DOI:
10.1039/D3SU00086A
(Critical Review)
RSC Sustain., 2023,
1, 1150-1167
A review on spent lithium-ion battery recycling: from collection to black mass recovery
Received
10th March 2023
, Accepted 28th June 2023
First published on 14th July 2023
Abstract
The advent of lithium-ion battery technology in portable electronic devices and electric vehicle applications results in the generation of millions of hazardous e-wastes that are detrimental to the ecosystem. A proper closed-loop recycling protocol reduces the environmental burden and strengthens a country with resource sustainability, circular economy, and the provision of raw materials. However, to date, only 3% of spent LIBs have been recycled. The recycling efficiency can be further increased upon strong policy incentives by the government and legislative pressure on the collection rate. This review sheds light on the pretreatment process of end-of-life batteries that includes storage, diagnosis, sorting, various cell discharge methods (e.g., liquid medium, cryogenic and thermal conditioning, and inert atmosphere processing), mechanical dismantling (crushing, sieving, sequential, and automated segregation), and black mass recovery (thermally and solvent leaching). The advantage of the stagewise physical separation and practical challenges are analyzed in detail. Disassembling the battery module pack at the cell level with the improved technology of processing spent batteries and implementing artificial intelligence-based automated segregation is worth it for high-grade material recovery for battery applications. Herein, we outline an industry-viable mechanochemical separation process of electrode materials in a profitable and ecofriendly way to mitigate the energy demand in the near future.
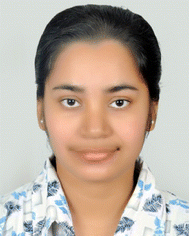 Madhushri Bhar | Madhushri Bhar received her B.Sc. degree in chemistry from the University of Burdwan in 2016 and then she received M.Sc. degree from Indian Institute of Technology Hyderabad (IITH) in 2018. Currently, she is a PhD research scholar at IITH under the supervision of Dr Surendra K. Martha. Her research is focused on the electrochemical analysis of recycled lithium-ion batteries as well as synthesis and applications of electrode materials in lithium and sodium-ion batteries. |
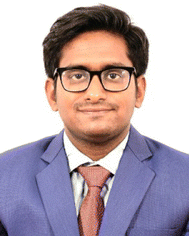 Shuvajit Ghosh | Shuvajit Ghosh received his Bachelor's degree from Presidency University, Kolkata, India in 2017 and Master's degree from Indian Institute of Technology (IIT) Hyderabad, India in 2019. Currently, he is a PhD candidate at IIT Hyderabad, India under the supervision of Dr Surendra K. Martha. His research focuses on anion storage electrochemistry and surface protection at high voltage. |
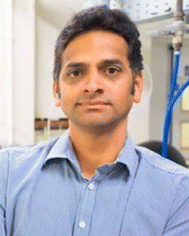 Satheesh Krishnamurthy | Professor Satheesh Krishnamurthy is a Fellow of Royal Society of Chemistry and Fellow of Institute of Materials, Minerals and Mining. He is currently the chair of energy technology at The Open University, Milton Keynes, UK. Prior to this position, he was a research fellow at Dublin City University and Trinity College Dublin. He did his PhD at Newcastle University, UK. He hails from Chennai, India and is a recipient of UK India research excellence award. His laboratory is involved in the research of advanced nanomaterials with specific application areas in alternative energy, multifunctional nanocomposites, wastewater treatment, and recycling of batteries. His research focuses on the materials science and engineering of several technologies that will impact our society in the future. |
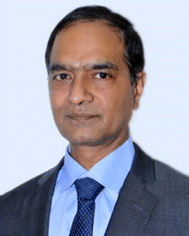 Y. Kaliprasad | Kaliprasad Yalamanchili currently serves as the CEO of NILE Limited, Hyderabad, India's leading recycler of lead–acid batteries. He is a mechanical engineering graduate with a qualification in general management and a qualified lead auditor for ISO management systems. He has over 34 years of industry experience in technical, project management, and business operations, widely in equipment building, heavy engineering, and chemical and metallurgical process industries. He has a core competency in design, product development, production operations, commercial operations, management systems, and general management. He has worked with Indian and multinational manufacturing companies as CTO and COO. |
