DOI:
10.1039/D3TA02642F
(Paper)
J. Mater. Chem. A, 2023,
11, 16127-16137
Ultralow loading of carbon quantum dots leading to significantly improved breakdown strength and energy density of P(VDF-TrFE-CTFE)†
Received
4th May 2023
, Accepted 28th June 2023
First published on 28th June 2023
Abstract
Relaxor ferroelectric polymer P(VDF-TrFE-CTFE) is attracting increasing attention for electronic applications due to its high dielectric constant and relaxation characteristic. However, the low breakdown strength and premature saturation of polarization of this polymer have hampered its practical application severely. In this work, carbon quantum dots (CQDs) with functional groups and size of about 5 nm have been incorporated to overcome these issues. The nanocomposite with 0.1 wt% CQDs presents significantly improved breakdown strength and discharge energy density. Its breakdown strength (Eb) has been increased by 30.7% up to 340 kV mm−1. Due to the Coulomb blockade effect, the electrons can be restrained in the deep traps, which is favorable for the improvement of breakdown strength. Besides, the abundant functional groups around CQDs are beneficial to form adequate hydrogen bonds, resulting in increased crystallinity and breakdown strength as well. Additionally, the discharge energy density has been improved by 41.7% up to 6.8 J cm−3. The study demonstrates the effective enhancement of breakdown strength and energy density by ultralow loading of CQDs for P(VDF-TrFE-CTFE) polymer.
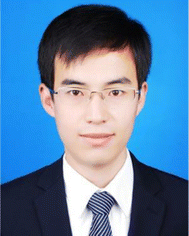 Hongshuai Hou | Dr Hongshuai Hou is a Professor of College of Chemistry and Chemical Engineering at Central South University. He received his master's degree from Tianjin University in 2013 and PhD degree from Central South University in 2016, and then worked at Central South University. His research focuses on the application of carbon dots in advanced energy materials, involving cathode/anode materials, electrolytes, and membrane materials for secondary batteries and capacitors. He has published more than 200 papers in international journals. |
Introduction
Capacitors with super-high power density and fast-speed charging–discharging processes have a broad application prospect, such as wind power, rail transportation, military equipment, and electrical energy storage.1–4 In the background of explosive development of the electronic information industry, breakthrough in key technology of capacitors is exigent for the application in new energy automobiles, intelligent terminals, and industry network.5–8 Dielectrics with high breakdown strength (Eb) and large discharge energy density (Ud) ought to be developed urgently.
For dielectrics with dipoles inside, energy charging–discharging can be realized through the polarization–depolarization process, which can be described by a displacement hysteresis loop (the D–E curve). Based on the D–E curve, the charging energy density or Ud of the dielectrics at a specific electric field can be calculated using the formula:
where U, E and D stand for the energy density, applied electric field, and displacement, respectively. ε0 is the permittivity of the vacuum (8.85 × 10−12), and εr is the relative permittivity (dielectric constant) of the dielectrics. Additionally, the electric field with the maximal displacement is called the dielectric breakdown strength (Eb).
In comparison with ceramic dielectrics, polymer dielectrics with greater flexibility and Eb have aroused widespread attention.9–12 For example, the Eb at room temperature of commercial BOPP can be as high as 700 kV mm−1.13,14 However, its Ud is limited due to the low dielectric constant (∼3). To overcome this problem, PVDF has been explored owing to its higher dielectric constant associated with the permanent dipoles. PVDF is a kind of crystallizable polymer and there are three main crystalline forms commonly, i.e. α, γ, and β phases.15,16 In the α phase, the dipoles orient randomly and show electric neutrality, which does not help to increase the dielectric constant; dipoles in the γ phase are oriented partially, resulting in a slight increase of polarity; the dipoles in the β phase orient in the same direction generating huge polarization.17–19 Yet, coupling among dipoles leads to larger energy loss because the energy barrier is higher for dipoles to switch back to the equilibrium state.20 It has been discovered that the introduction of CFE or CTFE monomer into the molecular chain of PVDF can enlarge the interchain spacing and weaken the interaction of the polar dipoles.21,22 Meanwhile, the P(VDF-TrFE-CTFE) ternary polymer possesses a high dielectric constant because of its strong polar bonds and the relaxation characteristic, attracting increasing attention in the energy storage field.23 Nevertheless, the weak Eb associated with the poor Young's modulus and the premature saturation of the polarization lead to the low Ud, limiting its application.3,24,25 Thus, it is a crucial task to enhance the Eb of the ternary polymer.
With regard to polymer dielectrics, the most threatening issue is the conductivity loss especially at high temperature and high electric field.24,26 The conductivity of polymers not only leads to huge dielectric loss but also produces a large amount of heat to increase the working temperature and accelerate the breakdown process. The charge carriers to form leakage current can originate from the intrinsic polymer and the charge injection.26 Furthermore, the electromechanical breakdown is the reason for the failure of the polymer dielectrics as well. This mechanism is related to the plastic deformation under electric fields. In summary, the main aim is to restrain the conductivity and reinforce the ternary polymer to reach higher Eb.
Efforts have been devoted to addressing this vital limitation. Liu et al. prepared P(VDF-TrFE-CTFE) film with Eb of 600 kV mm−1 and Ud of 17 J cm−3via grafting poly(styrene-methyl methacrylate) chains onto the polymer. Zhang et al. introduced hydrogen bonds into P(VDF-TrFE-CTFE) chains and increased the Young's modulus of the amorphous phase, resulting in an Eb of 500 kV mm−1.27 Zhu et al. constructed a sandwich structure with crosslinked PVDF clamped by P(VDF-TrFE-CTFE) on the outside, and an Eb of 550 kV mm−1 and a Ud of 18.3 J cm−3 were achieved.28 On the other hand, the modification towards polymer chains is effective but hard to conduct. Chi et al. prepared nanocomposite films with an Eb of 380 kV mm−1 and Ud of 12.93 J cm−3 by adding wide-bang-gap BNNs in gradient content.29 The addition of inorganic fillers into polymers is a feasible and facile method to improve the energy storage properties. However, filler loading needs to be high enough for greater εr, which will cause severe aggregation and higher local electric field, leading to more charge carriers and weaker Eb.30 Therefore, reasonable solutions need to be put forward to solve the problem.
