DOI:
10.1039/D4BM00155A
(Review Article)
Biomater. Sci., 2024,
12, 2460-2479
Multifunctional antibacterial hydrogels for chronic wound management
Received
28th January 2024
, Accepted 25th March 2024
First published on 5th April 2024
Abstract
Chronic wounds have gradually evolved into a global health challenge, comprising long-term non-healing wounds, local tissue necrosis, and even amputation in severe cases. Accordingly, chronic wounds place a considerable psychological and economic burden on patients and society. Chronic wounds have multifaceted pathogenesis involving excessive inflammation, insufficient angiogenesis, and elevated reactive oxygen species levels, with bacterial infection playing a crucial role. Hydrogels, renowned for their excellent biocompatibility, moisture retention, swelling properties, and oxygen permeability, have emerged as promising wound repair dressings. However, hydrogels with singular functions fall short of addressing the complex requirements associated with chronic wound healing. Hence, current research emphasises the development of multifunctional antibacterial hydrogels. This article reviews chronic wound characteristics and the properties and classification of antibacterial hydrogels, as well as their potential application in chronic wound management.
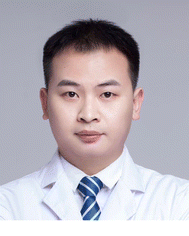 Yungang Hu | Yungang Hu is a graduate student in the Department of Burns Surgery, Beijing Jishuitan Hospital, Capital Medical University, Beijing, China. His main research interests include functional hydrogels and chronic wound healing. |
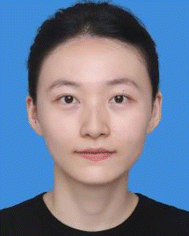 Lu Yu | Lu Yu received her Ph.D. in dermatology in 2023 from Capital Medical University, Beijing, China. Now she is a postdoctoral researcher at Beijing Jishuitan Hospital, Capital Medical University. Her current research interest focuses on wound healing. |
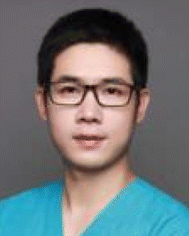 Qiang Dai | Qiang Dai, an attending physician of Beijing Jishuitan Hospital, is mainly engaged in chronic wound and scar treatment research. |
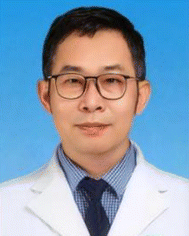 Xiaohua Hu | Xiaohua Hu, a chief physician of Beijing Jishuitan Hospital, specializes in the research of extensive burn injuries and diabetic ulcer wounds. |
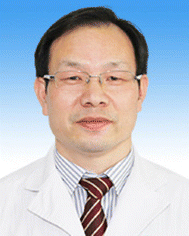 Yuming Shen | Yuming Shen is a professor at Beijing Jishuitan Hospital, Capital Medical University. His expertise and research interests are focused on the field of refractory wound repair, treatment of severe electrical burns, and development of wound biomaterials. |
Introduction
The skin, the body's first line of defence, plays a crucial role in protecting against external hazards; however, it is also susceptible to injury. Normal wounds typically heal through inflammation, cell proliferation, and tissue regeneration.1 However, those failing to heal following one month of conventional therapy while exhibiting no obvious healing tendency are defined as chronic wounds. These wounds are frequently associated with diabetes, ageing, peripheral vascular disease, or infections, leading to immune dysfunction, growth factor deficiency, bacterial biofilm formation, and prolonged inflammation, impeding normal and timely wound repair.2 Characterised by complex aetiology, persistent non-healing wounds, long treatment periods, significant incidences of amputations, notable recurrence frequencies, and elevated rates of mortality, chronic wounds impose significant psychological pressure and economic strain on patients, markedly affecting their quality of life.3 According to the World Health Organization, chronic wounds impact 2% of the global population, accounting for 4% of the total healthcare expenditure and 68% of care time.4,5 For example, there are over 550 million individuals worldwide diagnosed with diabetes, with approximately 18.6 million patients experiencing diabetic foot ulcers annually, of whom 20% require lower limb amputations. Moreover, the one-year recurrence rate following treatment for diabetic foot ulcers is approximately 42%, with a 5-year mortality rate of 65%.6 With the growing older adult population and increased incidence of chronic conditions, including obesity and diabetes, chronic wound cases are becoming more prevalent, posing a critical threat to human health.7 The most critical contributing factor is repeated infection of the local wound microenvironment, forming bacterial biofilms that impede the growth of granulation tissue and wound healing.8 Thus, effective management of wound infection and promotion of early wound healing are critical for reducing the rate of chronic wound development.
Wound dressings are typically used as temporary skin substitutes to protect wounds, stop bleeding, and prevent infection. While gauze and cotton pads are extensively used in clinical treatment, their application has numerous limitations, including poor moisture retention and adhesion to wound tissue.9 The principle of moist wound healing, first proposed by Winter in 1962,10 revolutionised wound care. As our knowledge of wound healing mechanisms expands and with the advancements in biomaterials, it has become clear that the ideal wound dressing should not only serve as an interim protective covering but also maintain a moist healing environment to promote wound repair.11 Various advanced wound dressings, including silver ion-containing dressings, foam dressings, alginate-based dressings, chitosan-based dressings, electrospun nanofiber scaffolds, and hydrogels, have been developed for clinical treatment.12
Hydrogels are hydrophilic polymers that are highly crosslinked and exhibit three-dimensional reticulations with excellent biocompatibility, degradability, and mechanical properties, making them ideal wound-dressing materials.13 Hydrogels are akin to the extracellular matrix (ECM), which provides significant advantages in wound healing.14 By maintaining a moist environment for the wound and consistently absorbing exudate, hydrogels facilitate the growth of granulation tissue and wound healing.15,16 Nonetheless, chronic wounds are refractory and can involve infection and bacterial biofilms, making it challenging to satisfy therapeutic requirements using standard hydrogel dressings with a simple structure and singular function. Wound infections are typically localised, while a few are accompanied by systemic infections. Hence, systemic antibiotics, whether administered orally or intravenously, might be less effective owing to poor wound blood supply, thus contributing to the development of antibiotic resistance.17,18 Therefore, a safe and effective delivery system for antibacterial drugs is crucial to reduce drug resistance and enhance therapeutic efficacy. The distinctive three-dimensional mesh structure of hydrogels can help slow the release of drugs and enable their localisation at the wound site, leading to sustained therapeutic outcomes.19 Indeed, various antibacterial materials have been loaded into hydrogels to construct multifunctional hydrogels capable of providing sustained pro-healing properties.20,21 This approach represents a new trend for chronic wound treatment, offering a promising direction for future research and development.
In this review, we summarise and discuss the characteristics of chronic wounds and the mechanisms and applications of multifunctional antibacterial hydrogels for chronic wound management. Furthermore, we explore the potential future advancements of these multifunctional antibacterial hydrogels. Ultimately, the goal of this article is to provide novel insights into the development of advanced wound dressings to improve outcomes in chronic wound management.
Characteristics of chronic wounds
Wound healing phases
Wound healing is a complex, highly ordered, and meticulously regulated biological process that comprises various cells, cytokines, and the ECM, to restore the structural integrity of tissues.22 Healing stages include haemostasis, inflammation, proliferation, and tissue remodelling, each seamlessly overlapping and transitioning, representing a complete, continuous, and dynamic process (Fig. 1).23 In acute wounds, skin tissue damage immediately enters the haemostatic phase, where local vasoconstriction occurs, platelets bind to collagen to undergo aggregation and adhere to damaged blood vessels, and thrombin activates the formation of a fibrin mesh structure. This structure undergoes a coagulation cascade with a coagulation factor exudate to stop the bleeding and temporarily protect the injured area.24,25 Subsequently, inflammatory cells, including macrophages and neutrophils, converge at the wound location to clear foreign bodies, damaged endogenous tissues, apoptotic cells, and bacterial proteases. Meanwhile, other immune cells are attracted to the wound site via the release of reactive oxygen species (ROS), chemokines, vasoactive factors, and other mediators to aid in tissue restoration.26 Next, to replace the lost cells/tissues, fibroblasts, keratinocytes, and vascular endothelial cells promote granulation tissue growth, vascular proliferation, collagen synthesis, wound contraction, and re-epithelialisation through migration, proliferation, and differentiation stimulated by various cytokines and growth factors.27,28 This stage is known as the proliferation phase. Although the granulation tissue of the wound is covered by epithelium, the healing process must go through the tissue remodelling phase. Through collagen degradation and rearrangement in the granulation tissue, type III collagen is progressively substituted with type I collagen, enhancing tissue strength. Overgrowth of the capillary network fades, and the small arterioles and venules in the dermis are restored to those of the normal dermis, achieving complete wound healing.29
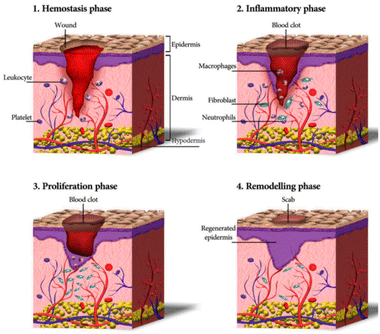 |
| Fig. 1 Schematic representation of the wound healing process.30 Copyright 2020, MDPI. | |
Pathology of chronic wounds
Chronic wounds often persist in the inflammatory stage, hindering the progression to the cell proliferation and tissue remodelling phases, leading to prolonged or non-healing wounds.31
These wounds can be categorised into clinical types based on the causative factors: ischaemic ulcers, traumatic ulcers, metabolic ulcers, pressure ulcers, venous ulcers, and others (e.g., infected ulcers, malignant ulcers, neurogenic ulcers, and radiological ulcers).32,33 Chronic wounds exhibit prolonged inflammation, elevated ROS levels, impaired angiogenesis, inadequate re-epithelialisation, imbalanced cytokine/growth factor expression, and heightened protease activity.34–36
Bacterial infection in wounds is a crucial factor contributing to the interruption of the wound healing process, generating inflammatory markers that impede granulation tissue growth and epithelialisation of the wound. Specifically, the existence of bacteria results in cell death, exacerbating the inflammatory response and prolonging the inflammatory phase.37 The excessive inflammatory response disrupts the body's immune barrier, increasing susceptibility to bacterial invasion. Macrophages, as an important component of intrinsic immunity, have a crucial role in each stage of the wound healing process.38 In chronic wounds, macrophages overproduce inflammatory cytokines and exhibit a disproportionate M1/M2 ratio, characterised by a predominance of the pro-inflammatory M1 phenotype and a deficiency of the pro-regenerative M2 phenotype.39 This imbalance leads to overexpression of pro-inflammatory factors, including interleukin-1β (IL-1β) and tumour necrosis factor-alpha (TNF-α).40 Inflammatory cells that accumulate in chronic wounds persistently produce ROS, resulting in impaired cell function, poor angiogenesis, and hindered re-epithelialisation.41 Additionally, excessive ROS and chronic inflammation upregulate the expression of matrix metalloproteinase-9 (MMP-9) through the nuclear factor-kappaB (NF-κB) pathway. This disrupts the balance between extracellular matrix deposition and remodelling and impedes epithelial closure.42 Collectively, these molecular and cellular abnormalities in the immune microenvironment severely impede ECM deposition, granulation tissue growth, and re-epithelialisation, contributing to chronic wound formation and maintenance (Fig. 2).
