DOI:
10.1039/D2TB02596E
(Review Article)
J. Mater. Chem. B, 2023,
11, 2830-2851
Smart microneedle patches for wound healing and management
Received
29th November 2022
, Accepted 24th January 2023
First published on 14th March 2023
Abstract
The number of patients with non-healing wounds is generally increasing globally, placing a huge social and economic burden on every country. The complexity of the wound-healing process remains a major health challenge despite the numerous studies that have been reported on conventional wound dressings. Therefore, a therapeutic system that combines diagnostic and therapeutic modalities is essential to monitor wound-related biomarkers and facilitate wound healing in real time. Microneedles, as a multifunctional platform, are promising for transdermal diagnostics and drug delivery. Their advantages are mainly reflected in painless transdermal drug delivery, good biocompatibility, and ease of self-administration. In this work, we review recent advances in the use of microneedle patches for wound healing and monitoring. The paper first provides a brief overview of the skin structure and the wound healing process, and then discusses the current state of research and prospects for the development of wound-related biomarkers and their real-time monitoring based on microneedle sensors. It summarizes the current state of research based on the unique design of microneedle patches, including biomimetic, conductive, and environmentally responsive, to achieve wound healing. It further summarizes the prospects for the application of different microneedle-based drug delivery modalities and drug delivery substances for wound healing, due to their superior transdermal drug delivery advantages. It concludes with challenges and expectations for the use of smart microneedle patches for wound healing and management.
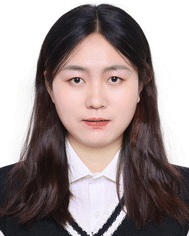
Fangfang Hu
| Fangfang Hu holds a BS degree in pharmaceutical engineering from Northwestern Normal University in 2021. She is a graduate student in biomedical engineering at the School of Life at Northwestern Polytechnical University. Her current interests include microneedling patches and wound repair materials. |
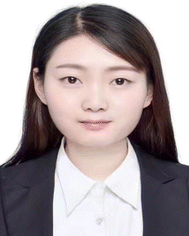
Qian Gao
| Qian Gao was born in Shaanxi Province, China. She holds a master's degree in pharmacology from Southwest Medical University. During her master's degree, she studied at the Cardiovascular Research Center on diabetes-related glucose regulation. She is currently a PhD candidate in biomedical engineering at Northwestern Polytechnical University. Her main research interests are metal–organic frameworks, hydrogels, molecular biology and biochemistry. |
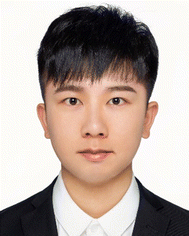
Jinxi Liu
| Jinxi Liu is a graduate student in the School of Life Sciences, Northwestern Polytechnical University. He graduated from Huaqiao University with his BS degree in 2019. His current research interests include the development of novel aggregation-induced luminescent molecules for use in disease marker diagnosis and phototherapy. |
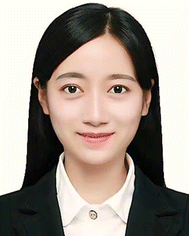
Wenting Chen
| Wenting Chen is a graduate student in the School of Life Sciences, Northwestern Polytechnical University. She graduated from Xi’an University with her BS degree in 2020. Her current research interests include the design and synthesis of multifunctional nanostructures for cancer therapy, focusing on photodynamic therapy and chemodynamic therapy. |
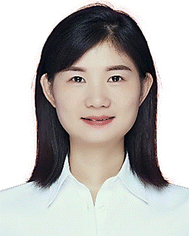 Caiyun Zheng | Caiyun Zheng holds a master's degree in biology from Jiangsu University of Science and Technology. During her master's degree, she studied algae genetic engineering at the Institute of Oceanology, Chinese Academy of Sciences. Currently, she is a PhD student in Biomedical Engineering at Northwestern Polytechnic University. Her research interests are porous nanomaterials, hemostatic materials, and photocatalytic traumatic antibacterial gel materials. |
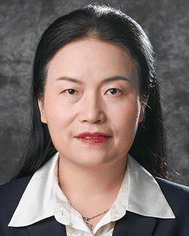
Tingli Lu
| Tingli Lu is a professor at Northwestern Polytechnical University (China). She received her PhD (2007) in Materialogy from Northwestern Polytechnical University, China. Since 2013, she has been working at the School of Life sciences, Northwestern Polytechnical University. Her research focuses on biological 3D printing, self-healing hydrogels, and medicinal carrier materials. |
1. Introduction
Skin wounds have a substantial negative impact on healthcare systems and economies worldwide. On the one hand, wound management is a core issue in clinical care due to the constant occurrence of traumatic injuries and the growing prevalence of chronic wounds such as diabetic ulcers and pressure sores.1 The increased prevalence also brings huge economic costs. First, diabetic foot ulcers are one of the most chronic wounds, with a lifetime risk of developing foot ulcers of up to 25%.2,3 The healthcare costs for diabetic ulcers and amputations in the UK in 2014–2015 were estimated to be between £837 million and £962 million.4 On the other hand, low healing rates exacerbate the problem.5 In the real world, cure rates for complex patients are reported to be no higher than 40%, which contradicts the reported rates almost close to 100%.6
While treatments including oxygen, growth factors, small drug molecules, autologous and stem cells, gene therapy and tissue engineering have been used to promote wound healing, to modulate various physiological processes, recent reports have pointed to an important factor-point delivery.7,8 In general, chronic wounds are covered with a layer of hard skin and necrotic tissue, and the various enzymes contained in the wound exudate locally flush out the therapeutic drugs, which greatly reduces their bioavailability.7,9 Microneedle patches with micro-nanostructures enable targeted therapeutic drug delivery to living tissues for optimal therapeutic results.10 The wound healing phase requires care to ensure a better healing state, mainly because the healing process is influenced by a variety of factors including environment, age, nutrition, and medications.11 Wounds are in an environment where both internal and external environments work together, and these factors affect the healing process.12 Limited by the subjective experience of traditional visual assessment of wounds, the specific conditions of wounds cannot be accurately reflected.13 For early diagnosis, it is necessary to determine wound characteristics including, most importantly, biomarkers, and to examine the wound environment to monitor the healing process.
In recent years, many wearable devices have been applied for wound monitoring to help understand the wound environment in real time by taking wound environment markers as input.11 Therefore, the new type of wound dressing should simultaneously satisfy real-time monitoring, and early diagnosis, and help achieve accurate treatment. In addition to being developed as a drug delivery system, a significant advantage of microneedle devices is their ability to accommodate multiple independent sensors on a single small array to provide timely multiplexed molecular information.14 Thus, the concept of microneedle patches combining diagnostic and therapeutic modalities in a therapeutic system to achieve real-time monitoring of wound-related biomarkers and promote wound healing was further elaborated (Fig. 1). Thus, the concept of microneedle patches combining diagnostic and therapeutic modalities in a therapeutic system to achieve real-time monitoring of wound-related biomarkers and promote wound healing is further elaborated. In this review, we describe the skin structure and wound healing process. Then the current research status and development prospects of microneedle sensor-based wound-related biomarkers and their real-time monitoring are discussed. The status of research on microneedle patches based on unique designs, including bionic, conductive, and environmentally responsive, for wound healing is summarized. The prospects of different microneedle-based drug delivery methods and drug delivery substances for wound healing are further summarized. It concludes with challenges and expectations for the use of smart microneedle patches for wound healing and management.
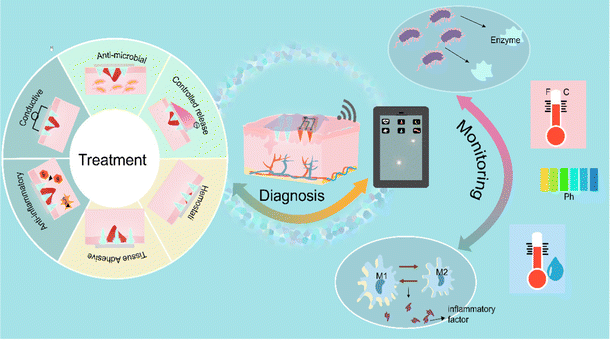 |
| Fig. 1 Schematic diagram of wound intelligent management microneedle patches. Smart microneedle patches combine treatment and monitoring for real-time diagnosis. To summarize, they have five therapeutic modalities: antibacterial, anti-inflammatory, hemostatic, tissue adhesion, and controlled-release drug release. From the monitoring perspective, microneedle patches enable the monitoring of relevant markers such as temperature, humidity, pH, inflammatory factors, and enzymes produced by bacteria. | |
2. Skin structure and the wound healing process
2.1 Skin structure
The human skin is a multilayered structure that primarily consists of the epidermis, dermis, and subcutaneous tissue.15 The epidermis, mainly composed of cuticles, is the outermost layer of skin. It could resist environmental disturbances such as ultraviolet radiation, pathogenic bacteria, and uncontrollable mechanical damage.16 It can also prevent dehydration by regulating moisture.15 The dermis, a thick, collagen-rich connective tissue, is the close-by layer. The extracellular matrix (ECM), living cells, nerve endings, and blood vessels that are abundant in the dermis support the skin's structural integrity, elasticity, and nutrition.16,17 The communication between the epidermis and dermis could establish, maintain, and restore tissue homeostasis.18 The deepest layer is subcutaneous tissue. It is mainly composed of vascularized loose peri areolar connective tissue and adipose tissue,19 which provides thermal isolation and mechanical protection to the body.16 A schematic diagram of the skin structure is shown in Fig. 2A.
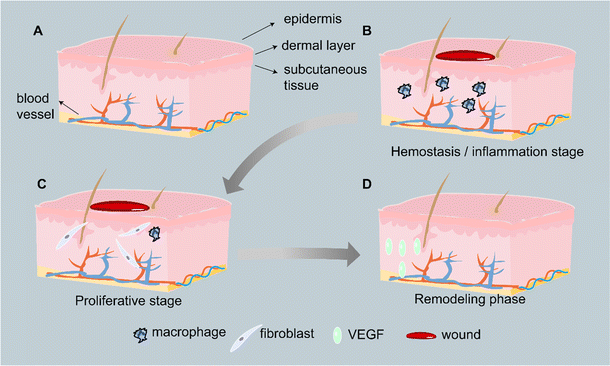 |
| Fig. 2 Wound healing stage. (A) Skin structure is mainly divided into three layers: epidermis, dermis, and subcutaneous tissue. (B) Hemostatic/inflammatory stage. (C) Proliferation stage. (D) Remodeling stage. | |
2.2 Wound healing process
The normal wound healing process has three stages and is a complex and dynamic process. The first stage is hemostasis and inflammation, which occurs immediately after tissue damage (Fig. 2B). Hemostasis begins with platelet degranulation and the coagulation cascade, culminating in the formation of a hemostatic fibrin clot.20 Fibrin clots then become a scaffold for the infiltration of inflammatory cells such as neutrophils and macrophages, which then migrate to the wound site to remove pathogens (such as bacteria) and cell/tissue debris.21 In order to stimulate fibroblasts and epithelial cells to migrate towards the wound site, macrophages also release cytokines such as TGF-α.22
The proliferative phase starts after the inflammatory phase and occurs after about 4–21 days (Fig. 2C).23,24 During this phase, dermal and epidermal cells migrate to and hyperproliferate at the wound site, with the typical events of regenerative epithelialization, collagen deposition, angiogenesis, and formation of granulation tissue.23 Regenerative epithelialization is the process of establishing an intact epidermis to repair skin damage. This involves the migration and proliferation of keratin-forming cells within the trabecular bed, the differentiation of new epithelial cells into a stratified epidermis, and the restoration of the intact basement membrane zone between the epidermis and dermis.25 Angiogenesis is a process of budding the preexisting blood vessels near the wound, involving endothelial cells migration, proliferation, and branching for the formation of new blood vessels.25 Based on newly formed blood vessels, proliferating fibroblasts invade the clot to form constricted granulation tissue.26
The longest reconstruction phase usually lasts from a few weeks to a few months and is the ultimate stage of wound healing (Fig. 2D).21 At this stage, cell proliferation and protein synthesis slow down, and collagen is remodeled into organized fibrils.6,21 Type III collagen is gradually being replaced with type I collagen.6
Wounds can be divided into acute wounds and chronic wounds according to their stage of healing. Acute wounds undergo the normal wound healing process. In contrast, chronic wounds undergo a disturbed wound repair phase that manifests as a persistent inflammatory phase.27 They may last for weeks, months, or even years and the wounds are highly susceptible to bacterial infections such as Staphylococcus aureus, Pseudomonas aeruginosa, Escherichia coli, and methicillin-resistant Staphylococcus aureus (MRSA) that can form biofilms at the wound site.28 Pathological features of chronic wounds include prolonged exposure to pro-inflammatory cytokines, imbalance in the expression of proteases and their inhibitors, altered availability and activity of growth factors, tissue hypoxia and high levels of oxidative stress.29 It is worth noting that chronic wounds are often associated with diseases such as diabetes, obesity, etc. Among the major problems of chronic wounds, diabetes is the most threatening.30 Angiogenesis plays a crucial role in wound healing by providing nutrients and oxygen and by removing metabolic waste products.31 In the high glucose environment caused by diabetes, vascular endothelial cells are damaged by oxidative stress, resulting in low cellular activity and reduced proliferation capacity, which delays diabetic wound healing.32 Achieving rapid healing of diabetic wounds is a pressing issue.
3. Microneedle patch for wound monitoring
3.1 Physiological data for monitoring the wound status
It is significant to monitor wound status, which is beneficial to predict the wound trends for real-time treatment.33,34 The physiological processes involved in wound healing are intricate. It is also susceptible to environmental factors that can impede or prolong healing, which may contribute to high treatment costs.13 Wound dressings that are tailored according to a specific healing situation based on the stage of healing, inflammatory state, moisture levels, and exudation rates are currently used to treat chronic wounds.35,36 However, the majority of the time, wound status is assessed through visual inspection, which carries the risk of secondary injury due to frequent replacement and visual errors.13,37 Next-generation wound dressings that are capable of real-time monitoring, early diagnosis, and on-demand therapy have received a lot of attention to overcome the wound-healing process's “black box” status.38–40 Injured tissue generates exudate, a fluid rich in proteins such as electrolytes, creatinine, fibrinogen, matrix metalloproteinases (MMPs) and c-reactive protein (CRP), and tumor necrosis factor-alpha (TNF-α).41,42 Additionally, certain physical wound environment factors, such as temperature, pH, humidity, and exercise, influence wound healing.43–48 To achieve early diagnosis of wounds, physiological data related to the wound environment become the main target of monitoring.
During the process of wound healing, pH is an important parameter. Intact skin has a pH of 4.8–6.0.49,50 Once stratum corneum is damaged, the underlying tissue is exposed, displaying a normally regulated pH of 7.4. On around day 2, a normally healing wound goes through a brief acidic period with a pH between 4 and 6.5, which helps prevent bacterial growth and promotes angiogenesis and epithelialization.49–51 After a transient decrease in pH, it returns to a neutral pH along with the wound closure.51 In the meantime, the newly formed skin recovers an acidic milieu. However, the pH tends to remain in a weakly alkaline range at the chronic wound as well as the wound with long-term bacterial colonization.51,52 Another crucial parameter in the wound-healing process is temperature. Inflammation or infection may be the cause of a sudden rise in temperature at the site of the wound.49 A decrease in temperature at the wound may be caused by ischemia, which tends to hamper wound healing.50 It has been demonstrated that a temperature change of approximately 2 °C is the threshold for wound deterioration.50 Accordingly, temperature monitoring is generally employed as a potential method of estimating wound status. An inflammatory marker linked to the onset of inflammatory conditions and infection is C-reactive protein (CRP).53 CRP concentrations in the blood suddenly rise from approximately 0.8 mg L−1 to 600–1000 mg L−1 during the acute phase response to inflammation, peaking after approximately 48 h.42,54 A biomarker known as urea (UA) has a strong connection to wound healing.55 Associated with oxidative stress and bacterial infection in the wound area, uric acid levels rise in unhealed chronic wounds and fall as the wound heals.42,56,57 Wound moisture is a key parameter in ensuring optimal healing conditions in wound care.47,58 However, moisture-induced infection might increase the exuding of tissue fluid, rendering wounds to be too wet and thus aggravating wound infection.47,58 Consequently, moisture monitoring is also important for wounds treated with moist dressings.47,58 In addition, studies have reported that glucose,59–61 inflammatory factors,62 exercise,63etc. can be used as indicators to monitor wound healing.