Carbon quantum dots (CQDs) are a novel type of semiconductor quantum material, attracting a wide range of attention in fields like advanced batteries, biomedicine, photocatalysis and so on.31–34 CQDs possess a lot of special characteristics such as functional groups, quantum effect, photoluminescence, and great biocompactivity, where the Coulomb blockade effect is the behaviour that prevents electron tunneling.35,36 Once the particle size is reduced to a certain degree, an energy barrier can be formed owing to the strong Coulomb repulsion among electrons.37 Consequently, electrons can be suppressed to hop under a certain threshold. As such, CQDs with quantum-scale size can provide large interfaces and thick permeators to capture the space charges potentially.38,39 Besides, the electrons captured by traps are prone to be confined to hop due to the Coulomb blockade effect. Additionally, the abundant functional groups on its surface may avoid the aggregation issue especially when the filler content is relatively low.
In this work, a series of nanocomposite films with different CQD filler contents are prepared via a solution-casting method. It turns out that the Eb and the energy efficiency have been improved effectively with ultralow loading. With the addition of CQDs, the crystallinity increased due to its interface effect, which is favourable for lengthening the electric tree path and generating a depolarization electric field. The nanocomposite with a CQD content of 0.1 wt% presents better performance. The Eb has been enhanced by 30.7%, up to 340 kV mm−1 and the energy efficiency at 160 kV mm−1 is as high as 68.0%. On account of the enhancement of Eb, the maximal Ud has been improved by 41.7%, from 4.8 J cm−3 to 6.8 J cm−3 for the pristine film and the nanocomposite film at 0.1 wt% CQD loading, respectively.
Results and discussion
The high-resolution TEM images are shown in Fig. 1a and b, illustrating that the CQDs are monodisperse spherical particles with diameter near 5 nm and good crystal structure. Fig. 1c shows the great dispersity of the CQDs in ethanol solvent. The FTIR spectrum of CQDs is presented in Fig. 1d. As demonstrated, CQDs contain several types of functional groups, such as the carbon–carbon double bond (C
C), hydroxyl (–OH), and carbonyl (C
O) double bond.40,41 The XPS spectra depicted in Fig. 1e and f correspond well with the FTIR spectrum. These functional groups can be favourable for the surface functionalization of CQDs and beneficial for their unique properties.
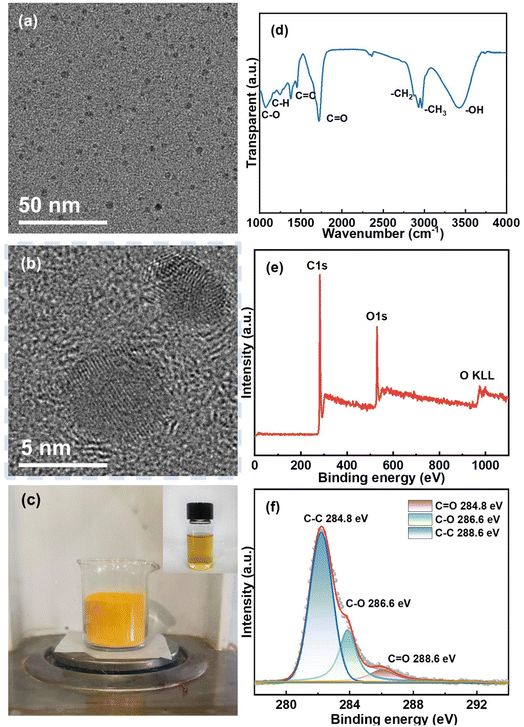 |
| Fig. 1 (a and b) High-res TEM images. (c) Digital images of CQDs and CQDs/ethanol solution. (d) FTIR spectrum. (e) Survey XPS spectrum. (f) C 1s high-resolution XPS spectra of CQDs. | |
The CQDs possess favourable dispersion in DMF solvent, as shown in Fig. 2a and S1 (ESI†). Since CQDs are a novel semiconductor quantum material with excellent photo-luminescence, LSCM is employed to confirm the dispersibility of CQDs in nanocomposite films. As illustrated in Fig. 2c–h, yellow dots scatter uniformly on each image. Besides, the yellow in different images becomes brighter with increasing the CQDs content. In other words, the CQDs are compatible with the homogeneous P(VDF-TrFE-CTFE) ternary polymer and well dispersed in the nanocomposite films with great stability. During the synthesis of CQDs, the aldol reaction process of acetone can occur under strong alkaline conditions as well as a series of side reactions. The products, unsaturated ketone, can further polymerize, forming oligomers with extended carbon chains whose components are functional groups (C–OH, CH2–/CH3, and C
O). Then the oligomers may curl and intertwine to produce several nanodots. Therefore, the CQDs possess a similar structure to the solvent and polymer matrix, accounting for their good compatibility.
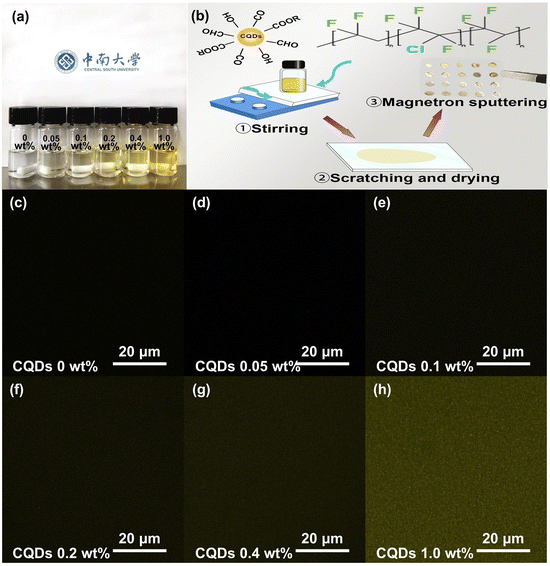 |
| Fig. 2 (a) Digital images of CQDs/P(VDF-TrFE-CTFE) solutions with different CQD content. (b) The scheme of the nanocomposite fabrication. (c–h) The fluorescence images of the nanocomposites recorded by LSCM. | |
Frequency dependence of dielectric permittivity under low electric field
The results of dielectric frequency spectroscopy are exhibited in Fig. 3a and b. There are five types of polarization in dielectrics, of which dipolar relaxation plays the most important role, ranging from 1 Hz to 100 MHz.42 The dielectric constant of all nanocomposites declines monotonously with increasing frequency, which is related to the weaker dielectric response of dipoles to higher frequency. The dielectric loss presents a peak at about 106 Hz with frequency, which is caused by the dipole relaxation.43 For a quantitative understanding of the influence on nanocomposites brought about by CQDs, εr and dielectric loss at 1 kHz of each nanocomposite are compared. As shown in Fig. 3c and d, the εr increases with addition of CQDs, and it changes from 29.4 to 34.3 at 0.1 wt% CQD loading. Since CQDs are a kind of spherical particle with a diameter of about 5 nm, a large specific area and interface can be generated. Space charges can accumulate in these interface and result in an increase of the permittivity.44–46 Besides, it is notable that the dielectric loss shows very little difference among all the CQDs/P(VDF-TrFE-CTFE) nanocomposites in Fig. 3b due to ultra-low filler loading.
