DOI:
10.1039/D3TA04686A
(Paper)
J. Mater. Chem. A, 2023,
11, 23922-23931
Post-synthetic modification of zeolitic imidazolate framework-90 via Schiff base reaction for ultrahigh iodine capture†
Received
6th August 2023
, Accepted 12th October 2023
First published on 31st October 2023
Abstract
The post-synthetic modification of zeolitic imidazolate frameworks (ZIFs) is an effective strategy for preparing new materials that exhibit superior performance compared to their parental ZIFs. Herein, we quantitatively transformed the aldehyde group of ZIF-90 into mono- and bis-Schiff bases, and the resulting compounds ZIF-90-I–ZIF-90-III still retained the crystallinity and their parental structures. Importantly, ZIF-90-III with the highest amount of mono-Schiff base showed the maximum iodine uptake capacities of 6600 mg g−1 and 1826 mg g−1 for iodine vapor and iodine/cyclohexane solution, respectively, which are 1.5- and 3.4-fold as much as that of ZIF-90, being among the highest reported to date for metal–organic frameworks (MOFs). Noteworthily, activation energies for iodine adsorption of ZIF-90 and its post-synthetic derivatives were speculated experimentally, which was firstly investigated for MOFs. Meanwhile, adsorption kinetics, iodine species during iodine adsorption process and the related theoretical calculations were also studied in detail.
1 Introduction
Nuclear energy is regarded one of excellent candidates to replace fossil energy because of its high efficiency and non-carbon-emission.1,2 However, nuclear waste pollutants, such as numerous volatile radionuclides 3H, 85Kr, 129I and 131I, are inevitably generated during the process of fuel fission, which damage the ecosystem and bring serious harm to humans and animals once they are accidently leaked.3 Among the above-mentioned pollutants, radioactive iodine has obtained worldwide interest, because both the long-lived 129I (t1/2 = 1.57 × 107 years) and short-lived 131I (t1/2 = 8.02 days) can affect human metabolic processes, further leading to thyroid cancer and hypothyroidism.4 However, the low solubility and easy mobility of iodine make it difficult to capture.5,6 Thus, the adsorption of iodine is an urgent and challenging worldwide issue.
In the past decades, many porous materials, such as silver-exchanged zeolite, mesoporous silica, porous organic cages, covalent organic frameworks, and metal–organic frameworks, have been reported to trap iodine.7–11 Among these materials, MOFs are viewed as an outstanding candidate for iodine adsorption owing to its high surface area, suitable pore size and good chemical and thermal stability. The zeolitic imidazolate framework (ZIF) is a very important subfamily of metal–organic frameworks. Previously reported studies identified that a charge-transfer complex was modestly formed between the electron-deficient iodine molecule and electron-rich aromatic carbon molecules in them; therefore, ZIFs often exhibited high iodine adsorption amounts. For example, ZIF-8 showed a good iodine vapor adsorption capacity of 1.25 g g−1 at 350 K under ambient pressure,12,13 and the iodine adsorption amount of single particle ZIF-90 with a size of 3.8 μm is 220 mg g−1 within 180 s at room temperature.14 Additionally, the high electron density of organic linkers usually contributes to generating strong interactions between the iodine molecule and framework. This further leads to extraordinary iodine adsorption capacities of the materials. Hence, the introduction of rich electron groups (such as C
N/C
C/C
C with π electron and OH/NH2 with a lone pair electron) and organic linker defects were regarded as an effective strategy to increase the electron density of the organic ligands. For example, the iodine vapor adsorption amount of UiO-67 was 530 mg g−1, while the values were increased up to 1071 mg g−1 and 1211 mg g−1 for UiO-67-NH2 and UiO-67-(NH2)2, respectively.15,16 When organic linker defects were anchored to ZIF-90, the partial broken Zn–N bonds decreased the delocalization of N atoms from imidazole-2-carboxaldehyde, and the electron density of the aromatic ring from imidazole was increased, so the iodine adsorption amount of single ZIF-90 particle was significantly improved to 400 mg g−1 at the same condition.14 However, the introduction of rich electron groups with both π electron and lone pairs in MOFs has rarely been reported except for the core–shell NH2-UiO-66@Br-COFs hybrid material, in which the maximum iodine vapor adsorption amount was up to 3.73 g g−1 at 75 °C and obviously superior to that of NH2-UiO-66 (565 mg g−1).16,17
In this context, we chose ZIF-90 with aldehyde groups as the precursor and reacted it with 1,2-diaminocyclohexane. The aldehyde groups of ZIF-90 were consequently converted into mono- and bis-Schiff bases, and both C
N and NH2 groups were introduced in ZIF-90 for improving the electron density of aromatic ring. A series of post-synthetic functionalizations of ZIF-90 derivatives (ZIF-90-I–ZIF-90-III) were prepared via controlling the molar ratio of ZIF-90 and 1,2-diaminocyclohexane. Moreover, iodine adsorption experiments of these materials were also carried out by means of weighting method for iodine vapor and monitoring on a UV-vis spectrometer for iodine/cyclohexane solution, respectively. Activation energies, adsorption kinetics, iodine species and related theoretical analysis of ZIF-90 and its post-synthetic derivatives during iodine adsorption process were also investigated.
2 Experiment
2.1. Materials and methods
All reagents in our research study were purchased from commercial sources and used without further purification. Powder X-ray diffraction (PXRD) patterns were obtained using a D/Max-3c X-ray diffractometer (Rigaku, Japan) with Cu Kα radiation. Fourier transform infrared spectroscopy (FTIR) spectra were collected using a Spectrum Two spectrometer (PerkinElmer, USA). 1H NMR spectra were measured on a Bruker Avance III HD 400 MHz spectrometer (Bruker, Germany). High-resolution electrospray ionization mass spectrometry (HRESI-MS) measurements were obtained on an Exactive mass spectrometer (Thermo Fisher Scientific, Germany). N2 adsorption isotherm measurements were performed on a BELSORP-max II adsorption apparatus (BEL, Japan) at 77 K. Scanning electron microscopy (SEM) was performed on a TESCAN MIRA LMS (TESCAN, Czech Republic). UV-vis absorption spectra were obtained on a CARY ECLIPSE JASCO-720 spectrophotometer (Agilent, USA). Raman spectra were obtained on a Renishaw Invia Raman spectrometer (Invia, UK). X-ray photoelectron spectra (XPS) were conducted on a Physical Electronics Model 5400 X-ray photoelectron spectrometer using an unmonochromatized Mg Kα X-ray source.
2.2. Synthetic procedures
2.2.1 Synthesis of (E)-2-(((1H-imidazol-2-yl)methylene)amino)cyclohexan-1-amine (L2, denoted mono-Schiff base).
1,2-Diaminocyclohexane (114.2 mg, 1.0 mmol) was dissolved in MeOH (20 mL) in a 100 mL flask. 1 M hydrochloric acid (1.1 mL) was slowly added to this solution and then stirred for 20 minutes. The solution of imidazole-2-carboxaldehyde (L1, 96.0 mg, 1.0 mmol) in MeOH (10 mL) was slowly added to the above system, and then the mixture was refluxed overnight. Finally, the resulting suspension was adjusted to a pH of ∼8.0 by the saturated NaHSO3 aqueous solution (Scheme S1†). The product was further purified by recrystallization using MeOH.
