DOI:
10.1039/D3GC03434H
(Critical Review)
Green Chem., 2024,
26, 42-56
High-value utilization of lignin: construction of an intelligent release system for targeting the delivery of pesticides
Received
11th September 2023
, Accepted 22nd November 2023
First published on 23rd November 2023
Abstract
The utilization of biomass for the production of agricultural green inputs is regarded as a crucial strategy for achieving low-carbon development in agriculture, while also fully harnessing the potential of renewable resources. Pesticides, as a vital agricultural input, often encounter issues pertaining to inefficient usage, resulting in significant environmental pollution and economic losses. As a main component of lignocellulosic biomass, lignin has become one of the most appealing biopolymers for the construction of advanced pesticide delivery systems. This review aims to provide a thorough summary of the advancements in lignin-based controlled release formulations (LCRFs) for the precise delivery of pesticides. The research in this field has experienced rapid growth in the past five years, making it an important area of study. Common LCRFs are introduced, and the factors influencing the release of active ingredients (AIs) within different LCRFs are analyzed. Special emphasis is placed on intelligent-responsive LCRFs, encompassing an overview of the existing formulations and an exploration of their potential application scenarios and development strategies. It is crucial to promote innovation in pesticide formulations based on the actual demands of agricultural production. We hope this review will stimulate the high-value utilization of lignin and the green development of plant protection technologies.
Introduction
Plant diseases are regarded as one of the most significant restrictions to agricultural productivity. According to statistics, globally, pests and diseases cause an average crop loss of 21.5% for wheat, 30.0% for rice, 22.6% for maize, 17.2% for potatoes, and 21.4% for soybeans.1 Global economic loss from plant diseases brought on by bacteria, fungi, nematodes, and viruses exceeds $220 billion annually.2 Pesticides have an important role in improving global crop yields, relieving hunger, and ensuring adequate food quality. Humans have used drugs to prevent and control agricultural diseases since ancient times. However, to date, the use of pesticides still poses some pressing issues. One of the most common phenomena is that during pesticide spraying, off-target losses occur due to evaporation, photolysis, splashing, and bouncing. Studies have shown that during the actual production process, harmful organisms were exposed to only 0.1% of the amount of pesticide applied.3 Furthermore, most farmers rely on agrochemicals to control pests and abuse pesticides to improve agricultural product yields.4,5 The misuse of pesticides not only generates financial losses but also causes severe risks to the environment and non-target creatures.6–8 The aim of maintaining high crop yields while reducing the use of pesticides remains a great challenge.
As an increasingly valued technology, pesticide-controlled release involves specific techniques to control the release rate, quantity, and pathway of pesticides in media, such as soil, air, water bodies, and plants. Compared with traditional pesticide application, the use of a pesticide-controlled release system (CRS) reduces issues associated with chemical drift, leaching, volatilization, and degradation, thus enhancing the pesticide utilization efficiency and reducing occupational exposure risks, as well as minimizing detrimental effects on ecosystems and non-target organisms.9,10 Pesticide-controlled release formulation (CRF) is a pesticide dosage form that can realize the gradual release of active ingredients (AIs) from processed products. Among the numerous parameters that influence CRS, the selection of carrier materials for the formulation plays a crucial role in determining the performance of the delivery system. The ideal pesticide carrier material should not react with the core contents and should possess appropriate characteristics, including mechanical strength, solubility, fluidity, emulsifying property, permeability, and stability.11 Various inorganic materials such as clay,12 silica,13 metals/metal oxides,14 synthetic polymers, such as polyethylene glycol,15 polyurea,16 and polyhydroxyalkanoates,17 and natural organic polymer materials, such as alginates,18 chitosan,19 and lignin,20 have been explored for CRF carriers. Inorganic materials have advantages, such as high surface area, high drug delivery efficiency, and stable physical and chemical properties, but are usually monofunctional and difficult to degrade after entering the natural environment. Synthetic polymers have the characteristics of low cost and stable performance, but they largely rely on fossil resources. With the continued depletion of fossil resources, society needs to shift towards a more sustainable development mode, promoting the use of bioresources to replace fossil ones.21
In recent years, natural polymers have emerged as promising additives and carriers for constructing environmentally-friendly and high-performance CRFs because of their easy access, low prices, and biodegradability. Lignin, in particular, is a highly compelling subject that continues to garner increasing interest in this field. As one of the primary components of lignocellulose, lignin, after cellulose, stands as the second most abundant renewable bioresource. Additionally, it is the only natural aromatic polymer that is obtainable in substantial quantities from terrestrial plants.22,23 The pulp & paper industry generates a significant amount of lignin (over 50 million tons per year) as by-products,24 most of which have not been effectively utilized. The valorization of lignin through the conversion into aromatic chemicals and advanced fuels has emerged as an appealing pathway. However, due to the low selectivity of desired products, achieving profitability from this process can be challenging. On the other hand, utilizing lignin as a substitute for synthetic polymers in various applications as functional materials proves to be a more lucrative option. In recent years, lignin has been widely tested for the preparation of industrial polymeric materials, such as polyurethanes, phenolic, and epoxy resins, exhibiting comparable performance to synthetic materials while offering cost reduction.25 However, to fully unlock the potential of lignin, an exciting avenue worth exploring lies in utilizing lignin for the production of high-value materials, particularly micro/nanomaterials, which find widespread applications in medical and agricultural fields. The development of lignin-based controlled release formulations (LCRFs) of pesticides is now emerging as a promising strategy, which is not only beneficial for agricultural production, but also for the high-value utilization of biomass.
There has been an increasing number of reports on LCRFs. As a pesticide carrier material, lignin has the following advantages: (1) from the standpoint of formulation functionality, lignin contains a variety of functional groups that can be chemically modified (e.g., hydroxyalkylation, esterification, and amination) to tailor LCRFs for different agricultural scenarios.26 (2) As for pesticide utilization efficiency, the phenolic, ketonic, and chromophoric structures in lignin impart the biopolymer UV resistance and antioxidant properties, which can reduce the degradation of pesticides under natural conditions and prolong their effectiveness.27,28 (3) From an environmental protection standpoint, lignin is non-toxic, harmless, and naturally degradable without exhibiting cytotoxicity.29,30 (4) Considering crop growth, lignin degradation can transform into humus, thereby enhancing soil fertility.30 Moreover, the benzene ring, phenolic hydroxyl groups, and methoxy groups in lignin endow it with natural antibacterial activity,31,32 which can alleviate the damage caused by pathogenic bacteria to crops and be beneficial to their growth and development. Based on the multiple advantages, lignin has been designed for various LCRFs in recent years, with intelligent ones being the most striking. Intelligent LCRFs possess the capability to convert various stimuli into predetermined outputs, thereby altering or activating specific functional characteristics of the system. This is more in line with the trend of precision agriculture (Fig. 1). There are several excellent reviews on lignin conversion to materials, including those of smart ones used as sensors, biomedical systems, and shape-programmable materials.26,33,34 However, a comprehensive summary and analysis of the most recent advancements in the utilization of lignin as intelligent materials, specifically focusing on applications in the targeted delivery of pesticides in the past five years, has yet to be presented. Therefore, this review offers a summary of recently published papers on intelligent-responsive LCRFs, introducing typical systems, application scenarios, development strategies, and summarizing their trigger factors and release characteristics. The highlight of this study is the emphasis on the response patterns of lignin to various environmental stimuli, revealing the potential of lignin as an intelligent material used in agriculture. We hope this review will stimulate the high-value utilization of lignin and the green development of plant protection technologies.
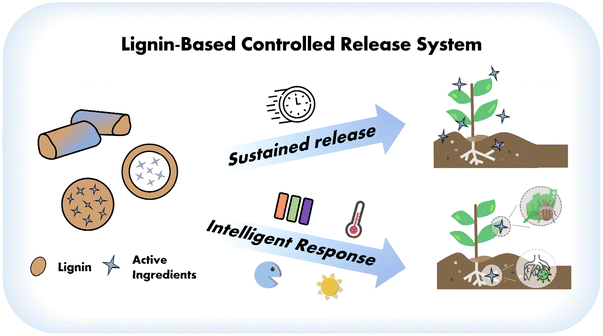 |
| Fig. 1 Development of lignin-based controlled release systems: from sustained release to intelligent response of active ingredients. | |
Typical LCRF systems
As compared to conventional burst-release formulations, sustained release systems gradually and consistently release AIs over an extended period of time, ensuring the long-lasting control of harmful organisms. By maintaining suitable concentrations of AIs around the target, sustained release technology allows for a reduction of environmental concerns associated with its implementation. This is achieved by incorporating AIs into specialized matrices or formulations. Since the late 20th century, attempts have been made to utilize lignin as the initial matrix for CRF.35,36 The source,35 structure,37 molecular weight,38 and concentration37 of lignin can all impact the release rate of AIs. Lignin macromolecules in various aggregation states form a variety of LCRF systems (Fig. 2). Some typical LCRF systems and their functional characteristics are summarized below.
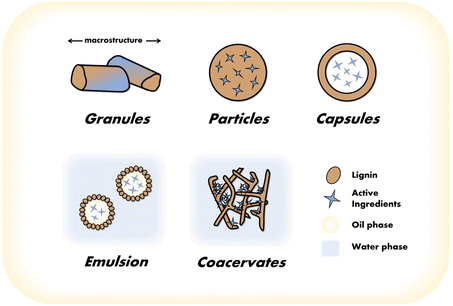 |
| Fig. 2 Different types of lignin-based controlled release systems. (In the figure, the granule system depicts the macrostructure, while the other systems illustrate the microstructure.) | |
Lignin-based granules
The lignin-AIs granular complex is the earliest system to be used for the sustained release of pesticides. The preparation method is relatively simple, involving the direct mixing of pesticides and lignin, followed by heating in a molten state for a certain period of time and subsequent cooling. Upon cooling, a glassy matrix is formed, from which the pesticide is released through diffusion. When preparing granules, the solubility parameters of the pesticide and lignin need to be considered. Only when the solubility parameter of the pesticide is similar to that of lignin can favorable compatibility be achieved.35 The size of granules is typically within the millimeter range, and it is the primary factor affecting the release rate. As the average granule size increases, it implies a longer distance for the AIs diffusion from the center of the matrix to the external surface, resulting in a slower release rate.39 Researchers have developed regression equations between the granule size and t50 (the time required for 50% of the AIs to be released), which indicates that the release rate of AIs from the granules can be tuned by regulating the particle size.39,40 However, due to only a portion of AIs having acceptable compatibility with lignin, as well as the potential loss of AIs during heating and melting, the application of granules is significantly restricted.
