DOI:
10.1039/D3TB00668A
(Review Article)
J. Mater. Chem. B, 2023,
11, 8315-8326
Beyond traditional light: NIR-II light-activated photosensitizers for cancer therapy
Received
28th March 2023
, Accepted 14th July 2023
First published on 19th July 2023
Abstract
With increasing demand for the accurate and safe treatment of cancer, non-invasive photodynamic therapy (PDT) has received widespread attention. However, most conventional photosensitizers are typically excited by short-wavelength visible light (400–700 nm), thus substantially hindering the penetration of light and the therapeutic effectiveness of the PDT procedure. Fortunately, near-infrared (NIR) light (>700 nm), in particular, light in the second near-infrared region (NIR-II, 1000–1700 nm) has a higher upper radiation limit, greater tissue tolerance, and deeper tissue penetration compared with traditional short-wavelength light excitation, and shows considerable potential in the clinical treatment of cancer. Therefore, it is of paramount importance and clinical value to develop photosensitizers that are excited by NIR-II light. In this review, for the first time we focus completely on recent progress made with various NIR-II photosensitizers for cancer treatment via PDT, and we briefly present the ongoing challenges and prospects of currently developed NIR-II photosensitizers for clinical practice in the near future. We believe that the above topics will inspire broad interest in researchers from interdisciplinary fields that include chemistry, materials science, pharmaceuticals, and clinical medicine, and provide insightful perspectives for exploiting new NIR-II photosensitizers for biomedical applications.
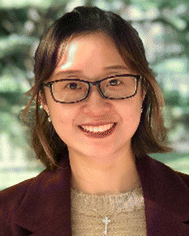
Jinfeng Zhang
| Jinfeng Zhang received her M.Sc. degree in organic chemistry in 2013 from the Technical Institute of Physics and Chemistry, Chinese Academy of Sciences, and her PhD from the Department of Biology and Chemistry at City University of Hong Kong, in 2017. Currently, she is an Associate Professor in the School of Life Sciences, Beijing Institute of Technology, China. Her research focuses mainly on the design and fabrication of self-assembled nanomaterials for drug delivery, bioimaging, and the treatment of various diseases, including cancer, inflammation-induced injuries as well as cardiovascular and neurodegenerative diseases. |
1. Introduction
Over the past few decades, cancer has emerged as one of the deadliest diseases worldwide.1,2 Compared with conventional methods of cancer treatment, such as surgery, radiotherapy, and chemotherapy, photodynamic therapy (PDT) is one of the most promising treatment modalities that has improved target specificity and reduced side effects, and has received significant attention from the perspective of both fundamental research and clinical practice.3–12 According to data from the ClinicalTrials.gov website, more than 500 studies on PDT are ongoing or have been completed, where some of these processes have been clinically approved for cancer therapy.13 In a typical type-II PDT process, photosensitizers are first activated by absorbing light energy, and they then interact with oxygen (O2) to generate reactive oxygen species (ROS), which ultimately trigger local or systemic cytotoxicity.14–17 In this regard, PDT relies on three components: photosensitizers, O2, and light. Up to now, a variety of chemically and nanotechnologically empowered photosensitizers have been developed to enhance the production of ROS for PDT.18–27
Although PDT has been studied widely as a promising treatment for cancer therapy, its wide clinical application has still been greatly limited.28–31 For example, most conventional photosensitizers are typically excited by visible light (400–700 nm). However, visible light of short wavelengths is not only easily absorbed by biological tissues but also has a limited tissue-penetration depth.32–34 Fortunately, due to the relatively weak interaction of near-infrared (NIR) light with biological tissues, photosensitizers excited by light in the first near-infrared window (NIR-I), i.e., in the wavelength range of 700–1000 nm, have drawn widespread attention for biomedical applications.35–37 Notably, compared with short-wavelength light, excitation in the second near-infrared window (NIR-II; 1000–1700 nm) shows a very weak interaction with biological tissues as well as minimal phototoxicity.38–43 More importantly, compared with shorter-wavelength light sources (0.33 W cm−2 at 808 nm), NIR-II light has a higher maximum permissible exposure (1 W cm−2 at 1064 nm) and shows deeper tissue penetration (5–20 mm), and is thus emerging as a more preferable light source for PDT.44–46 On account of the above advantages, photosensitizers stimulated by NIR-II light are particularly suitable for the treatment of deep-seated cancers. At present, numerous NIR-II-excited imaging and photothermal agents have been developed rapidly, which have been summarized in several previously reported reviews.47–54 However, there is currently no systematic summary of the NIR-II photosensitizers used for cancer therapy. In this review, we will describe the principle of the photosensitizer mechanism, summarize the recent applications of different types of NIR-II photosensitizers used in cancer treatment (Fig. 1), and discuss the existing challenges in the clinical transformation of photosensitizers based on NIR-II light activation. We believe that this review will provide insightful perspectives and clear prospects towards the rapid development of NIR-II photosensitizers in biomedicine, and will be highly valuable for the exploitation of new photosensitizer materials. This review will also appeal to scientists and researchers in the fields of chemical, physical, material, biological and medical sciences.
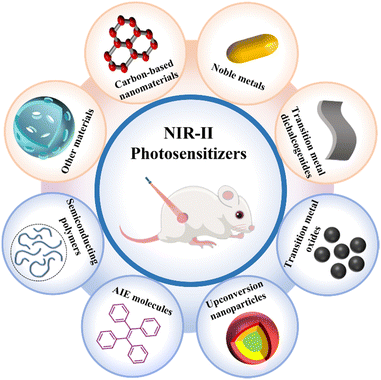 |
| Fig. 1 Schematic illustration of photosensitizers excited by NIR-II light for cancer therapy. | |
2. Mechanism of PDT
Up to now, three types of photodynamic mechanism have been exploited for cancer treatment (Fig. 2). When irradiated with light of the appropriate wavelength, photosensitizers are converted from the ground state (S0) to an unstable singlet excited state (Sn) followed by a fast internal conversion (IC) process to the lowest singlet excited state (S1). The excited photosensitizer is unstable and may change from the S1 state to a more stable excited triplet state (T1) through intersystem crossing (ISC), thus achieving PDT. According to different photochemical reaction processes, PDT can be divided into three types. For type-I PDT, T1 reacts directly with the surrounding substrates and transfers electrons/hydrogen atoms to produce free radicals, which may react further with other molecules (i.e., O2 and water) to generate ROS, which include superoxide anions (˙O2−) and hydroxyl radicals (˙OH).55–57 For type-II PDT, the energy of the triplet exciton is transferred to molecular oxygen (3O2) to produce singlet oxygen (1O2). Compared with type-II PDT, type-I PDT is less O2 dependent and is therefore considered to be more suitable for hypoxia conditions. In addition, very recently, Peng's group has provided evidence of a new concept and examples of type-III PDT for cancer treatment, in which type-III photosensitizers can specifically bind and transmit their excitation energy to nucleic acids (e.g., RNA), proteins, and other biological macromolecules in tumor cells upon light irradiation. Consequently, such novel type-III photosensitizers can kill different types of tumor cell directly via an O2-independent pathway.58
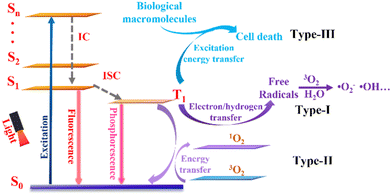 |
| Fig. 2 Three types of photodynamic mechanism exploited for cancer treatment. | |
3. Categories of NIR-II photosensitizer
In order to overcome the limitation of visible-light penetration and apply PDT to deep-seated or large tumors, a variety of functional photosensitizers that can be activated by NIR-II light have been reported. In this section, we will discuss the different types of NIR-II photosensitizer for cancer treatment, which include carbon-based nanomaterials, noble metals, transition metal chalcogenides, transition metal oxides, upconversion nanoparticles, aggregation-induced emission molecules, semiconducting polymers, and other NIR-II photosensitizers. Table 1 summarizes the recently developed NIR-II light-activated photosensitizers used in cancer treatment.