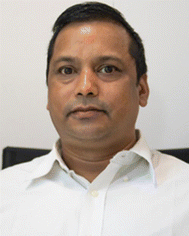 Surendra K. Martha | Surendra Kumar Martha is currently working as Associate Professor and Head of the Department of Chemistry, IIT Hyderabad. He graduated in the year 2006 from the Solid State and Structural Chemistry Unit, Indian Institute of Science, Bangalore, India, under the supervision of Professor Ashok K. Shukla. Previously, Dr Martha worked as a Postdoctoral Research Associate at the Oak Ridge National Laboratory, Tennessee, USA, and Bar-Ilan University, Israel. His recent research interests are in materials electrochemistry with particular emphasis on advanced metal-ion, dual carbon, lead–acid, Ni–Zn batteries, supercapacitors, and recycling Li-ion batteries. He has over 110 publications in peer-reviewed Material Science and Electrochemistry Journals and over 15 patents, 10 book chapters, and contributed to over 80 academic conferences. |
Sustainability spotlight
The massive use of lithium-ion batteries (LIBs) in commercial appliances has raised significant concern over the enormous e-waste caused by these end-of-life LIBs in both economic and environmental prospects. This article outlines e-waste logistics, collection, storage and various pre-treatment procedures to recover black mass from spent LIBs with a low level of contamination. The pre-treatment stage describes an eco-friendly and sustainable industry-viable mechanochemical separation process of cell components such as different cell discharge methods, mechanical dismantling by cursing, deep screening based on particle size fraction and sequential segregation. We emphasize all recovery stages with challenges and propose a feasible route to high-grade material recovery with a high recovery rate that might be a win–win situation for both environment and the manufacturer.
|
Introduction
Lithium-ion batteries (LIBs) have become the leading technology and multibillion-dollar market because of a plethora of applications in the automobile industry, portable electronics sector, power tools, and upcoming usage in powertrains, maritime markets, etc.1,2 The implementations of governmental policies on electromobility and the mitigation of the ever-growing energy demand have resulted in expeditious LIB manufacturing. As a result, experts forecast that the global market value of LIBs in commercial appliances will reach $139.36 billion in 2026.3 However, the rapid progress in battery research over the last three decades, incentivized by the huge demand, has downscaled the battery price from 57% of the total EV cost in 2015 to 33% in 2019 and is forecasted to drop to about 20% by 2025 for a medium-sized car.4 Nevertheless, the production cost of the cathode active material still makes up to 15–20% of total battery fabrication cost5,6 and, unfortunately, is expected to increase for high cobalt-containing compositions due to geopolitical conflicts centered on cobalt mining.7,8 Besides, the massive usage of LIBs might raise a significant concern over the enormous e-waste caused by these end-of-life retired LIBs in both economic and environmental prospects. The poisonous electrolyte and metal constituents from the end-of-life LIBs can penetrate groundwater, or burning batteries generate harmful gases such as COx that are detrimental to the ecosystem. Therefore, the closed-loop recycling of spent LIB components is paramount for waste management sustainability, the minimization of the supply chain risk of the raw materials among the countries, and price fluctuations with higher economic returns.9–13
A typical lithium-ion cell consists of lithium transition metal (Mn, Ni, Co, V, Fe) oxide/phosphate (LiFePO4)-based cathode and graphite as an anode. Each electrode comprises conductive metal foil current collectors, i.e., Al and Cu, for the cathode and anode. The electrolyte is Li-salt in carbonate-based solvent or polymer electrolytes, and a porous polyolefin is used as a separator. The commercial cells are cylindrical, prismatic, or pouch-type in a sealed container made of plastic, steel, or refined Al. The cells are connected in series or parallel as a module subunit of a battery system. This review explores the pretreatment processes, such as the collection of retired LIBs, sorting, various cell discharge methods (e.g., liquid medium, cryogenic and thermal conditioning, inert atmosphere processing), mechanical dismantling (crushing, sieving, sequential and automated segregation), and black mass recovery (thermally, solvent leaching). A detailed study from the retired cell collection to black mass recovery in a stepwise manner and the practical challenges are elucidated here. Besides, a perspective based on industry-viable battery module recycling is also prescribed in this article.