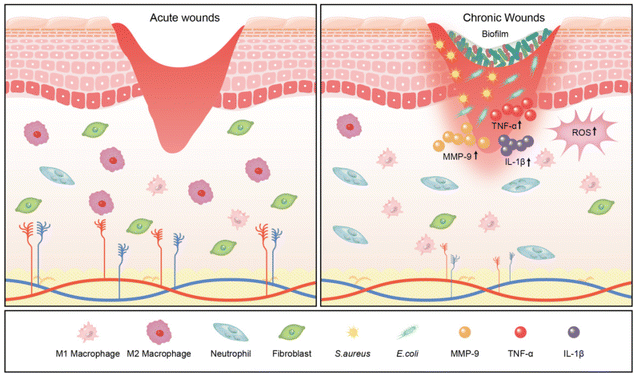 |
| Fig. 2 Schematic representation of acute and chronic wounds. | |
Biofilms in chronic wounds
Chronic wounds are often colonised by bacteria, such as Staphylococcus aureus (S. aureus), Escherichia coli (E. coli), Streptococci, Enterococcus faecalis, fungi, and Pseudomonas aeruginosa (P. aeruginosa), as well as multiple resistant bacteria, such as methicillin-resistant Staphylococcus aureus (MRSA) (Table 1).43,44 These microbes typically colonise and invade chronic wounds as biofilms that are unrecognised by the host cells. A biofilm comprises bacterial or fungal cells surrounded by extracellular polysaccharides, proteins, and extracellular DNA.45 Biofilms can increase the tolerance of intramembrane bacteria to various types of bactericides/inhibitors by limiting the diffusion of antibiotics within the membrane, upregulating the level of bacterial efflux pumps and horizontal transfer of drug-resistance genes, and reducing metabolism, leading to a state of persistent inflammation and non-healing wounds.46,47 James et al. revealed that biofilms are associated with 60% of chronic wounds contrasted with only 6% of acute wounds, making them a significant factor in chronic wound infection and resistance to conventional treatments.48
Table 1 Common bacteria that colonise chronic wounds
Group |
Pathogens |
Gram-positive |
Staphylococcus aureus, Staphylococcus epidermidis, Staphylococcus haemolyticus, Enterococcus faecalis, Streptococcus anginosus, Corynebacterium |
Gram-negative |
Pseudomonas aeruginosa, Escherichia coli, Morganella morganii, Klebsiella pneumoniae, Proteus mirabilis, Serratia marcescens, Acinetobacter baumannii |
Fungus |
Candida albicans, Candida parapsilosis, Aspergillus, Blastomycosis |
Multiple resistance |
Methicillin-resistant Staphylococcus aureus, Vancomycin-resistant enterococci, multi-drug resistant Pseudomonas aeruginosa, carbapenem-resistant Enterobacteriaceae |
Hence, to achieve healing, biofilms must be effectively removed from wounds. This typically involves surgical debridement, antibiotic administration, and regular local dressing changes.49 However, mechanical debridement can cause further damage to healthy tissues and trigger bacteria to enter the bloodstream, resulting in secondary infections.50 Emerging therapeutic strategies, including inorganic nanomaterials with antibacterial properties and carbon-based materials, such as silver nanoparticles (NPs) and graphene, have promising application prospects.51–53 In particular, hydrogels, possessing unique reticular structures, are an ideal delivery system for these materials to achieve antibacterial and pro-restorative effects.
Main functions of multifunctional antibacterial hydrogels
Hydrogels are a category of highly hydrophilic gels characterised by unique three-dimensional network structures created via physical or chemical crosslinking of hydrophilic polymers. Conventional hydrogels composed of natural or synthetic polymers exhibit substantial potential for wound-healing applications. However, they often fail to meet the complex requirements of chronic wound management.54 In contrast, multifunctional antibacterial hydrogels exhibit exceptional antibacterial and wound repair properties. As a favourable wound dressing, a multifunctional antibacterial hydrogel must satisfy several criteria: (1) high biocompatibility without inducing toxicity or inflammation; (2) effective moisturising capability to maintain a moist wound condition, facilitate cell hydration, and absorb wound exudate; (3) adequate physical and mechanical strength to maintain its integrity and prevent external bacterial invasion; (4) surface microstructures and biochemical characteristics that encourage cell adhesion, proliferation, and differentiation; (5) stable drug delivery properties for sustained action in wounds; and (6) excellent antibacterial properties and resistance to biofilm formation.55–57 The core functionalities of antibacterial hydrogels include antibacterial properties, anti-biofilm formation, and pro-repair functions (Fig. 3). This section explores these three aspects in detail.
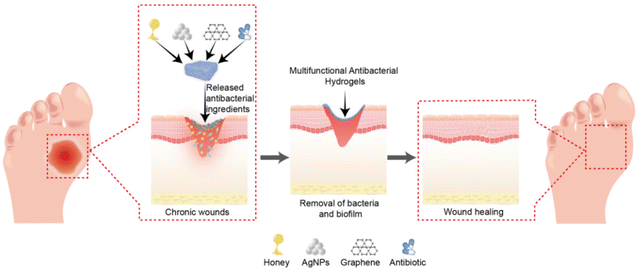 |
| Fig. 3 Schematic representation of multifunctional antibacterial hydrogels for chronic wound management. | |
Antibacterial properties
Persistent inflammation resulting from bacterial infection impedes granulation tissue growth and epithelialisation in wounds and is the predominant cause of chronic wounds.37 The primary efficacy of multifunctional hydrogels relies on good antibacterial properties, curbing the growth of harmful microorganisms in the wound and resisting wound infection. Recently, the employment of hydrogel materials to deliver antibacterial agents, including antibiotics or antibacterial NPs, has emerged as a prominent research area.58 Encapsulation of antibiotics or bacteriostatic drugs in hydrogels is a common method for treating chronic wounds. However, extended use of antibiotics may result in the emergence of drug-resistant bacterial strains or superbugs, significantly intensifying the difficulty of treating chronic wounds. As alternatives, inorganic metal NPs, such as silver, copper, and gold NPs, are extensively utilised owing to their diverse antibacterial mechanisms.59,60 Additionally, polychitosan, honey, and fullerenes have notable antibacterial properties and biocompatibility, which circumvent the misuse of antibiotics and mitigate the risk of bacterial resistance in chronic wounds.
Anti-biofilm formation
Biofilm formation is the primary cause of recurrent chronic wound infections, leading to refractory chronic infections.46 This results in reduced healing rates, extended healing durations, increased patient suffering, and increased medical expenses. Common bacteria in chronic wounds can form biofilms within 10 h.61 Although debridement procedures can effectively remove bacterial communities from wounds, biofilms begin to regrow within two days and large numbers of bacteria are present within mature biofilms three days post-debridement.62 However, detecting bacteria within biofilms is difficult with conventional microbial cultivation methods, further complicating the assessment of antibiotic resistance and biofilm formation trends.63 This poses significant challenges for clinical treatment and highlights the importance of targeting biofilm formation in chronic wound management.
Various nanomedicines and biomaterials have been exploited to treat biofilm infections.64–66 Hydrogels can achieve slow drug release and exhibit good biocompatibility and tissue adaptation, improving drug accumulation and bioavailability within biofilms. Hu et al. prepared an anti-biofilm hydrogel using aminoglycoside and norspermidine to treat P. aeruginosa biofilm infections.67 The hydrogel prevents biofilm formation through quorum-sensing regulation and by releasing norspermidine to reduce bacterial virulence after infection and aminoglycosides to eliminate planktonic bacteria. Thus, effective multifunctional antibacterial hydrogels should possess antibacterial and anti-biofilm effects.
Pro-repair function
Hydrogel matrix materials sourced from natural polymers, including gelatine, hyaluronic acid (HA), chitosan, and fibroin protein, as well as synthetic polymers, such as polyvinyl alcohol (PVA), polyacrylic acid (PAA), and polyethylene glycol (PEG), significantly influence cell behaviour and wound healing processes. These synthetic polymers can be chemically or physically crosslinked to construct different types of hydrogels that promote wound repair.68 Hydrogels are biocompatible, and their dense mesh structure prevents infection by foreign bacteria. Hydrophilic hydrogels help to sustain a moist environment in the wound area, conducive to the healing process.20 The moist environment can reduce the dehydration of tissue cells, promote the proliferation of fibroblasts and migration of keratin-forming cells, maintain an appropriate microenvironment for wound healing, and slow the progressive deepening of the wound.11,69 Therefore, achieving physical isolation and moisturisation are the most basic and important functions of hydrogels as wound dressings.
Hydrogels are similar to the ECM in structure and composition and can induce cell adhesion, migration, and ECM deposition. They provide temporary scaffolds for fibroblast proliferation, re-epithelialisation, pro-vascularisation, and chronic wound remodelling, thus promoting wound repair.70,71 In addition, owing to their efficient delivery properties, hydrogels can be loaded with different active molecules, including drugs, cytokines, growth factors, and exogenous cells, such as stem cells and fibroblasts, to construct a delivery system suitable for different chronic wounds, promoting wound repair.72,73
Classification and mechanism of multifunctional antibacterial hydrogels
Universally, antibacterial properties arise from antibacterial hydrogel matrices and the release of antibacterial substances from composite hydrogels. Antibacterial hydrogels are classified into six categories based on their antibacterial mode: (1) hydrogels with inherent antibacterial activity, (2) biological extract-loaded hydrogels, (3) antibiotic-loaded hydrogels, (4) inorganic NP-loaded hydrogels, (5) carbon material-loaded hydrogels, and (6) stimulus-responsive hydrogels (Fig. 4).
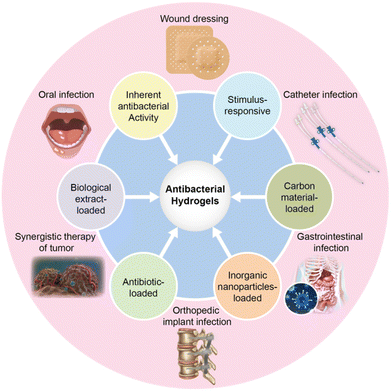 |
| Fig. 4 Classification and applications of antibacterial hydrogels. | |
Hydrogels with inherent antibacterial activity
Hydrogels with inherent antibacterial activity have become a significant area of clinical research and refer to those with antibacterial hydrogel matrix components. The antibacterial effect of these hydrogels primarily originates from three sources: antibacterial polymers, antibacterial peptides, and amphoteric ion hydrogels.74
Antibacterial polymer hydrogels.
Natural and synthetic antibacterial polymers, including chitosan, cellulose, PEG, PVA, and PAA, elicit their activity via distinctive functional groups.75 These materials are notable for their solubility, biocompatibility, simplicity in modification, and sustained antibacterial properties.76 Chitosan, a natural antibacterial cationic polymer derived from chitin through enzymatic or chemical deacetylation, has excellent antibacterial and biochemical properties, including biocompatibility, biodegradability, nontoxicity, and processability.77 It is particularly effective against S. aureus and E. coli. Mechanistically, when the pH falls below 6, chitosan becomes positively charged, enabling interaction with negatively charged regions on microbial membranes. This interaction disrupts nutrient transport into cells and damages bacterial membranes.78 Chitosan modification enhances the water solubility and antibacterial efficacy under neutral conditions. Carboxymethyl chitosan, a derivative obtained by carboxymethylation, demonstrates improved solubility, facilitating the creation of antibacterial hydrogels. In a mouse wound model, carboxymethyl chitosan hydrogels effectively promote wound repair and skin regeneration.79 Hao et al. incorporated the basic fibroblast growth factor (bFGF) into a carboxymethyl chitosan hydrogel for diabetic wound treatment.80 This accelerated the full-thickness repair of diabetic wounds, promoted epithelialisation and collagen production, and promoted angiogenesis and hair follicle formation by upregulating CD31 and CD34 expression. Meanwhile, cellulose exhibits enhanced antibacterial efficacy when combined with chitosan. A study on a bacterial cellulose–chitosan composite hydrogel revealed its significant anti-biofilm potential, reducing active biofilm by 90% and biofilm height by 65%, indicating its potential in treating chronic wounds.81
Although natural polymers boast several advantages, including biocompatibility, degradability, affordability, and abundant availability, they often present challenges in modification for specific wound treatments. Synthetic polymers can overcome this issue.82 For instance, Peng et al. utilised amine-modified PEG loaded with Cu5.4O ultra-small nanozymes to adsorb chemokines, remove ROS, and promote diabetic wound healing.83 PVA is particularly noteworthy for forming dense hydrogen bonds with other polymers, helping delay ROS release, thus, enabling prolonged bacterial elimination and wound repair. Moreover, a double-network hydrogel comprising PVA and PAA with tannic acid exhibits significant antibacterial properties against E. coli and S. aureus.84 To enhance antibacterial properties and promote wound healing, synthetic polymers are often used in conjunction with other materials or drugs, such as curcumin, silver NPs, and exosomes. Zhang et al. prepared multifunctional hydrogels with metformin-loaded exosomes using Ag+-crosslinked 4-arm PEG as a matrix for diabetic wound treatment.85 The composite hydrogel alleviated inflammation and vascular injury around the wound and promoted wound healing by triggering cell proliferation and angiogenesis.
Antimicrobial peptide hydrogels.
Antimicrobial peptides (AMPs) are present in animal and plant tissues and cells. They exhibit broad-spectrum antibacterial properties without inducing bacterial resistance and promote wound healing.86 Hence, AMPs have garnered increased attention as potential substitutes for antibiotics and novel treatment approaches. AMPs elicit their antimicrobial effects via two primary mechanisms. First, the positive charge of AMPs leads to electrostatic interactions with negatively charged cell membrane phospholipids, disrupting and destroying the cell membrane structure and ultimately causing cell death. Second, AMPs enter the cytoplasm and interact with intracellular components, leading to inhibition of DNA, RNA, and protein synthesis, hindrance of protein folding processes, obstruction of enzyme activity and cell wall formation, and induction of lyase release, which damages cell structures (Fig. 5).87 AMPs can effectively resist invasion by Gram-positive and Gram-negative bacteria, viruses, fungi, and other pathogens. However, their use is limited by their sensitivity to the wound microenvironment, including alkaline pH and protein or hydrolases, which can lead to hydrolysis, oxidation, or photolysis. Additionally, they exhibit high cytotoxicity and haemolytic activity toward mammalian cells, hindering their local application.88 To overcome these limitations and maximise the antibacterial and wound repair effects of AMPs, it is necessary to develop local preparations with sustained stability and release efficiency. These preparations would harness the full potential of AMPs in medical applications, particularly in wound management.