2.2.2 Synthesis of (1E,1′E)-N,N′-(cyclohexane-1,2-diyl)bis(1-(1H-imidazol-2-yl) methanimine) (L3, denoted bis-Schiff base).
1,2-Diaminocyclohexane (114.2 mg, 1.0 mmol) and imidazole-2-carboxaldehyde (192.0 mg, 2.0 mmol) were dissolved in MeOH (40 mL) in a 100 mL flask, and the mixture was refluxed overnight (Scheme S2†). The resulting product was obtained by filtration, and washed with fresh methanol.
2.2.3 Synthesis of ZIF-90.
ZIF-90 was prepared according to the previously reported procedure.18 A mixture of imidazole-2-carboxaldehyde (96.0 mg, 1.0 mmol) and sodium formate (17.0 mg, 0.25 mmol) was added in MeOH (10 mL), and then sonicated for 20 minutes to form a clear yellow solution, followed by the addition of Zn(NO3)2·6H2O (74.4 mg, 0.25 mmol). The obtained solution was transferred into a Teflon-lined stainless steel autoclave, and kept in an oven at 353 K for 24 h. The autoclave was then taken out and cooled to room temperature. The polyhedral crystals were collected by filtration, washed with fresh methanol, and then dried under vacuum at 373 K for one day.
2.2.4 Synthesis of ZIF-90-I–ZIF-90-III.
The synthetic routes of ZIF-90-I–ZIF-90-III are briefly described in Scheme 1.
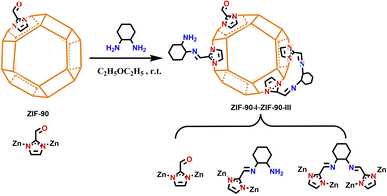 |
| Scheme 1 Transformation of ZIF-90 by Schiff base reaction with 1,2-diaminocyclohexane to obtain ZIF-90-I–ZIF-90-III. | |
2.2.5 Synthesis of ZIF-90-I.
ZIF-90 crystals (50.0 mg) and 1,2-diaminocyclohexane (46.0 mg) were suspended in ethoxyethane (10 mL), and stirred overnight at room temperature. The powder was then centrifuged and washed with fresh ethoxyethane (3 × 10 mL) before being immersed in fresh methanol (20 mL) for two days. The powder was finally collected by filtration, and then dried under vacuum at 373 K for one day. Molar ratio of L1
:
L2
:
L3 = 67.6%
:
25.7%
:
6.7%.
2.2.6 Synthesis of ZIF-90-II.
The method of preparing ZIF-90-II is similar to the procedure of ZIF-90-I, except that 120.0 mg 1,2-diaminocyclohexane was used. Molar ratio of L1
:
L2
:
L3 = 8.8%
:
58.4%
:
32.7%.
2.2.7 Synthesis of ZIF-90-III.
The method of preparing ZIF-90-III is similar to the procedure of ZIF-90-I, except that 250.0 mg 1,2-diaminocyclohexane was used. Molar ratio of L1
:
L2
:
L3 = 1.0%
:
96.1%
:
2.9%.
2.3. Iodine adsorption and desorption experiments
The iodine vapor adsorption experiments of ZIF-90 and ZIF-90-I–ZIF-90-III. 10 mg of activated ZIF-90 was placed in a pre-weighed 2 mL glass vial, and this glass vial was then put in a 20 mL sealed vial containing 300 mg of iodine pills, followed by being heated in an oven at 75 °C under ambient pressure. After a scheduled time, the iodine-loaded ZIF-90 was cooled down to room temperature in a desiccator and then weighed. The colorless ZIF-90 samples became dark brown. The iodine vapor adsorption experiments of ZIF-90-I–ZIF-90-III are similar to the adsorption process of ZIF-90, except that ZIF-90 is replaced by ZIF-90-I–ZIF-90-III, respectively.
The iodine adsorption experiments of ZIF-90 and ZIF-90-I–ZIF-90-III from iodine/cyclohexane solution. 5 mg of activated ZIF-90 was soaked in 5 mL of iodine/cyclohexane solution with different concentrations (200–800 mg L−1) under magnetic stirring at room temperature. The colorless ZIF-90 samples became dark brown, while the purple iodine/cyclohexane solution faded to colorless with increasing time. After a certain time, the supernatant was collected by centrifugal separation and its absorbance was monitored by a UV-vis spectrophotometer. Additionally, in order to obtain the maximum iodine adsorption amount of ZIF-90 in iodine/cyclohexane solution, 5 mg of activated ZIF-90 was soaked in 15 mL of 500, 700 and 1000 mg L−1 iodine/cyclohexane solution, and the abovementioned procedure was performed. The iodine adsorption experiments of ZIF-90-I–ZIF-90-III in iodine/cyclohexane solution are similar to the adsorption process of ZIF-90, except that ZIF-90 is replaced by ZIF-90-I–ZIF-90-III, respectively.
The iodine desorption experiments of iodine-loaded ZIF-90 and ZIF-90-I–ZIF-90-III. 10 mg of iodine-loaded ZIF-90 was placed in a pre-weighed 2 mL glass vial at 120 °C under ambient pressure. After a certain time, the glass vial was cooled down to room temperature in a desiccator and then weighed. The dark brown iodine-loaded ZIF-90 samples faded over time. The iodine desorption experiments of iodine-loaded ZIF-90-I–ZIF-90-III are similar to the desorption process of iodine-loaded ZIF-90, except that iodine-loaded ZIF-90 was replaced by iodine-loaded ZIF-90-I–ZIF-90-III, respectively.
3 Results and discussion
3.1. Characterization of ZIF-90-I–ZIF-90-III
The structures of ZIF-90-I–ZIF-90-III were characterized by powder X-ray diffraction (PXRD) and Fourier transform infrared spectroscopy (FTIR) measurements. As shown in Fig. S1,† the PXRD patterns of ZIF-90-I–ZIF-90-III are in agreement with the ones of experimental and simulated ZIF-90 generated from single crystal X-ray diffraction data, indicating that ZIF-90-I–ZIF-90-III retained the crystallinity of ZIF-90, and no crystal impurities were introduced during the post-synthetic modification procedure. The FTIR spectrum showed the presence of a C
O stretching vibration at 1680 cm−1 for ZIF-90, whereas there was the appearance of a C
N stretching vibration at 1640 cm−1 for ZIF-90-I–ZIF-90-III, indicating the transformation from the aldehyde group to Schiff bases of ZIF-90. Additionally, the features at 3300 and 3144 cm−1 can be assigned to the symmetric and asymmetric NH2 stretching vibrations, 2928 cm−1 belongs to the CH/CH2 stretching vibrations, 1581 cm−1 is attributed to the in-plane NH2 vibrations and the out-of-plane NH2 vibrations are situated in the 940–770 cm−1 region (Fig. S2†).19,20 These characteristics also revealed that 1,2-diaminocyclohexane has been anchored on ZIF-90via the Schiff base reaction. Additionally, the N2 adsorption isotherms of the activated samples of ZIF-90 and ZIF-90-I–ZIF-90-III were recorded at 77 K (Fig. S3†). The very low N2 uptakes of ZIF-90-I–ZIF-90-III relative to that of ZIF-90 may be attributed to an obvious constriction of the pores,21 resulting from the conversion of the small size aldehyde to the bigger size Schiff bases. The SEM images showed that the morphology of ZIF-90 was changed from a polyhedron to an irregular shape because of the post-synthetic modification. ZIF-90-I–ZIF-90-III exhibited almost the same particle size, which is obviously smaller than that of ZIF-90 (Fig. S4†). A smaller particle size of the materials generally leads to a higher gas adsorption capacity, but ZIF-90-I–ZIF-90-III presented a lower N2 adsorption capacity. Thus, the constriction of the pore through turning the aldehyde groups into Schiff bases probably played a major role in N2 adsorption.