Waste logistics
E-waste material collection is a significant step of any recycling protocol. Lead–acid battery (LAB) recycling is one of the oldest running circular economies, the efficiency of which heavily relies on waste collection legislation. European countries tax new batteries, and the revenue is invested in the recycling infrastructure. On the other hand, in some states of the USA, customers must deposit cash to be refunded on returning spent batteries.14 Therefore, proper collection procedures and strict no casual discard resulted in a more than 95% LAB recycling rate in Europe, nearly 100% in the USA and about 80% in other developing countries.15,16 LIBs, the latest addition to the recycling economy, are embedded with more polluting, scarce, hazardous, and costlier metals than LAB. However, the overall recycling rate of LIBs is not up to the mark to date on a global scale and is impeded by a poor collection rate. This rate varies drastically country-wise, such as less than 5% in the EU, around 70% in China and South Korea, 2–3% in Australia, 0.1% in South Africa, and yet to be initiated in many developing countries.17–19 Hence, stringent regulations, widespread awareness, and safety guidelines are required to vitalize an effective battery collection mechanism. A cooperative network (Fig. 1) among the government, battery manufacturers, distributors, retailers, consumers, local collectors, transporters, and recycling industries will undoubtedly push the initiative.
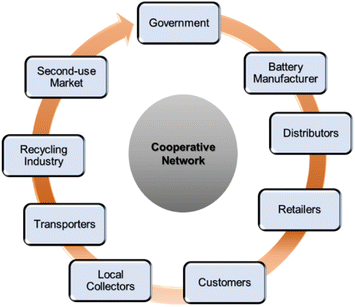 |
| Fig. 1 The cooperative network of LIB collection. | |
Collection, transport, and storage
LIBs have been classified as a 'highly combustible hazard' in all directives on waste batteries and are advised to be handled with utmost care. Small sparkles originating from any exposed section of the battery pack may be fuelled up by flammable electrolytes to cause self-ignition. Therefore, extreme safety measures have to be followed throughout the entire recycling web to cease short-circuit and electrolyte leakage. Japan Portable Rechargeable Battery Recycling Centre (JBRC) released a scheme on waste LIB logistics in 2014. According to it, all the uncovered conductive portions, such as connecting wires, electrode terminals, metal parts, and damaged electrode scraps must be insulated by tape at first. Then, the whole battery set should be fastened together to make a uniformly shielded bundle before shipment. Afterward, all properly taped battery sets are to be arranged inside heat-resistive robust containers with a clear warning sign on them and transported carefully to recycling plants to avoid excessive vibration.20
Waste LIB packs pose a severe threat even in nonfunctional conditions. Therefore, the storage precautions are necessary to follow. Large packs are safer to store in spacious chambers by keeping fair distances between one another and away from fire sources until further treatments. Storage buildings should be equipped with fire suppression systems, smoke detectors, climate-controlling accessories, mechanical ventilators, grated fiberglass flooring, and an adequate number of doors for entry and exit during emergency cases to check fire mishaps.21 External conditions such as abrupt temperature changes, mechanical impact, and higher humidity may disturb the safe ambience. Long-term storage beyond the recommended state of charge (SOC) that may induce irreversible side reactions must be avoided to secure a second service life for potential batteries. Fig. 2 represents the stepwise black mass recovery of the electrode materials from LIB e-waste.
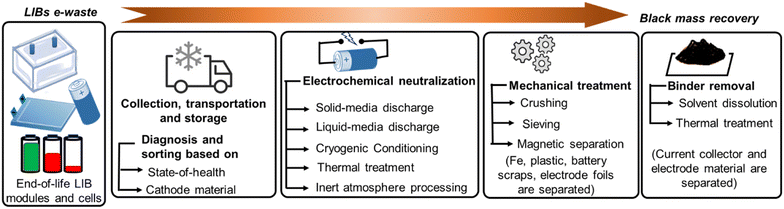 |
| Fig. 2 Process flowchart of electrode materials containing black mass recovery from LIBs e-waste. | |
Diagnosis and sorting
Many established battery recycling industries do not maintain a presorted route for spent LIB collection and storage. A large number of retired battery packs received from EV manufacturers, local collectors, or directly from customers are often amassed unsystematically, irrespective of active material composition, electrochemical characteristics, module geometry, and technical specifications.19 The management of waste batteries is more tedious for the industries newly adopting LIBs in their recycling ecosystem besides existing technologies such as NiMH, NiCd, primary lithium, and alkaline batteries.22 On the flip side, LIBs, on ending up in the wrong recycling stream, bring forth a huge risk. News of explosions has been reported on the accidental intrusion of LIBs in lead–acid battery recycling input streams. Prevention of such contamination through manual screening in recycling plants is impossible because the number of spent batteries delivered to recyclers may reach up to 70
000 per day.23 Hence, sorting LIBs by local collectors based on the battery type may conceivably be a safer practice for a properly functioning recycling chain.
