DOI:
10.1039/D3TB01674A
(Perspective)
J. Mater. Chem. B, 2023,
11, 10761-10777
Injectable organo-hydrogels influenced by click chemistry as a paramount stratagem in the conveyor belt of pharmaceutical revolution
Received
25th July 2023
, Accepted 22nd September 2023
First published on 9th October 2023
Abstract
The field of injectable hydrogels has demonstrated a paramount headway in the myriad of biomedical applications and paved a path toward clinical advancements. The innate superiority of hydrogels emerging from organic constitution has exhibited dominance in overcoming the bottlenecks associated with inorganic-based hydrogels in the biological milieu. Inorganic hydrogels demonstrate various disadvantages, including limited biocompatibility, degradability, a cumbersome synthesis process, high cost, and ecotoxicity. The excellent biocompatibility, eco-friendliness, and manufacturing convenience of organo-hydrogels have demonstrated to be promising in therapizing biomedical complexities with low toxicity and augmented bioavailability. This report manifests the realization of biomimetic organo-hydrogels with the development of bioresponsive and self-healing injectable organo-hydrogels in the emerging pharmaceutical revolution. Furthermore, the influence of click chemistry in this regime as a backbone in the pharmaceutical conveyor belt has been suggested to scale up production. Moreover, we propose an avant-garde design stratagem of developing a hyaluronic acid (HA)-based injectable organo-hydrogel via click chemistry to be realized for its pharmaceutical edge. Ultimately, injectable organo-hydrogels that materialize from academia or industry are required to follow the standard set of rules established by global governing bodies, which has been delineated to comprehend their marketability. Thence, this perspective narrates the development of injectable organo-hydrogels via click chemistry as a prospective elixir to have in the arsenal of pharmaceuticals.
1. Introduction
The development of injectable organo-hydrogels has marked an important stride in the clinical advancement of the field of materiobiology to act as an attribute in other domains such as regenerative medicine, therapeutics, and vehicles of therapies. Organo-hydrogels are unique biomimetic materials designed by either chemically or physically crosslinking polymeric frameworks derived from natural as well as synthetic sources. These hydrogels possess a highly hydrophilic nature due to their functional moieties leading to extensive water abundance, which complements their biomedical applicability along with other paramount characteristics, viz., porosity, softness, biocompatibility, flexibility, and permeability as well as absorptive activities.1,2 These attributes contribute to their biomimeticity, which broadens the horizon of the pharmaceutical industry. Consequently, the strategy of hydrogel preparation modifies the innate characteristics of hydrogels, which thereby influences their clinical applicability in drug delivery, bioimaging, tissue engineering, cancer therapy, gene delivery, wound dressing/healing and so on.3–22 Along these lines, physically engineered organo-hydrogels are developed by reversible physical interactions and their solubility can be tuned according to ambient conditions.4 Contrastingly, chemically prepared gels are more stable in physiological environments due to covalent bonds formed by means of chemical reactions, such as Schiff base reactions, click chemistry, enzyme-mediation, Diels–Alder reactions, thiol exchange/disulfide crosslinking, and Michael-type addition.23,24 Importantly, there are high chances of lixiviation of unreacted residual monomers post synthesis, and hence a further purification stage needs to be carried out.25
Contemporarily, injectable hydrogels have demonstrated to be a promising strategy in lieu of pre-developed hydrogels as they not only possess the characteristics of archetypal hydrogels but can also be introduced into human beings with nominal invasiveness to target sites of treatment, even those of irregular morphologies.26 Organo-hydrogels originating from organic constituents, viz., cellulose, agar, chitosan, polyethylene glycol (PEG), polyvinylpyrrolidone (PVP), polyacrylamide (PAAm), etc., and classified as natural or synthetic polymers are a highly biocompatible class of injectable hydrogels. Injectable organo-hydrogels can be conceptualized as simple liquid injections that gelate in situ to form a solid hydrogel when introduced into the human system.27 As a result, they have been integrated in the delivery of small and bioactive molecules to administer inflammations, cancers, and other infection-prone conditions as well as scaffolds for reparation and regeneration of hard and soft tissues.28 Recently, new additions have been introduced to the field of injectable hydrogels possessing self-healing, bioresponsive and shear-thinning features, which can be directly administered to patients in their gel phase.26,29–31 Injectable organo-hydrogels should possess certain hallmarks, which include the capacity to undergo gelation quickly, allowing for easy transportation of the pre-gel or sol to ensure the appropriate injectability at the administration site. Additionally, the hydrogel should be able to fill voids or vacancies effectively and maintain stability.23 Recently click-chemistry-based hydrogels have been gaining considerable interest due to their designability as per desire, versatility and simplicity of synthesis. Click chemistry refers to reactions that are selective and occur spontaneously with appreciably fast rates and high yields. Click-chemistry-based hydrogels are formed by spontaneous mixing of required compounds and can offer numerous functionalities to be used for biomedical as well as other applications. Such hydrogels can also encapsulate live cells and exhibit long-term viability.32 Click-chemistry-based hydrogels have also demonstrated 3D printing properties and can produce 3D tissue constructs.33 Thus, hydrogels bear tremendous potential to be used in regenerative medicine.
In a pool of contemporarily fascinating biomaterials, the rationale of this perspective manifests the clinical translation of biomimetic injectable organo-hydrogels with the development of bioresponsive and self-healing injectable organo-hydrogels in the emerging pharmaceutical revolution. In order to satisfy the ameliorating demand for novel drugs, we suggest click chemistry complimented by bio-orthogonal chemistry to lucratively influence upscaling of the production of clinically viable injectable organo-hydrogels. Likewise, we conceptualize an innovative design technique for developing a hyaluronic acid (HA)-based injectable hydrogel to be soon realised for its pharmaceutical precedence. Correspondingly, the marketability of injectable organo-hydrogels has been explained from the regulatory implications established by governing bodies such as the Food and Drug Administration (FDA) and European Medical Agency (EMA). Eventually, we summarize the potential outcomes of injectable organo-hydrogels as a pharmaceutical saviour in a cornucopia of medical events.
2. Clinical promise of injectable organo-hydrogels
The scope of clinical translation of injectable organo-hydrogels has attracted the attention of various researchers in academia and industry with a focus on pharmaceuticals and materiobiology. Thenceforth, exploration of this avenue has splurged over the years to improve the current strategies of treatments using advancing techniques of implementing hydrogels of organic composition in tissue regeneration, cancer therapy, and dental vis-à-vis maxillofacial remedies. Table 1 summarizes the benefits of implementing injectable organo-hydrogels in various biomedical applications.
Table 1 Clinical progress of injectable organo-hydrogels for various indications
Organo-hydrogel |
Origin |
Composition |
Preparation |
Mode of Injection |
Indication |
Clinical Status (https://clinicaltrials.gov) |
Ref. |
n/d – not defined. |
EUFLEXXA® (Ferring Pharmaceuticals Inc.) |
Natural |
Hyaluronic acid (HA) |
Physical interaction |
Intra-articular |
OA |
FDA (2003) |
34
|
EMA (2005) |
INFUSE® bone graft (Medtronic Sofamor Danek USA, Inc.) |
Natural |
Collagen and recombinant human bone morphogenetic protein-2 |
Physical interaction |
Spinal |
Spinal fusion, orthopaedic trauma along with spine and oral-maxillofacial surgeries |
FDA (2002) for spinal fusion |
35
|
Osteogenic protein 1 (OP-1®) implant, OP-1® Putty (Stryker Biotech) |
Natural |
Collagen, carboxymethylcellulose, and recombinant OP-1 |
Physical interaction |
Spinal |
Posterolateral lumbar spinal fusion |
FDA (2001) |
36
|
TraceIT® Hydrogel Tissue Marker (Augmenix, Inc.) |
Synthetic |
Polyethylene glycol (PEG) |
Chemical reaction |
Percutaneous |
To radiographically label soft tissue during or for subsequent surgical operations |
FDA (2013) |
37
|
Bulkamid® hydrogel (Searchlight Pharma) |
Synthetic |
Polyacrylamide |
Chemical reaction |
Transurethral |
Stress urinary incontinence in females |
FDA (2006) |
38 and 39
|
EMA (2003) |
Coaptite® (BioForm Medical, Inc.) |
Synthetic |
Calcium hydroxylapatite, sodium carboxymethylcellulose, glycerin |
Physical interaction |
Submucosal |
Female stress urinary incontinence |
FDA (2005) |
40
|
EMA (2001) |
Algisyl-LVR® Hydrogel Implant (LoneStar Heart, Inc.) |
Natural |
Alginate |
Physical interaction |
Percutaneous |
Advanced heart failure |
EMA (2014) |
41–45
|
Aquamid (Henning Bliddal) |
Synthetic |
Polyacrylamide |
Chemical reaction |
Intra-articular |
Knee OA |
Clinical trials |
NCT03060421 (NA) |
PAAG-OA (Contura) |
Synthetic |
Polyacrylamide |
Chemical reaction |
Intra-articular |
Knee OA |
Clinical trials |
NCT04045431 (NA) |
Aquamid (A2 Reumatologi Og Idrætsmedicin) |
Synthetic |
Polyacrylamide |
Chemical reaction |
Intra-articular |
Knee OA |
Clinical trials |
NCT03067090 (NA) |
Hymovis Viscoelastic Hydrogel (Fidia Farmaceutici s.p.a.) |
Natural |
High molecular weight hyaluronan |
Physical interaction |
Intra-articular |
OA |
Clinical trials (Phase III) |
NCT01372475 |
HYADD® 4 Hydrogel (Fidia Farmaceutici s.p.a.) |
Natural |
Non-crosslinked hyaluronic acid alkylamide |
Physical interaction |
Intra-articular |
Knee OA |
Clinical trials |
NCT02187549 (NA) |
Promedon |
Natural |
Hydroxyethyl cellulose |
Physical interaction |
Knee |
OA |
Clinical trials |
NCT04061733 (NA) |
Naofumi Takehara |
Natural |
Gelatin with basic fibroblast growth factor |
n/d |
Intra-myocardial |
Ischemic cardiomyopathy |
Clinical trials (phase I) |
NCT00981006 |
VentriGel (Ventrix, Inc.) |
Natural |
Native myocardial extracellular matrix |
Physical interaction |
Trans-endocardially |
Myocardial infarction |
Clinical trials |
NCT02305602 |
Neo-kidney augment (inRegen) |
Natural |
Gelatin with selected renal cells |
Chemical reaction |
Kidneys |
Type 2 diabetes and chronic kidney disease |
Clinical trials (phase II) |
NCT02525263 |
Renal autologous cell therapy (inRegen) |
Natural |
Gelatin with renal autologous cells |
Chemical reaction |
Renal cortex |
Chronic kidney disease from congenital anomalies of the kidneys and urinary tract |
Clinical trials (phase I) |
NCT04115345 |
GelStix® Nucleus augmentation device (Dr med. Paolo Maino Viceprimario Anestesiologia) |
Synthetic |
Polyacrylonitrile |
Chemical reaction |
Intra-discal |
DDD |
Clinical trials |
NCT02763956 |
The Second Affiliated Hospital of Chongqing Medical University |
Natural |
Unknown/human amniotic epithelial cells |
n/d |
Uterine cavity |
Asherman's syndrome |
Clinical trials (phase I) |
NCT03223454 |
Vantas® (Endo Pharmaceuticals) |
Synthetic |
Histrelin acetate, poly(2-hydroxyethyl methacrylate), poly(2-hydroxypropyl methacrylate) and gonadotropin releasing hormone |