In order to confirm the quantitative conversion from the aldehyde group of ZIF-90 to mono- and bis-Schiff bases (L2 and L3), activated ZIF-90-I–ZIF-90-III (10.0 mg) was put into 3 mL of MeOH to form a suspension under stirring. This mixture was then digested via the addition of 10% HCOOH/MeOH to adjust to a final pH of 5–6. The clear solution was evaporated and the resulting powder was dissolved in DMSO-d6 to measure 1H NMR, respectively. As shown in Fig. S5–S8,† the 1H NMR spectra of the digested ZIF-90-I–ZIF-90-III samples show that resonances occur at around 8.32, 8.15 and 8.04 ppm, which correspond to the imidazole-2-carboxaldehyde, mono- and bis-Schiff bases, indicating that approximately 32.4%, 91.2% and 99% conversion of the aldehyde group had been completed in ZIF-90-I–ZIF-90-III, respectively. The neighboring distance of the aldehyde groups in ZIF-90 is around 4.3 Å, which is close to the sum of the N…N distance from 1,2-diaminocyclohexane and C
N distance from the Schiff base. Thus, the aldehyde groups in ZIF-90 can be converted to bis-Schiff bases during the process of post-synthetic modification. However, the transformation of the bis-Schiff base is not very high (∼30%) owing to the steric hindrance effect. Meanwhile, we also carried out high-resolution electrospray ionization mass spectrometry (HRESI-MS) on these digested samples of ZIF-90-I–ZIF-90-III. As depicted in Fig. S9–S12,† compared with the HRESI-MS of the digested ZIF-90, four new m/z peaks were clearly observed for the digested ZIF-90-I–ZIF-90-III samples at 255.06, 273.06, 301.06 and 333.09, respectively. Fitting these peaks gives a formula of [Zn(L2)]+ (calcu. 255.06), [Zn(L2)(H2O)]+ (calcu. 273.07), [Zn(L2)(HCOOH)]+ (calcu. 301.06) and [Zn(L3)]+ (calcu. 333.08) (Fig. S13†). The existence of these m/z peaks relevant to L2 and L3 fragments also provided further evidence for the transformation from aldehyde group of ZIF-90 to Schiff bases. The speculated chemical formulae of the fragments are listed in Tables S1–S4.†
3.2. Iodine vapor capture of ZIF-90-I–ZIF-90-III
ZIFs such as ZIF-8 and ZIF-90 with suitable cage sizes and excellent chemical and thermal stability, easily form charge-transfer compounds between the iodine molecule and framework. Thus, they are viewed as ideal candidates for trapping iodine.11–13 Meanwhile, the introduction of C
N with a π electron and NH2 group featuring a lone pair electron will increase the electron density of the organic linkers and further enhances the iodine…framework interactions, leading to the improvement of the iodine adsorption amount. Iodine vapor capture experiments were performed on activated samples of ZIF-90 and ZIF-90-I–ZIF-90-III at 75 °C under ambient pressure. The iodine adsorption isotherms of these samples were depicted according to the equation of Qt = 1000 × (mt − m0)/m0, respectively, where Qt (mg g−1) is the adsorption amount of iodine vapor per gram of sample at time t, m0 (mg) is the initial mass of sample and mt (mg) is the mass of the sample after adsorption at time t.
As shown in Fig. 1a, the time of iodine adsorption equilibrium was about 60 hours with excellent iodine adsorption capacity values of 4490, 5270, 5680 and 6600 mg g−1 for ZIF-90 and ZIF-90-I–ZIF-90-III, respectively. The iodine adsorption capacity of ZIF-90-III is 1.5-fold that of ZIF-90. Interestingly, the iodine vapor adsorption capacities were enhanced with the increasing conversion of aldehyde to Schiff base, especially with the increasing ratio of mono-Schiff base. In addition, ZIF-90-I–ZIF-90-III possessed the approximate particle sizes, but they exhibited different iodine adsorption capacities, revealing that maybe the particle sizes did not distinctly affect the iodine adsorption of the ZIF-90 derivatives. Therefore, the iodine adsorption behavior of ZIF-90 and ZIF-90-I–ZIF-90-III indicates that not only suitable cage sizes and charge-transfer interaction between the iodine molecule and aromatic imidazole ring play an important role in iodine vapor adsorption, but the presence of C
N with the π electron and NH2 group with the lone pair electron also contributes to increasing interactions between the electron-deficient iodine molecule and framework, thus leading to the obvious increase of iodine adsorption in post-synthetic ZIF-90 derivatives. To our best knowledge, compared to the previously reported metal–organic frameworks (Fig. 1b and Table S5†), the iodine vapor adsorption capacities of ZIF-90 and ZIF-90-I–ZIF-90-III are only lower than that of IL@PCN-333 (Al) (7350 mg g−1)22 and obviously superior to those of most MOFs, such as PCN-333 (Al) (4420 mg g−1),23UPC-158-HCl (2920 mg g−1),24etc. Noteworthily, the iodine vapor adsorption value of ZIF-90-III ranks second among the reported MOFs under the same conditions to date.
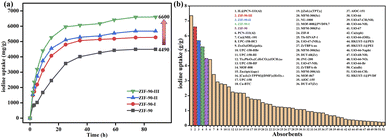 |
| Fig. 1 (a) Iodine adsorption isotherms of ZIF-90 and ZIF-90-I–ZIF-90-III at 75 °C under ambient pressure, and (b) comparison of the iodine vapor adsorption capacity by reported metal–organic frameworks and our materials. | |
To further investigate the iodine adsorption behavior of ZIF-90 and ZIF-90-I–ZIF-90-III, iodine vapor adsorption experiments of these materials were carried out at different temperatures (40, 50, 60 and 90 °C) under ambient pressure, respectively, and their corresponding iodine adsorption isotherms and the equilibrium adsorption amounts were obtained (Fig. S14 and Table S6†). For ZIF-90 and ZIF-90-I–ZIF-90-III, the times of iodine adsorption equilibrium were obviously shortened, while the iodine adsorption capacity values were significantly enhanced with increasing temperature. Furthermore, the iodine adsorption capacity of ZIF-90-II and ZIF-90-III at 90 °C is slightly lower than that at 75 °C.