3.2 Microneedle-based sensors
As the microneedle patches only penetrate the viable stratum corneum and viable epidermis and do not reach the nerve endings or blood vessels, they are minimally invasive procedures and therefore patients do not feel any pain.64,65 The microneedle technology is a transdermal drug delivery strategy that overcomes the disadvantages of hypodermic needles, reduces the risk of infection transmission, reduces anxiety, and allows patient compliance.66 Microneedle patches have shown high therapeutic advantages in aspects such as immunotherapy,65 cancer,67 Alzheimer's disease,68–71 drug delivery,72 and disease diagnosis.73,74 By combining electrodes with micronized array substrates, a variety of microneedle-based devices were proposed as sensors and diagnostic systems,75 including electrochemical, optical, magnetic, and paper-based biosensors.76 Microneedle sensors can be hollow microneedles with sensors on top, electrochemically bonded hollow microneedles, surface-functionalized solid microneedles, or metallized solid microneedles.77 Colorimetry, immunoassays, nucleic acid recognition, or electrochemistry can all be used to detect analytes with these microneedle-based biosensors.77 Among the existing research reports, electrochemical sensors account for the vast majority. The advantages are small size, speed, low cost, low power consumption, and no sample composition interference.14 Thanks to the superior biocompatibility and customizable microstructure of microneedle patches, innovative microneedle-based dressings are likely to be widely used in the future.78 Rongyan He and his team placed sensing reagents at the tips of microneedle patches to fabricate a colorimetric skin tattoo biosensor fabricated from a four-region segmented microneedle patch, realizing the detection of four typical biomarkers (pH, glucose, temperature, and uric acid) at the same time. Biosensors change color as markers change, allowing further semi-quantitative analysis by naked eye reading or smart camera capture.79 Researchers have recently developed smart colorimetric microneedle patches containing Fe ion-gallate coordination polymer nanodots (FNDs) for on-demand treatment of infected wounds and real-time reporting of these wounds (Fig. 3A).80 FDNs have pH-dependent catalase-mimicking activity, and can not only kill bacteria by catalyzing the generation of hydroxyl radicals (OH) from hydrogen peroxide, but also change color depending on pH and hydrogen peroxide. By observing the change in color of the microneedle patch, it is possible to distinguish between normal wounds and bacterially infected wounds.80 A colorimetric dermal tattoo biosensor fabricated from microneedle patches was developed for monitoring pH, glucose, uric acid, and temperature. The microneedle biosensor exhibits color changes in response to changes in the concentration of the marker allowing for visual semi-quantitative analysis.79
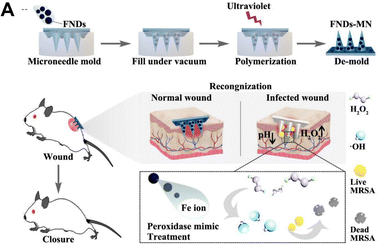 |
| Fig. 3 (A) Schematic diagram of how the ligand polymer nanoparticle enzyme integrated colorimetric microneedle patches are made and how they are used to detect and treat wound infections.80 Copyright 2022 Elsevier. | |
Microneedle patch sensors offer significant advantages in drug delivery and sensing physiological signals. For example, microneedle patches can increase permeability and can facilitate the delivery of various drugs. It has also been demonstrated that the same microneedle-based sensor prevents sweat contamination and signal noise during sensing while also minimizing damage to dermal nerves and blood vessels.81 For wound healing and sensing, an origami smart silk fibroin-based microneedle dressing (i-SMD) with an inverse opal photonic crystal (IO PC) structure, microfluidic channels, and microcircuits was proposed (Fig. 4A).82 By using capillary force, the microfluidic channels can direct the liquid flow to the area of detection, making it possible to detect biomarkers in liquid without the need for an external power supply.82 Microelectronic circuits can monitor activity in the trauma area and reduce secondary injuries caused by exercise. Among them, the temperature-responsive N-isopropyl acrylamide hydrogel realizes intelligent drug release. On the microneedle patch, a cross-shaped microfluidic channel is made, and the four regions of the channel have IO PC structures.82 Capillary force allows the liquid to spontaneously diffuse along the microfluidic channel after the smart patch is applied to the wound, bringing it into complete contact with the IO PC structure in the detection area. The relative levels of biomarkers in wound secretions can be read simply by detecting the fluorescent signal in the detection area. The intelligent microneedle patch integrating motion sensing, intelligent drug release, and wound biochemical analysis can realize intelligent management of wounds and provide a reference for management of other diseases. The article proposes that the microneedle patch can be used to analyze a variety of biochemical indicators at the wound healing stage, but the way to achieve this is to directly use a solution containing a high concentration of target molecules, which still has a certain gap with the actual demand.
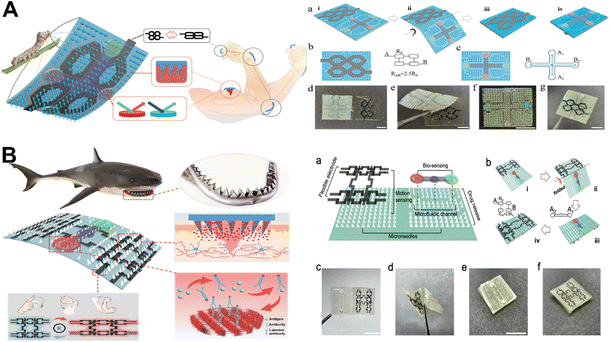 |
| Fig. 4 (A) Diagram of the highly integrated, intelligent, biocompatible, stretchable i-SMD for biochemical sensing, motion monitoring, and wound healing.82 Copyright 2020 Wiley-VCH. (B) Diagrammatic representation of a microneedle patches based on shark teeth for intelligent wound management.83 Copyright 2021 American Chemical Society. | |
The team proposed a microneedle patch for smart wound management inspired by the flat and angled structure of a shark's tooth to further enhance the feasibility of smart wound management (Fig. 4B).83 It was worth noting that the bionic shark teeth enabled the microneedle patches to penetrate the skin with improved grasping force. The microneedle patch was used for the analysis of the effect of IL-6 on inflammatory reaction at the wound site. Interestingly, IL-6 levels increased significantly from day 0 to day 3 and continued to decline from day 3 to day 9 due to treatment, consistent with normal wound healing changes.83
4. Microneedle design for wound healing
4.1 Biomimetic design
Many aspects of wound healing have been studied with microneedle patches. However, as some microneedle patches have poor adhesion to the skin, they require auxiliary equipment such as medical tape to achieve optimal penetration and fixation.84,85 The straight and symmetrical structure of the microneedle patches may be the main reason. Inspired by bionics, the researchers have further improved the structure of microneedle patches in order to improve their adhesion and avoid secondary cracking. For example, a microneedle patch with a claw-like tip, inspired by an eagle's claw, enables stable skin adhesion, and promotes wound healing (Fig. 5A).86 In addition, shark teeth-style patches are inspired by a shark's ability to bite and hold on to their prey, with their microneedle patches being designed to resemble the flat, angled structure of shark teeth.87 North American porcupines are known for their hair or feathers with tiny, backward-stretching barbs for self-defense, and inspire the new structural design of microneedle patches.88 Lamprey teeth-inspired microneedle patches are used for directional traction to shrink the wound area.89 Currently, it is still challenging to fabricate microneedle patches with complex microscopic features using traditional fabrication methods. Daehoon Han et al. proposed a 4D-printed microneedle patch with biomimetic reverse-curved barbs to enhance tissue adhesion. The results showed that the tissue adhesion of the prepared microneedle patch was 18 times higher than that of the non-barbed microneedle patch.90 Microneedle patches based on the bionic concept can be stabilized by 4D printing technology.
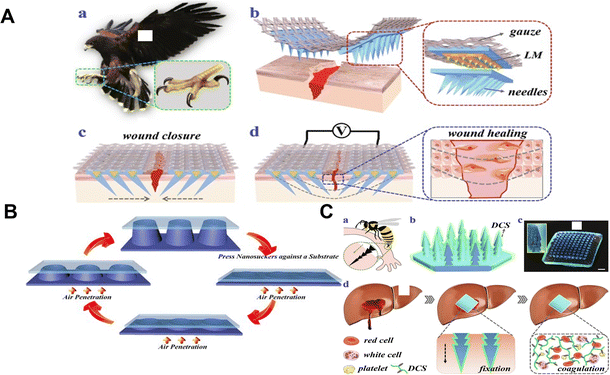 |
| Fig. 5 (A) Schematic illustration of the claw-inspired microneedle patch with liquid metal encapsulation and its wound healing application.86 Copyright 2021 Elsevier. (B) Schematic diagram of the working principle of the microneedle patch and the equipment process.91 Copyright 2017 American Chemical Society. (C) Schematic diagram of the preparation of a hemostatic and bionic pagoda microneedle patch.99 Copyright 2021 Elsevier. | |
A biphasic microneedle patch with shape-variably expanded microneedle tips was developed to mechanically interlock with tissue. The endoparasite Pomphorhynchus laevis, which swells its proboscis to attach to the intestinal wall of its host, served as inspiration for the insertion and fixation of microneedle patches.91 The conical microneedle sheet consists of a poly(styrene) block poly(acrylic) expandable tip and a non-expandable polystyrene core.91 Eun et al., inspired by the endoparasite Pomphorhynchus laevis, created a structured microneedle patch with a cross-linked biphasic structure.92 The microneedle patch comprises an absorbable adhesive external part based on mussel adhesion protein and a rigid internal part based on filament for effective tissue fixation without delamination. The designed novel adhesive microneedle patch achieves mechanical interlocking with tissue and exhibits excellent adhesion on wet and dynamic biological surfaces.92 Considering the effect of humid environments on microneedle patches, Chen designed an octopus nanosuction microneedle patch to exhibit strong adhesion in both dry and humid environments (Fig. 5B).93 The biomimetic microneedle patch can provide universal soft tissue adhesion with minimal damage and provide a platform for bioactive therapeutics, reducing the risk of infection and reducing traumatic removal.
The first stage of wound healing is hemostasis. Wound healing depends on hemostatic agents controlling bleeding quickly and effectively. Although currently available hemostatic materials such as cyanoacrylate,94 glutaraldehyde cross-linked albumin,95 or fibrin-based bandages96 can exert high hemostatic efficiency, they have limitations in the treatment of deep bleeding.96 In addition, hemostatic materials are often difficult to remove, which also brings a lot of trouble to patients.97,98 Therefore, hemostatic materials with high hemostatic efficiency and easy removal have become the focus of researchers to design new hemostatic materials. The layered microstructure of the feet or stings of insects like ladybugs, gadflies, and wasps served as inspiration for Zhang's team. To achieve rapid hemostasis, a novel dodecyl modified chitosan (DCS) coated pagoda shaped microneedle patch was proposed (Fig. 5C).99 The multilayered structure allows the microneedle patch to be firmly anchored to different tissues by physical interlocking, independent of massive blood loss. On the other hand, the DCS coating acts as an anchor on the cell membrane and causes blood cells to coagulate, thus actively promoting hemostasis.99
4.2 Environment-responsive design
It has been demonstrated that drug delivery that is intelligently controlled can prevent drug resistance around the wound caused by uncontrolled drug release. Precise regulation of the time, area, and flow rate of drug release can effectively improve the wound environment.100 Signals that can be responsive include physical and chemical signals, such as light,101–104 electricity,105 magnetism,106 temperature,87,107,108 pH,109 glucose,110 and ROS.111
Near infrared (NIR) light has received attention due to its ease of manipulation, ability to locally focus on a specific site, and its safety, allowing non-invasive propagation in deeper tissues.101,112 Light-activated microneedles perform their functions by converting light into heat and raising the ambient temperature.113 The creation of porous MOF microneedle patches to provide photo-thermally responsive nitric oxide is an example of this (Fig. 6A).114 The use of reactive graphene oxide (GO) encapsulated with an empty copper 1,3,5-tricarboxylate (HKUST-1) MOF enables the controlled release of NO. The photothermal effect of GO leads to a significant increase in the release of NO molecules at high temperatures.114 In the process of wound healing, nitric oxide is primarily responsible for cell proliferation, collagen formation, and wound shrinkage.115 The designed microneedle patch shortens the short half-life of NO. When the microneedle patch is applied to diabetic wounds, the wound closure rate can reach 99% within 13 days.114 In addition, a near-infrared responsive nitric oxide-releasing microneedle was prepared, using nitrosoglutathione (GSNO) as a nitric oxide donor, adding graphene oxide to enhance the photothermal conversion efficiency, and using near-infrared to achieve a controllable release of nitric oxide.116 Adequate oxygenation is important for cell proliferation, bacterial defense, angiogenesis, collagen synthesis, and epithelialization.117,118 Therefore, people also put forward higher requirements for oxygen carriers. Under the condition of meeting biocompatibility, finding a carrier with high oxygen-carrying capacity and controlled release that can act deep in the skin is a hot topic of interest. Zhang and his team have recently reported a microneedle patch that uses near-infrared light to achieve controlled oxygen delivery (Fig. 6B).119 Hemoglobin (Hb) utilizes the excellent photothermal efficiency of black-scale quantum dots (BP QDs) for its reversible oxygen binding capability.120 The two were encapsulated at the tip of a separable microneedle, and the responsive release of oxygen was achieved by using local heat generated from near-infrared light. The microneedle patch showed ideal wound healing ability in the treatment of diabetic mouse wounds.119 By activating the G protein-coupled adenosine receptor, adenosine, a systemic range of active ingredients, can alter cell behavior.121 Recently, several studies have shown that adenosine can promote angiogenesis and endothelial cell proliferation,122,123 exploit the ability of borates to dynamically covalently bind adenosine, combined with the powerful photothermal conversion of two-dimensional layered MXenes.124,125 The controlled release of adenosine is achieved, which promotes wound healing.126
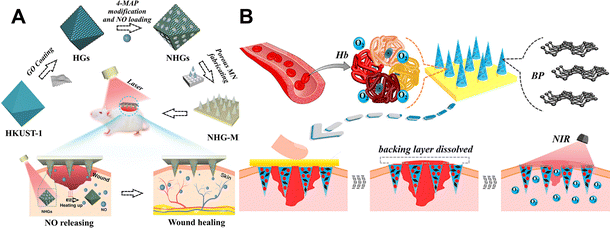 |
| Fig. 6 (A) Schematic diagram of the preparation and application of the porous MOF microneedle patches.114 Copyright 2021 Wiley-VCH. (B) Schematic diagram of using NIR response to rapidly release BP quantum dots and oxygen-carrying hemoglobin to promote wound healing.119 Copyright 2020 American Chemical Society. | |
The human body's normal physiological temperature is 37 °C. The epidermal temperature in the wound area will rise to 37 °C or higher when there is an infection.108 The microneedle patch achieves the release of the vascular endothelial growth factor (VEGF) encapsulated by NIPAM hydrogel.127N-iso-propyl acrylamide (NIPIM) hydrogel is a temperature-sensitive hydrogel. The NIPAM hydrogel has a volume phase transition temperature of 37 °C. At this point, the NIPAM hydrogel shrinks and then releases the encapsulated contents.108,128 Controllable and intelligent drug release is achieved through the temperature increase caused by the inflammatory response at the wound site. The microneedle patch achieves ideal wound healing by promoting inflammation inhibition, collagen deposition, angiogenesis, and tissue regeneration.127 In addition, Wang and his team have achieved controlled drug release from microneedle patches combined with temperature-responsive n-isopropylacrylamide (NIPAM) hydrogels.129 Inverse opal (IO) photonic crystals (PC) have an ordered three-dimensional porous microstructure. Thanks to the porous structure of IOPCs, the drug-carrying capacity of microneedle patches is greatly enhanced. Furthermore, thanks to the temperature-responsive NIPAM hydrogel, the microneedle patch can control drug release.129
The pH of the wound may change in accordance with the type of wound, the stage of the healing process, and other characteristics like infection.130 On a microneedle patch, Asad Ullah and colleagues created a porous polymer coating that automatically ‘releases’ therapeutic drugs according to the pH of the wound (Fig. 7A).131 The pH-sensitive polymer Eudragit S100 is soluble in tissue fluid with an alkaline pH (pH greater than or equal to 7) but insoluble in an acidic microenvironment.132 The test medium contained trace amounts of the microneedle patch released at a pH of 4.5. On the other hand, when the microneedle patch was exposed to wound pH (pH 7.5) conditions, the rate of drug release increased significantly. This study demonstrates the ability of the created microneedle patch to treat contaminated injuries.131
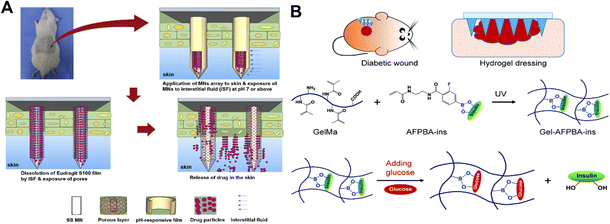 |
| Fig. 7 (A) Wound pH-dependent release system schematic illustration.131 Copyright 2021 Elsevier. (B) Diagram of glucose responsive microneedle dressing for wound healing in diabetes.133 Copyright 2022 Royal Society of Chemistry. | |
The dermal microcirculation is affected when the microneedle patches are inserted into the skin.113 For diabetic wounds, glucose-responsive microneedle patches enable the controlled release of drugs based on glucose concentration. For diabetic wound repair, a hydrogel microneedle patch that responds to glucose and releases insulin was developed (Fig. 7B).133 The glucose-responsive insulin release was achieved using hydrogels with phenylboronic acid (PBA) groups. By promoting angiogenesis, epithelial regeneration, and deposition of the extracellular matrix, insulin can act as a growth-like factor to promote wound healing.134–136 In conclusion, diabetic wounds may heal more quickly with this hydrogel-based microneedle patches.133
4.3 Electroconductive design
Electrical signals are the primary guiding cues for directed cell migration in wound healing.137 Exogenous electrical stimulation is applied to chronic wounds to stimulate electrotaxis (also known as electrotaxis).138 A spontaneous potential of 10–60 mV exists between the epidermis and sub-epidermal layer of undamaged skin.139 Ion channels and frequent depolarization and repolarization of the cells are the main causes of this phenomenon.140 When damaged, a short circuit occurs due to the low resistance of the injured part.141 The net movement of ions in the layers between the epidermis and dermis creates endogenous electric fields (EFs). EFs participate in the cell healing process by affecting cell migration. This phenomenon of cell migration under the action of an endogenous electric field is called electrotaxis.140
To facilitate wound healing, direct cell migration, and encourage cell metabolism, proliferation and differentiation, appropriate electrical stimulation can mimic endogenous wound healing mechanisms.142,143 Using the conductive properties of liquid metal, it is combined with microneedle patches to guide cell migration and accelerate wound healing under an electric field of 2 V cm−1 generated by a lithium-ion battery that can be recharged.86 Recently, a microneedle-based self-powered transcutaneous electrical stimulation system for improving epidermal growth factor pharmacodynamics to promote wound healing has been developed. The system consists of a sliding freestanding frictional electrical nanogenerator and a microneedle patch that enables continuous release of the epidermal growth factor for 24 hours. It is interesting to note that this method converts biomechanical energy generated by finger sliding into biosafe microcurrents.