Chemical reaction |
Subcutaneous |
Palliative treatment of prostate cancer |
FDA (2004) |
46
|
EMA (2005) |
SpaceOAR® Hydrogel (Augmenix, Inc.) |
Synthetic |
PEG |
Chemical reaction |
Percutaneous |
To shield exposed tissues during prostate cancer radiotherapy |
FDA (2015) |
47 and 48
|
EMA (2010) |
Memorial Sloan Kettering Cancer Center |
Synthetic |
PEG |
Chemical reaction |
Visceral pleura |
Lung biopsy |
Clinical trials (phase III) |
NCT02224924 |
Augmenix, Inc. |
Synthetic |
PEG/SpaceOAR® |
Chemical reaction |
Between the rectum and prostate |
Spacing in radiation therapy for prostate cancer |
Clinical trials (phase III) |
NCT01538628 |
University of Washington |
Synthetic |
PEG/TraceIT® |
Chemical reaction |
Near the circumference of tumour bed |
Imaging of bladder carcinoma |
Clinical trials |
NCT03125226 |
Absorbable Radiopaque Hydrogel Spacer (Thomas Zilli, University Hospital, Geneva) |
Synthetic |
PEG/TraceIT® |
Chemical reaction |
Between the prostate/vagina and the rectum |
Spacing in radiation therapy for rectal cancer |
Clinical trials |
NCT03258541 |
Royal North Shore Hospital |
Synthetic |
PEG/SpaceOAR® |
Chemical reaction |
Between the rectum and prostate |
Spacing in radiation therapy for prostate cancer |
Clinical trials |
NCT02212548 |
Absorbable Radiopaque Tissue Marker (Washington University School of Medicine) |
Synthetic |
PEG/TraceIT® |
Chemical reaction |
Resection bed |
Imaging of oropharyngeal cancer |
Clinical trials (phase I) |
NCT03713021 |
Absorbable Radiopaque Tissue Marker (Sidney Kimmel Comprehensive Cancer Center at Johns Hopkins) |
Synthetic |
PEG/TraceIT® |
Chemical reaction |
Between the pancreas and duodenum |
Imaging of pancreatic adenocarcinoma |
Clinical trials |
NCT03307564 |
Gut Guarding Gel (National Cheng-Kung University Hospital) |
Natural |
Sodium alginate/calcium lactate |
Physical interaction |
Submucosal |
Gastroenterological tumor and polyps |
Clinical trials |
NCT03321396 |
Radiesse® (Bioform Medical, Inc.) |
Synthetic |
Hydroxylapatite, carboxymethylcellulose |
Physical interaction |
Dermis |
Abet with the correction of facial folds and wrinkles |
FDA (2006) |
49
|
EMA (2004) |
Radiesse® (+) (Merz Pharmaceuticals) |
Synthetic |
Hydroxylapatite, carboxymethylcellulose with lidocaine |
Physical interaction |
Dermis |
For the purpose of stimulating natural collagen production along with treating wrinkles and facial folds |
FDA (2015) |
50
|
Bellafill® (Suneva Medical, Inc.) |
Synthetic |
Polymethylmethacrylate beads, collagen and lidocaine |
Physical interaction |
Dermis |
Facial wrinkles and folds |
FDA (2006) |
51
|
Sculptra® (Sanofi Aventis U.S.) |
Synthetic |
Poly-L-lactic acid |
Physical interaction |
Dermis |
For the purpose of correcting facial fat and shallow to deep nasolabial contour deficiencies in addition to treating wrinkles |
FDA (2004) |
52
|
EMA (2000) |
Fibrel® (Serono Laboratories) |
Natural |
Collagen |
Physical interaction |
Dermis |
Correction of depressed cutaneous scars |
FDA (1988) |
53
|
Zyplast(R)® and Zyderm(R)® (Inamed Corporation/Allergan, Inc.) |
Natural |
Bovine collagen |
Chemical reaction |
Dermis |
Correction of contour deficiencies |
FDA & EMA (1981) |
54
|
Restylane® Lyft, Restylane® Refyne, Restylane® Defyne (Galderma Laboratories, L.P.) |
Natural |
Hyaluronic acid with lidocaine |
Chemical reaction |
Subcutaneous, dermis, lips |
For treating conditions such as volume deficit, facial folds, wrinkles, midface contour deficiencies and perioral rhytids |
FDA (2012) |
55
|
EMA (2010) |
Belotero balance® (+) Lidocaine (Merz Pharmaceuticals) |
Natural |
Hyaluronic acid with lidocaine |
Chemical reaction |
Dermis |
Moderate to severe facial wrinkles and folds |
FDA (2019) |
56
|
Revanesse® Versa+ |
Natural |
Hyaluronic acid with lidocaine |
Chemical reaction |
Dermis |
Moderate to severe facial wrinkles and creases |
FDA (2018) |
57
|
Hylaform® (Hylan B gel), Captique Injectable Gel, Prevelle Silk (Genzyme Biosurgery) |
Natural |
Modified hyaluronic acid derived from a bird (avian) source |
Chemical reaction |
Dermis |
Correction of moderate to severe facial wrinkles and folds |
FDA (2004) |
58
|
EMA (1995) |
Teosyal® RHA (Teoxane SA) |
Natural |
Hyaluronic acid |
Chemical reaction |
Dermis |
Facial wrinkles and folds |
FDA (2017) |
59
|
EMA (2015) |
3. Neoteric breakthroughs of organo-hydrogels having biomedical implications
In this section, nascent advances in injectable organo-hydrogels, which have provided new horizons in solving biomedical predicaments, have been highlighted to display the salience and pharmaceutical promise of this biomimetic system from the pool of archetypal pharmaceutical agents and biomaterials. Herein, we aim to promote the notion of bioresponsive and self-healing injectable organo-hydrogels targeted for drug delivery, wound healing, and other means of tissue engineering and regenerative medicine to be predominantly employed in the coming pharmaceutical revolution.
3.1 Bioresponsive organo-hydrogels
Bioresponsive organo-hydrogels or synonymously referred to as stimuli-responsive organo-hydrogels are one such kind of new-age injectable gels predominantly explored in delivery systems of small and bioactive molecules. The benefit of implementing bioresponsive organo-hydrogels is the effective drug loading and controlled release of imperative compounds to the human systems without delay. In this section, we highlight the remarkable results of injectable bioresponsive organo-hydrogels as delivery vehicles and their application in regenerative medicine.
3.1.1 Delivery vehicles.
Injectable organo-hydrogels are being exploited as delivery vehicles for targeted delivery of drugs, proteins, genes, and other bioactive compounds and have been strongly established at the lab-scale. Extensive in vivo investigations validate the immaculate delivery performance of injectable organo-hydrogels derived from synthetic as well as natural constituents. Sustained drug delivery is of immense importance, especially in cases where a longer retention time of drugs is required, which in turn reduces the incidence of administering multiple maintenance doses of the same drug.60 Continuing on the same path, a biodegradable and thermally-responsive lysozyme-loaded hydrogel composed of N-isopropylacrylamide (NIPAAm), methacrylate-polylactide (MAPLA), and 2-hydroxyethyl methacrylate (HEMA) was fabricated as a means for protein delivery. When injected into the back of Sprague-Dawley rats, these systems underwent sol-to-gel transition, following which they released lysozyme in a sustained manner, thus avoiding the initial burst effect, which is quite common among conventional drug delivery systems. Moreover, these systems were also found to be non-toxic to fibroblasts, even at higher concentrations.61 Furthermore, to expedite the rate of diabetic wound healing, Lee et al. synthesised a hydrogel through the crosslinking of PEF–PLGA–PEG which was further utilised for the delivery of TGF-β 1 plasmid. In addition to providing sustained delivery of the plasmid, the hydrogel scaffold also exhibited the ability to accelerate the process of re-epithelialization, promote more organised extracellular matrix deposition and cell proliferation, and stimulate collagen production in a diabetic mouse model.62 A silk-elastin like protein polymer (SELP) hydrogel was synthesised as a carrier to deliver plasmid gene and the pRL-CMV vector released the plasmid effectively in a size dependent manner. In comparison to naked DNA, delivery of pRL-CMV from a SELP hydrogel significantly ameliorated transfection in a murine model of human breast cancer by 1–3 orders of magnitude. The concentration of the polymer in the hydrogel was found to be correlated with the release of a bioactive adenoviral vector.63
An intricately engineered bioresponsive hydrogel scaffold composing triglycerol monostearate was loaded with Rosiglitazone (Rosi-hydrogel), as shown in Fig. 1(A).64 It was observed that this hydrogel system could treat age-related meibomian gland dysfunction (ARGMD) in a mouse model, thus proving as an effective treatment to alleviate inflammation of the delicate eyelids, thus indicating its potential as a controlled release drug delivery system. Lee et al. synthesized a thermosensitive elastin-based polypeptide (EBP) and ligand-responsive calmodulin (CalM) injectable hydrogel as EBP–CalM–EBP (ECE) triblock copolypeptides for the purpose of controlled drug delivery. In similar circumstances, concentrated ECE triblock copolypeptides showed thermally induced gelation, leading to physically crosslinked hydrogels. Depending on the conformational change of the CalM middle block brought about by binding either Ca2+ or Ca2+ and trifluoperazines (TFPs) as ligands, they displayed controlled rheological and mechanical properties.65 Turabee et al. aimed at delivering protein molecules without deteriorating their inherent bioactivity through fabrication of a temperature- and pH-responsive bioresorbable injectable hydrogel system composed of polypeptide block copolymers, namely polyethylene glycol (PEG), temperature-responsive poly(γ-benzyl-L-glutamate) (PBLG), and pH-responsive oligo(sulfamethazine) (OSM), for the sustained delivery of proteins in vivo. The anionic properties of the scaffold allowed for the efficient loading of the model protein-lysozyme and when injected into the Sprague-Dawley rats subcutaneously, it was observed that the lysozyme loaded block copolymer formed a viscoelastic gel in vivo which could deliver the model protein in a sustained manner for at least a week.66
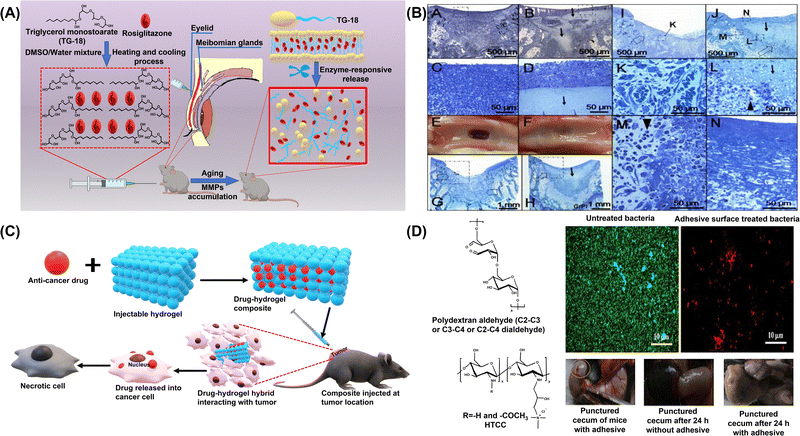 |
| Fig. 1 (A) Schematic representation of hydrogel formation and Rosi release for treating ARMGD. Reproduced from ref. 64. Copyright 2021, Elsevier. (B) In vivo delivery, retention of chitosan gel, and inflammatory response in example ∼3 mm deep osteochondral defects in 4-month-old NZW rabbits. Osteochondral holes 3 mm in diameter and ∼3 mm deep were drilled into the femoral-patellar groove (A–N) with one control defect allowed to bleed (A, C, E, G, I, K) and the other defect loaded with chitosan gel (B, D, F, H, J, L–N). After 1 day (A–D) or 8 days (E–N) of normal load-bearing, the joints were recovered and processed for histology. Reproduced from ref. 69. Copyright 2005, Elsevier. (C) Application of injectable organo-hydrogels in delivery of anti-cancer drugs. (D) Schematic illustration and in vivo wound healing activity of the injectable HTCC organo-hydrogel. Reproduced with permission.70 Copyright 2017, American Chemical Society. | |
As a long-term controlled release system, injectable hydrogels exhibit exceptional capacity to encapsulate drugs and biomolecules. For instance, injectable dopamine-based polydopamine hydrogels were fabricated through the crosslinking of a mixture of quaternised chitosan, gelatin and dopamine. These hydrogels have enabled sustained and effective treatment for Parkinson's disease. The release profiles of the drug loaded hydrogel scaffolds indicated their suitability as potential drug carriers for the enduring management of Parkinson's disease.67 Zubik et al. focussed on assembling thermo-responsive hydrogels composed of poly(N-isopropylacrylamide) (PNIPAAm) braced up with cellulose nanocrystals through covalent or non-covalent linkages. To test their application as drug-delivery vehicles, metronidazole, a drug used in wound dressing, was loaded into the system and it could be corroborated that the hydrogel scaffold had efficient drug-delivering capability as it exhibited an initial burst release of the drug at the beginning, following which the drug release occurred in a gradual and sustained manner at 37 °C.68 Furthermore, the loading and targeted in vivo delivery of anti-cancer compounds is schematically described in Fig. 1(C).