Lignin-based micro- or nanoparticles
Micro- and nanoparticles currently represent the most extensively studied LCRF system. Lignin-based micro- or nanoparticles typically possess adjustable sizes and shapes, with AIs being captured, encapsulated, or adhered to the surface of these nanoparticles.10 Special structures such as porous41 and hollow42 LCRF particle systems have also been reported. The anti-solvent method is commonly used for the preparation of micro- and nanoparticles, and the choice of solvent can serve as a means to adjust particle size. In the same solvent, an increase in lignin concentration leads to a reduction in particle diameter, but this reduction does not affect the encapsulation efficiency and drug loading of the system.43,44 Similar to granules, the release of AIs is mainly determined by their particle size, with smaller sizes resulting in faster release.45,46 Reducing the particle size can help to enhance the application performance of formulations (e.g., better dispersion at the foliar surface and better penetration into target organisms),47 but an excessively small particle size may alter the enrichment and fate of pesticides in the environment. Therefore, it is necessary to control the particle size to be within a range that can maintain the advantages of the small particle size, while mitigating risks.48
Lignin-based micro- or nanocapsules
Micro- or nanocapsules refer to egg-like structures with a core–shell architecture, which can encapsulate solid, liquid, or even gaseous AIs.63 Capsules can effectively control the release behavior of AIs, and protect them from degradation, as well as isolate harmful components from non-target organisms. In LCRFs, lignin often acts as the shell of the capsules. Unlike particle systems, which regulate release rates by adjusting the particle sizes, capsule systems modulate release by adjusting the thickness of the capsule shell. Increasing the thickness of the shell (or adding more layers) slows down the release. However, thicker shells typically result in a slight compromise in drug loading and encapsulation efficiency of the system.64,65 It should be noted that compared to particles, capsules usually exhibit a lag-time effect on the release of AIs (i.e., AIs usually take more time to release from the capsule) due to the physical separation between the AIs and the shell material.66
Lignin-based emulsions
An emulsion refers to a mixture of oil phase, water phase, and surfactant in varying proportions.67 It is also frequently used for encapsulation and the controlled release of sensitive chemicals. Due to the amphiphilic nature of lignin molecules,25 they can be partially or completely used as alternative surfactants after slight modification. Consequently, compared to particles and capsules, the use of lignin-based emulsion systems reduces the cost and potential environmental issues associated with the extensive use of surfactants. When all surfactants are replaced with lignin solid particles in an emulsion system, a specific emulsion called a lignin-based Pickering emulsion is formed.68 The release of AIs in emulsion systems is closely related to the stability of the emulsion, which is regulated by the content and amphiphilicity of lignin particles.59,60 Insufficient lignin at the oil–water interface hinders the formation of a stable emulsion, while an excessive content leads to aggregation and affects the emulsification performance. Lignin particles with excessive hydrophilicity or lipophilicity fail to stabilize well at the oil–water interface, requiring modification to achieve suitable amphiphilicity. Generally, higher stability of the emulsion results in a slower release of AIs.59,60
Lignin-based coacervates
In the current LCRFs system, aqueous-phase coacervates stand out as the only system that can be formed without the use of organic solvents, making them highly environmentally friendly.62 Lignin-based coacervates exhibit a rigid honeycomb-like network structure, providing a higher encapsulation efficiency for both hydrophilic and hydrophobic AIs. Simultaneously, it is precisely these network structures that can be entangled with the micro/nanostructures of the superhydrophobic foliage, resulting in superior wetting and anti-splash performance when the coacervate droplets are high-speed sprayed on leaf surfaces.62 The development of lignin-based coacervates is still in its nascent stage, but holds tremendous potential for various applications.
The applications of these aforementioned systems for the controlled release of pesticides are summarized in Table 1. It is important to note that there is no single exclusive and one universal lignin type that is best suited for the preparation of LCRFs. Thus, it should be selected based on the desired properties of the final products. For instance, sodium lignosulfonate is often employed as pesticide dispersants due to its favorable water solubility and polyanionic properties. Kraft lignin has low solubility under acidic conditions, and can be used to prepare lignin nanoparticles through acid precipitation method.69 Meanwhile, the pursuit of excessively prolonged release times should be avoided, and choices should be made based on specific agricultural scenarios to prevent the loss of maximum therapeutic effects.
Table 1 Typical lignin-based controlled release formulations
Systems |
Raw materials |
Active ingredients (AIs) |
AI classes |
Drug loading (%) |
Application properties |
Ref. |
Encapsulation efficiency (%) |
Not mentioned.
|
Granules |
Kraft lignin, alginate |
Azadirachtin |
Plant-derived insecticide |
2.81 |
Drug release followed the Higuchi mode. 58% azadirachtin was released in 550 h |
49
|
The encapsulated azadirachtin remained at 100% after irradiating by sunlight for 192 h |
99.5 |
Lignin as a biosorbent in alginate was used to control the release rate and stabilize the botanical insecticide |
Kraft lignin, ethylcellulose, polyethylene glycol |
Metribuzin and chloridazon |
Herbicide |
9.24–14.27 |
Reduced leaching of herbicides, thus mitigating the pollution of groundwater |
50
|
85.64–99.41 |
Particles |
Sodium lignosulfonate, cetyltrimethylammonium bromide (CTAB) |
Avermectin |
Insecticide and acaricide |
|
The cumulative release amounts ranged from 56.27% to 87.33% in 62 h |
45
|
The retention rates ranged from 46.67% to 63.41% after 50 h ultraviolet irradiation |
|
Enhanced plant surface adhesion and scouring resistance |
Benzoic acid esterified lignin sulfonates |
Difenoconazole |
Fungicide |
|
Drug release followed the Fickian diffusion. The cumulative release rate was 74% after 1248 h |
51
|
|
The decomposition rate was 66% after 24 h of UV radiation |
Better efficacy and foliar retention |
Alkaline lignin, cationic surfactant |
Abscisic acid |
Plant growth regulator |
20.66–26.15 |
Drug release followed the Fickian diffusion. The cumulative release amounts ranged from 22.4% to 34% in 72 h |
44
|
61.97–78.31 |
The retention rates of abscisic acid ranged from 34% to 75% in 60 h |
Highly effective in protecting rice seedlings from salinity stress |
Alginate, chitosan, sodium lignosulfonate |
Emamectin benzoate |
Insecticide |
62.6 |
Drug release followed the non-Fickian diffusion. The amount of AIs released was 49.4% within 60 h |
52
|
93.3 |
The degradation amount was 10% within 10 h |
Methacrylated lignin sulfonate |
Prothioconazole and pyraclostrobin |
Fungicide |
|
The cumulative release range was 45% after 5 days |
53
|
77–97 |
High stability and encapsulation efficiency |
Lignin-based azo polymer |
Avermectin |
Insecticide and acaricide |
16.80 |
The cumulative release amount within 120 h was 84.10% |
42
|
61.49 |
The decomposition rate was less than 41% after 72 h of UV irradiation |
Alkali lignin, cetyltrimethylammonium bromide (CTAB) |
Abscisic acid |
Plant growth regulator |
78 |
The cumulative release range was 35.5% at 72 h |
43
|
Greater than 75% of AIs could be retained after 60 h of irradiation |
>70 |
Thermal stability has been improved, leading to a good inhibition of seed germination and drought resistance performance |
Quaternary ammonium lignin, sodium dodecyl benzenesulfonate (SDBS) |
Avermectin |
Insecticide and acaricide |
42.34–63.19 |
The cumulative release amount within 72 h was 77% |
54
|
85.31–96.25 |
More than 85% of AIs could be preserved after 96 h of UV irradiation |
Capsules |
Alkali lignin, crosslinker |
Avermectin |
Insecticide and acaricide |
11.2–20.6 |
The cumulative release amounts ranged from 73.9% to 83.6% in 40 h |
55
|
61.2–76.4 |
After 70 h of UV irradiation, 88.9–93.7% of AIs were retained |
Kraft lignin, surfactants |
Propiconazole |
Fungicide |
5.6 |
The AIs remained at 15.7% by the end of the second week |
56
|
56.1 |
High stability |
Sodium lignosulfonate |
Pyraclostrobin |
Fungicide |
|
The cumulative release proportion reached 96.6% at 4 h |
46
|
>90 |
Excellent control against soilborne fungal diseases and lower residual risk |
Chitosan, sodium lignosulfonate |
Picloram |
Herbicide |
93.3–96.7 |
Drug release followed the Fickian diffusion. The time required for 50% release was 2.26 h |
57
|
49.8–87.6 |
More than 50% remained after being exposed to UV light irradiation for 30 min |
Alkali lignin, polyurea |
Avermectin |
Insecticide and acaricide |
|
The 85% cumulative release amount took 72 h |
58
|
|
The retention rate was up to 72% after being exposed to UV light for 120 h |
Kraft lignin |
Propiconazole |
Fungicide |
|
As a wood preservative, it exhibited enhanced antifungal resistance |
32
|
|
Emulsions |
Phenolated alkali lignin |
Avermectin |
Insecticide and acaricide |
52–72 |
The 300 h-cumulative release rates reached about 45% |
59
|
86–95 |
The retention rate after UV irradiation for 48 h was 78.65% |
High viscosity for reduced droplet bounce |
Amino acid-modified enzymatic hydrolysis lignin, surfactants |
Avermectin |
Insecticide and acaricide |
|
Drug release followed the non-Fickian diffusion. 100% of AIs were released after 6000 min |
60
|
|
The highest residual level was 80.1% after UV radiation for 72 h |
Cellulolytic enzyme lignin or alkali lignin or organosolv lignin |
Curcumin |
Drug |
|
The remaining curcumin content was greater than 70% after 36 h UV radiation |
61
|
|
Coacervates |
Sodium lignosulfonate, cationic surfactants |
Abscisic acid |
Plant growth regulator |
|
More than 40% of AIs remained after 72 h UV radiation |
62
|
40–90 |
Improved deposition performance on superhydrophobic leaves and high drought resistance of crops |
Intelligent response for LCRF: trigger factors and release characteristics
Due to the complex and ever-changing environmental conditions and diseases in agricultural production, the release performance of AIs from CRFs is susceptible to external influences. As a result, this often leads to a deviation in the release rate from the original design, which would weaken the benefits of the original formulation and reduce its efficacy. Therefore, there is a growing desire for AIs to exhibit targeted responsiveness to diseases or infestation occurrences. The controlled release of pesticides with an environmental intelligent-response is another breakthrough in plant protection technology after CRS. Based on the differences in the release sources of trigger signals, response factors can be broadly categorized into biogenic responses (e.g., pH response, enzyme response, redox response) and environmental-source responses (e.g., temperature response, photo response, CO2 response). Strategies for developing these two types of responsive formulations should have their own emphasis. Biogenic-responsive LCRFs should focus on the ability of the formulation to effectively penetrate pests or plants, because only after entering the body can the AIs be released by the trigger signal in the organism. It has been demonstrated that pesticide nanocarriers, such as lignin-based nanoparticles, can effectively penetrate plant tissues due to their small size, thereby increasing the magnitude of pesticide uptake and promoting the desired effect.70 Environmental-source responsive LCRFs are concerned with the sensitivity of the responsive material. Due to the strong feedback regulation of the ecosystem, the environmental changes caused by agricultural diseases are often relatively minor. When applied on the ground, the response material should be sensitive enough to respond to these minor changes. Environmental-source responsive LCRFs will be a fascinating challenge in the future.
