DOI:
10.1039/D3TA02142D
(Paper)
J. Mater. Chem. A, 2023,
11, 14052-14057
Engineering earth-abundant copper(I) sensitizing centers in metal–organic frameworks for efficient photosynthesis†
Received
10th April 2023
, Accepted 30th May 2023
First published on 30th May 2023
Abstract
Rational construction of earth-abundant heterogeneous photocatalysts is of great importance for efficient and sustainable solar energy utilization. Herein, we have developed a series of earth-abundant MOF photocatalysts (Cu-1-MOF–Cu-5-MOF) by incorporating Cu(I) complexes with different steric functional groups, which represents an efficient strategy for regulating the sensitizing ability of MOFs by restricting the excited state configuration of Cu(I) sensitizing centers. Remarkably, Cu-5-MOF with strong steric functional groups can efficiently drive photocatalytic oxidative coupling of benzylamine with a yield of 90.2%, 11 times higher than that with Cu-1-MOF without steric functional groups (8.2%). Systematic investigations revealed that the introduction of strong steric functional groups (e.g. sec-butyl) into Cu based MOFs can enhance their visible-light absorption, photochemical stability and electron–hole separation efficiency, which contributed to facilitating the utilization of solar energy and interface electron/energy transfer for efficient photosynthesis. This work provides a new insight into rationally constructing earth-abundant and efficient MOF photocatalysts by engineering copper(I) sensitizing centers with steric functional groups at the molecular level.
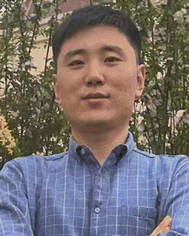 Song Guo | Dr Song Guo earned his PhD in Chemical Engineering and Technology in 2016 at the Dalian University of Technology (China). During the 2014–2015 year, he visited the University of Florida (USA) as a Joint PhD student. He is currently a Professor at the Institute for New Energy Materials and Low Carbon Technologies, Tianjin University of Technology. His current research interests focus on the development of artificial photosynthetic photosensitizers for solar energy conversion, including photochemical CO2 reduction, water splitting and organic synthesis. |
1 Introduction
Solar energy can be stored in carbohydrates via natural photosynthesis, which represents the largest scale matter and energy conversion process on the Earth. In this process, the magnesium porphyrin complex, as the active ingredient of chlorophyll in green leaves, is responsible for light absorption and electron transfer, largely determining the photosynthetic efficiency.1 Therefore, the development of artificial photosensitizers (PSs) by mimicking the structure and function of chlorophyll represents a promising strategy to construct efficient photosynthetic systems. In this field, transition-metal-based photoactive complexes have been employed as light-harvesting PSs for solar energy conversion.2–4 In particular, Ru- and Ir polypyridyl complexes can achieve long-lived metal-to-ligand charge transfer (MLCT) excited states, which have been widely applied to various photocatalytic reaction scenarios, such as organic synthesis,5,6 H2 evolution,7–9 and CO2 reduction.10–12 However, these metal complex PSs in homogeneous systems were usually limited by their poor photochemical stability and excited state self-quenching and were difficult to recycle. Given that metal–organic frameworks (MOFs) as a class of structurally defined porous solid materials can provide an ideal molecular platform to stabilize solution-inaccessible metal active sites and rationally control the distance between metal sites,13–19 typical [Ru(bpy)3]2+ and [Ir(ppy)2(bpy)]+ have been doped into MOFs for heterogeneous photosynthesis.20 Highly tunable MOFs can also hierarchically integrate Ru-/Ir complex PSs and catalysts to facilitate intra-framework energy and electron and mass transfers.21–25 Sorts of catalysts, such as single metal sites and nanoclusters, have been embedded into Ru-/Ir complex sensitized MOFs for synergistic catalysis.26–32 Despite significant progress, it's highly desirable but remains a great challenge to replace noble metal complexes with earth-abundant photoactive metal complexes in these crystalline MOF materials for efficient and sustainable photosynthesis.