3.1.2 Regenerative medicine.
In order to overcome palliative measures, materiobiologists have found it imperative to develop novel strategies for managing organ damage with successful transplantation or replacement. Many issues like those of graft rejection make it quite difficult for such surgeries to become successful and thus more efficient substitutes are of pressing need. For instance, an injectable temperature-responsive chitosan–beta glycerophosphate–hydroxyethyl cellulose (CH–GP–HEC) hydrogel was synthesized which was further encapsulated with mesenchymal cells (MSCs) to resolve defects related to cartilage tissue with minimal pain and invasion. When investigated for regenerative potential, it was found that the hydrogel scaffolds provided a suitable and optimum environment for human MSCs to differentiate.71 In another study, cartilage tissue engineering was effectuated using hydrogels such as PuraMatrix, alginate or atelopeptide collagen embedded with human auricular chondrocytes. When investigated for regenerative medicine, it was found that the hydrogel scaffold system could deliver the human auricular chondrocytes quite effectively.72 Moving further, an experimental design focussed on the fabrication of a chitosan-based hydrogel for the purpose of cartilage formation in vivo as well as in vitro. For the same, the newly synthesized hydrogel system was loaded with primary articular chondrocytes. It was found that, in such a manner, the hydrogel scaffold could treat chondral defects effectively, when tested in a rabbit chondral defect model while also leading to the accumulation of the cartilage matrix via chondrocytes, as presented in Fig. 1(B).69 Another experimental design focussed on hyaline cartilage repair in ovine microfracture defects by assembling and injecting chitosan–glycerol/blood implants. Upon histological examination of sheep, it was found that at 6 months the best hyaline tissue repair was associated with the implant while also showing aggravated levels of collagen and cells in the defects treated with our scaffold.73 Furthermore, an injectable hydrogel system was fabricated using an antibacterial polymer, N-(2-hydroxypropyl)-3-trimethylammonium chitosan chloride (HTCC), and a bioadhesive polymer, polydextran aldehyde, as shown in Fig. 1(D). When tested for its wound healing activity in the treatment group of Wistar rats, the adhesive hydrogel could treat the wounds effectively, especially after days 15 and 20.70
3.2 Self-healing organo-hydrogels
Interestingly, in the wide arena of hydrogels, self-healing hydrogel systems emerged as scaffolds, capable of restoring the morphological traits and mechanical traits following recurrent damage.74 The superior durability and stability of self-healing hydrogels allow them to reform their shape, injectability, and stretchability, thus acceding them to repair themselves autonomously when ruptured or traumatised.75 Self-healing hydrogels regenerate spontaneously after mechanical damage via dynamic covalent cross-linkages and covalent and non-covalent interactions.76 The hydrogel heals damages and reforms shapes due to the dynamic equilibrium between dissociation and recombination of various interactions. Dynamic covalent bonds typically have stable and slow dynamic equilibriums, whereas non-covalent interactions have fragile and fast dynamic equilibriums.77 In recent years, the dynamic properties of injectable organo-hydrogel systems have intrigued researchers to broaden their horizons in terms of establishing these scaffolds as those with superior and more effective biomedical applications such as wound healing,78 tissue repair and engineering,30 3D printing,79 and as drug delivery vehicles.80
3.2.1 Self-healing hydrogels for wound management.
The scope of injectable organo-hydrogels has been established to treat various kinds of wounds and multiple studies have shown how these systems can serve as better alternatives to conventional wound-healing treatments. For instance, an injectable, self-healing and antibacterial polypeptide-based FHE hydrogel (F127/OHA-EPL) was fabricated to release exosomes derived from adipose-derived mesenchymal stem cells (AMSCs-exo) upon responding to stimuli so as to facilitate synergistic healing of chronic wounds and skin regeneration. In in vivo culture, it was found that the multifunctional organo-hydrogel so formed effectuated faster wound closure rates, re-epithelialisation and angiogenesis along with redeposition of collagen on the site of diabetic wounds, as compared to the FHE hydrogel and exosomes alone.84 H. Xuan in another study synthesised an avant-garde injectable hydrogel system which was forged using Schiff's base reaction to link carboxymethyl chitosan and aldehyde functionalised sodium alginate along with the introduction of short nanofibers of carboxymethyl-functionalized polymethyl methacrylate (PMAA). The excellent self-healing activity of the system was attributed to the nanofibers. Further, when tested in rats, the synthesised hydrogel scaffolds displayed a faster rate of wound closure along with effective re-epithelialization and angiogenesis was observed which facilitated in accelerated wound closure rates.85 Furthermore, another study involved the fabrication of adhesive haemostatic antioxidant conductive photothermal antibacterial injectable nanocomposite hydrogel composed of a graft of hyaluronic acid and dopamine reduced graphene oxide for the purpose of wound dressing. The results showed that the synthesised hydrogel system surpassed the human skin in terms of mechanical properties while also augmenting the vascularization and collagen deposition, and improving the granulation tissue thickness, all of which contributed to the escalated rates of wound closure in rats. Further, their adhesive nature also contributed to a longer stay time over the wounds.86 Another study involved the modelling of injectable self-healing hydrogels for maintaining gastric homeostasis and promotion of wound healing. This hydrogel system was endowed with adhesive and pH-responsive qualities too. The hydrogel was composed of acryloyl-6-aminocaproic acid (AA) and AA-g-N-hydroxysuccinimide (AA-NHS) which enhanced the adhesive properties of the hydrogel, which is presented in Fig. 2(A). In addition, this hydrogel exhibited excellent self-healing and homeostatic properties, while from the results obtained in a gastric wound model, it could be concluded that this hydrogel scaffold effectuated enhanced gastric wound healing properties through deposition of type 1 collagen, revascularization and angiogenesis as well, which are crucial for proper would closure.81 Interestingly, another self-healing, injectable, platelet-rich plasma hydrogel was assembled based on chitosan, silk fibroin, and PRP (CBPGCTS-SF@PRP) for the purpose of treating diabetic wounds. The hydrogel showed promising results as it successfully led to the chemotactic migration of mesenchymal cells, protected PRP from rigorous enzyme hydrolysis and promoted cell proliferation, and when tested in a type 2 diabetic rat model, it effectuated heightened levels of collagen deposition, nerve repair and augmented rates of angiogenesis, thus proving to be a promising candidate in the nexus of wound healing.87
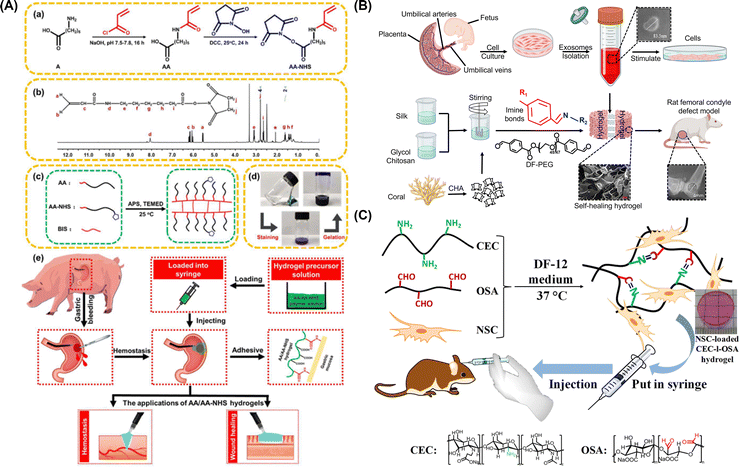 |
| Fig. 2 (A) Synthesis of AA and AA-NHS ester copolymers (a), 1H NMR spectrum of AA-NHS (b), preparation and network of the AA/AA-NHS hydrogel (c), optical images of AA/AA-NHS hydrogel polymer solution pre- and post-crosslinking and subsequent staining of solution with crystal violet (d), and gastric haemostasis and wound healing activities of AA/AA-NHS hydrogels (e). Reproduced from ref. 81. Copyright 2020, Springer Nature. (B) Schematic depiction of isolation and characterization of hucMSC-derived exosomes and creation of the CHA/SF/GCS/DF-PEG organo-hydrogel to be injected in Sprague-Dawley (SD) rats with induced femoral condyle defects. Reproduced from ref. 82. Copyright 2020, Frontiers. (C) Synthesis and transplantation strategy of injectable NSC-loaded, self-healing CEC-l-OSA hydrogels. Reproduced from ref. 83. Copyright 2016, Springer Nature. | |
