothermal,7–9 microwave-assisted,10–13 mechanochemical,14–16 and others, have proven essential for synthesizing MOFs with certain properties appropriate for specific uses.17 Designing MOFs with specific pore sizes, shapes, and functions is made possible by their capacity to modify the metal and organic components, which allows for the fine-tuning of their physical as well as chemical properties.18 Energy storage is one of the most promising uses of MOFs.19–21 Researchers have been exploring innovative materials and technologies in response to the global energy crisis and the increasing demand for sustainable and efficient energy storage solutions.22,23 MOFs have shown tremendous promise in this area due to their unique characteristics.19,24,25 They have been intensively researched for applications in a variety of energy storage devices such as batteries,26–28 supercapacitors,29–31 and fuel cells.32–34 Their high porosity and surface area allow for fast ion transport and high capacitance, while their structural diversity allows for the integration of active energy storage sites.35
Furthermore, MOFs may be used as outstanding electrode materials or as precursors for the production of other sophisticated materials.36 MOFs, for example, have been utilized to develop carbon-based materials,37,38 metal oxides,39,40 and metal sulfides41,42 with outstanding electrochemical performance.43 MOF design and synthesis for energy storage applications is a fast-emerging subject, with fresh discoveries and developments constantly extending the possibilities of these interesting materials.44 The purpose of this paper is to offer a complete understanding of MOF synthesis and their use in energy storage devices. We will investigate the different synthesis techniques and their effects on MOF characteristics, investigate the processes through which MOFs contribute to energy storage, and highlight some of the most recent, noteworthy advances in this sector. We hope that this analysis sheds light on the potential of MOFs in tackling the global energy crisis and inspires further research on this intriguing subject. More efficient and stable MOFs for energy storage applications are expected to be produced as synthetic methods increase and our knowledge of the structure–property linkages of MOFs deepens. The present study places particular emphasis on the advancement of energy storage devices generally referred to as ‘next-generation’ technologies. Considerable attention is devoted to the investigation of emerging technologies and their potential to revolutionize the field of energy storage. This comprehensive analysis involves advanced batteries, supercapacitors, and novel gas storage systems, all of which show promise in shaping the future of this fundamental field.
2 Properties of MOFs
MOFs have a wide range of tunable properties, including structural tuneability, high porosity, very large specific surface area, and superior conductivity as depicted in Fig. 1. They have unique functional materials with these properties that can be employed in a variety of applications.45 These qualities can be increased even further based on the structure units used in the MOF, rather than the stability that distinguishes it from other porous materials. MOFs can also be utilized to create other materials, such as carbon with a controlled porosity structure, as well as a variety of MOF-based composites with superior properties to the original material.46 MOFs have gained recognition for their ability to be customized using metal and organic ligands to build the structure that allows them to function efficiently in the applications they were designed for. There are several synthetic methods for altering the framework's chemistry, stability, dimension of particles, and flexibility.47 To enhance the attributes of MOFs, certain metal vertices and ligands within the framework can be interchanged, modified, or eliminated.48 MOF rigidity would be especially significant in sustaining structural integrity in some devices where the active element undergoes to volume change. Mechanical properties can also be improved by constructing frameworks with varied metal nodes, incorporating flexible linkers, and altering crystal sizes.45
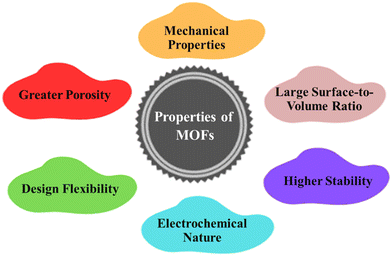 |
| Fig. 1 Distinguishing properties of MOFs. | |
MOFs provide a large number of uniformly distributed active sites in comparison to conventional materials, and the quick flow of electrolyte ions is facilitated by their distinct pore topologies. Because of this, MOFs are great options for supercapacitor materials.49 With repeated cycles of charge and discharge, MOF pore structures tend to irreversibly collapse.50 As a result, the electrode's specific surface area drastically decreases, reducing the electrolyte's conductivity and ion site diffusion. The pore structure and supercapacitor efficiency of MOFs are significantly influenced by their specific surface area (Fig. 2). Many charge storage sites are provided by a large surface area, yet in MOFs where pseudo-capacitance predominates, this may threaten stability.51 The utilization of porous materials has become more prevalent recently in the area of energy storage due to the advantages associated with abundant reactive sites and enhanced mass transfer.
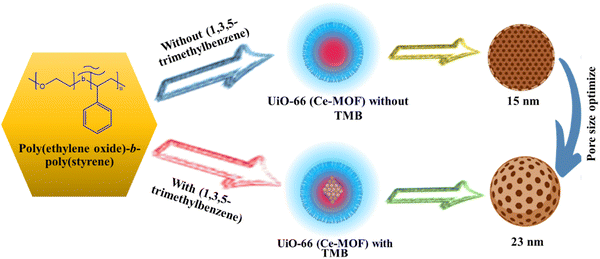 |
| Fig. 2 Porosity of Ce-MOFs optimized using 1,3,5-trimethylbenzene.52 | |
Ionic and electric conduction play a pivotal role in enhancing the performance of electrochemical devices. These devices often face challenges like large overpotentials due to high internal resistances arising from sluggish ionic motions.53 These issues lead to diminished power output and performance limitations. The utilization of novel materials for charge transport applications and the advancement of synthetic methods to enhance ionic and electric conductivity in MOFs provide valuable insights for material research as shown in Fig. 3. Scalability and processing capacity are vital considerations in the synthesis of metal–organic frameworks. To enhance scalability, the utilization of more affordable metal resources, such as metal mineral salts, oxides, hydroxides, nitrates, carbonates, along with organic binders, can yield further cost reductions. Moreover, the application of microwave radiation expedites reactions, consequently enhancing scalability.54
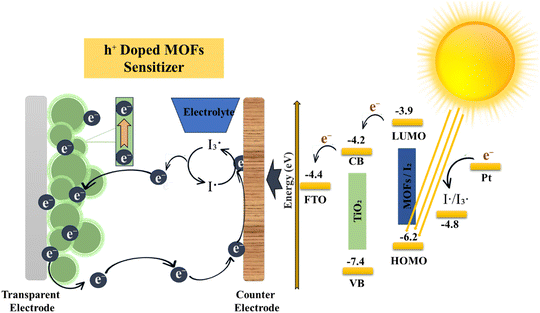 |
| Fig. 3 Electroactive and conductive nature of MOFs.55 | |
The capacity of MOFs to preserve their initial long-range organized structure in a certain chemical environment is referred to as stability. The reliability of frameworks is influenced by the strength of coordinate bonds, with increased bond strength leading to greater overall framework strength.56 To achieve durability in metal–organic frameworks one approach is to create a synthesis method by pairing hard bases with high-valent metal ions, or soft bases with bivalent metallic ions. Additionally, the operational conditions, specifically pH levels, can significantly influence framework solidity.57 External and internality stability of materials concerned with adsorption media surrounding it and the formation of metal ligand bonds respctively.58 The various factors involved in the stability of the MOFs are presented in pictorial form in Fig. 4.
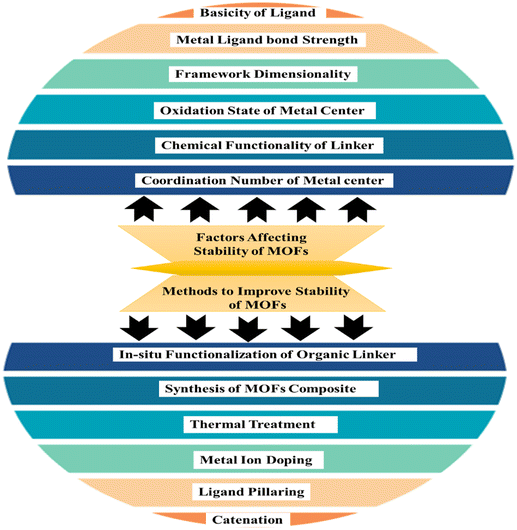 |
| Fig. 4 Factors affecting stability and methods to improve the stability of MOFs.59 | |
3 Sustainability and environmental impact
MOFs can considerably increase the efficacy of energy storage due to their enormous surface area and porosity. This enhances the absorption and storage of gases such as hydrogen and methane. For applications such as fuel cells and natural gas vehicles, efficient gas storage is essential, and MOFs can improve the energy density and performance.50 The synthesis and utilization of metal–organic frameworks have the potential to result in a noteworthy reduction in greenhouse gas emissions when contrasted with conventional materials. In the field of natural gas storage, it is worth noting that MOFs have emerged as an exciting opportunity for advancing the development of lightweight and high-capacity storage systems, particularly for vehicles.60 By exploiting the unique properties of MOFs, it becomes possible to facilitate the development of portable storage systems that possess a remarkable capacity to store natural gas while maintaining a reduced weight profile. This, in turn, has the potential to contribute significantly to the reduction of emissions by encouraging the adoption of cleaner fuels in various transportation applications.61 MOF fabrication requires energy-intensive procedures, especially in the process of activation and solvent removal. The utilization of this particular material may potentially yield a greater carbon footprint in comparison to conventional materials. The reduction of energy intensity in the synthesis of metal–organic frameworks can be achieved through the utilization of sustainable energy sources and the optimization of manufacturing techniques.62 Numerous methodologies for the synthesis of MOFs necessitate the utilization of organic solvents, thereby promoting adverse environmental implications. The advancement of solvent-free or environmentally friendly solvent synthesis pathways constitutes a pivotal domain of investigation with the objective of addressing this concern.63 The utilization of green synthesis methods has been shown to effectively mitigate the environmental impact associated with the production of metal–organic frameworks. By employing these environmentally friendly techniques, the overall carbon footprint and ecological consequences of MOF synthesis can be significantly reduced.14 The use of certain metal–organic framework precursors necessitates the inclusion of rare or expensive metals, thereby developing doubts regarding the accessibility of resources and the sustainability of the environment. In response to this clicking matter, diligent researchers are currently engaged in the investigation of alternative precursors and metal sources. This endeavor involves the exploration of recyclable materials and the utilization of abundant elements such as iron and aluminum.64
4 MOFs vs. traditional materials: a comparative analysis
4.1 Carbon capture and storage (CCS)
Carbon capture and storage (CCS) stands as a highly beneficial area for the utilization of metal–organic frameworks in the context of energy storage. They have exhibited exceptional promise in the realm of capturing carbon dioxide (CO2) from industrial flue gases.65 In contrast to conventional amine-based sorbents, metal–organic frameworks have been found to possess superior selectivity, capacity, and cyclic stability. The aforementioned observation implies that a diminished quantity of MOF materials suffices to achieve an equivalent level of CO2 capture, thereby resulting in a reduction in material consumption and the associated environmental implications.66 Due to their high surface area and superior adsorption performance, zeolite (ZSM-5) and a copper metal–organic framework (Cu-MOF) are chosen as adsorbents. The maximum particle size distribution and area for ZSM-5 were in the range of 25–66 m and 1.7–442 m2 respectively. Whereas for Cu-MOF they were 102–149 m and 1.7–1400 m2, respectively.67 The results demonstrate that Zn-MOF works well in the low-pressure system with a higher CO2 adsorption capability. Under ideal circumstances, the CO2 adsorption of Zn-MOF was 145.1.68 In order to create effective CO2 adsorbents, a combination of Cu-BTC framework with porous carbon materials such as ordered mesoporous non-activated carbon (OMC), ordered mesoporous activated carbon (AC), and nitrogen-containing microporous carbon (NC) was used to form a NC–Cu-BTC composite with the maximum CO2 capacity, measuring 8.24 and 4.51 mmol g−1 under 1 bar at 0 and 25 °C, respectively.69 The lowest parasitic energy (PE) for postcombustion CO2 collection has been observed in a nickel isonicotinate-based ultramicroporous MOF. Although a PE of 655 kJ kg−1 CO2 is less than that of Mg-MOF-74, the highest performing material that was previously been reported.70 In addition to the oxygen stored on the Ce(III)/Ce(IV) centers, the Pd nanoparticles supported on the Ce-MOF also store oxygen in the form of a thin palladium oxide layer at the particle–support interface. At 373 K, the CO oxidation process can cause the release of oxygen from these reservoirs. The Pd/CeMOF exhibits a substantial CO2 absorption of 3.5 mmol g−1 at lower temperatures (273 K).71
4.2 Hydrogen storage