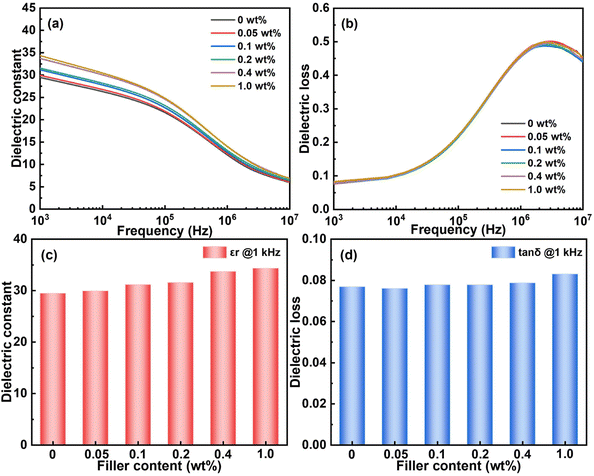 |
| Fig. 3 (a) Dielectric constant. (b) Dielectric loss as a function of frequency. (c) The dielectric constant. (d) Dielectric loss at 1 kHz of pristine ternary polymer thin film and CQDs/P(VDF-TrFE-CTFE) nanocomposites. | |
Weibull analysis of electric breakdown strength
The measured Eb is 260 kV mm−1, 300 kV mm−1, 340 kV mm−1, 320 kV mm−1, 280 kV mm−1 and 260 kV mm−1 for the pristine ternary polymer film and the CQDs/P(VDF-TrFE-CTFE) nanocomposite films with CQD content of 0.05 wt%, 0.1 wt%, 0.2 wt%, 0.4 wt% and 1.0 wt%, respectively. It is surprising to find that the Eb presents a 30.67% increase when CQDs, as low as 0.1 wt%, are incorporated into the ternary polymer film. This effective improvement is related to the Coulomb blockade effect and trap effect, which will be discussed later. The Weibull distribution is utilized to analyse the characteristic breakdown strength (EBD), under which the films have an 63.2% chance of failure. As illustrated in Fig. 4a and b, the EBD first increases from 265.03 kV mm−1 to 322.01 kV mm−1 then declines to 264.10 kV mm−1 with the addition of CQDs. Here, the characteristic Eb reaches the maximum at 0.1 wt% CQD loading.
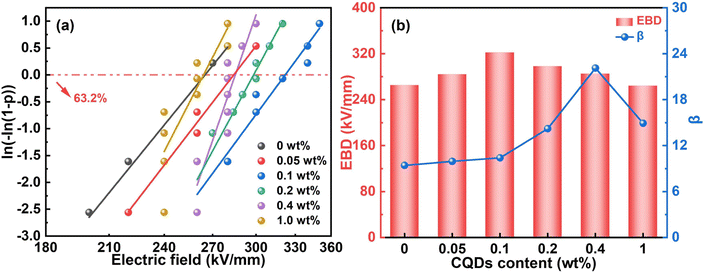 |
| Fig. 4 (a) The Weibull distribution. (b) The corresponding EBD and shape parameters of the samples with different CQD loadings. | |
Characterization of electric energy storage and energy efficiency
The Ud and energy efficiency are calculated through integrating the P–E loops, whose results are illustrated in Fig. 5. Among all the nanocomposite films, the one with CQD content of 0.1 wt% presents the highest Ud of 6.8 J cm−3, while that of the pristine ternary polymer film is only 4.8 J cm−3. Besides, this nanocomposite film possesses higher energy efficiency up to 68.0% under the electric field of 160 kV mm−1. That is, the Ud and the energy efficiency at 160 kV mm−1 are improved by 41.7% and 21.4%, respectively, for the CQD addition as low as 0.1 wt%. Additionally, the energy efficiency shows two turning points in Fig. 5b. The first is associated with the field-induced depolarization, meaning the dipoles can respond to the applied electric field.43 On the other hand, the ferroelectric domains can interact with each other and the coupling among them can become stronger when the electric field increases to a certain extent.46 As a result, it is difficult for dipoles to switch back to the equilibrium state, hampering the energy efficiency. In addition, the space charges accumulated on the interfaces can lead to severe conductivity loss.47 Notably, the Ud of the nanocomposite films are hardly distinguishable from each other, which will be discussed afterwards.
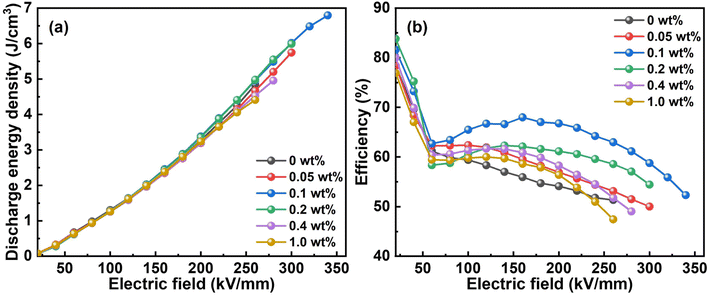 |
| Fig. 5 (a) The discharge energy density and (b) energy efficiency of the samples with different CQDs content. | |
Discussion
To understand the effect of CQDs on the breakdown behaviour of the nanocomposite films, DSC is utilized first. As shown in Fig. 6a, the melting point is around 132 °C, corresponding to that of the ternary polymer. It has been found that the crystallinity first reaches the apex at 0.1 wt% CQD loading then starts to decrease with increasing the filler content. It is speculated that the hydrogen bonds provided by abundant oxygenous functional groups on the CQDs surface are beneficial for polymer chains to align in order and for crystallinity to increase. Note that the sample with CQDs of 0.1 wt% also presents the maximal Eb. To our knowledge, a higher crystallinity is favourable for the improvement of the Eb due to the generated interfaces and extended tree-path brought about by more crystallites.44,48 On the one hand, polymer chains pack more densely and the free volume is declined in the crystallized area, resulting in a local higher breakdown strength. On the other hand, the electric stress develops along the interface between the crystallites and the polymer matrix when it hits the interface, which is favourable for diverging and weakening the electric stress and then for extending the growth of the electric tree-path. In addition, the crystallites can generate a depolarization electric field and reduce the local field, resulting in a decreased polarization to some extent and a higher electric field endurance.49 As a result, the sample with a higher crystallinity possesses better energy storage properties.