3.2.2 Self-healing hydrogels for tissue regeneration.
Tissue regeneration is a complex process that involves re-epithelialization, cell proliferation and extensive tissue repair. One of the most persistent problems regarding conventional regenerative medicine is immune rejection.88 To tackle this issue and other therapy related side effects as well as serious repercussions, injectable organo-hydrogels can be considered as a promising avenue for successful regeneration of the tissue. Various studies have been conducted to establish these scaffolds as successful candidates for tissue regeneration. For instance, to aid effective bone regeneration, a self-healing hydrogel composed of a human umbilical cord mesenchymal stem cell (hucMSC)-derived exosome carrier was fabricated, as depicted in Fig. 2(B). The results indicated that these scaffolds effectuated differentiation while also promoting the proliferation and migration of human umbilical vein endothelial cells. These results could be confirmed after the hydrogel system could cure a bone defect in a rat model while also exhibiting good biocompatibility.82 In another study, injectable conductive self-healing hydrogels were assembled by carrying out a Schiff's base reaction between dextran-graft-aniline tetramer-graft-4-formylbenzoic acid and N-carboxyethyl chitosan as carriers for myoblast cells, which were further used for muscle regeneration. The results indicated that the synthesized hydrogel scaffolds had good biocompatibility while also leading to muscle regeneration by effectuating successful release of myoblasts from the hydrogel system into the tissue microenvironment.89 In another study, self-healing hydrogels were synthesised using adamantine- and cyclodextrin-modified HA, encapsulated with endothelial progenitor cells (EPCs) which was then injected into the ischemic myocardium. The results were observed in a rodent model of acute myocardial infarction and it was found that an increase in vasculogenesis was observed compared to treatment with EPCs or the hydrogel alone.90,91
3.2.3 Delivery vehicles.
Since conventional systems of drug delivery have a myriad of drawbacks and repercussions associated with them, the organ-hydrogel scaffold system can be considered and established as a substitute to deliver drugs and biomolecules more effectively inside the body. For instance, chitosan–PEG based hydrogels were synthesized with the aim of making them anti-inflammatory, anti-bacterial and applicable in drug delivery and wound healing of ulcers.92 An experimental study reported the fabrication of an injectable self-healing hydrogel composed of N-carboxyethyl chitosan and oxidised sodium alginate for the delivery of neural stem cells, as illustrated in Fig. 2(C). With a marked expression of the neuronal markers nestin and b-III tubulin but not the glial marker GFAP, the hydrogel supported the homing, proliferation (up to 5 days), and differentiation of the encapsulated NSCs, suggesting the promotion of NSC differentiation into neurons rather than glial cells. One week after injection, NSC-loaded hydrogels completely filled the lesion site with cells evenly distributed throughout the hydrogel in mice with focal cerebral ischemia.83 Similarly, the delivery of optogenetics (genetically targeted neurons expressing light-responsive opsin molecules) was realised by co-injecting bacteriorhodopsin (HEBR) plasmid in conjugation with a chitosan-based self-healing hydrogel with strong shear-thinning properties as a potential temporal–spatial remedy against neurodegeneration.93 Furthermore, an organo-hydrogel based on a 4-arm star PEG functionalized with vinyl sulfone and short dithiol crosslinkers was created to resemble cartilage. Chondrocytes were introduced into osteochondral defects in immunodeficient mice using the hydrogel. Following 12 weeks, there was an aggravation in proliferation, chondrogenic differentiation, and ECM deposition. The coalescence of hyaline cartilage with the surrounding cartilage allowed for cartilage repair.94
4. Pharmaceutical expansion of injectable organo-hydrogels complimented by click chemistry
Click chemistry is a novel approach gaining interest owing to its benefits including one-step reactions that are rapid, simple to perform, regiospecific involving benign solvents.95 These reactions are biorthogonal, as the azide moiety is absent in nature, and hence do not react with natural biomolecules.96 This section conceptualizes click chemistry to act as a backbone in the conveyor belt in mass production of injectable organo-hydrogels for the new-age pharmaceutical revolution. Along these lines, the current development of bio-orthogonal chemistry in this regime has also been discussed. Ultimately, we propose an avant-garde design stratagem to create a hyaluronic acid (HA)-based injectable hydrogel via click chemistry to be exploited for its biomedical advantage.
4.1 Biomedical applications of the organo-hydrogel developed via click chemistry
Click chemistry provides the much-required multifunctional hydrogel materials, which are interesting biomaterials for tissue engineering and bioprinting ink applications owing to their excellent ability to form hydrogels with printability instantly and to retain live cells in their 3D network without losing the mechanical integrity even when swollen. Cu(I)-catalyzed processes for hydrogel synthesis were quite popular and are popularly referred to as click chemistry. This was the case for all the hydrogels that were investigated.97 SP-AAC click chemistry was used to create a hydrogel that was generated from dextran that had been modified with azide and cyclooctyne components. To evaluate the efficacy of this injectable hydrogel for cartilage tissue engineering, chondrocyte cells were included both singly and in the form of spheroids. Spheroids, on the other hand, led to a greater formation of chondrocyte extracellular matrices than did individual cells.98 A biocompatible, in situ cross-linking HA-based injectable hydrogel was developed by Han et al. for cartilage regeneration. The gel was formed by modifying HA with 4-arm PEG azide and dibenzyl cyclooctyne independently to produce the desired level of high cross-linking, which was accomplished using the SP-AAC mechanism. The gel was produced because of the reaction that occurred between the azide and dibenzyl cyclooctyne groups during the copper-free click reaction. In vitro testing was conducted using a variety of different compositions to evaluate the varied necessary qualities of the injectable hydrogel. They administered the hydrogel subcutaneously to mice and monitored the change in the volume of the gel across a variety of time intervals. After performing in vivo tests on mice to regenerate cartilage, they observed the removal of white solid tissue-like hydrogels from the animals. This hydrogel technology has potential for the regeneration of cartilage in vivo, showing positive outcomes.99 Ethylenediaminetetraacetic acid (EDTA) is the chemical used to remove copper ions from hydrogels. However, it may be challenging to employ the same procedure in the context of injectable hydrogels for regenerative medicine or targeted drug administration.100 Even though scaffold-free approaches are being researched for tissue regeneration, injectable in situ hydrogels, with or without live cells, growth factors, biomolecules, nanoparticles, or microspheres, are promising candidates for tissue engineering applications with improved mechanical properties and biocompatibility.101 In general, click-chemistry-based hydrogels for tissue engineering applications are promising and have been extensively explored all over the world in recent years for a variety of biomedical applications. These applications include 3D bioprinting, drug delivery, diagnosis, tissue engineering, and regenerative medicine, among other things. Experiments should be designed to create innovative biomaterials that are suitable for certain applications, taking into consideration the numerous benefits and drawbacks associated with the many different synthesis pathways. For instance, it has been shown that thiol-based hydrogels with residual initiators might generate a slight immunogenic response at the cellular level. For this reason, extreme caution should be exercised during the hydrogel synthesis process to ensure that any potentially harmful catalysts or initiators are eliminated. Alternatively, time- and cost-saving purification methods should be utilised to obtain higher-quality products by means of click chemistry reactions. Combining the benefits obtained from various click chemistry methods or its main biomaterials to generate multifunctional and biocompatible biomaterials is essential for the development of such hydrogels with superior qualities. This is one of the most critical criteria. At the same time, the reactions should not disrupt or interfere with other processes, and it should not have any unintended side effects or cross reactions with other unanticipated reaction groups. Recently, new technologies have been proposed, such as the surface modification of living cells with ligands for crosslinking with biocompatible hydrogels. This is just one example of the tremendous expansion that is occurring in this field. Fig. 3 describes the various approaches of click reactions in the preparation of injectable organo-hydrogels and their corresponding biomedical applications.
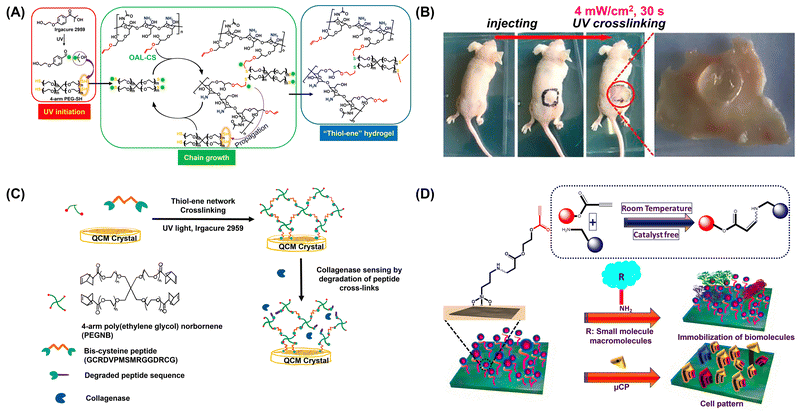 |
| Fig. 3 (A) Click reaction mechanism of organo-hydrogel preparation and (B) in situ cross-linking of the hydrogel in the mouse model within 30 s. Reproduced with permission.102 Copyright 2020, Elsevier. (C) Click chemistry preparation of the collagenase sensing organo-hydrogel. Reproduced from ref. 103. Copyright 2019, MDPI. (D) Schematic depiction of quick surface immobilization of native bioconjugates via spontaneous click chemistry. Adapted from ref. 104. | |
4.2 Biomedical applications of bio-orthogonally derived injectable organo-hydrogels
In order to characterise the reactions that occur in the presence of macromolecules such as proteins or living cells, etc., many words have been utilised. Some of these phrases are bio-orthogonal and bio-click. The fact that bio-orthogonal approaches often do not interfere with other regular biochemical processes is the primary benefit of utilising these approaches.105 Using bio-orthogonal click cross-linking processes of azide-modified mammalian cells with alkyne-modified biocompatible polymers, another group generated cell cross-linked live bulk hydrogels (bAlg-DBCO). The purpose of the research was to provide evidence that the covalent coupling of live cells, which acted as active cross-linking sites, paves the way to produce multifunctional hydrogels that possess one-of-a-kind functions that are derived from the cells themselves, as presented in Fig. 4(D). To show the usefulness of their methodology, they chose to focus on two fundamental biological functions: autonomous cell growth and selective cell adhesion. As a result of the autonomous development of cells that are used as active cross-linking sites, CxGels have the potential to both self-grow and self-degrade, and this effectively demonstrates that these are distinctive qualities of CxGels. Furthermore, the capacity of cells incorporated into CxGels to preferentially stick to surfaces is the source of the selective adhesion shown by CxGels. Therefore, they hypothesise that the selective adhesive ability of CxGels is unparalleled in comparison to that of hydrogels currently on the market. Together, they have successfully demonstrated that the functions of cells that have been covalently incorporated into CxGels as active cross-linking points are helpful for endowing CxGels with unique functionalities.