The intelligent response of AIs in CRFs needs the support of intelligent materials. Although overlooked by many, lignin is actually a kind of intelligent material. The following section will illustrate the release triggers and mechanisms of intelligent-responsive LCRFs from the nature of lignin in combination with environmental changes in agricultural production.
pH response
Triggers of pH response in agricultural scenarios.
The pH features of pests, bacterial colonies, and plants themselves offer a basis for setting triggers. The digestive tracts of different insect species exhibit specific pH values under long-term evolution due to the nutrients they feed on. Most lepidopteran13 and coleopteran71 insects have relatively higher alkaline pH in their midguts. The intestinal lumen of Drosophila is alkali, while the central “stomach” of the midgut is naturally acidic.72 The pH value of different plant tissues also varies. Most plant tissues are weakly acidic, but phloem is weakly alkaline.73,74 Many plants are more vulnerable to phloem pathogens, most notably the Citrus Huanglongbing caused by Candidatus Liberibacter asiaticus.75 The specific alkalinity of plant phloem can act as a trigger for pH-responsive pesticide release.
The interaction of environmental stress with the crop might also result in specific pH values. When some pathogenic bacteria infect the host, they secrete acidic chemicals like citric acid and gluconic acid, thus acidifying the host. It has been found that the process of environmental acidification caused by pathogenic bacteria facilitates the enhancement of their pathogenicity.76 In response to biotic or abiotic stress, crops also release acidic root exudates, which are related to the initiation of plant defense mechanisms.77
pH is the most representative and widely used stimulus. When designing intelligent materials, it is vital to examine not only the sensitivity of the material to the response, but also its specific usage scenarios to achieve the transition from laboratory to farmland.
Release characteristics of the LCRFs response to pH.
Alkali lignin shows different solubility under different pH conditions, which is an important reason why lignin can be applied as a pH-responsive intelligent material. In an alkaline aqueous solution, acidic groups such as phenolic hydroxyl and carboxyl groups of alkali lignin are deprotonated and negatively charged. The electrostatic repulsion between these groups stretches the macromolecular network of lignin, causing it to dissolve in the solution (Fig. 3a).59 The predominant pH-responsive LCRFs commonly observed are alkaline-responsive releases. In the study by Wu et al.,41 porous lignin microspheres with bifunctional adsorption and controlled release were prepared by an inverse suspension copolymerization method. The lignin microspheres loaded with 2,4-dichlorophenoxyacetic acid showed an explosive release at pH = 11 with a release rate of 81.95% within 4 h. Conversely, it was only 25.78% at pH = 2 and achieved almost no release under neutral conditions. Under alkaline conditions, the microcapsules created by our group64 through the layer-by-layer assembly of chitosan and lignin also displayed notable release characteristics (Fig. 3b). In addition to the solubility of lignin, the weaker interactions between the two materials under alkaline conditions were responsible for the abrupt release of AIs.64 Plant phloem diseases, lepidopteran and coleopteran pests with alkaline intestinal tracts can be well prevented and controlled with alkali-responsive LCRFs.
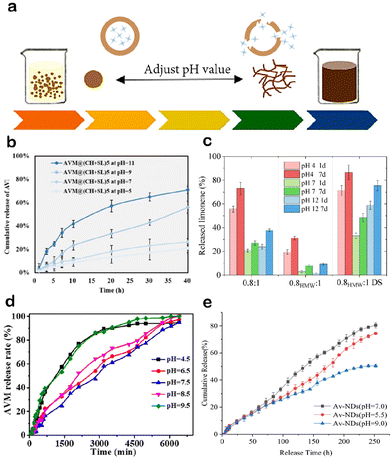 |
| Fig. 3 pH-Responsive LCRFs: (a) mechanism of alkali-responsive LCRFs mediated by the solubility of lignin. Adapted with permission from ref. 59. Copyright 2022 from Elsevier. (b) Effects of pH on the release performance of avermectin (AVM) from AVM@(CH + SL)5. Adapted with permission from ref. 64. Copyright 2023 from Royal Society of Chemistry. (c) The pH stability of lignin-based microcapsules samples. Adapted from ref. 79. (d) Influence of the environmental pH on the release behavior of lignin-based emulsion. Adapted with permission from ref. 60. Copyright 2021 from American Chemical Society. (e) Release curve of avermectin nano-delivery system (Av-NDs) in 70% methanol with different pH values. Adapted with permission from ref. 80. Copyright 2021 from Springer Nature. | |
There have been scattered reports on acid-responsive release, acid/alkali-responsive release, and neutral-responsive release by using lignin as intelligent material. The “cation–π” effect is primarily responsible for the theory underlying the release of AIs in acidic environments.78,79 Lignin, as an aromatic polymer, interacts with a large amount of H+ (and other possible cations) in acidic solutions, favoring the formation of extended conformations, leading to the disassembly of LCRF and the release of AIs (Fig. 3c).78,79 Chen et al.60 developed a lignin-based emulsion for encapsulating avermectin (AVM), where the release of AVM under neutral conditions was relatively low (Fig. 3d). This is because the carboxylic acid groups in lignin ionize in a medium with a pH of 7.5, generating a strong electrostatic repulsion that maintains the stability of the emulsion.60 Microspheres with the highest release rate of AVM under neutral conditions have also been reported (Fig. 3e).80
The pH value also influences the intermolecular forces within the LCRF, thereby controlling the release of AIs.81,82 Furthermore, grafting pH-sensitive polymers onto lignin is an excellent strategy for preparing pH-responsive LCRFs.83,84
Enzyme response
Triggers of enzyme response in agricultural scenarios.
Enzymatic triggers play a pervasive role in agricultural production, rendering their application in intelligent agrochemical delivery highly beneficial. This strategy capitalizes on the exceptional efficiency and specificity of enzymatic reactions. Pests, plant pathogenic fungi, and bacteria can all create a variety of enzymes to gain nutrients from the outside world. Phytophagous insects possess a diverse array of digestive enzymes in their gastrointestinal tract. These enzymes can be categorized into distinct groups based on their digestive targets, such as amylase, protease, lipase, and cellulase.85–87 In addition, during the process of infecting host plants, phytopathogenic fungi and bacteria secrete a series of cell wall-degrading enzymes such as pectinase, cellulase, glycosidase, and xylanase, which are conducive to the invasion, colonization, and expansion of pathogens.88,89 Research studies have reported on the use of zein or α-cyclodextrin as carriers in CRF that can be triggered for release by proteases or α-amylase in the gut of insects.90,91
Various enzymes with lignin-degrading functions have been recognized, including lignin peroxidase, manganese peroxidase, versatile peroxidase, laccase, and others.92 Lignin-degrading enzymes catalyze the cleavage of a series of lignin linkages, and play an essential role in the efficient utilization of lignin.93,94 Among them, laccase is a digestive enzyme that is present in the gut of lepidopteran species,95 and is also abundant in fungus secretions.96 Taking advantage of the fact that lignin can be degraded by laccases, many enzyme-responsive LCRFs have been designed, which will be discussed in detail below.
Release characteristics of the LCRFs response to enzymes.
In enzyme-responsive drug-loading systems, two mechanisms are typically used in design applications. The first mechanism entails the conversion of prodrugs into active pharmaceutical compounds facilitated by specific enzymes. This transformation enables the desired therapeutic effects, and is commonly observed in medical delivery systems.97 The second mechanism involves the degradation of controlled-release matrix by specific enzymes, thereby achieving targeted drug delivery. Most of the reported mechanisms for enzyme-responsive LCRF are of the latter kind. Specifically, enzymes destroy the drug-loading system by degrading lignin itself or lignin grafts, thereby leading to the release of AIs.