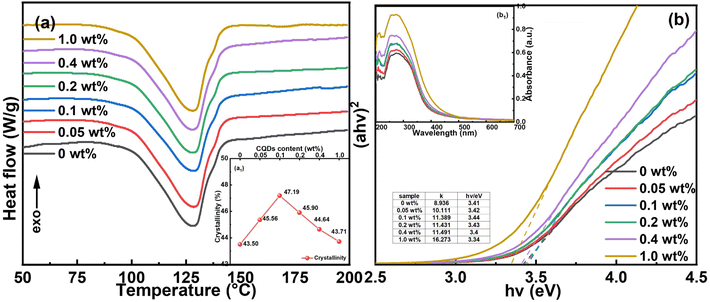 |
| Fig. 6 (a) The DSC curves. (b) The Tauc plot. (a1) The crystallinity. (b1) The UV-vis absorption spectra of the samples with different CQD content. | |
For another perspective, UV-vis absorption is employed. The band gap energy (Eg) can be estimated by using eqn (2). The UV-vis absorption spectra and the Tauc plot are shown in Fig. 6b1 and b, respectively. Notably, the A of the y axis of Fig. 6b is different from the one in eqn (2) due to mathematical analysis. By drawing the tangent line at the steepest position of the curve, the Eg can be found at the intersection of the tangent line with the horizontal axis. The Eg first increases to the peak value at 0.1 wt% CQD loading and then starts to decrease, though the variation is relatively small. Intriguingly, the changing trend here corresponds well with the enhancement of the Eb. By incorporating CQDs, impurity energy level and traps can be introduced, which is favourable for establishing the energy barrier and reducing the charge carriers. Besides, CQDs can help prevent carriers from hopping due to the Coulomb blockade effect by restricting carriers in the deep traps.37,50 According to Tanaka's multi-layer interface model for dielectric nanocomposites, there are bonded, bound, and loose layers, three types of interface layers, in the interface area, where the chain mobility increases successively. Deep traps can be formed in the bound layer at the interface, which are capable of capturing electrons and preventing them from moving.39 Therefore, the total concentration of charge carriers can be decreased with decreased leakage current density shown in Fig. S2a (ESI†). In this instance, the possibility for dielectric failure goes down accordingly. Moreover, it has been reported that the influence of the interface will become dominant when the filler size is small enough.51 Hence, the interface effect brought about by CQDs has played a significant role in improving the Eb of the nanocomposites. Nevertheless, the electron clouds will overlap and more space charges accumulate when the CQD content is over a certain value, leading to a nonuniform local electric field.52 Moreover, shallow traps in the loose layer at the interface can improve the charge carrier mobility because the electrons trapped by them are more prone to detrap.39,53,54 Under these conditions, the conductivity loss will emerge and the traps can no longer restrict free carriers. Therefore, the Eb of the nanocomposite film at 1.0 wt% CQD loading is reduced to values close to the initial values.
To further understand the energy storage behaviour of the nanocomposite films, the polarization curves are studied. As illustrated in Fig. 7a, the pristine ternary polymer film reaches the high maximal polarization (Pmax) quickly due to its premature saturation while a higher remanent polarization (Pr) is retained. Summarizing the Pmax and Pr with electric field increment of 20 kV mm−1, the differences become clearer in Fig. 7b. It is apparent that the slope belonging to the sample with CQD content of 0.1 wt% is the lowest, which means a valid delay for polarization saturation. According to the P–E curve, a larger integrated area and Ud can be obtained with a delayed polarization. The reason for this phenomenon lies in the O–H⋯F hydrogen bonds formed at the interfaces due to the strong polymer/filler interaction.20,47 Because of the hydrogen bonds, the energy barrier for dipoles to respond to the electric field becomes higher, which also explains the inferior polarization and less remanent polarization.27,55 On the other hand, the depolarization electric field generated by crystallites also plays a vital role in diminishing the local field and postponing the polarization saturation. Usually, the larger the integrated area in the P–E curve, the higher the energy density. However, there is seldom any difference among those samples as mentioned before. The Ud depends on the discharge curve which is associated with both Pmax and Pr. Take the sample at 1.0 wt% CQD loading for instance: the Pmax is higher than that of the sample at 0.1 wt% CQD loading while the Pr is higher as well, leading to a very small variation in the calculated Ud. On the other hand, the total energy density of the sample with CQD content of 1.0 wt% is higher absolutely because it is mainly depending on the Pmax. For this instance, the efficiency of the sample with CQD content of 0.1 wt% is much more satisfactory without a doubt.
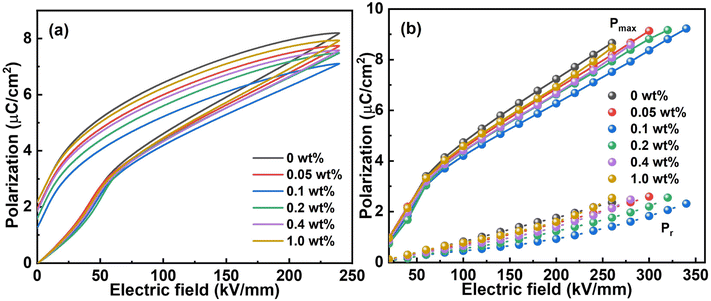 |
| Fig. 7 (a) The P–E loops at 240 kV mm−1 and (b) the maximum polarization and remanent polarization at different electric fields of the samples with different CQD content. | |
For dielectric capacitors, the charge–discharge property is one of the most important indexes to assess whether they can be put into practical applications or not. The Wd and the overdamped current versus time curves of the sample with CQD content of 0.1 wt% are shown in Fig. 8a and b. The energy is released rapidly as well as stably and the Wd is up to 5.5 J cm−3 at the electric field of 260 kV mm−1. Meanwhile, the discharge current increases gradually with higher electric field. To verify the stability of the nanocomposite films, they are divided into 6 regions factitiously where several points are measured to calculate the average energy density. The results are summarized in Fig. 8c. The 0.1 wt% CQD nanocomposite presents a better cycling stability than the pristine polymer as shown in Fig. S2b (ESI†). Through the comprehensive consideration of the dielectric properties of all the nanocomposite films, the sample at 0.1 wt% CQD loading is thought to be the best performing nanocomposite film with ultralow addition of CQD filler. The comparison between the pristine polymer film and the greatest one is summed up in Fig. 8d. The property comparisons with similar material systems are provided in Table S1 (ESI†).