Metal–organic frameworks have drawn significant attention in the discipline of hydrogen storage for fuel cell applications. The conventional techniques employed for hydrogen storage, namely high-pressure tanks and cryogenic liquefaction, have been subject to investigation due to their inherent safety risks and inappropriate energy efficiency.72 Metal–organic frameworks present an appealing option as they offer a more energy-efficient and safer alternative for hydrogen storage. This is achieved through their unique ability to facilitate hydrogen storage at reduced pressures and moderate temperatures. By harnessing the remarkable properties of MOFs, the energy industry can potentially overcome the challenges associated with conventional hydrogen storage methods.73 The data showed that two novel zinc(II)-pyrazolate metal–organic frameworks have very excellent chemical and thermal stability. Most importantly, Zn(1,4-benzenedi(4-pyrazolyl)) demonstrates an excess H2 uptake of 4.7 wt% at 40 bar and 77 K with a BET surface area of 1710 m2 g−1.74 Based on the binuclear [Sc2(μ2-OH)(O2CR)4] building block, the metal–organic frameworks NOTT-400 and NOTT-401 were synthesized and characterized; the framework NOTT-401 exhibits a BET surface area of 1514 m2 g−1 and a total H2 uptake of 4.44 wt% at 77 K and 20 bar.75 The MOF hydrogen delivery capabilities between 100 bar/77 K and 5 bar/160 K were determined using molecular modeling on a vast and structurally varied collection of 13512 possible MOF structures based on 41 distinct topologies. The greatest volumetric deliverable capacity (compressing hydrogen to 700 bar at ambient temperature) was 57 g L−1 of MOF, above the 37 g L−1 of tank of the existing method.76 The usable volumetric H2 storage capacities of the metal–organic frameworks containing Co, Ni; dobdc4- = 1,4-dioxido-1,3-benzenedicarboxylate, which are known to have open metal cation sites that strongly interact with H2, were assessed over a range of near-ambient temperatures relevant to on-board storage, with a useable volumetric capacity between 100 and 5 bar of 11.0 g L−1 at 25 °C and 23.0 g L−1 with a temperature swing between 75 and 25 °C. Ni2(m-dobdc) was discovered to be the best physisorptive storage material based on adsorption isotherm data.77 In order to create effective adsorbents for hydrogen gas, trimesic acid, a very electron-deficient and polar molecule, is produced as an organic ligand. This results in nanostructured Zr(IV) metal organic frameworks (MOFs-808) with excellent stability. The improved MOF-808's hydrogen storage capacity at 4 MPa is 7.31 wt% at 77 K, which is near to the maximum hydrogen storage capacity specified.78
4.3 Natural gas storage
In the subject of natural gas storage for vehicle applications, it is worth noting that metal–organic frameworks present notable advantages in the form of weight reduction and enhanced volumetric capacity. The utilization of compressed natural gas (CNG) as an alternative fuel source for transportation has been hindered by the weight and volumetric constraints associated with traditional CNG storage systems.79 These limitations have impeded the widespread adoption of CNG as a viable option for powering vehicles. Metal–organic frameworks, renowned for their exceptional storage capabilities, have the potential to significantly mitigate the carbon footprint associated with compressed natural gas (CNG) vehicles by facilitating the utilization of smaller and lighter storage tanks.80 MIL-101(Cr) MOF (metal–organic framework) production, characterization, and property assessment for CH4 adsorption was carried out. The total volumetric absorption of CH4 on MIL-101(Cr) MOF is reported to be about 30 cm3 cm−3 at 5 bar, 150 cm3 cm−3 at 35 bar, and 215 cm3 cm−3 at 65 bar at 298 K. It has been discovered that MIL-101(Cr) exhibits a high CH4 delivery or working capacity using LNG-ANG coupling.81 Five novel zinc-based metal–organic frameworks (MOFs) were synthesized and also have crystal structures and methane adsorption capabilities. All of these MOFs are made up of benzene-1,3,5-tri-acrylate, or BTAC, and secondary building units (SBUs) of Zn4O(CO2)6. With a volumetric working capacity (desorption at 5 pressure) of 203 cm3 cm−3 at 80 bar and 298 K, MOF-905 is one of the best-performing methane storage materials, rivaling the value of HKUST-1 (200 cm3 cm−3), the industry-standard compound for methane storage in MOFs.82 For methane storage with a high deliverable capacity, the flexibility and stability of hydroxy-functionalized MIL-53(Al) MOFs were explored. In addition to effective stacking, the location of the hydroxy groups encourages weak hydrogen bonding, which regulates the breathing behavior of these MOFs. In MIL-53(Al)–OH at 65 bar and 298 K (regarding crystallographic density), a promising deliverable capacity of 164 v/v was obtained. This value is near to the record of 197 v/v by Co-BDP.83 Purified multi-walled carbon nanotubes (MWCNTs) have been added in situ during the production of MIL-53-Cu to create a composite MOF material called MIL-53-Cu with MWCNTs. At 298 K and 35 bar, it was shown that the methane sorption capabilities of MIL-53-Cu increased from 8.52 to 13.72 mmol g−1.84 In a different study, polyvinyl alcohol (PVA) nanofibers were combined with a tantalum(V) metal organic framework (Ta-MOF) nanostructure to create an electro spun porous composite that served as a new CH4 adsorbent. The greatest methane adsorption may be reached at 24.40 °C and 3.70 bar in 23.60 min, according to the results of response surface methodology (RSM) optimization.85 A comparison of the MOFs with other conventional materials has been tabulated in Table 1. It is depicted in the table that MOFs have great potential for energy storage as compared to metal oxide, polymer or carbon-based materials.
Table 1 Comparison of the MOFs and other conventional materials for energy storage
Material |
Porosity |
Surface area (m2 g−1) |
Conductivity |
Charge/discharge rate |
Energy density |
Cyclability |
Environmental impact |
Ref. |
Metal–organic frameworks (MOFs) |
High porosity due to a well-defined structure |
Extremely high surface area |
Generally low electrical conductivity |
Typically slow charge/discharge rates |
Moderate energy density |
Can suffer from low cyclability due to structural instability |
MOF production can have high a environmental impact |
86–89
|
Traditional metal oxides |
Limited porosity |
Moderate to high |
Insulating or semiconducting |
Varies depending on material |
Moderate to high |
Generally good cyclability |
Varies depending on material |
90 and 91
|
Conducting polymers |
Low porosity |
Moderate to low |
High electrical conductivity |
Fast charge/discharge rates |
Moderate to low |
Varies depending on material |
Moderate environmental impact |
92 and 93
|
Carbon-based materials |
Variable porosity based on structure and activation methods |
High surface area for activated carbons |
Varies from insulating to highly conducting |
Moderate to fast charge/discharge rates |
Moderate to high energy density |
Generally good cyclability |
Low environmental impact |
94 and 95
|
5 Energy storage applications of MOFs
Metal–organic frameworks, which are made up of metal ions/clusters and organic ligands, have recently emerged as one of the most popular porous crystalline inorganic–organic materials.96 MOFs have already been used in a variety of applications because of their unique compositional and structural properties. Significant effort has lately been directed toward the fabrication of MOF micro/nanomaterials with adjustable morphologies for improved performance in a variety of applications.97 Furthermore, due to their customized compositions and porous architectures, MOFs can serve as effective models and precedents in high-temperature operations to build nanostructures with tunable pore topologies, huge specific surface area, great chemical stabilities, and a wide range of functions.98 To cope with this, as well as environmental concerns, researchers have focused on designing MOFs for clean energy applications such as lithium-based batteries, supercapacitors, and hydrogen production and storage.99 Greater charge/discharge rates, higher theoretical capacities, and improved electronic stability are all required for energy storage systems to operate at peak output effectiveness and performance.100 Batteries and supercapacitors are more practical and cost-effective energy storage technologies. The most significant component of development is energy storage, and the uses of renewable sources of energy are essential in our daily life. MOF-based electrodes have huge potential and several benefits, such as a large specific surface area, highly changeable porosities, efficient active sites, and rapid response.101
5.1 Lithium-ion-based batteries (LIBs)
Due to their high storage capacity, long lifespan, high energy density, light mass, excellent cycle performance, and environmental friendliness, rechargeable lithium-ion batteries are essential energy storage devices.102 During the charging process, lithium ions leave the cathode and enter the anode as they move through the electrolyte, storing energy; when the lithium ions return to the cathode, the (LIBs) discharge.103 The very porous structure of MOFs may result in massive lithium ion accommodation and adequate lithium ion routes. MOFs have a three-dimensional structure that allows metal cations to be accommodated in interstitial space.100 Significant attention has been paid to the use of metal organic frameworks (MOFs) as innovative and promising electrode materials in lithium-ion batteries (LIBs) as presented in Fig. 5. A straightforward solvothermal approach for the fabrication of Fe-MOF/reduced graphene oxide (RGO) composites. The synthesized Fe-MOF/RGO (5%) composite exhibits outstanding Li storage when utilized as anode materials for LIBs, with a reversible capacity of 1010.3 mA h g−1 after 200 cycles and a good rate performance.104 The metal–organic frameworks (MOFs) NENU-506 and NENU-507, two new isostructural polyoxometalate-based MOFs with diamond topology, were produced hydrothermally. Notably, NENU-507, used as an anode material in lithium-ion batteries, demonstrated a high reversible capacity of 640 mA h g−1 after 100 cycles.105 A foam-like CoO@N,S-codoped carbon hybrid composite was made using an N,S-containing precursor that was first synthesized and described. Within 500 cycles, the specific capacity can maintain a constant value of 809 mA g−1 at a current of 1000 mA g−1.106 A successful method has been developed for producing silica-based anodes for lithium-ion batteries (LIBs) beginning with the precursor zeolitic imidazolate framework-67 (ZIF67)/mesopores silica (mSiO2). The engineered hybrid silica-based electrode (EHSiE), which has a high reversible capacity of 410 mA h g−1 at 5 A g−1vs. Li/Li+ in the LP30 electrolyte, has outstanding stability for 1000 cycles.107 Li-NTA (2-nitro terephthalate) s-block MOFs synthesised using a scalable microwave-assisted method and then converted to carbonaceous material (Li-NTA-C) for electrochemical energy storage (EES) applications as an anode for Li-ion batteries with significant low-rate capacities and cycling stability are shown in Fig. 5.108
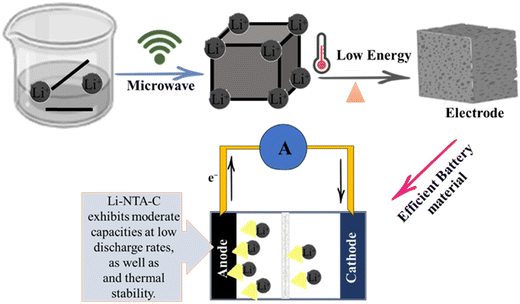 |
| Fig. 5 The application of MOFs in lithium ion batteries.108 | |
5.2 Lithium–sulfur batteries (LSBs)
LSBs have gained attention due to their high specific capacity and energy density which are much higher than the commercialized LIBs.109 Commercialization of LSBs is problematic due to huge gaps between theoretical and real capacity, energy density, and poor cycle stability; nevertheless, the use of plentiful and non-toxic sulfur may reduce battery manufacture costs.110 LSBs are a form of secondary battery with negative electrode lithium and positive electrode sulfur that has a high specific capacity of 1675 mA h g−1 and an energy density of up to 2600 W h kg−1. Because the sulfur element is environmentally favorable, found in abundance on earth, and affordable, LSBs are one of the most important next-generation secondary batteries.111 When paired with conductive carbon nanotubes (CNTs), the antiferroelectric perovskite dimethyl ammonium zinc formate MOFs serve as a potent molecular filter to reduce polysulfide (PS) migration in lithium–sulfur batteries (LSBs). With a sulfur loading of 5 mg cm−2, the hybrid MOF based electrode provides an initial high specific capacity of 1260 mA h g−1 at 0.05 °C and 1007 mA h g−1 at 0.1 °C with just 0.07% deterioration after 120 cycles.112 The rate capability of LSB based on a hierarchically porous TiO2–S cathode exhibits a considerably improved specific capacity and coulombic efficiency. Due to the Ti–S chemical bonding occurring during the charge/discharge process, the diffusion coefficient of Li-ions significantly increases and is about 17 times greater than that of a fresh TiO2–S hybrid cathode.113 By annealing and phosphorizing Ni-ZIF-67 precursor at high temperatures, Ni/Co bimetallic phosphides were enclosed in a nitrogen-doped dual carbon conductive network (NiCoP@NC). With an initial capacity of 1083.4 4 mA h g−1 at 0.5 °C and exceptional cycle stability with a capacity degradation rate of just 0.09% per cycle for 300 cycles, the cells demonstrated excellent performance when utilized as a modified separator for LSBs.114 The iron single-atom (FeSA-CN) catalyst is decorated on the nitrogen-rich, metal organic framework (MOF)-derived carbon nanocage to initiate the surface-mediated reaction of LiPSs. The FeSA-CN/S electrode produced a specific capacity of 1123 mA h g−1 at 0.2 °C and showed an exceptional rate performance of 605 mA h g−1 at 4.0 °C with an ultralow capacity fading rate of 0.06% each cycle for 500 cycles.115 A schematic presentation of the lithium sulfur based battery is presented in Fig. 6. The use of nickel-based hexa-iminotriphenylene MOFs, with an ordered microporous and a 2D structure with a large specific surface as a separator, not only increased the overall conductivity but also had a strong absorption capacity for lithium polysulfides. Fig. 6 depicts the Ni3(HITP)2/PP production process which is used as a separator in lithium sulfur batteries.116
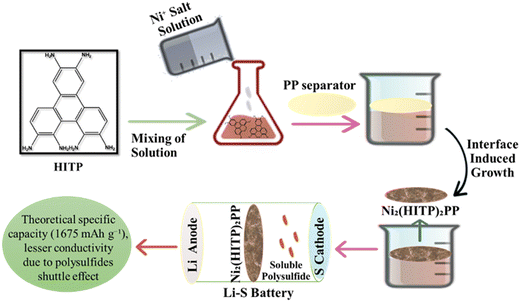 |
| Fig. 6 The application of MOFs in lithium sulfur batteries.116 | |
5.3 Lithium–air batteries (LABs)