In enzyme-responsive LCRFs, the most commonly used triggering enzymes are laccases. Wurm and coauthors have conducted several years of research to develop pesticide formulations for the treatment of the grapevine trunk disease Esca.98–100 Esca is a very contagious and devastating fungal disease caused by a fungus that secretes lignin-degrading enzymes like laccase. A combination of miniemulsion polymerization and solvent evaporation was used to produce lignin nanocarriers. The fungicides encapsulated by these nanocarriers were released only when infected, while no leakage of AIs was detected during storage or in the absence of infection (Fig. 4a and c). Zhang et al. have successfully developed lignin-based nanogel101 and nanocapsule48 suspensions as a means to combat lepidopteran pests. These formulations display selective reactivity towards the endogenous laccases present in these pests. In the latter research study, the authors ingeniously introduced polysaccharides into the nanocapsule suspension to optimize the drug delivery system, thereby conferring responsiveness to both pectinases and cellulases present within the digestive tracts of pests. In vivo experiments have conclusively shown the accelerated release of AIs from the carrier composed of lignin and polysaccharides.48
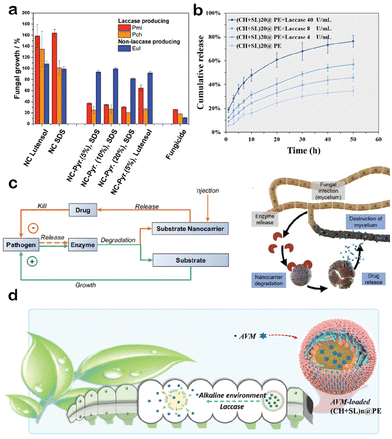 |
| Fig. 4 Enzyme-responsive LCRFs: (a) in vitro investigations of lignin nanocarriers (NC). Pyr stands for pyraclostrobin. Adapted with permission from ref. 98. Copyright 2019 from Wiley. (b) Effects of laccase on the release performance of avermectin (AVM) from (CH + SL)20@PE. Adapted with permission from ref. 65. Copyright 2023 from Wiley. (c) Concept of the nanocarriers-mediated drug delivery. Adapted with permission from ref. 98. Copyright 2019 from Wiley. (d) Schematic diagram of pH/laccase-responsive release of AVM-loaded microcapsules (CH + SL)n@PE in pests. Adapted with permission from ref. 65. Copyright 2023 from Wiley. | |
Researchers often combine enzyme response with pH response, and these dual-responsive LCRFs exhibit enhanced targeted lethality towards lepidopteran pests. Zhang et al.102 have established lignin nanoparticles with core–shell structures through electrostatic self-assembly, which accelerates the release of lambda-cyhalothrin under both alkaline conditions and the presence of laccases, with the latter triggering a more pronounced release. Our group65 has utilized the alternating deposition of chitosan and lignosulfonate on an alkali lignin-based Pickering emulsion to form microcapsules. Similarly, these microcapsules display dual responsiveness to pH and laccase. The shell thickness of the microcapsule can be adjusted through the deposition process, thereby enabling the tunable release behavior of the AIs (Fig. 4b and d).
In addition to degrading lignin itself, the enzymatic degradation of lignin-grafted materials can be used to prepare enzyme-responsive LCRFs.103 The high efficiency of the enzymatic reaction enables the rapid release of AIs when necessary, minimizing the negative “lag-time effect”.
Temperature response
Triggers of temperature response in agricultural scenarios.
The aforementioned reaction triggers are derived from the characteristics of crops, pests, and weeds, as well as their interactions, which exhibit strong response signals. In contrast, ambient temperature, being a climatic variable, does not experience significant fluctuations in a short period, yet some elements are highly sensitive to even minuscule changes of temperature. On the one hand, the severity of disease, pest, and weed damage is affected by temperature. A rise in ambient temperature may cause weeds to germinate earlier and increase the severity of weed damage. Large temperature fluctuations will also promote some weed germination.104 For diseases, each “plant–pathogen” interaction has an optimal temperature range. For example, 15 °C is the optimum temperature for cyst nematodes to infect potatoes,105 and rice blast is prone to epidemics at 24–26 °C and below.106 Temperature can influence disease incidence by impacting the behavior of vector insects as well.107 On the other hand, the infestation of diseases, pests, and weeds can cause temperature changes. Symptoms of scab disease on apple leaves appear to be associated with disruption of the apple cuticle by V. inaequalis conidiophores, resulting in a localized reduction in leaf temperature.108 For wheat stripe rust, irrespective of the crop growth stages, the canopy mean temperature increases with the disease progression.109 In comparison, changes in foliar temperature caused by crop infestation by pathogenic microorganisms such as fungi and viruses are more significant. Based on this change, researchers have built remote sensing technology to detect plant diseases non-destructively.110,111
When designing temperature-responsive LCRFs, two temperature values of lignin deserve special attention: the glass transition temperature (Tg) and the critical solution temperature. Lignin itself exhibits a high Tg, ranging from 90 °C to 145 °C, depending on the source and isolation process of lignin.26 By tuning Tg, lignin can be designed as different shape memory materials for smart applications, including vitrimer materials.112 The critical solution temperature is generally divided into the upper critical solution temperature (UCST) and the lower critical solution temperature (LCST). Limited by the relatively high Tg, researchers tend to exploit the critical solution temperature of lignin for the development of intelligent LCRFs.
Currently, most temperature-responsive polymers are LCST-type. Lignin-based LCST intelligent materials are primarily prepared by grafting classical temperature-sensitive polymers.113 The research on UCST is relatively less extensive compared to the widespread studies on LCST. Alcohol/water solvent mixtures are considered as promising solvents for various polymers to obtain UCST behavior.114 Related works have looked at the UCST behavior of lignin in ethanol/water mixed solvents, pointing out that it is the result of the combined action of the hydrogen bond and hydrophobic force of lignin.115 High temperature breaks the hydrogen bonds between lignin molecules, while also reducing the polarity of the ethanol/water mixed solvent. Consequently, the hydrophobic force of the solvent on lignin is stronger than that between lignin, resulting in lignin solvation. Thus, lignin slowly dissolves during the heating process in ethanol/water, changing from two-phase to single-phase, and the UCST behavior occurs (Fig. 5a).115 However, the UCST behavior of lignin is solvent-dependent, and it does not exhibit UCST behavior in pure water.116
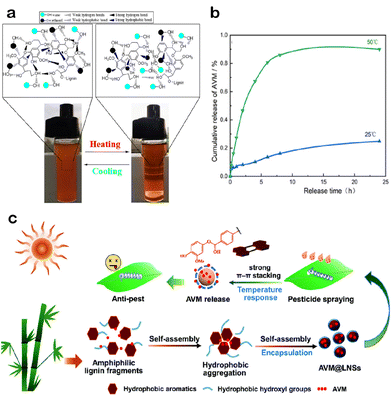 |
| Fig. 5 Temperature-responsive LCRFs: (a) mechanism of UCST behavior of enzymatic hydrolysis lignin (EHL) in 50% (v/v) ethanol/water. (b) Drug release of avermectin-loaded EHL microspheres at different temperatures. Adapted with permission from ref. 115. Copyright 2021 from American Chemical Society. (c) The process from encapsulation to the release of avermectin (AVM) by lignin nanospheres. Adapted with permission from ref. 117. Copyright 2022 from Royal Society of Chemistry. | |
Release characteristics of the LCRFs response to temperature.
Lin et al.115 revealed the UCST behavior of lignin in ethanol/water mixtures, and applied it to the controlled release of pesticides. The anti-solvent method was used to prepare enzymatic hydrolysis lignin microspheres loaded with AVM, and the lignin retained its UCST behavior after microsphere formation via microstructure regulation. Experiments revealed that at 25 °C, only 20% of avermectin was released. However, at 50 °C, this value was up to 90% (Fig. 5b). In fact, this research can be optimized further because agricultural production under natural conditions rarely reaches 50 °C. Shen et al.117 fabricated lignin nanospheres for encapsulating AVM. Under xenon lamp-replicated solar radiation, the nanosphere solution undergoes photothermal conversion, rapidly heating up. Consequently, the structure of lignin nanospheres gradually swelled and even dissolved, releasing AVM in an ethanol/water solution (Fig. 5c). Experimental evidence demonstrates that these nanospheres exhibit better pest control efficacy at 32 °C compared to 12 °C.
It has been reported that graft polymerization of lignin with NIPAAm can form thermosensitive hydrogels.118,119 The LCST of NIPAAm is close to the natural temperature, which is approximately 32 °C.120 Furthermore, the LCST of the lignin–NIPAAm copolymer can be flexibly adjusted according to the lignin content. This adaptability enables the copolymer to effectively respond to diverse agricultural application scenarios, explaining its growing popularity in recent years. However, the NIPAAm monomer exhibits cytotoxicity.121 Thus, its effects on crops, non-target organisms, and the environment must be carefully investigated in order to develop green and safe temperature-sensitive LCRFs.
Promising and underexploited intelligent LCRFs: potentials and ideas
The microenvironment of crop growth is closely linked and mutually influenced by disease occurrence. In addition to the aforementioned environmental triggering factors, light intensity, redox potential, gas concentration, and other factors are key components of the microenvironment for crop growth (Fig. 6). Understanding the variation patterns between the two can help develop the spatiotemporal targeting LCRFs. It should be pointed out that these response triggers may have been explored in other fields. However, there are few publications on the LCRFs. Lignin can be used to create lignin-based intelligent materials through grafting or crosslinking with responsive polymers. Additionally, its wide availability and rich functional groups make the biopolymer a promising candidate for exploring its own potential as an intelligent material. The following section will delve into the application potential and research directions of intelligent LCRFs activated by other stimuli.