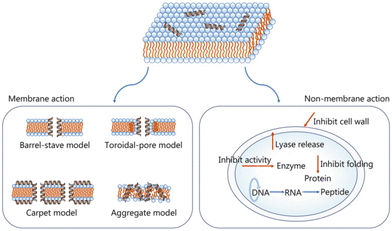 |
| Fig. 5 AMP antibacterial mechanisms.87 Copyright 2021, BMC. | |
The three-dimensional structure of hydrogels can achieve a local stable release of AMPs through various mechanisms (e.g., electrostatic interactions, covalent binding, and degradation).89 The hydrogel's high water content preserves AMP activity, and the polymerised structure of the hydrogel minimises AMP degradation by proteases. Suo et al. prepared an AMP–HA composite hydrogel with a crosslinking agent via a Schiff base reaction.90 The AMP–HA composite demonstrated remarkable antibacterial activity against S. aureus and E. coli. Moreover, in a mouse infectious wound model, it reduced the inflammatory response, promoted fibroblast proliferation and angiogenesis, and accelerated wound healing. Antibacterial peptide hydrogels’ regenerative and reparative effects can also be enhanced by loading active ingredients. A hydrogel comprising oxidised dextran (ODEX), antibacterial peptide-modified hyaluronic acid (HA–AMP), and platelet-rich plasma (PRP) exhibits significant antibacterial activity against E. coli, S. aureus, and P. aeruginosa. In a diabetic mouse infectious wound model, this hydrogel enhanced wound healing by modulating inflammation, collagen deposition, and angiogenesis.91
Zwitterionic hydrogels.
Zwitterionic hydrogels exhibit antibacterial mechanisms similar to those of AMPs. Their electrostatic interactions enable their attachment to bacterial cell membranes that possess a negative charge. This binding disrupts the bacterial membrane structure, alters the osmotic balance, causes intracellular matrix outflow, and ultimately leads to bacterial death.57 Zwitterionic polymers primarily include polybetaine, amino acid derivatives, and mixed charge polyampholytes. Compared to the traditional antibacterial material PEG, zwitterionic materials have a series of unique properties, including resistance to protein adsorption, bacterial adhesion, and biofilm formation, reduced immune rejection, and enhanced protein stability.92 Consequently, zwitterionic materials have gained significant attention in biomedical applications. Qiu et al. developed zwitterionic dextran-based hydrogels utilising carboxybetaine glucan (CB-Dex) and sulfoxide betaine glucan (SB-Dex).93 This hydrogel demonstrated antibacterial adhesion to S. aureus and E. coli and promoted skin wound healing in mice. Other antibacterial components can also be added to zwitterionic hydrogels to exert a synergistic antibacterial effect. For example, tannic acid (TA), which has antioxidant and antibacterial properties, was incorporated into a zwitterionic polysulfone betaine (polySBMA) hydrogel, demonstrating an efficient free radical scavenging ability and excellent antibacterial capacity against Gram-positive and Gram-negative bacteria. In a diabetic mouse wound model, the TA–polySBMA hydrogel notably expedited wound repair.94 Hence, this simple and effective preparation method is promising for diabetic wound management.
Some hydrogel matrices exhibit limited antibacterial properties, thereby restricting their applications. To enhance their inherent antibacterial effects and achieve optimal antibacterial properties, hydrogel matrices can be modified with antibacterial groups. Currently, commonly employed antibacterial groups include quaternary ammonium salts, quaternary phosphine salts, and thiazole salts.95 Orlando et al. introduced glycidyl ester trimethyl ammonium chloride modification into bacterial cellulose to improve its antimicrobial activity.96 Following this modification, the hydrogel exhibited an inhibition rate of 53% against S. aureus and 43% against E. coli, while not affecting keratinocyte growth. Moreover, it promoted wound healing effectively owing to its excellent antibacterial properties and biocompatibility. Enhancement of the antibacterial properties of hydrogels can also be achieved through the incorporation of small molecule compounds or polymer compounds with antibacterial attributes. Shen et al. successfully modified PVA by incorporating polyaspartic acid (PASP) derivatives containing quaternary ammonium and boric acid groups, which exhibit both antibacterial and luminescence properties, thereby promoting wound healing.97 Gallic acid, TA, catechol derivatives, and other compounds are frequently employed for hydrogel modification to fabricate multifunctional antibacterial hydrogels with antimicrobial, antioxidant, and wound healing properties, owing to their inherent antibacterial, anti-inflammatory, and antioxidant characteristics. Gong et al. synthesised gallic acid-modified water-soluble polysaccharide agarose that exhibits remarkable antibacterial activity and biocompatibility while significantly mitigating inflammation and expediting wound healing.98
Biological extract-loaded hydrogels
Biological extracts encompass plant and animal extracts that possess anti-inflammatory and anti-infective properties owing to the presence of alkaloids, flavonoids, terpenoids, tannins, polyphenols, and other components. Common sources include honey, green tea, turmeric, and seaweed.99 These extracts are characterised by low toxicity and excellent bactericidal properties. The broad-spectrum bactericidal properties of honey aid in preventing wound infections and promoting epithelial cell proliferation while reducing oedema around wounds.100 Incorporating honey into chitosan hydrogels significantly boosts their antibacterial effects against S. aureus, Bacillus cereus, E. coli, P. aeruginosa, and Candida albicans. In a mouse model with full-thickness skin defects, a honey–chitosan hydrogel accelerated wound healing and contraction.101 Additionally, Park et al. developed a chestnut honey-infused sodium carboxymethylcellulose hydrogel that effectively inhibited the growth of S. aureus and E. coli, promoting wound healing in diabetic mice.102 However, many types of honey are available, and their antibacterial properties are impacted by nectar type, flowering period, and geographical area.
Curcumin, a polyphenolic compound extracted from turmeric rhizome, is recognised for its anti-infection, anti-inflammatory, and antioxidant properties, making it a potential wound-healing agent.103 Curcumin facilitates wound healing by enhancing granulation tissue growth, tissue remodelling, and collagen deposition. However, its clinical application is hindered by its unstable structure, poor solubility, and rapid elimination.104 To address these limitations, Le et al. prepared hydrogels containing curcumin nanoemulsions that enhanced the antibacterial properties of curcumin in vitro and wound healing effects in vivo.105 This hydrogel enabled stable curcumin release and sustained its antibacterial properties. Combining curcumin NPs with hydrogels improves the stability, solubility, and bioavailability while prolonging the action time. Curcumin NPs encapsulated in hydrogels enhance the wound healing process in diabetic rats, leading to complete re-epithelialisation, full dermal–epidermal connection, dermal recombination, a significant increase in collagen deposition, and elevated vascular endothelial growth factor (VEGF) expression.106 Green tea, chrysanthemum, hesperidin, Periplaneta americana extract, and plant essential oils have similar anti-inflammatory, antioxidant, and antibacterial effects.99 Nevertheless, their application for chronic wounds is restricted by poor water solubility, low bioavailability, and short action time. Currently, combining extracts and hydrogel scaffolds is a promising antibacterial strategy. However, the complex nature of biological extracts necessitates further optimisation and purification to identify key components for enhanced efficacy.
Antibiotic-loaded hydrogels
Antibiotic resistance remains a significant challenge in the development and application of antibiotics; however, they remain the most prevalent and efficient choice for the clinical management of bacterial infections. A critical clinical issue is reducing systemic antibiotic use to minimise bacterial resistance while ensuring that local antibiotic treatments achieve effective bactericidal doses.107 The three-dimensional porous network structure of hydrogels makes them ideal antibiotic delivery systems, facilitating the local release of drugs and decreasing bacterial resistance. Localised drug delivery ensures sufficient long-term bactericidal doses.108 Hence, this represents a strategic solution to achieve effective bacterial infection treatment and reduce the risk of antibiotic resistance development. The primary bactericidal mechanisms of traditional antibiotics include the hindrance of bacterial cell wall synthesis, disturbance of protein synthesis pathways, and suppression of nucleic acid synthesis (Fig. 6).109,110 Commonly loaded antibiotics onto hydrogels include imipenem, gentamicin, ciprofloxacin, vancomycin, chloramphenicol, lincomycin, and minocycline (Table 2).
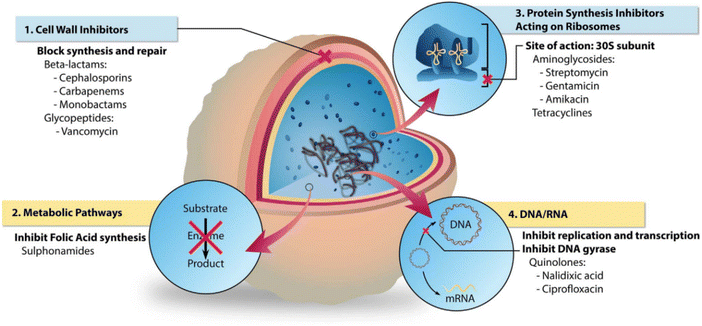 |
| Fig. 6 The different bacterial targets of antibiotic agents.110 Copyright 2018, Elsevier. | |
Table 2 Application of antibiotic-loaded hydrogels for chronic wound management
Type of Antibiotic |
Mechanism |
Hydrogel matrix |
Antibacterial capability |
Type of wound |
Wound healing effect |
Ref. |
Imipenem |
Inhibits the synthesis of cell wall mucins; impedes cell wall formation |
PLGA–PEG–PLGA |
Completely inhibits MDR-PA growth |
Infected mouse wound model |
Reduces inflammation; regulates macrophages; promotes wound healing |
112
|
Gentamicin |
Disrupts protein synthesis; compromises bacterial cell membrane integrity |
Sodium alginate, glyceryl methacrylate gelatine |
Good antibacterial performance against E. coli and S. aureus |
Diabetic wound mouse model |
Reduces inflammation; accelerates re-epithelialisation and granulation tissue formation |
116
|
Ciprofloxacin |
Hinders DNA replication; inflicts irreversible DNA damage |
Glycol chitosan |
Antibacterial activity against S. aureus (61%) |
Infected mouse wound model |
Reduces bacterial load in the infected sites; effectively promotes wound healing |
118
|
Vancomycin |
Inhibits the production of cell wall phospholipids and peptides |
Quaternary ammonium salt chitosan |
Antibacterial rate of 99.953 ± 0.080% against S. aureus and 98.676 ± 0.654% against E. coli |
Infected rat wound model |
Facilitates blood vessel regeneration at the wound site; promotes collagen deposition; expedites wound healing |
121
|
Chloramphenicol |
Inhibits peptide acyltransferase; inhibits protein synthesis |
κ-Carrageenan |
Effective antibacterial properties against E. coli and S. aureus |
— |
Requires further animal experimental observation |
122
|
Lincomycin |
Similar mechanism to chloramphenicol |
PVA, chitosan |
Notable antibacterial activity against E. coli and S. aureus |
— |
Requires further animal experimental observation |
123
|
Minocycline |
Similar mechanism to gentamicin |
Alginate |
Strong anti-S. aureus activity |
Infected rat wound model |
Promotes fibroblast proliferation and angiogenesis; accelerates wound healing |
124
|
Imipenem, a broad-spectrum β-lactam antibiotic with potent antibacterial properties, is particularly effective against infections caused by various pathogens, including aerobic and anaerobic bacteria.111 Pan et al. developed a thermosensitive hydrogel loaded with imipenem-encapsulated liposomes containing a gold shell and a lipopolysaccharide-targeting aptamer (ILGA).112 ILGA@Gel exerted a strong bactericidal effect against multidrug-resistant P. aeruginosa, reduced inflammation by regulating macrophages, and promoted the healing of infectious wounds, demonstrating its significant potential in managing complex, infected wounds.