Pioneered by the work of McMillin, Cu(I)–bis-phenanthrolines ([Cu(NN)2]+) with Cu(I) MLCT excited states have emerged as a most promising class of non-noble metal complexes for solar energy conversion.33–35 In the ground state, the bis(diimine) Cu(I) structural motif with d10 configuration presented a pseudotetrahedral (D2d) molecular geometry.36 Upon light excitation, the resulting Cu(II) coordination center has a d9 configuration via MLCT, which can trigger a Jahn–Teller (J–T) distortion owing to the unequal occupation of electrons in the degenerate 3d molecular orbitals.37,38 After J–T distortion, a flattened conformation formed for the Cu(II) complex with a D2 symmetry, which contributes to fully exposing the Cu(II) center to solvents and makes the Cu(II) center susceptible to nucleophilic attack from the donor solvents. Therefore, [Cu(NN)2]+ complexes usually presented a short-lived excited state and poor photochemical stability in Lewis basic solvents due to the formation of Cu(II)-solvent exciplexes. In the past half a century, attempts have been made to introduce steric functional groups in the 2- and 9- positions of the phenanthroline (Phen) ligands in [Cu(NN)2]+ for restricting their J–T distortion and improving their excited state properties.39–42 However, to the best of our knowledge, the influence of excited state configuration on the sensitizing ability of Cu(I) complexes has never been explored in crystalline solid materials for heterogeneous photosynthesis.
In this work, for the first time, we have constructed a series of earth-abundant MOF photocatalysts via incorporation of Cu(I) complexes (Cu-1-MOF–Cu-5-MOF). The sensitizing ability of Cu-MOFs can be improved by systemically tuning the steric functional groups in the 2- and 9- positions of Phen. The catalytic activities for photo-oxidative coupling of benzylamine with these Cu(I)-based MOFs were in the order of Cu-5-MOF > Cu-4-MOF > Cu-3-MOF > Cu-2-MOF > Cu-1-MOF, well consistent with that of the steric resistance of their steric functional groups (Fig. 1). Remarkably, the conversion rates for benzylamine oxidative coupling can reach over 90.2% with Cu-5-MOF as the photocatalyst, representing the highest yield among all the Cu-MOFs. Systematic investigations reveal that the introduction of steric functional groups in Phen can improve the visible-light absorption, photochemical stability and electron–hole separation efficiency of photoactive MOFs, which contributed to facilitating the utilization of solar energy and interface electron/energy transfer for boosting photosynthesis. This work opened up a new avenue to develop efficient and earth-abundant MOF photocatalysts by restricting the excited state configuration of Cu(I) complexes.
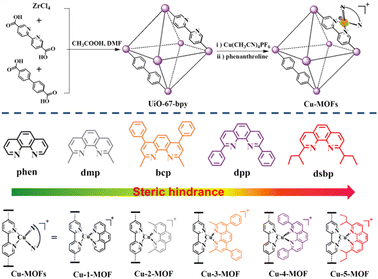 |
| Fig. 1 Schematic view of the synthetic process (up) and the structures (down) of Cu-MOFs. | |
2 Experimental
2.1. Photocatalytic measurements
2.1.1 Aerobic oxidation coupling of benzylamine.
5.0 mg photocatalyst was added into the mixed solvent of 0.75 mL CH3CN and 0.75 mL CH2Cl2 in a glass vial for ultrasonic dispersion. 0.02 mmol benzylamine was added into the above mixture, which was stirred in air upon irradiation with a 300 W Xe lamp (100 mW cm−2, λ > 400 nm). The reaction process was monitored by TLC analysis. After the reaction is complete, the solvent is concentrated to dryness under reduced pressure. The conversion rate of crude products was determined by 1H NMR. For the recycling experiments, the photocatalyst was collected by centrifugation and washed with CH2Cl2. After drying in a vacuum oven at 50 °C for 3 h, the sample powder was used for the next round of photocatalysis.
2.2. Synthesis of MOFs
2.2.1 Synthesis of UiO-67-bpy.
ZrCl4 (10.0 mg, 0.042 mmol), 4,4′-biphenyldicarboxylic acid (H2bdc, 6.0 mg, 0.025 mmol), 2,2′-bipyridine-5,5′-dicarboxylic acid (H2bpydc, 4.0 mg, 0.016 mmol) and glacial acetic acid (83 μL) were mixed in 1.5 mL DMF. After being sealed in a vial, the mixture was placed in an oven. The temperature was kept at 100 °C for 24 h. After cooling down to room temperature, the resulting precipitate was collected by centrifugation, washed with DMF/CH3OH and dried under vacuum at 100 °C to afford 12.5 mg UiO-67-bpy with a yield of ∼84.2%.