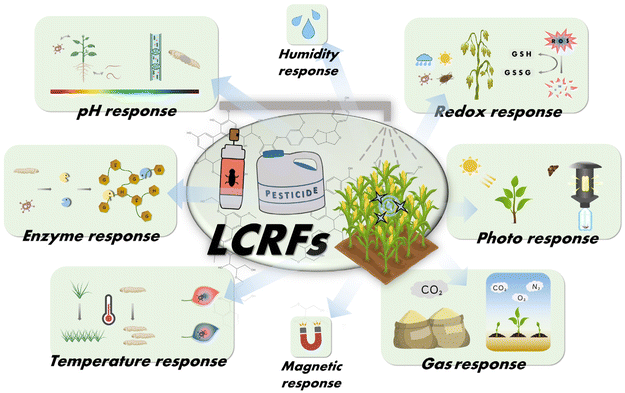 |
| Fig. 6 Various intelligent lignin-based controlled release formulations (LCRFs) activated by different stimuli used in agricultural production. | |
Photo-responsive LCRFs
Photo-responsive intelligent systems for the controlled release of pesticides have been widely reported in recent years, with a wide range of application settings.122,123 In one aspect, photosynthesis is a unique and vital physiological and biochemical process in green plants. By utilizing photosynthesis-inhibiting herbicides that only release when the weeds are exposed to sunlight, the effectiveness of the pesticide application can be greatly enhanced.19 In another aspect, light trapping technology for pest control is one of the main methods used in physical pest management. By combining photo-responsive pesticide delivery systems with light-trapping devices, pests can be attracted and eradicated simultaneously. Photo-responsive CRF also holds great application prospects in greenhouses equipped with lighting devices.
The molecular structure of lignin contains numerous aromatic rings and conjugated functional groups, resulting in strong conjugation and π–π interactions between molecules. These characteristics grant lignin a range of unique optical properties, including aggregation-induced luminescence, UV absorption, and sustainable photothermal conversion, among others.124,125 Several reports have explored the coupling of lignin with photosensitive groups,126–128 some of which revealed its potential for preparing photo-responsive materials.126 However, its application in drug delivery is relatively scarce.
Photo-responsive LCRFs can be further converted into temperature-responsive LCRFs due to the photothermal conversion action of lignin,124 as introduced in the preceding content.117 The inherent excellent optical properties of lignin can revitalize photo-responsive LCRFs.
Redox-responsive LCRFs
Many factors influence redox reactions in agricultural output. For crops, most abiotic and biotic stresses induce changes in cellular redox homeostasis, and increased the generation and accumulation of reactive oxygen species (ROS).129 Correspondingly, pests and pathogens have evolved various strategies to evade plant defense systems. Among them, some insects maintain the reducing state of the midgut by secreting antioxidants like glutathione.130 It has been found that glutathione accumulates in many plant–pathogen interactions to minimize the adverse impacts of ROS on cells.131
Utilizing redox reactions to trigger the release of AIs in the context of drug delivery systems is a well-established approach. A promising strategy to induce this response involves incorporating disulfide bonds within the carriers. Glutathione, present in the environment, can act as a “scissor” to cleave the disulfide bonds in pesticide carriers, acting as an intelligent “switch” to release AIs. It has been reported that disulfide bonds are inserted into lignin modified with polyetheramine as an adhesive,132 while LCRFs with disulfide bonds have not been widely reported.
Lignin possesses antioxidant properties due to the capacity of its phenolic groups to scavenge free radicals.133 When lignin undergoes oxidative reactions, some covalent bonds will break and its molecular weight will decrease.134 In fact, the enzymatic degradation mechanism of lignin is also the process of oxidative degradation.93,94 By employing the characteristic feature of lignin that is prone to decomposition in the presence of oxidative conditions caused by ROS generated from crops under stress, there is promising potential for the development of redox-responsive LCRFs. This novel approach shows great potential in enhancing crop resilience in the face of stressful conditions.
Gas-responsive LCRFs
Present studies in the field of gas-responsive systems predominantly concentrate on CO2, and CO2 triggers are of interest because of their cost-effectiveness, widespread availability, ecological and biological compatibility, as well as the ease with which they can be eliminated.135 CO2 is also a key factor influencing agricultural production. In the storage of grains, elevated levels of CO2 indicate the presence of mold spoilage or pest activity within the grain.136 If fungicides/pesticides can respond to the ambient CO2 concentration, it will effectively ensure the safety of grain storage. Besides pesticides, other gas-responsive agrochemicals are also worth considering. CO2 is a necessary raw material in agricultural photosynthesis. CO2 fertilization, a process that stimulates plant growth by increasing CO2 concentration,137 has been widely used in greenhouse cultivation. Studies have shown that the growth of plant biomass is limited by low levels of nitrogen and phosphorus when CO2 fertilization is implemented.138 Therefore, nitrogen or phosphorus fertilizers can be applied together with CO2 fertilization to enhance its efficiency. The development of CO2-responsive nitrogen and phosphorus fertilizers could reduce the labor-intensive nature of greenhouse horticulture.
CO2-switchability is induced by the presence of CO2-functional groups such as amidine, tertiary amine, imidazole, guanidine, or carboxylic acid, and lignin is advantageous for grafting these groups to elicit the response.135 DEAEMA-grafted lignin nanoparticles can be easily dispersed in water by CO2 bubbling, and swiftly precipitated out by N2 bubbling. Lignin-based Pickering emulsions made with these particles can switch between emulsified and demulsified states by bubbling different gases, which has great potential for drug delivery applications.139 Moreover, CO2 dissolved in water produces carbonic acid, so the gas response initiated by CO2 can be further turned into a pH response.
Challenges coupled and future outlook
In order to better capitalize on the remarkable features of LCRFs and better serve the need of actual agricultural production, the selection of pesticide formulations is crucial. In recent years, there have been numerous reports on novel and functional LCRFs, including seed coating,140 trunk injection,98–100 liquid mulching film,141 controlled-release adsorbent,41 and super-spreading agent.62 These advancements have expanded the application scenarios of lignin-based CRS. Pesticide formulations are currently evolving towards broad functionality, labor-saving efficiency, and enhanced pesticide utilization (Fig. 7).
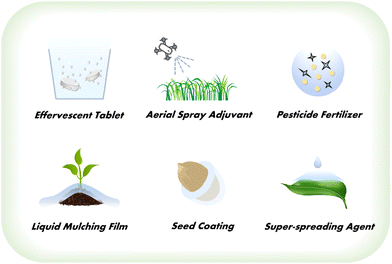 |
| Fig. 7 Versatile lignin-based controlled release system. | |
In recent years, the widespread application of plant protection unmanned aerial vehicle (UAV) has seen rapid progress in hardware and flight control systems. However, there exists a significant deficiency in the corresponding aerial spraying agents. Compared to conventional ground spraying, UAV application is characterized by low volume, high concentration, fine mist, elevated operational altitude, and extended spraying distance. These factors make it more susceptible to issues like evaporation, photolysis, and splashing, leading to ineffective application and higher frequency of drug damage accidents. LCRFs have the potential to reduce unintended losses when applying pesticides through conventional spraying methods, making them valuable assets in aerial crop protection. Numerous studies support this claim by demonstrating that certain LCRF systems have high viscosity, which promotes better adhesion of the agents to leaf surfaces.59,101 Additionally, some LCRFs form a topological structure with the wax layer on foliage surfaces, making them more resistant to rainfall.51 It is important to acknowledge that emerging LCRF systems based on lignin-based coacervates possess exceptional wetting and spreading properties of their own. These properties largely arise from the internal disordered network structure of coacervates and the entanglement of micro/nano structures on the surfaces of leaves, as previously mentioned.62 Overall, the use of LCRFs can enhance the precise application characteristics of intelligent-responsive systems, ultimately leading to improved efficiency in delivering pesticides to the target areas. In addition to aerial spray adjuvant, looking ahead, pesticide formulations combining drugs and fertilizers are a promising labor-saving agent. Researchers should drive pesticide formulation innovation based on practical agricultural production needs, promoting sustainable and environmentally friendly practices in the field of agriculture.
The research and development of LCRFs is still under continuous exploration, with several pressing issues awaiting resolution: (1) to enhance the reproducibility and repeatability of LCRF products, there is a need for an economical, eco-friendly, and efficient pretreatment technique, which would extract structurally ideal and stable lignin from pollutants or lignocellulose. (2) Although some intelligent-responsive LCRFs have been developed, further validation under field conditions is essential. This validation aims to confirm whether this responsiveness truly synchronizes with pest infestations, adapting to the intricate and ever-changing realities of agricultural production. (3) Tracing the remaining amounts of AIs in LCRF is crucial. This information is vital for farmers to determine when to replenish drugs. However, there is a lack of reports on this aspect in the existing literature. Comprehensive laboratory investigations are a necessary prerequisite for the commercial application of new products.
Before LCRFs can be widely commercialized, it is essential to subject them to rigorous toxic evaluation. The toxicity of new products often stands as a primary concern for the public. Being a natural substance, the basic ecotoxicity of lignin is not a problem as such. The problem may arise from necessary lignin modifications during the production process and the addition of surfactants or other substances. Indeed, many studies have evaluated the impact of LCRFs on seed vitality,142 plant growth,142 as well as their acute toxicity to natural insect predators and aquatic organisms48,65,102,143 under laboratory conditions. All of these studies concluded that lignin-based agrochemical encapsulation systems do not pose negative ecological impacts, and even partially exhibit enhanced safety quotient. Nonetheless, despite these findings, larger-scale studies of the long-term effects of LCRFs on ecosystems under actual field conditions should be necessary. Additionally, the occupational chronic toxicity of LCRFs to agricultural workers, and their potential accumulation in human cells through the food chain deserve attention.
Another pertinent issue pertaining to the commercial utilization of LCRFs is their costs. Given the critical nature of agriculture as a primary sector, responsible companies should prioritize the provision of affordable products to farmers in order to safeguard their livelihoods. Simultaneously, these companies should also bear the negative external costs associated with pesticides, which encompass the contamination of drinking water, loss of biodiversity, and potential effects on human health.144 Fortunately, the conversion from lignin to high-value materials is known to be commercially competitive.25 Substantial economic returns not only facilitate the successful transition of products from laboratory settings to actual production plants, but also drive the continuous innovation and improvement of factory-produced goods. Therefore, it is imperative for companies, research institutions, and all relevant stakeholders to collaboratively address these issues, and enable LCRFs to make significant contributions to the overall well-being of humanity.