Need for SoH-based sorting
The volume of retired EV battery packs is far exceeding the capacity of the steadily evolving second use-market of LIBs at the current status quo. However, an exponential upturn in market growth is expected in the not so distant future.24 Hence, a triage based on state-of-health (SoH) diagnosis is performed to detect and differentiate all exhausted and malfunctioning modules of an EV battery pack.25,26 These modules are subjected to subsequent steps of recycling. The remaining prospective modules of the battery pack are refurbished according to the demands of the second-use market and finally included in the recycling loop after the completion of the second service life. Again, manually screening faulty modules or cells at this level is painstaking. Automated setups can solve the problem. Advancements in automatic sorting based on electrical conditions of end-of-life batteries have already been made.27,28 A literature report where the measurement of computed cell health is performed on account of X-ray radiographic scanning and digital image contrast computation reveals the function with an appreciable speed of 5–6 cells per second and with 79% accuracy.29 The method is applicable for imaging a single 18
650-type cell from different angles at a time, but detecting a damaged cell from a module or drawing conclusions on an entire module basis is challenging. This necessitates module dismantling, which is a drawback. Therefore, artificial intelligence-driven complex algorithms are needed to realize automated sorting industrially.
Need for cathode material-based sorting
To ensure cell longevity and better public safety at lower cost, EV companies are showing more interest in low cobalt chemistries, such as a mixture of NMC-811 with LMO or LNMO as the price of NMC-111 (20.36 wt% Co) underwent 63.56% inflation between January 2017 and March 2018. In contrast, the increase is only 27.72% for NMC-811 (6.05 wt% Co). However, the cost scenario is altered after 2020, when the price inflation of raw nickel increased the price of nickel-rich cathode active materials. The ∼20 USD per kg of both NMC811 and NMC532 in January 2021 reached ∼60 and ∼48 USD per kg, respectively, in January 2022.30 Therefore, the bottom-line is that the increasing prices of Ni and Co are increasing the cost of layered oxide cathodes. Conversely, LFP is a trendy choice for electric buses in China as the price fluctuation of LFP is much more stable. It is intended to be used in the first all-electric motorhome: the Iridium E Mobil.31–33 Thus, LFP and NMC are expected to capture 65% of the total LIB cathode market by 2025, and their recycling may become a large-scale business.34Fig. 3 represents a schematic diagram of the diagnosis and sorting of spent LIBs. Nevertheless, sequestering all elements (Ni, Co, and Mn) from NMC in the purest form is hard to control because of chemical similarities among 3d transition metals originating from their juxtaposition in the periodic table. There is no unified recycling protocol for all types of cathode materials (NMC, LMO, NCA, LCO, and LFP) that exists today. The accepted routes and applied treatments for recovery vary from one cell chemistry to another. Therefore, contamination may severely threaten reusability if not sorted beforehand based on the cathode active material as battery-grade raw materials should be more than 99% pure. Similar sorting on the ground of active anode materials is not so substantial because the superiority of graphite has let only 2% market penetration for LTO and other chemistries.
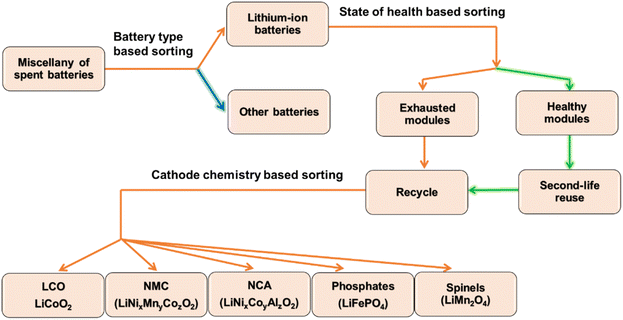 |
| Fig. 3 A schematic diagram for diagnosis and sorting of spent LIBs. | |
Electrochemical neutralization
A cathode is the principal source of lithium-ion in LIBs. Electrical energy is applied to extract Li+ from stable cathode materials during charging. It is solvated by the electrolyte, moves through the pores of the separator, and sits inside layers of graphite at the anode. Thus, the applied electrical energy is converted into chemical energy and stored within both electrodes. The charged state is a chemically destabilized condition of the LIBs. The preserved chemical energy is released in electrical form during discharge. Battery Management System (BMS) is designed to resist users from discharging a battery below a specific voltage, i.e., cut-off voltage, to avoid irreversible damage. Therefore, batteries decommissioned even at cut-off voltage, especially those from EVs, are not energy neutral. There always remains some amount of energy. Mechanical or electrical abuse at this stage may trigger an internal short-circuit that will consume the contained energy to generate heat quickly and consequently lead to an explosion if heat is not dissipated properly.35 Therefore, all battery packs designated for recycling must undergo treatment to drain the rest of the energy before the dismantling or mechanical crushing step.