5. Microneedle-based drug delivery in wound healing
5.1 Delivering antibacterial agents for combating infection
Bacterial infection of wounds is a common and unavoidable challenge in the wound healing process. At the point when a bacterial contamination happens at the injury site, there might be a persevering provocative reaction at the injury site because of the microscopic organisms, drawing out the injury recuperating time. At present, the treatment of bacterial infection mainly relies on various antibiotics, but the problem of bacterial resistance emerges one after another.19 Therefore, how to achieve an efficient sterilization strategy is the focus at present.
It has been found that approximately 75% of chronic wounds suffer from a bacterial infection caused by biofilms produced by bacterial colonization at the wound site.144 Bacterial biofilms are characterized as different bacterial networks joined in total and encompassed by polysaccharides, disciple fimbriae, extracellular DNA, lipids, and proteins; self-blending grids called hydrated extracellular polymers (EPS).145,146 Planktonic bacteria are less resistant to antimicrobial compounds than bacterial biofilms.147 Existing strategies to combat microbial biofilms are limited by the difficulty faced by antimicrobial agents in penetrating their physical barriers.148 EPS' net negative charge could trap positively charged antimicrobials or repel them. Additionally, some bacteria produce enzymes like beta-lactamases, which accumulate within biofilms and aid in bacterial survival by deactivating specific antibiotic molecules.149 Microneedle patches appear to be a viable strategy to penetrate these barriers and deliver the loaded cargo to a predetermined depth of the dermis without causing painful, skin damage.148 We summarize the current status of research on microneedle patch-based delivery of antimicrobial drugs, including delivery drugs, functions, and materials for microneedle preparation as shown in Table 1.
Table 1 Summary of microneedle-based delivery of antibacterial agents to promote wound healing
Delivery substance |
Function |
Preparation method |
Ref. |
Vancomycin |
Figureht skin infections caused by MRSA (methicillin-resistant Staphylococcus aureus) |
Polyvinyl alcohol polymethylmethacrylate |
150
|
Tetracycline |
Provide an appealing approach to preventing infections in the local area and prevent pathogen growth. |
Silk fibroin |
151
|
Bacteriophages |
An effective method of treating biofilm infections of the skin. |
Polyvinyl alcohols |
152
|
Calcium peroxide |
Overcome the bacterial biofilm present in chronic nonhealing wounds and co-deliver oxygen and bactericidal agents |
Polyvinylpyrrolidone |
153
|
Carvacrol |
At infection sites, achieve a lasting antimicrobial effect. |
Poly(caprolactone) |
154
|
Antimicrobial Peptide |
Treatment of biofilms formed by multidrug-resistant bacteria |
Poly(ε-caprolactone) polyvinylpyrrolidone |
144
|
Lysozyme |
Lyse bacterial infections for dermal applications |
Polyvinylpyrrolidone |
155
|
Hyaluronic acid |
Poly lactic-co-glycolic acid |
Erythromycin Azithromycin |
Enhanced wound healing and anti-bacterial effect |
Polyvinylpyrrolidone |
156
|
Hyaluronic acid |
Chloramphenicol |
Improve the delivery of a wide range of antimicrobial agents to biofilm contaminated sites. |
Polyvinylpyrrolidone |
149
|
Gelatin nanoparticles |
Levofloxacin polydopamine nanoparticles |
Combating wound infections-associated bacterial biofilms. |
Polyvinyl alcohol |
157
|
Silver nanoparticles |
Fast dissolution rates and potent antibacterial activity |
Carboxymethylcellulose |
158
|
Silver nanoparticles |
Overcome the problem of skin infection associated with bacterial biofilms |
Polyvinyl alcohol Polyvinylpyrrolidone |
159
|
Zn-MOF |
Dramatically accelerate epithelial regeneration and neovascularization |
Methacrylated hyaluronic acid |
160
|
Zn2+ |
Eradication of bacterial biofilms |
Chitosan |
161
|
Mg and panax notoginseng saponins |
Achieves antibacterial, neovascularization, and activating a benign immune response |
Chitosan polyvinylpyrrolidone |
162
|
5.1.1 Antibacterial drugs.
Antibiotics are still the main strategy for treating clinical wound infection. The researchers encapsulated macrolide (erythromycin and azithromycin) antimicrobial agents in polymeric microneedle patches made of hyaluronic acid (HA) and polyvinylpyrrolidone (PVP). Five days after the treatment, the damage is fully recovered and the hair follicles, dermis, and other skin forms are restored.156 Researchers have reported the fabrication of water-soluble polymer microneedle patches by encapsulating the antibiotic chloramphenicol in gelatin nanoparticles and a polymer matrix (Fig. 8A).149 When applied to an infected site, the microneedle patches enter the EPS and genuinely upset the biofilm structure. Following rapid dissolution of the polymeric microneedle patches, the gelatin nanoparticles are dissociated by gelatinase, allowing the release of the encapsulated chloramphenicol into the biofilm matrix. This approach speeds up wound healing and improves targeting compared to direct chloramphenicol usage.149
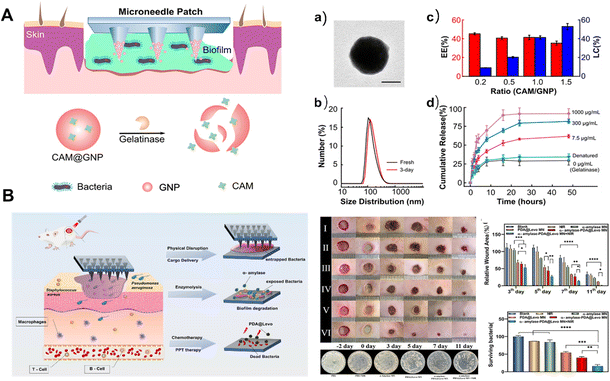 |
| Fig. 8 (A) Schematic illustration of the microneedle-mediated biofilm treatment.149 Copyright 2019 American Chemical Society. (B) Schematic illustration of the mechanism for the treatment using biofilms with dissolvable microneedle patches in infected wounds and biofilm elimination.157 Copyright 2022 Elsevier. | |
Extracellular polysaccharides are one of the important components of EPS. They enhance bacterial adhesion and aggregation, mechanical stability, nutrient and ion adsorption, and nutrient storage and act as a protective barrier for antimicrobial drugs.163 Enzymatic degradation has been reported to disrupt EPS structural integrity.164 Dissolvable microneedle patches combined with enzymatic and anti-infective agents and photothermal therapy eliminate biofilms from bacterial disease in wounds (Fig. 8B).157 The microneedle patches have rapid dissolution properties of PVA. The fine tip of the microneedle patch penetrates the biofilm matrix and delivers α-amylase and levofloxacin adsorbed polydopamine nanoparticles into the biofilm. In addition, photothermal response to near infrared light can accelerate the release of therapeutic agents, speeding up the healing process. However, photothermal therapy will inevitably cause damage to surrounding normal cells and tissues, and how to reasonably control the treatment time still needs further research.
Antimicrobial peptides are host defense molecules in multicellular organisms due to their special antibacterial mechanism compared with antibiotics.165 The antimicrobial peptides have broad-spectrum antibacterial properties and low biotoxicity, which can help solve the problem of drug resistance of traditional antibiotics.166 Su et al. combined electrostatic spinning, microneedle patches and antimicrobial peptides to remove biofilms produced by bacterial infections in wounds, based on a database of antimicrobial peptides.167
5.1.2 Antibacterial nanoparticles.
For potential antimicrobial applications, inorganic metal nanoparticles, such as silver,168 zinc oxide,169 copper,170 and other metal-containing nanoparticles,171 have been extensively investigated. The mechanism of action of nanoparticles on bacteria is mainly manifested in their ability to adsorb on bacterial membranes through electrostatic interactions, destroying the integrity of bacterial membranes.172 Despite the safety concerns of nanoparticles, it still does not prevent them from becoming one of the most used antibacterial agents besides antibiotics.
Silver nanoparticles can not only kill bacteria but also inhibit their exopolysaccharide synthesis.173,174 For example, silver nanoparticles and carboxymethylcellulose are used to create dissolvable microneedle patches with powerful antibacterial properties.158 Although silver nanoparticles are reported to be effective, they may be harmful to human cells.175 As a result, a method for selective drug delivery that prevents silver nanoparticles from being delivered to undesirable locations is required. For instance, Andi utilized silver nanoparticles synthesized with green tea extract due to their potential as antibacterial agents against biofilms of Staphylococcus aureus (SA) and Pseudomonas aeruginosa (PA) (Fig. 9A).159 Due to the toxicity of silver nanoparticles, they were incorporated into bacterial reaction particles (MPs) made of chitosan-modified poly(-caprolactone). In the presence of SA and PA, the MPs showed a 9-fold increase in the in vitro release of silver NPs, indicating some selectivity.159
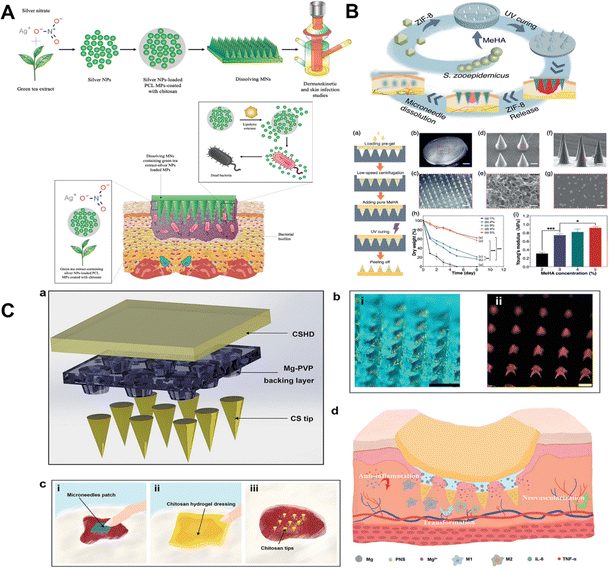 |
| Fig. 9 (A) Schematic illustration of bacteria-responsive microparticles for selective delivery of silver nanoparticles with microneedle patches.159 Copyright 2020 Elsevier. (B) Schematic diagram of Zn-MOF microneedle patches.177 Copyright 2021 Wiley-VCH. (C) Schematic diagram of microneedle patches and brief introduction to the process of promoting wound healing.162 Copyright 2022 Wiley-VCH. | |
Metal organic backbones (MOFs) are non-porous materials consisting of metal ions and organic ligands linked to ether by covalent bonds.176 Metal–organic frameworks (MOFs) are considered third-generation antimicrobials. They have advantages over conventional bactericidal materials due to their adjustable pore structure, controllable ion release rate, and high specific surface area.176 The metal ions are gradually released using the metal disassembly of the MOFs to provide long-lasting antimicrobial activity and to achieve the antimicrobial durability of expensive MOFs.176 For example, Yao et al. proposed a novel Zn-MOF-encapsulated microneedle patch with antibacterial activity and degradable properties to promote wound healing, and the Zn2+ released from the Zn-MOF can destroy the integrity of the bacterial envelope, catalyzing the production of oxygen free radicals, resulting in bacterial death. Additionally, the rough surface of ZIF-8 nanoparticles has the potential to expand the area of contact between MOFs and bacteria, enhancing antibacterial activity (Fig. 9B).177
Chitosan (CS) is one of the most encouraging regular polymers known to have antibacterial properties.178 CS is a natural cationic polysaccharide consisting of (1 → 4)-2-amino-2-deoxy-β-D-glucan, a partially to fully deacetylated form of chitin.179,180 CS is biodegradable, non-toxic, biocompatible, antibacterial and hemostatic, and is of great value in biomedical applications.127 The electrostatic interaction between the positively charged CS molecules and the negatively charged cell membrane alters the permeability of the cell membrane, leading to lysis, one of the known mechanisms by which CS acts on microorganisms.127,181 However, in practical applications, CS and other antibacterial substances are usually used to have a synergistic effect to achieve the antibacterial treatment of wounds. For example, taking advantage of the antibacterial properties of CS and zinc ions (Zn2+), composite microneedle patches of CS and zinc nitrate were prepared to eliminate bacterial biofilms.161 Due to the needle-like structure, microneedle patches can pierce EPS and can deliver CS and Zn2+ directly into bacterial biofilms. Compared with the patch without needle structure, the needle design increased the area of contact between the drug transporter and the bacterial biofilm by nearly 14–23%, facilitating drug dispersion. More importantly, the synergistic effect of CS and Zn2+ enabled the composite microneedle patches to show excellent anti-biofilm properties.161 Furthermore, Ning et al. proposed a synergistic detachable microneedle patch with a bilayer structure (Fig. 9C).162 The bilayer microneedle structure consists of a CS needle tip, CS hydrogel dressing, and a magnesium (Mg)-doped polyvinylpyrrolidone (PVP) backing. It provides a simple method for the programmed treatment of chronic wound management.