Gentamicin exhibits efficacy against a broad spectrum of bacteria and is frequently employed in the treatment of skin, soft tissue, and wound infections. However, its clinical application is restricted owing to nephrotoxicity, ototoxicity, and low plasma concentrations.113,114 To address these limitations, local application via drug-loaded hydrogels has emerged as an effective solution. Mohd Razali et al. prepared a composite scaffold with a structure resembling a sandwich, comprising a hydrogel layer positioned between two layers of aligned nanofibers. Gentamicin, preloaded into a hydrogel, was gradually released during the healing process.115 In a rat full-thickness skin defect model, this scaffold achieved a 97.49% wound healing rate, characterised by low inflammation, rapid re-epithelialisation, and angiogenesis, leading to accelerated wound healing. Treatment of diabetic wounds, often complicated by bacterial infection and persistent inflammation, benefits from innovative treatments such as dual-network multifunctional hydrogels. One such hydrogel, comprising gentamicin sulphate, sodium alginate oxide (OSA), and glyceryl methacrylate gelatine (GelGMA), shows promise for diabetic wound care. Bacteriostatic experiments demonstrated that gentamicin effectively inhibits S. aureus and E. coli. In skin wound models of diabetes, the GelGMA-OSA@GS hydrogel markedly decreased inflammation and expedited re-epithelialisation and granulation tissue growth, enhancing wound healing.116
Ciprofloxacin is a fluoroquinolone antibacterial agent that effectively targets Gram-positive and Gram-negative bacteria.117 When incorporated into a chitosan hydrogel, ciprofloxacin enhances skin retention, wound healing, and anti-biofilm properties. This hydrogel exhibited 72% anti-biofilm activity against Enterobacter aerogenes and 63% against P. aeruginosa. In vitro scratch healing experiments in the treated group revealed significant increases in wound healing rates at 24 h (28.9%) and 72 h (57.7%), compared to controls. An in vivo study demonstrated that the retention rate of the ciprofloxacin-loaded hydrogel was 3.65 times that of free ciprofloxacin after 5 h. Therefore, the loaded ciprofloxacin hydrogel not only improves the drug retention rate to achieve local sustained release but also promotes wound healing.118 This localised treatment method addresses the challenges associated with high drug dosages, toxicity, and drug resistance while providing enhanced efficacy directly at the infection site.
Vancomycin, a glycopeptide antibiotic, is essential for treating serious infections induced by MRSA, methicillin-resistant coagulase-negative staphylococci, and enterococci. These infections include conditions such as sepsis, pulmonary infections, and skin and soft tissue infections.119 In the management of chronic wounds, utilising hydrogels as drug delivery systems enhances the protection and efficacy of vancomycin. This approach ensures targeted antibacterial action and promotes healing by leveraging the hydrogel properties for sustained and localised drug release. Upon incorporating vancomycin hydrochloride, a hydrogel exhibited sustained release and robust antibacterial activity against MRSA. It also demonstrated notable therapeutic effects in a full-thickness rat burn wound model, significantly enhancing collagen deposition, stimulating granulation tissue formation, and accelerating wound closure. Additionally, the hydrogel promoted neovascularisation and demonstrated anti-inflammatory properties.120 Huang et al. prepared a multifunctional composite hydrogel integrating curcumin and vancomycin coated with a quaternary ammonium salt chitosan (QCS) organic framework (QCSMOF-Van).121 This composition effectively inhibited bacterial metabolism, enabling efficient bacterial capture and rapid eradication. The QCSMOF-Van hydrogel also precisely regulated the balance between M1 and M2 macrophage phenotypes, fostering nerve and blood vessel regeneration. This mechanism significantly accelerates chronic wound healing, highlighting the potential of antibacterial hydrogels in advanced wound care.
A chloramphenicol agar/κ-carrageenan/montmorillonite hydrogel exhibited effective antibacterial activity against E. coli and S. aureus, with significant potential in wound care applications.122 Qing et al. prepared a PVA/n-succinyl chitosan (NSCS)/lincomycin wound-dressing hydrogel using a freezing/thawing method.123 This hydrogel, incorporating lincomycin, exhibited notable antibacterial properties against E. coli and S. aureus, with a 77.71% inhibition rate against S. aureus at 75 μg mL−1. However, further animal experiments are required to verify the associated effects and wound repair mechanisms.
Minocycline, an effective tetracycline against MRSA strains, has been used in a minocycline-loaded alginate injectable hydrogel (SA@MC). This formulation ensures sustained release, high biocompatibility, and strong anti-S. aureus activity. In vivo, SA–MC hydrogels effectively promote wound healing in S. aureus-infected wounds.124
Although hydrogel drug loading can achieve local sustained drug release for large wounds, particularly for burns, excessive use of antibiotic-containing hydrogels carries the risk of bacterial resistance. Therefore, future research should focus on minimising antibiotic dosages or even avoiding antibiotic use to prevent the emergence of bacterial resistance.
Inorganic nanoparticle-loaded hydrogels
Inorganic NPs have garnered significant interest owing to their potent bactericidal capabilities, low cytotoxicity, and broad-spectrum antibacterial performance.125 Integration of nanomaterials into drug delivery systems has opened new avenues for controlling microbial infections and enhancing wound healing. Metal and metal oxide NPs, including silver (Ag), gold (Au), copper (Cu), zinc oxide (ZnO), and titanium dioxide (TiO2), possess good antibacterial properties and drug resistance, making them pivotal constituents in the fabrication of antibacterial hydrogels based on inorganic materials.126 Hydrogels loaded with inorganic antibacterial materials not only enhance antibacterial efficiency but also sustain this activity over extended periods. This helps reduce the likelihood of bacterial resistance. Among them, silver NPs (AgNPs) are the most extensively utilised and boast effective antibacterial action, wound healing facilitation, and low toxicity, making them excellent choices for inclusion in hydrogel formulations for medical and therapeutic applications.127
Metal nanoparticle-loaded hydrogels
Silver has a long history of medicinal use, dating back to Hippocrates, who utilised silver powder for treating wound ulcers. Before the advent of antibiotics, silver compounds were the primary means for combating wound infections.128 With the advancements in nanoscience and nanotechnology, Ag is primarily utilised in the form of AgNPs, highly effective antibacterial agents.129 The exceptional antibacterial properties of AgNPs are attributed to their large specific surface area, enhanced contact with bacterial membranes, and cytoplasmic leakage, which inhibit bacterial growth.130 Additionally, AgNPs have a strong binding capacity for macromolecules, which, upon contact with bacterial membranes, result in cell disintegration and death.131 The precise antibacterial mechanism of AgNPs is not fully established; however, several theories have been proposed: (i) disruption of the bacterial membrane and release of cellular contents; (ii) generation of ROS, leading to respiratory chain disruption; (iii) destruction of the DNA structure and interference with DNA replication; and (iv) inactivation of enzymes and denaturation of proteins (Fig. 7).132 Each of these actions contributes to the potent antibacterial effects of AgNPs, making them valuable in antimicrobial treatments.
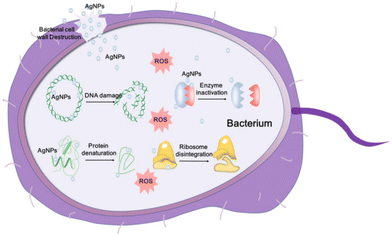 |
| Fig. 7 Schematic representation of AgNP antibacterial mechanisms.132 Copyright 2020, Ivyspring International Publisher. | |
AgNP-loaded antibacterial hydrogels exhibit excellent antibacterial, antioxidant, pro-vascularisation, and pro-epithelialisation properties in animal experiments and clinical applications. Basha et al. synthesised a fumaric acid-incorporated agar-silver hydrogel (AA–Ag–FA) using fumaric acid, AgNPs, and agar.133 This hydrogel demonstrated potent antibacterial properties against E. coli, S. aureus, and P. aeruginosa, common culprits in wound infections. AA–Ag–FA also exhibits significant antioxidant properties and effectively promotes neovascularisation, granulation tissue formation, and epithelialisation. However, the safety of AgNPs has been questioned. The nonspecific dispersion of AgNPs may produce cytotoxicities, such as dermatotoxicity, ophthalmotoxicity, respiratory toxicity, hepatobiliary toxicity, neurotoxicity, and reproduction toxicity, limiting their application.134,135 Current knowledge regarding the cytotoxicity, long-term health impacts, and specific mechanisms of AgNP accumulation in different tissues and organs remains limited. Therefore, more in-depth research is warranted to mitigate these risks, ensuring safer and more effective use of AgNP-loaded hydrogels in medical and therapeutic contexts.
The green synthesis of metal and metal oxide NPs has garnered growing attention in the biomedical field, offering enhanced efficiency over traditional physical and chemical methods in terms of antibiotic and metal ion utilisation.136,137 This approach is considered superior for NP preparation as it reduces toxicity, improves stability and provides an environmentally friendly and cost-effective solution.59 Aldakheel et al. synthesised AgNPs using a microwave irradiation technique with green tea extract as a natural reducing agent, which was incorporated into a chitosan-grafted PVA hydrogel.138 This hydrogel promoted cell proliferation and was non-cytotoxic. Enhanced wound healing was observed in rat experiments and in vitro fibroblast cultures. Additionally, it demonstrated effective antibacterial activity against E. coli and S. aureus.
Gold NPs (AuNPs), copper NPs (CuNPs), zinc NPs (ZnNPs), iron NPs (FeNPs), and gallium NPs (GaNPs) have approximately the same antibacterial, antioxidant, and pro-restorative effects, although the functionality of each NP varies depending on the inherent characteristics of the metal. We have summarised the mechanisms and efficacy of these metal NPs when incorporated into hydrogels for wound healing in Table 3. When loaded into hydrogels, these NPs enhance the hydrogel properties, including increased antibacterial efficacy, improved wound healing, and controlled drug release. The hydrogel matrix provides a supportive structure for the NPs, allowing for sustained release and targeted delivery to the wound site. This synergy between the NPs and hydrogel matrix is crucial for optimising wound care and enhancing the healing process.
Table 3 Application of metal nanoparticle-loaded antibacterial hydrogels for chronic wound management
Type of metal nanoparticle |
Mechanism(s) |
Hydrogel matrix |
Antibacterial capability |
Type of wound |
Wound healing effect |
Ref. |
Ag |
Bacterial membrane destruction; ROS generation; DNA damage; protein denaturation; enzyme inactivation |
Methacrylate gelatine |
Antibacterial ratio > 95% for S. aureus, E. coli, and P. aeruginosa |
Infected burn wound model in rats |
Reduces the number of bacteria; accelerates wound healing; promotes early angiogenesis; regulates immune reaction |
139
|
Au |
Bacterial ATP synthase disruption; ROS generation; regulates cytokines; promotes collagen and VEGF expression; promotes fibroblast proliferation; reduces apoptosis; accelerates angiogenesis |
Sodium alginate |
Bacterial killing of >95% for P. aeruginosa and E. coli; up to 60% for Gram-positive S. aureus |
Infected rat wound |
Effective sterilisation; promotes angiogenesis and collagen fibre deposition; accelerates wound healing |
140
|
Cu |
Bacterial adhesion; destruction of the cell wall; promotes IL-2; enhances immunity; promotes fibroblast production of collagen |
Polyacrylamide |
Decreases viability of E. coli from 26.8% to 5.1% when increased from 2 mol% to 10 mol% |
Infected mouse wound |
Kills bacteria and promotes collagen production and deposition; reduces the inflammatory response; accelerates wound healing |
141
|
Zn |
Disrupts cell membranes; imposes oxidant injury; promotes migration of keratinocytes and ECM regeneration |
Gelatine |
Effectively kills E. coli and S. aureus |
Infected mouse wound |
Improves wound healing by controlling the inflammatory response; enhances epidermal and granulation tissue formation; stimulates angiogenesis |
142
|
Fe |
Induces bacterial death, membrane damage, DNA degradation, and lipid peroxidation |
Chitin and tannic acid |
Antibacterial activity against E. coli (92%) and S. aureus (97%) |
Infected mouse wound |
Effective and rapid bactericidal effects; accelerates wound closure; promotes skin tissue repair |
143
|
Ga |
Impairs bacterial iron metabolism; disrupts enzyme activity |
Carboxymethyl cellulose |
Kills >60% of S. aureus |
Diabetic rat wound |
Prevents infection and accelerates diabetic wound healing |
144
|
Metal oxide nanoparticle-loaded hydrogels
Certain metal oxide NPs, such as zinc oxide (ZnO), copper oxide (CuO), cerium oxide (CeO2), manganese oxide (MnO2), and titanium oxide (TiO2), also show excellent antibacterial properties.145 The antibacterial mechanism comprises two primary aspects: the release of metal ions and photocatalysis. Photocatalysis is a unique antibacterial mechanism of metal oxide NPs. When exposed to ultraviolet irradiation, many strongly oxidising free radicals, namely hydroxyl radicals and hydrogen peroxide, are generated on the surface of metal oxide NPs, which damage bacterial cell membranes and cell components through oxidative stress.146
Among the various metal oxides, TiO2 has a high oxidation activity, strong stability, and is non-toxic to the human body. ROS produced under light irradiation can kill microorganisms that are in contact with them for a short time. Concurrently, in the absence of light, TiO2 can catalyse the surface decomposition of hydrogen peroxide, generating ROS.147 Indeed, TiO2 NPs can kill E. coli, S. aureus, P. aeruginosa, Enterococcus faecalis, and Candida albicans, among others and promote chronic wound healing.148,149 Ulu et al. designed a chitosan/polypropylene glycol/TiO2 composite hydrogel, which effectively suppressed the activity of S. aureus, E. coli, and Candida lipolytica.150 The incorporation of TiO2 NPs enhanced the material's antibacterial properties, demonstrating their potential biomedical application in chronic wound repair.