Meanwhile, the pseudo-first and pseudo-second order kinetic models were adopted to probe the iodine adsorption kinetics of ZIF-90 and ZIF-90-I–ZIF-90-III, and the corresponding kinetic equations were listed as follows:
Pseudo-first order kinetic model: Qt = Qe − Qe × e−k1t |
Pseudo-second order kinetic model: t/Qt = 1/k2Qe2 + t/Qe |
where
k1 (h
−1) and
k2 (g mg
−1 h
−1) are the pseudo-first and pseudo-second order rate constants, respectively,
Qt (mg g
−1) and
Qe (mg g
−1) are respectively the adsorption amount of iodine vapor by per gram of sample at time
t and equilibrium. As depicted in
Fig. 2 and S15–S23,
† and according to the correlation coefficients (
R2) and calculated value of
Qe (mg g
−1) obtained by the pseudo-first and pseudo-second order kinetic equations at different temperatures (Tables S7–S10
†), the iodine vapor adsorption of
ZIF-90 can be described by the pseudo-first order kinetic equation, indicating that the diffusion of the iodine molecule
via pores was the rate-controlling mechanism for iodine vapor adsorption by
ZIF-90.
25,26 While the pseudo-second order kinetic equation better favored the iodine vapor adsorption by
ZIF-90-I–ZIF-90-III, revealing that chemisorption is predominant during the absorption progress for these post-synthetic
ZIF-90 derivatives.
27,28 Meanwhile, the order rate constants of
k1 and
k2 obviously increased with increasing temperature, probably ascribed to the more mobile iodine at higher temperature.
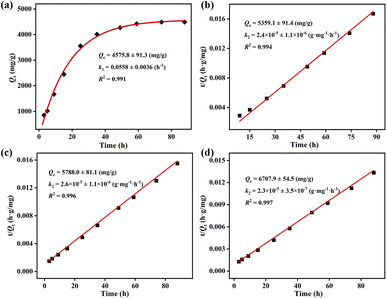 |
| Fig. 2 (a) The pseudo-first kinetic model for the iodine vapor adsorption kinetics of ZIF-90, the pseudo-second kinetic models for the iodine vapor adsorption kinetics of (b) ZIF-90-I, (c) ZIF-90-II and (d) ZIF-90-III at 75 °C under ambient pressure. | |
The Arrhenius equation k = A
exp(−Ea/RT) was adopted to calculate the activation energy (Ea) during the iodine vapor adsorption process by ZIF-90 and ZIF-90-I–ZIF-90-III, where k is the pseudo-first order rate constant for ZIF-90 and the pseudo-second order rate constant for ZIF-90-I–ZIF-90-III, respectively, A is the pre-exponential factor, R = 8.314 J mol−1 K−1 and T is the Kelvin temperature. As shown in Fig. 3, the values of activation energy are 34.2 ± 1.5, 25.2 ± 0.9, 24.0 ± 0.9 and 23.1 ± 0.8 kJ mol−1 for ZIF-90 and ZIF-90-I–ZIF-90-III, and all of them are lower than 40 kJ mol−1. The lower activation energy implies the quicker diffusion of the iodine molecule, further leading to the high iodine adsorption capacity.29,30 Thus, the sequence of the iodine vapor adsorption amount was ZIF-90-III > ZIF-90-II > ZIF-90-I > ZIF-90, which is coincident with the experimental values.
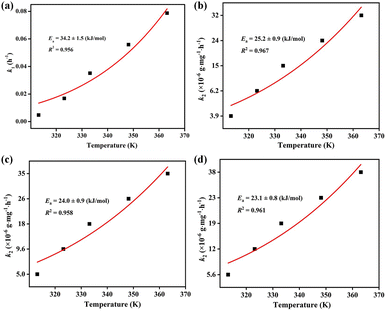 |
| Fig. 3 Arrhenius fitting of the iodine vapor adsorption by (a) ZIF-90, (b) ZIF-90-I, (c) ZIF-90-II and (d)ZIF-90-III. | |
3.3. Iodine adsorption from iodine/cyclohexane solution by ZIF-90-I–ZIF-90-III
To determine the iodine adsorption capacity of ZIF-90 and ZIF-90-I–ZIF-90-III in the iodine/cyclohexane solution, the standard curve of the iodine/cyclohexane solution was also measured (Fig. S24†). The absorbance at 522 nm and the concentration of the iodine/cyclohexane solution display an excellent linear relationship, which can be described using the equation of y = 0.00388x − 0.01509, where y and x are the absorbance at 522 nm and the concentration of iodine/cyclohexane solution, respectively. As shown in Fig. S25–S32,† the absorbance at 522 nm of the iodine/cyclohexane solution obviously decreased with increasing time after ZIF-90 and ZIF-90-I–ZIF-90-III were soaked in this solution, respectively, indicating that iodine was adsorbed by these materials. The residual concentration of iodine in cyclohexane can be speculated by the equation of y = 0.00388x − 0.01509, and the iodine adsorption values were calculated using the following equation: Qt = (C0 − Ct) × V÷m, where Qt (mg g−1) is the iodine adsorption amount from the iodine/cyclohexane solution per gram of samples at time t, C0 (mg L−1) is the initial concentration of the iodine/cyclohexane solution, Ct (mg L−1) is the concentration of the iodine/cyclohexane solution after adsorption at time t, V (mL) is the volume of iodine/cyclohexane solution, and m (mg) is the mass of the sample.
As shown in Fig. 4, S33 and Table S11,† the maximum iodine equilibrium adsorption amounts were 538, 1005, 1461 and 1826 mg g−1 for ZIF-90 and ZIF-90-I–ZIF-90-III, respectively, and the iodine adsorption capacity of ZIF-90-III is 3.4-fold the value for ZIF-90. Similar to the iodine vapor adsorption behavior, the iodine adsorption capacity of ZIF-90 in the iodine/cyclohexane solution was also enhanced with the increasing conversion of the aldehyde to a Schiff base, especially with increasing ratio of the mono-Schiff base. This behavior also indicates that the iodine adsorption capacity of the materials in the iodine/cyclohexane solution is not only ascribed to the suitable cage size and charge-transfer interaction between the iodine molecule and aromatic imidazole ring, but also results from the introduction of C
N and NH2 groups with rich electrons. These factors are beneficial to forming multipoint interactions between the iodine molecule and framework, and further improving the iodine adsorption capacity of compounds. To our best knowledge, compared to the previously reported MOFs (Fig. 4 and Table S12†), the iodine adsorption values of ZIF-90-II and ZIF-90-III are lower than those for IL@PCN-333 (Al) (3400 mg g−1),22 Ti16Pb5O16(C6H5CO2)2(OCH3)40 (3127 mg g−1)31 and Ag@MIL-101 (2140 mg g−1),32 but higher than that of most known MOFs, among the highest iodine adsorption capacity.