5.2 Delivering drugs for regulating inflammation
An excessive or prolonged inflammatory response can cause an undesired polarization of immune cells and lead to wound deterioration.182 Therefore, the development of dressings with inflammation modulating ability is of great importance to promote wound healing. Macrophages are key players in the wound healing process and serve as important targets for wound healing.183 Studies have shown that chronic wounds are closely associated with an impaired phenotypic shift of pro-inflammatory macrophages to an anti-inflammatory phenotype in wounds.184 Focusing on restoring the normal transformation of the macrophage phenotype facilitates normal wound healing. Numerous immune cells and non-lymphocytes use reactive oxygen species (ROS) as second messengers, regulate angiogenesis, and are necessary for the normal wound healing response.185 However, it should be noted that while high ROS production can result in oxidative stress and hinder wound healing, low ROS levels may actually help wound healing.186 In addition to causing severe inflammation, excessive reactive oxygen species (ROS) in wounds also inhibit the function of endogenous stem cells and macrophages, preventing cell proliferation and wound tissue regeneration.187,188 Therefore, to form a normal wound environment with an appropriate amount of reactive oxygen species, it is necessary to remove excess reactive oxygen species around the wound.
The new microneedle patch designed by Ma and his colleagues encapsulates polydopamine nanoparticles (PDA NPs) in a shell of hyaluronic acid methacrylate (HAMA).189 The structure of polydopamine is rich in reducing functional groups, such as catechol and imines, which allow the polydopamine molecule to exhibit good antioxidant properties.19,190 Polydopamine nanoparticles were continuously released to scavenge ROS as HAMA slowly degraded (Fig. 10A).189 Microneedle patches show antioxidant, anti-inflammatory, and pro-angiogenic properties, and are of some value.
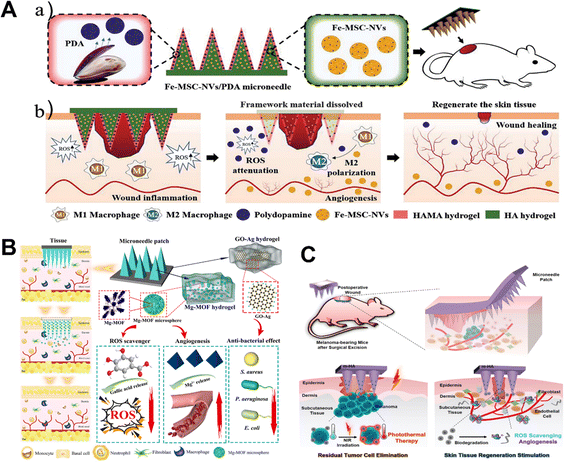 |
| Fig. 10 (A) Schematic illustrations of Fe-MSC-NVs/PDA MN patches for diabetic wound healing.189 Copyright 2022 Wiley-VCH. (B) Schematic illustration of magnesium organic framework-based MN patches (denoted as MN-MOF-GO-Ag) for accelerating diabetic wound healing.194 Copyright 2021 American Chemical Society. (C) Schematic diagram of the biomineralized melanin nanoparticle-loaded microneedle patches for the subcutaneous melanoma postoperative wound.195 Copyright 2022 Wiley-VCH. | |
Gallic acid (GA) is a specific plant compound in the phenol group that is also used to promote wound healing.191 GA has many biological activities, including anti-inflammatory, anti-tumor, immunomodulatory, hypoglycemic, and hyperlipidemic.192 GA has strong antioxidant activity due to its three hydroxyl groups.193 A schematic diagram of the multifunctional MOF magnesium microneedle patch prepared with gallic acid and Mg2+ is shown in Fig. 10B.194 The Mg-MOFs in an acidic microenvironment slowly release Mg2+ and gallic acid. The gallic acid released acts as an antioxidant, scavenging excessive production of intracellular reactive oxygen species (ROS), thereby reducing ROS-induced inflammation.194In vivo, therapeutic effects on diabetic wound healing were significantly improved.
Melanin exists widely in nature and has functions such as antioxidation, temperature regulation, and photoprotection.196 Cuttlefish ink contains many melanin nanoparticles, which can be extracted as well-dispersed spherical melanin nanoparticles.197,198 Qi Lei and colleagues presented a hyaluronic acid (HA) microneedle patch functionalized with biomineralized melanin nanoparticles. The microneedle patch enables simultaneous tumor photothermal therapy (PTT) and promotes skin tissue regeneration. Microneedle patches have great potential as adjuvant therapy after skin tumor resection (Fig. 10C).195 In addition, the researchers designed herbal microneedle patches using two herbs found in nature, Premna microphylla and Centella Asiatica.199 The microneedle patch actively shows excellent antioxidant and anti-inflammatory properties of asiaticoside (AS), a natural ingredient is Centella asiatica. AS hinders angiogenesis during the remodeling phase in addition to promoting angiogenesis during the inflammatory phase.200 The preparation of Chinese herbal microneedle patches also serves as a booster for the global promotion of Chinese herbal medicine. In addition, Wang's team successfully manufactured a microneedle patch integrated with Mxenes to achieve a controlled release of AS, which is beneficial for wound healing.201 The diabetic wound microenvironment is characterized by hyperglycemia, hypoxia, overproduction of oxidative stress and bacterial infection, making wound healing difficult.202
5.3 Delivering living cells and bioactive factors for skin regeneration
Growth factors, gene delivery, and cell therapy are some of the methods that have been used to speed up the healing of non-healing wounds.23 Growth factors such as the PDGF (platelet-derived growth factor), VEGF (vascular endothelial growth factor) and bFGF (basic fibroblast growth factor) have been found to have dysregulated biological activity in chronic trauma in both in vitro and in vivo studies.23,203,204 By delivering the right amount of growth factors, the wound can be provided with a better environment for growth.205 Cell-based therapeutic methods for healing wounds have also received attention. In vivo, the incorporation of fibroblasts, keratinocytes, and stem/progenitor cells significantly speeds up wound healing.23,206–208
Chen and his colleagues fabricated polymer microneedle patches for keratinocyte delivery. The delivery rate can reach 83.2%.209 The design of microneedle patches loaded with mesenchymal stem cells (MSCs) for wound healing was first proposed by Kwangju Lee et al. (Fig. 11A).210 Benefiting from the outer layer of the polylactic acid–ethanolic acid shell, the MSCs in the GelMA-MSC mixture within the microneedle tip remain viable. Results showed that the vitality and function of MSCs could be maintained up to 24 h, which increased wound closure rates and improved re-epithelialization.210
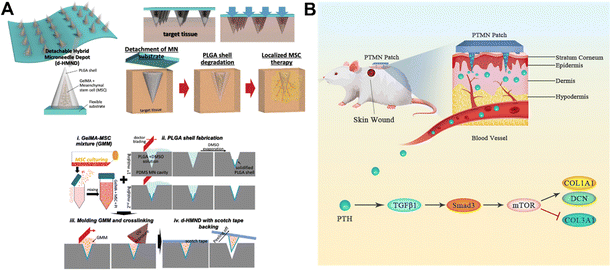 |
| Fig. 11 (A) Conceptual diagram of the microneedle patch and the preparation process.210 Copyright 2020 Wiley-VCH. (B) New polyvinyl alcohol microneedle patch releases the parathyroid hormone.214 Copyright 2021 Elsevier. | |
MengYuan and colleagues used a gelatin methacrylate microneedle patch to achieve controlled transdermal release of tazarotene and exosomes. Tazarotene is a retinoid that promotes angiogenesis and hair follicle and collagen regeneration.211 The MCS-exosome is involved in skin regeneration by promoting macrophage polarization and reducing the release of pro-inflammatory factors.212 Microneedle patches are used for the slow release of exosomes and tazarotene deep in the skin to promote cell migration and angiogenesis.213 In addition, the researchers achieved a non-invasive sustained release of the parathyroid hormone in rats through the microneedle patches, which promoted wound healing by accelerating extracellular matrix deposition and improving the structure of the extracellular matrix (Fig. 11B).214 Not only this but microneedle patches that deliver collagen to promote wound healing have also been developed.215,216 Long et al. prepared the first recombinant human-derived collagen (rhCol III) and naproxen (Nap) loaded polylactic acid glycolic acid (PLGA) nanoparticle microneedle patch for the treatment of diabetic chronic wounds.217In vitro cell culture results show that microneedles loaded with rhCol III can effectively promote the proliferation and migration of fibroblasts and endothelial cells.217
6. Conclusions and prospects
Wound healing is affected by old age, a weakened immune system and malnutrition, which imposes a significant economic burden on society.127 Functional dressings for various types of wounds have become increasingly available, but these wound dressings are expected to be able to monitor wound status in real time and provide personalized treatment. A new generation of smart wound dressings with real-time monitoring and on-demand treatment is currently under development to enable real-time tracking of the wound environment. Due to their powerful drug delivery capabilities, customizable microstructure and their superior biocompatibility, microneedle patches have great potential as wound dressings. This article discusses the current application of microneedle patches in promoting wound healing and smart wound management. The microneedle-based sensor enables real-time monitoring of a wide range of biomarkers, enabling early diagnosis of a variety of diseases such as Parkinson's disease and diabetes. However, microneedle-based sensors are still in the preliminary stages of exploration in smart wound management, although microneedle sensors have already realized the monitoring of inflammatory factors at the wound bed and the tracking of motion sensing. The complex changes in the wound healing environment require them to realize real-time monitoring of more biomarkers and more intelligent wound management. In addition, enabling the monitoring of multiple markers means the interconnection of multiple sensors, and microneedle-based sensors are highly reproducible and stable. Low detection limits are a key requirement for medical problems. Microneedle patches as dressings to facilitate wound healing have undergone tremendous development, from designing individualized microstructures to meeting different adhesion requirements, to enabling the delivery of different functional substances. Based on the bionic, responsive, and conductive properties of microneedle patches, the application of microneedle patches in wound healing is described in three aspects. At the same time, a microneedle patch that promotes wound healing has achieved the delivery of various substances that promote wound healing, including antibacterial agents, living cells, and bioactive factors. However, the adhesion of the microneedle patches to the skin, the delivery efficiency, and the therapeutic effect are the main factors to be investigated. The skin and wounds on the skin are elastic tissues with complicated surface topography and counteracting forces, which can make penetration uneven.100 Second, skin elasticity and thickness vary according to age, gender, and weight. These complex factors impose higher requirements on the design of microneedle patches. A phase III study of Zosano Pharmaceuticals' microneedle-delivered patch for sumatriptan (migraine) has recently been concluded. However, the FDA requested more data to address differences in the exposure of zolmitriptan and insufficient bridging of the pharmacokinetics of microneedle patches across batches when subjects received different microneedle patches.218,219
The smart microneedle patch combines on-demand drug delivery and real-time monitoring and is a new way forward for intelligent wound management. The therapeutic, diagnostic system should include controllable drug delivery patches, tiny electronic controllers, and high-sensitivity biosensors. The sensors send data to the control via bluetooth or WiFi after continuously monitoring different biomarkers in the wound environment. A microneedle patch-based system that personalizes treatment through early analysis and diagnosis. However, as far as the current research is concerned, more biomarkers related to wound healing are worth exploring. At the same time, the coordinated detection of multiple markers can provide more accurate data. As far as the existing research articles are concerned, the smart microneedle wound patch only realizes the monitoring of inflammatory factors and motion sensing. It is necessary to prepare microneedle patches with multiple monitoring properties.
Author contributions
Fangfang Hu: data curation, writing and original draft preparation. Qian Gao, Jinxi Liu, and Wenting Chen: data collection and obtained the permission. Caiyun Zheng Que Bai, Na Sun, and Wenhui Zhang: supervision. Fangfang Hu, Yanni Zhang, and Tingli Lu: writing, reviewing, and editing. All the authors participated in the conception and design, or analysis and interpretation of the data; drafting of the paper or revising it critically for intellectual content; and the final approval of the version to be published; and all authors agree to be accountable for all aspects of the work.
Conflicts of interest
There are no conflicts to declare.
Acknowledgements
We acknowledge funding support from the National Natural Science Foundation of China (program no. 81803491), the Key Research and Development Program of Shaanxi (program no. 2021SF-106 and 2022SF-081), and the China Postdoctoral Science Foundation (no. 2021M702633). We appreciate the Experimental Center, College of Life Sciences of Northwestern Polytechnical University for supporting our research on the cell tests.
References
- S. O. Blacklow, J. Li, B. R. Freedman, M. Zeidi, C. Chen and D. J. Mooney, Bioinspired Mechanically Active Adhesive Dressings to Accelerate Wound Closure, Sci. Adv., 2019, 5, eaaw3963 CrossRef CAS PubMed
.
- M. Farahani and A. Shafiee, Wound Healing: From Passive to Smart Dressings, Adv. Healthcare Mater., 2021, 10, 2100477 CrossRef CAS PubMed
.
- C. Lindholm and R. Searle, Wound Management for the 21st Century: Combining Effectiveness and Efficiency, Int. Wound J., 2016, 13, 5–15 CrossRef PubMed
.
- M. Kerr, E. Barron, P. Chadwick, T. Evans, W. M. Kong, G. Rayman, M. Sutton-Smith, G. Todd, B. Young and W. J. Jeffcoate, The Cost of Diabetic Foot Ulcers and Amputations to the National Health Service in England, Diabetic Med., 2019, 36, 995–1002 CrossRef CAS PubMed
.
- K. Raziyeva, Y. Kim, Z. Zharkinbekov, K. Kassymbek, S. Jimi and A. Saparov, Immunology of Acute and Chronic Wound Healing, Biomolecules, 2021, 11, 700 CrossRef CAS PubMed
.
- C. E. Fife, K. A. Eckert and M. J. Carter, Publicly Reported Wound Healing Rates: The Fantasy and the Reality, Adv. Wound Care, 2018, 7, 77–94 CrossRef PubMed
.
- H. Derakhshandeh,
et al., A Wirelessly Controlled Smart Bandage with 3d-Printed Miniaturized Needle Arrays, Adv. Funct. Mater., 2020, 30, 1905544 CrossRef CAS PubMed
.