Table 1 Summary of NIR-II light-activated photosensitizers for cancer therapy
Category |
Photosensitizer |
Laser (nm) |
Power |
PDT types |
Pros |
Cons |
Ref. |
Carbon-based nanomaterials |
CDs-NO |
1064 |
0.8 W cm−2 |
Type-I and type-II |
High loading rate |
Poor water solubility |
66
|
Strong NIR absorption |
Ti3C2–Cu–PEG |
1064 |
1 W cm−2 |
Type-I and type-II |
Excellent photon stability |
Dose-dependent toxicity |
71
|
Tunable optical properties |
Easy modification |
Noble metals |
AuNC@HSA/CAT |
1064 |
0.2 W cm−2 |
Type-I and type-II |
Unique physicochemical and optical properties |
Difficult to clear |
79
|
MnO2/Ag3SbS3 |
1064 |
1 W cm−2 |
Type-II |
Tunable optical scattering and absorption |
Low molar extinction coefficient |
80
|
Au/Ag NR |
1064 |
1 W cm−2 |
Type-II |
81
|
Transition metal chalcogenides |
FeS2/CoS2@PEG |
1064 |
0.8 W cm−2 |
Type-I |
Strong NIR absorption |
Disruption of cellular homeostasis |
84
|
Poor water solubility |
Transition metal oxides |
ZrO2−x-B@SiO2-HA |
1064 |
1 W cm−2 |
Type-I |
Tunable localized surface plasmon resonance |
Weak light absorption in the NIR region |
86
|
PEG-MoOx |
1064 |
1 W cm−2 |
Type-II |
90
|
CeO2−x@HA |
1064 |
1 W cm−2 |
Type-I |
91
|
Upconversion nanoparticles |
TiO2@UCN/Qr/LA |
1060 |
0.6 W cm−2 |
Type-I and type-II |
Intrinsic upconversion luminescence properties |
Limited excitation wavelengths |
95
|
UCNPs-ZnPc |
1532 |
— |
Type-II |
Great photostability |
Requirement of high laser intensity |
96
|
Aggregation-induced emission molecules |
TQ-BTPE |
1200 |
200 J cm−2 |
Type-II |
Unique luminescence enhancement |
Limited NIR-II absorption intensity |
107
|
TPBPy |
1000 |
10 J cm−2 |
Type-II |
Improved ROS generation ability |
Low molar extinction coefficient |
111
|
Poor water solubility |
Organic semiconducting polymers |
PTTe |
1064 |
1 W cm−2 |
Type-I |
High photostability |
Low quantum yield |
116
|
Flexible optical properties |
Liver enrichment and long-term retention |
Layered double hydroxides |
LA and LDH |
1270 |
0.5 W cm−2 |
Type-I |
Large specific surface area |
Poor stability |
118
|
Tunable chemical composition and structure |
Difficult to control size and poor repeatability |
Difficult to clear |
Mesoporous materials |
ER-HMCu2−xS/CXB |
1064 |
0.8 W cm−2 |
— |
Porous structure and surface area |
Poor dispersion |
121
|
TCLP |
1064 |
0.8 W cm−2 |
Type-I and type-II |
Easy functionalization |
Complex synthesis process |
122
|
pH response degradation |
3.1. Carbon-based nanomaterials
Compared with other traditional photosensitizers, carbon-based nanomaterials have become increasingly attractive in PDT due to their high loading rate, strong NIR absorption, excellent photon stability, tunable optical properties, easy modification and functionalization, and low toxicity.59–63 Among them, carbon dots and MXene nanomaterials have been used widely as NIR-II photosensitizers for PDT in recent years.
3.1.1. Carbon dots.
Carbon-based nanomaterials with diameters between 1 and 10 nm are commonly referred to as carbon dots (CDs), and are generally considered to be core–shell structures. The outer layer of the graphite core usually contains rich functional groups, which include –NH2, –OH, and –COOH. Such hydrophilic groups endow the CDs with good water solubility and the capability of further functionalization.64,65 In addition, due to their wide absorption spectra, tunable emission region, photostability, and good biocompatibility, recent studies have shown that CDs have potential applications in NIR-II PDT. For example, Wang and co-authors used methylene blue (MB) as a carbon source, H2SO4 as an oxidant and a dehydration (carbonization) agent to synthesize CDs via a hydrothermal method for 1064 nm laser-activated PDT.66 The results showed that the aromatic structure of MB and the heteroatoms of the carbon source have an important impact on the ROS generation performance of the as-synthesized CDs. In addition, due to the hydrothermal process, a large number of –OH groups were retained on the surface of the CDs, which could then be subject to a simple nitrification reaction to induce nitrification of the CDs (CDs-NO). More interestingly, the CDs-NO could release NO for gas therapy (GT) in the presence of glutathione (GSH) which is overexpressed in the tumor microenvironment. Under NIR-II irradiation, the ROS can capture NO rapidly and form ONOO− free radicals with a high cytotoxicity. Besides, the depletion of GSH can alleviate the antioxidant system in the tumor microenvironment, which is beneficial for increasing the oxidative stress of PDT. Finally, the synergetic therapy can arouse an immune response and effectively inhibit tumor metastasis.
3.1.2. Metal carbides and nitrides.
MXene is a two-dimensional material formed of transition metal carbides and nitrides or carbonitrides. In recent years, MXene nanosheets have become an ideal carrier candidate for research into tumor phototherapy due to their large specific surface area, easy functionalization, and uniform composition.67–70 Ti3C2, as a common MXene nanosheet, is usually used to load drugs or metal nanoparticles for combined tumor treatment, although it suffers from poor stability against O2 and water. Zhang et al. proposed and fabricated a biocompatible and O2-resistant Cu-decorated Ti3C2 nanocomposite (Ti3C2–Cu–PEG), which could effectively consume the GSH, responding smartly to NIR-II light to achieve photothermally enhanced PDT (Fig. 3a).71 Specifically, after irradiation with 1064 nm laser light (1 W cm−2) for 5 min, the temperature of Ti3C2–Cu–PEG reached 55.8 °C, indicating a good photothermal performance (Fig. 3b–d). Attractively, the Ti3C2–Cu–PEG not only reacted with O2 to generate 1O2via NIR-II light radiation but also catalyzed the endogenous H2O2 to produce ˙OH (Fig. 3e–g). Furthermore, Ti3C2–Cu–PEG could consume GSH to promote oxidative stress, finally achieving NIR-II-triggered PDT synergistic anti-tumor therapy both in vitro and in vivo.
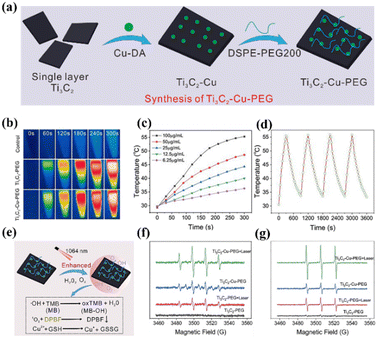 |
| Fig. 3 (a) Schematic illustration of the preparation of Ti3C2–Cu–PEG. (b) Temperature variation infrared thermal images of Ti3C2–Cu–PEG (100 μg mL−1). (c) Ti3C2–Cu–PEG at different concentrations under 1064 nm irradiation. (d) Recycling–heating profiles of Ti3C2–Cu–PEG (1064 nm, 1 W cm−2) for four laser on/off cycles. (e) Schematic diagram of the detection of ROS generated by Ti3C2–Cu–PEG and its GSH consumption. (f) Electron spin resonance (ESR) spectra of ˙OH with or without laser irradiation. (g) ESR spectra of 1O2 with or without laser irradiation. Reproduced with permission from ref. 71. Copyright 2022, De Gruyter. | |
3.2. Noble metals
In recent years, noble metal nanomaterials have attracted wide interest because of their unique physicochemical and optical properties.72–74 Among them, gold (Au) and silver (Ag) show unique size- and shape-dependent optoelectronic characteristics. Therefore, it is possible to adjust the optical properties of noble metal materials by changing the size and shape, enhancing their therapeutic properties as photosensitizers.75–78 In addition, the possibility of further chemical modification improves their bioavailability, making noble metal nanomaterials a potent candidate for the clinical treatment of cancer.
3.2.1. Gold nanoparticles.
In 2018, Liu's group developed a novel and multifunctional photosensitizer that simultaneously achieves tumor hypoxia relief, in vivo fluorescence imaging, and NIR-II light-activated PDT.79 In this study, they used human serum albumin (HSA) and catalase (CAT) to co-modify alkyl thiolated gold nanoclusters (AuNCs), namely AuNC@HSA/CAT nanoparticles. In the AuNC@HSA/CAT system, HSA greatly promoted the physiological stability of the nanoparticles, and the AuNCs could efficiently generate ˙OH and 1O2 under 1064 nm light irradiation. The coating of CAT triggered the degradation of endogenous tumor H2O2 to produce O2, thus improving the effect of PDT through relieving tumor hypoxia.