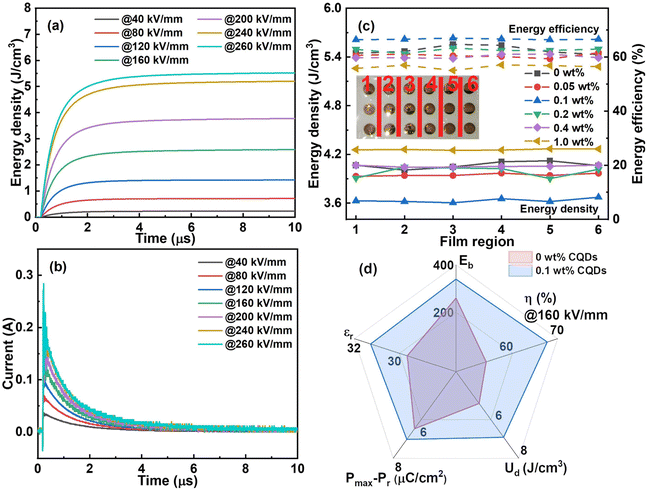 |
| Fig. 8 (a) The Wd as a function of time and (b) pulsed overdamped discharge behaviour of the sample with CQD content of 0.1 wt% at various electric fields. (c) The film stability at 160 kV mm−1 of the samples with different CQD content and (d) the comparison between the pristine polymer film and the sample with CQD content of 0.1 wt%. | |
Conclusions
P(VDF-TrFE-CTFE) added ultra-low CQD filler content nanocomposite films are prepared via solution-casting. The best performing one among all the samples is the nanocomposite film with 0.1 wt% CQD loading. The dielectric properties have been improved effectively, especially the breakdown strength. The dielectric constant increases gradually with the addition of CQDs, reaching 34.6 with the CQD content of 1.0 wt%. The Eb has been enhanced 30.7%, as high as 340 kV mm−1. The origin is that depolarization electric field can be generated in the crystallites of the nanocomposites, weakening the local field. Furthermore, CQDs hamper carriers from hopping via the Coulomb blockade effect. Consequently, the maximal Ud is up to 6.8 J cm−3 which presents a 41.7% increase compared to the pristine ternary polymer film.
It is obvious that the ultralow amount of filler addition facilitates the improvement of the dielectric properties, however, there is still a limitation for practical application. More improvement methods suitable for the P(VDF-TrFE-CTFE) polymer should be conducted in the future.
Experimental
Raw materials
All NaOH, 40% acetaldehyde and HCl are purchased from Sinopharm Chemical Reagent Beijing Co., Ltd. Poly(vinylidenefluoride-trifluoroethylene-chlorotrifluoroethylene) (P(VDF-TrFE-CTFE)) powder is purchased from Polyk Technologies, China; N,N-dimethylformamide (DMF, ≥99.5%) is purchased from Sinopharm, China; CQDs are synthesized by a simple chemical method mentioned in previous work.40,56
The synthesis of CQDs
In brief, 60 g NaOH was dissolved in a moderate amount of water and then the solution was added to 200 mL acetaldehyde aqueous solution (40%), followed by stirring vigorously for 2 h and being placed at ambient air, temperature, and pressure. Then the product is separated by centrifugation and washed with 1 M HCl and deionized water successively, followed by drying in a vacuum oven for 12 h.
The preparation of CQDs/P(VDF-TrFE-CTFE) nanocomposite films
CQDs powder with various mass fractions is dispersed in DMF solvent, followed by a 30 minute sonication to obtain a homogeneous light-yellow solution. P(VDF-TrFE-CTFE) powder is dissolved in the above solution under magnetic stirring at 55 °C for more than 18 h to obtain a clear solution. The final solution is solution-cast in a glass substrate and dried at 70 °C for 48 h to prepare thin nanocomposite films. The thickness of those films is about 12 μm. Finally, the films are coated golden electrodes via magnetron sputtering for dielectric properties testing.
Characterization and instrumentation
The transmission electron microscope (TEM, Titan G2 60-300), SEM (TESCAN MIRA3 LMH), laser scanning confocal microscopy (LSCM, Leica TCS SP8, Germany, λ = 538 nm), and XPS (Kratos AXIS SUPRA+) are employed to characterize the morphology and microstructure of the CQDs and nanocomposite films. DSC (DSC-25, America, heating rate of 10 °C min−1 under a nitrogen atmosphere) is utilized to calculate the crystallinity of the nanocomposites with the formula below:57 | 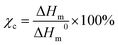 | (1) |
where χc is the crystallinity, ΔHm is the melting enthalpy od the nanocomposites and the ΔHm0 is the melting enthalpy of the polymer with 100% of crystallinity (42.0 J g−1 for the P(VDF-TrFE-CTFE)).55
UV-vis absorption (Shimadzu UV-3600i Plus) is employed to analyse the direct Eg of the nanocomposite films by the Tauc plot method. The Eg can be calculated as follows:58
where
α,
h, and
ν represent the light absorption index, Planck constant and the frequency, respectively; and
A is a constant while
Eg is defined before.
An Agilent 4294A LCR meter with frequency ranging from 1 kHz to 10 MHz and TF analyser 3000 ferroelectric polarization tester (aixACT, Germany) were utilized to test the dielectric properties and measure the P–E loops of the nanocomposites, respectively. Afterwards, the discharged energy density and efficiency were calculated. The characteristic Eb of the nanocomposites is analysed via the Weibull distribution:59
where
P,
E,
Eb, and
β represent the cumulative possibility of electric failure, breakdown strength at a 63.2% possibility of cumulative failure, EBD and the shape parameter assessing the scatter degree of data, respectively.