- L. Barnum, M. Samandari, T. A. Schmidt and A. Tamayol, Microneedle Arrays for the Treatment of Chronic Wounds, Expert Opin. Drug Delivery, 2020, 17, 1767–1780 CrossRef CAS PubMed
.
- T. N. Demidova-Rice, M. R. Hamblin and I. M. Herman, Acute and Impaired Wound Healing: Pathophysiology and Current Methods for Drug Delivery, Part 1: Normal and Chronic Wounds: Biology, Causes, and Approaches to Care, Adv. Skin Wound Care, 2012, 25, 304–314 CrossRef PubMed
.
- M. Samandari,
et al., Miniaturized Needle Array-Mediated Drug Delivery Accelerates Wound Healing, Adv. Healthcare Mater., 2021, 10, 2001800 CrossRef CAS PubMed
.
- H. Sattar, I. S. Bajwa, R. ul Amin, J. Muhammad, M. F. Mushtaq, R. Kazmi, M. Akram, M. Ashraf and U. Shafi, Smart Wound Hydration Monitoring Using Biosensors and Fuzzy Inference System, Wirel. Commun. Mob. Comput., 2019, 2019, 1–15 CrossRef
.
- C. R. Kruse, K. Nuutila, C. C. Y. Lee, E. Kiwanuka, M. Singh, E. J. Caterson, E. Eriksson and J. A. Sorensen, The External Microenvironment of Healing Skin Wounds, Wound Repair Regen., 2015, 23, 456–464 CrossRef PubMed
.
- F. Mariani, M. Serafini, I. Gualandi, D. Arcangeli, F. Decataldo, L. Possanzini, M. Tessarolo, D. Tonelli, B. Fraboni and E. Scavetta, Advanced Wound Dressing for Real-Time Ph Monitoring, ACS Sens., 2021, 6, 2366–2377 CrossRef CAS PubMed
.
- H. Teymourian, F. Tehrani, K. Mahato and J. Wang, Lab under the Skin: Microneedle Based Wearable Devices, Adv. Healthcare Mater., 2021, 10, 2002255 CrossRef CAS PubMed
.
- A. W. C. Chua, Y. C. Khoo, B. K. Tan, K. C. Tan, C. L. Foo and S. J. Chong, Skin Tissue Engineering Advances in Severe Burns: Review and Therapeutic Applications, Int. J. Burns Trauma, 2016, 4 DOI:10.1186/s41038-016-0027-y
.
- A. Moeini, P. Pedram, P. Makvandi, M. Malinconico and G. G. d’Ayala, Wound Healing and Antimicrobial Effect of Active Secondary Metabolites in Chitosan-Based Wound Dressings: A Review, Carbohydr. Polym., 2020, 233, 115839 CrossRef CAS PubMed
.
- D. M. Supp and S. T. Boyce, Engineered Skin Substitutes: Practices and Potentials, Clin. Dermatol., 2005, 23, 403–412 CrossRef PubMed
.
- D. Breitkreutz, N. Mirancea and R. Nischt, Basement Membranes in Skin: Unique Matrix Structures with Diverse Functions?, Histochem. Cell Biol., 2009, 132, 1–10 CrossRef CAS PubMed
.
- Y. P. Liang, J. H. He and B. L. Guo, Functional Hydrogels as Wound Dressing to Enhance Wound Healing, ACS Nano, 2021, 15, 12687–12722 CrossRef CAS PubMed
.
- E. E. Tredget, B. Nedelec, P. G. Scott and A. Ghahary, Hypertrophic Scars, Keloids, and Contractures - the Cellular and Molecular Basis for Therapy, Surg. Clin. North Am., 1997, 77, 701–730 CrossRef CAS PubMed
.
- B. M. Delavary, W. M. van der Veer, M. van Egmond, F. B. Niessen and R. H. J. Beelen, Macrophages in Skin Injury and Repair, Immunobiology, 2011, 216, 753–762 CrossRef CAS PubMed
.
- K. A. Bielefeld, S. Amini-Nik and B. A. Alman, Cutaneous Wound Healing: Recruiting Developmental Pathways for Regeneration, Cell. Mol. Life Sci., 2013, 70, 2059–2081 CrossRef CAS PubMed
.
- H. S. Kim, X. Y. Sun, J. H. Lee, H. W. Kim, X. B. Fu and K. W. Leong, Advanced Drug Delivery Systems and Artificial Skin Grafts for Skin Wound Healing, Adv. Drug Delivery Rev., 2019, 146, 209–239 CrossRef CAS PubMed
.
- M. E. Okur, I. D. Karantas, Z. Senyigit, N. U. Okur and P. I. Siafaka, Recent Trends on Wound Management: New Therapeutic Choices Based on Polymeric Carriers, Asian J. Pharm. Sci., 2020, 15, 661–684 CrossRef PubMed
.
- J. Li, J. Chen and R. Kirsner, Pathophysiology of Acute Wound Healing, Clin. Dermatol., 2007, 25, 9–18 CrossRef PubMed
.
- M. Rodrigues, N. Kosaric, C. A. Bonham and G. C. Gurtner, Wound Healing: A Cellular Perspective, Physiol. Rev., 2019, 99, 665–706 CrossRef CAS PubMed
.
- E. M. Tottoli, R. Dorati, I. Genta, E. Chiesa, S. Pisani and B. Conti, Skin Wound Healing Process and New Emerging Technologies for Skin Wound Care and Regeneration, Pharmaceutics, 2020, 12, 735 CrossRef CAS PubMed
.
- Z. Wang, F. Rong, Z. Li, W. Li, K. Kaur and Y. Wang, Tailoring Gas-Releasing Nanoplatforms for Wound Treatment: An Emerging Approach, Chem. Eng. J., 2023, 452, 139297 CrossRef CAS
.
- W. Dai, Y. C. Dong, T. Han, J. Wang, B. Gao, H. Guo, F. Xu, J. Li and Y. F. Ma, Microenvironmental Cue-Regulated Exosomes as Therapeutic Strategies for Improving Chronic Wound Healing. Npg Asia, Materials, 2022, 14, 75 CAS
.
- G. Han and R. Ceilley, Chronic Wound Healing: A Review of Current Management and Treatments, Adv. Ther., 2017, 34, 599–610 CrossRef PubMed
.
- P. Yang, S. Li, H. Zhang, X. Ding and Q. Tan, Lrg1 Accelerates Wound Healing in Diabetic Rats by Promoting Angiogenesis Via the Wnt/Beta-Catenin Signaling Pathway, Int. J. Lower Extremity Wounds, 2022 DOI:10.1177/15347346221081610
.
- C. C. Yan,
et al., Human Umbilical Cord Mesenchymal Stem Cell-Derived Exosomes Accelerate Diabetic Wound Healing Via Ameliorating Oxidative Stress and Promoting Angiogenesis, Front. Bioeng. Biotechnol., 2022, 10, 7 Search PubMed
.
- Q. Pang, D. Lou, S. J. Li, G. M. Wang, B. B. Qiao, S. R. Dong, L. Ma, C. Y. Gao and Z. H. Wu, Smart Flexible Electronics-Integrated Wound Dressing for Real-Time Monitoring and on-Demand Treatment of Infected Wounds, Adv. Sci., 2020, 7, 1902673 CrossRef CAS PubMed
.
- Q. Wang, S. Ling, X. Liang, H. Wang, H. Lu and Y. Zhang, Self-Healable Multifunctional Electronic Tattoos Based on Silk and Graphene, Adv. Funct. Mater., 2019, 29, 1808695 CrossRef
.
- M. Qin, H. Guo, Z. Dai, X. Yan and X. Ning, Advances in Flexible and Wearable Ph Sensors for Wound Healing Monitoring, J. Semicond., 2019, 40, 111607 CrossRef CAS
.
- S. D. Milne, I. Seoudi, H. Al Hamad, T. K. Talal, A. A. Anoop, N. Allahverdi, Z. Zakaria, R. Menzies and P. Connolly, A Wearable Wound Moisture Sensor as an Indicator for Wound Dressing Change: An Observational Study of Wound Moisture and Status, Int. Wound J., 2016, 13, 1309–1314 CrossRef PubMed
.
- L. Wang, Q. H. Hou, W. F. Zheng and X. Y. Jiang, Fluorescent and Antibacterial Aminobenzeneboronic Acid (Aba)-Modified Gold Nanoclusters for Self-Monitoring Residual Dosage and Smart Wound Care, ACS Nano, 2021, 15, 17885–17894 CrossRef CAS PubMed
.
- Q. Pang, D. Lou, S. Li, G. Wang, B. Qiao, S. Dong, L. Ma, C. Gao and Z. Wu, Smart Flexible Electronics-Integrated Wound Dressing for Real-Time Monitoring and on-Demand Treatment of Infected Wounds, Adv. Sci., 2020, 7, 1902673 CrossRef CAS PubMed
.
- K. Zheng,
et al., Flexible Bicolorimetric Polyacrylamide/Chitosan Hydrogels for Smart Real-Time Monitoring and Promotion of Wound Healing, Adv. Funct. Mater., 2021, 31, 2102599 CrossRef CAS
.
- Z. Zhang, H. Yan, B. Qiu, P. Ran, W. Cao, X. Jia, K. Huang and X. Li, Persistent Luminescence-Based Theranostics for Real-Time Monitoring and Simultaneously Launching Photodynamic Therapy of Bacterial Infections, Small, 2022, 18, 2200813 CrossRef CAS PubMed
.
- P. Salvo, V. Dini, F. Di Francesco and M. Romanelli, The Role of Biomedical Sensors in Wound Healing, Wound Medicine, 2015, 8, 15–18 CrossRef
.
- P. Salvo, V. Dini, A. Kirchhain, A. Janowska, T. Oranges, A. Chiricozzi, T. Lomonaco, F. Di Francesco and M. Romanelli, Sensors and Biosensors for C-Reactive Protein, Temperature and Ph, and Their Applications for Monitoring Wound Healing: A Review, Sensors, 2017, 17, 2952 CrossRef PubMed
.
- P. Mostafalu,
et al., Smart Bandage for Monitoring and Treatment of Chronic Wounds, Small, 2018, 14, 1703509 CrossRef PubMed
.
- G. Xu,
et al., Battery-Free and Wireless Smart Wound Dressing for Wound Infection Monitoring and Electrically Controlled on-Demand Drug Delivery, Adv. Funct. Mater., 2021, 31, 2100852 CrossRef CAS
.
- Y. N. Zhu,
et al., A Multifunctional Pro-Healing Zwitterionic Hydrogel for Simultaneous Optical Monitoring of Ph and Glucose in Diabetic Wound Treatment, Adv. Funct. Mater., 2020, 30, 1905493 CrossRef CAS
.
- X. Zhang, R. Lv, L. Chen, R. Sun, Y. Zhang, R. Sheng, T. Du, Y. Li and Y. Qi, A Multifunctional Janus Electrospun Nanofiber Dressing with Biofluid Draining, Monitoring, and Antibacterial Properties for Wound Healing, ACS Appl. Mater. Interfaces, 2022, 14, 12984–13000 CrossRef CAS PubMed
.
- M. Tessarolo, L. Possanzini, I. Gualandi, F. Mariani, L. D. Torchia, D. Arcangeli, F. Melandri, E. Scavetta and B. Fraboni, Wireless Textile Moisture Sensor for Wound Care, Frontiers Physics, 2021, 9, 616 Search PubMed
.
- C. Cheng, Y. M. Qiu, S. M. Tang, B. Y. Lin, M. Z. Guo, B. B. Gao and B. F. He, Artificial Spider Silk Based Programmable Woven Textile for Efficient Wound Management, Adv. Funct. Mater., 2022, 32, 2107707 CrossRef CAS
.
- N. Tang, Y. Zheng, D. Cui and H. Haick, Multifunctional Dressing for Wound Diagnosis and Rehabilitation, Adv. Healthcare Mater., 2021, 10, 2101292 CrossRef CAS PubMed
.
- N. Tang, Y. Zheng, X. Jiang, C. Zhou, H. Jin, K. Jin, W. Wu and H. Haick, Wearable Sensors and Systems for Wound Healing-Related Ph and Temperature Detection, Micromachines, 2021, 12, 430 CrossRef PubMed
.
- A. McLister, J. McHugh, J. Cundell and J. Davis, New Developments in Smart Bandage Technologies for Wound Diagnostics, Adv. Mater., 2016, 28, 5732–5737 CrossRef CAS PubMed
.
- C. Watters, T. T. Yuan and K. P. Rumbaugh, Beneficial and Deleterious Bacterial-Host Interactions in Chronic Wound Pathophysiology, Chronic Wound Care Manag. Res., 2015, 2, 53–62 Search PubMed
.
- R. Pourkarim, M. R. Farahpour and S. A. Rezaei, Comparison Effects of Platelet-Rich Plasma on Healing of Infected and Non-Infected Excision Wounds by the Modulation of the Expression of Inflammatory Mediators: Experimental Research, Eur. J. Trauma Emerg. Surg., 2022, 48, 3339–3347 CrossRef PubMed
.
- D. Braig, B. Kaiser, J. R. Thiele, H. Bannasch, K. Peter, G. B. Stark, H. G. Koch, S. U. Eisenhardt and A. Conformational, Change of C-Reactive Protein in Burn Wounds Unmasks Its Proinflammatory Properties, Int. Immunol., 2014, 26, 467–478 CrossRef CAS PubMed
.
- S. RoyChoudhury,
et al., Continuous Monitoring of Wound Healing Using a Wearable Enzymatic Uric Acid Biosensor, J. Electrochem. Soc., 2018, 165, B3168–B3175 CrossRef CAS
.
- X. Liu and P. B. Lillehoj, Embroidered Electrochemical Sensors on Gauze for Rapid Quantification of Wound Biomarkers, Biosens. Bioelectron., 2017, 98, 189–194 CrossRef CAS PubMed
.
- A. Pal, D. Goswami, H. E. Cuellar, B. Castro, S. H. Kuang and R. V. Martinez, Early Detection and Monitoring of Chronic Wounds Using Low-Cost, Omniphobic Paper-Based Smart Bandages, Biosens. Bioelectron., 2018, 117, 696–705 CrossRef CAS PubMed
.
- Y.-C. Du and W.-S. Ciou, A Sensor Gauze with Multi-Channel Moisture and Ph Monitoring for Chronic Wound Care, IEEE Access, 2019, 7, 29185–29192 Search PubMed
.
- H. S. Guo,
et al., Pro-Healing Zwitterionic Skin Sensor Enables Multi-Indicator Distinction and Continuous Real-Time Monitoring, Adv. Funct. Mater., 2021, 31, 2106406 CrossRef CAS
.
- D. A. Jankowska, M. B. Bannwarth, C. Schulenburg, G. Faccio, K. Maniura-Weber, R. M. Rossi, L. Scherer, M. Richter and L. F. Boesel, Simultaneous Detection of Ph Value and Glucose Concentrations for Wound Monitoring Applications, Biosens. Bioelectron., 2017, 87, 312–319 CrossRef CAS PubMed
.
- Y. Zhu,
et al., A Multifunctional Pro-Healing Zwitterionic Hydrogel for Simultaneous Optical Monitoring of Ph and Glucose in Diabetic Wound Treatment, Adv. Funct. Mater., 2020, 30, 1905493 CrossRef CAS
.
- Y. Gao,
et al., A Flexible Multiplexed Immunosensor for Point-of-Care in Situ Wound Monitoring, Sci. Adv., 2021, 7, eabg9614 CrossRef CAS PubMed
.
- M. Zheng,
et al., Skin-Inspired Gelatin-Based Flexible Bio-Electronic Hydrogel for Wound Healing Promotion and Motion Sensing, Biomaterials, 2021, 276, 121026 CrossRef CAS PubMed
.