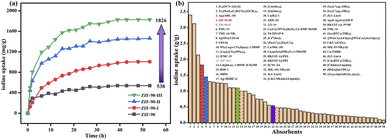 |
| Fig. 4 (a) Iodine adsorption isotherms of ZIF-90 and ZIF-90-I–ZIF-90-III from 15 mL of 1000 mg L−1 iodine/cyclohexane solution, and (b) comparison of the iodine adsorption capacity from iodine solution by reported metal–organic frameworks and our materials. | |
The pseudo-first and pseudo-second order kinetic models were also adopted to study the iodine adsorption kinetics of ZIF-90 and ZIF-90-I–ZIF-90-III in the iodine/cyclohexane solution. As shown in Fig. S34–S49 and Tables S13–S16,† and according to R2 and the calculated values of Qe (mg g−1) obtained via the pseudo-first and pseudo-second order kinetic equations, the pseudo-second order kinetic equation better describes the iodine adsorption by ZIF-90 and ZIF-90-I–ZIF-90-III, indicating that chemisorption exists in the iodine absorption progress.33,34 Additionally, the iodine absorption isotherms of ZIF-90 and ZIF-90-I–ZIF-90-III in the iodine/cyclohexane solution were also further explored via Langmuir and Freundlich isotherm model, respectively, and these two isotherm models are expressed by the following equations:
Langmuir isotherm model: Qe = (QmKLCe)/(1 + KLCe) |
Freundlich isotherm model: Qe = KFCe1/n |
where
Qe (mg g
−1) and
Ce (mg L
−1) are the equilibrium iodine adsorption amount and concentration of the iodine/cyclohexane solution, respectively,
Qm (mg g
−1) is the theoretical maximum adsorption value,
KL (L mg
−1) and
KF (mg g
−1) are the adsorption constants of Langmuir and Freundlich isotherm model, respectively, and
n is the Freundlich linearity index. As shown in
Fig. 5 and the related parameters obtained from the Langmuir and Freundlich isotherm models (Table S17
†), the Langmuir isotherm model is in better agreement with the experimental adsorption isotherm for
ZIF-90 and
ZIF-90-I–ZIF-90-III than the Freundlich isotherm model, indicating that the iodine adsorption process in the iodine/cyclohexane solution by these materials probably due to the monolayer chemisorption occurring on the heterogeneous surfaces.
5,33
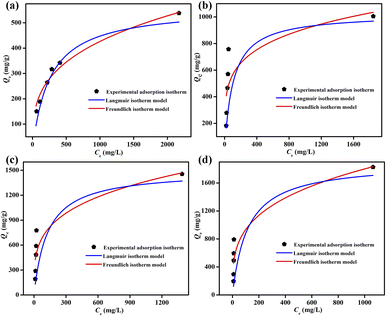 |
| Fig. 5 The fitting of the iodine adsorption isotherms adopting the Langmuir and Freundlich isotherm models for (a) ZIF-90, (b) ZIF-90-I, (c) ZIF-90-II and (d) ZIF-90-III. High Ce values were approximatively obtained using the adsorption data of 15 mL iodine/cyclohexane solution with different concentrations. | |
3.4. Characterization of iodine-loaded ZIF-90 and ZIF-90-I–ZIF-90-III
Raman spectra and XPS were carried out on pristine and iodine-loaded ZIF-90 and ZIF-90-I–ZIF-90-III (including these samples that adsorbed iodine from iodine vapor and iodine/cyclohexane solution, respectively) to detect the probable species of iodine in these iodine-loaded materials, and explore the interactions between the iodine molecule and framework. As shown in Fig. 6, two strong peaks of 112 and 168 cm−1 were detected on the Raman spectrum of the iodine-loaded ZIF-90 and ZIF-90-I–ZIF-90-III. The peak of 168 cm−1 may be ascribed to the iodine molecule, which was shifted by 12 cm−1 and is comparable to the I–I vibration of solid iodine (180 cm−1) (Fig. S50†). This reveals that the adsorbed iodine molecules were bound to the framework, and probably due to the formation of hydrogen bonds between the iodine molecule and the NH2 group from the framework or the formation of a partial dipole moment between the iodine molecule and electron-rich organic linkers with the C
N bond and aromatic ring.34 The peak of 112 cm−1 probably corresponds to the I3− species. This may be a result from the charge transfer between the anti-bond I2 molecular orbital and the lone pair orbital of the O/N atom from organic linkers, and the iodine molecule was subsequently polarized to form I3− anions.35
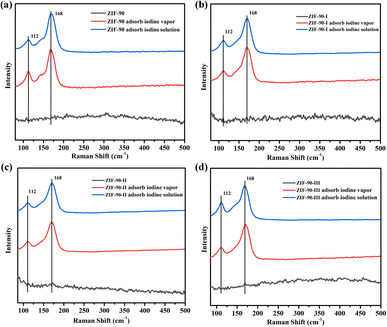 |
| Fig. 6 Raman spectra of the pristine and iodine-loaded (a) ZIF-90, (b) ZIF-90-I, (c) ZIF-90-II and (d) ZIF-90-III. | |
Meanwhile, the binding energies for the 3d5/2 and 3d3/2 orbitals of iodine theoretically are 620.1 and 630.6 eV, respectively, and the binding energies will slightly shift due to the host–guest interaction. As shown in Fig. 7, two couples binding energies were observed by XPS of the iodine-loaded ZIF-90 and ZIF-90-I–ZIF-90-III. The binding energies of 620.6 and 632.0 eV probably are ascribed to the iodine molecules, and the binding energies of 619.0 (619.1) and 630.4 (630.5) eV probably occur because of the existence of the I3− species.36 Thus, both Raman spectra and XPS suggested that both I2 and I3− species existed in the iodine-loaded ZIF-90 and its post-synthetic derivatives. The production of the I3− species also proved that chemical adsorption occurred in the process of iodine adsorption from iodine vapor and iodine/cyclohexane solution by these mentioned materials, further confirming the formation of charge-transfer compounds between the iodine molecule and aromatic ring.11–13
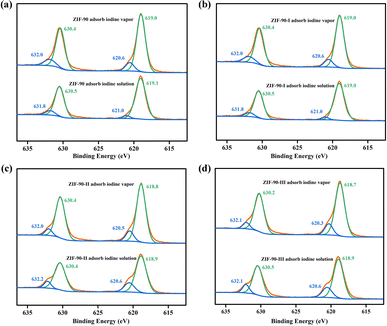 |
| Fig. 7 XPS of the iodine-loaded (a) ZIF-90, (b) ZIF-90-I, (c) ZIF-90-II and (d) ZIF-90-III. | |
3.5. Theoretical insight into the iodine molecule adsorption of ZIF-90 and ZIF-90-I–ZIF-90-III
Taking the characteristic of the multicomponent structure of ZIF-90-I–ZIF-90-III into consideration, it is difficult to directly analyze the interactions between iodine and ZIF-90-I–ZIF-90-III. So many efforts have been carried out on synthesizing single crystals of ZIF-90 with pure mono-Schiff base (ZIF-90-mono), ZIF-90 with pure bis-Schiff base (ZIF-90-bis) and ZIF-90 with mono-Schiff base and bis-Schiff base (ZIF-90-mono-bis). Unfortunately, no corresponding crystals were gained. Therefore, we performed density functional theory (DFT) calculations37,38 with Gaussian 16 A.03 program39 to investigate the interactions between iodine and compounds ZIF-90-mono/ZIF-90-bis based on the cluster model.40,41 The geometry optimizations and electronic structure calculations were implemented at the PBE1PBE level42 with GD3BJ dispersion corrections.43 The def2-TZVPD basis set44 was adopted for Zn and I atoms, while the 6-31++G(d,p) basis set was used for other atoms. In order to further improve the computational efficiency, the diaminocyclohexane molecules were replaced by NH3 to generate one side-edge Schiff base in the studied clusters. The final optimized clusters for ZIF-90-mono and ZIF-90-bis are shown in Fig. S51.† The binding energy (ΔE) for an iodine molecule loaded on the specified cluster can be expressed as
ΔE = Ecomposite − EI2 − Ecluster |
where Ecomposite is the energy of an iodine molecule absorbed on the specified cluster, and EI2 and Ecluster are the energies of an iodine molecule and the specified cluster, respectively. In addition, the Mulliken charges45 were adopted to evaluate the charge transfers between the clusters and iodine molecules.