Treatments to neutralize batteries can be classified into four broad categories according to the reported literature: (1) pulling all Li+ back to its root (cathode) from the anode by forcibly overdischarging the battery, (2) freezing all inner ionic movements via cryogenic conditioning and pushing LIB to a virtual dead mode, (3) thermally evaporating the flammable electrolyte under vacuum or inert atmosphere, and (4) passivating the reactive lithium surface through inert atmosphere processing.
Overdischarge method
Discharge in solid media.
Battery packs that run battery electric vehicles (BEVs) may be in the range of 20–90 kW h, 80–500 V, 200–550 kg, and 400–650 L in terms of energy, voltage, weight, and volume, respectively. From a circular economy perspective, employing the energy recuperated from discharging such large packs into power grids is more remunerative than wasting it as heat. A reported estimation suggests that one 60 kW h battery pack at 50% SoC and 75% SoH can power a UK home for nearly 2 h.36 Hence, an alternative use of the remaining energy is essential. Commercially available electronically adjustable load (EA-ELR-9500-30 supplied by EPS Stromversorgung GmbH) having maximum voltage and current input limit of 500 V and 30 A, respectively, can be used for this purpose. These instruments convert DC energy flow from the battery into a power-synchronized sinusoidal current, which can be utilized later for public power supplies.37
For disassembled modules from BEV battery packs, energy, voltage, weight, and volume vary in the range of 0.1–1 kW h, 5–80 V, 3–25 kg, and 2–20 L, respectively. Energy reclaimed from discharging a single module is inadequate for stationary applications and can be controllably converted into heat through electrical resistors. Considering the dangers of high module voltages, instruments such as TORKEL 860 from Megger GmbH (uses a resistor) and developed circuits with MOSFET (metal-oxide semiconductor field-effect-transistors) having advanced cooling systems are safer options (Fig. 4).37 A vital point is that battery packs decommissioned from EVs at lower SoC cannot deliver usable energy during overdischarge. It is best to treat these module levels weight and volume-wise; it is more compatible for the human to handle. On the other hand, the cell level discharge is more time-consuming and labor-intensive because a battery pack contains larger cell numbers depending on the battery manufacturer and vehicle company. For example, an EV battery pack may have 72–192 prismatic or pouch cells within 8–48 modules, whereas the Tesla Model S uses a total 7104 number of cylindrical cells distributed among 16 modules.36
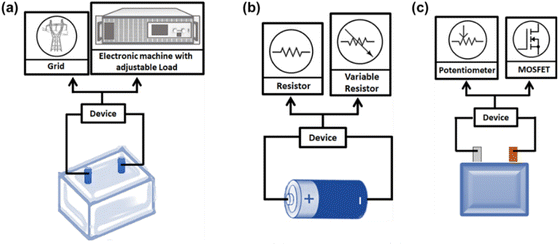 |
| Fig. 4 A pictorial representation of solid medium discharge by (a) adjustable load, (b) electrical resistors for cells, and (c) MOSFET for modules. | |
Individual cells gathered from different lower-power fields of application cover the compass of 2–20 W h, 0–5 V, 0.4–1 kg, and 0.1–1 L for energy, voltage, weight, and volume, respectively. Solid state technologies to passivate them involve discharging with a single resistor system with a maximum current limit of 10 A. Upgraded versions consisting of multiple selectable resistors are more flexible as voltage drop during discharge can be controlled by switching over one resistance value to another accordingly. In addition, covering the cells with sand is used as a protective measure against electrolyte leakage.
Discharge in liquid media.