Because of their greater energy density, lithium-ion batteries (LABs) with an oxygen cathode and lithium metal anode show enormous potential as yet another set of advantageous LIB alternatives,117 as shown in Fig. 7. As an example, Co3O4 carbon nanofiber composites made from ZIF-9 by electrospinning and thermal treatment act as LAB cathodes and have exceptional mechanical flexibility, which explains their prospective use in energy storage systems for electronics.101 According to electrochemical characterizations, mesopores in MCu-BTC increased the discharging capacity (approximately 7000 mA h g−1) of Co-10/MCu-BTC in an aprotic Li–air battery because they allowed for electrolyte infiltration for both mass and charge transfer and gave more space to store the discharge product.118 Electrospinning and post-thermal processing were used to create a self-standing, binder-free ZIF-9 Co3O4/carbon nanofiber composite for use as the cathode in a non-aqueous Li–air battery. Co3O4/carbon nanofiber composites have an initial discharge capacity of more than 760 mA h g−1, which is much larger than the initial discharge capacity of pure carbon nanofiber (72 mA h g−1).119 A conductive metal organic framework (c-MOF) has an exceptional property that encourages the formation of nanocrystalline Li2O2 with amorphous areas. For large capacity (1000–2000 mA h g−1), direct charge transfer provides a minimal charge potential of 3.7 V at high current densities (1–2 A g−1) sustained for a long cycle life (100–300 cycles).120 Additionally, the produced UiO-67-Li@rGO aerogel demonstrates continuous and plentiful O2 diffusion channels as well as Li+/e transfer channels. The solid-state Li–O2 battery accomplishes suppression of anode dendrite development, resistance to air-corrosion, and existence of numerous low-impedance wetting interfaces including anode/electrolyte and electrolyte/cathode by making use of the special chemical features of the UiO-67-Li SSEs layer. The solid-state Li–O2 battery benefits from this clever design by having a low overpotential (0.8 V), improved rate capability, and consistent cycling life of 115 cycles.121 The synthesis of hollow N-doped porous carbon sphere-structured atomically distributed Co–N–C catalysts has been achieved, as has the design of aggregate fruit electrocatalysts for Li–O2 batteries. Because of the strong metal-support interactions, this unique structure produces more stable Co SACs as shown in Fig. 7.122
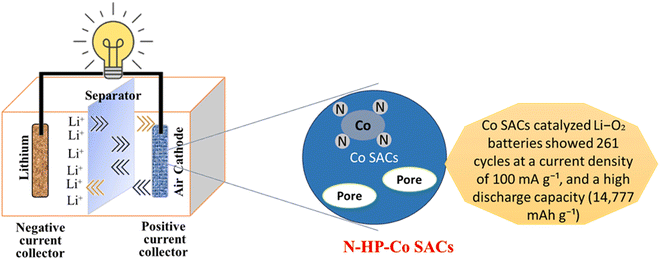 |
| Fig. 7 The application of MOFs in lithium air batteries.122 | |
5.4 Sodium-ion batteries (SIBs)
SIBs have vast applications in the development of electric vehicles and consumer electronics due to their low cost and globally high demand. The sodium ion battery in which MOF is being used as an electrode is presented in Fig. 8. SIBs are gaining importance as they can be used even at room temperature without any problem.123 Moreover, sodium is very abundant with low extraction costs, which is advantageous over LIBs and in this way, SIBs are promising alternatives to LIBs.124 SIBs are characterized by the movement of sodium ions, whereas LIBs involve the migration of lithium ions. However, it is important to note that despite this distinction, the fundamental components and overall structure of SIBs closely resemble those of LIBs.125 Porosity must be introduced into materials to increase the better cycle life of SIBs. Through an easy and practical process called ion exchange, a new calcium metal organic framework has been successfully synthesized. Calcium terephthalate (C8H4CaO4) as-prepared displays a sufficient voltage plateau at 0.5 V for sodium-ion storage and offers a specific capacity of 235.2 mA h g−1 at a current density of 0.2 A g−1.126 Ni-doped Co/CoO/N-doped carbon (NC) hybrids were synthesized employing bimetallic Ni-Co-ZIF as the initial precursor. The Ni-doped Co/CoO/NC hybrid demonstrated a good rate performance when used as an anode material for sodium-ion batteries, with a high discharge capacity of 218 mA h g−1 at a high current density of 500 mA g−1, and good cycling stability, as a high discharge capacity of 218.7 mA h g−1 can be retained after 100 cycles at 500 mA g−1, corresponding to a high capacity retention of 87.5%.127 By enclosing nanosized amorphous red P in a nitrogen-doped microporous carbon matrix (referred to as P@N-MPC) generated from zeolitic imidazolate framework-8 (ZIF-8). The P@N-MPC composite exhibits an increased rate capacity (450 mA h g−1 at 1 A g−1 after 1000 cycles with a very low capacity fading rate of 0.02% per cycle) when used as an anode for NIBs and a high reversible specific capacity of 600 mA h g−1 at 0.15 A g−1.128 The resultant Bi–Sb alloy nanoparticles synthesized from MOF-836 have homogeneous particle sizes of 30 nm, and the amount of Sb in MOF-836 was adjusted to accurately determine their composition. The nanosized MOF-836 alloys showed remarkable cycling performance, a capacity retention of 180.9 mA h g−1 at 1 A g−1 over 1000 cycles with a capacity loss of just 0.055% each cycle, and a specific capacity of 259.8 mA h g−1 at 200 mA g−1 after 500 cycles.129 Metal–organic frameworks (MOFs) were synthesized and utilized as an electrode for an aqueous sodium-ion rechargeable battery (ASIB). MOFs are crystal structure of metal hexacyanometallate (NaP[R(CN6)]), whereas, P and R are transition metals as shown in Fig. 8.130
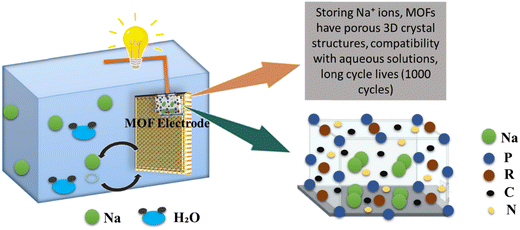 |
| Fig. 8 The application of MOFs in sodium ion batteries.130 | |
5.5 Zinc-based batteries (ZIBs)
Zn-based batteries have shown a higher probability for use in future energy storage devices for handheld electronic devices due to sufficient Zn sources, low cost, high safety, and excellent aqueous electrolyte compatibility.131 There is also great interest in ZIBs like for LIBs because of the low redox potential of Zn metal and chemical stability is developed (Fig. 9). Aqueous electrolytes provide an adaptable option for ZIBs due to their minimal toxicity and mild impact on the surrounding system.132 The important feature of ZIBs is the manufacturing of cathode materials with suitable capacity and stability.133 For example, V-MOF-derived V2O3/C and V2O5/C are used as intercalation cathodes for aqueous zin-ion batteries. The V-MOFs have distinctive channels, suitable pore size distribution, improved conductivity, and electrochemical performance. Zn/a-V2O5@C showed a rate performance that was maintained at 72.8 mA h g−1 with a current density of 200 A g−1 with a 2000 cycling performance at 40.0 A g−1.134 A typical V-MOF (MIL-47) product is successfully created using a single hydrothermal process, and its one-dimensional, layered nanorod-like structure is examined. The aqueous MOF-Zn battery, which has a high initial specific capacity of 320 mA h g−1 at 0.1 A g−1 and a good rate capability and cycling performance, uses the V-MOF product as the cathode material.135 MnO/C@rGO composites are created using an in situ process that combines a one-step solvothermal approach developed from MOFs by calcinating at 570 °C. It can sustain a discharge capacity of 170.6 mA h g−1 after 300 cycles at 500 mA g−1 when used as a cathode for AZIBs, according to subsequent evaluation. Importantly, it still yields a reversible capacity of up to 110.1 mA h g−1 even when the current density reaches up to 2.0 A g−1.136 ZnVO-800 is a brand-new cathode material made of a V2O3/V3O5/Zn2VO4@NC composite with a hierarchical structure and heterojunction that was created by self-sacrificing the zeolitic imidazolate framework-8 (abbreviated ZIF-8). Particularly, for aqueous zinc ion batteries at an initial discharge of 0.5 A g−1, the activated electrode's reversible capacity can reach up to 314.0 mA h g−1.137 Through the use of a metal–organic framework template, a novel and highly reversible Mn-based cathode material with a porous framework and N-doping (MnOx@N-C) is synthesized. It shows superior performance to most reported ZIB cathode materials, exhibiting a high capacity of 305 mA h g−1 after 600 cycles at 500 mA g−1, and maintaining a feasible capacity of 100 mA h g−1 at a relatively high rate of 2000 mA g−1 with long-term cycling of up to 1600 cycles.138 The cathode material for aqueous rechargeable zinc ion batteries using Cu3(HHTP)2 was a two-dimensional (2D) conductive metal–organic framework (MOF) that also offers high diffusion rates, low interfacial resistance and allows the Cu3(HHTP)2 cathode to use the intercalation pseudo-capacitance process as shown in Fig. 9.139
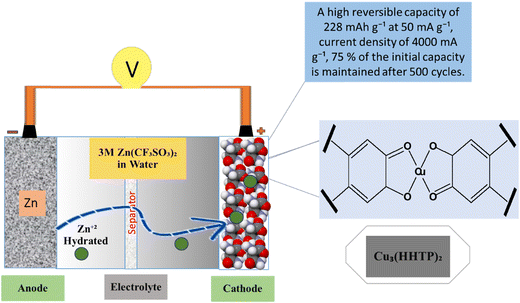 |
| Fig. 9 The application of MOFs in zinc ion batteries.139 | |
5.6 Potassium-ion batteries (KIBs)
KIBs have shown their advantages of high energy and power density at low cost since 2004.140 Moreover, potassium is one of the most common elements on the planet, accounting for about 2.1–2.4% of the earth's crust. It is important to use those cathode materials which can manage overweight sized K ions because generous sized K ions may cause KIBs to fail.141 For the extended cycle life anode of PIBs, new ultrathin carbon film@carbon nanorods@Bi MOFs nanoparticle materials were created. The MOF-based anodes demonstrate a remarkable capacity of 425 mA h g−1 at 100 mA g−1 and a capacity degradation of 0.038% per cycle over 600 cycles because to their distinctive structure. The capacity degradation is as low as 0.036% per cycle over 700 cycles, even at a greater current density of 1000 mA g−1.142 Investigations were conducted on a metal organic framework-5 (MOF-5) based high-temperature PIB with extremely high stability. The PIBs have initial discharge specific capacities of 1183 and 210 mA h g−1 at a current density of 200 mA g−1, respectively, at a fixed working temperature of 62.5 °C, and have a specific capacity of 160 mA h g−1 with a low decay of 0.15% per cycle over 150 cycles.143 By electrospinning a Zn-based zeolitic imidazolate framework (ZIF-8), ultrafine ZnSe nanocrystals were effectively incorporated into a new N-doped porous carbon nanofiber, which was then thermally processed to provide an improved anode material for KIBs. With a reversible capacity of 270 mA h g−1 at 0.5 A g−1 and a high-rate capacity of 139 mA h g−1 at 2.0 A g−1, the 1D porous nanostructured electrodes demonstrated outstanding long-term cycling stability for 1000 cycles when evaluated as anodes for KIBs.144 An anode material for PIBs made of a cobalt(II) terephthalate-based multilayer MOF with a high reversible capacity of 188 mA h g−1 after 600 extended cycles at 1 A g−1, the extraordinary potassium storage performance of L-Co2(OH)2BDC is shown, highlighting the significant advantages of MOFs as better potassium storage anodes.145
5.7 Magnesium-ion batteries (MIBs)
Due to their greater stability, divalent character, and high melting point, MIBs will be used frequently in the future as electrical energy storage devices. MIB abundance is 104 times greater than lithium and the movement of solid-state transport into an inorganic cathode material is slow which results in a poor reversible capacity and less production of power.146 To reduce these difficulties, the cathode material must be mesoporous and nanostructured. Enormous efforts have been made recently to develop the cathode materials for MIBs. Bimetallic metal–organic frameworks were effectively constructed for cubic spinel MgMn2O4 cathode materials using this technique. MgMn2O4 progressively undergoes a self-activation effect during cycling, which is essential for enhancing the kinetics of Mg2+ ions in the host spinel structure. The MgMn2O4 cathode is given an evident platform at 2 V vs. Mg/Mg2+ in complete cells as a result of this activation, which offers fresh perspectives for creating high-performance Mg-ion battery cathode materials.147 Due to its low price, high volumetric energy density, and minimal danger of dendrite development, magnesium batteries are attractive contenders for post-lithium energy storage systems. A Metal–organic framework (MOF) structure called UiO-66 that has been impregnated with magnesium bis[(trifluoromethyl)sulfonyl]imide in 1-ethyl-3-methylimidazolium bis[(trifluoromethyl)sulfonyl]imide, an ionic liquid. At room temperature, it is possible to reach a comparably high conductivity of 5.7 10−5 S cm−1.148 Through the use of a sulfurization technique based on MOF, we can create an in situ carbon-encapsulated cuprous sulfide (Cu2S@C) composite and evaluate its electrochemical performance as a displacement reaction cathode for hybrid Mg2+/Li+ batteries. The Cu2S@C composite exhibits 399.2 mA h g−1 discharge capacity and the capacity is maintained at roughly 150 mA h g−1 at 0.05 °C after 50 cycles in the nucleophilic hybrid electrolyte thanks to the intrinsic property of Cu2S, the well-defined hybrid porous structure, and the carbon encapsulation derived from MOFs.149 The benefits of magnesium batteries include a high volumetric energy density, and dendrite-free plating and stripping of magnesium anodes. The Mg–S discharge capacity reaches a maximum of 600 mA h g−1 during the first cycle and remains stable for at least 200 cycles at 400 mA h g−1.150
5.8 Aluminum air batteries (AABs)