Conclusions
Lignin polymers, due to their cost-effectiveness, green carbon footprint, tunable properties, and resistance to UV radiation, are considered ideal raw materials for constructing CRFs. As summarized in this review, numerous innovative LCRF systems have been developed and refined, resulting in improved effectiveness and environmental friendliness. The development of intelligent-responsive LCRFs is considered a noteworthy advancement in the field of plant protection, as it contributes to the progress of precision agriculture and low-carbon development. In this system, AIs are released based on the timing and location of disease occurrence, thereby increasing pesticide utilization efficiency while minimizing its harmful impact on the environment and non-target organisms. Many of the examples discussed in this review demonstrate that the sensitivity to various environmental stimuli allows lignin to be employed as an intelligent material in the construction of environmentally responsive LCRFs. In summary, LCRFs are a new pesticide formulation that is flourishing, experiencing a leap from sustained release to intelligent response, and will do much more in the foreseeable future.
Author contributions
Yitong Wang: conceptualization, writing – original draft. Xiaona Yu: conceptualization, writing – review & editing. Shuaishuai Ma: writing – review & editing. Shuling Cao: writing – review & editing. Xufeng Yuan: supervision. Wanbin Zhu: funding acquisition, supervision. Hongliang Wang: conceptualization, writing – review & editing, funding acquisition, supervision.
Conflicts of interest
There are no conflicts to declare.
Acknowledgements
This work was supported by the National Key R&D Program of China (2021YFA0716700), National Natural Science Foundation of China (22278422), the 2115 Talent Development Program of China Agricultural University Fund (1011-00109018), and Beijing Innovation Team of the Modern Agricultural Research System (BAIC08-2023-FQ02).
Notes and references
- M. Carvajal-Yepes, K. Cardwell, A. Nelson, K. A. Garrett, B. Giovani, D. G. O. Saunders, S. Kamoun, J. P. Legg, V. Verdier, J. Lessel, R. A. Neher, R. Day, P. Pardey, M. L. Gullino, A. R. Records, B. Bextine, J. E. Leach, S. Staiger and J. Tohme, Science, 2019, 364, 1237–1239 CrossRef CAS PubMed.
- S. He and K. M. Creasey Krainer, Mol. Plant, 2020, 13, 933–934 CrossRef CAS PubMed.
- Y. Zhang, B. Y. Liu, K. X. Huang, S. Y. Wang, R. L. Quirino, Z. X. Zhang and C. Q. Zhang, ACS Appl. Mater. Interfaces, 2020, 12, 37607–37618 CrossRef CAS PubMed.
- M. F. A. Jallow, D. G. Awadh, M. S. Albaho, V. Y. Devi and B. M. Thomas, Sci. Total Environ., 2017, 574, 490–498 CrossRef CAS.
- X. Guo, C. Wang and F. Zhang, Front. Agric. Sci. Eng., 2022, 9, 511–522 CrossRef.
- A. I. Sankoh, R. Whittle, K. T. Semple, K. C. Jones and A. J. Sweetman, Environ. Int., 2016, 94, 458–466 CrossRef CAS PubMed.
- A. Sharma, A. Shukla, K. Attri, M. Kumar, P. Kumar, A. Suttee, G. Singh, R. P. Barnwal and N. Singla, Ecotoxicol. Environ. Saf., 2020, 201, 110812 CrossRef CAS.
- A. Wang, A. Padula, M. Sirota and T. J. Woodruff, Fertil. Steril., 2016, 106, 905–929 CrossRef CAS PubMed.
- E. V. R. Campos, J. L. de Oliveira, L. F. Fraceto and B. Singh, Agron. Sustainable Dev., 2015, 35, 47–66 CrossRef CAS.
- A. Singh, N. Dhiman, A. K. Kar, D. Singh, M. P. Purohit, D. Ghosh and S. Patnaik, J. Hazard. Mater., 2020, 385, 121525 CrossRef CAS PubMed.
- T. Li, D. Teng, R. Mao, Y. Hao, X. Wang and J. Wang, J. Biomed. Mater. Res., Part A, 2019, 107, 2371–2385 CrossRef CAS.
- A. Chevillard, H. Angellier-Coussy, V. Guillard, N. Gontard and E. Gastaldi, J. Hazard. Mater., 2012, 205–206, 32–39 CrossRef CAS PubMed.
- Y. Gao, Y. Zhang, S. He, Y. Xiao, X. Qin, Y. Zhang, D. Li, H. Ma, H. You and J. Li, Chem. Eng. J., 2019, 364, 361–369 CrossRef CAS.
- J. Tang, G. Ding, J. Niu, W. Zhang, G. Tang, Y. Liang, C. Fan, H. Dong, J. Yang, J. Li and Y. Cao, Chem. Eng. J., 2019, 359, 225–232 CrossRef CAS.
- B. Liu, J. Zhang, C. Chen, D. Wang, G. Tian, G. Zhang, D. Cai and Z. Wu, J. Agric. Food Chem., 2021, 69, 6981–6988 CrossRef CAS PubMed.
- D. Zhang, B. Li, X. Zhang, Z. Zhang, W. Wang and F. Liu, J. Agric. Food Chem., 2016, 64, 2841–2846 CrossRef CAS PubMed.
- S. V. Prudnikova, A. N. Boyandin, G. S. Kalacheva and A. J. Sinskey, J. Polym. Environ., 2013, 21, 675–682 CrossRef CAS.
- S. Kumar, G. Bhanjana, A. Sharma, M. C. Sidhu and N. Dilbaghi, Carbohydr. Polym., 2014, 101, 1061–1067 CrossRef CAS PubMed.
- Z. Ye, J. Guo, D. Wu, M. Tan, X. Xiong, Y. Yin and G. He, Carbohydr. Polym., 2015, 132, 520–528 CrossRef CAS PubMed.
- Y. Li, M. Zhou, Y. Pang and X. Qiu, ACS Sustainable Chem. Eng., 2017, 5, 3321–3328 CrossRef CAS.
- R. Tao, C. You, Q. Qu, X. Zhang, Y. Deng, W. Ma and C. Huang, Environ. Sci.: Nano, 2023, 10, 351–371 RSC.
- H. Wang, Y. Pu, A. Ragauskas and B. Yang, Bioresour. Technol., 2019, 271, 449–461 CrossRef CAS PubMed.
- J. Xu, C. Li, L. Dai, C. Xu, Y. Zhong, F. Yu and C. Si, ChemSusChem, 2020, 13, 4284–4295 CrossRef CAS.
- D. S. Bajwa, G. Pourhashem, A. H. Ullah and S. G. Bajwa, Ind. Crops Prod., 2019, 139, 111526 CrossRef CAS.
- X. Yu, B. Yang, W. Zhu, T. Deng, Y. Pu, A. Ragauskas and H. Wang, Ind. Crops Prod., 2023, 200, 116824 CrossRef CAS.
- A. Moreno and M. H. Sipponen, Mater. Horiz., 2020, 7, 2237–2257 RSC.
- Z. Sun, B. Fridrich, A. de Santi, S. Elangovan and K. Barta, Chem. Rev., 2018, 118, 614–678 CrossRef CAS.
- Y. Zhang and M. Naebe, ACS Sustainable Chem. Eng., 2021, 9, 1427–1442 CrossRef CAS.
- M. Tortora, F. Cavalieri, P. Mosesso, F. Ciaffardini, F. Melone and C. Crestini, Biomacromolecules, 2014, 15, 1634–1643 CrossRef CAS.
- M. Tuomela, M. Vikman, A. Hatakka and M. Itävaara, Bioresour. Technol., 2000, 72, 169–183 CrossRef CAS.
- W. Yang, E. Fortunati, D. Gao, G. M. Balestra, G. Giovanale, X. He, L. Torre, J. M. Kenny and D. Puglia, ACS Sustainable Chem. Eng., 2018, 6, 3502–3514 CrossRef CAS.
- R. C. Andeme Ela, S. H. Chipkar, T. L. Bal, X. Xie and R. G. Ong, ACS Sustainable Chem. Eng., 2021, 9, 2684–2692 CrossRef CAS.
- D. Kai, M. J. Tan, P. L. Chee, Y. K. Chua, Y. L. Yap and X. J. Loh, Green Chem., 2016, 18, 1175–1200 RSC.
- S. Sugiarto, Y. Leow, C. L. Tan, G. Wang and D. Kai, Bioact. Mater., 2022, 8, 71–94 Search PubMed.
- J. V. Cotterill, R. M. Wilkins and F. T. Da Silva, J. Controlled Release, 1996, 40, 133–142 CrossRef CAS.
- M. Fernández-Pérez, E. González-Pradas, M. D. Ureña-Amate, R. M. Wilkins and I. Lindup, J. Agric. Food Chem., 1998, 46, 3828–3834 CrossRef.
- J. Cao, R. H. Guenther, T. L. Sit, S. A. Lommel, C. H. Opperman and J. A. Willoughby, Cellulose, 2016, 23, 673–687 CrossRef CAS.
- J. Rao, A. N. Chandrani, A. Powar and S. Chandra, Green Chem. Lett. Rev., 2021, 14, 204–220 CrossRef.
- M. Fernández-Pérez, M. Villafranca-Sánchez, F. Flores-Céspedes and I. Daza-Fernández, Carbohydr. Polym., 2011, 83, 1672–1679 CrossRef.
- F. J. Garrido-Herrera, I. Daza-Fernández, E. González-Pradas and M. Fernández-Pérez, J. Hazard. Mater., 2009, 168, 220–225 CrossRef CAS PubMed.
- H. Wu, L. Gong, X. Zhang, F. He and Z. Li, Chem. Eng. J., 2021, 411, 128539 CrossRef CAS.
- Y. Deng, H. Zhao, Y. Qian, L. Lü, B. Wang and X. Qiu, Ind. Crops Prod., 2016, 87, 191–197 CrossRef CAS.
- J. Yin, H. Wang, Z. Yang, J. Wang, Z. Wang, L. Duan, Z. Li and W. Tan, J. Agric. Food Chem., 2020, 68, 7360–7368 CrossRef CAS PubMed.