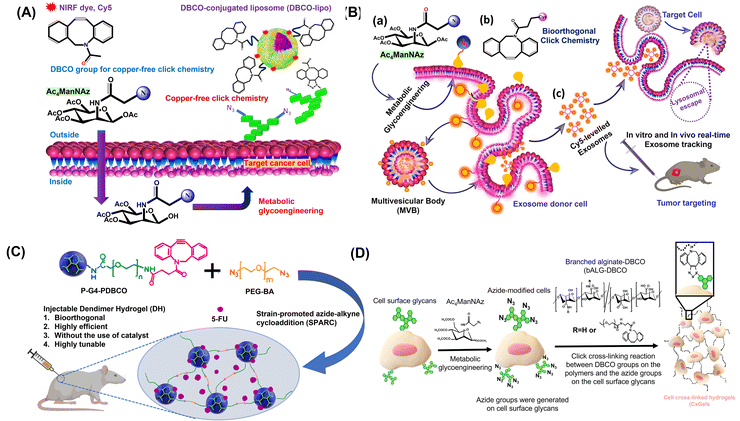 |
| Fig. 4 (A) In vivo tumour-targeting via bio-orthogonal copper-free click chemistry. Adapted from ref. 110. (B) One-step bio-orthogonal click chemistry of metabolite-treated cancer cell exosomes. Adapted from ref. 111. (C) Synthesis and localized drug delivery using the injectable bio-orthogonal dendrimer organo-hydrogel. Reproduced with permission.109 Copyright 2017, American Chemical Society. (D) Illustration of construction of cell cross-linked hydrogels (CxGels) via a bio-orthogonal click cross-linking reaction between azide-modified cells and alkyne-modified polymers. Reproduced from ref. 105. Copyright 2018, Springer Nature. | |
The ‘click’ reaction of CuAAC was utilised by Li and her colleagues in order to successfully build novel thermo-responsive polyurethane–poly(ethylene glycol) (PU–PEG) hydrogels. These hydrogels were based on azido-pendent PU–PEG and dialkynyl PEG (DAKPEG). The PU–PEG hydrogels were equipped with a number of exceptional qualities, such as thermo-responsivity, remarkable mechanical properties, and excellent bio-orthogonality, and because of this they were considered to be a promising choice for the fabrication of injectable hydrogels.106 The production of bio-orthogonal hydrogels based on PEG will likely play a significant role in the field of future biomedicine. Bio-orthogonal PEG hydrogels were produced by Hodgson and colleagues using the SPAAC ‘click’ reaction. The synthesis was based on aza-dibenzocyclooctyne (DIBAC) and azide ended polymers. The gelation duration could be adjusted from 10 to 60 seconds at room temperature, and the Young modulus of each hydrogel could range from 1 to 18 kPa by adjusting the ratio and concentration of the two precursors. Both parameters were governed by the gelation time. After that, the PEG hydrogel exhibited remarkable biocompatibility.107 A new thermosensitive and dual-crosslinking furyl-modified hydroxypropyl chitin polymer was produced by Bi et al. and it was used to fabricate injectable hydrogels. The component that was responsible for temperature sensitivity was hydroxypropyl chitin, and the component that was responsible for chemical crosslinking was a Diels–Alder ‘click’ reaction that took place between maleimide-terminated PEG and furyl-modified hydroxypropyl chitin polymer. The injectable hydrogels possessed high bio-orthogonality and outstanding biocompatibility. The encapsulated cells exhibited sustained proliferation and a remarkable capacity for producing multicellular spheroids within the injectable hydrogels. The authors believed there was a significant amount of scope for the application of hydrogels in tissue engineering.108
On the basis of bio-orthogonal chemistry, the Yang group conceived and created innovative injectable bio-orthogonal dendrimer hydrogels.109 The platform provides a great degree of capability and is also quite modular, as depicted in Fig. 4(C). To fine-tune the chemical and physical properties of dendrimer hydrogels, it is possible to readily manipulate a wide variety of structural parameters, such as the dendrimer generation, degree of PEGylation, loading density of clickable DBCO groups, PEG–BA chain length, and the ratio of the dendrimer to PEG–BA. In particular, the injectable bio-orthogonal DH that was synthesised under the conditions that were disclosed in this study displayed great cytocompatibility as well as prolonged drug release. It was utilised in the production of an injectable formulation for the anticancer medication 5-FU that included both sustained release and localised administration. The DH/5-FU formulation dramatically reduced the formation of tumours in HN12 tumor-bearing mice and increased the overall survival rate of the animals. This was accomplished by increasing the rate of cancer cell death as well as decreasing the rate of cancer cell proliferation and tumour angiogenesis.
4.3 An avant-garde design stratagem for the development of hyaluronic acid (HA)-based injectable organo-hydrogels via click chemistry
Click chemistry based injectable hydrogels obtained using hyaluronic acid (HA) and its derivatives are utilized for synthesis of novel injectable hydrogels. Hyaluronic acid-based hydrogels exhibit structural and mechanical properties mimicking the extracellular matrix (ECM).112 Owing to its properties such as biocompatibility, biodegradability, and low immunogenicity, HA is fabricated into a desirable biomaterial for regenerative medicine, tissue engineering and cosmetology.113,114 However, due to the enzymatic activity of hyaluronidase which degrades hyaluronic acid inside the body, chemical crosslinking of HA is essential.115 Viscosity, sheer thinning and self-healing are important parameters which impart injectable properties to a hydrogel.116
4.3.1 Click-chemistry-based injectable organo-hydrogels.
We propose the development of injectable hydrogels via click chemistry using hyaluronic acid (HA) and its derivatives. The synthesis of a click tetrafunctional poly(ethylene glycol) hyaluronic acid hydrogel is illustrated in Fig. 5(A), where 4-arm PEG and hyaluronic acid serve as precursors. Extensive studies have shown that 4-arm PEG imparts high strength and yields an ideal homogeneous network structure to the hydrogels.117 Furthermore, the synthesis of a hyaluronic acid–thiolated gelatin azide injectable hydrogel (Fig. 5(B)) involves the crosslinking of gelatin with cystamine, followed by click reaction with cyclooctyne–hyaluronic acid. The exceptional reactivity of cyclooctyne rings with azide functional groups allows for a rapid click reaction to occur under mild conditions, obviating the need for copper catalysts.118 Additionally, the inherent stability of the cyclooctyne ring facilitates the formation of a stable triazole ring upon reaction with the azide group. The resulting triazole linkages provide long-term stability to the modified molecules.119
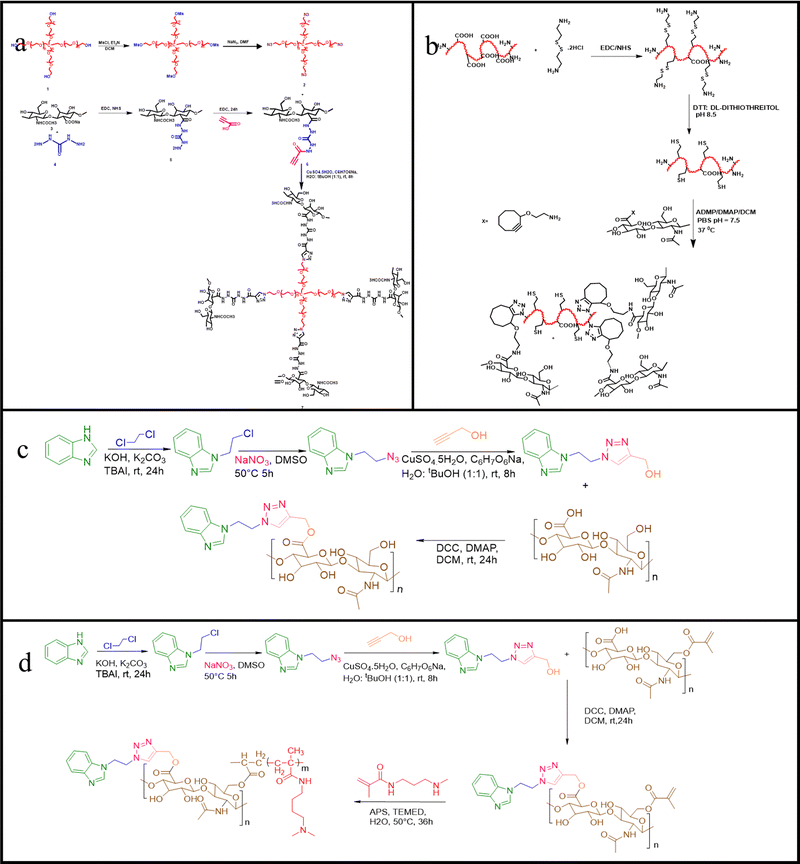 |
| Fig. 5 Intelligent design strategies for the development of HA-based injectable organo-hydrogels. | |
4.3.2 Click-chemistry-based injectable organo-hydrogels with inherent antimicrobial properties.
Further, we propose the development of a click chemistry derived injectable hydrogel using functionalized HA and its derivatives possessing antimicrobial properties. Combination of two or more forms of heterocycles in a molecule impart an enhanced antimicrobial spectrum due to rich electron density clouds.120 The hydrogel consists of 1,2,3-triazole rings along with benzimidazole, thereby increasing its antimicrobial potency. Hence, in addition to the technological advancements, these hydrogels will possess bioactive properties from the click monomeric unit exhibiting remarkable efficacy as an antibacterial agent, in conjunction with injectable properties. The new click monomer (CM) (1-(2-(1H-benzo[d]imidazole-1-yl)ethyl)-H-1,2,3-triazol-4-yl)methanol is synthesized via an azide–alkyne cycloaddition ‘click reaction’. The CM is further co-polymerized with HA or its derivative, wherein, the benzimidazole functionalized monomer containing 1,2,3-triazole groups is synthesized via a copper catalysed 1,3-dipolar cycloaddition reaction. Post that HA was amalgamated with free radical aqueous polymerization, under physical crosslinking of co-networks, leads to the formation of (1-(2-(1H-benzo[d]imidazole-1-yl)ethyl)-H-1,2,3-triazol-4-yl)methanol is depicted in Fig. 5(C). In Fig. 5(D), methacrylated HA (HA derivative) is further combined with dimethylaminopropyl methacrylamide (DMAPMA) via free radical aqueous copolymerization. Methacrylated HA (HA derivative) is further combined with dimethyl aminopropyl methacrylamide (DMAPMA) via free radical aqueous copolymerization. Injectable hydrogels being life-saving carrier agents require special emphasis on their design and development and click chemistry can offer a wide designability to such systems, an area that has huge scope to be explored.