2.2.2 Synthesis of Cu-MOFs.
UiO-67-bpy (50.0 mg) was dispersed in 10 mL CH3CN and transferred into a dried 50 mL three-neck round-bottom flask. Cu(CH3CN)4PF6 (93.2 mg, 0.25 mmol) in 10 mL CH2Cl2 was added to the above flask and the mixture was stirred for 24 h under argon. After the reaction was complete, the solid was collected by centrifugation and washed with CH2Cl2 several times to remove excess Cu salt. The resulting solid and phenanthroline ligands (0.10 mmol) were added into the mixed solvent of 2 mL CH2Cl2 and 1 mL CH3CN, which was stirred for 24 h. After the reaction was complete, the product was collected by centrifugation, washing with CH2Cl2 and drying under vacuum at 50 °C to afford the Cu-MOFs.
3 Results and discussion
3.1. Synthesis and characterization
A series of Cu(I)-based MOF photocatalysts (Cu-MOFs) were successfully synthesized through a modular step-by-step assembly strategy (Fig. 1). Using UiO-67-bpy as a molecular platform, Cu(CH3CN)4PF6 was first coordinated to the diimide sites of the bpy ligands in MOFs, and then Phen with different steric functional groups could be coordinated to the Cu(I) sites to afford the Cu(I) complex sensitized MOFs (Cu-1-MOF–Cu-5-MOF) (Fig. S1†). Cu(I)-based MOFs were fully characterized using the powder X-ray diffraction pattern (PXRD), X-ray photoelectron spectroscopy (XPS), a scanning electron microscope (SEM) and a transmission electron microscope (TEM) (Fig. S2–S5†). As shown in Fig. S2,† the characteristic peaks of PXRD for UiO-67-bpy were well consistent with that of the simulated PXRD pattern of UiO-67, indicating the correct structure and good phase purity of UiO-67-bpy. The PXRD patterns of Cu-MOFs were similar to those of UiO-67-bpy, confirming the well maintained structure and crystallinity before and after incorporating Cu(I) complexes. As shown in Fig. S4,† the two main peaks at 932.2 and 952.0 eV correspond to Cu 2p1/2 and Cu 2p3/2, respectively, which could be attributed to Cu(I).
SEM and TEM analyses revealed that Cu-5-MOF exhibited a regular uniform octahedral morphology with a size of ca. 400 nm (Fig. S5†).
Besides, elemental mapping images showed the even distribution of Zr, Cu, C, N and O elements in the Cu-5-MOF skeleton, further supporting that Cu(I) complexes were successfully introduced into UiO-67-bpy (Fig. 2). Inductively coupled plasma mass spectrometry (ICP-MS) analysis manifested that these different Cu-MOFs exhibited a similar Cu content (Table S1†), precluding the influence of Cu content differences in different MOFs on their catalytic performance.
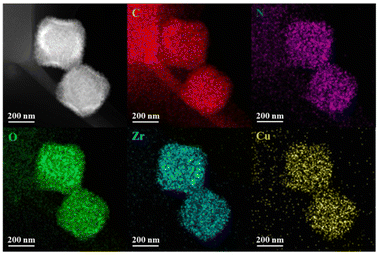 |
| Fig. 2 High angle annular dark field scanning transmission electron microscopy and elemental mapping images of Cu-5-MOF. | |
3.2. Photophysical and electrochemical characterization
Visible light harvesting ability and photoelectrochemical properties of Cu-MOFs were systematically investigated using steady/transient spectra and photocurrent/electro-chemical impedance spectroscopy (Fig. 3). As shown in Fig. 3a, the absorption of UiO-67-bpy was mainly limited in the ultraviolet region, which was harmful to the utilization of solar energy. After the introduction of Cu(I) complexes, the absorption of UiO-MOFs can be expanded to the visible light region. Cu-5-MOF exhibited a visible light absorption peak at around 450 nm, similar to that of Cu-2-MOF. This indicated that 2,9-di(sec-butyl)Phen and 2,9-dimethylPhen modified Cu-based MOFs possessed a similar electronic configuration in the ground state. The absorption of Cu-3-MOF extended to 490 nm as