- J. Yin, M. Quan, Z. Wang, J. Wang, Z. Yang, L. Duan, Z. Li, Q. X. Li, H. Wang and W. Tan, Ind. Crops Prod., 2022, 177, 114573 CrossRef CAS.
- R. Peng, D. Yang, X. Qiu, Y. Qin and M. Zhou, Int. J. Biol. Macromol., 2020, 151, 421–427 CrossRef CAS PubMed.
- J. Luo, D.-x. Zhang, T. Jing, G. Liu, H. Cao, B.-x. Li, Y. Hou and F. Liu, Chem. Eng. J., 2020, 394, 124854 CrossRef CAS.
- S. Kumar, M. Nehra, N. Dilbaghi, G. Marrazza, A. A. Hassan and K.-H. Kim, J. Controlled Release, 2019, 294, 131–153 CrossRef CAS.
- D. Zhang, R. Wang, C. Ren, Y. Wang, B. Li, W. Mu, F. Liu and Y. Hou, ACS Appl. Mater. Interfaces, 2022, 14, 41337–41347 CrossRef CAS PubMed.
- F. Flores-Céspedes, G. P. Martínez-Domínguez, M. Villafranca-Sánchez and M. Fernández-Pérez, J. Agric. Food Chem., 2015, 63, 8391–8398 CrossRef.
- F. Flores-Céspedes, I. Daza-Fernández, M. Villafranca-Sánchez, M. Fernández-Pérez, E. Morillo and T. Undabeytia, J. Hazard. Mater., 2018, 343, 227–234 CrossRef.
- W. Liang, J. Zhang, F. R. Wurm, R. Wang, J. Cheng, Z. Xie, X. Li and J. Zhao, Int. J. Biol. Macromol., 2022, 220, 472–481 CrossRef CAS PubMed.
- G. Huang, G. Qian, Y. Yan, D. Xu, C. Xu, L. Fu and B. Lin, React. Funct. Polym., 2020, 146, 104429 CrossRef CAS.
- S. Beckers, S. Peil and F. R. Wurm, ACS Sustainable Chem. Eng., 2020, 8, 18468–18475 CrossRef CAS PubMed.
- Y. Li, D. Yang, S. Lu, S. Lao and X. Qiu, J. Agric. Food Chem., 2018, 66, 3457–3464 CrossRef CAS.
- Y. Pang, Y. Sun, Y. Luo, M. Zhou, X. Qiu, C. Yi and H. Lou, Ind. Crops Prod., 2021, 167, 113468 CrossRef CAS.
- R. C. Andeme Ela, M. Tajiri, N. K. Newberry, P. A. Heiden and R. G. Ong, ACS Sustainable Chem. Eng., 2020, 8, 17299–17306 CrossRef CAS.
- X. Wang and J. Zhao, J. Agric. Food Chem., 2013, 61, 3789–3796 CrossRef CAS.
- Y. Pang, X. Li, S. Wang, X. Qiu, D. Yang and H. Lou, React. Funct. Polym., 2018, 123, 115–121 CrossRef CAS.
- X. Yu, S. Chen, W. Wang, T. Deng and H. Wang, J. Cleaner Prod., 2022, 339, 130769 CrossRef CAS.
- K. Chen, S. Yuan, D. Wang, Y. Liu, F. Chen and D. Qi, Langmuir, 2021, 37, 12179–12187 CrossRef CAS PubMed.
- H. Zhang, F. Yue, S. Hu, H. Qi and F. Lu, Int. J. Biol. Macromol., 2023, 228, 178–185 CrossRef CAS PubMed.
- J. Wang, Y. Fan, H. Wang, J. Yin, W. Tan, X. Li, Y. Shen and Y. Wang, Chem. Eng. J., 2022, 430, 132920 CrossRef CAS.
- T. He, X. Xu, B. Ni, H. Lin, C. Li, W. Hu and X. Wang, Angew. Chem., Int. Ed., 2018, 57, 10148–10152 CrossRef CAS.
- X. Yu, J. Wang, X. Li, S. Ma, W. Zhu and H. Wang, Mater. Adv., 2023, 4, 1089–1100 RSC.
- X. Yu, X. Li, S. Ma, Y. Wang, W. Zhu and H. Wang, Adv. Funct. Mater., 2023, 33, 2214911 CrossRef CAS.
- B. D. Mattos, B. L. Tardy, W. L. E. Magalhães and O. J. Rojas, J. Controlled Release, 2017, 262, 139–150 CrossRef CAS PubMed.
- P. Fernandez, V. André, J. Rieger and A. Kühnle, Colloids Surf., A, 2004, 251, 53–58 CrossRef CAS.
- J. Wu and G.-H. Ma, Small, 2016, 12, 4633–4648 CrossRef CAS PubMed.
- M. Stanisz, Ł. Klapiszewski, M. N. Collins and T. Jesionowski, Mater. Today Chem., 2022, 26, 101198 CrossRef CAS.
- O. E. Mendez, C. E. Astete, R. Cueto, B. Eitzer, E. A. Hanna, F. Salinas, C. Tamez, Y. Wang, J. C. White and C. M. Sabliov, J. Agric. Food Res., 2022, 7, 100259 CAS.
- T. Lemke, U. Stingl, M. Egert, M. W. Friedrich and A. Brune, Appl. Environ. Microbiol., 2003, 69, 6650–6658 CrossRef CAS PubMed.
- C. T. J. Ferguson, A. A. Al-Khalaf, R. E. Isaac and O. J. Cayre, PLoS One, 2018, 13, e0201294 CrossRef PubMed.
- D. Mendoza Cozatl, E. Butko, F. Springer, J. Torpey, E. Komives, J. Kehr and J. Schroeder, Plant J., 2008, 54, 249–259 CrossRef CAS.
- B. J. Shelp, J. Exp. Bot., 1987, 38, 1619–1636 CrossRef CAS.
- C.-Y. Huang, K. Araujo, J. N. Sánchez, G. Kund, J. Trumble, C. Roper, K. E. Godfrey and H. Jin, Proc. Natl. Acad. Sci. U. S. A., 2021, 118, e2019628118 CrossRef CAS.
- D. Prusky, J. L. McEvoy, R. Saftner, W. S. Conway and R. Jones, Phytopathology, 2004, 94, 44–51 CrossRef.
- I. Lager, O. Andréasson, T. L. Dunbar, E. Andreasson, M. A. Escobar and A. G. Rasmusson, Plant, Cell Environ., 2010, 33, 1513–1528 CAS.
- L. Zongo, H. Lange and C. Crestini, ACS Omega, 2019, 4, 6979–6993 CrossRef CAS PubMed.
- M. Sgarzi, M. Gigli, C. Giuriato and C. Crestini, Materials, 2022, 15, 1857 CrossRef CAS PubMed.
- D. Mo, X. Li, Y. Chen, Y. Jiang, C. Gan, Y. Zhang, W. Li, Y. Huang and J. Cui, Sci. Rep., 2021, 11, 23248 CrossRef CAS.
- J. Cui, D. Mo, Y. Jiang, C. Gan, W. Li, A. Wu, X. Li, J. Xiao, Q. Hu, H. Yuan, R. Lu and Y. Huang, Ind. Eng. Chem. Res., 2019, 58, 19741–19751 CrossRef CAS.
- G. Huang, Y. Deng, Y. Zhang, P. Feng, C. Xu, L. Fu and B. Lin, Chem. Eng. J., 2021, 403, 126342 CrossRef CAS.
- D. Yiamsawas, W. Kangwansupamonkon and S. Kiatkamjornwong, Eur. Polym. J., 2021, 145, 110241 CrossRef CAS.
- N. Chen, L. A. Dempere and Z. Tong, ACS Sustainable Chem. Eng., 2016, 4, 5204–5211 CrossRef CAS.
-
G. Tokuda, in Advances in Insect Physiology, ed. R. Jurenka, Academic Press, Okinawa, 2019, vol. 57, pp. 97–136 Search PubMed.
- M. Hafeez, X. W. Li, J. M. Zhang, Z. J. Zhang, J. Huang, L. K. Wang, M. M. Khan, S. K. Shah, G. M. Fernandez-Grandon and Y. B. Lu, Insect Sci., 2021, 28, 611–626 CrossRef CAS.
- A. Filipović, M. Mrdaković, L. Ilijin, A. Grčić, D. Matić, D. Todorović, M. Vlahović and V. Perić-Mataruga, Comp. Biochem. Physiol., Part C: Toxicol. Pharmacol., 2021, 249, 109123 Search PubMed.
- C. P. Kubicek, T. L. Starr and N. L. Glass, Annu. Rev. Phytopathol., 2014, 52, 427–451 CrossRef PubMed.
- K. Hématy, C. Cherk and S. Somerville, Curr. Opin. Plant Biol., 2009, 12, 406–413 CrossRef.
- R. A. Monteiro, M. C. Camara, J. L. de Oliveira, E. V. R. Campos, L. B. Carvalho, P. L. de Freitas Proença, M. Guilger-Casagrande, R. Lima, J. do Nascimento, K. C. Gonçalves, R. A. Polanczyk and L. F. Fraceto, J. Hazard. Mater., 2021, 417, 126004 CrossRef CAS PubMed.
- A. E. Kaziem, Y. Gao, Y. Zhang, X. Qin, Y. Xiao, Y. Zhang, H. You, J. Li and S. He, J. Hazard. Mater., 2018, 359, 213–221 CrossRef CAS.
- C. Weng, X. Peng and Y. Han, Biotechnol. Biofuels, 2021, 14, 84 CrossRef CAS PubMed.
- G. Janusz, A. Pawlik, U. Świderska-Burek, J. Polak, J. Sulej, A. Jarosz-Wilkołazka and A. Paszczyński, Int. J. Mol. Sci., 2020, 21, 966 CrossRef CAS.
- G. Janusz, A. Pawlik, J. Sulej, U. Świderska-Burek, A. Jarosz-Wilkołazka and A. Paszczyński, FEMS Microbiol. Rev., 2017, 41, 941–962 CrossRef CAS.
- Z. Liu, H. Wang and C. Xue, J. Integr. Agric., 2018, 17, 2310–2319 CrossRef CAS.