3.2.2. Silver nanoparticles.
As a typical example of silver-based semiconductors, Ag3SbS3 nanoparticles with a narrow band gap (1.5–1.7 eV) exhibit superb optical absorption in the NIR-II window, show negligible invasiveness, and no accumulation of multidrug resistance, displaying an excellent feasibility for NIR-II PA imaging, photothermal therapy (PTT) and PDT. As shown in Fig. 4a, Wang et al. synthesized Ag and Ag3SbS3 nanoparticles using a typical hydrothermal method, then the Ag3SbS3 NPs were decorated with PEG and loaded with MnO2 to prepare MnO2/Ag3SbS3 (MA).80 Intriguingly, the tumor microenvironment could trigger the degradation of MA into Mn2+ ions and ultrasmall Ag3SbS3 nanoparticles (Fig. 4b), which can be rapidly excreted via liver and kidney pathways, avoiding their accumulation in normal tissues. The released Mn2+ ions could catalyze H2O2 to generate ˙OH through a Fenton-like reaction (Fig. 4c). As shown in Fig. 4d, under different pH conditions and 1064 nm laser irradiation, the generation of 1O2 in the MA solution is detected using the 1,3-diphenylisobenzofuran (DPBF) probe sensor. The results showed that acidic conditions were helpful to promote the formation of 1O2. In addition, MA could constantly deplete GSH and produce O2 for enhancing the efficiency of chemodynamic therapy (CDT) and PDT. In another study, Jin et al. reported a corn-like Au/Ag nanorod (Au/Ag NR) for NIR-II activated PDT/PTT, which could further reprogram the immunosuppressive tumor microenvironment and sensitize tumors to immune checkpoint blocking (ICB) antibodies, especially aCTLA4, for synergistic antitumor treatment.81 The surface plasmon resonance (SPR) band of Au/Ag NRs could be adjusted to the NIR-II region by tuning the thickness of the Ag shell. The Au/Ag NRs could generate hyperthermia and ROS under 1064 nm laser irradiation and consequently trigger the immunogenic cell death (ICD) of cancer cells by increasing the T cell invasion of tumors, converting the cold tumor microenvironment into a hot microenvironment. Finally, Au/Ag NR-mediated NIR-II PDT/PTT synergized with ICB antibodies showed a powerful immunological memory result, preventing tumor recurrence.
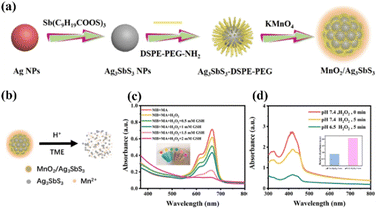 |
| Fig. 4 (a) Schematic diagram of MnO2/Ag3SbS3 preparation. (b) Ag3SbS3 and Mn2+ were released from MnO2/Ag3SbS3 under acid stimulation of the tumor microenvironment. (c) ˙OH generated from MA nanoparticles was detected by MB. (d) 1O2 generation by MA nanoparticles at different pH values. Reproduced with permission from ref. 80. Copyright 2022, American Chemical Society. | |
3.3. Transition metal chalcogenides
Due to their narrow band gap, transition metal chalcogenides show a strong harvest in the NIR range and have been applied extensively in photocatalysis as well as phototherapy (PDT and PTT).82,83 As shown in Fig. 5a, Wang et al. used Fe–Co layered double hydroxides (LDHs) as precursors to prepare the Z-scheme nanostructure FeS2/CoS2@PEG (FCs@PEG).84 The Z-scheme mechanism endows FCs@PEG with a high redox potential of photogenerated charge to oxidize water into O2 and subsequently capture O2 to produce ˙O2− (Fig. 5b). Importantly, FCs@PEG exhibited significant NIR-II absorption and an excellent photothermal conversion efficiency of 50.5% upon 1064 nm irradiation. Fig. 5 shows that the high temperature provides additional energy to achieve the co-excitation of CoS2 (1.37 eV) and FeS2 (1.16 eV) through 1064 nm laser irradiation (1.16eV). Moreover, the as-fabricated FCs@PEG exhibited improved simulated CAT and peroxidase (POD) activities due to the lower resistance for charge transfer in the heterostructure, efficiently catalyzing endogenous H2O2 into O2 and ˙OH, thereby alleviating hypoxia (Fig. 5c). Finally, the tumor treated with FCs@PEG and NIR-II light was almost ablated, demonstrating the excellent synergistic antitumor effect of PDT/PTT.
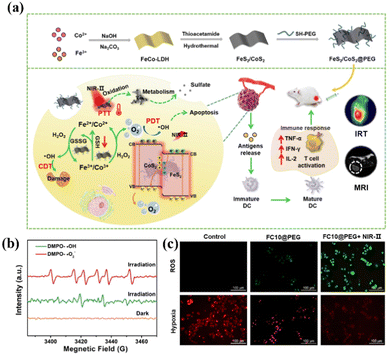 |
| Fig. 5 (a) Schematic illustration of the synthesis of FeS2/CoS2 nanosheets using Fe–Co LDHs as the precursor. (b) ESR spectra of FCs@PEG trapped by DMPO under 1064 nm irradiation. (c) Detection of ROS production with DCFH-DA. Reproduced with permission from ref. 84. Copyright 2022, Elsevier. | |
3.4. Transition metal oxides
Semiconductor nanomaterials based on transition metal oxides have garnered extensive attention due to their tunable localized surface plasmon resonance (LSPR).85 Considerable efforts have been made using plasmonic semiconductor nanomaterials for NIR-II light-activated PDT. Zhu and co-authors discovered a tumor-targeting ZrO2-based phototherapeutic agent to achieve NIR-II PA imaging-guided PTT/PDT synergistic phototherapy, where boron-doped O2-deficient zirconia (ZrO2−x-B) was formed via NaBH4 reduction, and was functionalized further with a layer of SiO2 covalently linked with hyaluronic acid (HA), termed as ZrO2−x-B@SiO2-HA (Fig. 6a).86 The ZrO2−x-B@SiO2-HA agent exhibited full spectral absorption properties due to the oxygen vacancies and boron doping (Fig. 6b), enabling high NIR-II photothermal conversion and significant ROS generation (Fig. 6c). Fig. 6d illustrates the mechanism of NIR-II light-induced ROS production, in which the light firstly irradiates the ZrO2−x-B@SiO2-HA to produce pairs of electrons and holes. Notably, the oxygen vacancies can be used as trapping sites of photogenerated electrons to enhance charge separation. Then, the generated electrons and holes can combine with water and O2 on the surface of ZrO2−x-B to generate ˙O2− and ˙OH, respectively. The mechanism was further confirmed using ESR spectroscopy, as shown in Fig. 6e, which shows the presence of oxygen vacancies in ZrO2−x-B@SiO2-HA. Furthermore, the decoration of HA made ZrO2−x-B@SiO2-HA with a water dispersion ability as well as a targeting ability toward CD44 overexpressed on tumor cells. Hence, the as-prepared ZrO2−x-B@SiO2-HA reveals accurate NIR-II laser-activated cancer-targeting PTT/PDT.
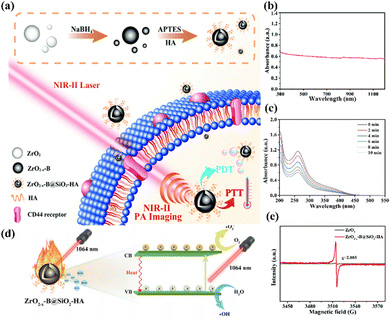 |
| Fig. 6 (a) Schematic of the fabrication of ZrO2−x-B@SiO2-HA for NIR-II PA imaging-mediated tumor-targeting therapy. (b) UV-vis-NIR spectrum of ZrO2−x-B@SiO2-HA. (c) Nitro blue tetrazolium was employed to test ˙O2− of ZrO2−x-B@SiO2-HA under 1064 nm laser irradiation. (d) Schematic of the PTT/PDT mechanism of ZrO2−x-B@SiO2-HA. (e) ESR detected the oxygen vacancies of ZrO2−x-B@SiO2-HA. Reproduced with permission from ref. 86. Copyright 2020, Royal Society of Chemistry. | |
In addition to ZrO2, oxygen-deficient molybdenum oxide nanoparticles (MoOx NPs) can also be utilized in PTT/PDT synergistic therapy because the LSPR of the MoOx NPs can be regulated to the NIR window by adjusting its chemical composition.87–89 Yin et al. reported PEGylated MoOx nanoparticles (PEG-MoOx NPs), which were prepared through a facile hydrothermal method.90 The PEG-MoOx NPs exhibited extensive absorption in the NIR region and a significant photothermal conversion efficiency under both 808 nm and 1064 nm light irradiation. Interestingly, the PEG-MoOx NPs could only conduct limited PTT of cancer cells when exposed to 808 nm irradiation, whereas under irradiation at 1064 nm the PEG-MoOx NPs could not only efficiently convert NIR light into heat but also were sensitized to form ROS. In recent years, oxygen-deficient cerium oxide (CeO2−x) has also been studied in the field of NIR-II PDT where the introduction of oxygen vacancies can reduce the band gap of CeO2−x and enable its optical absorption located in the NIR region. Li et al. first used CeO2−x with plenty of oxygen vacancies as a single NIR-II light-triggered nanotheranostic for sonodynamic therapy (SDT)-enhanced phototherapy.91 CeO2−x, with surface crystalline disorder, exhibited a broad absorption in the NIR-II region. In addition, CeO2−x contains a large number of oxygen defects, which can facilitate the separation of holes and electrons under ultrasound, significantly enhancing the efficacy of its photothermal and photodynamic capabilities. In vitro and in vivo experiments confirmed that CeO2−x@HA showed an outstanding therapeutic effect of SDT-enhanced PDT/PTT upon NIR-II laser irradiation, realizing complete tumor ablation with good biocompatibility.