compared with that of Cu-2-MOF, which could be attributed to an extended conjugated system of ligands due to the introduction of phenyl at the 4 and 7-positions of Phen. Besides, Cu-4-MOF presented a much broader visible absorption than other Cu-MOFs, well consistent with the absorbing feature of the reported PS [Cu(dpp)2]+ (dpp = 2,9-diphenylphenanthroline).43 Both the emission and photoluminescence lifetimes of UiO-67-bpy significantly quenched after introducing Cu(I) complexes (Fig. 3b and S6, S7†). This could be ascribed to the transformation from the singlet state of UiO-67-bpy into the triplet state of the Cu(I) complex.42,44 The photocurrent intensity was in the order of Cu-5-MOF > Cu-4-MOF > Cu-3-MOF > Cu-2-MOF > Cu-1-MOF, well consistent with that of the steric resistance of their steric functional groups (Fig. 3c). This result revealed that Cu(I) complexes with large steric functional groups contributed to facilitating photogenerated electron–hole separation of photoactive MOFs. Notably, Cu-5-MOF exhibited the most efficient electron–hole separation among Cu-MOFs due to the restricted J–T distortion of sec-butyl steric functional groups to reduce the excited state loss via non-radiation transition.40 This viewpoint was further supported by their electrochemical impedance spectra (EIS), where their EIS radii were in the order of Cu-5-MOF < Cu-4-MOF < Cu-3-MOF < Cu-2-MOF < Cu-1-MOF (Fig. 3d). Furthermore, the energy level diagram of the highest occupied molecular orbital (HOMO) and lowest unoccupied molecular orbital (LUMO) for UiO-67-bpy and Cu-MOFs can be estimated by Mott–Schottky (MS) measurements and Tauc plot analysis (Fig. S8 and S9†). The calculated LUMO level of Cu-MOFs was determined to be −0.60 V − −0.85 V, more negative than the reduction potential from O2 to superoxide radicals (E(O2/O2˙−) = −0.33 V vs. NHE) (Fig. S10†), indicating that the electron transfer from the LUMO of photoactive Cu-MOFs to O2 was thermodynamically feasible. As a result, Cu-5-MOF with large steric functional groups exhibited favorable visible absorption and efficient electron–hole separation, highlighting its great potential for solar energy conversion.
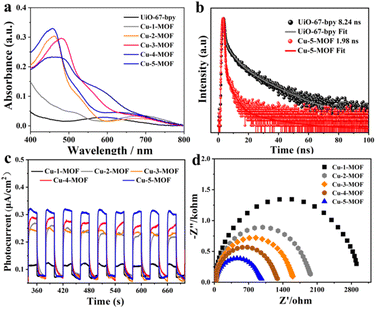 |
| Fig. 3 (a) UV-vis absorption spectra of UiO-67-bpy and Cu-MOFs, (b) Photoluminescence lifetimes of UiO-67-bpy and Cu-5-MOF, and (c) and (d) photocurrent response and Nyquist plots of the electrochemical impedance spectra (EIS) of Cu-MOFs under 300 W Xe lamp irradiation. | |
3.3. Photocatalytic oxidation reactions
Imines, as active intermediates, played an important role in the synthesis of dyes, drugs and agricultural chemicals. Photocatalytic oxidative coupling of amines represented a mild, clean and efficient approach for the synthesis of imines.45 Here we examined the sensitizing ability of Cu-MOFs by photo-oxidative coupling of benzylamines. As shown in Fig. 4a, upon irradiation with visible light, the yield of product 2a (N-benzylidenebenzylamine) was in the order of Cu-5-MOF > Cu-4-MOF > Cu-3-MOF > Cu-2-MOF > Cu-1-MOF > UiO-67-bpy (Table S2†). Remarkably, the yield of 2a with the Cu-5-MOF photocatalyst can reach 90.2% within 1 hour, 11 times higher than that with Cu-1-MOF (8.2%). The poor catalytic performance of Cu-1-MOF could be mainly ascribed to its excitation energy loss by J–T distortion due to no steric functional groups in Phen ligands. There was almost no product formed in the absence of oxygen, visible light or photocatalyst, indicating that these factors were essential for efficient photosynthesis (Fig. S11†).