Aluminum air batteries appeared as useful energy storage devices with an energy density of 8100 W h k g−and a discharge capacity of 2980 mA h g−1. The electrical energy is produced in AABs when aluminum (Al) reacts with oxygen at the anode, while the reduction of oxygen occurs at the cathode.151 AABs are more capable than LIBs because the emission of electrons in AABs is three times greater than in LIBs. The drawbacks of AABs are self-corrosion and slowed electrochemical reactions due to the by-products formation.100 Fe2O3@-rGO, CoFe2O4@-rGO, and Co3O4@-rGO are used as electrocatalysts in AABs152 as shown in Fig. 10. CoFe2O4@-rGO showed a stable discharge cycle with a good columbic efficiency of 99.6%. Therefore, MOFs and their composites play a vital role in the development of aluminum–air batteries.153 A new, high-performance Co3O4@MWCNTs polyhedron composite cathode was studied in a rechargeable aluminum-ion battery (RAIB) system. MOFs can deliver an initial discharge capacity of approximately 266.3 mA h g−1 thanks to the well-defined morphology of MOF-derived Co3O4 driver reversible specific capacity near 125 mA h g−1 at 100 mA g−1 over 150 cycles.154 The Co-MOF exhibited a superior reversible rate capacity (86 mA h g−1 at 5.0 A g−1) and remarkable cycling performance (125 mA h g−1 after 1000 cycles at 2.0 A g−1).155 Co3O4, Fe2O3 and CoFe2O4 modified with rGO were proposed as affordable metal–oxide cathodes for AIBs. The Co3O4@rGO outperformed the most recent state-of-the-art cathode material described in the scientific literature in terms of both capacity and longevity. CoFe2O4@rGO also demonstrates sensible electrochemical capabilities and an incredibly steady charge/discharge process with a superb coulombic efficiency of 99.6%.152 More crucially, ZIF-67 nanocrystals may be used as precursors for the quick and easy carbonization/tellurization process that yields CoTe2 nanoparticles@nitrogen-doped porous carbon polyhedral composites (CoTe2@N-PC). Even at high discharge cut-off voltages (voltage window: 0.5–2.3 V), CoTe2@N-PC can generate an extremely high reversible initial capacity of 635.8 mA h g−1 at a current density of 200 mA g−1.156 The acidic treatment of Fe-MOF resulted in yolk–shell hollow Fe-MOF spheres with many active sites to promote electrolyte penetration. The dopamine was coated and calcined to increase the electrode's electron conductivity and regulate volume expansion during cycling. The yolk–shell MOF-derived FeS2@C was produced after sulfurization as shown in Fig. 10.157
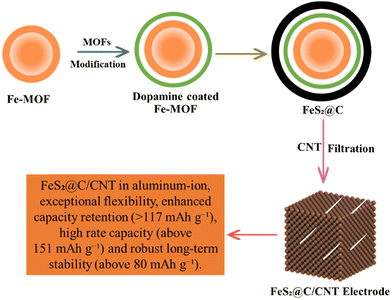 |
| Fig. 10 The application of MOFs in aluminum air batteries.157 | |
5.9 Electrode materials
Due to the presence of abundant metallic and organic redox sites within crystallographic porous materials, alongside their distinct pores and extensive surface area, these materials prove valuable for electrode applications. The investigation of cyclic voltammetry reveals insights through the correlation between peak currents and scan rates, elucidating the electrochemical storage processes.158 Notably, in 2006 and 2007, both MOF-177 and MIL-53 demonstrated effective utilization as cathode and anode materials in LIBs. For instance, CuTNCQ, which is conductive and redox-active, was formed on carbon nanofibers. This material served as a cathode for SIBs, showcasing a remarkable capacity of 252 mA h g−1 at 0.1C. Additionally, it exhibited exceptional redox flexibility across 1200 cycles spanning from 2.5 to 4.1 V. This underscores the advancements in utilizing these materials for enhancing energy storage systems.159 M3V2(PO4)3 (M indicates Li or Na) acts as an excellent cathode that has sparked attention on both lithium and sodium ion batteries in recent years. For example, Li3V2(PO 4)3 (LVP) has a substantial mean voltage (4.0 V) with a particular conductance of 197 mA h g, along with a low ion mobility and strong thermal stability.134
5.10 Supercapacitors (SCs)
Supercapacitors as an electrochemical capacitor which is an efficient energy storage device between traditional capacitors and secondary batteries. Supercapacitors are associated with various advantages due to the high power density of traditional capacitors and the high energy density of secondary batteries.160 These properties indicate efficient rate performances, high safety performances, a long cycle life, and environment-friendly nature. Recently supercapacitors were used in various fields which include power supplies, the automobile industry, and industrial sectors.161 There are two types of energy storage in supercapacitors, one is through the use of an electrical double layer to reach capacitance storage and the second is through Faraday's capacitor energy storage which used a reversible redox reaction on an electrode surface. As energy storage occurs at the surface of the electrode, porous electrodes with a suitable surface area are necessary.111 Using a simple chemical oxidation method, an electrode of composite material Ni-MOF@PPy was manufactured from a Ni-based metal–organic framework (Ni-MOF) doped with poly-pyrrole (PPy) to enhance its electron-transport property. The composite material had a specific capacitance of 1815.4 F g−1 at a current density of 1 A g−1.162 Pristine Fe-MOF has been used as an electrode material in supercapacitors due to their unique structures. In comparison with batteries which are associated with high energy density, supercapacitors are efficient electrical energy storage devices with a high power density.163 Liu et al. (2022) effectively manufactured the Zn-BTC by collecting zinc acetate and Na3BTC in a single process. Calcinating this precursor resulted in efficient porous carbon (BTCC) manufactured with a rich pore structure. The generated BTCC has a high SSA (1464 m2 g−1) and an appropriate pore size of 3.9 nm. The densely porous structures of BTCC provide a remarkable supercapacitor performance, including a high 310 F g−1 at 10 mV s−1, a charging time of only 0.9 s, and excellent cycle stability.164
5.11 Hydrogen evolution reaction (HER)
Hydrogen, being a sustainable energy storage medium with the highest gravimetric energy density, has received much interest as a potential replacement for fossil fuels. Water electrolysis can easily produce high-purity hydrogen, but the best Pt-based electrocatalysts utilized in this method are expensive and scarce in platinum.165 CoSe2–NiSe2/NPFC demonstrated outstanding catalytic activity during the HER in both acidic and alkaline solutions, requiring only 57 and 86mV overpotentials to reach a current density of 10 mA cm−2, respectively.166 Exorbitant costs and a paucity of resources have limited the broad deployment of the HER. As a result, significant efforts are being directed toward developing metal-based materials as highly efficient electrocatalysts, such as transition metal oxides, nitrides, oxides, selenides, phosphides, and so on, which are expected to replace noble platinum-based electrocatalysts in large-scale applications due to their low cost, intrinsic catalytic properties, and stability.167 He et al. (2022), demonstrated a freestanding HER catalytic molecular organic framework (MOF) (CS@CNC NAs/CC). CS@CNC NAs/CC revealed exceptional HER performance with an overpotential value of 84 mV within 10 mA cm−2, which was roughly the same as the efficacy exhibited by the platinum electrodes (35 mV). The CS@CNC NAs/CC metal electrode additionally showed greater structural stability, with an elevated potential of 84 mV maintained for 72 hours.101
6 Stability of metal–organic frameworks for advanced energy storage and conversion
MOFs have become very promising materials for enhanced energy conversion and storage because of their large surface areas, adjustable designs, and remarkable porosity. On the other hand, their actual use depends on the crucial factor of stability. The stability of MOFs for energy storage and conversion is represented in Table 2. It is clear from Table 2, that MOFs of the different metals have great potential for use as either different types of batteries or as supercapacitors. They have unique stability in energy storage devices on the basis of the cyclic stability, cycles and specific capacity. In 2019, Zhao and colleagues successfully synthesized a metal–organic framework material based on anthraquinone and subsequently explored its potential in energy storage applications. This synthesized material demonstrated a remarkable specific capacity of 240 mA h g−1 after undergoing 300 cycles. The documented specific capacity establishes this material as a promising candidate for use in next-generation battery technologies.168
Table 2 Stability parameters of MOF-based synthetic materials
Materials |
Applications |
Specific capacity (mA h g−1) |
Cyclic stability (%) |
Cycles |
Ref. |
Anthraquinone |
LIBs |
240 |
— |
300 |
168
|
Ni-MOF coating MoS2 |
Supercapacitors |
1590.24 |
87.97 |
20 000 |
169
|
Co/Zn bimetallic oxides |
Electrochemical |
25.9 |
71.43 |
2000 |
170
|
Hybrid metal oxides (NiO/NiCo2O4) |
LIBs |
732.0 |
92.5 |
3000 |
171
|
NiZnCoP and S-α-Fe2O3 |
Energy storage |
902 |
88.6 |
3000 |
172
|
Nickel pyrophosphate |
Energy storage |
1517 |
58 |
3000 |
173
|
Vanadium(V-MOFs, MIL-47) |
Zinc ion batteries |
101.8 |
81.5 |
300 |
174
|
Graphite carbon nitride |
Supercapacitors |
495 |
— |
5000 |
175
|
MWCNTs@Ni(TA) |
Energy storage |
115 |
81.6 |
5000 |
176
|
HCSN |
Energy storage |
720 |
82.1 |
10 000 |
177
|
Co-MOF nanocrystals |
LIBs |
1301 |
— |
2000 |
178
|
Hexagonal Ni |
Energy storage |
977.04 |
92.34 |
5000 |
179
|
Porous carbon |
Supercapacitors |
323 |
97.9 |
20 000 |
180
|
MCo2O4 (M = Mn and Zn) |
LIBs |
1289 |
95–5 |
20 000 |
181
|
ZnS |
Energy storage |
160 |
92.9 |
1000 |
182
|
2D carbon |
LIBs |
553 |
100 |
1000 |
183
|
CuCo2O4 |
Supercapacitors |
701 |
93.6 |
6000 |
184
|
Co3O4/Fe2O3 |
Energy storage |
312 |
89.4 |
10 000 |
185
|
CoHCF |
Na ion batteries |
97.8 |
94 |
5000 |
186
|
NiCo–P |
Energy storage |
894 |
80.4 |
10 000 |
187
|
CC@CoMoO4 Co(OH)2 |
Supercapacitors |
2028 |
94.5 |
5000 |
188
|
Mn-BDC |
Supercapacitors |
1590 |
82 |
3000 |
189
|
Co-MOF/NF |
Supercapacitors |
13.6 |
69.7 |
2000 |
190
|
CoS2 |
Supercapacitors |
146 |
89 |
5000 |
191
|
NiCo2O4 |
Supercapacitors |
208.8 |
100 |
6000 |
192
|
Zn–Co–O@CC |
Supercapacitors |
1750 |
94 |
5000 |
193
|
Fe-MIL-88B |
LIBs |
744.5 |
∼80 |
400 |
100
|
NiO/C@CNF |
Supercapacitors |
742 |
— |
5000 |
98
|
ppy-S-in-PCN-224 |
LSBs |
440 |
91.3 |
1000 |
194
|
Zn MOF |
Supercapacitors |
310 |
89.4 |
10 000 |
164
|
ZIF-67-ppy |
Supercapacitors |
597.6 |
95.5 |
10 000 |
159
|
MIL-47 |
Supercapacitors |
572 |
— |
1000 |
134
|
Ni-MOF-ppy |
Supercapacitors |
1815.4 |
90.2 |
3000 |
162
|
MIL-53 Fe |
LIBs |
93 |
87 |
— |
166
|
c-MOF Ni3(HITP)2 |
Supercapacitors |
111 |
89 |
10 000 |
29
|
Cu-MOF/G |
Supercapacitors |
482 |
95 |
1000 |
195
|
PET derived- MILL-53 |
ZIC |
391 |
92.2 |
10 000 |
196
|
MOF-199- PANI |
Supercapacitors |
766 |
92 |
1000 |
197
|
UPJS-15 |
LSBs |
235 |
— |
100 |
198
|
6.1 Disadvantages of MOFs for energy storage
Recently, there has been a lot of interest in metal–organic frameworks (MOFs) as possible materials for energy storage applications, especially in the fields of gas storage, hydrogen storage, and battery technologies. They do, however, have a number of disadvantages and challenges that must be resolved in order to put them into implementation. In the area of energy storage, it is imperative to critically evaluate the drawbacks associated with metal–organic frameworks (MOFs).199
Limited stability.
Numerous metal–organic frameworks (MOFs) exhibit a notable vulnerability to moisture and undergo degradation when exposed to water, thereby imposing restrictions on their persistent reliability and stability within achievable energy storage applications.200
Synthesis complexity.
The synthesis of metal–organic frameworks (MOFs) is a complex procedure, often requiring careful attention to specifics in terms of the conditions and precursors employed.201 This sophisticated nature of MOF synthesis poses significant challenges when attempting to enhance fabrication for industrial purposes. The complicated composition of MOFs can give rise to diverse expressions in their properties, thereby placing an obvious effect on their overall performance.202
Low volumetric energy density.
MOFs frequently exhibit comparably lowered volumetric energy densities, thereby requiring enhanced storage volumes in order to accommodate equivalent energy storage capacities compared to alternative substances, such as conventional metal hydrides.203
Kinetic limitations.
Some MOFs may have slower kinetics when it comes to the adsorption or desorption of gases, which may be a major disadvantage in applications where rapid energy absorption or release is essential.204
Chemical compatibility.
MOFs have demonstrated remarkable potential in the field of energy storage. However, it is important to acknowledge that their compatibility with certain chemicals and gases may impose limitations on their applicability in specific energy storage scenarios.20
Cost and scalability.
The cost associated with the fabrication of MOFs is a notable concern, particularly when considering their potential utilization in energy storage applications of practical significance. Moreover, the process of upscaling the synthesis of MOFs for such purposes presents a set of significant difficulties. The commercial viability of a product can be inhibited by the presence of high production costs.20
Cycling stability.
MOFs have been observed to exhibit a drawback in terms of their cycling stability, wherein they experience degradation over successive charge–discharge cycles within energy storage systems. This particular limitation hinders their practical applicability in batteries and supercapacitors.111
Tuning challenges.