Carbon material-loaded hydrogels
Recently, carbon materials, including graphene, carbon dots (CDs), and carbon nanotubes (CNTs), have garnered significant interest in the biomedical field owing to their unique antibacterial properties. However, the poor stability of graphene often necessitates chemical modifications, such as conversion into graphene oxide (GO).151 Functional groups are present on the edge and base surface of GO, and are highly hydrophilic and can be dispersed in the solution to form a stable aqueous colloid and promote the assembly of a three-dimensional structure. ROS generated by GO induce oxidative stress, leading to microbial DNA damage, mitochondrial dysfunction, and cell death.152 Wang et al. incorporated chemically modified GO into chitosan hydrogels.153 The resulting CS–CGO composite hydrogels exhibited good biocompatibility and promoted fibroblast adhesion and proliferation. In a rat skin wound model, the CS–CGO hydrogel significantly accelerated wound healing, achieving a 92.2% healing rate within 21 days. Additionally, Ningrum et al. prepared a PVA hydrogel containing GO and Moringa leaf (MOL) extract for diabetic foot ulcer treatment.154 The PVA/MOL/GO hydrogel demonstrated excellent antibacterial properties against Gram-positive S. aureus and Gram-negative E. coli, with rates of 94% and 82%, respectively. The incorporation of GO improved the mechanical properties of the hydrogel and synergised with MOL to enhance antibacterial action.
The CDs represent a novel class of zero-dimensional carbon-based nanomaterials with excellent biocompatibility, good water solubility, and outstanding optical properties. The abundance of functional groups on the surface of CDs and their photodynamic ability to generate ROS confer unique advantages in combating drug-resistant bacteria and emerging viruses.155 The coupling of a CD variant, CD31, with polylysine (Plys) created an injectable self-healing hydrogel (CD-Plys) with broad-spectrum antibacterial activity, efficient wound healing, and good biocompatibility. CD-Plys effectively inhibited the activity of E. coli and S. aureus and significantly enhanced wound healing and angiogenesis in rats infected with these bacteria (Fig. 8).156
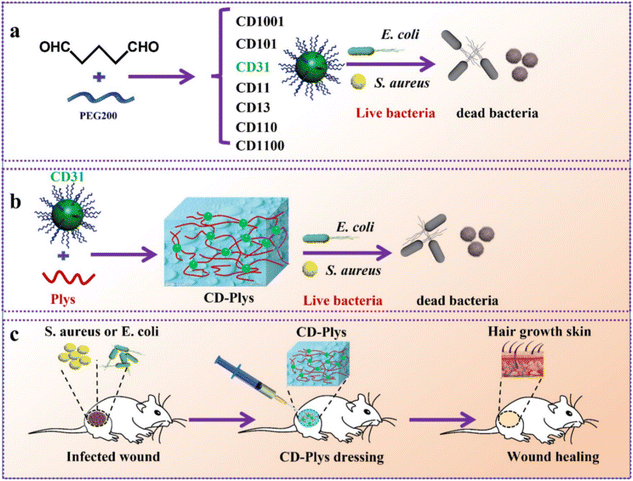 |
| Fig. 8 Schematic illustration of (a) preparation of CDs and their antimicrobial activity against Gram-negative and Gram-positive bacteria. (b) Synthesis of the CD-Plys hydrogel and its inhibitory effect on E. coli and S. aureus. (c) The CD-Plys hydrogel acts as a wound dressing to prevent bacterial infection and promote wound healing in mouse models.156 Copyright 2022, BMC. | |
CNTs—one-dimensional quantum materials with a unique structure—share similar antibacterial mechanisms with CDs. Liang et al. designed a GT–DA/CS/CNT composite hydrogel using gelatine-grafted dopamine (GT–DA) and polydopamine coating (CNT–PDA).157 This hydrogel exhibits antibacterial, adhesive, antioxidant, and conductive properties. The GT-DA/CS/CNT hydrogel effectively promotes wound healing and collagen deposition in mice with full-thickness skin defect wounds. As a new type of antibacterial material, the hydrogel composite material formed by combining carbon nanomaterials and hydrogels has a strong antibacterial ability, a wide source of raw materials, low cytotoxicity, and good biocompatibility. It can prevent bacterial resistance, demonstrating promising prospects for antibacterial applications. However, the preparation process for carbon nanomaterials is complicated, with a low yield, limiting their clinical transformation and commercialisation.
Stimuli-responsive hydrogels
The microenvironment of chronic wounds is complex and characterised by dynamic changes in cells and growth factors at various healing stages. Traditional hydrogel wound dressings, limited to the passive release of active substances, struggle to meet these changing requirements. In contrast, stimuli-responsive hydrogels are designed to react to alterations in external (e.g., light, electricity, magnetism, ultrasound) and internal (e.g., pH, temperature, enzymes, ROS) wound conditions. This responsiveness facilitates the controlled release of active substances tailored to the specific needs of each wound-healing stage, including antibacterial, anti-inflammatory, and proangiogenic requirements. This adaptability makes stimuli-responsive hydrogels an effective solution for the dynamic and complex nature of chronic wound healing.
Photo/thermal-responsive hydrogels.
Recent advances in the development of antibacterial hydrogels have extended beyond relying solely on chemical agents for bacterial eradication. Photothermal and photodynamic therapies (PTT and PDT, respectively) are effective antibacterial strategies. Hydrogels embedded with photothermal agents (PTAs) can generate high temperatures under specific light wavelengths, effectively killing bacteria. Similarly, hydrogels containing photodynamic agents (PDAs) induce the production of ROS, which can rupture bacterial cell membranes and denature proteins, serving antibacterial purposes.158,159 The high temperatures produced by PTT also improve the permeability of bacterial cell membranes, facilitating the entry of ROS and bolstering the antibacterial efficacy of PDT. Du et al. created a novel NIR light-triggered multifunctional injectable hydrogel by incorporating photothermal sodium nitroprusside into a Pt-modified porphyrin metal–organic framework and, in situ, modifying it with AuNPs.160 Upon dual NIR irradiation, the PSPG hydrogel generates high temperatures and ROS, resulting in nitric oxide release. This multifaceted approach effectively eliminated MRSA and E. coli biofilms and destroyed bacterial cell membranes. By synergising PDT and PTT, this hydrogel achieves efficient sterilisation and biofilm removal. In vivo, the bacterial load of wounds was decreased by 99.9%. Additionally, the hydrogel promoted wound healing in MRSA and P. aeruginosa infections by enhancing angiogenesis, collagen deposition, and reducing inflammation. Furthermore, photo-responsive hydrogels can be gelatinised and dissolved using different illumination times to prevent secondary damage from dressing changes. Photo-responsive hydrogels can utilise light signals of different wavelengths to generate photo-responsive reactions through photosensitive groups, converting light signals into various physical or chemical signals and altering the physical or chemical properties of the hydrogels. The common types of photochemical reactions for photosensitive groups include dimerisation, cleavage, and isomerisation reactions.161 Photo-responsive groups represented by thiol–ene undergo photoinduced dimerisation under illumination conditions, providing abundant crosslinking points for hydrogels.162 Therefore, certain dimeric groups can undergo crosslinking–decrosslinking under light conditions, achieving the gel–sol transition of light-responsive hydrogels. Additionally, by integrating photolytic groups, such as ortho-nitrobenzyl esters, with the hydrogel framework, irreversible damage to the hydrogel network occurs upon exposure to light, leading to changes in its physical or chemical properties.163 Li et al. designed an all-optical operating hydrogel dressing for chronic wounds composed of hyaluronic acid methacrylate (MeHA) and the photostable copulation agent o-nitrobenzene derivative (o-NB), which achieved rapid and remote control of dressing changes (30 s of gelation and 4 min of light irradiation dissolution).164 In diabetic mouse models, photo-responsive hydrogels can reduce secondary damage caused by repeated dressing changes and promote wound epithelisation, collagen deposition, and cell proliferation, accelerating wound healing. However, the use of photo-responsive antibacterial hydrogels for chronic wound management poses several challenges. The primary limitation is the restricted penetration depth of light, which reduces the effectiveness of hydrogels in deeper tissues. Additionally, issues such as low photothermal conversion efficiency, limited biosafety and biodegradability, and potential cytotoxicity of these hydrogels must be addressed. Despite these challenges, the potential of photo-responsive antibacterial hydrogels for advancing intelligent wound-dressing technologies is undeniable.
pH-responsive hydrogels.
The pH of the wound microenvironment plays a critical role in wound healing. Healthy, intact skin typically has an acidic pH of approximately 4–6. Meanwhile, acute wounds expose the underlying neutral tissue, raising the pH to approximately 7.4. As healing progresses, the pH typically reverts to acidic. However, chronic wounds often exhibit a pH range between 7 and 9, influenced by factors such as the blood, interstitial fluid, and ammonia. Bacterial infections in wounds can lower the pH due to acidic substances, including lactic and carbonic acid produced by bacterial metabolism.165,166 A pH-responsive hydrogel wound dressing can adapt to the pH change of the wound local environment and increase its interaction with the bacteria by ionising the functional groups to effectively penetrate the bacterial cell membrane. They can also release loaded antibacterial components.167 Based on the pH value of bacteria-infected wounds, Zhang et al. prepared a multifunctional hydrogel (ZC-QPP) loaded with ZnO@CeO2, chitosan quaternary ammonium salt (QCS), PVA, and PEG.168 Under the acidic conditions of a bacterial infection microenvironment, the borate ester bond in the ZC-QPP hydrogel breaks down, releasing ZnO. Gradual dissociation of Zn2+ under acidic conditions directly destroys the bacterial membrane. This hydrogel is effective against E. coli and S. aureus, promotes wound healing in S. aureus-infected wounds, and serves as a multifunctional dressing to monitor wound pH changes.
Other stimuli-responsive hydrogels.
Stimulation response methods, such as to ultrasound, enzymes, and ROS, are also used in antibacterial hydrogels to enhance the permeability of bacterial membranes, reduce cell membrane fluidity, and depolarise membranes, killing bacteria.169 Zhang et al. designed a nanoenzyme–hydrogel scaffold (PNAs).170 Under ultrasound, the surface plasmon resonance effect enhanced electron polarisation, effectively catalysing hydrogen peroxide, promoting glutathione synthesis, and enhancing the ROS scavenging ability. PNAs promote in vitro cell proliferation, migration, and angiogenesis, promote diabetic wound healing in vivo, and control the release of antibacterial components according to changes in specific active substances in infected wounds. For example, when a curcumin-encapsulated liposome hydrogel came into contact with phospholipase A2 (PLA2) in the wound exudate, it triggered the hydrolysis of lecithin in liposomes, destroying the liposomes and releasing curcumin to achieve antibacterial effects.171 This hydrogel inhibited drug degradation during delivery, improved drug release efficiency, and released drugs in response to changes in PLA2 activity in infected tissues. In chronic wounds, redox imbalance leads to excessive ROS production, which inhibits wound healing. Responsive antibacterial hydrogels can accelerate drug release and promote wound healing by breaking open when ROS levels increase. Zhao et al. developed a ROS-responsive hydrogel by crosslinking a borate ester bond between a ROS-responsive crosslinking agent and polyvinyl alcohol.172 In infectious diabetic wounds, this hydrogel removes excessive ROS in the wound while cleaving borate ester bonds, releases mupirocin that kills bacteria, and promotes angiogenesis and collagen production to accelerate wound healing. Therefore, it can be used to effectively treat various types of wounds, including diabetic wounds that are difficult to heal and bacterial infections.
Multi-responsive hydrogels.
Single-response hydrogel dressings often fail to achieve efficient and targeted drug delivery, making them less effective for chronic wound treatment. To overcome this, researchers are developing dual/multi-stimuli-responsive hydrogel wound dressings that allow for more precise control over the release of active substances to cater to the varying needs of different wound healing stages.173 Zha et al. prepared a NIR and pH dual-responsive hydrogel membrane composed of Cu–humic acid (HAs) NPs embedded in a PVA matrix and loaded with the macrophage recruiter SEW2871, to target bacterially infected skin wounds.174 Cu-HA NPs eradicate bacterial infections via NIR-induced local hyperthermia, while the pH response increases the solubility of HAs under acidic conditions. This hydrogel effectively promotes infectious wound regeneration by fostering M2 macrophage polarisation, reducing oxidative stress, encouraging angiogenesis, and aiding in collagen deposition. According to the special physiological environment of chronic wounds, hydrogels with responsiveness to photothermal, ROS, pH, and glucose stimuli can be designed to release drugs on demand and improve the microenvironment of wounds to cater to the requirements of different stages of wound healing. For example, high glucose concentrations, high MMP-9 expression, and long-term inflammatory reactions can lead to chronic diabetic wounds that are difficult to heal. Accordingly, Zhou et al. constructed a glucose and MMP-9 dual-responsive temperature-sensitive self-adaptive hydrogel (CBP/GMs@Cel & INS) to treat chronic diabetic wounds (Fig. 9).175 This hydrogel encapsulates insulin (INS) and celecoxib-loaded gelatine microspheres (GMs@Cel) within a matrix of PVA and chitosan-grafted phenylboronic acid (CS-BA). The hydrogel releases insulin and celecoxib in response to high glucose and MMP-9 levels, helping to regulate these factors and reduce inflammation in diabetic rat wound models, accelerating wound healing. These innovative hydrogels, with their dual-response release strategies, offer novel approaches for chronic wound treatment. We have summarised common stimuli-responsive antibacterial hydrogels for chronic wound management in Table 4.