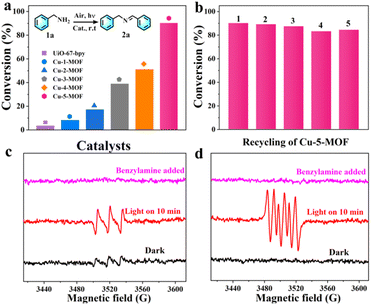 |
| Fig. 4 (a) Catalytic activities of UiO-67-bpy and Cu-MOFs for aerobic photocatalytic oxidative coupling of benzylamine. (b) Recycling experiments with Cu-5-MOF. (c) and (d) ESR spectra of Cu-5-MOF in the presence of 4-oxo-TMP in CH3CN and DMPO in DMSO. | |
Remarkably, there was no significant loss of catalytic activity for Cu-5-MOF after five consecutive recycles (Fig. 4b), indicating its excellent catalytic stability. This viewpoint was further supported by the results of PXRD and SEM, where the structure, crystallinity and octahedral configuration of Cu-5-MOF were well maintained after the photocatalytic reaction (Fig. S12 and S13†). As compared with Cu-5-MOF, Cu-1-MOF and Cu-3-MOF presented a poor photochemical stability (Fig. S14†). In addition, benzylamine derivatives with different substituents could be effectively transformed into imines with Cu-5-MOF photocatalysts under visible light. As shown in Table S3,† the conversion of all the substrates can reach over 74% within 1.5 h, confirming a broad substrate tolerance for the Cu-5-MOF photocatalyst.
In order to unveil the superior catalytic performance of Cu-5-MOF, a series of control experiments were carried out and electron spin resonance (ESR) spectra were recorded to comprehensively study its catalytic mechanism (Fig. 4c, d and Table S4†). p-Benzoquinone (BQ), NaN3 and isopropanol (IPA) were used as O2˙−, 1O2 and ˙OH scavengers, respectively. The conversion of benzylamine significantly decreased upon the addition of BQ and NaN3, which preliminarily confirmed that both 1O2 and O2˙− were reactive oxygen species (ROS) for efficient photooxidation. After adding IPA, the catalytic yield was well maintained, excluding the possibility of ˙OH participating in the reaction. As shown in Table S4† the conversion rate of benzylamine decreased in the presence of hydroquinone (HQ), KI and AgNO3 as free radicals, holes and electron scavengers, respectively, further supporting that the catalytic process was mediated by the electron transfer pathway. To further discern ROS in the catalytic process, ESR experiments were performed with 4-oxo-TMP and 5, 5-dimethyl-1-pyrroline N-oxide (DMPO) as 1O2 and O2˙− trapping agents, respectively (Fig. 4c and d). Upon light irradiation, the characteristic signals of the complexes 1O2-4-oxo-TMP and O2˙−-DMPO were observed, which almost completely disappeared after the addition of benzylamine. This further verified that both 1O2 and O2˙− indeed participated in the photosynthetic reaction. As shown in Fig. S15,† a characteristic absorption band between 450–600 nm was observed for reaction solution in the presence of DPD (N,N-diethyl-p-phenylenediamine)/POD (horseradish peroxidase), confirming the production of H2O2 in the photocatalysis process of benzylamine oxidative coupling.
Based on the above systematic investigations, the catalytic mechanism for the photocatalytic aerobic coupling of benzylamine was proposed as follows (Fig. 5): upon visible light irradiation, the triplet state of Cu(I) complexes in MOFs was populated by a series of intramolecular photophysical processes, which can facilitate photogenerated electron–hole (e−–h+) separation of MOFs. Subsequently, the photogenerated electrons and holes can transfer to O2 and benzylamine to generate O2˙− and PhCH2NH2˙+, respectively. PhCH2NH2˙+ can react with O2˙− to generate PhCH
NH. In the meantime, the excitation energy of the Cu(I) complex can be transferred to O2via the Dexter mechanism to afford 1O2, which can react with benzylamine to generate PhCH
NH. Ultimately, PhCH
NH coupled with benzylamine to produce N-benzylidenebenzylamine. As a result, the catalytic performance of Cu-MOFs highly depended on their visible light harvesting ability, electron–hole separation ability and interface energy/electron efficiency.