As shown in Fig. S52,† I2 is adjacent with the aldehyde groups from imidazole-2-carboxaldehyde in the iodine-loaded ZIF-90, and the relatively short I…framework distances ranged from 2.775 to 3.890 Å. In the iodine-loaded ZIF-90-mono, I2 approaches a –NH2 group from an individual diaminocyclohexane molecule, as well as near the imidazole and cyclohexane rings. The distances between I and these groups are in the range of 2.947–3.349 Å. In the iodine-loaded ZIF-90-bis, I2 is close to the imidazole rings from the bis-Schiff base, and the longer distances of 3.146 and 3.996 Å exist between iodine and the framework. Meanwhile, the I–I distances are 2.674, 2.659 and 2.656 Å in the iodine-loaded ZIF-90, ZIF-90-mono and ZIF-90-bis, respectively, slightly longer than the distance of the free iodine molecule optimized at the PBE1PBE/def2-TZVPD level (2.654 Å), revealing that affinities exist in these frameworks and iodine atom. As listed in Table S18,† the transferred charges from ZIF-90, ZIF-90-mono and ZIF-90-bis to the iodine molecule are −0.172, −0.183 and −0.134e, respectively. The binding energies between the iodine molecule and the abovementioned compounds are −0.664, −0.687 and −0.574 eV, respectively, implying that the strongest interaction may be generated between ZIF-90-mono and the iodine molecule, while the weakest affinity probably formed between ZIF-90-bis and the iodine molecule. Based on these calculation results, we speculate that materials with a higher ratio of mono-Schiff base and lower ratio of bis-Schiff base will generate stronger binding forces between iodine and the framework, further leading to a higher iodine uptake capacity for these materials. This deduction is consistent with the aforementioned iodine adsorption experiments, in which ZIF-90-III with the highest amount of mono-Schiff bases has the maximum iodine uptake capacity, and also matches well with the subsequent iodine release from iodine-loaded materials.
3.6. Iodine release from iodine-loaded ZIF-90 and ZIF-90-I–ZIF-90-III
In order to probe the reversibility of ZIF-90 and ZIF-90-I–ZIF-90-III, the iodine desorption experiments of these iodine-loaded materials (including these samples adsorbed iodine from iodine vapor and iodine/cyclohexane solution) were performed via heating at 120 °C, respectively. The iodine desorption capacity was estimated by the equation of
, where R% is the iodine release from the iodine-loaded material at time t,
is the initial mass of the iodine-loaded material and
is the mass of the iodine-loaded material after desorption at time t. As shown in Fig. S53,† the release rate of iodine was very fast in the initial first hour and then it became slow. Finally, the desorption approached the equilibrium after 2 hours with a recovery rate of 93.1%, 85.1%, 82.6% and 80.7% for the iodine-loaded ZIF-90 and ZIF-90-I–ZIF-90-III with iodine coming from iodine vapor, and the recovery rate of 94.2%, 87.2%, 84.8% and 82.7% for iodine-loaded ZIF-90 and ZIF-90-I–ZIF-90-III with iodine coming from iodine/cyclohexane, respectively. This phenomenon implied that strong interactions existed between iodine and the framework, and the sequence of the interactions between iodine and the framework is ZIF-90-III > ZIF-90-II > ZIF-90-I > ZIF-90, which is consistent with the theoretical calculation. The next iodine adsorption process cycle was carried out when one desorption process finished. After three cycles of the adsorption–desorption process, the iodine adsorption capacity of iodine vapor by ZIF-90 and ZIF-90-I–ZIF-90-III still retained a high adsorption amount of 4290, 4990, 5490, and 6450 mg g−1, corresponding to 95.6%, 94.7%, 96.7% and 97.7% of their initial capacity, respectively (Fig. S54†). It is noteworthy that the iodine adsorption capacities of ZIF-90-I–ZIF-90-III after three cycles is still superior to the initial ZIF-90 and only lower than the best iodine adsorption MOF material IL@PCN-333(Al),22 still being among the highest iodine adsorption values. Meanwhile, after three cycles of the adsorption–desorption process, the retained iodine adsorption capacities from the iodine/cyclohexane solution by ZIF-90 and ZIF-90-I–ZIF-90-III were 489, 897, 1288, and 1695 mg g−1, corresponding to 90.9%, 89.3%, 88.2% and 92.8% of their initial capacities, respectively (Fig. S55†). Furthermore, the iodine adsorption amount of ZIF-90-III was still ranked in the front of MOFs with iodine trap properties from the iodine solution. Additionally, PXRD patterns of the recovered ZIF-90 and ZIF-90-I–ZIF-90-III were carried out (Fig. S56†), and matched well with those of the as-synthesized ones. This indicated that these materials still kept their structures and crystallinity, further confirming the good stability and renewability of ZIF-90 and these ZIF-90 post-synthetic derivatives.
4 Conclusions
In conclusion, we synthesized a series of ZIF-90 derivatives with rich electrons via post-synthetic modification, and the aldehyde group of ZIF-90 was quantitatively converted to mono- and di-Schiff bases. Iodine adsorption experiments revealed that the uptake capacities of the ZIF-90 derivatives are superior to that of ZIF-90. Among these ZIF-90 derivatives, ZIF-90-III showed the maximum iodine adsorption capacity of 6600 mg g−1 and 1826 mg g−1 for iodine vapor and iodine/cyclohexane solution, respectively, being among the highest known to date for metal–organic frameworks. The values of the activation energies are 34.2 ± 1.5, 25.2 ± 0.9, 24.0 ± 0.9 and 23.1 ± 0.8 kJ mol−1 for ZIF-90 and ZIF-90-I–ZIF-90-III, respectively, and the iodine adsorption kinetics of the ZIF-90 and ZIF-90 derivatives were investigated in detail. Meanwhile, both I2 and I3− were observed in iodine-loaded ZIF-90 and ZIF-90 derivatives by XPS and Raman measurements. The renewable experiments indicated that our synthesized ZIF-90 derivatives still can retain high iodine adsorption values after three cycles. This research broadens the materials with high iodine adsorption properties, and provides a good method for the improvement of iodine…framework interactions, which is beneficial to developing more iodine trap materials.
Author contributions
Z. Zhang prepared and characterized ZIF-90 and its derivates, and analysed the data; Y. Chen and Y. Sun carried out the iodine adsorption experiments; Z.-Y. Zhang performed the theoretical calculation. F. Liang discussed the results of the theoretical calculation. Z. Chen and H.-C. Hu guided this research and wrote the manuscript. All authors commented on the manuscript.
Conflicts of interest
There are no conflicts to declare.
Acknowledgements
This work was supported by the National Natural Science Foundation of China (Grant No. 22261007, 12064002 and 22061004) and Guangxi Technology Base and Talent Subject (No. GUIKE AD23026067).