MOFs with tailored properties suitable for targeted energy storage applications present an immense difficulty, as careful manipulation of both their structural and chemical attributes is required.205
7 Commercialization and industrial adoption
Metal–organic frameworks have emerged as a subject of considerable interest and evaluation within the scientific community in recent years due to their immense potential in a multitude of energy storage applications, most notably batteries and supercapacitors. The current state of research in metal–organic frameworks for energy storage primarily resides within the realm of experimental and developmental stages. However, it is worth noting that significant progress, collaborative efforts, and pilot initiatives have emerged, indicating the potential for MOF-based energy storage to transition into a commercially viable solution in the near future.206
7.1 Recent developments and partnerships
The widespread use of MOFs in the realm of energy storage devices has garnered substantial momentum, owing to a number of noteworthy advancements and collaborative efforts. The esteemed scholars from Northwestern University have engaged in a fruitful collaboration with A123 Systems, a distinguished purveyor of energy storage solutions, in order to embark upon the development of an electrode material for lithium-ion batteries that is based on metal–organic frameworks.207 The researchers successfully engineered a carbon composite material derived from metal–organic frameworks, which exhibited remarkable electrochemical characteristics, notably in terms of its substantial capacity and extended cycle life. The aforementioned collaboration serves as a testament to the profound interest exhibited by well-established corporations in leveraging the potential of metal–organic framework technology for the purpose of energy storage.208
7.2 PNNL's research on MOF-derived electrodes
The diligent and industrious Pacific Northwest National Laboratory (PNNL) has been deeply engaged in the pursuit of knowledge and understanding regarding the subject matter of MOF-derived materials, with a particular focus on their potential applications in the domain of energy storage. An investigation of metal–organic framework derived carbon materials as potential anode materials for lithium-ion batteries has been undertaken. The research conducted by Pacific Northwest National Laboratory (PNNL) is primarily centered around enhancing the energy density and stability of these materials, thereby advancing the feasibility of metal–organic frameworks (MOFs) for integration into commercial battery systems.209
7.3 Collaboration between MOF technologies and EnergyNest
In a collaborative effort, MOF Technologies, a distinguished enterprise focusing on the advancement and commercialization of metal–organic frameworks, has joined forces with EnergyNest, a prominent provider of cutting-edge energy storage technologies. The primary objective of this partnership is to delve into the potential applications of MOFs in the discipline of thermal energy storage. The primary objective of their collaborative effort is to advance the field of thermal storage by devising economically viable and highly efficient solutions through the utilization of MOFs.210
8 Future directions and research opportunities
This analysis seeks to delve deeper into the present condition of energy storage utilizing metal–organic frameworks. By doing so, we will endeavor to identify research directions that have promise, while also shedding light on areas that require further investigation. This will enable us to address prevailing challenges and unlock novel applications in this field.
8.1 Promising research directions
MOF synthesis and tailoring.
The research should concentrate on establishing scalable and cost-effective MOF synthesis techniques. To tune MOF characteristics for particular energy storage applications, novel techniques such as post-synthetic modification and defect engineering may be investigated. Rational design approaches, led by computer modeling, may help in the development of customized MOFs with improved characteristics.211,212
Stability enhancement.
One of the most difficult difficulties in MOF-based energy storage is maintaining stability under demanding working environments. MOFs with increased stability should be developed via research, particularly in the presence of moisture, high temperatures, and chemical reactions. Techniques for encapsulation and protective coatings may also be researched.213,214
Electrode design for batteries and supercapacitors.
Advancing battery and supercapacitor technology requires optimizing MOF-based electrode materials. To improve electrical conductivity and charge–discharge rates, researchers should investigate the integration of MOFs with other conductive materials or develop composite structures. This might include creating MOF-based composite electrodes with specific porosity and composition.215,216
In situ characterization.
In situ characterisation methods that have advanced may give useful insights into the electrochemical behavior of MOF-based energy storage materials. Researchers may use in situ investigations to better understand the structural changes, redox reactions, and ion dynamics that occur inside MOFs during energy storage operations. This information may be used to guide the development of new materials and technology.217
8.2 Challenges and research needs
Scalability and cost-effectiveness.
While MOFs have outstanding characteristics, synthesizing them on a big scale remains a difficulty. To make MOFs practicable for energy storage applications, research is required to develop cost-effective and scalable production procedures. In this context, collaboration between materials scientists and engineers is critical.218
Safety concerns.
MOFs may be dangerous in some energy storage applications, particularly when exposed to high temperatures or chemically reactive conditions. More investigation is needed to create safe handling and storage practices for MOF-based energy storage materials and devices.219
Long-term stability.
It is critical to ensure the long-term stability of MOFs in real-world applications. Researchers should undertake thorough durability testing and evaluate the effects of cycling, temperature changes, and pollutant exposure on MOF performance.220,221
Integration and system-level research.
It is a difficult challenge to integrate MOF-based energy storage materials into practical devices and systems. The development of entire energy storage systems, including design concerns, scalability, and compatibility with current technologies, should be the focus of research.222
9 Conclusions
Finally, in the context of sustainable energy storage, this study has offered an in-depth review of the rapidly developing discipline of metal–organic frameworks (MOFs). MOFs as a game-changing material for next-generation energy storage systems, owing to their unique features, including as tunability, large surface area, and various metal–organic combinations. The hybrid systems, which integrate MOFs with other materials such as polymers, graphene, or nanoparticles, are an emerging idea. These combinations may improve MOF performance while also addressing stability difficulties. The possibilities of developing functional MOF structures with advanced characteristics such as redox-active sites, charge transport channels, or catalytic centers for improved energy storage and conversion is also a major concern of future study. These applications of MOFs provide opportunities for inventive approaches to energy storage and conversion, in addition to showing promise for tackling the current pressing issues of environmental impact and energy sustainability. But it's important to recognize the hurdles that need to be removed in order to turn these promising ideas into actualities. Furthermore, multidisciplinary cooperation between materials scientists, chemists, engineers, and policymakers is necessary for the integration of MOFs into practical energy systems. The possibilities for MOFs in sustainable energy storage are still very exciting and enormous as we go towards the future. To fully use MOF materials, researchers and industry stakeholders need to keep funding the investigation of new materials, synthesis techniques, and applications. When combined with persistent work, MOFs have the potential to significantly contribute to the worldwide shift toward sustainable, effective, and clean energy storage technologies.
Conflicts of interest
There are no conflicts to declare.
Acknowledgements
The authors extend their appreciation to the Deanship of Scientific Research at King Khalid University for supporting this work through research groups program under grant number RGP.2/273/44.
References
- L. Bojic, Metaverse through the prism of power and addiction: what will happen when the virtual world becomes more attractive than reality?, Eur. J. Futures Res., 2022, 10(1), 1–24 CrossRef
.
- S. Koohi-Fayegh and M. A. Rosen, A review of energy storage types, applications and recent developments, J. Energy Storage, 2020, 27, 101047 CrossRef
.
- A. A. Kebede,
et al., A comprehensive review of stationary energy storage devices for large scale renewable energy sources grid integration, Renewable Sustainable Energy Rev., 2022, 159, 112213 CrossRef CAS
.
- H. Wang, X. Qian and X. An, Introducing lanthanide metal–organic framework and perovskite onto pulp fibers for fluorescent anti-counterfeiting and encryption, Cellulose, 2022, 1–13 Search PubMed
.
- Q. Huang, Y. Yang and J. Qian, Structure-directed growth and morphology of multifunctional metal-organic frameworks, Coord. Chem. Rev., 2023, 484, 215101 CrossRef CAS
.
- H. Kim and C. S. Hong, MOF-74-type frameworks: Tunable pore environment and functionality through metal and ligand modification, CrystEngComm, 2021, 23(6), 1377–1387 RSC
.
- P.-F. Hsieh,
et al., Understanding solvothermal growth of Metal–Organic Framework colloids for CO2 capture applications, Langmuir, 2022, 38(14), 4415–4424 CrossRef CAS
.
- A. Mukherjee, P. Dhak and D. Dhak, The solvothermal synthesis of a 3D rod-like Fe–Al bimetallic metal–organic-framework for efficient fluoride adsorption and photodegradation of water-soluble carcinogenic dyes, Environ. Sci.: Adv., 2022, 1(2), 121–137 CAS
.
- K. Kamal,
et al., Optimization of washing processes in solvothermal synthesis of nickel-based MOF-74, Materials, 2020, 13(12), 2741 CrossRef CAS PubMed
.
- C. Chen,
et al., Microwave-assisted rapid synthesis of well-shaped MOF-74 (Ni) for CO2 efficient capture, Inorg. Chem., 2019, 58(4), 2717–2728 CrossRef CAS
.
- H.-T. T. Nguyen,
et al., Microwave-assisted solvothermal synthesis of bimetallic metal-organic framework for efficient photodegradation of organic dyes, Mater. Chem. Phys., 2021, 272, 125040 CrossRef CAS
.
- Y. T. Dang,
et al., Microwave-assisted synthesis of nano Hf-and Zr-based metal-organic frameworks for enhancement of curcumin adsorption, Microporous Mesoporous Mater., 2020, 298, 110064 CrossRef CAS
.
- H. Liu,
et al., Microwave-assisted synthesis of Zr-based metal–organic framework (Zr-fum-fcu-MOF) for gas adsorption separation, Chem. Phys. Lett., 2021, 780, 138906 CrossRef CAS
.
- S. Głowniak,
et al., Mechanochemistry: Toward green synthesis of metal–organic frameworks, Mater. Today, 2021, 46, 109–124 CrossRef
.
- J. Beamish-Cook,
et al., Insights into the Mechanochemical Synthesis of MOF-74, Cryst. Growth Des., 2021, 21(5), 3047–3055 CrossRef CAS
.
- D. Chen,
et al., Mechanochemical synthesis of metal–organic frameworks, Polyhedron, 2019, 162, 59–64 CrossRef CAS
.
- C. Duan, Y. Yu and H. Hu, Recent progress on synthesis of ZIF-67-based materials and their application to heterogeneous catalysis, Green Energy Environ., 2022, 7(1), 3–15 CrossRef CAS
.
- V. Ve Butova,
et al., Metal-organic frameworks: structure, properties, methods of synthesis and characterization, Russ. Chem. Rev., 2016, 85(3), 280 CrossRef CAS
.
- X. Zhang,
et al., Metal–organic frameworks (MOFs) and MOF-derived materials for energy storage and conversion, Electrochem. Energy Rev., 2019, 2, 29–104 CrossRef CAS
.
- V. Shrivastav,
et al., Metal-organic frameworks (MOFs) and their composites as electrodes for lithium battery applications: Novel means for alternative energy storage, Coord. Chem. Rev., 2019, 393, 48–78 CrossRef CAS
.
- G. Zou,
et al., Metal–organic framework-derived materials for sodium energy storage, Small, 2018, 14(3), 1702648 CrossRef
.
- Z. Ding, W. Wu and M. Leung, Advanced/hybrid thermal energy storage technology: material, cycle, system and perspective, Renewable Sustainable Energy Rev., 2021, 145, 111088 CrossRef CAS
.
- A. Chen, X. Zhang and Z. Zhou, Machine learning: accelerating materials development for energy storage and conversion, InfoMat, 2020, 2(3), 553–576 CrossRef CAS
.
- Y. Zhao,
et al., Metal organic frameworks for energy storage and conversion, Energy Storage Mater., 2016, 2, 35–62 CrossRef
.
- T. Mehtab,
et al., Metal-organic frameworks for energy storage
devices: batteries and supercapacitors, J. Energy Storage, 2019, 21, 632–646 CrossRef
.
- Y. Xu,
et al., Application of metal-organic frameworks, covalent organic frameworks and their derivates for the metal-air batteries, Nano Res. Energy, 2023, 2(2), e9120052 CrossRef
.
- R. Yan,
et al., Metal–organic-framework-derived nanostructures as multifaceted electrodes in metal–sulfur batteries, Adv. Mater., 2021, 33(27), 2008784 CrossRef CAS
.
- R. Yuksel,
et al., Metal-organic framework integrated anodes for aqueous zinc-ion batteries, Adv. Energy Mater., 2020, 10(16), 1904215 CrossRef CAS
.
- L. Niu,
et al., Conductive metal–organic frameworks for supercapacitors, Adv. Mater., 2022, 2200999 CrossRef CAS PubMed
.
- B. Xu,
et al., Recent progress in metal-organic framework-based supercapacitor electrode materials, Coord. Chem. Rev., 2020, 420, 213438 CrossRef CAS
.
- A. Mohanty,
et al., An extensive review on three dimension architectural Metal-Organic Frameworks towards supercapacitor application, J. Power Sources, 2021, 488, 229444 CrossRef CAS
.
- X. Wen, Q. Zhang and J. Guan, Applications of metal–organic framework-derived materials in fuel cells and metal-air batteries, Coord. Chem. Rev., 2020, 409, 213214 CrossRef CAS
.
- X. Ao,
et al., Sulfurization-functionalized 2D metal-organic frameworks for high-performance urea fuel cell, Appl. Catal., B, 2022, 315, 121586 CrossRef CAS
.
- Z. H. Li,
et al., Multivariate Synergistic Flexible Metal-Organic Frameworks with Superproton Conductivity for Direct Methanol Fuel Cells, Angew. Chem., 2021, 133(51), 26781–26785 CrossRef
.
- J. Liu,
et al., 2D conductive metal–organic frameworks: an emerging platform for electrochemical energy storage, Angew. Chem., 2021, 133(11), 5672–5684 CrossRef
.
- Y. Li,
et al., MOF-derived metal oxide composites for advanced electrochemical energy storage, Small, 2018, 14(25), 1704435 CrossRef
.
- J. A. Cruz-Navarro, F. Hernandez-Garcia and G. A. A. Romero, Novel applications of metal-organic frameworks (MOFs) as redox-active materials for elaboration of carbon-based electrodes with electroanalytical uses, Coord. Chem. Rev., 2020, 412, 213263 CrossRef CAS
.