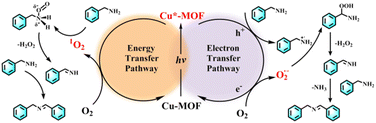 |
| Fig. 5 The proposed catalytic mechanism of the aerobic oxidative coupling of benzylamine with O2 using Cu-5-MOF as the photocatalyst. | |
2-Chloroethyl ethyl sulfide (CEES) as a chemical warfare agent simulant can be transformed into nontoxic 2-chloroethyl ethyl sulfoxide (CEESO) by the photochemical strategy.46–48 In order to verify the universality of the regulation strategy of Cu-MOF sensitizing ability by restricting their excited state configuration, these Cu-MOFs were applied to photo-oxidative degradation of CEES to produce CEESO. Remarkably, the catalytic yield of CEESO with Cu-5-MOF can reach 100% under visible light irradiation, significantly higher than that with Cu-1-MOF and Cu-2-MOF (Table S5†). This result revealed that Cu(I) complexes with strong steric functional groups in MOFs exhibited a much stronger sensitizing ability than that with weak or no steric functional groups in MOFs. This could be ascribed to that Cu(I) complexes with strong steric functional groups in MOFs can restrict its J–T distortion to reduce the excitation energy loss. Control experiments and ESR spectra confirmed that both 1O2 and O2˙− were ROS in the catalytic process (Table S6, Fig. S16 and S17†). As shown in Fig. S18,† the excited Cu-5-MOF sensitized O2 to produce 1O2 and O2˙− by the energy/electron transfer process, which can further oxidize the substrates to CEESO.
4 Conclusions
In summary, for the first time, we constructed a series of earth-abundant and efficient MOF photocatalysts by incorporating Cu(I) complexes with different steric functional groups. The influence of excited state configuration of Cu(I) complexes on MOF sensitizing ability was first explored by systemically varying steric functional groups. Remarkably, the conversion rates for benzylamine oxidative coupling can reach over 90.2% with Cu-5-MOF as the photocatalyst, 11 and 5.2 times higher than that with Cu-1-MOF (8.2%) and Cu-2-MOF (17.2%), respectively. In addition, the Cu-5-MOF photocatalyst can be recycled and reused for over 5 times without the obvious activity loss, representing a robust photochemical stability, much superior to that of Cu-1-MOF and Cu-3-MOF. Cu-5-MOF with large steric functional groups in Phen can increase torsional resistance and reduce the excitation loss of MOF photocatalysts, resulting in its advantages in strong visible light absorption, efficient electron–hole separation and good photochemical stability, which greatly contributed to boosting photosynthesis. This work opens a new horizon for the development of highly efficient and earth-abundant MOF photocatalysts by restricting the excited state configuration at the molecular level.
Author contributions
S. G. conceived and designed the project, G. C. G. and X. D. L. performed the experiments, G. C. G., L. H. M., X. D. L., S. G., T. B. L. and Z. M. Z. analyzed the data and wrote the article.
Conflicts of interest
There are no conflicts to declare.
Acknowledgements
This work was supported by the National Natural Science Foundation of China (No. 22171209 and 22071180).
References
- T. Mirkovic, E. E. Ostroumov, J. M. Anna, R. van Grondelle, Govindjee and G. D. Scholes, Chem. Rev., 2017, 117, 249–293 CrossRef CAS.
- D. N. Tritton, F.-K. Tang, G. B. Bodedla, F.-W. Lee, C.-S. Kwan, K. C.-F. Leung, X. Zhu and W.-Y. Wong, Coord. Chem. Rev., 2022, 459, 214390 CrossRef CAS.
- Y. J. Yuan, Z. T. Yu, D. Q. Chen and Z. G. Zou, Chem. Soc. Rev., 2017, 46, 603–631 RSC.
- J. Zhao, W. Wu, J. Sun and S. Guo, Chem. Soc. Rev., 2013, 42, 5323–5351 RSC.
- Q. Liu and L.-Z. Wu, Natl. Sci. Rev., 2017, 4, 359–380 CrossRef CAS.
- C. K. Prier, D. A. Rankic and D. W. MacMillan, Chem. Rev., 2013, 113, 5322–5363 CrossRef CAS PubMed.
- M. Wang, K. Han, S. Zhang and L. Sun, Coord. Chem. Rev., 2015, 287, 1–14 CrossRef CAS.
- P. Wang, S. Guo, H. J. Wang, K. K. Chen, N. Zhang, Z. M. Zhang and T. B. Lu, Nat. Commun., 2019, 10, 3155 CrossRef PubMed.