5. Bench-to-bedside implementation of injectable organo-hydrogels
The translation of materiobiological innovations to clinical therapy is frequently referred to as bench-to-bedside, which is mediated by governing institutions such as the FDA and EMA.121 The regulation of the marketability of pharmaceutical products provides medical professionals agency over the pharmacokinetics of the products they prescribe to patients. Bioinspired injectable organo-hydrogels were primarily synthesised on a pilot-plant scale on the grounds of conducting pre-clinical studies. This stipulates the notion of them being manufactured in small-scale batches, thus implying the need to scale up their fabrication techniques, while also adhering to the official guidelines and practices that have been proposed and established for their successful synthesis and commercialisation. As is evident, the subtle sophistication of crosslinking various biomimetic/synthetic/natural starting materials in an effort to devise clinically sound hydrogels makes it even more important for the manufacturing giants to comply with the cGMP (Current Good Manufacturing Practices) which have been put forward for aspects encompassing materiobiological procedures in an effort to strike out any chances of batch variations, therapeutic efficacy, mechanical robustness and adulteration during scale-up of manufacturing techniques. Moreover, the fabrication techniques of hydrogels of natural/biomimetic grade are more demanding as such natural polymers are of heterogeneous nature due to which their molecular characteristics, sterilization and storage have to be taken care of effectively.8,122
5.1 Pilot-scale organo-hydrogel fabrication
The pilot-scale fabrication of organo-hydrogels as an injectable aid requires addressing the critical challenges associated with the final batch size, dispersion process, homogeneous heating of biopolymer solution, and purification of organo-hydrogels.23 These parameters can be summarised as follows:
• The use of industrial reactors of a volume relating to the final batch size (∼10 kg) is necessitated.
• Dispersing elements along with the heating system enable the homogeneous heating of the organo-hydrogel solution.
• The dispersion process is regulated via time, speed, as well as size parameters of the dispersing element.
• The reproducibility of the dispersing element must be consistent for maximum performance.
• Organo-hydrogel purification is conducted with a cartridge device to facilitate concomitant purification of numerous membranes.
• Scale-up of organo-hydrogel production is to be done with minimal manipulation by preparing specifically tailored solution for respective manufacturers.
• Commercially marketed bench-top filling devices enable automatic filling of syringes.
5.2 Regulatory implications
Presently, under Section 201(g) of the FD&C Ac, the FDA has classified hydrogels under ‘devices’ as opposed to drugs which are available commercially. The aforementioned act covers “any product which does not achieve its primary intended purposes through chemical action within or on the body” which clearly implies that ‘a product that has chemical action could be a device if it does not achieve its primary intended purposes through that chemical action’, as stated by the FDA in its regulatory guidelines.123 Furthermore, with a few exceptions, the majority of hydrogel-based products must go through additional FDA review of a 510(k) PreMarket Notification submission in order to obtain legal marketing rights in the United States. Hydrogel scaffolds containing a drug or drug-secreting cells are considered combination products, and thus regulatory approval can take up to 7–10 years, further limiting their commercial viability.122Fig. 6 schematically summarizes the regulatory implications and procedure of clinical investigation of injectable medical products.
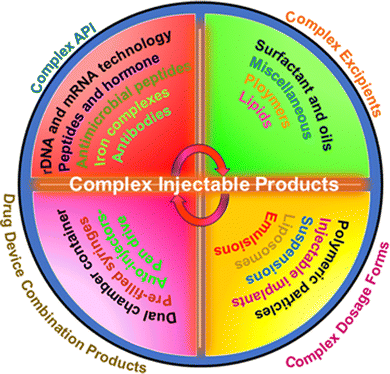 |
| Fig. 6 Procedure of clinical investigation of injectable medical products. Adapted from ref. 124. | |
The procedure for injectable organo-hydrogels to receive the CE mark to demonstrate their compliance with the European regulation is summarised as follows:
• Requires determination of the regulatory guideline to which the medicine applies; hydrogels are active implantable medical devices;
• Classification of medical devices into one of the following categories: class I, IIa, IIb, or III; hydrogels are class III;
• Enforcing a quality management system (QMS);
• Elucidation of all available technical information regarding chemical and physical characteristics as well as properties, along with clinical data about the organo-hydrogel to validate its safe use;
• Audit of the technical information and QMS by a notified body, which includes clinical evaluation and post-marketing supervision to be conducted;
• Receiving the CE marking certificate, which has a validity for three years for hydrogels, and the ISO 13485 certificate, which has a validity of one year for the manufacturer facilities (some countries require manufacture license);
• Declaration of conformity and compliance of the organo-hydrogel to regulatory guidelines;
• State-wise registration of hydrogels where their national regulatory guidelines mandate it, and the process can be repeated on expiration of the CE mark/ISO13485 validity.
6. Conclusion
In conclusion, this perspective proposes biomimetic injectable organo-hydrogels to be translated in the emerging regime of pharmaceutical revolution with the development of bioresponsive and self-healing injectable organo-hydrogels by providing a bird's eye view on their tissue engineering and regenerative medical applicability. Although these classes of organo-hydrogels have not yet made to the market, they exhibit optimistic results in wound healing and drug delivery, and can effectively unleash their versatility in other tissue engineering and regenerative medical applications. Furthermore, with the focus of pharmaceutical revolution, it is imperative to address concerns regarding the demand for novel drugs and efficient techniques of mass production. Along these lines, click chemistry complimented by bio-orthogonal chemistry comes as a paramount stratagem in the conveyor belt of pharmaceutics. Ultimately, it is necessary for them to conform to the regulatory guidelines put forward by governing institutions such as the FDA and EMA for their safe distribution, which have been extensively investigated and elucidated in this perspective. In this approach, we believe that the clinical advancement of injectable organo-hydrogels via click chemistry is an elixir in the arsenal of pharmaceuticals.
Nevertheless, looking at the future directions of establishing click-synthesis-derived injectable organo-hydrogels for various biomedical applications, there are certain bottlenecks associated with organo-hydrogels that need to be overcome. These challenges include achieving desired mechanical properties, immunogenicity and inflammation as some organo-hydrogels may trigger immune response and/or inflammation when implanted. When cells are injected into ischemic tissue for therapeutic repair, they are exposed to a hypoxic microenvironment, which results in immunogenic response at the injection site. Furthermore, the difficulty in biofunctionalization and inhomogeneity is a critical aspect to look at. Some organo-hydrogels are sensitive to common sterilization practices, and finally having an appropriate shelf-life would strongly benefit the medical implementation of these injectable organo-hydrogels.
Author contributions
Abhyavartin Selvam: conceptualization, investigation, methodology, data curation, writing – original draft preparation, writing – review & editing. Misba Majood: investigation, methodology, data curation, writing – original draft preparation, writing – review & editing. Radhika Chaurasia: conceptualization, investigation, writing – original draft preparation, conceptualization of the HA-based injectable organo-hydrogel. Rupesh: conceptualization of the HA-based injectable organo-hydrogel. Akanksha Singh: investigation, writing – original draft preparation. Tapan Dey: visualization. Omnarayan Agrawal: writing – review & editing. Yogesh Kumar Verma: writing – review & editing. Monalisa Mukherjee: conceptualization, methodology, resources, supervision, formal analysis, project administration, funding acquisition, writing – review & editing.
Conflicts of interest
There are no conflicts to declare.
Acknowledgements
MM thanks the Department of Biotechnology (DBT; BT/PR21866/NNT/28/1145/2016) and MM and RC thank the Department of Science and Technology (DST; DST/WOS-A/CS-106/2021). The authors would like to acknowledge the Biorender software for figures. We are also thankful to Amity Institute of Click Chemistry Research and Studies, for providing the research infrastructure.
References
- A. S. Hoffman, Adv. Drug Delivery Rev., 2012, 64, 18–23 CrossRef.
- E. Caló and V. v Khutoryanskiy, Eur. Polym. J., 2015, 65, 252–267 CrossRef.
- A. M. Vasi, M. I. Popa, M. Butnaru, G. Dodi and L. Verestiuc, Mater. Sci. Eng., C, 2014, 38, 177–185 CrossRef CAS PubMed.
- S. Maiz-Fernández, L. Pérez-álvarez, L. Ruiz-Rubio, J. L. Vilas-Vilela and S. Lanceros-Mendez, Polymers, 2020, 12, 2261–2294 CrossRef PubMed.
- N. Carballo-Pedrares, I. Fuentes-Boquete, S. Díaz-Prado and A. Rey-Rico, Pharmaceutics, 2020, 12, 752–773 CrossRef CAS PubMed.
- Q. Chai, Y. Jiao and X. Yu, Gels, 2017, 3, 1–15 CrossRef PubMed.
- A. Vashist, A. Kaushik, K. Alexis, R. Dev Jayant, V. Sagar, A. Vashist and M. Nair, Curr. Pharm. Des., 2017, 23(24), 3595–3602 CrossRef CAS PubMed.
- A. Mandal, J. R. Clegg, A. C. Anselmo and S. Mitragotri, Bioeng. Transl. Med., 2020, 5(2), e10158 CrossRef CAS PubMed.
- Y. Chao, Q. Chen and Z. Liu, Adv. Funct. Mater., 2020, 30, 1902785 CrossRef CAS.
- F. Rizzo and N. S. Kehr, Adv. Healthcare Mater., 2021, 10, 2001341 CrossRef CAS PubMed.
- S. Zhou, D. Yang, D. Yang, Y. Guo, R. Hu, Y. Li, X. Zan and X. Zhang, Macromol. Rapid Commun., 2023, 44, 2200674 CrossRef CAS PubMed.
- P. Bertsch, M. Diba, D. J. Mooney and S. C. G. Leeuwenburgh, Chem. Rev., 2023, 123, 834–873 CrossRef CAS PubMed.
- A. Singh, R. Bhattacharya, A. Shakeel, A. K. Sharma, S. Jeevanandham, A. Kumar, S. Chattopadhyay, H. B. Bohidar, S. Ghosh, S. Chakrabarti, S. K. Rajput and M. Mukherjee, Mater. Horiz., 2019, 6, 274–284 RSC.
- A. K. Mittal, R. Bhardwaj, R. Arora, A. Singh, M. Mukherjee and S. K. Rajput, ACS Omega, 2020, 5, 24239–24246 CrossRef CAS PubMed.
- A. Singh, D. Kochhar, S. Jeevanandham, C. Kar, R. Bhattacharya, A. Shakeel and M. Mukherjee, ACS Appl. Polym. Mater., 2020, 2, 5743–5755 CrossRef CAS.