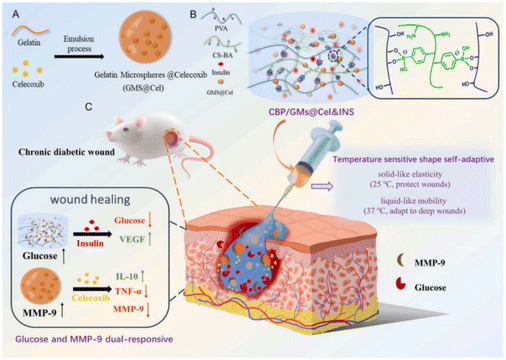 |
| Fig. 9 Design strategy for glucose and MMP-9 dual-responsive self-adaptive hydrogels for treating chronic diabetic wounds.175 Copyright 2022, Elsevier. | |
Table 4 Application of common stimuli-responsive antibacterial hydrogels for chronic wound management
Type of stimuli-responsiveness |
Stimulus condition |
Responder |
Antibacterial mechanism(s) |
Ref. |
Photothermal-responsive |
NIR |
Photothermal; sodium nitroprusside |
Generates high temperatures under specific light wavelengths, effectively killing bacteria |
160
|
Photodynamic-responsive |
NIR |
Gold particles |
Induces ROS production, rupturing bacterial cell membranes |
160
|
pH-responsive |
pH |
Chitosan quaternary ammonium salt; ZnO |
With a change in wound pH, increases the interaction with bacteria by ionising the functional groups to effectively penetrate the bacterial cell membrane |
168
|
Sound-responsive |
Ultrasound |
Nanoenzyme hydrogel scaffold |
The surface plasmon resonance effect enhances electron polarisation, effectively catalyses hydrogen peroxide, and enhances ROS scavenging ability |
170
|
Enzyme-responsive |
Enzyme |
Phospholipase; A2 |
Controls the release of antibacterial components based on changes in specific active substances in chronic wounds |
171
|
ROS-responsive |
ROS |
ROS-responsive crosslinking agent |
Controls the release of antibacterial components based on changes in ROS levels in chronic wounds |
172
|
Multi-responsive |
Photo–pH |
Cu–humic acid |
Controls continuous antibacterial component release through multiple conditioned stimuli |
174
|
Smart-responsive hydrogels can respond to specific wound environments, such as low pH, high ROS, high glucose, and overexpressed enzymes, or external conditions, such as temperature, light, and magnetism, to achieve intelligent control of drug release on demand and improve the local microenvironment of chronic wounds.176 However, variations in individual responses may lead to large differences in the role of single-response hydrogels in chronic wounds, whereas multifunctional smart-responsive hydrogels are more suitable for the chronic wound microenvironment to achieve better therapeutic results.177 Moreover, as the environmental state of the wound changes over time, different drugs and molecules may be required at different times. Multifunctional smart-responsive hydrogels enable time–space controlled release of drugs, avoiding drug resistance or other adverse side effects.178 Yang et al. designed a smart hydrogel drug delivery system based on an MXene, which has multiple response capabilities towards light and magnetism, with controllable drug delivery ability, and is suitable for deep wound treatment.179 It is composed of magnetic colloids coated with an MXene and an n-isopropyl acrylamide-alginate double-network hydrogel. Loaded with AgNPs, the system temperature rises rapidly under near-red external and alternating magnetic field (AMF) strips, thus triggering the release of AgNPs in a controlled manner. The hydrogel not only has good cytocompatibility and biopracticability but can also reduce drug toxicity and promote wound healing. At present, many studies focus on wound dressings that combine diagnosis and treatment to promote wound healing while monitoring pH, blood sugar levels, and wound infection.180,181 This integrated approach represents the future direction of the development of multifunctional smart-responsive antibacterial hydrogels.
Summary and prospects
With the ageing population and an increase in the prevalence of chronic diseases, the incidence of chronic wounds has gradually increased. Owing to metabolic abnormalities and persistent inflammation of the local tissue, wounds are susceptible to bacterial infection, causing the wound to remain unhealed for an extended period and even requiring amputation. Hydrogels boast a strong water absorption performance, which can maintain the wet environment of the wound, provide a stable and favourable environment for cell proliferation, promote the growth of new granulation tissue in the wound, and reduce secondary damage caused by dressing changes. Simultaneously, the unique three-dimensional network structure of hydrogels can realise the sustained release of antibacterial components and bioactive substances to achieve the effects of bacterial inhibition and accelerated wound healing. Therefore, the application of multifunctional antibacterial hydrogels represents a new approach to chronic wound management. This article focuses on the pathological characteristics of chronic wounds and the properties and classification of antibacterial hydrogels.
Although various antibacterial hydrogels have shown potential for application in chronic wound treatment owing to their unique antibacterial mechanisms, they all have shortcomings. These include the potential toxicity of metal nanoions, bacterial resistance to antibiotics, and low synthesis rate of carbon-containing materials. However, achieving effective and safe antibacterial effects using a single mechanism is relatively challenging. Combining two or more antibacterial materials can ensure effective bacteriostasis through synergistic antibacterial activity, reduce the disadvantages of a single material, and play a role in complementing each other. The most common combination includes metal NPs with antibiotics or GO. The demand for chronic wound treatment has steadily increased with the development and integration of biomedicine and materials science. Multifunctional intelligent stimuli-responsive antibacterial hydrogels show unique advantages in wound treatment. According to the changes in the chronic wound microenvironment, hydrogels with responsiveness to single or multiple stimuli, such as temperature, ROS, pH, and glucose, can be designed to release drugs on demand and improve the microenvironment to meet the needs of different wound healing stages.
Multifunctional antibacterial hydrogels have made progress in the treatment of chronic wounds and are expected to become widely used; however, there remains room for further development. In particular, the preparation process of hydrogels must be optimised to improve the structure of materials and enhance their functional properties. Moreover, various combinations of antibacterial components and bioactive substances should be evaluated to improve the antibacterial and reparative properties of hydrogels through the stimulation response mode and reduce the related side effects. The development of new antibacterial hydrogels with high efficiency, intelligence, and adaptability to the wound microenvironment will quickly become an important research direction. Specifically, an integrated multifunctional hydrogel with sensing therapy to dynamically monitor changes in the wound microenvironment and achieve accurate drug release to promote wound healing represents the metaphorical “golden goose” in the field.
Author contributions
Y. H. conceived and wrote the manuscript with the assistance of Y. S. L. Y. summarised the literature studies. Q. D. and X. H. revised the manuscript. Y. S. secured funding. All authors read and approved the final manuscript.
Conflicts of interest
There are no conflicts to declare.
Acknowledgements
The study was financially supported by the National Key Research and Development Program of China (Grant No. 2022YFC2403004).
References
- H. E. Talbott, S. Mascharak, M. Griffin, D. C. Wan and M. T. Longaker, Wound healing, fibroblast heterogeneity, and fibrosis, Cell Stem Cell, 2022, 29(8), 1161–1180 CrossRef CAS PubMed.
- S. Bowers and E. Franco, Chronic Wounds: Evaluation and Management, Am. Fam. Physician, 2020, 101(3), 159–166 Search PubMed.
- R. Yan, F. Yu, K. Strandlund, J. Han, N. Lei and Y. Song, Analyzing factors affecting quality of life in patients hospitalized with chronic wound, Wound Repair Regen., 2021, 29(1), 70–78 CrossRef PubMed.
- M. J. Carter, J. DaVanzo, R. Haught, M. Nusgart, D. Cartwright and C. E. Fife, Chronic wound prevalence and the associated cost of treatment in Medicare beneficiaries: changes between 2014 and 2019, J. Med. Econ., 2023, 26(1), 894–901 CrossRef PubMed.
- S. Kapp and N. Santamaria, The financial and quality-of-life cost to patients living with a chronic wound in the community, Int. Wound J., 2017, 14(6), 1108–1119 CrossRef PubMed.
- D. G. Armstrong, T. W. Tan, A. Boulton and S. A. Bus, Diabetic Foot Ulcers: A Review, J. Am. Med. Assoc., 2023, 330(1), 62–75 CrossRef CAS PubMed.
- Y. Huang, B. Q. Mao, P. W. Ni, Q. Wang, T. Xie and L. Hou, Investigation of the status and influence factors of caregiver's quality of life on caring for patients with chronic wound during COVID-19 epidemic, Int. Wound J., 2021, 18(4), 440–447 CrossRef PubMed.
- C. Pouget, C. Dunyach-Remy, C. Magnan, A. Pantel, A. Sotto and J. P. Lavigne, Polymicrobial Biofilm Organization of Staphylococcus aureus and Pseudomonas aeruginosa in a Chronic Wound Environment, Int. J. Mol. Sci., 2022, 23(18), 10761 CrossRef CAS PubMed.
- W. Peng, D. Li and K. Dai,
et al., Recent progress of collagen, chitosan, alginate and other hydrogels in skin repair and wound dressing applications, Int. J. Biol. Macromol., 2022, 208, 400–408 CrossRef CAS PubMed.
- G. D. Winter, Formation of the scab and the rate of epithelization of superficial wounds in the skin of the young domestic pig, Nature, 1962, 193, 293–294 CrossRef CAS PubMed.
- K. Nuutila and E. Eriksson, Moist Wound Healing with Commonly Available Dressings, Adv. Wound Care, 2021, 10(12), 685–698 CrossRef PubMed.
- B. Cullen and A. Gefen, The biological and physiological impact of the performance of wound dressings, Int. Wound J., 2023, 20(4), 1292–1303 CrossRef PubMed.
- T. C. Ho, C. C. Chang and H. P. Chan,
et al., Hydrogels: Properties and Applications in Biomedicine, Molecules, 2022, 27(9), 2902 CrossRef CAS PubMed.
- W. Liu, R. Gao and C. Yang,
et al., ECM-mimetic immunomodulatory hydrogel for methicillin-resistant Staphylococcus aureus-infected chronic skin wound healing, Sci. Adv., 2022, 8(27), eabn7006 CrossRef CAS PubMed.
- C. Wu, L. Long and Y. Zhang,
et al., Injectable conductive and angiogenic hydrogels for chronic diabetic wound treatment, J. Controlled Release, 2022, 344, 249–260 CrossRef CAS PubMed.
- Y. Luo, L. Cui and L. Zou,
et al., Mechanically strong and on-demand dissoluble chitosan hydrogels for wound dressing applications, Carbohydr. Polym., 2022, 294, 119774 CrossRef CAS PubMed.
- A. H. Rasmi, E. F. Ahmed, A. Darwish and G. Gad, Virulence genes distributed among Staphylococcus aureus causing wound infections and their correlation to antibiotic resistance, BMC Infect. Dis., 2022, 22(1), 652 CrossRef CAS PubMed.
- W. J. Caputo, P. Monterosa and D. Beggs, Antibiotic Misuse in Wound Care: Can Bacterial Localization through Fluorescence Imaging Help, Diagnostics, 2022, 12(12), 3207 CrossRef PubMed.
- M. Amiri, P. Khazaeli, A. Salehabadi and M. Salavati-Niasari, Adv. Colloid Interface Sci., 2021, 288, 102316 CrossRef CAS PubMed.
- J. Y. Ji, D. Y. Ren and Y. Z. Weng, Int. J. Nanomed., 2022, 17, 3163–3176 CrossRef PubMed.
- B. Jia, G. Li, E. Cao, J. Luo, X. Zhao and H. Huang, Mater. Today Bio, 2023, 19, 100582 CrossRef CAS PubMed.
- P. H. Wang, B. S. Huang, H. C. Horng, C. C. Yeh and Y. J. Chen, J. Chin. Med. Assoc., 2018, 81, 94–101 CrossRef PubMed.
- M. Rodrigues, N. Kosaric, C. A. Bonham and G. C. Gurtner, Physiol. Rev., 2019, 99, 665–706 CrossRef CAS PubMed.
- K. Raziyeva, Y. Kim, Z. Zharkinbekov, K. Kassymbek, S. Jimi and A. Saparov, Biomolecules, 2021, 11, 700 CrossRef CAS PubMed.