The discharge energy density (Wd) is an important performance index to directly estimate the practicability of dielectric capacitors. The charge–discharge curves are measured through a simple R–C circuit. And the Wd is calculated from the formula below:60
| 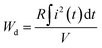 | (4) |
where
R and
V represent the inner resistance (10
4 Ω) and the sample volume, respectively.
Author contributions
D. Zhang and H. Luo conceived and supervised the project. G. Zou, X. Ji and H. Hou provided the CQDs. X. Jiang carried out the nanocomposite material preparations and data analysis. H. Xie and Y. Liu: helped in the material characterization. F. Wang and X. Li: conducted the electrical properties testing. All the authors participated in the discussion and preparation of the manuscript.
Conflicts of interest
There are no conflicts to declare.
Acknowledgements
The authors acknowledge the support from the National Natural Science Foundation of China (52172265 and 52002404), Scientific research project of Hunan Provincial Department of Education (21B0009), Excellent Youth Science Foundation of Hunan Province (2022JJ20067), The Science and Technology Innovation Program of Hunan Province (2022RC1074), Central South University Innovation-Driven Research Program (2023CXQD010) and State Key Laboratory of Powder Metallurgy, Central South University, Changsha, China.
References
- Z. Ran, B. Du, M. Xiao, H. Liu and J. Xing, Dielectric property improvement of polypropylene films doped with POSS for HVDC polymer film capacitors, IEEE Trans. Dielectr. Electr. Insul., 2021, 28(4), 1308–1316 CAS.
- G. Wang, Z. Lu, Y. Li, L. Li, H. Ji, A. Feteira, D. Zhou, D. Wang, S. Zhang and I. M. Reaney, Electroceramics for high-energy density capacitors: current status and future perspectives, Chem. Rev., 2021, 121(10), 6124–6172 CrossRef CAS PubMed.
- M. Singh, I. E. Apata, S. Samant, W. Wu, B. V. Tawade, N. Pradhan, D. Raghavan and A. Karim, Nanoscale strategies to enhance the energy storage capacity of polymeric dielectric capacitors: review of recent advances, Polym. Rev., 2021, 62(2), 211–260 CrossRef.
- J. H. Bae and S. H. Chang, PVDF-based ferroelectric polymers and dielectric elastomers for sensor and actuator applications: a review, Funct. Compos. Struct., 2022, 1, 012003 CrossRef.
- X. Wu, X. Chen, Q. Zhang and D. Q. Tan, Advanced dielectric polymers for energy storage, Energy Storage Mater., 2022, 44, 29–47 CrossRef.
- Q. Feng, J. Ping, J. Zhu, J. Pei, L. Huang, D. Zhang, Y. Zhao, S. Zhong and Z. Dang, All-organic dielectrics with high breakdown strength and energy storage density for high-power capacitors, Macromol. Rapid Commun., 2021, 42(12), e2100116 CrossRef PubMed.
- Y. Zhuang, S. Han, W. Liu, X. Wei and Z. Xu, An interdigital electrode type sensor based on P(VDF-TrFE) nanofibers, J. Alloys Compd., 2020, 831, 154657 CrossRef CAS.
- Y. Lu, Y. Jiang, Z. Lou, R. Shi, D. Chen and G. Shen, Wearable supercapacitor self-charged by P(VDF-TrFE) piezoelectric separator, Prog. Nat. Sci.: Mater. Int., 2020, 30(2), 174–179 CrossRef CAS.
- J. Qiu, Q. Gu, Y. Sha, Y. Huang, M. Zhang and Z. Luo, Preparation and application of dielectric polymers with high permittivity and low energy loss: a mini review, J. Appl. Polym. Sci., 2022, 139(24), 52367 CrossRef CAS.
- H. Chen, Z. Pan, W. Wang, Y. Chen, S. Xing, Y. Cheng, X. Ding, J. Liu, J. Zhai and J. Yu, Ultrahigh discharge efficiency and improved energy density in polymer-based nanocomposite for high-temperature capacitors application, Compos. Part A: Appl. Sci. Manuf., 2021, 142, 106266 CrossRef CAS.
- J. S. Ho and S. G. Greenbaum, Polymer capacitor dielectrics for high temperature applications, ACS Appl. Mater. Interfaces, 2018, 10(35), 29189–29218 CrossRef CAS PubMed.
- T. Zhang, X. Chen, Y. Thakur, B. Lu, Q. Zhang, J. Runt and Q. Zhang, A highly scalable dielectric metamaterial with superior capacitor performance over a broad temperature, Sci. Adv., 2020, 6(4), eaax6622 CrossRef CAS PubMed.
- Y. Zhu, P. Jiang and X. Huang, Poly(vinylidene fluoride) terpolymer and poly(methyl methacrylate) composite films with superior energy storage performance for electrostatic capacitor application, Compos. Sci. Technol., 2019, 179, 115–124 CrossRef CAS.
- J. Lu, B. Zhu, X. Zhang and X. Wang, Dielectric strength structure-activity relationship of BOPP film for high energy density pulse capacitor, IEEE Trans. Plasma Sci., 2019, 47(9), 4342–4349 CAS.
- L. Lu, W. Ding, J. Liu and B. Yang, Flexible PVDF based piezoelectric nanogenerators, Nano Energy, 2020, 78, 105251 CrossRef CAS.
- A. Salimi and A. Yousefi, FTIR studies of β-phase crystal formation in stretched PVDF films, Polym. Test., 2003, 22(6), 699–704 CrossRef CAS.
- Y. j. Cho, J. Jeong, M. Choi, G. Baek, S. Park, H. Choi, S. Ahn, S. Cha, T. Kim and D. Kang, BaTiO3@ PVDF-TrFE nanocomposites with efficient orientation prepared via phase separation nano-coating method for piezoelectric performance improvement and application to 3D-PENG, Chem. Eng. J., 2022, 427, 131030 CrossRef CAS.
- S. Barrau, A. Ferri, A. Da Costa, J. Defebvin, S. Leroy, R. Desfeux and J.-M. Lefebvre, Nanoscale investigations of α-and γ-crystal phases in PVDF-based nanocomposites, ACS Appl. Mater. Interfaces, 2018, 10(15), 13092–13099 CrossRef CAS PubMed.