- Y. Cong,
et al., Metal–Organic Frameworks-Derived Self-Supported Carbon-Based Composites for Electrocatalytic Water Splitting, Chem. – Eur. J., 2021, 27(64), 15866–15888 CrossRef CAS
.
- R. C. K. Reddy,
et al., Progress of nanostructured metal oxides derived from metal–organic frameworks as anode materials for lithium–ion batteries, Coord. Chem. Rev., 2020, 420, 213434 CrossRef CAS
.
- S. Wu,
et al., A review of performance optimization of MOF-derived metal oxide as electrode materials for supercapacitors, Int. J. Energy Res., 2019, 43(2), 697–716 CrossRef CAS
.
- Y. Liu, X. Xu and Z. Shao, Metal-organic frameworks derived porous carbon, metal oxides and metal sulfides-based compounds for supercapacitors application. Energy Storage, Materials, 2020, 26, 1–22 Search PubMed
.
- J. Wang,
et al., MOFs-derived transition metal sulfide composites for advanced sodium ion batteries. Energy Storage, Materials, 2021, 41, 404–426 Search PubMed
.
- X.-C. Xie, K.-J. Huang and X. Wu, Metal–organic framework derived hollow materials for electrochemical energy storage, J. Mater. Chem. A, 2018, 6(16), 6754–6771 RSC
.
- C. Li,
et al., Nickel metal–organic framework nanosheets as novel binder-free cathode for advanced fibrous aqueous rechargeable Ni–Zn battery, J. Mater. Chem. A, 2020, 8(6), 3262–3269 RSC
.
- M. A. Abdelkareem,
et al., High-performance effective metal–organic frameworks for electrochemical applications, J. Sci.: Adv. Mater. Devices, 2022, 7(3), 100465 CAS
.
- B. Y. Guan,
et al., Complex nanostructures from materials based on metal–organic frameworks for electrochemical energy storage and conversion, Adv. Mater., 2017, 29(47), 1703614 CrossRef
.
- Y. Liang,
et al., Organo-macrocycle-containing hierarchical metal–organic frameworks and cages: design, structures, and applications, Chem. Soc. Rev., 2022, 51, 8378–8405 RSC
.
- A. Dutta,
et al., Multicomponent isoreticular metal-organic frameworks: Principles, current status and challenges, Coord. Chem. Rev., 2021, 445, 214074 CrossRef CAS
.
- D. Tian, C. Wang and X. Lu, Metal–organic frameworks and their derived functional materials for supercapacitor electrode application, Adv. Energy Sustainability Res., 2021, 2(7), 2100024 CrossRef CAS
.
- X. Luo,
et al., Trimetallic metal–organic frameworks and derived materials for environmental remediation and electrochemical energy storage and conversion, Coord. Chem. Rev., 2022, 461, 214505 CrossRef CAS
.
- W. Zhao,
et al., Flexible transparent supercapacitors: materials and devices, Adv. Funct. Mater., 2021, 31(11), 2009136 CrossRef CAS
.
- J. Chen,
et al., Hierarchical large-pore MOFs templated from poly (ethylene oxide)-b-polystyrene diblock copolymer with tuneable pore sizes, Chem. Commun., 2022, 58(72), 10028–10031 RSC
.
- N. A. Kukhta, A. Marks and C. K. Luscombe, Molecular design strategies toward improvement of charge injection and ionic conduction in organic mixed ionic–electronic conductors for organic electrochemical transistors, Chem. Rev., 2021, 122(4), 4325–4355 CrossRef PubMed
.
- R. Ramos,
et al., Metal-supported biochar catalysts for sustainable biorefinery, electrocatalysis, and energy storage applications: a review, Catalysts, 2022, 12(2), 207 CrossRef CAS
.
- D. Y. Lee,
et al., Facile interfacial charge transfer across hole doped cobalt-based MOFs/TiO 2 nano-hybrids making MOFs light harvesting active layers in solar cells, J. Mater. Chem. A, 2015, 3(45), 22669–22676 RSC
.
- B. Han,
et al., Recent Advances in Metal-Organic Framework-Based Nanomaterials for Electrocatalytic Nitrogen Reduction, Small Methods, 2023, 2300277 CrossRef CAS
.
- D. G. Atinafu,
et al., A novel enhancement of shape/thermal stability and energy-storage capacity of phase change materials through the formation of composites with 3D porous (3, 6)-connected metal–organic framework, Chem. Eng. J., 2020, 389, 124430 CrossRef CAS
.
- S. Zheng,
et al., A highly alkaline-stable metal oxide@ metal–organic framework composite for high-performance electrochemical energy storage, Natl. Sci. Rev., 2020, 7(2), 305–314 CrossRef CAS
.
- N. ul Qadir, S. A. Said and H. M. Bahaidarah, Structural stability of metal organic frameworks in aqueous media–controlling factors and methods to improve hydrostability and hydrothermal cyclic stability, Microporous Mesoporous Mater., 2015, 201, 61–90 CrossRef
.
- X. Wang,
et al., MOF-based electrocatalysts for high-efficiency CO2 conversion: structure, performance, and perspectives, J. Mater. Chem. A, 2021, 9(40), 22710–22728 RSC
.
- X. Gao,
et al., MOFs and COFs for batteries and supercapacitors, Electrochem. Energy Rev., 2020, 3, 81–126 CrossRef
.
- S. Pioquinto-García,
et al., Environmental assessment of metal-organic framework DUT-4 synthesis and its application for siloxane removal, J. Environ. Chem. Eng., 2021, 9(6), 106601 CrossRef
.
- S. Kumar,
et al., Green synthesis of metal–organic frameworks: A state-of-the-art review of potential environmental and medical applications, Coord. Chem. Rev., 2020, 420, 213407 CrossRef CAS
.
- D. M. Maklavany,
et al., Eco-Environmental Analysis of Different Routes for the Synthesis of MIL-53 (Fe): An Integrated Life Cycle Assessment and Life Cycle Cost Approaches, ACS Sustainable Chem. Eng., 2023, 11(26), 9816–9832 CrossRef CAS
.
- O. T. Qazvini, R. Babarao and S. G. Telfer, Selective capture of carbon dioxide from hydrocarbons using a metal-organic framework, Nat. Commun., 2021, 12(1), 197 CrossRef CAS PubMed
.
- Y. Dong, Y. Ji and Z. Lai, Carbon Dioxide Capture in Metal-Organic Frameworks, Highlights Sci. Eng. Technol., 2022, 21, 50–56 CrossRef
.
- N. Daud and N. Najib, Adsorption of CO2 on ZSM-5 and Cu-MOF at room temperature and low pressure conditions for Carbon Capture and Storage (CCS) application, Mater. Today Proc., 2022, 57, 1345–1355 CrossRef CAS
.
- N. Daud, CO2 adsorption performance of AC and Zn-MOF for the use of carbon capture and sequestration (CCS), Mater. Today Proc., 2023 DOI:10.1016/j.matpr.2023.03.231
.
- Y. Liu, P. Ghimire and M. Jaroniec, Copper benzene-1, 3, 5-tricarboxylate (Cu-BTC) metal-organic framework (MOF) and porous carbon composites as efficient carbon dioxide adsorbents, J. Colloid Interface Sci., 2019, 535, 122–132 CrossRef CAS
.
- S. Nandi,
et al., Ultralow parasitic energy for postcombustion CO2 capture realized in a nickel isonicotinate metal–organic framework with excellent moisture stability, J. Am. Chem. Soc., 2017, 139(5), 1734–1737 CrossRef CAS PubMed
.
- A. Lin,
et al., Palladium nanoparticles supported on Ce-metal–organic framework for efficient CO oxidation and low-temperature CO2 capture, ACS Appl. Mater. Interfaces, 2017, 9(21), 17961–17968 CrossRef CAS
.
- Z. Zhu and Q. Zheng, Investigation of cryo-adsorption hydrogen storage capacity of rapidly synthesized MOF-5 by mechanochemical method, Int. J. Hydrogen Energy, 2023, 48(13), 5166–5174 CrossRef CAS
.
- X. Zhang, P. Liu and Y. Zhang, The application of MOFs for hydrogen storage, Inorg. Chim. Acta, 2023, 121683 CrossRef CAS
.
- H. J. Choi,
et al., Hydrogen storage in water-stable metal–organic frameworks incorporating 1, 3-and 1, 4-benzenedipyrazolate, Energy Environ. Sci., 2010, 3(1), 117–123 RSC
.
- I. A. Ibarra,
et al., Highly porous and robust scandium-based metal–organic frameworks for hydrogen storage, Chem. Commun., 2011, 47(29), 8304–8306 RSC
.
- D. A. Gómez-Gualdrón,
et al., Evaluating topologically diverse metal–organic frameworks for cryo-adsorbed hydrogen storage, Energy Environ. Sci., 2016, 9(10), 3279–3289 RSC
.
- M. T. Kapelewski,
et al., Record high hydrogen storage capacity in the metal–organic framework Ni2 (m-dobdc) at near-ambient temperatures, Chem. Mater., 2018, 30(22), 8179–8189 CrossRef CAS
.
- J. Xu,
et al., Optimized synthesis of Zr (iv) metal organic frameworks (MOFs-808) for efficient hydrogen storage, New J. Chem., 2019, 43(10), 4092–4099 RSC
.
- T. Jia, Y. Gu and F. Li, Progress and potential of metal-organic frameworks (MOFs) for gas storage and separation: A review, J. Environ. Chem. Eng., 2022, 108300 CrossRef CAS
.
- H. Yang,
et al., Advances in metal-organic frameworks for efficient separation and purification of natural gas, Chin. J. Struct. Chem., 2023, 42(2), 100034 CrossRef
.
- S. Kayal, B. Sun and A. Chakraborty, Study of metal-organic framework MIL-101 (Cr) for natural gas (methane) storage and compare with other MOFs (metal-organic frameworks), Energy, 2015, 91, 772–781 CrossRef CAS
.
- J. Jiang,
et al., High methane storage working capacity in metal–organic frameworks with acrylate links, J. Am. Chem. Soc., 2016, 138(32), 10244–10251 CrossRef CAS PubMed
.
- T. Kundu,
et al., Functionalization-induced breathing control in metal–organic frameworks for methane storage with high deliverable capacity, Chem. Mater., 2019, 31(8), 2842–2847 CrossRef CAS
.
- M. Anbia and S. Sheykhi, Preparation of multi-walled carbon nanotube incorporated MIL-53-Cu composite metal–organic framework with enhanced methane sorption, J. Ind. Eng. Chem., 2013, 19(5), 1583–1586 CrossRef CAS
.
- G. Sargazi,
et al., A novel composite derived from a metal organic framework immobilized within electrospun nanofibrous polymers: An efficient methane adsorbent, Appl. Organomet. Chem., 2020, 34(3), e5448 CrossRef CAS
.
- C.-L. Chen,
et al., Conductive Lanthanide Metal–Organic Frameworks with Exceptionally High Stability, J. Am. Chem. Soc., 2023, 145(31), 16983–16987 CrossRef CAS PubMed
.
- Z. Yu,
et al., Efficient Generation of Large Collections of Metal–Organic Framework Structures Containing Well-Defined Point Defects, J. Phys. Chem. Lett., 2023, 14(29), 6658–6665 CrossRef CAS PubMed
.
- C. Sandoval-Pauker, G. Molina-Aguirre and B. Pinter, Status report on copper (I) complexes in photoredox catalysis; photophysical and electrochemical properties and future prospects, Polyhedron, 2021, 199, 115105 CrossRef CAS
.
- L. R. Redfern and O. K. Farha, Mechanical properties of metal–organic frameworks, Chem. Sci., 2019, 10(46), 10666–10679 RSC
.
- Q. Liu,
et al., A review on metal-and metal oxide-based nanozymes: properties, mechanisms, and applications, Nano-Micro Lett., 2021, 13, 1–53 CrossRef
.
- A. A. Oun, S. Shankar and J.-W. Rhim, Multifunctional nanocellulose/metal and metal oxide nanoparticle hybrid nanomaterials, Crit. Rev. Food Sci. Nutr., 2020, 60(3), 435–460 CrossRef CAS PubMed
.
- K. Namsheer and C. S. Rout, Conducting polymers: A comprehensive review on recent advances in synthesis, properties and applications, RSC Adv., 2021, 11(10), 5659–5697 RSC
.
- B. Lakard, Electrochemical biosensors based on conducting polymers: A review, Appl. Sci., 2020, 10(18), 6614 CrossRef CAS
.
- N. Wu,
et al., Review on the electromagnetic interference shielding properties of carbon based materials and their novel composites: Recent progress, challenges and prospects, Carbon, 2021, 176, 88–105 CrossRef CAS
.
- P. Gholami,
et al., A review on carbon-based materials for heterogeneous sonocatalysis: fundamentals, properties and applications, Ultrason. Sonochem., 2019, 58, 104681 CrossRef CAS PubMed
.
- J. Ru,
et al., UiO series of metal-organic frameworks composites as advanced sorbents for the removal of heavy metal ions: Synthesis, applications and adsorption mechanism, Ecotoxicol.
Environ. Saf., 2021, 208, 111577 CrossRef CAS
.
- Y. Liu, W. Zhang and H. Wang, Synthesis and application of core–shell liquid metal particles: A perspective of surface engineering, Mater. Horiz., 2021, 8(1), 56–77 RSC
.
- Y. S. Wei,
et al., Micro/nano-scaled metal-organic frameworks and their derivatives for energy applications, Adv. Energy Mater., 2022, 12(4), 2003970 CrossRef CAS
.
- H. Wang,
et al., Metal-organic frameworks for energy applications, Chem, 2017, 2(1), 52–80 CAS
.