- T. Waghule, G. Singhvi, S. K. Dubey, M. M. Pandey, G. Gupta, M. Singh and K. Dua, Microneedles: A Smart Approach and Increasing Potential for Transdermal Drug Delivery System, Biomed. Pharmacother., 2019, 109, 1249–1258 CrossRef CAS PubMed
.
- H. Amani, M. A. Shahbazi, C. D'Amico, F. Fontana, S. Abbaszadeh and H. A. Santos, Microneedles for Painless Transdermal Immunotherapeutic Applications, J. Controlled Release, 2021, 330, 185–217 CrossRef CAS PubMed
.
- E. Larraneta, R. E. M. Lutton, A. D. Woolfson and R. F. Donnelly, Microneedle Arrays as Transdermal and Intradermal Drug Delivery Systems: Materials Science, Manufacture and Commercial Development, Mater. Sci. Eng. R Rep., 2016, 104, 1–32 CrossRef
.
- Y. W. Naguib, A. Kumar and Z. Cuin, The Effect of Microneedles on the Skin Permeability and Antitumor Activity of Topical 5-Fluorouracil, Acta Pharm. Sin. B, 2014, 4, 94–99 CrossRef PubMed
.
- M.-C. Kearney, E. Caffarel-Salvador, S. J. Fallows, H. O. McCarthy and R. F. Donnelly, Microneedle-Mediated Delivery of Donepezil: Potential for Improved Treatment Options in Alzheimer's Disease, Eur. J. Pharm. Biopharm., 2016, 103, 43–50 CrossRef CAS PubMed
.
- J.-Y. Kim, M.-R. Han, Y.-H. Kim, S.-W. Shin, S.-Y. Nam and J.-H. Park, Tip-Loaded Dissolving Microneedles for Transdermal Delivery of Donepezil Hydrochloride for Treatment of Alzheimer's Disease, Eur. J. Pharm. Biopharm., 2016, 105, 148–155 CrossRef CAS PubMed
.
- N. U. Rehman, C. Song, J. Kim, I. Noh, Y.-S. Rhee and H. J. Chung, Pharmacokinetic Evaluation of a Novel Donepezil-Loaded Dissolving Microneedle Patch in Rats, Pharmaceutics, 2022, 14, 5 CrossRef CAS PubMed
.
- M. Kale, T. Kipping and A. K. Banga, Modulated Delivery of Donepezil Using a Combination of Skin Microporation and Iontophoresis, Int. J. Pharm., 2020, 589, 119853 CrossRef CAS PubMed
.
- L. Dong,
et al., Au Nanocage-Strengthened Dissolving Microneedles for Chemo-Photothermal Combined Therapy of Superficial Skin Tumors, ACS Appl. Mater. Interfaces, 2018, 10, 9247–9256 CrossRef CAS PubMed
.
- A. M. V. Mohan, J. R. Windmiller, R. K. Mishra and J. Wang, Continuous Minimally-Invasive Alcohol Monitoring Using Microneedle Sensor Arrays, Biosens. Bioelectron., 2017, 91, 574–579 CrossRef CAS PubMed
.
- S. A. Ranamukhaarachchi, C. Padeste, U. O. Hafeli, B. Stoeber and V. J. Cadarso, Design Considerations of a Hollow Microneedle-Optofluidic Biosensing Platform Incorporating Enzyme-Linked Assays, J. Micromech. Microeng., 2018, 28, 024002 CrossRef
.
- O. Hosu, S. Mirel, R. Sandulescu and C. Cristea, Minireview: Smart Tattoo, Microneedle, Point-of-Care, and Phone-Based Biosensors for Medical Screening, Diagnosis, and Monitoring, Anal. Lett., 2019, 52, 78–92 CrossRef CAS
.
- O. Erdem, I. Es, G. A. Akceoglu, Y. Saylan and F. Inci, Recent Advances in Microneedle-Based Sensors for Sampling, Diagnosis and Monitoring of Chronic Diseases, Biosensors-Basel, 2021, 11, 296 CrossRef CAS PubMed
.
- G.-S. Liu, Y. Kong, Y. Wang, Y. Luo, X. Fan, X. Xie, B.-R. Yang and M. X. Wu, Microneedles for Transdermal Diagnostics: Recent Advances and New Horizons, Biomaterials, 2020, 232, 119740 CrossRef CAS PubMed
.
- S. Yao, Y. Luo and Y. Wang, Engineered Microneedles Arrays for Wound Healing, Engineered Regeneration, 2022, 3, 232–240 CrossRef
.
- R. Y. He, H. Liu, T. S. Fang, Y. Niu, H. Q. Zhang, F. Han, B. Gao, F. Li and F. Xu, A Colorimetric Dermal Tattoo Biosensor Fabricated by Microneedle Patch for Multiplexed Detection of Health-Related Biomarkers, Adv. Sci., 2021, 8, 2103030 CrossRef CAS PubMed
.
- J. Y. Shan, X. X. Zhang, B. Kong, Y. J. Zhu, Z. X. Gu, L. J. Ren and Y. J. Zhao, Coordination Polymer Nanozymes-Integrated Colorimetric Microneedle Patches for Intelligent Wound Infection Management, Chem. Eng. J., 2022, 444, 136640 CrossRef CAS
.
- K. Lee,
et al., Non-Transdermal Microneedles for Advanced Drug Delivery, Adv. Drug Delivery Rev., 2020, 165–166, 41–59 CrossRef CAS PubMed
.
- B. Gao, M. Guo, K. Lyu, T. Chu and B. He, Intelligent Silk Fibroin Based Microneedle Dressing (I-Smd), Adv. Funct. Mater., 2021, 31, 2006839 CrossRef CAS
.
- M. Z. Guo, Y. Q. Wang, B. B. Gao and B. F. He, Shark Tooth-Inspired Microneedle Dressing for Intelligent Wound Management, ACS Nano, 2021, 15, 15316–15327 CrossRef CAS PubMed
.
- X. Zhang, G. Chen, Y. Yu, L. Sun and Y. Zhao, Bioinspired Adhesive and Antibacterial Microneedles for Versatile Transdermal Drug Delivery, Research, 2020, 2020 DOI:10.34133/2020/3672120
.
- P. Makvandi,
et al., Bioinspired Microneedle Patches: Biomimetic Designs, Fabrication, and Biomedical Applications, Matter, 2022, 5, 390–429 CrossRef CAS
.
- X. Zhang, G. Chen, L. Sun, F. Ye, X. Shen and Y. Zhao, Claw-Inspired Microneedle Patches with Liquid Metal Encapsulation for Accelerating Incisional Wound Healing, Chem. Eng. J., 2021, 406, 126741 CrossRef CAS
.
- M. Guo, Y. Wang, B. Gao and B. He, Shark Tooth-Inspired Microneedle Dressing for Intelligent Wound Management, ACS Nano, 2021, 15, 15316–15327 CrossRef CAS PubMed
.
- W. K. Cho,
et al., Microstructured Barbs on the North American Porcupine Quill Enable Easy Tissue Penetration and Difficult Removal, Proc. Natl. Acad. Sci. U. S. A., 2012, 109, 21289–21294 CrossRef CAS PubMed
.
- Y. Deng, C. Yang, Y. Y. Zhu, W. Y. Liu, H. L. Li, L. P. Wang, W. Chen, Z. Wang and L. Wang, Lamprey-Teeth-Inspired Oriented Antibacterial Sericin Microneedles for Infected Wound Healing Improvement, Nano Lett., 2022, 22, 2702–2711 CrossRef CAS PubMed
.
- D. Han, R. S. Morde, S. Mariani, A. A. La Mattina, E. Vignali, C. Yang, G. Barillaro and H. Lee, 4d Printing of a Bioinspired Microneedle Array with Backward-Facing Barbs for Enhanced Tissue Adhesion, Adv. Funct. Mater., 2020, 30, 1909197 CrossRef CAS
.
- S. Y. Yang, E. D. O’Cearbhaill, G. C. Sisk, K. M. Park, W. K. Cho, M. Villiger, B. E. Bouma, B. Pomahac, J. M. Karp and A. Bio-Inspired, Swellable Microneedle Adhesive for Mechanical Interlocking with Tissue, Nat. Commun., 2013, 4, 1702 CrossRef PubMed
.
- E. Y. Jeon, J. Lee, B. J. Kim, K. I. Joo, K. H. Kim, G. Lim and H. J. Cha, Bio-Inspired Swellable Hydrogel-Forming Double-Layered Adhesive Microneedle Protein Patch for Regenerative Internal/External Surgical Closure, Biomaterials, 2019, 222, 119439 CrossRef CAS PubMed
.
- Y.-C. Chen and H. Yang, Octopus-Inspired Assembly of Nanosucker Arrays for Dry/Wet Adhesion, ACS Nano, 2017, 11, 5332–5338 CrossRef CAS PubMed
.
- K. Jiang, Y.-Z. Long, Z.-J. Chen, S.-L. Liu, Y.-Y. Huang, X. Jiang and Z.-Q. Huang, Airflow-Directed in Situ Electrospinning of a Medical Glue of Cyanoacrylate for Rapid Hemostasis in Liver Resection, Nanoscale, 2014, 6, 7792–7798 RSC
.
- D. H. Park, S. B. Kim, K. D. Ahn, E. Y. Kim, Y. J. Kim and D. K. Han, In Vitro Degradation and Cytotoxicity of Alkyl 2-Cyanoacrylate Polymers for Application to Tissue Adhesives, J. Appl. Polym. Sci., 2003, 89, 3272–3278 CrossRef CAS
.
- X. Zhao, B. Guo, H. Wu, Y. Liang and P. X. Ma, Injectable Antibacterial Conductive Nanocomposite Cryogels with Rapid Shape Recovery for Noncompressible Hemorrhage and Wound Healing, Nat. Commun., 2018, 9, 2784 CrossRef PubMed
.
- Y. Huang, X. Zhao, Z. Zhang, Y. Liang, Z. Yin, B. Chen, L. Bai, Y. Han and B. Guo, Degradable Gelatin-Based Ipn Cryogel Hemostat for Rapidly Stopping Deep Noncompressible Hemorrhage and Simultaneously Improving Wound Healing, Chem. Mater., 2020, 32, 6595–6610 CrossRef CAS
.
- Z. Qiao,
et al., A Mussel-Inspired Supramolecular Hydrogel with Robust Tissue Anchor for Rapid Hemostasis of Arterial and Visceral Bleedings, Bioact. Mater., 2021, 6, 2829–2840 CrossRef CAS PubMed
.
- X. Zhang, G. Chen, L. Cai, Y. Wang, L. Sun and Y. Zhao, Bioinspired Pagoda-Like Microneedle Patches with Strong Fixation and Hemostasis Capabilities, Chem. Eng. J., 2021, 414, 128905 CrossRef CAS
.
- Y. Q. Wang, M. Z. Guo, B. F. He and B. B. Gao, Intelligent Patches for Wound Management: In Situ Sensing and Treatment, Anal. Chem., 2021, 93, 4687–4696 CrossRef CAS PubMed
.
- M. C. Chen, M. H. Ling, K. W. Wang, Z. W. Lin, B. H. Lai and D. H. Chen, Near-Infrared Light-Responsive Composite Microneedles for on-Demand Transdermal Drug Delivery, Biomacromolecules, 2015, 16, 1598–1607 CrossRef CAS PubMed
.
- Z. Zheng, H. Ye, J. Wang, T. Zhang, Q. You, H. Li, R. He, Y. Chen, W. Zhang and Y. Cao, Visible-Light-Controllable Drug Release from Multilayer-Coated Microneedles, J. Mater. Chem. B, 2017, 5, 7014–7017 RSC
.
- L. Fan, X. Zhang, M. Nie, Y. Xu, Y. Wang, L. Shang, Y. Zhao and Y. Zhao, Photothermal Responsive Microspheres-Triggered Separable Microneedles for Versatile Drug Delivery, Adv. Funct. Mater., 2022, 32, 2110746 CrossRef CAS
.
- Y. Zhang, D. F. Wang, M. Y. Gao, B. Xu, J. Y. Zhu, W. J. Yu, D. P. Liu and G. H. Jiang, Separable Microneedles for near-Infrared Light-Triggered Transdermal Delivery of Metformin in Diabetic Rats, ACS Biomater. Sci. Eng., 2018, 4, 2879–2888 CrossRef CAS PubMed
.
- S. A. Gaware, K. A. Rokade, P. Bala and S. N. Kale, Microneedles of Chitosan-Porous Carbon Nanocomposites: Stimuli (Ph and Electric Field)-Initiated Drug Delivery and Toxicological Studies, J. Biomed. Mater. Res., Part A, 2019, 107, 1582–1596 CAS
.
- X. Zhang, G. Chen, X. Fu, Y. Wang and Y. Zhao, Magneto-Responsive Microneedle Robots for Intestinal Macromolecule Delivery, Adv. Mater., 2021, 33, 2104932 CrossRef CAS PubMed
.
- Y. Zhang, D. N. Chai, M. Y. Gao, B. Xu and G. H. Jiang, Thermal Ablation of Separable Microneedles for Transdermal Delivery of Metformin on Diabetic Rats, Int. J. Polym. Mater. Polym. Biomater., 2019, 68, 850–858 CrossRef CAS
.
- Y. Wang, H. Lu, M. Guo, J. Chu, B. Gao and B. He, Personalized and Programmable Microneedle Dressing for Promoting Wound Healing, Adv. Healthcare Mater., 2022, 11, 2101659 CrossRef CAS PubMed
.
- Y. Q. Zhang, J. Q. Wang, J. C. Yu, D. Wen, A. R. Kahkoska, Y. Lu, X. D. Zhang, J. B. Buse and Z. Gu, Bioresponsive Microneedles with a Sheath Structure for H2o2 and Ph Cascade-Triggered Insulin Delivery, Small, 2018, 14, 1704181 CrossRef PubMed
.
- J. Yu, J. Wang, Y. Zhang, G. Chen, W. Mao, Y. Ye, A. R. Kahkoska, J. B. Buse, R. Langer and Z. Gu, Glucose-Responsive Insulin Patch for the Regulation of Blood Glucose in Mice and Minipigs, Nat. Biomed. Eng., 2020, 4, 499–506 CrossRef CAS PubMed
.
- Y. Zhang, P. Feng, J. Yu, J. Yang, J. Zhao, J. Wang, Q. Shen and Z. Gu, Ros-Responsive Microneedle Patch for Acne Vulgaris Treatment, Adv. Ther., 2018, 1, 1800035 CrossRef
.
- X. Yue, Q. Zhang and Z. Dai, Near-Infrared Light-Activatable Polymeric Nanoformulations for Combined Therapy and Imaging of Cancer, Adv. Drug Delivery Rev., 2017, 115, 155–170 CrossRef CAS PubMed
.
- P. Makvandi,
et al., Stimuli-Responsive Transdermal Microneedle Patches, Mater. Today, 2021, 47, 206–222 CrossRef CAS PubMed
.
- S. Yao, Y. Wang, J. Chi, Y. Yu, Y. Zhao, Y. Luo and Y. Wang, Porous Mof Microneedle Array Patch with Photothermal Responsive Nitric Oxide Delivery for Wound Healing, Adv. Sci., 2022, 9, 2103449 CrossRef CAS PubMed
.
- M. B. Witte and A. Barbul, Role of Nitric Oxide in Wound Repair, Am. J. Surg., 2002, 183, 406–412 CrossRef CAS PubMed
.
- C. J. Ma, Y. He, X. Jin, Y. Zhang, X. Zhang, Y. Li, M. Xu, K. Liu, Y. Yao and F. Lu, Light-Regulated Nitric Oxide Release from Hydrogel-Forming Microneedles Integrated with Graphene Oxide for Biofilm-Infected-Wound Healing, Biomater. Adv., 2022, 134, 112555 CrossRef PubMed
.