- L. Jin, S. Ma, W. Deng, C. Yan, T. Yang, X. Chu, G. Tian, D. Xiong, J. Lu and W. Yang, Polarization-free high-crystallization β-PVDF piezoelectric nanogenerator toward self-powered 3D acceleration sensor, Nano Energy, 2018, 50, 632–638 CrossRef CAS.
- C. Zhao, Y. Hong, X. Chu, Y. Dong, Z. Hu, X. Sun and S. Yan, Enhanced ferroelectric properties of P(VDF-TrFE) thin film on single layer graphene simply adjusted by crystallization condition, Mater. Today Energy, 2021, 20, 100678 CrossRef CAS.
- M. R. Gadinski, Q. Li, G. Zhang, X. Zhang and Q. Wang, Understanding of relaxor ferroelectric behavior of poly(vinylidene fluoride–trifluoroethylene–chlorotrifluoroethylene)terpolymers, Macromolecules, 2015, 48(8), 2731–2739 CrossRef CAS.
- F. Bargain, D. Thuau, G. Hadziioannou, F. Domingues Dos Santos and S. Tencé-Girault, Phase diagram of poly(VDF-ter-TrFE-ter-CTFE)copolymers: relationship between crystalline structure and material properties, Polymer, 2021, 213, 123203 CrossRef CAS.
- Y. Huang, T. Gu, G. Rui, P. a. Shi, W. Fu, L. Chen, X. Liu, J. Zeng, B. Kang, Z. Yan, F. J. Stadler, L. Zhu, F. Kang and Y. He, A relaxor ferroelectric polymer with an ultrahigh dielectric constant largely promotes the dissociation of lithium salts to achieve high ionic conductivity, Energy Environ. Sci., 2021, 14(11), 6021–6029 RSC.
- Q. Feng, S. Zhong, J. Pei, Y. Zhao, D. Zhang, D. Liu, Y. Zhang and Z. Dang, Recent progress and future prospects on all-organic polymer dielectrics for energy storage capacitors, Chem. Rev., 2022, 122(3), 3820–3878 CrossRef CAS PubMed.
- B. Chu, X. Zhou, K. Ren, B. Neese, M. Lin, Q. Wang, F. Bauer and Q. M. Zhang, A dielectric polymer with high electric energy density and fast discharge speed, Science, 2006, 313(5785), 334–336 CrossRef CAS PubMed.
- J. Dong, X. Deng, Y. Niu, Z. Pan and H. Wang, Research progress of polymer based dielectrics for high-temperature capacitor energy storage, Acta Phys. Sin., 2020, 69(21), 217701 CrossRef.
- M. Zhang, S. Tan, J. Xiong, C. Chen, Y. Zhang, X. Wei and Z. Zhang, Tailoring dielectric and energy storage performance of PVDF-based relaxor ferroelectrics with hydrogen bonds, ACS Appl. Energy Mater., 2021, 4(8), 8454–8464 CrossRef CAS.
- X. Li, Y. Yang, Y. Wang, S. Pang, J. Shi, X. Ma and K. Zhu, Enhanced energy storage density of all-organic fluoropolymer composite dielectric via introducing crosslinked structure, RSC Adv., 2021, 11(25), 15177–15183 RSC.
- Y. Shang, Y. Feng, C. Li, C. Zhang, T. Zhang, Y. Zhang, Y. Zhang, C. Song and Q. Chi, Energy storage properties of P(VDF-TrFE-CTFE)-based composite dielectrics with uniform and gradient-doped boron nitride nanosheets, IET Nanodielectr., 2021, 5(1), 50–61 CrossRef.
- L. Li, J. Cheng, Y. Cheng, T. Han, Y. Liu, Y. Zhou, G. Zhao, Y. Zhao, C. Xiong, L. Dong and Q. Wang, Significant improvements in dielectric constant and energy density of ferroelectric polymer nanocomposites enabled by ultralow contents of nanofillers, Adv. Mater., 2021, 33(35), e2102392 CrossRef.
- R. Guo, L. Li, B. Wang, Y. Xiang, G. Zou, Y. Zhu, H. Hou and X. Ji, Functionalized carbon dots for advanced batteries, Energy Storage Mater., 2021, 37, 8–39 CrossRef.
- T. C. Wareing, P. Gentile and A. N. Phan, Biomass-based carbon dots: current development and future perspectives, ACS Nano, 2021, 15(10), 15471–15501 CrossRef CAS PubMed.
- B. Domingo-Tafalla, E. Martinez-Ferrero, F. Franco and E. Palomares-Gil, Applications of carbon dots for the photocatalytic and electrocatalytic reduction of CO2, Molecules, 2022, 27(3), 1081 CrossRef CAS.
- M. Ji, Y. Liu, J. Di, R. Chen, Z. Chen, J. Xia and H. Li, N-CQDs accelerating surface charge transfer of Bi4O5I2 hollow nanotubes with broad spectrum photocatalytic activity, Appl. Catal., B, 2018, 237, 1033–1043 CrossRef CAS.
- V. A. e. Burdov, The quantum control of electron states in a double quantum dot under coulomb blocking conditions, J. Exp. Theor. Phys., 2002, 76(1), 21–25 CAS.
- A. Donarini, G. Begemann and M. Grifoni, Interference effects in the Coulomb blockade regime: current blocking and spin preparation in symmetric nanojunctions, Phys. Rev. B: Condens. Matter Mater. Phys., 2010, 82(12), 125451 CrossRef.
- L. F. Schelp, A. Fert, F. Fettar, P. Holody, S. F. Lee, J. L. Maurice, F. Petroff and A. Vaures, Spin-dependent tunneling with Coulomb blockade, Phys. Rev. B: Condens. Matter Mater. Phys., 1997, 56(10), R5747–R5750 CrossRef CAS.
- T. Tanaka, M. Kozako, N. Fuse and Y. Ohki, Proposal of a multi-core model for polymer nanocomposite dielectrics, IEEE Trans. Dielectr. Electr. Insul., 2005, 12(4), 669–681 CrossRef CAS.
- Y. Zhou, J. Hu, B. Dang and J. He, Titanium oxide nanoparticle increases shallow traps to suppress space charge accumulation in polypropylene dielectrics, RSC Adv., 2016, 6(54), 48720–48727 RSC.
- H. Hou, L. Shao, Y. Zhang, G. Zou, J. Chen and X. Ji, Large-area carbon nanosheets doped with phosphorus: a high-performance anode material for sodium-ion batteries, Adv. Sci., 2017, 4(1), 1600243 CrossRef.