- H. Wang and L. Yang, Theranostics, 2023, 13, 4615–4635 CrossRef CAS PubMed.
- Y. Jiang, X. Xu, L. Xiao, L. Wang and S. Qiang, Front. Immunol., 2022, 13, 852419 CrossRef CAS PubMed.
- Y. Liu, Y. Liu and W. He,
et al.
, Front. Immunol., 2022, 13, 918223 CrossRef CAS PubMed.
- F. Cialdai, C. Risaliti and M. Monici, Front. Bioeng. Biotechnol., 2022, 10, 958381 CrossRef PubMed.
- A. F. Spielman, M. F. Griffin, J. Parker, A. C. Cotterell, D. C. Wan and M. T. Longaker, Adv. Wound Care, 2023, 12, 57–67 CrossRef PubMed.
- S. Tavakoli and A. S. Klar, Biomolecules, 2020, 10, 1169 CrossRef CAS PubMed.
- D. Prakashan, A. Roberts and S. Gandhi, Biotechnol. Genet. Eng. Rev., 2023, 1–29 Search PubMed.
- B. Durand, C. Pouget, C. Magnan, V. Molle, J. P. Lavigne and C. Dunyach-Remy, Microorganisms, 2022, 10, 1500 CrossRef CAS PubMed.
- W. Alam, J. Hasson and M. Reed, J. Am. Geriatr. Soc., 2021, 69, 2327–2334 CrossRef PubMed.
- M. Kandhwal, T. Behl, A. Kumar and S. Arora, Curr. Pharm. Des., 2021, 27, 1999–2014 CrossRef CAS PubMed.
- M. Maaz Arif, S. M. Khan and N. Gull,
et al.
, Int. J. Pharm., 2021, 598, 120270 CrossRef CAS PubMed.
- J. Hurlow and P. G. Bowler, J. Wound Care, 2022, 31, 436–445 CrossRef PubMed.
- C. N. Elangwe, S. N. Morozkina, R. O. Olekhnovich, A. Krasichkov, V. O. Polyakova and M. V. Uspenskaya, Polymers, 2022, 14, 5163 CrossRef CAS PubMed.
- A. Hassanshahi, M. Moradzad, S. Ghalamkari, M. Fadaei, A. J. Cowin and M. Hassanshahi, Cells, 2022, 11, 2953 CrossRef CAS PubMed.
- H. Al Sadoun, Cells, 2022, 11, 2430 CrossRef CAS PubMed.
- K. Geng, X. Ma and Z. Jiang,
et al.
, Front. Pharmacol., 2021, 12, 653940 CrossRef CAS PubMed.
- S. Polaka, P. Katare and B. Pawar,
et al.
, ACS Omega, 2022, 7, 30657–30672 CrossRef CAS PubMed.
- M. Chang and T. T. Nguyen, Acc. Chem. Res., 2021, 54, 1080–1093 CrossRef CAS PubMed.
- M. D. Caldwell, Surg. Clin. North Am., 2020, 100, 757–776 CrossRef PubMed.
- N. Graves, C. J. Phillips and K. Harding, Br. J. Dermatol., 2022, 187, 141–148 CrossRef CAS PubMed.
- I. C. Thaarup, A. Iversen, M. Lichtenberg, T. Bjarnsholt and T. H. Jakobsen, Microorganisms, 2022, 10, 775 CrossRef CAS PubMed.
- C. K. Sen, S. Roy, S. S. Mathew-Steiner and G. M. Gordillo, Plast. Reconstr. Surg., 2021, 148, 275e–288e CrossRef CAS PubMed.
- Z. Versey, W. S. da Cruz Nizer and E. Russell,
et al.
, Front. Immunol., 2021, 12, 648554 CrossRef CAS PubMed.
- G. A. James, E. Swogger and R. Wolcott,
et al.
, Wound Repair Regen., 2008, 16, 37–44 CrossRef PubMed.
- M. Dietrich, M. Besser, E. S. Debus, R. Smeets and E. K. Stuermer, J. Wound Care, 2023, 32, 446–455 CrossRef PubMed.
- K. Ousey and L. Ovens, J. Wound Care, 2023, 32, S4–S10 CrossRef PubMed.
- M. M. Mihai, M. B. Dima, B. Dima and A. M. Holban, Materials, 2019, 12, 2176 CrossRef CAS PubMed.
- I. H. Ali, A. Ouf and F. Elshishiny,
et al.
, ACS Omega, 2022, 7, 1838–1850 CrossRef CAS PubMed.
- X. Chen, Y. Zhang, W. Yu, W. Zhang, H. Tang and W. E. Yuan, J Nanobiotechnology, 2023, 21, 129 CrossRef CAS PubMed.
- S. Nasra, M. Patel, H. Shukla, M. Bhatt and A. Kumar, Life Sci., 2023, 334, 122232 CrossRef CAS PubMed.
- H. Hu and F. J. Xu, Biomater. Sci., 2020, 8, 2084–2101 RSC.
- Y. Liang, J. He and B. Guo, ACS Nano, 2021, 15, 12687–12722 CrossRef CAS PubMed.
- J. Liu, W. Jiang, Q. Xu and Y. Zheng, Gels, 2022, 8, 503 CrossRef CAS PubMed.
- M. O. Teixeira, J. C. Antunes and H. P. Felgueiras, Antibiotics, 2021, 10, 248 CrossRef CAS PubMed.
- F. M. Aldakheel, M. Sayed, D. Mohsen, M. H. Fagir and D. K. El Dein, Gels, 2023, 9, 530 CrossRef CAS PubMed.
- M. Sari, A. F. Cobre, R. Pontarolo and L. M. Ferreira, Pharmaceutics, 2023, 15, 874 CrossRef CAS PubMed.
- P. J. Kim and J. S. Steinberg, Semin. Vasc. Surg., 2012, 25, 70–74 CrossRef PubMed.
- R. D. Wolcott, K. P. Rumbaugh and G. James,
et al.
, J. Wound Care, 2010, 19, 320–328 CrossRef CAS PubMed.
- N. K. Devanga Ragupathi, B. Veeraraghavan, E. Karunakaran and P. N. Monk, Front. Med., 2022, 9, 987011 CrossRef PubMed.
- X. Song, P. Liu and X. Liu,
et al.
, Mater. Sci. Eng., C, 2021, 128, 112318 CrossRef CAS PubMed.
- Q. Wang, W. Qiu and M. Li,
et al.
, J. Colloid Interface Sci., 2022, 617, 542–556 CrossRef CAS PubMed.
- G. K. Mannala, M. Rupp and N. Walter,
et al.
, Eur. Cells Mater., 2022, 43, 66–78 CrossRef CAS PubMed.
- J. Hu, S. Chen and Y. Yang,
et al.
, Adv. Healthc. Mater., 2022, 11, e2200299 CrossRef PubMed.
- V. Brumberg, T. Astrelina, T. Malivanova and A. Samoilov, Biomedicines, 2021, 9, 1235 CrossRef CAS PubMed.
- G. Sun, Y. I. Shen and J. W. Harmon, Adv. Healthc. Mater., 2018, 7, e1800016 CrossRef PubMed.
- P. Sánchez-Cid, M. Jiménez-Rosado, A. Romero and V. Pérez-Puyana, Polymers, 2022, 14, 3023 CrossRef PubMed.
- I. Firlar, M. Altunbek, C. McCarthy, M. Ramalingam and G. Camci-Unal, Gels, 2022, 8, 127 CrossRef CAS PubMed.
- S. Schaly, P. Islam, A. Abosalha, J. L. Boyajian, D. Shum-Tim and S. Prakash, Pharmaceuticals, 2022, 15, 1382 CrossRef CAS PubMed.
- S. Afrin, M. Shahruzzaman and P. Haque,
et al.
, Gels, 2022, 8, 340 CrossRef CAS PubMed.
- S. Li, S. Dong and W. Xu,
et al.
, Adv. Sci., 2018, 5, 1700527 CrossRef PubMed.
- E. F. Palermo, K. Lienkamp, E. R. Gillies and P. J. Ragogna, Angew. Chem., Int. Ed., 2019, 58, 3690–3693 CrossRef CAS PubMed.
- S. O. Ebhodaghe, J. Biomater. Sci., Polym. Ed., 2022, 33, 1595–1622 CrossRef CAS PubMed.
- C. H. Wang, J. H. Cherng and C. C. Liu,
et al.
, Int. J. Mol. Sci., 2021, 22, 7067 CrossRef CAS PubMed.
- M. A. Matica, F. L. Aachmann, A. Tøndervik, H. Sletta and V. Ostafe, Int. J. Mol. Sci., 2019, 20, 5889 CrossRef CAS PubMed.
- J. Cao, P. Wu, Q. Cheng, C. He, Y. Chen and J. Zhou, ACS Appl. Mater. Interfaces, 2021, 13, 24095–24105 CrossRef CAS PubMed.
- Y. Hao, W. Zhao, H. Zhang, W. Zheng and Q. Zhou, Carbohydr. Polym., 2022, 287, 119336 CrossRef CAS PubMed.
- D. Z. Zmejkoski, N. M. Zdravković and D. D. Trišić,
et al.
, Int. J. Biol. Macromol., 2021, 191, 315–323 CrossRef CAS PubMed.
- Z. Pan, H. Ye and D. Wu, APL Bioeng., 2021, 5, 011504 CrossRef CAS PubMed.
- Y. Peng, D. He and X. Ge,
et al.
, Bioact. Mater., 2021, 6, 3109–3124 CAS.
- J. Park, T. Y. Kim and Y. Kim,
et al.
, Adv. Sci., 2023, 10, e2303651 CrossRef PubMed.
- Y. Zhang, M. Li and Y. Wang,
et al.
, Bioact. Mater., 2023, 26, 323–336 CAS.
- R. Zhang, L. Xu and C. Dong, Protein Pept. Lett., 2022, 29, 641–650 CrossRef CAS PubMed.
- Q. Y. Zhang, Z. B. Yan and Y. M. Meng,
et al.
, Mil. Med. Res., 2021, 8, 48 CAS.
- C. Wang, T. Hong, P. Cui, J. Wang and J. Xia, Adv. Drug Delivery Rev., 2021, 175, 113818 CrossRef CAS PubMed.
- B. C. Borro, R. Nordström and M. Malmsten, Colloids Surf., B, 2020, 187, 110835 CrossRef CAS PubMed.
- H. Suo, M. Hussain and H. Wang,
et al.
, Biomacromolecules, 2021, 22, 3049–3059 CrossRef CAS PubMed.
- S. Wei, P. Xu and Z. Yao,
et al.
, Acta Biomater., 2021, 124, 205–218 CrossRef CAS PubMed.
- Q. Li, C. Wen and J. Yang,
et al.
, Chem. Rev., 2022, 122, 17073–17154 CrossRef CAS PubMed.
- X. Qiu, J. Zhang and L. Cao,
et al.
, ACS Appl. Mater. Interfaces, 2021, 13, 7060–7069 CrossRef CAS PubMed.
- K. Fang, Q. Gu and M. Zeng,
et al.
, J. Mater. Chem. B, 2022, 10, 4142–4152 RSC.
- B. Jia, G. Li, E. Cao, J. Luo, X. Zhao and H. Huang, Mater. Today Bio, 2023, 19, 100582 CrossRef CAS PubMed.
- I. Orlando, P. Basnett, R. Nigmatullin, W. Wang, J. C. Knowles and I. Roy, Front. Bioeng. Biotechnol., 2020, 8, 557885 CrossRef PubMed.
- J. Shen, Z. Zhou and D. Chen,
et al.
, Colloids Surf., B, 2021, 200, 111568 CrossRef CAS PubMed.
- W. Gong, R. Wang and H. Huang,
et al.
, Int. J. Biol. Macromol., 2023, 227, 698–710 CrossRef CAS PubMed.
- O. Kapusta, A. Jarosz, K. Stadnik, D. A. Giannakoudakis, B. Barczyński and M. Barczak, Int. J. Mol. Sci., 2023, 24, 2191 CrossRef CAS PubMed.
- H. Tashkandi, Open Life Sci., 2021, 16, 1091–1100 CrossRef CAS PubMed.
- J. Movaffagh, B. S. Fazly Bazzaz and A. T. Yazdi,
et al.
, Wounds, 2019, 31, 228–235 Search PubMed.
- J. S. Park, S. J. An, S. I. Jeong, H. J. Gwon, Y. M. Lim and Y. C. Nho, Polymers, 2017, 9, 248 CrossRef PubMed.
- C. Mohanty and S. K. Sahoo, Drug Discovery Today, 2017, 22, 1582–1592 CrossRef CAS PubMed.
- A. Kumari, N. Raina and A. Wahi,
et al.
, Pharmaceutics, 2022, 14, 2288 CrossRef CAS PubMed.