- A. Agarwal, A. Kumar, P. Garg, A. Chakraborty, R. Verma, M. Sarwat, A. Gupta, P. K. Sasmal, Y. K. Verma, C. Chowdhury and M. Mukherjee, ACS Appl. Polym. Mater., 2022, 4, 5800–5812 CrossRef.
- M. Majood, A. Shakeel, A. Agarwal, S. Jeevanandham, R. Bhattacharya, D. Kochhar, A. Singh, D. Kalyanasundaram, S. Mohanty and M. Mukherjee, Biomed. Mater., 2022, 17, 065004 CrossRef PubMed.
- K. Shivam, A. Selvam, S. Sangam, M. Majood, S. Pahari, R. Patra, A. K. Sharma and M. Mukherjee, Biomater. Adv., 2023, 149, 213395 CrossRef CAS PubMed.
- A. Selvam, T. Aggarwal, M. Mukherjee and Y. K. Verma, Biotechnol. Adv., 2023, 68, 108237 CrossRef PubMed.
- M. Majood, A. Selvam, O. Agrawal, R. Chaurasia, S. Rawat, S. Mohanty and M. Mukherjee, ACS Appl. Mater. Interfaces, 2023, 15, 19997–20011 CrossRef CAS PubMed.
-
Y. R. Singh, A. Selvam, P. E. Lokhande and S. Chakrabarti, in Bioinspired and Green Synthesis of Nanostructures: a Sustainable Approach, ed. M. Sen and M. Mukherjee, Scrivener Publishing LLC, Beverly, MA, 2023, pp. 65–113 Search PubMed.
- A. Agarwal, A. Selvam, M. Majood, O. Agrawal, S. Chakrabarti and M. Mukherjee, Drug Discovery Today, 2023, 28, 103586 CrossRef CAS PubMed.
- J. Maria Alonso, J. Andrade del Olmo, R. Perez Gonzalez, V. Saez-Martinez Citation, A. del Olmo, P. Gonzalez and H. Velasco, Polymers, 2021, 13, 650–672 CrossRef PubMed.
- B. Balakrishnan and R. Banerjee, Chem. Rev., 2011, 111, 4453–4474 CrossRef CAS PubMed.
- S. Lin-Gibson, S. Bencherif, J. A. Cooper, S. J. Wetzel, J. M. Antonucci, B. M. Vogel, F. Horkay and N. R. Washburn, Biomacromolecules, 2004, 5, 1280–1287 CrossRef CAS PubMed.
- J. H. Lee, Biomater. Res., 2018, 22, 1–14 CrossRef PubMed.
-
H. Deyhle, G. Schulz and B. Müller, in Encyclopedia of Nanotechnology, ed. B. Bhushan, Springer, Dordrecht, 2012, pp. 1049–1056 Search PubMed.
- M. D. Baumann, C. E. Kang, J. C. Stanwick, Y. Wang, H. Kim, Y. Lapitsky and M. S. Shoichet, J. Controlled Release, 2009, 138, 205–213 CrossRef CAS PubMed.
- S. Uman, A. Dhand and J. A. Burdick, J. Appl. Polym. Sci., 2020, 137, 48668 CrossRef CAS.
- C. Loebel, C. B. Rodell, M. H. Chen and J. A. Burdick, Nat. Protoc., 2017, 12, 1521–1541 CrossRef CAS PubMed.
- A. Mellati and J. Akhtari, Res. Mol. Med., 2019, 6, 1–14 CrossRef.
- S. R. Caliari and J. A. Burdick, Nat. Methods, 2016, 13, 405–414 CrossRef CAS PubMed.
- X. Li and Y. Xiong, ACS Omega, 2022, 7, 36918–36928 CrossRef CAS PubMed.
- EUFLEXXA, https://www.euflexxa.com/, (accessed 9 October 2022).
- Bone Grafting – Spine and Trauma Surgery – Infuse Bone Graft|Medtronic, https://www.medtronic.com/us-en/healthcare-professionals/products/spinal-orthopaedic/bone-grafting/infuse-bone-graft.html, (accessed 9 October 2022).
- G. E. Friedlaender, C. R. Perry, J. D. Cole, S. D. Cook, G. Cierny, G. F. Muschler, G. A. Zych, J. H. Calhoun, A. J. LaForte and S. Yin, J. Bone Jt. Surg., Am. Vol., 2001, 83-A(Suppl 1), S151 Search PubMed.
- TraceIT Tissue Marker 3 mL 1-UP TH-1031 Medical Device Identification, https://fda.report/GUDID/00864661000133, (accessed 9 October 2022).
- Home – Bulkamid, https://bulkamid.com/en-US, (accessed 9 October 2022).
- S. Mohr, C. Marthaler, S. Imboden, A. Monga, M. D. Mueller and A. Kuhn, Int. Urogynecol. J., 2017, 28, 1657–1661 CrossRef PubMed.
- FDA Approvals: Coaptite, Resolution Endovascular System, ACB Test, https://www.medscape.com/viewarticle/521537, (accessed 9 October 2022).
- L. C. Lee, S. T. Wall, D. Klepach, L. Ge, Z. Zhang, R. J. Lee, A. Hinson, J. H. Gorman, R. C. Gorman and J. M. Guccione, Int. J. Cardiol., 2013, 168, 2022–2028 CrossRef PubMed.
- A Randomized, Controlled Study to Evaluate Algisyl-LVR™ as a Method of Left Ventricular Augmentation for Heart Failure – Full Text View – ClinicalTrials.gov, https://www.clinicaltrials.gov/ct2/show/NCT01311791, (accessed 9 October 2022).
- A Pivotal Trial to Establish the Efficacy and Safety of Algisyl in Patients With Moderate to Severe Heart Failure – Full Text View – ClinicalTrials.gov, https://clinicaltrials.gov/ct2/show/NCT03082508, (accessed 9 October 2022).
- D. L. Mann, R. J. Lee, A. J. S. Coats, G. Neagoe, D. Dragomir, E. Pusineri, M. Piredda, L. Bettari, B. A. Kirwan, R. Dowling, M. Volterrani, S. D. Solomon, H. N. Sabbah, A. Hinson and S. D. Anker, Eur. J. Heart Failure, 2016, 18, 314–325 CrossRef CAS PubMed.
- S. D. Anker, A. J. S. Coats, G. Cristian, D. Dragomir, E. Pusineri, M. Piredda, L. Bettari, R. Dowling, M. Volterrani, B. A. Kirwan, G. Filippatos, J. L. Mas, N. Danchin, S. D. Solomon, R. J. Lee, F. Ahmann, A. Hinson, H. N. Sabbah and D. L. Mann, Eur. Heart J., 2015, 36, 2297–2309 CrossRef CAS PubMed.
- Vantas|C70H94N18O16 – PubChem, https://pubchem.ncbi.nlm.nih.gov/compound/Vantas, (accessed 18 October 2022).
- SpaceOAR™ Hydrogel|Prostate Cancer Hydrogel Spacer, https://www.spaceoar.com/, (accessed 18 October 2022).
- A. Balen, D. Sobel, S. Elsamra and D. Golijanin, J. Endourol. Case Rep., 2020, 6, 442 CrossRef PubMed.
- Home – Radiesse Injectables, https://radiesse.com/, (accessed 5 November 2022).
- Merz Aesthetics, https://www.merzaesthetics.ca/solutions/radiesse/, (accessed 8 November 2022).
- BELLAFILL® TO REPLACE ARTEFILL® AS NEW BRAND – Suneva Medical, https://www.sunevamedical.com/bellafill-to-replace-artefill-as-new-brand/, (accessed 12 November 2022).
- Sculptra®: A Poly-L-lactic Acid Facial Injection, https://www.sculptrausa.com/, (accessed 5 November 2022).
- M. H. Gold, J. Dermatol. Surg. Oncol., 1994, 20, 586–590 CrossRef CAS PubMed.
- B. A. Matti, F. V. Nicolle, M. Chit and F. R. C. S. London, Aesthetic Plast. Surg., 1990, 14, 227–234 CrossRef CAS PubMed.
- Restylane|Galderma, https://www.galderma.com/mx/en/restylane, (accessed 5 November 2022).
- Home – Belotero BALANCE, https://www.belotero.com/, (accessed 5 November 2022).
- R. T. Adamyan, O. N. Aleshina, E. I. Abdeeva and M. Y. Sinelnikov, Plast. Reconstr. Surg. Glob. Open, 2021, 9, e3842 CrossRef PubMed.
- L. Hallén, C. Johansson and C. Laurent, Acta Otolaryngol., 2009, 119, 107–111 Search PubMed.
- TEOSYAL® Products|Teoxane Laboratories, https://www.teoxane.com/en/dermal-fillers/teosyalr-products, (accessed 5 November 2022).
- K. K. Jain, Methods Mol. Biol., 2020, 2059, 1–54 CrossRef CAS PubMed.
- H. J. Sim, T. Thambi and D. S. Lee, J. Mater. Chem. B, 2015, 3, 8892–8901 RSC.
- P. Y. Lee, Z. Li and L. Huang, Pharm. Res., 2003, 20, 1995–2000 CrossRef CAS PubMed.
- Z. Megeed, M. Haider, D. Li, B. W. O’Malley, J. Cappello and H. Ghandehari, J. Controlled Release, 2004, 94, 433–445 CrossRef CAS PubMed.
- L. Chen, D. Yan, N. Wu, Q. Yao, H. Sun, Y. Pang and Y. Fu, Bioact. Mater., 2021, 6, 3062–3073 CrossRef CAS PubMed.
- J. S. Lee, M. J. Kang, J. H. Lee and D. W. Lim, Biomacromolecules, 2022, 23(5), 2051–2063 CrossRef CAS PubMed.
- M. H. Turabee, T. Thambi, H. T. T. Duong, J. H. Jeong and D. S. Lee, Biomater. Sci., 2018, 6, 661–671 RSC.
- Y. Ren, X. Zhao, X. Liang, P. X. Ma and B. Guo, Int. J. Biol. Macromol., 2017, 105, 1079–1087 CrossRef CAS PubMed.
- K. Zubik, P. Singhsa, Y. Wang, H. Manuspiya and R. Narain, Polymers, 2017, 9, 119 CrossRef PubMed.
- C. D. Hoemann, J. Sun, A. Légaré, M. D. McKee and M. D. Buschmann, Osteoarthr. Cartil., 2005, 13, 318–329 CrossRef CAS PubMed.
- J. Hoque, R. G. Prakash, K. Paramanandham, B. R. Shome and J. Haldar, Mol. Pharmaceutics, 2017, 14, 1218–1230 CrossRef CAS PubMed.
- H. Naderi-Meshkin, K. Andreas, M. M. Matin, M. Sittinger, H. R. Bidkhori, N. Ahmadiankia, A. R. Bahrami and J. Ringe, Cell Biol. Int., 2014, 38, 72–84 CrossRef CAS PubMed.