Notes and references
- P. C. Burns, R. C. Ewing and A. Navrotsky, Nuclear fuel in a reactor accident, Science, 2012, 335, 1184–1188 CrossRef CAS PubMed.
- X. Zhang, J. Maddock, T. M. Nenoff, M. A. Denecke, S. Yang and M. Schröder, Adsorption of iodine in metal–organic framework materials, Chem. Soc. Rev., 2022, 51, 3243–3262 RSC.
- T. Pan, K. Yang, X. Dong and Y. Han, Adsorption-based capture of iodine and organic iodides: status and challenges, J. Mater. Chem. A, 2023, 11, 5460–5475 RSC.
- W. Xie, D. Cui, S.-R. Zhang, Y.-H. Xu and D.-L. Jiang, Iodine capture in porous organic polymers and metal–organic frameworks materials, Mater. Horiz., 2019, 6, 1571–1595 RSC.
- M. Avais and S. Chattopadhyay, Porous polyaminoamides via an exotemplate synthesis approach for ultrahigh multimedia iodine adsorption, J. Mater. Chem. A, 2022, 10, 20090–20100 RSC.
- J. Chang, H. Li, J. Zhao, X. Guan, C. Li, G. Yu, V. Valtchev, Y. Yan, S. Qiu and Q. Fang, Tetrathiafulvalene-based covalent organic frameworks for ultrahigh iodine capture, Chem. Sci., 2021, 12, 8452–8457 RSC.
- L. Xie, Z. Zheng, Q. Lin, H. Zhou, X. Ji, J. L. Sessler and H. Wang, Calix[4]pyrrole-based crosslinked polymer networks for highly effective iodine adsorption from water, Angew. Chem., Int. Ed., 2022, 61, e202113724 CrossRef CAS PubMed.
- B. Azambre, M. Chebbi, O. Leroy and L. Cantrel, Effects of zeolitic parameters and irradiation on the retention properties of silver zeolites exposed to molecular iodine, Ind. Eng. Chem. Res., 2018, 57, 1468–1479 CrossRef CAS.
- X. He, L. Chen, X. Xiao, Y. Gan, J. Yu, J. Luo, H. Dan, Y. Wang, Y. Ding and T. Duan, Improved utilization of Cu0 for efficient adsorption of iodine in gas and solution by mesoporous Cu0-SBA-15 via solvothermal reduction method, Chem. Eng. J., 2023, 462, 142175 CrossRef CAS.
- S. Yao, W.-H. Fang, Y. Sun, S.-T. Wang and J. Zhang, Mesoporous assembly of aluminum molecular rings for iodine capture, J. Am. Chem. Soc., 2021, 143, 2325–2330 CrossRef CAS PubMed.
- C. Liu, Y. Jin, Z. Yu, L. Gong, H. Wang, B. Yu, W. Zhang and J. Jiang, Transformation of porous organic cages and covalent organic frameworks with efficient iodine vapor capture performance, J. Am. Chem. Soc., 2022, 144, 12390–12399 CrossRef CAS PubMed.
- D. F. Sava, M. A. Rodriguez, K. W. Chapman, P. J. Chupas, J. A. Greathouse, P. S. Crozier and T. M. Nenoff, Capture of volatile iodine, a gaseous fission product, by zeolitic imidazolate framework-8, J. Am. Chem. Soc., 2011, 133, 12398–12401 CrossRef CAS PubMed.
- J. T. Hughes, D. F. Sava, T. M. Nenoff and A. Navrotsky, Thermochemical evidence for strong iodine chemisorption by ZIF-8, J. Am. Chem. Soc., 2013, 135, 16256–16259 CrossRef CAS PubMed.
- Y. Lei, G. Zhang, Q. Zhang, L. Yu, H. Li, H. Yu and Y. He, Visualization of gaseous iodine adsorption on single zeolitic imidazolate framework-90 particles, Nat. Commun., 2021, 12, 4483 CrossRef CAS PubMed.
- M. Leloire, C. Walshe, P. Devaux, R. Giovine, S. Duval, T. Bousquet, S. Chibani, J. F. Paul, A. Moissette, H. Vezin, P. Nerisson, L. Cantrel, C. Volkringer and T. Loiseau, Capture of gaseous iodine in isoreticular zirconium-based UiO-n metal-organic frameworks:
influence of amino functionalization, DFT calculations, Raman and EPR spectroscopic investigation, Chem. – Eur. J., 2022, 28, e202104437–e202104453 CrossRef CAS PubMed.
- P. Chen, X. He, M. Pang, X. Dong, S. Zhao and W. Zhang, Iodine capture using Zr-based metal–organic frameworks (Zr-MOFs): adsorption performance and mechanism, ACS Appl. Mater. Interfaces, 2020, 12, 20429–20439 CrossRef CAS PubMed.
- J. Wang, L. Wang, Y. Wang, F. Yang, J. Li, X. Guan, J. Zong, F. Zhou, J. Huang and Y.-N. Liu, Covalently connected core-shell NH2-UiO-66@Br-COFs hybrid materials for CO2 capture and I2 vapor adsorption, Chem. Eng. J., 2022, 438, 135555 CrossRef CAS.
- W. Morris, C. J. Doonan, H. Furukawa, R. Banerjee and O. M. Yaghi, Crystals as molecules: postsynthesis covalent functionalization of zeolitic imidazolate frameworks, J. Am. Chem. Soc., 2008, 130, 12626–12627 CrossRef CAS PubMed.
- P. Kupser, K. Pagel, J. Oomens, N. Polfer, B. Koksch, G. Meijer and G. von. Helden, Amide-I and -II vibrations of the cyclic β-sheet model peptide gramicidins in the gas phase, J. Am. Chem. Soc., 2010, 132, 2085–2093 CrossRef CAS PubMed.
- A. K. Eckhardt and P. R. Schreiner, Spectroscopic evidence for aminomethylene (H–C–NH2) – the simplest amino carbine, Angew. Chem., Int. Ed., 2018, 57, 5248–5252 CrossRef CAS PubMed.
- B. Ghalei, K. Wakimoto, C. Y. Wu, A. P. Isfahani, T. Yamamoto, K. Sakurai, M. Higuchi, B. K. Chang, S. Kitagawa and E. Sivaniah, Rational tuning of zirconium metal–organic framework membranes for hydrogen purification, Angew. Chem., Int. Ed., 2019, 58, 19034–19040 CrossRef CAS PubMed.
- Y. Tang, H. Huang, J. Li, W. Xue and C. Zhong, IL-induced formation of dynamic complex iodide anions in IL@MOF composites for efficient iodine capture, J. Mater. Chem. A, 2019, 7, 18324–18329 RSC.
- B. Qi, Y. Liu, T. Zheng, Q. Gao, X. Yan, Y. Jiao and Y. Yang, Highly efficient capture of iodine by Cu/MIL-101, J. Solid State Chem., 2018, 258, 49–55 CrossRef CAS.
- B. Guo, F. Li, C. Wang, L. Zhang and D. Sun, A rare (3,12)-connected zirconium metal–organic framework with efficient iodine adsorption capacity and pH sensing, J. Mater. Chem. A, 2019, 7, 13173–13179 RSC.