3.5. Upconversion nanoparticles
Rare-earth doped upconversion nanoparticles (UCNPs) have emerged as one of the most promising inorganic nanomedicines for cell labeling, bioimaging, and cancer therapy owing to their intrinsic upconversion luminescence (UCL) properties of low-energy NIR light into high-energy ultraviolet or visible light, excellent photostability, and low toxicity.92–94 In particular, compared with carbon-based materials and organic fluorophores, UCNPs are highly suitable as NIR-II photosensitizers because they are inherently activated by NIR laser irradiation. Recently, Zhang and colleagues fabricated a NIR-II-excited therapeutic agent formed of ytterbium (Yb)- and erbium (Er)-doped titanium dioxide nano-shovel/quercetin/l-arginine (TiO2@UCN/Qr/LA) on a titanium bone implant (Fig. 7a), in which the doped rare-earth elements enabled up-conversion to generate ROS upon illumination with a 1060 nm laser.95 Encouragingly, the introduction of rare-earth elements not only enhanced the optical absorption and reduced the band gap but also produced abundant oxygen vacancies to promote the separation of electrons and holes, thus improving the photocatalytic performance and the resultant ROS generation ability (e.g., 1O2 and ˙OH) of the TiO2@UCN/Qr/LA under NIR-II irradiation (Fig. 7b and c). More intriguingly, the generated ROS further catalyzed LA to NO to promote therapy. Both in vitro and in vivo evaluations demonstrated that TiO2@UCN/Qr/LA could ablate tumors and eliminate biofilms via NIR-II PDT combined with other therapies.
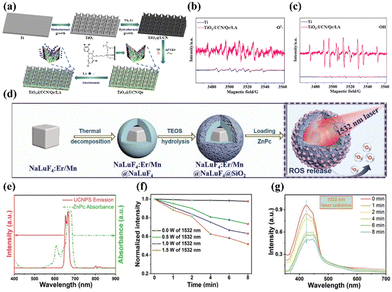 |
| Fig. 7 (a) Preparation process of the TiO2@UCN/Qr/LA nano-shovel on the Ti implant. Detection of (b) 1O2 and (c) ˙OH from Ti and TiO2@UCN/Qr/LA with DMPO. Reproduced with permission from ref. 95. Copyright 2022 Elsevier. (d) Preparation of Er-sensitized UCNPs and the process of activating PDT with 1532 nm light. (e) Normalized absorbance spectrum of ZnPc and UCL spectrum of the UCNPs. (f) ROS generation via irradiation at different light intensities. (g) ROS release with 1532 nm laser radiation. Reproduced with permission from ref. 96. Copyright 2022, John Wiley & Sons Inc. | |
Besides, to solve the problem that the excitation band of the upconversion material is limited to short wavelengths (<1000 nm) and thus expand its application in the biomedical field, Bi et al. introduced Er3+ as a sensitizer to construct novel Er/Mn co-doped NIR-II photo-responsive UCNPs, which were further loaded with the zinc phthalocyanine (ZnPc) photosensitizer (Fig. 7d).96 NIR-II-excited PDT can be easily achieved via a typical Förster resonance energy transfer (FRET) process in which energy can be efficiently transferred from the UCNPs to the ZnPc, because the emission spectrum of the Er-sensitized UCNPs overlaps well with the absorbance band of ZnPc (Fig. 7e). Subsequent experimental results demonstrated that a significant ROS signal can be detected upon 1532 nm laser irradiation, and the intensity was related to the laser power density (Fig. 7f and g). In addition, by tuning the concentration of Mn2+ in the Er/Mn co-doped UCNPs, their crystal phase, size, and emitting colour were readily manipulated. Subsequently, the inert shell of the Er/Mn co-doped UCNP can overcome surface defects, further enhancing its red UCL intensity by ∼8-fold. In short, this work successfully achieved NIR-II light-activated red upconversion bioimaging and PDT through the loading of the ZnPc photosensitizer for the first time, providing a novel method for the application of NIR-II light-activated single-band red emissive UCNPs in biomedicine.
3.6. Aggregation-induced emission molecules
So far, several conventional organic molecules, such as squaric acid and cyanine dyes, have been exploited as NIR-II photosensitizers.97,98 However, these conventional organic dyes suffer from fluorescence quenching effects upon aggregation to form nanoparticles with poor photostability, which significantly impede their wider bio-applications.99,100 Inspiringly, over the past two decades, molecules with aggregation-induced emission (AIE) characteristics that exhibit unique luminescence enhancement properties, due to the restriction of molecular motion in the aggregated state, have attracted great attention in the field of biological imaging.101–103 More importantly, their light-triggered ROS-generating ability is substantially improved in the aggregated state, making AIE molecules promising photosensitizers for PDT applications.104,105 However, the absorption and emission of most AIE molecules are in the visible-light range, which severely limits their practical application in deep tissue therapy. Fortunately, AIE molecules with favourable two-photon absorption features can be excited by concurrently absorbing two long-wavelength photons, thus providing great prospects for building AIE photosensitizers for NIR-II PDT.106 For example, Wang et al. designed an AIE photosensitizer (TQ-BTPE) with long two-photon absorption and significant 1O2 production for NIR-II PDT (Fig. 8a).107 TQ-BTPE displayed bright NIR two-photon fluorescence (about 700 nm) and satisfactory 1O2 generation upon excitation at the NIR-II wavelength of 1200 nm. Noticeably, TQ-BTPE exhibited a much greater 1O2 production ability than that of chlorin e6 (Ce6), suggesting its efficient two-photon photosensitization (Fig. 8b). This work exhibits the first example of two-photon activated organic photosensitizers for NIR-II PDT, and provides new insights for the development of NIR-excited photosensitizers.
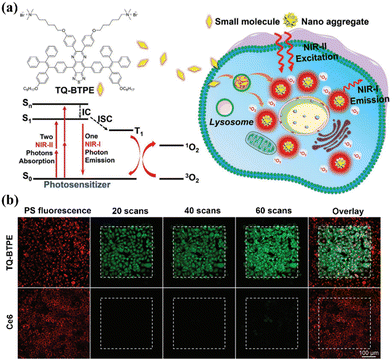 |
| Fig. 8 (a) Chemical structure of TQ-BTPE and schematic of the NIR-II light-activated two-photon photodynamics used to kill tumor cells. (b) TQ-BTPE and Ce6 were irradiated with an NIR-II laser to generate 1O2 in cells, with scanning at different time points. Reproduced with permission from ref. 107. Copyright 2020, John Wiley & Sons Inc. | |
In addition, the construction of a donor–acceptor (D–A) structure is one of the most effective methods to design photosensitizers with long-wavelength absorption, where the strong intramolecular charge transfer in the D–A structure will promote a redshifted absorption and enhance the resultant long-wavelength two-photon absorption.108–110 For instance, Luo's group synthesized an AIE photosensitizer (TPBPy) with a donor–π–receptor (D–π–A) core structure for NIR-II PDT.111 On the one hand, the D–π–A structure could be beneficial for redshifting the absorption peak, endowing the TPBPy with an NIR-II light excitation capability. Furthermore, the conjugated system could reduce the energy gap considerably between the singlet excited state and the triplet excited state, further improving the ISC process and significantly enhancing the 1O2 production ability. To this end, tumor growth was greatly inhibited after treatment via TPBPy-mediated NIR-II two-photon PDT.
3.7. Organic semiconducting polymers
Compared with traditional small-molecule-based photosensitizers, organic semiconducting polymers (OSPs) have unique advantages of high photostability, flexible optical properties, and good biocompatibility due to the entirely organic constituents, which make OSPs more promising for in vivo phototherapy.112–115 For instance, Wen et al. constructed three semiconducting polymers, i.e., PTS, PTSe, and PTTe, that showed strong absorption in the NIR-II region through different D–A molecular structures, in which thiophenoindole (T) served as the strong electron-absorbing unit and thiophene (S), selenophenol (Se) or benzene telluride (Te) acted as the donor unit, respectively (Fig. 9).116 More attractively, by introducing heavy atoms (selenium and tellurium) into the molecular skeleton, the rate constant of ISC (kISC) was improved markedly to generate triplet excitons, which is favorable for yielding ROS. Besides, the Gibbs free energy change between PTS, PTSe, or PTTe and 3O2 was less than zero, which facilitated type-I PDT via intermolecular electron transfer. In addition, the PTTe nanoparticles efficiently produced more ˙O2− and a higher photothermal conversion efficiency than the other two nanoparticles upon NIR-II light irradiation, showing outstanding type-I PDT synergetic therapy performance both in vitro and in vivo.