- Z. Lu, J. Deng, H. Wang, X. Zhao, Z. Luo, C. Yu and Y. Zhang, Environ. Microbiol., 2021, 23, 1256–1274 CrossRef CAS.
- R. Walther, J. Rautio and A. N. Zelikin, Adv. Drug Delivery Rev., 2017, 118, 65–77 CrossRef CAS PubMed.
- J. Fischer, S. J. Beckers, D. Yiamsawas, E. Thines, K. Landfester and F. R. Wurm, Adv. Sci., 2019, 6, 1802315 CrossRef.
- T. O. Machado, S. J. Beckers, J. Fischer, B. Müller, C. Sayer, P. H. H. de Araújo, K. Landfester and F. R. Wurm, Biomacromolecules, 2020, 21, 2755–2763 CrossRef CAS.
- S. Peil, S. J. Beckers, J. Fischer and F. R. Wurm, Mater. Today Bio, 2020, 7, 100061 CrossRef CAS.
- D. Zhang, R. Wang, H. Cao, J. Luo, T. Jing, B. Li, W. Mu, F. Liu and Y. Hou, Colloids Surf., B, 2022, 209, 112166 CrossRef CAS.
- D. Zhang, J. Du, R. Wang, J. Luo, T. Jing, B. Li, W. Mu, F. Liu and Y. Hou, Adv. Funct. Mater., 2021, 31, 2102027 CrossRef CAS.
- Y. Jiang, Y. Chen, D. Tian, F. Shen, X. Wan, L. Xu, Y. Chen, H. Zhang, J. Hu and F. Shen, Soft Matter, 2020, 16, 9083–9093 RSC.
- V. Nichols, N. Verhulst, R. Cox and B. Govaerts, Field Crops Res., 2015, 183, 56–68 CrossRef.
- L. M. Jones, A. K. Koehler, M. Trnka, J. Balek, A. J. Challinor, H. J. Atkinson and P. E. Urwin, Global Change Biol., 2017, 23, 4497–4507 CrossRef PubMed.
- J. Qiu, J. Xie, Y. Chen, Z. Shen, H. Shi, N. I. Naqvi, Q. Qian, Y. Liang and Y. Kou, Mol. Plant, 2022, 15, 723–739 CrossRef CAS.
- A. C. Velásquez, C. D. M. Castroverde and S. Y. He, Curr. Biol., 2018, 28, R619–R634 CrossRef.
- E. C. Oerke, P. Fröhling and U. Steiner, Precis. Agric., 2011, 12, 699–715 CrossRef.
- R. N. Singh, P. Krishnan, V. K. Singh and K. Banerjee, Ecol. Inform., 2022, 71, 101774 CrossRef.
- J. Zhang, Y. Huang, R. Pu, P. Gonzalez-Moreno, L. Yuan, K. Wu and W. Huang, Comput. Electron. Agric., 2019, 165, 104943 CrossRef.
- V. Singh, N. Sharma and S. Singh, Artif. Intell. Agric., 2020, 4, 229–242 Search PubMed.
- S. Zhang, T. Liu, C. Hao, L. Wang, J. Han, H. Liu and J. Zhang, Green
Chem., 2018, 20, 2995–3000 RSC.
- R. Liu, T. Ding, P. Deng, X. Yan, F. Xiong, J. Chen and Z. Wu, Int. J. Biol. Macromol., 2022, 194, 358–365 CrossRef CAS.
- Q. Zhang and R. Hoogenboom, Chem. Commun., 2015, 51, 70–73 RSC.
- Y. A. Lin, Y. X. Pang, Z. P. Li, M. S. Zhou, H. M. Lou and X. Q. Qiu, ACS Sustainable Chem. Eng., 2021, 9, 15634–15640 CrossRef CAS.
- Y. Qian, Y. H. Deng and X. Q. Qiu, Adv. Mater. Res., 2012, 550–553, 1321–1326 CAS.
- F. Shen, S. Wu, M. Huang, L. Zhao, J. He, Y. Zhang, S. Deng, J. Hu, D. Tian and F. Shen, Green Chem., 2022, 24, 5242–5254 RSC.
- P. Jiang, Y. Cheng, S. Yu, J. Lu and H. Wang, Polymers, 2018, 10, 1109 CrossRef.
- P. Jiang, X. R. Sheng, S. Yu, H. M. Li, J. Lu, J. H. Zhou and H. S. Wang, Sci. Rep., 2018, 8, 14450 CrossRef PubMed.
- A. Halperin, M. Kroger and F. M. Winnik, Angew. Chem., Int. Ed., 2015, 54, 15342–15367 CrossRef CAS PubMed.
- A. S. Wadajkar, B. Koppolu, M. Rahimi and K. T. Nguyen, J. Nanopart. Res., 2009, 11, 1375–1382 CrossRef CAS.
- X. Xu, B. Bai, H. Wang and Y. Suo, ACS Appl. Mater. Interfaces, 2017, 9, 6424–6432 CrossRef CAS.
- C. Chen, G. Zhang, Z. Dai, Y. Xiang, B. Liu, P. Bian, K. Zheng, Z. Wu and D. Cai, Chem. Eng. J., 2018, 349, 101–110 CrossRef CAS.
- X. Jin, X. Li, X. Liu, L. Du, L. Su, Y. Ma and S. Ren, Int. J. Biol. Macromol., 2023, 228, 528–536 CrossRef CAS.
- J. Li, W. Liu, X. Qiu, X. Zhao, Z. Chen, M. Yan, Z. Fang, Z. Li, Z. Tu and J. Huang, Green Chem., 2022, 24, 823–836 RSC.
- Y. Deng, Y. Liu, Y. Qian, W. Zhang and X. Qiu, ACS Sustainable Chem. Eng., 2015, 3, 1111–1116 CrossRef CAS.
- H.-Y. Tse, S.-C. Cheng, C. S. Yeung, C.-Y. Lau, W.-H. Wong, C. Dong and S.-Y. Leu, Green Chem., 2019, 21, 1319–1329 RSC.
- H.-Y. Tse, C. S. Yeung, C. Y. Lau, M. Y. Cheung, J. Guan, M. K. Islam, P. T. Anastas and S.-Y. Leu, Green Chem., 2022, 24, 2904–2918 RSC.
- M. A. Farooq, A. K. Niazi, J. Akhtar, Saifullah, M. Farooq, Z. Souri, N. Karimi and Z. Rengel, Plant Physiol. Biochem., 2019, 141, 353–369 CrossRef CAS.
- J. Dong, W. Chen, J. Feng, X. Liu, Y. Xu, C. Wang, W. Yang and X. Du, ACS Appl.
Mater. Interfaces, 2021, 13, 19507–19520 CrossRef CAS PubMed.
- G. Noctor, A. Mhamdi, S. Chaouch, Y. I. Han, J. Neukermans, B. Marquez-Garcia, G. Queval and C. H. Foyer, Plant, Cell Environ., 2012, 35, 454–484 CrossRef CAS.
- W. Liu, C. Fang, F. Chen and X. Qiu, ChemSusChem, 2020, 13, 4691–4701 CrossRef CAS PubMed.
- T. Dizhbite, G. Telysheva, V. Jurkjane and U. Viesturs, Bioresour. Technol., 2004, 95, 309–317 CrossRef CAS PubMed.
- B. Jiang, Y. Zhang, H. Zhao, T. Guo, W. Wu and Y. Jin, Int. J. Biol. Macromol., 2019, 139, 21–29 CrossRef CAS PubMed.
- A. Darabi, P. G. Jessop and M. F. Cunningham, Chem. Soc. Rev., 2016, 45, 4391–4436 RSC.
- A. Müller, M. T. Nunes, V. Maldaner, P. C. Coradi, R. S. D. Moraes, S. Martens, A. F. Leal, V. F. Pereira and C. K. Marin, Rice Sci., 2022, 29, 16–30 CrossRef.
- C. Huntingford and R. J. Oliver, Nature, 2021, 600, 224–225 CrossRef CAS PubMed.
- C. Terrer, R. B. Jackson, I. C. Prentice, T. F. Keenan, C. Kaiser, S. Vicca, J. B. Fisher, P. B. Reich, B. D. Stocker, B. A. Hungate, J. Peñuelas, I. McCallum, N. A. Soudzilovskaia, L. A. Cernusak, A. F. Talhelm, K. Van Sundert, S. Piao, P. C. D. Newton, M. J. Hovenden, D. M. Blumenthal, Y. Y. Liu, C. Müller, K. Winter, C. B. Field, W. Viechtbauer, C. J. Van Lissa, M. R. Hoosbeek, M. Watanabe, T. Koike, V. O. Leshyk, H. W. Polley and O. Franklin, Nat. Clim. Change, 2019, 9, 684–689 CrossRef CAS.
- Y. Qian, Q. Zhang, X. Qiu and S. Zhu, Green Chem., 2014, 16, 4963–4968 RSC.
- Y. Gu, Y. Pang, D. Yang, Y. Qian, H. Lou and M. Zhou, Ind. Crops Prod., 2022, 182, 114877 CrossRef CAS.
- D. Tian, J. Zhang, J. Hu, M. Huang, L. Zhao, Y. Lei, J. Zou, S. Zhang and F. Shen, Chem. Eng. J., 2023, 452, 139383 CrossRef CAS.
- T. Kacsó, E. A. Hanna, F. Salinas, C. E. Astete, E. Bodoki, R. Oprean, P. P. Price, V. P. Doyle, C. A. R. Bonser, J. A. Davis and C. M. Sabliov, Appl. Nanosci., 2022, 12, 1557–1569 CrossRef.
- N. Zhao, L. Zhu, M. Liu, L. He, H. Xu and J. Jia, J. Agric. Food Chem., 2023, 71, 3790–3799 CrossRef CAS PubMed.
- N. G. Shakhramanyan, U. A. Schneider and B. A. McCarl, Clim. Change, 2013, 117, 711–723 CrossRef.
|
This journal is © The Royal Society of Chemistry 2024 |