- H. Yamaoka, H. Asato, T. Ogasawara, S. Nishizawa, T. Takahashi, T. Nakatsuka, I. Koshima, K. Nakamura, H. Kawaguchi, U. il Chung, T. Takato and K. Hoshi, J. Biomed. Mater. Res., Part A, 2006, 78A, 1–11 CrossRef CAS PubMed.
- C. D. Hoemann, M. Hurtig, E. Rossomacha, J. Sun, A. Chevrier, M. S. Shive and M. D. Buschmann, J. Bone Jt. Surg., 2005, 87, 2671–2686 CrossRef.
- Y. Liu and S. H. Hsu, Front. Chem., 2018, 6, 449 CrossRef CAS PubMed.
- V. K. Anupama Devi, R. Shyam, A. Palaniappan, A. K. Jaiswal, T. H. Oh and A. J. Nathanael, Polymers, 2021, 13, 3782 CrossRef PubMed.
- Z. Deng, H. Wang, P. X. Ma and B. Guo, Nanoscale, 2020, 12, 1224–1246 RSC.
- W. Zou, J. Dong, Y. Luo, Q. Zhao and T. Xie, Adv. Mater., 2017, 29, 1606100 CrossRef PubMed.
- B. Liu, Y. Wang, Y. Miao, X. Zhang, Z. Fan, G. Singh, X. Zhang, K. Xu, B. Li, Z. Hu and M. Xing, Biomaterials, 2018, 171, 83–96 CrossRef CAS PubMed.
- L. L. Wang, C. B. Highley, Y. C. Yeh, J. H. Galarraga, S. Uman and J. A. Burdick, J. Biomed. Mater. Res., Part A, 2018, 106, 865–875 CrossRef CAS PubMed.
- S. H. Hong, S. Kim, J. P. Park, M. Shin, K. Kim, J. H. Ryu and H. Lee, Biomacromolecules, 2018, 19, 2053–2061 CrossRef CAS PubMed.
- J. He, Z. Zhang, Y. Yang, F. Ren, J. Li, S. Zhu, F. Ma, R. Wu, Y. Lv, G. He, B. Guo and D. Chu, Nanomicro Lett., 2021, 13, 1–17 Search PubMed.
- L. Wang, J. Wang, X. Zhou, J. Sun, B. Zhu, C. Duan, P. Chen, X. Guo, T. Zhang and H. Guo, Front. Bioeng. Biotechnol., 2020, 8, 1047 Search PubMed.
- Z. Wei, J. Zhao, Y. M. Chen, P. Zhang and Q. Zhang, Sci. Rep., 2016, 6, 1–12 CrossRef PubMed.
- C. Wang, M. Wang, T. Xu, X. Zhang, C. Lin, W. Gao, H. Xu, B. Lei and C. Mao, Theranostics, 2019, 9, 65–76 CrossRef CAS PubMed.
- H. Xuan, S. Wu, S. Fei, B. Li, Y. Yang and H. Yuan, Mater. Sci. Eng., C, 2021, 128, 112264 CrossRef CAS PubMed.
- Y. Liang, X. Zhao, T. Hu, B. Chen, Z. Yin, P. X. Ma and B. Guo, Small, 2019, 15, 1900046 CrossRef PubMed.
- Z. Qian, H. Wang, Y. Bai, Y. Wang, L. Tao, Y. Wei, Y. Fan, X. Guo and H. Liu, ACS Appl. Mater. Interfaces, 2020, 12, 55659–55674 CrossRef CAS PubMed.
- A. Shpichka, D. Butnaru, E. A. Bezrukov, R. B. Sukhanov, A. Atala, V. Burdukovskii, Y. Zhang and P. Timashev, Stem Cell Res. Ther., 2019, 10, 1–16 CrossRef PubMed.
- B. Guo, J. Qu, X. Zhao and M. Zhang, Acta Biomater., 2019, 84, 180–193 CrossRef CAS PubMed.
- A. C. Gaffey, M. H. Chen, C. M. Venkataraman, A. Trubelja, C. B. Rodell, P. V. Dinh, G. Hung, J. W. Macarthur, R. V. Soopan, J. A. Burdick and P. Atluri, J. Thorac. Cardiovasc. Surg., 2015, 150, 1268–1277 CrossRef PubMed.
- C. B. Rodell, J. W. MacArthur, S. M. Dorsey, R. J. Wade, L. L. Wang, Y. J. Woo and J. A. Burdick, Adv. Funct. Mater., 2015, 25, 636–644 CrossRef CAS PubMed.
- H. Liu, C. Wang, C. Li, Y. Qin, Z. Wang, F. Yang, Z. Li and J. Wang, RSC Adv., 2018, 8, 7533–7549 RSC.
- F. Y. Hsieh, H. W. Han, X. R. Chen, C. S. Yang, Y. Wei and S. Hui Hsu, Biomaterials, 2018, 174, 31–40 CrossRef CAS PubMed.
- J. Wang, F. Zhang, W. P. Tsang, C. Wan and C. Wu, Biomaterials, 2017, 120, 11–21 CrossRef CAS PubMed.
- C. D. Hein, X. M. Liu and D. Wang, Pharm. Res., 2008, 25, 2216–2230 CrossRef CAS PubMed.
- K. Nwe and M. W. Brechbiel, Cancer Biother. Radiopharm., 2009, 24, 289–302 CrossRef CAS PubMed.
- Z. Xu and K. M. Bratlie, ACS Biomater. Sci. Eng., 2018, 4, 2276–2291 CrossRef CAS PubMed.
- X. Wang, Z. Li, T. Shi, P. Zhao, K. An, C. Lin and H. Liu, Mater. Sci. Eng., C, 2017, 73, 21–30 CrossRef CAS PubMed.
- S. S. Han, H. Y. Yoon, J. Y. Yhee, M. O. Cho, H. E. Shim, J. E. Jeong, D. E. Lee, K. Kim, H. Guim, J. H. Lee, K. M. Huh and S. W. Kang, Polym. Chem., 2017, 9, 20–27 RSC.
- S. Liu, M. Dong, Z. Zhang and G. Fu, Polym. Adv. Technol., 2017, 28, 1065–1070 CrossRef CAS.
- S. I. H. Abdi, J. Y. Choi, J. S. Lee, H. J. Lim, C. Lee, J. Kim, H. Y. Chung and J. O. Lim, Tissue Eng. Regener. Med., 2012, 9, 1–9 CrossRef CAS.
- H. Ding, B. Li, Y. Jiang, G. Liu, S. Pu, Y. Feng, D. Jia and Y. Zhou, Carbohydr. Polym., 2021, 251, 117101 CrossRef CAS PubMed.
- N. Ahmad, B. Colak, D. W. Zhang, M. J. Gibbs, M. Watkinson, C. Remzi Becer, J. E. Gautrot and S. Krause, Sensors, 2019, 19, 1677 CrossRef CAS PubMed.
- Y. Zhang, J. Shen, R. Hu, X. Shi, X. Hu, B. He, A. Qin and B. Z. Tang, Chem. Sci., 2020, 11, 3931–3935 RSC.
- K. Nagahama, Y. Kimura and A. Takemoto, Nat. Commun., 2018, 9, 1–11 CAS.
- K. Li, C. Zhou, S. Liu, F. Yao, G. Fu and L. Xu, React. Funct. Polym., 2017, 117, 81–88 CrossRef CAS.
- S. M. Hodgson, E. Bakaic, S. A. Stewart, T. Hoare and A. Adronov, Biomacromolecules, 2016, 17, 1093–1100 CrossRef CAS PubMed.
- B. Bi, M. Ma, S. Lv, R. Zhuo and X. Jiang, Carbohydr. Polym., 2019, 212, 368–377 CrossRef CAS PubMed.
- L. Xu, R. C. Cooper, J. Wang, W. A. Yeudall and H. Yang, ACS Biomater. Sci. Eng., 2017, 3, 1641–1653 CrossRef CAS PubMed.
- H. Koo, S. Lee, J. H. Na, S. H. Kim, S. K. Hahn, K. Choi, I. C. Kwon, S. Y. Jeong and K. Kim, Angew. Chem., Int. Ed., 2012, 51, 11836–11840 CrossRef CAS PubMed.
- S. Song, M. K. Shim, S. Lim, Y. Moon, S. Yang, J. Kim, Y. Hong, H. Y. Yoon, I. S. Kim, K. Y. Hwang and K. Kim, Bioconjugate Chem., 2020, 31, 1562–1574 CrossRef CAS PubMed.
- S. C. Owen, S. A. Fisher, R. Y. Tam, C. M. Nimmo and M. S. Shoichet, Langmuir, 2013, 29, 7393–7400 CrossRef CAS PubMed.
- L. Zhang, J. J. Chang, S. L. Zhang, G. L. V. Damu, R. X. Geng and C. H. Zhou, Bioorg. Med. Chem., 2013, 21, 4158–4169 CrossRef CAS PubMed.
- A. M. Juncan, D. G. Moisă, A. Santini, C. Morgovan, L. L. Rus, A. L. Vonica-ţincu and F. Loghin, Molecules, 2021, 26, 4429 CrossRef CAS PubMed.
- A. la Gatta, C. Schiraldi, A. Papa and M. de Rosa, Polym. Degrad. Stab., 2011, 96, 630–636 CrossRef CAS.
- S. Uman, A. Dhand and J. A. Burdick, J. Appl. Polym. Sci., 2020, 137, 48668 CrossRef CAS.
- T. Sakai, T. Matsunaga, Y. Yamamoto, C. Ito, R. Yoshida, S. Suzuki, N. Sasaki, M. Shibayama and U. Il Chung, Macromolecules, 2008, 41, 5379–5384 CrossRef CAS.
- Z. S. Chinoy and F. Friscourt, Isr. J. Chem., 2023, 63, e202200055 CrossRef CAS.
- J. Dommerholt, F. P. J. T. Rutjes and F. L. van Delft, Top. Curr. Chem., 2016, 374, 1–20 CrossRef CAS PubMed.
- Y. Bansal, M. Kaur and G. Bansal, Mini-Rev. Med. Chem., 2019, 19, 624–646 CrossRef CAS PubMed.
- P. S. Knoepfler, Adv. Drug Delivery Rev., 2015, 82–83, 192–196 CrossRef CAS PubMed.
- J. Li and D. J. Mooney, Nat. Rev. Mater., 2016, 1, 1–17 Search PubMed.
- Classification of Products as Drugs and Devices and Additional Product Classification Issues|FDA, https://www.fda.gov/regulatory-information/search-fda-guidance-documents/classification-products-drugs-and-devices-and-additional-product-classification-issues#_ftn11, (accessed 5 November 2022).
- K. Panchal, S. Katke, S. K. Dash, A. Gaur, A. Shinde, N. Saha, N. K. Mehra and A. Chaurasiya, Drug Delivery Transl. Res., 2022, 1–40 Search PubMed.
Footnote |
† These authors have contributed equally. |
|
This journal is © The Royal Society of Chemistry 2023 |