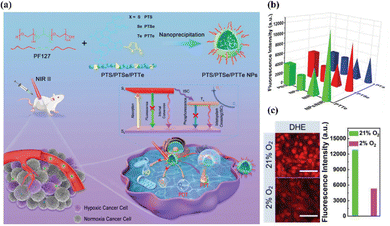 |
| Fig. 9 (a) Synthesis of PTS, PTSe, and PTTe nanoparticles, and their application in NIR-II PDT/PTT synergistic cancer therapy under hypoxic conditions. (b) ˙O2− production of different semiconducting polymers with or without the NIR-II irradiation. (c) ˙O2− production of PTTe nanoparticles was detected under normoxia or hypoxic conditions. Scale bar, 100 μm. Reproduced with permission from ref. 116. Copyright 2022, John Wiley & Sons Inc. | |
3.8. Other materials as photosensitizers
In addition to the above-mentioned carbon-based nanomaterials, noble metals, transition metal chalcogenides, transition metal oxides, UCNPs, AIE molecules, and semiconducting polymers, there are some other NIR-II photosensitizers, such as two-dimensional layered double hydroxides (LDHs) and mesoporous materials. As a typical two-dimensional nanomaterial, LDHs have a large specific surface area and unique physical and chemical properties. In recent years, due to their adjustable chemical composition and good biocompatibility, LDHs have attracted widespread research attention in the biomedical field.117 Yang and co-authors coupled Lactobacillus acidophilus probiotics with CoCuMo-LDH nanosheets (LA and LDH) to construct tumor-microenvironment-responsive photosensitizers for accurate NIR-II PDT.118 Specifically, the hypoxia tropism properties of LA endow LA and LDH with excellent tumor targeting. In addition, the low pH (∼5.4) of LA metabolites and GSH transform the CoCuMo-LDH nanosheets into amorphous states, significantly improving the production of 1O2 upon 1270 nm laser irradiation, with a quantum yield of 0.75/1.06. This work provides an excellent strategy for the in situ activation of photosensitizers to achieve accurate NIR-II PDT.
Mesoporous materials, mesoporous silicas in particular, are used widely in anti-tumor drug-delivery studies due to their porous structure and surface area, easy surface functionalization, and pH response degradation.119,120 Very recently, some studies have shown that mesoporous nanoparticles can be used in NIR-II PDT. Wan et al. obtained ER-HMCu2−XS/CXB by modifying hollow mesoporous Cu2−XS with p-toluenesulfonamide (for endoplasmic reticulum (ER) targeting) and loading it with a vascular inhibitor (celecoxib; CXB).121 The as-prepared ER-HMCu2−XS showed good synergistic treatment of NIR-II-mediated PDT/PTT as well as induced ICD for triple-negative breast cancer ablation, which also greatly inhibited cancer recurrence. In another study by Zhang et al., black mesoporous titanium (H-TiO2) was first loaded with chloroperoxidase (CPO) and β-lapachone (β-Lap), and then encapsulated with polyacrylic acid (PAA), to form TCLP nanocomposites.122 Notably, the H-TiO2 within the TCLP could be used as a NIR-II-activated photothermal agent and photosensitizer, while the β-Lap could enhance the 1O2 formation from CPO due to an increase in H2O2. As a result, the TCLP mediated the production of plenty of ROS and significantly overcame heat shock protein (HSP)-dependent tumor resistance, thereby improving the synergistic anti-tumor effect of NIR-II PTT/PDT.
4. Conclusion and outlook
As a promising cancer treatment modality, PDT, with non-invasiveness and remarkable therapeutic selectivity, has been developed significantly and implemented in clinical practice. However, so far, most traditional photosensitizers are still excited by short-wavelength visible light, which not only substantially discounts their therapeutic performance but also severely limits their widespread use, especially for the treatment of deep tumors. Recently, great progress has been made in NIR-II-activated photosensitizers that use a long-wavelength light from 1000 to 1700 nm for PDT, and these exhibit minimal scattering loss, deeper tissue penetration, an improved photon-capturing ability, and satisfactory biosafety. In this review, we describe the latest principles of the photosensitizer mechanism, and, for the first time, summarize the various types of NIR-II-excited photosensitizer as well as their applications in cancer therapy.
Despite the encouraging features of NIR-II PDT for cancer treatment, such cutting-edge therapeutic technology is still in its infancy, and several major concerns remain to be resolved for further clinical applications (Fig. 10).
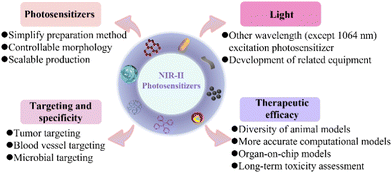 |
| Fig. 10 Typical ongoing challenges for enhancing the clinical translation of NIR-II photosensitizers. | |
(1) In the clinical transformation of NIR-II photosensitizers, the complex synthesis steps required not only lead to increased production costs but also increase the toxicity of the photosensitizers, and there are still problems regarding their large-scale and repeatable synthesis.123 Moreover, the instability of nanomaterials requires strict storage conditions, which also hinders the clinical application of NIR-II photosensitizer-based nanomedicines. In addition, the morphology, size and surface charge of nanomaterials have important effects on blood circulation, tumor penetration and accumulation. Therefore, simple preparation methods, controllable morphologies, and the large-scale production of NIR-II photosensitizer molecules or nanomaterials are particularly important and require further exploration.
(2) NIR-II photosensitizers have poor specificity for tumors, which may lead to low treatment efficiencies and potential damage to other healthy tissues. Several studies have shown that the tumor microenvironment promotes the development of malignant tumors and becomes a potential therapeutic target. Researchers have designed new targeted antitumor photosensitizers that are based on the general physiological features of the tumor microenvironment. However, pH values, enzymes, and reducing agents in clinical applications may change, leading to poor responsiveness and low sensitivity.124 Following the in-depth study of tumors, strategies based on blood-vessel-targeted anticancer therapy are being developed. Photosensitizers can be selectively accumulated in the tumor vasculature by targeting blood vessels, where photosensitizers are more likely to obtain oxygen to alleviate the hypoxia of PDT, and achieve tumor-specific killing by causing vessel constriction and thrombus. However, tumor specificity is not only related to the type of target but also relies heavily on the enhanced permeability and retention (EPR) effect, which is greatly influenced by the physical and chemical properties of the photosensitizers. Recently, several studies have utilized the living attributes of microorganisms to develop new specific anti-tumor approaches. Compared with traditional therapies, treatments using microorganisms and their derivatives have the advantages of reduced systemic toxicity, active mobility, precise accurate tumor self-propulsion and penetration, and flexible adaptation to the tumor microenvironment to evoke innate immunity.125,126 Nevertheless, excessive immune responses can also lead to inflammation; thus, it is necessary to balance appropriate immune responses with severe inflammation. Despite all efforts to improve the aggregation of photosensitizers at the tumor site, tumor-specific therapy remains in the early stages. In the future, more efforts should be made to develop different targeting strategies through pathological studies according to the heterogeneity of the disease; in addition, multiple targeting strategies may also improve the anti-tumor efficiency.
(3) Due to difficulties in the preparation of photosensitizers excited at a specific range of wavelengths and the high expense of NIR-II-related instruments, currently, most NIR-II photosensitizers still use excitation at the 1064 nm wavelength, ignoring the study of other wavelength excitation photosensitizers. Therefore, the joint efforts of researchers in various fields to develop photosensitizers that are excited by other wavelengths and the relevant equipment are both required. In addition, recent evidence suggests that NIR-II light has a lower phototoxicity, a higher maximum permissible exposure (MPE), and a deeper tissue-penetration depth. However, the lower energy of the NIR-II photons results in less efficient ROS generation. Therefore, it is urgent to develop photosensitizers with a high light-conversion efficiency. Moreover, research into the antitumor effects of NIR-II photosensitizers remains in its infancy, and the phototoxicity of NIR-II light laser needs to be evaluated further in clinical applications.
(4) In preclinical studies, mouse xenograft cancer models are commonly used, which are significantly different from real human biological and physiological parameters, leading to failures in clinical translation. In order to better evaluate the clinical treatment effects, the following methods can be used. Firstly, the selection of large animal models such as primates should be added to in vivo evaluation studies. Secondly, a better computational model could more accurately evaluate drug accumulation and pharmacokinetics, effectively assessing differences between the preclinical and clinical efficacy. Furthermore, organ-on-chip models are another method to address the poor in vitro–in vivo correlation of photosensitizers, which is similar to human physiological functions, thus obtaining more accurate drug response data.127,128 Finally, the long-term chronic toxicity of NIR-II photosensitizers is a key issue that affects their clinical application, and needs further evaluation.
Based on the above discussion, current high-efficiency NIR-II photosensitizers remain scarce, and will bring new hotspots to phototherapy research. Besides, the clinical application of NIR-II PDT still needs to be explored further, which requires favourable collaboration between interdisciplinary fields, including chemistry, materials science, nanoscience, and pharmaceuticals. In short, we believe that rapid progress on NIR-II-activated photosensitizers will advance new horizons in precision medicine, especially for the accurate treatment of cancer.