- S. Schreml, R. M. Szeimies, L. Prantl, S. Karrer, M. Landthaler and P. Babilas, Oxygen in Acute and Chronic Wound Healing, Br. J. Dermatol., 2010, 163, 257–268 CrossRef CAS PubMed
.
- W. L. Yip, Influence of Oxygen on Wound Healing, Int. Wound J., 2015, 12, 620–624 CrossRef PubMed
.
- X. Zhang, G. Chen, Y. Liu, L. Sun, L. Sun and Y. Zhao, Black Phosphorus-Loaded Separable Microneedles as Responsive Oxygen Delivery Carriers for Wound Healing, ACS Nano, 2020, 14, 5901–5908 CrossRef CAS PubMed
.
- Z. B. Sun, H. H. Xie, S. Y. Tang, X. F. Yu, Z. N. Guo, J. D. Shao, H. Zhang, H. Huang, H. Y. Wang and P. K. Chu, Ultrasmall Black Phosphorus Quantum Dots: Synthesis and Use as Photothermal Agents, Angew. Chem., Int. Ed., 2015, 54, 11526–11530 CrossRef CAS PubMed
.
- Y. Zeng, Y.-R. V. Shih, G. S. Baht and S. Varghese, In Vivo Sequestration of Innate Small Molecules to Promote Bone Healing, Adv. Mater., 2020, 32, 1906022 CrossRef CAS PubMed
.
- Z. Bonyanian, M. Walker, E. Du Toit and R. B. Rose'Meyer, Multiple Adenosine Receptor Subtypes Stimulate Wound Healing in Human Ea.Hy926 Endothelial Cells, Purinergic Signalling, 2019, 15, 357–366 CrossRef CAS PubMed
.
- M. C. Montesinos, A. Desai, J. F. Chen, H. Yee, M. A. Schwarzschild, J. S. Fink and B. N. Cronstein, Adenosine Promotes Wound Healing and Mediates Angiogenesis in Response to Tissue Injury Via Occupancy of a(2a) Receptors, Am. J. Pathol., 2002, 160, 2009–2018 CrossRef CAS PubMed
.
- S. Wang, Z. Zhang, S. Wei, F. He, Z. Li, H.-H. Wang, Y. Huang and Z. Nie, Near-Infrared Light-Controllable Mxene Hydrogel for Tunable on-Demand Release of Therapeutic Proteins, Acta Biomater., 2021, 130, 138–148 CrossRef CAS PubMed
.
- P. Xue,
et al., Near-Infrared Light-Driven Shape-Morphing of Programmable Anisotropic Hydrogels Enabled by Mxene Nanosheets, Angew. Chem., Int. Ed., 2021, 60, 3390–3396 CrossRef CAS PubMed
.
- L. Y. Sun, L. Fan, F. K. Bian, G. P. Chen, Y. T. Wang and Y. J. Zhao, Mxene-Integrated Microneedle Patches with Innate Molecule Encapsulation for Wound Healing, Research, 2021, 2021, 9838490 CAS
.
- J. Chi, X. Zhang, C. Chen, C. Shao, Y. Zhao and Y. Wang, Antibacterial and Angiogenic Chitosan Microneedle Array Patch for Promoting Wound Healing, Bioact. Mater., 2020, 5, 253–259 CrossRef PubMed
.
- D. Y. Shan, E. Gerhard, C. J. Zhang, J. W. Tierney, D. Xie, Z. W. Liu and J. Yang, Polymeric Biomaterials for Biophotonic Applications, Bioact. Mater., 2018, 3, 434–445 CrossRef PubMed
.
- B. B. Gao, M. Z. Guo, K. Lyu, T. S. Chu and B. F. He, Intelligent Silk Fibroin Based Microneedle Dressing (I-Smd), Adv. Funct. Mater., 2021, 31, 2006839 CrossRef CAS
.
- Y. Ren, X. Yu, Z. Li, D. Liu and X. Xue, Fabrication of Ph-Responsive Ta-Keratin Bio-Composited Hydrogels Encapsulated with Photoluminescent Go Quantum Dots for Improved Bacterial Inhibition and Healing Efficacy in Wound Care Management: In Vivo Wound Evaluations, J. Photochem. Photobiol., B, 2020, 202, 111676 CrossRef CAS PubMed
.
- A. Ullah, M. Jang, H. Khan, H. J. Choi, S. An, D. Kim, Y.-R. Kim, U.-K. Kim and G. M. Kim, Microneedle Array with a Ph-Responsive Polymer Coating and Its Application in Smart Drug Delivery for Wound Healing, Sens. Actuators, B, 2021, 345, 130441 CrossRef CAS
.
- V. C. Ibekwe, H. M. Fadda, G. E. Parsons and A. W. Basit, A Comparative in Vitro Assessment of the Drug Release Performance of Ph-Responsive Polymers for Ileo-Colonic Delivery, Int. J. Pharm., 2006, 308, 52–60 CrossRef CAS PubMed
.
- Z. Guo, H. Liu, Z. Shi, L. Lin, Y. Li, M. Wang, G. Pan, Y. Lei and L. Xue, Responsive Hydrogel-Based Microneedle Dressing for Diabetic Wound Healing, J. Mater. Chem. B, 2022, 10, 3501–3511 RSC
.
- Y. Liu, M. Petreaca and M. Martins-Green, Cell and Molecular Mechanisms of Insulin-Induced Angiogenesis, J. Cell. Mol. Med., 2009, 13, 4492–4504 CrossRef CAS PubMed
.
- Y. Liu, M. Petreaca, M. Yao and M. Martins-Green, Cell and Molecular Mechanisms of Keratinocyte Function Stimulated by Insulin During Wound Healing, BMC Cell Biol., 2009, 10, 1–15 CrossRef PubMed
.
- P. Yang,
et al., Insulin-Containing Wound Dressing Promotes Diabetic Wound Healing through Stabilizing Hif-1 Alpha, Front. Bioeng. Biotechnol., 2020, 8, 592833 CrossRef PubMed
.
- M. Zhao, Electrical Fields in Wound Healing-an Overriding Signal That Directs Cell Migration, Semin. Cell Dev. Biol., 2009, 20, 674–682 CrossRef CAS PubMed
.
- K. Y. Nishimura, R. R. Isseroff and R. Nuccitelli, Human Keratinocytes Migrate to the Negative Pole in Direct Current Electric Fields Comparable to Those Measured in Mammalian Wounds, J. Cell Sci., 1996, 109, 199–207 CrossRef CAS PubMed
.
- L. F. Jaffe and J. W. Vanable, Jr., Electric Fields and Wound Healing, Clin. Dermatol., 1984, 2, 34–44 CrossRef CAS PubMed
.
- Y.-S. Sun, Electrical Stimulation for Wound-Healing: Simulation on the Effect of Electrode Configurations, BioMed Res. Int., 2017, 2017, 5289041 Search PubMed
.
- S. B. Rajendran, K. Challen, K. L. Wright and J. G. Hardy, Electrical Stimulation to Enhance Wound Healing, J. Funct. Biomater., 2021, 12, 40 CrossRef CAS PubMed
.
- Y. Long,
et al., Effective Wound Healing Enabled by Discrete Alternative Electric Fields from Wearable Nanogenerators, ACS Nano, 2018, 12, 12533–12540 CrossRef CAS PubMed
.
- A. Guo, B. Song, B. Reid, Y. Gu, J. V. Forrester, C. A. B. Jahoda and M. Zhao, Effects of Physiological Electric Fields on Migration of Human Dermal Fibroblasts, J. Invest. Dermatol., 2010, 130, 2320–2327 CrossRef CAS PubMed
.
- Y. Su,
et al., Dissolvable Microneedles Coupled with Nanofiber Dressings Eradicate Biofilms Via Effectively Delivering a Database-Designed Antimicrobial Peptide, ACS Nano, 2020, 14, 11775–11786 CrossRef CAS PubMed
.
- A. D. Permana, M. Mir, E. Utomo and R. F. Donnelly, Bacterially Sensitive Nanoparticle-Based Dissolving Microneedles of Doxycycline for Enhanced Treatment of Bacterial Biofilm Skin Infection: A Proof of Concept Study, Int. J. Pharm.: X, 2020, 2, 100047 CAS
.
- H. C. Flemming and J. Wingender, The Biofilm Matrix, Nat. Rev. Microbiol., 2010, 8, 623–633 CrossRef CAS PubMed
.
- R. Jamaledin, C. K. Y. Yiu, E. N. Zare, L.-N. Niu, R. Vecchione, G. Chen, Z. Gu, F. R. Tay and P. Makvandi, Advances in Antimicrobial Microneedle Patches for Combating Infections, Adv. Mater., 2020, 32, 2002129 CrossRef CAS PubMed
.
- H. Koo, R. N. Allan, R. P. Howlin, P. Stoodley and L. Hall-Stoodley, Targeting Microbial Biofilms: Current and Prospective Therapeutic Strategies, Nat. Rev. Microbiol., 2017, 15, 740–755 CrossRef CAS PubMed
.
- J. H. Xu, R. Danehy, H. W. Cai, Z. Ao, M. Pu, A. Nusawardhana, D. Rowe-Magnus and F. Guo, Microneedle Patch-Mediated Treatment of Bacterial Biofilms, ACS Appl. Mater. Interfaces, 2019, 11, 14640–14646 CrossRef CAS PubMed
.
- J. Ziesmer, P. Tajpara, N.-J. Hempel, M. Ehrstrom, K. Melican, L. Eidsmo and G. A. Sotiriou, Vancomycin-Loaded Microneedle Arrays against Methicillin-Resistant Staphylococcus Aureus Skin Infections, Adv. Mater. Technol., 2021, 6, 2001307 CrossRef CAS PubMed
.
- K. Tsioris, W. K. Raja, E. M. Pritchard, B. Panilaitis, D. L. Kaplan and F. G. Omenetto, Fabrication of Silk Microneedles for Controlled-Release Drug Delivery, Adv. Funct. Mater., 2012, 22, 330–335 CrossRef CAS
.
- S. Sillankorva, L. Pires, L. M. Pastrana and M. Banobre-Lopez, Antibiofilm Efficacy of the Pseudomonas Aeruginosa Pbunavirus Vb_Paem-Sms29 Loaded onto Dissolving Polyvinyl Alcohol Microneedles, Viruses-Basel, 2022, 14, 964 CrossRef CAS PubMed
.
- I. Woodhouse,
et al., Flexible Microneedle Array Patch for Chronic Wound Oxygenation and Biofilm Eradication, ACS Appl. Bio Mater., 2021, 4, 5405–5415 CrossRef CAS PubMed
.
- M. Mir, A. D. Permana, N. Ahmed, G. M. Khan, A. U. Rehman and R. F. Donnelly, Enhancement in Site-Specific Delivery of Carvacrol for Potential Treatment of Infected Wounds Using Infection Responsive Nanoparticles Loaded into Dissolving Microneedles: A Proof of Concept Study, Eur. J. Pharm. Biopharm., 2020, 147, 57–68 CrossRef CAS PubMed
.
- A. Panda, A. Shettar, P. K. Sharma, M. A. Repka and S. N. Murthy, Development of Lysozyme Loaded Microneedles for Dermal Applications, Int. J. Pharm., 2021, 593, 120104 CrossRef CAS PubMed
.
- M. S. Arshad, A. T. Zahra, S. Zafar, H. Zaman, A. Akhtar, M. M. Ayaz, I. Kucuk, M. Maniruzzaman, M. W. Chang and Z. Ahmad, Antibiofilm Effects of Macrolide Loaded Microneedle Patches: Prospects in Healing Infected Wounds, Pharm. Res., 2021, 38, 165–177 CrossRef CAS PubMed
.
- X. Yu, J. Zhao and D. Fan, A Dissolving Microneedle Patch for Antibiotic/Enzymolysis/Photothermal Triple Therapy against Bacteria and Their Biofilms, Chem. Eng. J., 2022, 437, 135475 CrossRef CAS
.
- L. E. G. Garcia, M. N. MacGregor, R. M. Visalakshan, N. Ninan, A. A. Cavallaro, A. D. Trinidad, Y. P. Zhao, A. J. D. Hayball and K. Vasilev, Self-Sterilizing Antibacterial Silver-Loaded Microneedles, Chem. Commun., 2019, 55, 171–174 RSC
.
- A. D. Permana,
et al., Selective Delivery of Silver Nanoparticles for Improved Treatment of Biofilm Skin Infection Using Bacteria-Responsive Microparticles Loaded into Dissolving Microneedles, Mater. Sci. Eng., C, 2021, 120, 111786 CrossRef CAS PubMed
.
- S. Yao, J. Chi, Y. Wang, Y. Zhao, Y. Luo and Y. Wang, Zn-Mof Encapsulated Antibacterial and Degradable Microneedles Array for Promoting Wound Healing, Adv. Healthcare Mater., 2021, 10, 2100056 CrossRef CAS PubMed
.
- X. Yi, C. Wang, X. Yu, W. Su and Z. Yuan, Chitosan/Zinc Nitrate Microneedles for Bacterial Biofilm Eradication, J. Biomed. Mater. Res., Part B, 2021, 109, 911–920 CrossRef CAS PubMed
.
- T. Ning,
et al., Synergistically Detachable Microneedle Dressing for Programmed Treatment of Chronic Wounds, Adv. Healthcare Mater., 2022, 11, 2102180 CrossRef CAS PubMed
.
- L. K. Jennings,
et al., Pel Is a Cationic Exopolysaccharide That Cross-Links Extracellular DNA in the Pseudomonas Aeruginosa Biofilm Matrix, Proc. Natl. Acad. Sci. U. S. A., 2015, 112, 11353–11358 CrossRef CAS PubMed
.
- T. F. Bahamondez-Canas, L. A. Heersema and H. D. C. Smyth, Current Status of in Vitro Models and Assays for Susceptibility Testing for Wound Biofilm Infections, Biomedicines, 2019, 7, 34 CrossRef CAS PubMed
.
- P. Kaiser, J. Wachter and M. Windbergs, Therapy of Infected Wounds: Overcoming Clinical Challenges by Advanced Drug Delivery Systems, Drug Delivery Transl. Res., 2021, 11, 1545–1567 CrossRef CAS PubMed
.
- Y. H. Yan, Y. Z. Li, Z. W. Zhang, X. H. Wang, Y. Z. Niu, S. H. Zhang, W. L. Xu and C. G. Ren, Advances of Peptides for Antibacterial Applications, Colloids Surf., B, 2021, 202, 111682 CrossRef CAS PubMed
.
- Y. J. Su,
et al., Dissolvable Microneedles Coupled with Nanofiber Dressings Eradicate Biofilms Via Effectively Delivering a Database-Designed Antimicrobial Peptide, ACS Nano, 2020, 14, 11775–11786 CrossRef CAS PubMed
.
- S. S. Khan, A. Mukherjee and N. Chandrasekaran, Studies on Interaction of Colloidal Silver Nanoparticles (Snps) with Five Different Bacterial Species, Colloids Surf., B, 2011, 87, 129–138 CrossRef CAS PubMed
.
- R. Sinha, R. Karan, A. Sinha and S. K. Khare, Interaction and Nanotoxic Effect of Zno and Ag Nanoparticles on Mesophilic and Halophilic Bacterial Cells, Bioresour. Technol., 2011, 102, 1516–1520 CrossRef CAS PubMed
.
- B. Wu, R. Huang, M. Sahu, X. Y. Feng, P. Biswas and Y. J. Tang, Bacterial Responses to Cu-Doped Tio2 Nanoparticles, Sci. Total Environ, 2010, 408, 1755–1758 CrossRef CAS PubMed
.