Another technique to neutralize smaller battery units and individual cells is to submerge them in a conductive liquid. Here, the liquid acts as a resistor and heat sink. Water is the most feasible option as a liquid due to its wide availability, ease of handling, neutral pH (acidic or basic pH can induce corrosion of battery case), and high specific heat (can absorb high heat from the battery body). The required voltage for water oxidation is 1.23 V vs. NHE (a little higher considering kinetic barriers in real cases). The lithium-ion cells with a cut-off voltage of ∼2.5–3.0 V can easily promote the electrolysis of water, which drives the overdischarge process.38 However, the poor conductivity of pure water makes the entire process sluggish, and the addition of salt is necessary to catalyze the voltage drop rate.
A basic setup of liquid media discharge is given in Fig. 4a. Hence, there exists a research incentive to optimize the proper salt. NaCl is widely used as a salt additive because of its low cost. Its aqueous solution is the most studied among all liquid-phase discharging techniques.39–47 Competitive Cl2 gas evolution reaction having higher overpotential than O2 evolution at the anode is the rate-determining step in this process. Corrosion induced by counter anions is one of the essential factors in choosing salt for swift and nonviolent proceedings. Highly acidic salt solutions (pH 1–4) cause severe damage to nickel-plated steel casing, whereas highly alkaline solutions (pH 11–14) perforate the can, leading to electrolyte leakage. Mild acidic (pH 4–6) and mild alkaline (8–11) solutions demonstrate slower corrosion rates, but mild acidic solutions render less visible damage to battery terminals than mild alkaline solutions.42 Halides can also penetrate the outer case by dissolving the rubber gasket and reacting with Al-foil to produce Al(OH)3 foams besides electrolyte leakage.43 NaNO2 solution with a near neutral pH of 7.3 is reported to be the fastest under the described circumstances and safest as nitrites are noncorrosive to steel despite the human hazards of handling nitrites and the possibility of the presence of toxic NOx in the headspace gas.42 However, this underwater overdischarge method cannot opt for high-energy batteries. Large EV battery packs initiate rampant electrolysis and raise the aqueous solution temperature at a steeper rate. Gaseous bubbles evolved through electrolysis undergo rapid breakdown under the water at higher temperatures, producing high-energy electric arcs that may melt most of the materials. Then, a considerable amount of leaked electrolytes will self-ignite.44
The main drawback of this setup (Fig. 5a) originates from the direct dipping of batteries into the liquid media. Therefore, to bypass all physical contact of the battery with conductive liquids, an alternative strategy incorporates connecting the anticorrosive Pt wires to the poles of the battery, thereby closing the external circuit by dipping the wire in the salt solution. Metallic powder is used as the discharge accelerator,45 and a beaker containing the salt solution was kept stirring to dissolve the formed precipitates (Fig. 5b). A BL-4B mobile phone battery (Nokia Company, 3.7 V, 700 mA h) can be discharged from 3.7 V to 0 V within 15 min using Fe chips (particle size <2 mm) in 20 wt% NaCl solution under a similar experimental setup.46
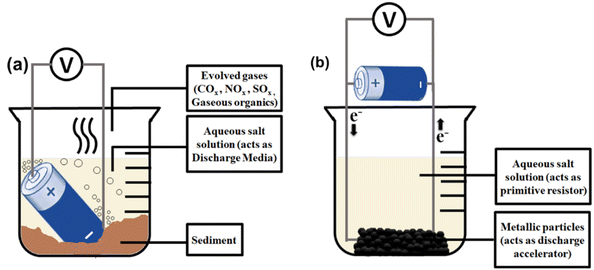 |
| Fig. 5 A pictorial representation of (a) in situ and (b) ex situ salt discharge in a liquid medium. | |
The discharge efficiency in liquid media cannot be evaluated on a generic platform as in different reported techniques, parameters such as the configuration of used batteries, SoH and SoC of the spent batteries, concentration of the salt solution, temperature while performing the experiments, detection of corroded elements in sediments and supernatants, and the purity of chemicals varied. However, it can be stated from the chemical kinetics point of view that a higher salt concentration increases the number of dissociated ions per unit volume in an aqueous solution. As a result, the conductivity of the media rises, and faster gas evolution occurs at the battery poles, which results in a quick discharge. However, after a certain concentration, conductivity does not increase significantly; rather, the rate of corrosion enhances due to the presence of a large number of salt anions.47 Discharging with powders is also reported. Conductive metal powders can result in faster discharge but the generated heat cannot escape from the system rapidly, bringing the possibility of dust explosion. However, the discharge in semiconductive graphite powder progresses at a mild rate.48 Neither of the methods is congruent to the industrial scale.