- S. Chuhadiya,
et al., Metal organic frameworks as hybrid porous materials for energy storage and conversion devices: A review, Coord. Chem. Rev., 2021, 446, 214115 CrossRef CAS
.
- B. He,
et al., Freestanding metal–organic frameworks and their derivatives: an emerging platform for electrochemical energy storage and conversion, Chem. Rev., 2022, 122(11), 10087–10125 CrossRef CAS
.
- Y. Qiao,
et al., A high-energy-density and long-life lithium-ion battery via reversible oxide–peroxide conversion, Nat. Catal., 2019, 2(11), 1035–1044 CrossRef CAS
.
-
Y. Li, Improvement of the performance for perovskite solar cell, 2020.
- Y. Jin,
et al., Facile synthesis of Fe-MOF/RGO and its application as a high performance anode in lithium-ion batteries, RSC Adv., 2016, 6(36), 30763–30768 RSC
.
- Y.-Y. Wang,
et al., Diamondoid-structured polymolybdate-based metal–organic frameworks as high-capacity anodes for lithium-ion batteries, Chem. Commun., 2017, 53(37), 5204–5207 RSC
.
- F. Wang,
et al., Foam-like CoO@ N, S-codoped carbon composites derived from a well-designed N, S-rich Co-MOF for lithium-ion batteries, J. Mater. Chem. A, 2017, 5(44), 22964–22969 RSC
.
- Z. Parviz,
et al., Fabrication of Sustainable Hybrid MOF/Silica Electrodes for Current Lithium-ion Batteries and Beyond, ACS Appl. Energy Mater., 2022, 5(12), 15155–15165 CrossRef CAS
.
- A. V. Desai,
et al., Conversion of a microwave synthesized alkali-metal MOF to a carbonaceous anode for Li-ion batteries, RSC Adv., 2020, 10(23), 13732–13736 RSC
.
- J. Sun,
et al., Will lithium-sulfur batteries be the next beyond-lithium ion batteries and even much better?, InfoMat, 2022, 4(9), e12359 CrossRef CAS
.
- F. Zhao,
et al., Toward high-sulfur-content, high-performance lithium–sulfur batteries: Review of materials and technologies, J. Energy Chem., 2023, 80, 625–657 CrossRef CAS
.
- J. Ren,
et al., Recent progress on MOF-derived carbon materials for energy storage, Carbon Energy, 2020, 2(2), 176–202 CrossRef CAS
.
- M. Rana,
et al., Oriented nanoporous MOFs to mitigate polysulfides migration in lithium-sulfur batteries, Nano Energy, 2020, 75, 105009 CrossRef CAS
.
- C. Li,
et al., MOFs derived hierarchically porous TiO2 as effective chemical and physical immobilizer for sulfur species as cathodes for high-performance lithium-sulfur batteries, Electrochim. Acta, 2016, 215, 689–698 CrossRef CAS
.
- H. Zhu,
et al., MOF derived cobalt-nickel bimetallic phosphide (CoNiP) modified separator to enhance the polysulfide adsorption-catalysis for superior lithium-sulfur batteries, J. Colloid Interface Sci., 2023, 641, 942–949 CrossRef CAS PubMed
.
- C. Wang,
et al., Iron single-atom catalyst anchored on nitrogen-rich MOF-derived carbon nanocage to accelerate polysulfide redox conversion for lithium sulfur batteries, J. Mater. Chem. A, 2020, 8(6), 3421–3430 RSC
.
- N. Yuan,
et al., Multifunctional MOF-Based Separator Materials for Advanced Lithium–Sulfur Batteries, Adv. Mater. Interfaces, 2021, 8(9), 2001941 CrossRef CAS
.
-
S. Peng, Zinc-Air Batteries: Fundamentals, Key Materials and Application, Springer Nature, 2023 Search PubMed
.
- H. Wang,
et al., Engineering mesopores and unsaturated coordination in metal–organic frameworks for enhanced oxygen reduction and oxygen evolution activity and Li–air battery capacity, ACS Sustainable Chem. Eng., 2021, 9(12), 4509–4519 CrossRef CAS
.
- M. J. Song,
et al., Self-standing, binder-free electrospun Co3O4/carbon nanofiber composites for non-aqueous Li-air batteries, Electrochim. Acta, 2015, 182, 289–296 CrossRef CAS
.
- L. Majidi,
et al., Nanostructured Conductive Metal Organic Frameworks for Sustainable Low Charge Overpotentials in Li–Air Batteries, Small, 2022, 18(4), 2102902 CrossRef CAS
.
- X. X. Wang,
et al., Metal–Organic Frameworks Derived Electrolytes Build Multiple Wetting Interfaces for Integrated Solid-State Lithium–Oxygen Battery, Adv. Funct. Mater., 2022, 32(30), 2113235 CrossRef CAS
.
- L.-N. Song,
et al., Tuning lithium-peroxide formation and decomposition routes with single-atom catalysts for lithium–oxygen batteries, Nat. Commun., 2020, 11(1), 2191 CrossRef CAS PubMed
.
-
Z. Guo, et al., Progress in electrode materials for the industrialization of sodium-ion batteries, Progress in Natural Science: Materials International, 2022.
- H. Hou,
et al., Carbon anode materials for advanced sodium-ion batteries, Adv. Energy Mater., 2017, 7(24), 1602898 CrossRef
.
- P. K. Nayak,
et al., From lithium-ion to sodium-ion batteries: advantages, challenges, and surprises, Angew. Chem., Int. Ed., 2018, 57(1), 102–120 CrossRef CAS
.
- F. Xiao,
et al., A new calcium metal organic frameworks (Ca-MOF) for sodium ion batteries, Mater. Lett., 2021, 286, 129264 CrossRef CAS
.
- Y. V. Kaneti,
et al., Fabrication of an MOF-derived heteroatom-doped Co/CoO/carbon hybrid with superior sodium storage performance for sodium-ion batteries, J. Mater. Chem. A, 2017, 5(29), 15356–15366 RSC
.
- W. Li,
et al., Confined amorphous red phosphorus in MOF-derived N-doped microporous carbon as a superior anode for sodium-ion battery, Adv. Mater., 2017, 29(16), 1605820 CrossRef
.
- W. Zhang,
et al., Uniform Bi–Sb alloy nanoparticles synthesized from MOFs by laser metallurgy for sodium-ion batteries, ACS Sustainable Chem. Eng., 2019, 8(1), 335–342 CrossRef
.
- D. Choi, S. Lim and D. Han, Advanced metal–organic frameworks for aqueous sodium-ion rechargeable batteries, J. Energy Chem., 2021, 53, 396–406 CrossRef CAS
.
- S. Mallick and C. R. Raj, Aqueous Rechargeable Zn-ion Batteries: Strategies for Improving the Energy Storage Performance, ChemSusChem, 2021, 14(9), 1987–2022 CrossRef CAS
.
- J. Hao,
et al., Deeply understanding the Zn anode behaviour and corresponding improvement strategies in different aqueous Zn-based batteries, Energy Environ. Sci., 2020, 13(11), 3917–3949 RSC
.
- S. Nagappan,
et al., Metal–Organic Frameworks-Based Cathode Materials for Energy Storage Applications: A Review, Energy Technol., 2023, 11(3), 2201200 CrossRef CAS
.
- J. Zhu,
et al., Vanadium-based metal-organic frameworks and their derivatives for electrochemical energy conversion and storage, SmartMat, 2022, 3(3), 384–416 CrossRef CAS
.
- Y. Ru,
et al., Layered V-MOF nanorods for rechargeable aqueous zinc-ion batteries, Mater. Today Chem., 2021, 21, 100513 CrossRef CAS
.
- F. Tang,
et al., Graphene-wrapped MnO/C composites by MOFs-derived as cathode material for aqueous zinc ion batteries, Electrochim. Acta, 2020, 353, 136570 CrossRef CAS
.
- X. Wu,
et al., The intercalation cathode of MOFs-driven vanadium-based composite embedded in N-doped carbon for aqueous zinc ion batteries, Chem. Eng. J., 2023, 452, 139573 CrossRef CAS
.
- Y. Fu,
et al., High-performance reversible aqueous Zn-ion battery based on porous MnOx nanorods coated by MOF-derived N-doped carbon, Adv. Energy Mater., 2018, 8(26), 1801445 CrossRef
.
- K. W. Nam,
et al., Conductive 2D metal-organic framework for high-performance cathodes in aqueous rechargeable zinc batteries, Nat. Commun., 2019, 10(1), 4948 CrossRef PubMed
.
- Z. Wu,
et al., Potassium-ion battery cathodes: Past, present, and prospects, J. Power Sources, 2021, 484, 229307 CrossRef CAS
.
- X. Du and B. Zhang, Robust solid electrolyte interphases in localized high concentration electrolytes boosting black phosphorus anode for potassium-ion batteries, ACS Nano, 2021, 15(10), 16851–16860 CrossRef CAS PubMed
.
- S. Su,
et al., Control of SEI formation for stable potassium-ion battery anodes by Bi-MOF-derived nanocomposites, ACS Appl. Mater. Interfaces, 2019, 11(25), 22474–22480 CrossRef CAS PubMed
.
- X. Lu,
et al., MOF-5 as anodes for high-temperature potassium-ion batteries with ultrahigh stability, Chem. Eng. J., 2022, 432, 134416 CrossRef CAS
.
- J. H. Na, Y. C. Kang and S.-K. Park, Electrospun MOF-based ZnSe nanocrystals confined in N-doped mesoporous carbon fibers as anode materials for potassium ion batteries with long-term cycling stability, Chem. Eng. J., 2021, 425, 131651 CrossRef
.
- C. Li, X. Hu and B. Hu, Cobalt (II) dicarboxylate-based metal-organic framework for long-cycling and high-rate potassium-ion battery anode, Electrochim. Acta, 2017, 253, 439–444 CrossRef CAS
.
- F. Bella,
et al., An overview on anodes for magnesium batteries: Challenges towards a promising storage solution for renewables, Nanomaterials, 2021, 11(3), 810 CrossRef CAS PubMed
.
- C. Dong, H. Kobayashi and I. Honma, Self-activation effect in bimetallic MgMn2O4 and boosting its electrochemical performance using metal-organic framework template for magnesium-ion battery cathodes, Mater. Today Energy, 2022, 30, 101143 CrossRef CAS
.
- Z. Wei,
et al., Ionic Liquid-Incorporated Metal-Organic Framework with High Magnesium Ion Conductivity for Quasi-Solid-State Magnesium Batteries, Batteries Supercaps, 2022, 5(12), e202200318 CrossRef CAS
.
- W. Wang,
et al., Metal Organic Framework (MOF)-Derived carbon-encapsulated cuprous sulfide cathode based on displacement reaction for Hybrid Mg2+/Li+ batteries, J. Power Sources, 2020, 445, 227325 CrossRef CAS
.
- X. Zhou,
et al., High rate magnesium–sulfur battery with improved cyclability based on metal–organic framework derivative carbon host, Adv. Mater., 2018, 30(7), 1704166 CrossRef
.
-
R. K. Harchegani, Strategies to Improve the Electrochemical Performance of Aluminum Anodes in Primary Alkaline Aluminum-Air Batteries, University of Windsor, Canada, 2023 Search PubMed
.
- K. Zhang,
et al., Metal-organic framework-derived metal oxide nanoparticles@ reduced graphene oxide composites as cathode materials for rechargeable aluminium-ion batteries, Sci. Rep., 2019, 9(1), 13739 CrossRef PubMed
.
-
K. Zhang, Metal–Organic Frameworks Derived Heterogeneous Materials: Toward High-Performance Electrochemical Applications of Aluminum-and Lithium-Ion Storage. 2020.
- X. Xiao,
et al., Metal–organic framework-derived Co3O4@ MWCNTs polyhedron as cathode material for a high-performance aluminum-ion battery, ACS Sustainable Chem. Eng., 2019, 7(19), 16200–16208 CrossRef CAS
.
- H. Hong,
et al., Ordered macro–microporous metal–organic framework single crystals and their derivatives for rechargeable aluminum-ion batteries, J. Am. Chem. Soc., 2019, 141(37), 14764–14771 CrossRef CAS
.
- B. Zhang,
et al., In situ growth of metal–organic framework-derived CoTe2 nanoparticles@ nitrogen-doped porous carbon polyhedral composites as novel cathodes for rechargeable aluminum-ion batteries, J. Mater. Chem. A, 2020, 8(11), 5535–5545 RSC
.
- Y. Hu,
et al., All-Climate Aluminum-Ion Batteries Based on Binder-Free MOF-Derived FeS2@C/CNT Cathode, Nano-Micro Lett., 2021, 13, 1–12 CrossRef
.
- A. S. Rajpurohit, N. S. Punde and A. K. Srivastava, A dual metal organic framework based on copper-iron clusters integrated sulphur doped graphene as a porous material for supercapacitor with remarkable performance characteristics, J. Colloid Interface Sci., 2019, 553, 328–340 CrossRef CAS
.
- H. Zhang,
et al., Hybridization of emerging crystalline porous materials: synthesis dimensionality and electrochemical energy storage application, Adv. Energy Mater., 2022, 12(4), 2100321 CrossRef CAS
.
- M. M. Baig,
et al., 2D MXenes: Synthesis, properties, and electrochemical energy storage for supercapacitors–A review, J. Electroanal. Chem., 2022, 904, 115920 CrossRef CAS
.
- F. B. Ajdari,
et al., A review on the field patents and recent developments over the application of metal organic frameworks (MOFs) in supercapacitors, Coord. Chem. Rev., 2020, 422, 213441 CrossRef
.
- Z. Qin,
et al., Ni-MOF composite polypyrrole applied to supercapacitor energy storage, RSC Adv., 2022, 12(45), 29177–29186 RSC
.