- T. Le, T. Nguyen and V. M. Nguyen,
et al.
, Molecules, 2023, 28, 6433 CrossRef CAS PubMed.
- S. S. Kamar, D. H. Abdel-Kader and L. A. Rashed, Ann. Anat., 2019, 222, 94–102 CrossRef PubMed.
- M. Zhang, H. Feng, Y. Gao, X. Gao and Z. Ji, Int. Wound J., 2023, 20, 4015–4022 CrossRef PubMed.
- P. Kaur, V. S. Gondil and S. Chhibber, Int. J. Pharm., 2019, 572, 118779 CrossRef CAS PubMed.
- R. F. Eyler and K. Shvets, Clin. J. Am. Soc. Nephrol., 2019, 14, 1080–1090 CrossRef CAS PubMed.
- D. Simões, S. P. Miguel, M. P. Ribeiro, P. Coutinho, A. G. Mendonça and I. J. Correia, Eur. J. Pharm. Biopharm., 2018, 127, 130–141 CrossRef PubMed.
- M. López-Pérez, J. M. Haro-Moreno, C. Molina-Pardines, M. P. Ventero and J. C. Rodríguez, mSphere, 2021, 6, e0083621 CrossRef PubMed.
- W. Pan, B. Wu and C. Nie,
et al.
, ACS Nano, 2023, 17, 11253–11267 CrossRef CAS PubMed.
- H. Staecker, J. Cystic Fibrosis, 2021, 20, 5 CrossRef CAS PubMed.
- T. A. Le, T. Hiba and D. Chaudhari,
et al.
, Adv. Ther., 2023, 40, 1357–1365 CrossRef CAS PubMed.
- N. A. Mohd Razali and W. C. Lin, Mater. Today Bio, 2022, 16, 100347 CrossRef CAS PubMed.
- B. Cao, C. Wang and P. Guo,
et al.
, Int. J. Biol. Macromol., 2023, 245, 125528 CrossRef CAS PubMed.
- P. S. Dube, L. J. Legoabe and R. M. Beteck, Mol. Divers., 2023, 27, 1501–1526 CrossRef CAS PubMed.
- A. M. Shehabeldine, A. A. Al-Askar and H. AbdElgawad,
et al.
, Appl. Biochem. Biotechnol., 2023, 195, 6421–6439 CrossRef CAS PubMed.
- J. Hwang, H. Huang, M. O. Sullivan and K. L. Kiick, Mol. Pharm., 2023, 20, 1696–1708 CrossRef CAS PubMed.
- M. Babaluei, Y. Mojarab, F. Mottaghitalab and M. Farokhi, Int. J. Biol. Macromol., 2023, 249, 126051 CrossRef CAS PubMed.
- K. Huang, W. Liu and W. Wei,
et al.
, ACS Nano, 2022, 16, 19491–19508 CrossRef CAS PubMed.
- T. G. Polat, O. Duman and S. Tunç, Int. J. Biol. Macromol., 2020, 164, 4591–4602 CrossRef CAS PubMed.
- X. Qing, G. He and Z. Liu,
et al.
, Carbohydr. Polym., 2021, 261, 117875 CrossRef CAS PubMed.
- C. Xie, Q. Zhang, Z. Li, S. Ge and B. Ma, Polymers, 2022, 14, 1816 CrossRef CAS PubMed.
- J. Yang, K. Wang, D. G. Yu, Y. Yang, S. Bligh and G. R. Williams, Mater. Sci. Eng., C, 2020, 111, 110805 CrossRef CAS PubMed.
- J. Balcucho, D. M. Narváez, N. A. Tarazona and J. L. Castro-Mayorga, Polymers, 2023, 15, 920 CrossRef CAS PubMed.
- S. Amer, N. Attia, S. Nouh, M. El-Kammar, A. Korittum and H. Abu-Ahmed, J. Biomater. Appl., 2020, 35, 287–298 CrossRef CAS PubMed.
- P. Mathur, S. Jha, S. Ramteke and N. K. Jain, Artif. Cells, Nanomed., Biotechnol., 2018, 46, 115–126 CrossRef CAS PubMed.
- A. N. Cadinoiu, D. M. Rata and O. M. Daraba,
et al.
, Int. J. Mol. Sci., 2022, 23, 10671 CrossRef CAS PubMed.
- P. Hayat, I. Khan and A. Rehman,
et al.
, Molecules, 2023, 28, 637 CrossRef CAS PubMed.
- A. M. Mohammed, K. T. Hassan and O. M. Hassan, Int. J. Biol. Macromol., 2023, 233, 123580 CrossRef CAS PubMed.
- L. Xu, Y. Y. Wang, J. Huang, C. Y. Chen, Z. X. Wang and H. Xie, Theranostics, 2020, 10, 8996–9031 CrossRef CAS PubMed.
- S. I. Basha, S. Ghosh and K. Vinothkumar,
et al.
, Mater. Sci. Eng., C, 2020, 111, 110743 CrossRef CAS PubMed.
- M. Azizi-Lalabadi, F. Garavand and S. M. Jafari, Adv. Colloid Interface Sci., 2021, 293, 102440 CrossRef CAS PubMed.
- M. Noga, J. Milan, A. Frydrych and K. Jurowski, Int. J. Mol. Sci., 2023, 24, 5133 CrossRef CAS PubMed.
- A. Q. Malik, T. Mir and D. Kumar,
et al.
, Environ. Sci. Pollut. Res. Int., 2023, 30, 69796–69823 CrossRef CAS PubMed.
- J. Jeevanandam, S. F. Kiew and S. Boakye-Ansah,
et al.
, Nanoscale, 2022, 14, 2534–2571 RSC.
- F. M. Aldakheel, D. Mohsen, M. M. El Sayed, K. A. Alawam, A. S. Binshaya and S. A. Alduraywish, Molecules, 2023, 28, 3241 CrossRef CAS PubMed.
- Y. Xiong, Y. Xu and F. Zhou,
et al.
, Bioeng. Transl. Med., 2023, 8, e10373 CrossRef CAS PubMed.
- S. Kaul, P. Sagar, R. Gupta, P. Garg, N. Priyadarshi and N. K. Singhal, ACS Appl. Mater. Interfaces, 2022, 14, 44084–44097 CrossRef CAS PubMed.
- G. Guo, J. Sun and Y. Wu,
et al.
, J. Mater. Chem. B, 2022, 10, 6414–6424 RSC.
- B. Tao, C. Lin and X. Qin,
et al.
, Mater. Today Bio, 2022, 13, 100216 CrossRef CAS PubMed.
- M. Ma, Y. Zhong and X. Jiang, J. Mater. Chem. B, 2021, 9, 4567–4576 RSC.
- G. Chen, K. Ren, H. Wang, L. Wu, D. Wang and L. Xu, J. Biomater. Appl., 2023, 37, 1676–1686 CrossRef CAS PubMed.
- A. Czyżowska and A. Barbasz, Int. J. Environ. Health Res., 2022, 32, 885–901 CrossRef PubMed.
- R. Li, Z. Chen, N. Ren, Y. Wang, Y. Wang and F. Yu, J. Photochem. Photobiol., B, 2019, 199, 111593 CrossRef CAS PubMed.
- C. Guo, K. Wang and S. Hou,
et al.
, J. Hazard. Mater., 2017, 323, 710–718 CrossRef CAS PubMed.
- M. Nilavukkarasi, S. Vijayakumar, M. Kalaskar, N. Gurav, S. Gurav and P. K. Praseetha, Biochem. Biophys. Res. Commun., 2022, 623, 127–132 CrossRef CAS PubMed.
- M. A. Ansari, H. M. Albetran and M. H. Alheshibri,
et al.
, Antibiotics, 2020, 9, 572 CrossRef CAS PubMed.
- A. Ulu, E. Birhanlı, S. Köytepe and B. Ateş, Int. J. Biol. Macromol., 2020, 163, 529–540 CrossRef CAS PubMed.
- A. Raslan, L. Saenz Del Burgo, J. Ciriza and J. L. Pedraz, Int. J. Pharm., 2020, 580, 119226 CrossRef CAS PubMed.
- P. Zare, M. Aleemardani, A. Seifalian, Z. Bagher and A. M. Seifalian, Nanomaterials, 2021, 11, 1083 CrossRef CAS PubMed.
- Y. Wang, S. Liu and W. Yu, Macromol. Biosci., 2021, 21, e2000432 CrossRef PubMed.
- D. R. Ningrum, W. Hanif, D. F. Mardhian and L. Asri, Polymers, 2023, 15, 468 CrossRef CAS PubMed.
- M. Ghirardello, J. Ramos-Soriano and M. C. Galan, Nanomaterials, 2021, 11, 1877 CrossRef CAS PubMed.
- C. Mou, X. Wang, J. Teng, Z. Xie and M. Zheng, J. Nanobiotechnol., 2022, 20, 368 CrossRef CAS PubMed.
- Y. Liang, X. Zhao, T. Hu, Y. Han and B. Guo, J. Colloid Interface Sci., 2019, 556, 514–528 CrossRef CAS PubMed.
- Y. Chen, Y. Gao, Y. Chen, L. Liu, A. Mo and Q. Peng, J. Controlled Release, 2020, 328, 251–262 CrossRef CAS PubMed.
- M. Kolarikova, B. Hosikova and H. Dilenko,
et al.
, Med. Res. Rev., 2023, 43, 717–774 CrossRef CAS PubMed.
- T. Du, Z. Xiao and G. Zhang,
et al.
, Acta Biomater., 2023, 161, 112–133 CrossRef CAS PubMed.
- K. Peng, L. Zheng, T. Zhou, C. Zhang and H. Li, Acta Biomater., 2022, 137, 20–43 CrossRef CAS PubMed.
- T. Fang, X. Chen and C. Yang,
et al.
, J. Colloid Interface Sci., 2022, 621, 205–212 CrossRef CAS PubMed.
- D. Anugrah, K. Ramesh, M. Kim, K. Hyun and K. T. Lim, Carbohydr. Polym., 2019, 223, 115070 CrossRef CAS PubMed.
- Z. Y. Li, X. J. Zhang and Y. M. Gao,
et al.
, Adv. Healthc. Mater., 2023, 12, e2202770 CrossRef PubMed.
- Z. Han, M. Yuan and L. Liu,
et al.
, Nanoscale Horiz., 2023, 8, 422–440 RSC.
- L. A. Wallace, L. Gwynne and T. Jenkins, Ther. Delivery, 2019, 10, 719–735 CrossRef CAS PubMed.
- X. Wang, M. Shan and S. Zhang,
et al.
, Adv. Sci., 2022, 9, e2104843 CrossRef PubMed.
- Z. Zhang, J. Wang and Y. Luo,
et al.
, J. Mater. Chem. B, 2023, 11, 9300–9310 RSC.
- Q. He, D. Liu, M. Ashokkumar, X. Ye, T. Z. Jin and M. Guo, Ultrason. Sonochem., 2021, 73, 105509 CrossRef CAS PubMed.
- F. Zhang, Y. Kang and L. Feng,
et al.
, Mater. Horiz., 2023, 10, 5474–5483 RSC.
- D. Li, X. An and Y. Mu, Chem. Phys. Lipids, 2019, 223, 104783 CrossRef CAS PubMed.
- H. Zhao, J. Huang and Y. Li,
et al.
, Biomaterials, 2020, 258, 120286 CrossRef CAS PubMed.
- P. C. McCarthy, Y. Zhang and F. Abebe, Molecules, 2021, 26, 4735 CrossRef CAS PubMed.
- K. Zha, Y. Xiong and W. Zhang,
et al.
, ACS Nano, 2023, 17, 17199–17216 CrossRef CAS PubMed.
- W. Zhou, Z. Duan, J. Zhao, R. Fu, C. Zhu and D. Fan, Bioact. Mater., 2022, 17, 1–17 CAS.
- Y. Chen, X. Wang and S. Tao,
et al.
, Mil. Med. Res., 2023, 10, 37 Search PubMed.
- S. Hu, Y. Zhi, S. Shan and Y. Ni, Carbohydr. Polym., 2022, 275, 118741 CrossRef CAS PubMed.
- A. Kasiński, M. Zielińska-Pisklak, E. Oledzka and M. Sobczak, Int. J. Nanomed., 2020, 15, 4541–4572 CrossRef PubMed.
- X. Yang, C. Zhang, D. Deng, Y. Gu, H. Wang and Q. Zhong, Small, 2022, 18, e2104368 CrossRef PubMed.
- M. Mu, X. Li, A. Tong and G. Guo, Expert Opin. Drug Delivery, 2019, 16, 239–250 CrossRef CAS PubMed.
- J. García-Torres, S. Colombi, L. P. Macor and C. Alemán, Int. J. Biol. Macromol., 2022, 219, 312–332 CrossRef PubMed.
|
This journal is © The Royal Society of Chemistry 2024 |