- S. Guo, K.-K. Chen, R. Dong, Z.-M. Zhang, J. Zhao and T.-B. Lu, ACS Catal., 2018, 8, 8659–8670 CrossRef CAS.
- Y. Kuramochi, O. Ishitani and H. Ishida, Coord. Chem. Rev., 2018, 373, 333–356 CrossRef CAS.
- Y.-H. Luo, L.-Z. Dong, J. Liu, S.-L. Li and Y.-Q. Lan, Coord. Chem. Rev., 2019, 390, 86–126 CrossRef CAS.
- Y. Hu, F. Zhan, Q. Wang, Y. Sun, C. Yu, X. Zhao, H. Wang, R. Long, G. Zhang, C. Gao, W. Zhang, J. Jiang, Y. Tao and Y. Xiong, J. Am. Chem. Soc., 2020, 142, 5618–5626 CrossRef CAS PubMed.
- K. Sun, Y. Qian and H. L. Jiang, Angew. Chem., Int. Ed., 2023, 62, e202217565 CrossRef CAS PubMed.
- B. Li, M. Chrzanowski, Y. Zhang and S. Ma, Coord. Chem. Rev., 2016, 307, 106–129 CrossRef CAS.
- C. D. Wu and M. Zhao, Adv. Mater., 2017, 29, 1605446 CrossRef.
- J. Liu, L. Chen, H. Cui, J. Zhang, L. Zhang and C. Y. Su, Chem. Soc. Rev., 2014, 43, 6011–6061 RSC.
- L. Zeng, X. Guo, C. He and C. Duan, ACS Catal., 2016, 6, 7935–7947 CrossRef CAS.
- A. Kirchon, L. Feng, H. F. Drake, E. A. Joseph and H. C. Zhou, Chem. Soc. Rev., 2018, 47, 8611–8638 RSC.
- Y. Bai, Y. Dou, L. H. Xie, W. Rutledge, J. R. Li and H. C. Zhou, Chem. Soc. Rev., 2016, 45, 2327–2367 RSC.
- C. Wang, Z. Xie, K. E. deKrafft and W. Lin, J. Am. Chem. Soc., 2011, 133, 13445–13454 CrossRef CAS PubMed.
- C.-C. Hou, T.-T. Li, S. Cao, Y. Chen and W.-F. Fu, J. Mater. Chem. A, 2015, 3, 10386–10394 RSC.
- G. Lan, Z. Li, S. S. Veroneau, Y. Y. Zhu, Z. Xu, C. Wang and W. Lin, J. Am. Chem. Soc., 2018, 140, 12369–12373 CrossRef CAS PubMed.
- P. M. Stanley, J. Haimerl, C. Thomas, A. Urstoeger, M. Schuster, N. B. Shustova, A. Casini, B. Rieger, J. Warnan and R. A. Fischer, Angew. Chem., Int. Ed., 2021, 60, 17854–17860 CrossRef CAS PubMed.
- P. M. Stanley, C. Thomas, E. Thyrhaug, A. Urstoeger, M. Schuster, J. Hauer, B. Rieger, J. Warnan and R. A. Fischer, ACS Catal., 2021, 11, 871–882 CrossRef CAS.
- D. Kim, D. R. Whang and S. Y. Park, J. Am. Chem. Soc., 2016, 138, 8698–8701 CrossRef CAS PubMed.
- Z.-H. Yan, B. Ma, S.-R. Li, J. Liu, R. Chen, M.-H. Du, S. Jin, G.-L. Zhuang, L.-S. Long, X.-J. Kong and L.-S. Zheng, Sci. Bull., 2019, 64, 976–985 CrossRef CAS PubMed.
- T. C. Zhuo, Y. Song, G. L. Zhuang, L. P. Chang, S. Yao, W. Zhang, Y. Wang, P. Wang, W. Lin, T. B. Lu and Z. M. Zhang, J. Am. Chem. Soc., 2021, 143, 6114–6122 CrossRef CAS PubMed.
- S. Guo, L. H. Kong, P. Wang, S. Yao, T. B. Lu and Z. M. Zhang, Angew. Chem., Int. Ed., 2022, 61, e202206193 CAS.
- C. Wang, K. E. deKrafft and W. Lin, J. Am. Chem. Soc., 2012, 134, 7211–7214 CrossRef CAS.