- K. M. A. Qasem, S. Khan, M. N. Ahamad, H. A. M. Saleh, M. Ahmad and M. Shahid, Radioactive iodine capture by metal organic frameworks in liquid and vapour phases: an experimental, kinetic and mechanistic study, J. Environ. Chem. Eng., 2021, 9, 106720 CrossRef CAS.
- B. Liu, X. Ren, L. Chen, X. Ma, Q. Chen, Q. Sun, L. Zhang, P. Si and L. Ci, High efficient adsorption and storage of iodine on S, N co-doped graphene aerogel, J. Hazard. Mater., 2019, 373, 705–715 CrossRef CAS PubMed.
- Z. J. Li, Y. Ju, Y. Ju, B. Yu, X. Wu, H. Lu, Y. Li, J. Zhou, X. Guo, Z. H. Zhang, J. Lin, J. Q. Wang, J. Q. Wang and S. Wang, Modulated synthesis and isoreticular expansion of Th-MOFs with record high pore volume and surface area for iodine adsorption, Chem. Commun., 2020, 56, 6715–6718 RSC.
- Z. Changani, A. Razmjou, A. Taheri-Kafrani, M. E. Warkiani and M. Asadnia, Surface modification of polypropylene membrane for the removal of iodine using polydopamine chemistry, Chemosphere, 2020, 249, 126079 CrossRef CAS PubMed.
- M. Al-Ghouti, M. A. M. Khraisheh, M. N. M. Ahmad and S. Allen, Thermodynamic
behaviour and the effect of temperature on the removal of dyes from aqueous solution using modified diatomite: a kinetic study, J. Colloid Interface Sci., 2005, 287, 6–13 CrossRef CAS PubMed.
- V. Vaid and R. Jindal, An efficient pH-responsive kappa-carrageenan/tamarind kernel powder hydrogel for the removal of brilliant green and rose bengal from aqueous solution, J. Appl. Polym. Sci., 2022, 139, e52218 CrossRef.
- A. Said, C. Gao, C. Liu, H. Niu, D. Wang, Y. Liu, L. Du, C. H. Tung and Y. Wang, A mesoporous lead-doped titanium oxide compound with high performance and recyclability in I2 uptake and photocatalysis, Inorg. Chem., 2022, 61, 586–596 CrossRef CAS PubMed.
- P. Mao, B. Qi, Y. Liu, L. Zhao, Y. Jiao, Y. Zhang, Z. Jiang, Q. Li, J. Wang, S. Chen and Y. Yang, Highly efficient capture of iodine by Cu/MIL-101, J. Solid State Chem., 2016, 237, 274–283 CrossRef CAS.
- S. Sun, X. Sha, J. Liang, G. Yang, X. Hu, Z. He, M. Liu, N. Zhou, X. Zhang and Y. Wei, Rapid synthesis of polyimidazole functionalized MXene via microwave-irradiation assisted multi-component reaction and its iodine adsorption performance, J. Hazard. Mater., 2021, 420, 126580 CrossRef CAS PubMed.
- C. Liu, W. Li, Y. Liu, H. Wang, B. Yu, Z. Bao and J. Jiang, Porous organic cages for efficient gas selective separation and iodine capture, Chem. Eng. J., 2022, 428, 131129 CrossRef CAS.
- K. Cheng, H. Li, J.-R. Wang, P.-Z. Li and Y. Zhao, From supramolecular organic cages to porous covalent organic frameworks for enhancing iodine adsorption capability by fully exposed nitrogen-rich sites, Small, 2023, 2301998 CrossRef CAS PubMed.
- H.-J. Noh, Y.-K. Im, S.-Y. Yu, J.-M. Seo, J. Mahmood, T. Yildirim and J.-B. Baek, Vertical two-dimensional layered fused aromatic ladder structure, Nat. Commun., 2020, 11, 2021 CrossRef CAS PubMed.
- W. Kohn and L. J. Sham, Self-consistent equations including exchange and correlation effects, Phys. Rev., 1965, 140, A1133 CrossRef.
-
R. G. Parr and W. Yang, Density-Functional Theory of Atoms and Molecules, Oxford Univ. Press, Oxford, 1989 Search PubMed.
-
M. J. Frisch, G. W. Trucks, H. B. Schlegel, G. E. Scuseria, M. A. Robb, J. R. Cheeseman, G. Scalmani, V. Barone, G. A. Petersson, H. Nakatsuji, X. Li, M. Caricato, A. V. Marenich, J. Bloino, B. G. Janesko, R. Gomperts, B. Mennucci, H. P. Hratchian, J. V. Ortiz, A. F. Izmaylov, J. L. Sonnenberg, D. Williams-Young, F. Ding, F. Lipparini, F. Egidi, J. Goings, B. Peng, A. Petrone, T. Henderson, D. Ranasinghe, V. G. Zakrzewski, J. Gao, N. Rega, G. Zheng, W. Liang, M. Hada, M. Ehara, K. Toyota, R. Fukuda, J. Hasegawa, M. Ishida, T. Nakajima, Y. Honda, O. Kitao, H. Nakai, T. Vreven, K. Throssell, J. A. Montgomery Jr, J. E. Peralta, F. Ogliaro, M. J. Bearpark, J. J. Heyd, E. N. Brothers, K. N. Kudin, V. N. Staroverov, T. A. Keith, R. Kobayashi, J. Normand, K. Raghavachari, A. P. Rendell, J. C. Burant, S. S. Iyengar, J. Tomasi, M. Cossi, J. M. Millam, M. Klene, C. Adamo, R. Cammi, J. W. Ochterski, R. L. Martin, K. Morokuma, O. Farkas, J. B. Foresman and D. J. Fox, Gaussian, Wallingford CT, 2016 Search PubMed.
- L. Grajciar, O. Bludský and P. Nachtigall, Water adsorption on coordinatively unsaturated sites in CuBTC MOF, J. Phys. Chem. Lett., 2010, 1, 3354–3359 CrossRef CAS.
- P. Chen, X. He, M. Pang, X. Dong, S. Zhao and W. Zhang, Iodine capture using Zr-Based metal–organic Frameworks (ZrMOFs): adsorption performance and mechanism, ACS Appl. Mater. Interfaces, 2020, 12, 20429–20439 CrossRef CAS PubMed.
- C. Adamo and V. Barone, Toward reliable density functional methods without adjustable parameters: the PBE0 model, J. Chem. Phys., 1999, 110, 6158–6170 CrossRef CAS.
- S. Grimme, S. Ehrlich and L. Goerigk, Effect of the damping function in dispersion corrected density functional theory, J. Comput. Chem., 2011, 32, 1456–1465 CrossRef CAS PubMed.
- D. Rappoport and F. Furche, Property-optimized Gaussian basis sets for molecular response calculations, J. Chem. Phys., 2010, 133, 134105 CrossRef PubMed.
- R. S. Mulliken, Electronic population analysis on LCAO-MO molecular wave functions, J. Chem. Phys., 1955, 23, 1833–1840 CrossRef CAS.
Footnotes |
† Electronic supplementary information (ESI) available: PXRD patterns, IR spectra, 1H NMR, HRESI-MS, UV-vis absorption spectra, etc. See DOI: https://doi.org/10.1039/d3ta04686a |
‡ These authors contributed equally to this work. |
|
This journal is © The Royal Society of Chemistry 2023 |