Conflicts of interest
There are no conflicts to declare.
Acknowledgements
This work was funded by the National Natural Science Foundation of China (No. 32001010) and the Beijing Institute of Technology Science, Technology Innovation Plan Project (2022CX01029), and The Young Elite Scientist Sponsorship Program of Beijing Association for Science and Technology (No. BYESS2021162). J. Z. would like to thank Biological & Medical Engineering Core Facilities (Beijing Institute of Technology) for providing advanced equipment.
References
- L. Huang, S. Zhao, F. Fang, T. Xu, M. Lan and J. Zhang, Biomaterials, 2021, 268, 120557 CrossRef CAS PubMed.
- Y. Zhang, F. Fang, L. Li and J. Zhang, ACS Biomater. Sci. Eng., 2020, 6, 4816–4833 CrossRef CAS PubMed.
- J. Chen, T. Fan, Z. Xie, Q. Zeng, P. Xue, T. Zheng, Y. Chen, X. Luo and H. Zhang, Biomaterials, 2020, 237, 119827 CrossRef CAS PubMed.
- C. Liang, X. Zhang, Z. Wang, W. Wang, M. Yang and X. Dong, J. Mater. Chem. B, 2020, 8, 4748–4763 RSC.
- C. Hopper, Lancet Oncol., 2000, 1, 212–219 CrossRef CAS PubMed.
- R. Vankayala and K. C. Hwang, Adv. Mater., 2018, 30, 1706320 CrossRef PubMed.
- Y. Wan, G. Lu, J. Zhang, Z. Wang, X. Li, R. Chen, X. Cui, Z. Huang, Y. Xiao, J. Chelora, W. Zhang, Y. Liu, M. Li, H.-Y. Xie and C.-S. Lee, Adv. Funct. Mater., 2019, 29, 1903436 CrossRef.
- J. Zhang, F. Fang, B. Liu, J.-H. Tan, W.-C. Chen, Z. Zhu, Y. Yuan, Y. Wan, X. Cui, S. Li, Q.-X. Tong, J. Zhao, X.-M. Meng and C.-S. Lee, ACS Appl. Mater. Interfaces, 2019, 11, 41051–41061 CrossRef CAS.
- F. Fang, S. Wang, Y. Song, M. Sun, W.-C. Chen, D. Zhao and J. Zhang, Nat. Commun., 2023, 14, 1660 CrossRef CAS.
- T. Yano, T. Minamide, K. Takashima, K. Nakajo, T. Kadota and Y. Yoda, J. Clin. Med., 2021, 10, 2785 CrossRef CAS.
- R. R. Allison, G. H. Downie, R. Cuenca, X.-H. Hu, C. J. H. Childs and C. H. Sibata, Photodiagn. Photodyn. Ther., 2004, 1, 27–42 CrossRef CAS.
- R. R. Allison and C. H. Sibata, Photodiagn. Photodyn. Ther., 2010, 7, 61–75 CrossRef CAS.
- S. Das, M. Tiwari, D. Mondal, B. R. Sahoo and D. K. Tiwari, J. Mater. Chem. B, 2020, 8, 10897–10940 RSC.
- S. Yin, J. Song, D. Liu, K. Wang and J. Qi, Molecules, 2022, 27, 6649 CrossRef CAS PubMed.
- H. Dai, Q. Shen, J. Shao, W. Wang, F. Gao and X. Dong, Innovation, 2021, 2, 100082 CAS.
- X. Zhao, J. Liu, J. Fan, H. Chao and X. Peng, Chem. Soc. Rev., 2021, 50, 4185–4219 RSC.
- L. Zhu, M. Luo, Y. Zhang, F. Fang, M. Li, F. An, D. Zhao and J. Zhang, Coord. Chem. Rev., 2023, 475, 214875 CrossRef CAS.
- Y. Liu, J. Huang and J. Liu, J. Mater. Chem. B, 2022, 10, 7760–7771 RSC.
- P. Ran, T. Xia, H. Zheng, F. Lei, Z. Zhang, J. Wei and X. Li, Acta Biomater., 2023, 155, 292–303 CrossRef CAS PubMed.
- M. Qiu, D. Wang, H. Huang, T. Yin, W. Bao, B. Zhang, Z. Xie, N. Xie, Z. Wu, C. Ge, Q. Wang, M. Gu, H. L. Kutscher, L. Liu, S. Bao, P. N. Prasad and H. Zhang, Adv. Mater., 2021, 33, 2102562 CrossRef CAS.
- Y. Yu, S. Wu, L. Zhang, S. Xu, C. Dai, S. Gan, G. Xie, G. Feng and B. Z. Tang, Biomaterials, 2022, 280, 121255 CrossRef CAS PubMed.
- X. Li, F. Fang, B. Sun, C. Yin, J. Tan, Y. Wan, J. Zhang, P. Sun, Q. Fan, P. Wang, S. Li and C.-S. Lee, Nanoscale Horiz., 2021, 6, 177–185 RSC.
- X. Cui, G. Lu, S. Dong, S. Li, Y. Xiao, J. Zhang, Y. Liu, X. Meng, F. Li and C.-S. Lee, Mater. Horiz., 2021, 8, 571–576 RSC.
- Y. Wan, G. Lu, W.-C. Wei, Y.-H. Huang, S. Li, J.-X. Chen, X. Cui, Y.-F. Xiao, X. Li, Y. Liu, X.-M. Meng, P. Wang, H.-Y. Xie, J. Zhang, K.-T. Wong and C.-S. Lee, ACS Nano, 2020, 14, 9917–9928 CrossRef CAS PubMed.
- X. Cui, J. Zhang, Y. Wan, F. Fang, R. Chen, D. Shen, Z. Huang, S. Tian, Y. Xiao, X. Li, J. Chelora, Y. Liu, W. Zhang and C.-S. Lee, ACS Appl. Bio Mater., 2019, 2, 3854–3860 CrossRef CAS PubMed.
- J. Zhang, W. Chen, R. Chen, X.-K. Liu, Y. Xiong, S. V. Kershaw, A. L. Rogach, C. Adachi, X. Zhang and C.-S. Lee, Chem. Commun., 2016, 52, 11744–11747 RSC.
- M. Tavakkoli Yaraki, B. Liu and Y. N. Tan, Nanomicro Lett., 2022, 14, 123 Search PubMed.
- J. Xie, Y. Wang, W. Choi, P. Jangili, Y. Ge, Y. Xu, J. Kang, L. Liu, B. Zhang, Z. Xie, J. He, N. Xie, G. Nie, H. Zhang and J. S. Kim, Chem. Soc. Rev., 2021, 50, 9152–9201 RSC.
- B. Sun, J. N. Bte Rahmat and Y. Zhang, Biomaterials, 2022, 291, 121875 CrossRef CAS PubMed.
- Y. Qian, J. Wang, W. Bu, X. Zhu, P. Zhang, Y. Zhu, X. Fan and C. Wang, Biomater. Sci., 2023, 11, 704–718 RSC.
- X. Li, J. F. Lovell, J. Yoon and X. Chen, Nat. Rev. Clin. Oncol., 2020, 17, 657–674 CrossRef.
- C. Yin, X. Lu, Q. Fan and W. Huang, View, 2021, 2, 20200070 CrossRef CAS.
- G. Hong, A. L. Antaris and H. Dai, Nat. Biomed. Eng., 2017, 1, 1–22 CrossRef.
- X. Zhang, L. An, Q. Tian, J. Lin and S. Yang, J. Mater. Chem. B, 2020, 8, 4738–4747 RSC.
- F. Fang, L. Zhu, M. Li, Y. Song, M. Sun, D. Zhao and J. Zhang, Adv. Sci., 2021, 8, 2102970 CrossRef CAS.
- D. Yang, G. Yang, J. Li, S. Gai, F. He and P. Yang, J. Mater. Chem. B, 2017, 5, 4152–4161 RSC.
- F. Fang, Y. Yuan, Y. Wan, J. Li, Y. Song, W.-C. Chen, D. Zhao, Y. Chi, M. Li, C.-S. Lee and J. Zhang, Small, 2022, 18, 2106215 CrossRef CAS.
- Y. Jiang, P. K. Upputuri, C. Xie, Y. Lyu, L. Zhang, Q. Xiong, M. Pramanik and K. Pu, Nano Lett., 2017, 17, 4964–4969 CrossRef CAS.
- Q. Shen, S. Wang, N.-D. Yang, C. Zhang, Q. Wu and C. Yu, J. Lumin., 2020, 225, 117338 CrossRef CAS.