- W. Huang, X. Wang, W. Zhang, S. Zhang, Y. Tian, Z. Chen, W. Fang and J. Ma, Appl. Catal., B, 2020, 273, 119087 CrossRef CAS.
- W. Wang, X.-W. Song, Z. Hong, B. Li, Y. Si, C. Ji, K. Su, Y. Tan, Z. Ju, Y. Huang, C.-N. Chen and D. Yuan, Appl. Catal., B, 2019, 258, 117979 CrossRef CAS.
- W.-M. Liao, J.-H. Zhang, Z. Wang, S.-Y. Yin, M. Pan, H.-P. Wang and C.-Y. Su, J. Mater. Chem. A, 2018, 6, 11337–11345 RSC.
- R. M. Everly, R. Ziessel, J. Suffert and D. R. McMillin, Inorg. Chem., 1991, 30, 559–561 CrossRef CAS.
- M. Iwamura, S. Takeuchi and T. Tahara, Acc. Chem. Res., 2015, 48, 782–791 CrossRef CAS.
- D. G. Cuttell, S. M. Kuang, P. E. Fanwick, D. R. McMillin and R. A. Walton, J. Am. Chem. Soc., 2002, 124, 6–7 CrossRef CAS PubMed.
- N. A. Gothard, M. W. Mara, J. Huang, J. M. Szarko, B. Rolczynski, J. V. Lockard and L. X. Chen, J. Phys. Chem. A, 2012, 116, 1984–1992 CrossRef CAS PubMed.
- M. Iwamura, S. Takeuchi and T. Tahara, J. Am. Chem. Soc., 2007, 129, 5248–5256 CrossRef CAS PubMed.
- S. Garakyaraghi, E. O. Danilov, C. E. McCusker and F. N. Castellano, J. Phys. Chem. A, 2015, 119, 3181–3193 CrossRef CAS PubMed.
- M. Iwamura, S. Takeuchi and T. Tahara, Phys. Chem. Chem. Phys., 2014, 16, 4143–4154 RSC.
- C. T. Cunningham, K. L. Cunningham, J. F. Michalec and D. R. McMillin, Inorg. Chem., 1999, 38, 4388–4392 CrossRef CAS PubMed.
- M. C. Rosko, K. A. Wells, C. E. Hauke and F. N. Castellano, Inorg. Chem., 2021, 60, 8394–8403 CrossRef CAS PubMed.
- K. K. Chen, S. Guo, H. Liu, X. Li, Z. M. Zhang and T. B. Lu, Angew. Chem., Int. Ed., 2020, 59, 12951–12957 CrossRef CAS PubMed.
- M. Ruthkosky, F. N. Castellano and G. J. Meyer, Inorg. Chem., 1996, 35, 6406–6412 CrossRef CAS PubMed.
- M. W. Mara, D. N. Bowman, O. Buyukcakir, M. L. Shelby, K. Haldrup, J. Huang, M. R. Harpham, A. B. Stickrath, X. Zhang, J. F. Stoddart, A. Coskun, E. Jakubikova and L. X. Chen, J. Am. Chem. Soc., 2015, 137, 9670–9684 CrossRef CAS PubMed.
- C. Xu, H. Liu, D. Li, J. H. Su and H. L. Jiang, Chem. Sci., 2018, 9, 3152–3158 RSC.
- H. Wang, G. W. Wagner, A. X. Lu, D. L. Nguyen, J. H. Buchanan, P. M. McNutt and C. J. Karwacki, ACS Appl. Mater. Interfaces, 2018, 10, 18771–18777 CrossRef CAS PubMed.
- A. Atilgan, M. M. Cetin, J. Yu, Y. Beldjoudi, J. Liu, C. L. Stern, F. M. Cetin, T. Islamoglu, O. K. Farha, P. Deria, J. F. Stoddart and J. T. Hupp, J. Am. Chem. Soc., 2020, 142, 18554–18564 CrossRef CAS PubMed.
- H. Wang, S. Jiang, S. Chen, D. Li, X. Zhang, W. Shao, X. Sun, J. Xie, Z. Zhao, Q. Zhang, Y. Tian and Y. Xie, Adv. Mater., 2016, 28, 6940–6945 CrossRef CAS PubMed.
|
This journal is © The Royal Society of Chemistry 2023 |