- X. Jin,
et al., Applications of Transition Metal (Fe, Co, Ni)-Based Metal–Organic Frameworks and their Derivatives in Batteries and Supercapacitors, Trans. Tianjin Univ., 2022, 28(6), 446–468 CrossRef CAS
.
- N. Liu, X. Liu and J. Pan, A new rapid synthesis of hexagonal prism Zn-MOF as a precursor at room temperature for energy storage through pre-ionization strategy, J. Colloid Interface Sci., 2022, 606, 1364–1373 CrossRef CAS
.
- R. Maric and H. Yu, Proton exchange membrane water electrolysis as a promising technology for hydrogen production and energy storage, Nanostruct. Energy Gener., Transm. Storage, 2019, 13 Search PubMed
.
- F. Y. Yi,
et al., Metal–organic frameworks and their composites: synthesis and electrochemical applications, Small Methods, 2017, 1(11), 1700187 CrossRef
.
- L. Liu,
et al., In Situ/Operando Insights into the Stability and Degradation Mechanisms of Heterogeneous Electrocatalysts, Small, 2022, 18(7), 2104205 CrossRef CAS
.
- L. Zhao,
et al., Nanopore confined anthraquinone in MOF-derived N-doped microporous carbon as stable organic cathode for lithium-ion battery. Energy Storage, Materials, 2019, 22, 433–440 Search PubMed
.
- L. Yue,
et al., Ni-MOF coating MoS2 structures by hydrothermal intercalation as high-performance electrodes for asymmetric supercapacitors, Chem. Eng. J., 2019, 375, 121959 CrossRef CAS
.
- G. J. Lim,
et al., Co/Zn bimetallic oxides derived from metal organic frameworks for high performance electrochemical energy storage, Electrochim. Acta, 2018, 291, 177–187 CrossRef CAS
.
- C. Yu,
et al., MOF-74 derived porous hybrid metal oxide hollow nanowires for high-performance electrochemical energy storage, J. Mater. Chem. A, 2018, 6(18), 8396–8404 RSC
.
- Q. Zhang,
et al., All-metal-organic framework-derived battery materials on carbon nanotube fibers for wearable energy-storage device, Adv. Sci., 2018, 5(12), 1801462 CrossRef
.
- P. Suganya,
et al., MOF assisted synthesis of new porous nickel phosphate nanorods as an advanced electrode material for energy storage application, J. Solid State Electrochem., 2019, 23, 3429–3435 CrossRef CAS
.
- B. He,
et al., Self-sacrificed synthesis of conductive vanadium-based metal–organic framework nanowire-bundle arrays as binder-free cathodes for high-rate and high-energy-density wearable Zn-ion batteries, Nano Energy, 2019, 64, 103935 CrossRef CAS
.
- L. Kong,
et al., MOF derived nitrogen-doped carbon polyhedrons decorated on graphitic carbon nitride sheets with enhanced electrochemical capacitive energy storage performance, Electrochim. Acta, 2018, 265, 651–661 CrossRef CAS
.
- Q. Wang,
et al., Flower-shaped multiwalled carbon nanotubes@ nickel-trimesic acid MOF composite as a high-performance cathode material for energy storage, Electrochim. Acta, 2018, 281, 69–77 CrossRef CAS
.
- X. Wei,
et al., Metal-organic framework-derived hollow CoS nanobox for high performance electrochemical energy storage, Chem. Eng. J., 2018, 341, 618–627 CrossRef CAS
.
- P. Xiao,
et al., Sub-5 nm ultrasmall metal–organic framework nanocrystals for highly efficient electrochemical energy storage, ACS Nano, 2018, 12(4), 3947–3953 CrossRef CAS
.
- Y. Li,
et al., Exposing {001} crystal plane on hexagonal Ni-MOF with surface-grown cross-linked mesh-structures for electrochemical energy storage, Small, 2019, 15(36), 1902463 CrossRef
.
- W. Jiang,
et al., A coin like porous carbon derived from Al-MOF with enhanced hierarchical structure for fast charging and super long cycle energy storage, Carbon, 2019, 154, 428–438 CrossRef CAS
.
- T. Huang,
et al., Metal-Organic-Framework-Derived MCo2O4 (M= Mn and Zn) Nanosheet Arrays on Carbon Cloth as Integrated Anodes for Energy Storage Applications, ChemElectroChem, 2019, 6(23), 5836–5843 CrossRef CAS
.
- Z.-B. Zhai,
et al., Metal–organic framework derived small sized metal sulfide nanoparticles anchored on N-doped carbon plates for high-capacity energy storage, Dalton Trans., 2019, 48(14), 4712–4718 RSC
.
- K. Zhao,
et al., High-yield bottom-up synthesis of 2D metal–organic frameworks and their derived ultrathin carbon nanosheets for energy storage, J. Mater. Chem. A, 2018, 6(5), 2166–2175 RSC
.
- F. Saleki,
et al., MOF assistance synthesis of nanoporous double-shelled CuCo2O4 hollow spheres for hybrid supercapacitors, J. Colloid Interface Sci., 2019, 556, 83–91 CrossRef CAS
.
- X. Wei,
et al., A novel functional material of Co3O4/Fe2O3 nanocubes derived from a MOF precursor for high-performance electrochemical energy storage and conversion application, Chem. Eng. J., 2019, 355, 336–340 CrossRef CAS
.
- L. Ren,
et al., Metal-organic-framework-derived hollow polyhedrons of prussian blue analogues for high power grid-scale energy storage, Electrochim. Acta, 2019, 321, 134671 CrossRef CAS
.
- Z. Liang,
et al., Synergistic effect of Co–Ni hybrid phosphide nanocages for ultrahigh capacity fast energy storage, Adv. Sci., 2019, 6(8), 1802005 CrossRef PubMed
.
- Y. Zhao,
et al., Carbon Cloth Modified with Metal-Organic Framework Derived CC@CoMoO4-Co(OH)2 Nanosheets Array as a Flexible Energy-Storage Material, ChemElectroChem, 2019, 6(13), 3355–3366 CrossRef CAS
.
- S. Sundriyal,
et al., Redox additive electrolyte study of Mn–MOF electrode for supercapacitor applications, ChemistrySelect, 2019, 4(9), 2585–2592 CrossRef CAS
.
- G. Zhu,
et al., A self-supported hierarchical Co-MOF as a supercapacitor electrode with ultrahigh areal capacitance and excellent rate performance, Chem. Commun., 2018, 54(74), 10499–10502 RSC
.
- H. Jia,
et al., Controlled synthesis of MOF-derived quadruple-shelled CoS2 hollow dodecahedrons as enhanced electrodes for supercapacitors, Electrochim. Acta, 2019, 312, 54–61 CrossRef CAS
.
- G. Li,
et al., Construction of hierarchical NiCo2O4@ Ni-MOF hybrid arrays on carbon cloth as superior battery-type electrodes for flexible solid-state hybrid supercapacitors, ACS Appl. Mater. Interfaces, 2019, 11(41), 37675–37684 CrossRef CAS
.
- M. S. Javed,
et al., An ultra-high energy density flexible asymmetric supercapacitor based on hierarchical fabric decorated with 2D bimetallic oxide nanosheets and MOF-derived porous carbon polyhedra, J. Mater. Chem. A, 2019, 7(3), 946–957 RSC
.
- H. Fan,
et al., One-Step MOF-Templated Strategy to Fabrication of Ce-Doped ZnIn2S4 Tetrakaidecahedron Hollow Nanocages as an Efficient Photocatalyst
for Hydrogen Evolution, Adv. Sci., 2022, 9(9), 2104579 CrossRef CAS
.
- S. K. Shinde,
et al., MOFs-graphene composites synthesis and application for electrochemical supercapacitor: a review, Polymers, 2022, 14(3), 511 CrossRef CAS PubMed
.
- J. Li,
et al., Facile synthesis of accordion-like porous carbon from waste PET bottles-based MIL-53 (Al) and its application for high-performance Zn-ion capacitor, Green Energy Environ., 2023 DOI:10.1016/j.gee.2023.01.002
.
- M. Z. Iqbal,
et al., Exploring MOF-199 composites as redox-active materials for hybrid battery-supercapacitor devices, RSC Adv., 2023, 13(5), 2860–2870 RSC
.
- N. Király,
et al., Sr(II) and Ba(II) Alkaline Earth Metal–Organic Frameworks (AE-MOFs) for Selective Gas Adsorption, Energy Storage, and Environmental Application, Nanomaterials, 2023, 13(2), 234 CrossRef
.
- X. Li,
et al., Metal–organic frameworks as a platform for clean energy applications, EnergyChem, 2020, 2(2), 100027 CrossRef
.
- C. G. Piscopo and S. Loebbecke, Strategies to Enhance Carbon Dioxide Capture in Metal-Organic Frameworks, ChemPlusChem, 2020, 85(3), 538–547 CrossRef CAS PubMed
.
- G. Cai,
et al., Metal–organic framework-based hierarchically porous materials: synthesis and applications, Chem. Rev., 2021, 121(20), 12278–12326 CrossRef CAS PubMed
.
- W. L. Teo,
et al., Industrializing metal–organic frameworks: Scalable synthetic means and their transformation into functional materials, Mater. Today, 2021, 47, 170–186 CrossRef CAS
.
- S. Sanati,
et al., Metal–organic framework derived bimetallic materials for electrochemical energy storage, Angew. Chem., Int. Ed., 2021, 60(20), 11048–11067 CrossRef CAS PubMed
.
- A. J. Rieth, A. M. Wright and M. Dincă, Kinetic stability of metal–organic frameworks for corrosive and coordinating gas capture, Nat. Rev. Mater., 2019, 4(11), 708–725 CrossRef CAS
.
- S. Li,
et al., Design principles and direct applications of cobalt-based metal-organic frameworks for electrochemical energy storage, Coord. Chem. Rev., 2021, 438, 213872 CrossRef CAS
.
- T. Chen,
et al., Recent progress on metal–organic framework-derived materials for sodium-ion battery anodes, Inorg. Chem. Front., 2020, 7(3), 567–582 RSC
.
-
L. M. Steele and Y. Agyeman, An Investigation of Innovative Energy Technologies Entering the Market between 2009-2015, Enabled by EERE-Funded R&D, Pacific Northwest National Lab.(PNNL), Richland, WA (United States), 2021 Search PubMed
.
- S.-H. Hwang, S.-D. Seo and D.-W. Kim, Metal–organic-framework-derived vanadium (iii) phosphate nanoaggregates for zinc-ion battery cathodes with long-term cycle stability, J. Mater. Chem. A, 2022, 10(19), 10638–10650 RSC
.
- S. Fu,
et al., Porous carbon-hosted atomically dispersed iron–nitrogen moiety as enhanced electrocatalysts for oxygen reduction reaction in a wide range of pH, Small, 2018, 14(12), 1703118 CrossRef
.
- V. Novotny,
et al., Review of carnot battery technology commercial development, Energies, 2022, 15(2), 647 CrossRef CAS
.
- Z. Chen,
et al., Reticular chemistry for highly porous metal–organic frameworks: the chemistry and applications, Acc. Chem. Res., 2022, 55(4), 579–591 CrossRef CAS
.
- S. M. Majhi,
et al., Metal–organic frameworks for advanced transducer based gas sensors: review and perspectives, Nanoscale Adv., 2022, 4(3), 697–732 RSC
.
- I. E. Khalil,
et al., The role of defects in metal–organic frameworks for nitrogen reduction reaction: when defects switch to features, Adv. Funct. Mater., 2021, 31(17), 2010052 CrossRef CAS
.
- Y. Fang, X. Y. Yu and X. W. Lou, Formation of hierarchical Cu-doped CoSe2 microboxes via sequential ion exchange for high-performance sodium-ion batteries, Adv. Mater., 2018, 30(21), 1706668 CrossRef
.
- R. Matheu,
et al., Three-dimensional phthalocyanine metal-catecholates for high electrochemical carbon dioxide reduction, J. Am. Chem. Soc., 2019, 141(43), 17081–17085 CrossRef CAS
.
- Y. Fang,
et al., Synthesis of CuS@CoS2 double-shelled nanoboxes with enhanced sodium storage properties, Angew. Chem., Int. Ed., 2019, 58(23), 7739–7743 CrossRef CAS PubMed
.
- C. Wang,
et al., Metal–organic frameworks and their derived materials: emerging catalysts for a sulfate radicals-based advanced oxidation process in water purification, Small, 2019, 15(16), 1900744 CrossRef
.
- S. C. Sekhar,
et al., Metal–Organic Framework-Derived Co3V2O8@CuV2O6 Hybrid Architecture as a Multifunctional Binder-Free Electrode for Li-Ion Batteries and Hybrid Supercapacitors, Small, 2020, 16(48), 2003983 CrossRef CAS PubMed
.
- W. Li,
et al., Sorption Thermal Energy Storage Performance of Nanoporous Metal–Organic Frameworks and Covalent Organic Frameworks by Grand Canonical Monte Carlo Simulations, ACS Appl. Nano Mater., 2023, 6(14), 13363–13373 CrossRef
.
- V. Nozari,
et al., Ionic liquid facilitated melting of the metal-organic framework ZIF-8, Nat. Commun., 2021, 12(1), 5703 CrossRef CAS PubMed
.
- Y. Fang,
et al., Rationally designed three-layered Cu2S@ Carbon@ MoS2 hierarchical nanoboxes for efficient sodium storage, Angew. Chem., 2020, 132(18), 7245–7250 CrossRef
.
- P. Li,
et al., Metal-organic frameworks with photocatalytic bactericidal activity for integrated air cleaning, Nat. Commun., 2019, 10(1), 2177 CrossRef
.
|
This journal is © The Royal Society of Chemistry 2024 |