- X. Ding, C. H. Liow, M. Zhang, R. Huang, C. Li, H. Shen, M. Liu, Y. Zou, N. Gao, Z. Zhang, Y. Li, Q. Wang, S. Li and J. Jiang, J. Am. Chem. Soc., 2014, 136, 15684–15693 CrossRef CAS.
- H. Lin, Z. Lin, K. Zheng, C. Wang, L. Lin, J. Chen and J. Song, Adv. Optical Mater., 2021, 9, 2002177 CrossRef CAS.
- Q. Xin, H. Ma, H. Wang and X.-D. Zhang, Exploration, 2023, 20220011 CrossRef.
- K. Wanderi and Z. Cui, Exploration, 2022, 2, 20210097 CrossRef.
- T. Shi, C. Huang, Y. Li, F. Huang and S. Yin, Biomaterials, 2022, 285, 121535 CrossRef CAS.
- Y. Ma, Y. Zhang, X. Li, Y. Zhao, M. Li, W. Jiang, X. Tang, J. Dou, L. Lu, F. Wang and Y. Wang, ACS Nano, 2019, 13, 11967–11980 CrossRef CAS PubMed.
- R. Tian, W. Sun, M. Li, S. Long, M. Li, J. Fan, L. Guo and X. Peng, Chem. Sci., 2019, 10, 10106–10112 RSC.
- D. An, J. Fu, B. Zhang, N. Xie, G. Nie, H. Ågren, M. Qiu and H. Zhang, Adv. Funct. Mater., 2021, 31, 2101625 CrossRef CAS.
- C. Xu and K. Pu, Chem. Soc. Rev., 2021, 50, 1111–1137 RSC.
- Y. Liu, Y. Li, S. Koo, Y. Sun, Y. Liu, X. Liu, Y. Pan, Z. Zhang, M. Du, S. Lu, X. Qiao, J. Gao, X. Wang, Z. Deng, X. Meng, Y. Xiao, J. S. Kim and X. Hong, Chem. Rev., 2022, 122, 209–268 CrossRef CAS.
- B. Li, M. Zhao, J. Lin, P. Huang and X. Chen, Chem. Soc. Rev., 2022, 51, 7692–7714 RSC.
- S. He, J. Song, J. Qu and Z. Cheng, Chem. Soc. Rev., 2018, 47, 4258–4278 RSC.
- Q. Qu, Z. Zhang, X. Guo, J. Yang, C. Cao, C. Li, H. Zhang, P. Xu, Z. Hu and J. Tian, J. Nanobiotechnol., 2022, 20, 143 CrossRef CAS.
- Z. Hu, C. Fang, B. Li, Z. Zhang, C. Cao, M. Cai, S. Su, X. Sun, X. Shi, C. Li, T. Zhou, Y. Zhang, C. Chi, P. He, X. Xia, Y. Chen, S. S. Gambhir, Z. Cheng and J. Tian, Nat. Biomed. Eng., 2020, 4, 259–271 CrossRef.
- X. Shi, Z. Zhang, Z. Zhang, C. Cao, Z. Cheng, Z. Hu, J. Tian and N. Ji, IEEE Trans. Biomed. Eng., 2022, 69, 1889–1900 Search PubMed.
- C. S. Foote, Photochem. Photobiol., 1991, 54, 659 CrossRef CAS.
- M. R. Younis, G. He, J. Qu, J. Lin, P. Huang and X.-H. Xia, Adv. Sci., 2021, 8, 2102587 CrossRef CAS PubMed.
- M. Lan, S. Zhao, W. Liu, C.-S. Lee, W. Zhang and P. Wang, Adv. Healthcare Mater., 2019, 8, 1900132 CrossRef.
- Q. Yao, J. Fan, S. Long, X. Zhao, H. Li, J. Du, K. Shao and X. Peng, Chem, 2022, 8, 197–209 CAS.
- M. Hassan, V. G. Gomes, A. Dehghani and S. M. Ardekani, Nano Res., 2018, 11, 1–41 CrossRef CAS.
- L. D. Dias, H. H. Buzzá, M. D. Stringasci and V. S. Bagnato, Photochem, 2021, 1, 434–447 CrossRef.
- R. G. Mendes, A. Bachmatiuk, B. Büchner, G. Cuniberti and M. H. Rümmeli, J. Mater. Chem. B, 2013, 1, 401–428 RSC.
- L. D. Dias and I. S. Mfouo-Tynga, Small, 2020, 5, 53 CAS.
- S. Masoudi Asil, E. D. Guerrero, G. Bugarini, J. Cayme, N. De Avila, J. Garcia, A. Hernandez, J. Mecado, Y. Madero, F. Moncayo, R. Olmos, D. Perches, J. Roman, D. Salcido-Padilla, E. Sanchez, C. Trejo, P. Trevino, M. Nurunnabi and M. Narayan, View, 2023, 4, 20220056 CrossRef CAS.
- J. Du, N. Xu, J. Fan, W. Sun and X. Peng, Small, 2019, 15, 1805087 CrossRef.
- B.-P. Jiang, B. Zhou, Z. Lin, H. Liang and X.-C. Shen, Chem. – Eur. J., 2019, 25, 3993–4004 CrossRef CAS.
- L. Wang, K. Kang, Y. Ma, F. Zhang, W. Guo, K. Yu, K. Wang, F. Qu and H. Lin, Chem. Eng. J., 2022, 444, 136512 CrossRef CAS.
- S. Iravani and R. S. Varma, Chem. Commun., 2022, 58, 7336–7350 RSC.
- H. Lin, S. Gao, C. Dai, Y. Chen and J. Shi, J. Am. Chem. Soc., 2017, 139, 16235–16247 CrossRef CAS PubMed.
- H. Xiang, H. Lin, L. Yu and Y. Chen, ACS Nano, 2019, 13, 2223–2235 CAS.
- Z. Guo, S. Chen, Z. Wang, Z. Yang, F. Liu, Y. Xu, J. Wang, Y. Yi, H. Zhang, L. Liao, P. K. Chu and X.-F. Yu, Adv. Mater., 2017, 29, 1703811 CrossRef.
- Y. Zhang, S. Li, X. Fang, B. Miao, Y. Wang, J. Liu, G. Nie and B. Zhang, Nanophotonics, 2022, 11, 5189–5204 CrossRef CAS.
- R. R. Arvizo, S. Bhattacharyya, R. A. Kudgus, K. Giri, R. Bhattacharya and P. Mukherjee, Chem. Soc. Rev., 2012, 41, 2943–2970 RSC.
- M. Kim, J.-H. Lee and J.-M. Nam, Adv. Sci., 2019, 6, 1900471 CrossRef.
- D. Yang, F. Deng, D. Liu, B. He, B. He, X. Tang and Q. Zhang, Asian J. Pharm., 2019, 14, 349–364 Search PubMed.
- P. K. Jain, X. Huang, I. H. El-Sayed and M. A. El-Sayed, Acc. Chem. Res., 2008, 41, 1578–1586 CrossRef CAS PubMed.
- N. Kuthala, M. Shanmugam, X. Kong, C.-S. Chiang and K. C. Hwang, Nanoscale Horiz., 2022, 7, 589–606 RSC.
- Y. Huang, L. Dai, L. Song, L. Zhang, Y. Rong, J. Zhang, Z. Nie and T. Chen, ACS Appl. Mater. Interfaces, 2016, 8, 27949–27955 CrossRef CAS.
- J. Hu, Z. Wang and J. Li, Sensors, 2007, 7, 3299–3311 CrossRef CAS.
- Q. Chen, J. Chen, Z. Yang, L. Zhang, Z. Dong and Z. Liu, Nano Res., 2018, 11, 5657–5669 CrossRef CAS.
- Q. Wang, B. Qu, J. Li, Y. Liu, J. Dong, X. Peng and R. Zhang, ACS Appl. Mater. Interfaces, 2022, 14, 4980–4994 CrossRef CAS.
- L. Jin, S. Shen, Y. Huang, D. Li and X. Yang, Biomaterials, 2021, 268, 120582 CrossRef CAS.
- Y. Zhao, S.-B. Wang, A.-Z. Chen and R. K. Kankala, Coord. Chem. Rev., 2022, 472, 214765 CrossRef CAS.
- Z. Bao, X. Liu, Y. Liu, H. Liu and K. Zhao, Asian J. Pharm., 2016, 11, 349–364 Search PubMed.
- L. Wang, K. Kang, H. Hou, Y. Ma, K. Yu, F. Qu and H. Lin, J. Colloid Interface Sci., 2022, 625, 145–157 CrossRef CAS PubMed.
- W. A. Murray and W. L. Barnes, Adv. Mater., 2007, 19, 3771–3782 CrossRef CAS.
- C. Zhu, Z. Ding, Z. Guo, X. Guo, A. Yang, Z. Li, B.-P. Jiang and X.-C. Shen, Biomater. Sci., 2020, 8, 6515–6525 RSC.