- W. Jiang, H. Mashayekhi and B. Xing, Bacterial Toxicity Comparison between Nano- and Micro-Scaled Oxide Particles, Environmental Pollution, 2009, 157, 1619–1625 CrossRef CAS PubMed
.
- M. J. Hajipour, K. M. Fromm, A. A. Ashkarran, D. J. de Aberasturi, I. R. de Larramendi, T. Rojo, V. Serpooshan, W. J. Parak and M. Mahmoudi, Antibacterial Properties of Nanoparticles, Trends Biotechnol., 2012, 30, 499–511 CrossRef CAS PubMed
.
- K. Kalishwaralal, S. BarathManiKanth, S. R. K. Pandian, V. Deepak and S. Gurunathan, Silver Nanoparticles Impede the Biofilm Formation by Pseudomonas Aeruginosa and Staphylococcus Epidermidis, Colloids Surf., B, 2010, 79, 340–344 CrossRef CAS PubMed
.
- A. R. Shahverdi, A. Fakhimi, H. R. Shahverdi and S. Minaian, Synthesis and Effect of Silver Nanoparticles on the Antibacterial Activity of Different Antibiotics against Staphylococcus Aureus and Escherichia Coli, Nanomedicine, 2007, 3, 168–171 CrossRef CAS PubMed
.
- M. van der Zande,
et al., Distribution, Elimination, and Toxicity of Silver Nanoparticles and Silver Ions in Rats after 28-Day Oral Exposure, ACS Nano, 2012, 6, 7427–7442 CrossRef CAS PubMed
.
- M. F. Shen, F. Forghani, X. Q. Kong, D. H. Liu, X. Q. Ye, S. G. Chen and T. Ding, Antibacterial Applications of Metal-Organic Frameworks and Their Composites, Compr. Rev. Food Sci. Food Saf., 2020, 19, 1397–1419 CrossRef CAS PubMed
.
- S. Yao, J. J. Chi, Y. T. Wang, Y. J. Zhao, Y. Luo and Y. A. Wang, Zn-Mof Encapsulated Antibacterial and Degradable Microneedles Array for Promoting Wound Healing, Adv. Healthcare Mater., 2021, 10, 2100056 CrossRef CAS PubMed
.
- N. A. Kamel, S. L. Abd El-Messieh and N. M. Saleh, Chitosan/Banana Peel Powder Nanocomposites for Wound Dressing Application: Preparation and Characterization, Mater. Sci. Eng., C, 2017, 72, 543–550 CrossRef CAS PubMed
.
- M. Hosseinnejad and S. M. Jafari, Evaluation of Different Factors Affecting Antimicrobial Properties of Chitosan, Int. J. Biol. Macromol., 2016, 85, 467–475 CrossRef CAS PubMed
.
- G. F. Yuan, H. Lv, W. Y. Tang, X. J. Zhang and H. Y. Sun, Effect of Chitosan Coating Combined with Pomegranate Peel Extract on the Quality of Pacific White Shrimp During Iced Storage, Food Control, 2016, 59, 818–823 CrossRef CAS
.
- F. R. Tamara, C. Lin, F.-L. Mi and Y.-C. Ho, Antibacterial Effects of Chitosan/Cationic Peptide Nanoparticles, Nanomaterials, 2018, 8, 88 CrossRef PubMed
.
- M. Tian, L. Zhou, C. Fan, L. Wang, X. Lin, Y. Wen, L. Su and H. Dong, Bimetal-Organic Framework/Gox-Based Hydrogel Dressings with Antibacterial and Inflammatory Modulation for Wound Healing, Acta Biomater., 2022, 158, 252–265 CrossRef PubMed
.
- S. Y. Kim and M. G. Nair, Macrophages in Wound Healing: Activation and Plasticity, Immunol. Cell Biol., 2019, 97, 258–267 CrossRef PubMed
.
- A. E. Boniakowski, A. S. Kimball, B. N. Jacobs, S. L. Kunkel and K. A. Gallagher, Macrophage-Mediated Inflammation in Normal and Diabetic Wound Healing, J. Immunol., 2017, 199, 17–24 CrossRef CAS PubMed
.
- C. Dunnill, T. Patton, J. Brennan, J. Barrett, M. Dryden, J. Cooke, D. Leaper and N. T. Georgopoulos, Reactive Oxygen Species (Ros) and Wound Healing: The Functional Role of Ros and Emerging Ros-Modulating Technologies for Augmentation of the Healing Process, Int. Wound J., 2017, 14, 89–96 CrossRef PubMed
.
- H. Zhao, J. Huang, Y. Li, X. J. Lv, H. T. Zhou, H. R. Wang, Y. Y. Xu, C. Wang, J. Wang and Z. Liu, Ros-Scavenging Hydrogel to Promote Healing of Bacteria Infected Diabetic Wounds, Biomaterials, 2020, 258, 120286 CrossRef CAS PubMed
.
- H. Wu, F. Li, W. Shao, J. Gao and D. Ling, Promoting Angiogenesis in Oxidative Diabetic Wound Microenvironment Using a Nanozyme-Reinforced Self-Protecting Hydrogel, ACS Cent. Sci., 2019, 5, 477–485 CrossRef CAS PubMed
.
- J. Zhu, Q. Jin, H. Zhao, W. Zhu, Z. Liu and Q. Chen, Reactive Oxygen Species Scavenging Sutures for Enhanced Wound Sealing and Repair, Small Structures, 2021, 2, 2100002 CrossRef CAS
.
- W. Ma, X. Zhang, Y. Liu, L. Fan, J. Gan, W. Liu, Y. Zhao and L. Sun, Polydopamine Decorated Microneedles with Fe-Msc-Derived Nanovesicles Encapsulation for Wound Healing, Adv. Sci., 2022, 9, 2103317 CrossRef CAS PubMed
.
- X. Bao, J. Zhao, J. Sun, M. Hu and X. Yang, Polydopamine Nanoparticles as Efficient Scavengers for Reactive Oxygen Species in Periodontal Disease, ACS Nano, 2018, 12, 8882–8892 CrossRef CAS PubMed
.
- S. Sharma, D. Mittal, A. K. Verma and I. Roy, Copper-Gallic Acid Nanoscale Metal-Organic Framework for Combined Drug Delivery and Photodynamic Therapy, ACS Appl. Bio Mater., 2019, 2, 2092–2101 CrossRef CAS PubMed
.
- A. Ahangarpour, H. Sharifinasab, H. Kalantari, M. A. Dehghani, N. S. Maram and F. Golfakhrabadi, Gallic Acid and Gallic Acid Nanoparticle Modulate Insulin Secretion Pancreatic Beta-Islets against Silica Nanoparticle-Induced Oxidative Damage, Biol. Trace Elem. Res., 2022, 200, 5159–5171 CrossRef CAS PubMed
.
- D. L. Tran, P. L. Thi, T. T. H. Thi and K. D. Park, Novel Enzymatically Crosslinked Chitosan Hydrogels with Free-Radical-Scavenging Property and Promoted Cellular Behaviors under Hyperglycemia, Prog. Nat. Sci.: Mater. Int., 2020, 30, 661–668 CrossRef CAS
.
- M. Yin,
et al., Multifunctional Magnesium Organic Framework-Based Microneedle Patch for Accelerating Diabetic Wound Healing, ACS Nano, 2021, 15, 17842–17853 CrossRef CAS PubMed
.
- Q. Lei,
et al., Microneedle Patches Integrated with Biomineralized Melanin Nanoparticles for Simultaneous Skin Tumor Photothermal Therapy and Wound Healing, Adv. Funct. Mater., 2022, 32, 2113269 CrossRef CAS
.
- E. Di Mauro, O. Carpentier, S. I. Y. Sanchez, N. I. Ignoumba, M. Lalancette-Jean, J. Lefebvre, S. Zhang, C. F. O. Graeff, F. Cicoira and C. Santato, Resistive Switching Controlled by the Hydration Level in Thin Films of the Biopigment Eumelanin, J. Mater. Chem. C, 2016, 4, 9544–9553 RSC
.
- Y. Liang, R. Li, H. Sun, J. Dan, Z. Su, Y. Kang, Q. Zhang, S. Shi, J. Wang and W. Zhang, Functionalized Natural Melanin Nanoparticle Mimics Natural Peroxidase for Total Antioxidant Capacity Determination, Sens. Actuators, B, 2022, 359, 131541 CrossRef CAS
.
- J. Zhou, W. Liu, X. Y. Zhao, Y. F. Xian, W. Wu, X. Zhang, N. N. Zhao, F. J. Xu and C. Y. Wang, Natural Melanin/Alginate Hydrogels Achieve Cardiac Repair through Ros Scavenging and Macrophage Polarization, Adv. Sci., 2021, 8, 2100505 CrossRef CAS PubMed
.
- J. Chi, L. Sun, L. Cai, L. Fan, C. Shao, L. Shang and Y. Zhao, Chinese Herb Microneedle Patch for Wound Healing, Bioact. Mater., 2021, 6, 3507–3514 CrossRef CAS PubMed
.
- J. Huang, X. Zhou, L. Xia, W. Liu, F. Guo, J. Liu and W. Liu, Inhibition of Hypertrophic Scar Formation with Oral Asiaticoside Treatment in a Rabbit Ear Scar Model, Int. Wound J., 2021, 18, 598–607 CrossRef PubMed
.
- P. Wang, Y. Wang, Y. Yi, Y. Gong, H. Ji, Y. Gan, F. Xie, J. Fan and X. Wang, Mxenes-Integrated Microneedle Combined with Asiaticoside to Penetrate
the Cuticle for Treatment of Diabetic Foot Ulcer, J. Nanobiotechnol., 2022, 20, 259 CrossRef CAS PubMed
.
- J. Shan, X. Zhang, Y. Cheng, C. Song, G. Chen, Z. Gu and Y. Zhao, Glucose Metabolism-Inspired Catalytic Patches for Nir-Ii Phototherapy of Diabetic Wound Infection, Acta Biomater., 2023, 157, 200–209 CrossRef CAS PubMed
.
- A. P. Veith, K. Henderson, A. Spencer, A. Univer and A. B. Baker, Therapeutic Strategies for Enhancing Angiogenesis in Wound Healing, Adv. Drug Delivery Rev., 2019, 146, 97–125 CrossRef CAS PubMed
.
- N. S. Greaves, K. J. Ashcroft, M. Baguneid and A. Bayat, Current Understanding of Molecular and Cellular Mechanisms in Fibroplasia and Angiogenesis During Acute Wound Healing, J. Dermatol. Sci., 2013, 72, 206–217 CrossRef CAS PubMed
.
- S. Yamakawa and K. Hayashida, Advances in Surgical Applications of Growth Factors for Wound Healing, Int. J. Burns Trauma, 2019, 7 DOI:10.1186/s41038-019-0148-1
.
- G. Mascre, S. Dekoninck, B. Drogat, K. K. Youssef, S. Brohee, P. A. Sotiropoulou, B. D. Simons and C. Blanpain, Distinct Contribution of Stem and Progenitor Cells to Epidermal Maintenance, Nature, 2012, 489, 257–262 CrossRef CAS PubMed
.
- D. T. Woodley, Distinct Fibroblasts in the Papillary and Reticular Dermis: Implications for Wound Healing, Dermatol. Clin., 2017, 35, 95–100 CrossRef CAS PubMed
.
- L. Y. Liu, Y. H. Yu, Y. S. Hou, J. K. Chai, H. J. Duan, W. L. Chu, H. J. Zhang, Q. Hu and J. D. Du, Human Umbilical Cord Mesenchymal Stem Cells Transplantation Promotes Cutaneous Wound Healing of Severe Burned Rats, PLoS One, 2014, 9, e88348 CrossRef PubMed
.
- Y. H. Chen, D. C. Lin, E. Chern and Y. Y. Huang, The Use of Micro-Needle Arrays to Deliver Cells for Cellular Therapies, Biomed. Microdevices, 2020, 22, 1–8 CrossRef PubMed
.
- K. Lee,
et al., A Patch of Detachable Hybrid Microneedle Depot for Localized Delivery of Mesenchymal Stem Cells in Regeneration Therapy, Adv. Funct. Mater., 2020, 30, 2000086 CrossRef CAS PubMed
.
- Z. Zhu, Y. Liu, Y. Xue, X. Cheng, W. Zhao, J. Wang, R. He, Q. Wan and X. Pei, Tazarotene Released from Aligned Electrospun Membrane Facilitates Cutaneous Wound Healing by Promoting Angiogenesis, ACS Appl. Mater. Interfaces, 2019, 11, 36141–36153 CrossRef CAS PubMed
.
- D. H. Ha,
et al., Mesenchymal Stem/Stromal Cell-Derived Exosomes for Immunomodulatory Therapeutics and Skin Regeneration, Cells, 2020, 9, 1157 CrossRef CAS PubMed
.
- M. Yuan,
et al., Gelma/Pegda Microneedles Patch Loaded with Huvecs-Derived Exosomes and Tazarotene Promote Diabetic Wound Healing, J. Nanobiotechnol., 2022, 20, 147 CrossRef CAS PubMed
.
- Z. X. Yao, T. Xue, H. Xiong, C. A. D. Cai, X. D. Liu, F. Wu, S. Liu and C. Y. Fan, Promotion of Collagen Deposition During Skin Healing through Smad3/Mtor Pathway by Parathyroid Hormone-Loaded Microneedle, Mater. Sci. Eng., C, 2021, 119, 111446 CrossRef CAS PubMed
.
- L.-y Long, W. Liu, L. Li, C. Hu, S. He, L. Lu, J. Wang, L. Yang and Y.-b Wang, Dissolving Microneedle-Encapsulated Drug-Loaded Nanoparticles and Recombinant Humanized Collagen Type Iii for the Treatment of Chronic Wound Via Anti-Inflammation and Enhanced Cell Proliferation and Angiogenesis, Nanoscale, 2022, 14, 1285–1295 RSC
.
- W. Sun, M. Inayathullah, M. A. C. Manoukian, A. V. Malkovskiy, S. Manickam, M. P. Marinkovich, A. T. Lane, L. Tayebi, A. M. Seifalian and J. Rajadas, Transdermal Delivery of Functional Collagen Via Polyvinylpyrrolidone Microneedles, Ann. Biomed. Eng., 2015, 43, 2978–2990 CrossRef PubMed
.
- L. Y. Long, W. Q. Liu, L. Li, C. Hu, S. Y. He, L. Lu, J. Wang, L. Yang and Y. B. Wang, Dissolving Microneedle-Encapsulated Drug-Loaded Nanoparticles and Recombinant Humanized Collagen Type Iii for the Treatment of Chronic Wound Via Anti-Inflammation and Enhanced Cell Proliferation and Angiogenesis, Nanoscale, 2022, 14, 1285–1295 RSC
.
- B. Creelman, C. Frivold, S. Jessup, G. Saxon and C. Jarrahian, Manufacturing Readiness Assessment for Evaluation of the Microneedle Array Patch Industry: An Exploration of Barriers to Full-Scale Manufacturing, Drug Delivery Transl. Res., 2022, 12, 368–375 CrossRef PubMed
.
- E. L. H. Spierings, J. L. Brandes, D. B. Kudrow, J. Weintraub, P. C. Schmidt, D. J. Kellerman and S. J. Tepper, Randomized, Double-Blind, Placebo-Controlled, Parallel-Group, Multi-Center Study of the Safety and Efficacy of Adam Zolmitriptan for the Acute Treatment of Migraine, Cephalalgia, 2018, 38, 215–224 CrossRef PubMed
.
|
This journal is © The Royal Society of Chemistry 2023 |