- P. Ge, H. Hou, X. Cao, S. j. Li, G. Zhao, T. Guo, C. Wang and X. Ji, Multidimensional evolution of carbon structures underpinned by temperature-induced intermediate of chloride for sodium-ion batteries, Adv. Sci., 2018, 5(6), 1800080 CrossRef.
- L. Zhu, Exploring strategies for high dielectric constant and low loss polymer dielectrics, J. Phys. Chem. Lett., 2014, 5(21), 3677–3687 CrossRef CAS.
- M. Yuan, B. Li, S. Zhang, R. Rajagopalan and M. T. Lanagan, High-field dielectric properties of oriented poly(vinylidene fluoride-co-hexafluoropropylene): structure–dielectric property relationship and implications for energy storage applications, ACS Appl. Polym. Mater., 2020, 2(3), 1356–1368 CrossRef CAS.
- Y. Guo, N. Meng, Y. Zhang, J. Xu, E. Pawlikowska, C. y. Wu, M. Szafran and F. Gao, Characterization and performance of plate-like Ba0.6Sr0.4TiO3/poly(vinylidene fluoride-trifluoroethylene-chlorotrifluoroethylene)
composites with high permittivity and low loss, Polymer, 2020, 203, 122777 CrossRef CAS.
- J. Wang, S. Liu, C. Chen, H. Hao and J. Zhai, Interface modification and energy storage properties of barium titanate-based/polyvinylidene fluoride composite, Acta Phys. Sin., 2020, 69(21), 217702 CrossRef.
- N. Meng, X. Zhu, R. Mao, M. J. Reece and E. Bilotti, Nanoscale interfacial electroactivity in PVDF/PVDF-TrFE blended films with enhanced dielectric and ferroelectric properties, J. Mater. Chem. C, 2017, 5(13), 3296–3305 RSC.
- Y. Feng, Q. Deng, C. Peng, J. Hu, Y. Li, Q. Wu and Z. Xu, An ultrahigh discharged energy density achieved in an inhomogeneous PVDF dielectric composite filled with 2D MXene nanosheets via interface engineering, J. Mater. Chem. C, 2018, 6(48), 13283–13292 RSC.
- T. Soulestin, V. Ladmiral, F. D. Dos Santos and B. Améduri, Vinylidene fluoride- and trifluoroethylene-containing fluorinated electroactive copolymers. How does chemistry impact properties?, Prog. Polym. Sci., 2017, 72, 16–60 CrossRef CAS.
- F. Guan, J. Wang, L. Yang, J.-K. Tseng, K. Han, Q. Wang and L. Zhu, Confinement-induced high-field antiferroelectric-like behavior in a poly(vinylidene fluoride-co-trifluoroethylene-co-chlorotrifluoroethylene)-graft-polystyrene graft copolymer, Macromolecules, 2011, 44(7), 2190–2199 CrossRef CAS.
- S. Li, J. Dong, Y. Niu, L. Li, F. Wang, R. Hu, J. Cheng, L. Sun, Z. Pan, X. Xu and H. Wang, Enhanced high-temperature energy storage properties of polymer composites by interlayered metal nanodots, J. Mater. Chem. A, 2022, 10(36), 18773–18781 RSC.
- T. J. Lewis, Interfaces: nanometric dielectrics, J. Phys. D, 2005, 38(2), 202–212 CrossRef CAS.
- Y. Thakur, T. Zhang, C. Iacob, T. Yang, J. Bernholc, L. Chen, J. Runt and Q. Zhang, Enhancement of the dielectric response in polymer nanocomposites with low dielectric constant fillers, Nanoscale, 2017, 9(31), 10992–10997 RSC.
- R. Qiao, C. Wang, S. Chen, G. He, Z. Liu, H. Luo and D. Zhang, High-temperature dielectric polymers with high breakdown strength and energy density via constructing the electron traps in blends, Compos. Part A: Appl. Sci. Manuf., 2022, 152, 106679 CrossRef CAS.
- S. Wang, Z. Luo, J. Liang, J. Hu, N. Jiang, J. He and Q. Li, Polymer nanocomposite dielectrics: understanding the matrix/particle interface, ACS Nano, 2022, 16(9), 13612–13656 CrossRef CAS.
- R. Wang, H. Xu, S. Cheng, J. Liang, B. Gou, J. Zhou, J. Fu, C. Xie, J. He and Q. Li, Ultrahigh-energy-density dielectric materials from ferroelectric polymer/glucose all-organic composites with a cross-linking network of hydrogen bonds, Energy Storage Mater., 2022, 49, 339–347 CrossRef.
- W. Hong, Y. Zhang, L. Yang, Y. Tian, P. Ge, J. Hu, W. Wei, G. Zou, H. Hou and X. Ji, Carbon quantum dot micelles tailored hollow carbon anode for fast potassium and sodium storage, Nano Energy, 2019, 65, 104038 CrossRef CAS.
- T. Soulestin, P. Marcelino Dos Santos Filho, V. Ladmiral, C. Totée, G. Silly, T. Lannuzel, F. Domingues Dos Santos and B. Ameduri, Influence of trans-1,3,3,3-tetrafluoropropene on the structure–properties relationship of VDF- and TrFE-based terpolymers, Macromolecules, 2017, 50(2), 503–514 CrossRef CAS.
- S. Mandal, A. C. Reber, M. c. Qian, P. S. Weiss, S. N. Khanna and A. Sen, Controlling the band gap energy of cluster-assembled materials, Acc. Chem. Res., 2013, 46(11), 2385–2395 CrossRef CAS.
- L. Sun, Z. Shi, B. He, H. Wang, S. Liu, M. Huang, J. Shi, D. Dastan and H. Wang, Asymmetric trilayer all-polymer dielectric composites with simultaneous high efficiency and high energy density: a novel design targeting advanced energy storage capacitors, Adv. Funct. Mater., 2021, 31(35), 2100280 CrossRef CAS.
- X. Zhao, C. Li, J. k. Liu, Y. Ding, W. Bai, P. Zheng, P. Li, J. Zhang and J. Zhai, (Bi0.5Na0.5)TiO3-based relaxor ferroelectrics with simultaneous high energy storage properties and remarkable charge-discharge performances under low working electric fields for dielectric capacitor applications, Ceram. Int., 2021, 47(18), 25800–25809 CrossRef CAS.
|
This journal is © The Royal Society of Chemistry 2023 |