- G. Song, J. Shen, F. Jiang, R. Hu, W. Li, L. An, R. Zou, Z. Chen, Z. Qin and J. Hu, ACS Appl. Mater. Interfaces, 2014, 6, 3915–3922 CrossRef CAS.
- G. Song, J. Hao, C. Liang, T. Liu, M. Gao, L. Cheng, J. Hu and Z. Liu, Angew. Chem. Int. Ed., 2016, 55, 2122–2126 CrossRef CAS.
- Y. Wang, N. Gong, Y. Li, Q. Lu, X. Wang and J. Li, J. Am. Chem. Soc., 2020, 142, 1735–1739 CrossRef CAS.
- W. Yin, T. Bao, X. Zhang, Q. Gao, J. Yu, X. Dong, L. Yan, Z. Gu and Y. Zhao, Nanoscale, 2018, 10, 1517–1531 RSC.
- J. Li, H.-L. Peng, C. Wen, P. Xu, X.-C. Shen and C. Gao, Langmuir, 2022, 38, 5502–5514 CrossRef CAS.
- H. S. Naher, B. A. H. Al-Turaihi, S. H. Mohammed, S. M. Naser, M. A. Albark, H. A. Madlool, H. A. M. Al- Marzoog and A. Turki Jalil, J. Drug Delivery Sci. Technol., 2023, 80, 104175 CrossRef CAS.
- R. Rafique, S. K. Kailasa and T. J. Park, Trends Analyt. Chem., 2019, 120, 115646 CrossRef.
- J. F.-C. Loo, Y.-H. Chien, F. Yin, S.-K. Kong, H.-P. Ho and K.-T. Yong, Coord. Chem. Rev., 2019, 400, 213042 CrossRef CAS.
- G. Zhang, Z. Wu, Y. Yang, J. Shi, J. Lv, Y. Fang, Z. Shen, Z. Lv, P. Li, X. Yao, W. Chen, X. Wei, P. K. Chu and X. Zhang, Chem. Eng. J., 2022, 428, 131155 CrossRef CAS.
- S. Bi, Z. Deng, J. Huang, X. Wen and S. Zeng, Adv. Mater., 2023, 35, 2207038 CrossRef CAS.
- K. Wang, Y. Xu, Z. Chen, H. Li, R. Hu, J. Qu, Y. Lu and L. Liu, Nanophotonics, 2022, 11, 5089–5100 CrossRef CAS.
- H. Bian, D. Ma, X. Zhang, K. Xin, Y. Yang, X. Peng and Y. Xiao, Small, 2021, 17, 2100398 CrossRef CAS.
- S. Wang, X. Wang, L. Yu and M. Sun, Photodiagn. Photodyn. Ther., 2021, 34, 102254 CrossRef CAS.
- W. Park, S. Cho, J. Han, H. Shin, K. Na, B. Lee and D.-H. Kim, Biomater. Sci., 2018, 6, 79–90 RSC.
- J. Luo, Z. Xie, J. W. Y. Lam, L. Cheng, H. Chen, C. Qiu, H. S. Kwok, X. Zhan, Y. Liu, D. Zhu and B. Z. Tang, Chem. Commun., 2001, 1740–1741 RSC.
- Z. Zhuang, F. Bu, W. Luo, H. Peng, S. Chen, R. Hu, A. Qin, Z. Zhao and B. Z. Tang, J. Mater. Chem. C, 2017, 5, 1836–1842 RSC.
- Y. Li, Z. Zhao, J. Zhang, R. T. K. Kwok, S. Xie, R. Tang, Y. Jia, J. Yang, L. Wang, J. W. Y. Lam, W. Zheng, X. Jiang and B. Z. Tang, Adv. Funct. Mater., 2018, 28, 1804632 CrossRef.
- Z. Zhao, H. Zhang, J. W. Y. Lam and B. Z. Tang, Angew. Chem., Int. Ed., 2020, 59, 9888–9907 CrossRef CAS PubMed.
- Y. Wang, S. Xu, L. Shi, C. Teh, G. Qi and B. Liu, Angew. Chem., Int. Ed., 2021, 60, 14945–14953 CrossRef CAS PubMed.
- S. Wang, X. Li, S. Y. Chong, X. Wang, H. Chen, C. Chen, L. G. Ng, J.-W. Wang and B. Liu, Adv. Mater., 2021, 33, 2007490 CrossRef CAS PubMed.
- S. Wang, H. Chen, J. Liu, C. Chen and B. Liu, Adv. Funct. Mater., 2020, 30, 2002546 CrossRef CAS.
- J.-S. Ni, P. Zhang, T. Jiang, Y. Chen, H. Su, D. Wang, Z.-Q. Yu, R. T. K. Kwok, Z. Zhao, J. W. Y. Lam and B. Z. Tang, Adv. Mater., 2018, 30, 1805220 CrossRef PubMed.
- Y. Gao, G. Feng, T. Jiang, C. Goh, L. Ng, B. Liu, B. Li, L. Yang, J. Hua and H. Tian, Adv. Funct. Mater., 2015, 25, 2857–2866 CrossRef CAS.
- J. Zhang, W. Chen, S. Kalytchuk, K. F. Li, R. Chen, C. Adachi, Z. Chen, A. L. Rogach, G. Zhu, P. K. N. Yu, W. Zhang, K. W. X. Shi, Z. Zhang, Z. Zhang, C. Cao, Z. Cheng, Z. Hu, J. Tian and N. Ji, IEEE Trans. Biomed. Eng., 2022, 69, 1889–1900 Search PubMed.
- Z. He, Y. Gao, H. Zhang, Y. Xue, F. Meng and L. Luo, Adv. Healthcare Mater., 2021, 10, 2101056 CrossRef CAS.
- N. Yu, L. Zhao, D. Cheng, M. Ding, Y. Lyu, J. Zhao and J. Li, J. Colloid Interface Sci., 2022, 619, 219–228 CrossRef CAS.
- C. Yin, X. Li, G. Wen, B. Yang, Y. Zhang, X. Chen, P. Zhao, S. Li, R. Li, L. Wang, C.-S. Lee and L. Bian, Biomaterials, 2020, 232, 119684 CrossRef CAS PubMed.
- C. Yin, X. Zhen, Q. Fan, W. Huang and K. Pu, ACS Nano, 2017, 11, 4174–4182 CrossRef CAS.
- J. Li, J. Rao and K. Pu, Biomaterials, 2018, 155, 217–235 CrossRef CAS.
- K. Wen, H. Tan, Q. Peng, H. Chen, H. Ma, L. Wang, A. Peng, Q. Shi, X. Cai and H. Huang, Adv. Mater., 2022, 34, 2108146 CrossRef CAS PubMed.
- J. Wen, K. Yang, J. Huang and S. Sun, Mater. Des., 2021, 198, 109298 CrossRef CAS.
- Y. Yang, T. Hu, Y. Bian, F. Meng, S. Yu, H. Li, Q. Zhang, L. Gu, X. Weng, C. Tan and R. Liang, Adv. Mater., 2023, 35, 2211205 CrossRef CAS.
- S. L. Suib, Chem. Rec., 2017, 17, 1169–1183 CrossRef CAS PubMed.
- V. Cauda and G. Canavese, Pharmaceutics, 2020, 12, 1108 CrossRef.
- G. Wan, X. Chen, J. Chen, R. Gou, H. Wang, S. Liu, M. Zhang, H. Chen, D. Wang and Q. Zhang, Biomater. Sci., 2023, 11, 1876–1894 RSC.
- W. Zhang, M. Wang, B. Liu, M. Yuan, Z. Yang, J. Tan, P. A. Ma and J. Lin, Chem. Eng. J., 2023, 460, 141818 CrossRef CAS.
- M. Souri, M. Soltani, F. Moradi Kashkooli, M. Kiani Shahvandi, M. Chiani, F. S. Shariati, M. R. Mehrabi and L. L. Munn, Mater. Today Bio., 2022, 13, 100208 CrossRef CAS.
- D. Rosenblum, N. Joshi, W. Tao, J. M. Karp and D. Peer, Nat. Commun., 2018, 9, 1410 CrossRef PubMed.
- Q.-W. Chen, J.-Y. Qiao, X.-H. Liu, C. Zhang and X.-Z. Zhang, Chem. Soc. Rev., 2021, 50, 12576–12615 RSC.
- W. Wu, Y. Pu, S. Gao, Y. Shen, M. Zhou, H. Yao and J. Shi, Nanomicro Lett., 2022, 14, 220 CAS.
- B. Zhang, A. Korolj, B. F. L. Lai and M. Radisic, Nat. Rev. Mater., 2018, 3, 257–278 CrossRef.
- J. Zhu, L. Ji, Y. Chen, H. Li, M. Huang, Z. Dai, J. Wang, D. Xiang, G. Fu, Z. Lei and X. Chu, Cell Death Discovery, 2023, 9, 72 CrossRef.
|
This journal is © The Royal Society of Chemistry 2023 |