Literature reports on the technical developments of electrochemical neutralization via the overdischarge method are plenty. But standardized setup, optimum level of discharge, and suitable time are not well-outlined. Furthermore, the additional cost of sophisticated instruments, cooling facilities, and other safety arrangements to run the process pushes it far from being widely accepted in the industry, notwithstanding the technical benefits. Hence, insights into the chemical mechanism of the overdischarge process are essential to understand the origin of the loophole. LIBs can relax back to open circuit voltage (OCV) after discharge. To avoid this, internal short-circuit (ISC) induced by forced overdischarge is performed.49 Overdischarge is initiated below the cut-off voltage. In this case, Li+ from the anode moves toward the cathode. This phenomenon reaches completion at zero volts (0% SOC), where the rest of the capacity is released. During discharge below 0 V, pole reversal occurs first. Subsequently, the battery is forced to deliver the charged ions. At about −2 V (−11% to −12% SOC), the potential of the anode reaches a high enough value (∼3.4–3.5 V) to actuate the electrochemical oxidation of the Cu-current collector. The released Cu2+ ions move to the cathode in a similar manner to Li+ but are deposited on the surface instead of intercalating. Finally, a dendrite growth occurs, puncturing the separator to establish a direct internal connection between two electrodes, i.e., ISC.50,51 To secure a lower voltage after the relaxation period, a longer discharging time is recommended, such as 24 h for 5 V cells and several days for 400 V EV packs. It is because an electric shock of DC current emitted from a short-circuited battery can cause burn injuries and internal damage to workers.37 Before ISC, the produced gas from solid-electrolyte interphase (SEI) decomposition creates high internal pressure and cell swelling at about −10% SOC. As a result, active cathode material loses its morphology via solid-state amorphization. It is one of the major drawbacks of the discharging method. The contamination of all internal components due to copper deposition is another issue.51,52
Cryogenic conditioning
To and fro motion of Li+ between electrodes is the essential part of an LIB. It causes interconversion between electrical and chemical energy and keeps the cell lively. Exposing a battery to a lower temperature slows down the ionic mobility. Extreme low temperatures can even cease all ionic movements inside. Hence, immersing an LIB in liquefied cryogenic gas freezes the electrolyte (liquid media that channels Li+) and pushes the LIB into a virtual dead mode.53–55 Temperature lesser than −50 °C is helpful for this purpose. Liquid argon (−90 °C) and liquid helium (−269 °C) can also be used, but liquid nitrogen (−200 °C) is the cheapest option.51 Small cells should be dipped within liquefied gas for a few minutes (4 min)54 to freeze and harden, while dismantling treatment is performed under a continuous flow of liquified gas for larger battery packs before proceeding to the next steps.55
Thermal treatment
Thermal energy is the cheapest source of energy to activate a chemical process. However, a battery cannot be heated in an aerial atmosphere because the internal pressure of produced combustible gases, such as POF3, PF5, CF4, HF, H2, alkanes, alkenes, and alkyl halides, may cause a violent explosion.56,57 But the rapid energy release can be controlled if these processes are carried out in a controlled manner under the presence of an inert atmosphere and within instruments such as vacuum induction furnaces or rotatory kilns.58,59 Heating slowly at elevated temperatures put a stop to all Li+ movements by evaporating the electrolyte. However, a temperature higher than 200 °C is strictly avoided as the electrolyte is susceptible to decomposing at that range. Pyrolysis techniques are mainly adapted at the industry level but can also be performed at the lab scale with a slower degree of temperature rise. However, thermal treatment decays the binder, damages the separator, affects the crystallinity of active materials, and produces a mixed melt of several components that are difficult to separate later.57,60 Hence, the suitability of this method to be included in the all-component-recovery scheme is questionable.
Inert atmosphere processing
Inert atmosphere processing slows the rate of exothermic reactions between the deposited metallic lithium and electrolyte with oxygen and atmospheric moisture. Crushing or shredding in inert gases such as nitrogen, argon, carbon dioxide, or a mixture of argon and carbon dioxide (<5000 ppm of molecular oxygen) is an economical and safe industrial practice.61 Due to higher density, carbon dioxide and argon (1.98 kg m−3 and 1.78 kg m−3, respectively) are more effective than nitrogen (1.229 kg m−3). Carbon dioxide reacts with metallic lithium to initiate the formation of lithium carbonate and deactivates the surface. However, this process mandates an inert atmosphere chamber. Considering the large number of cells to be recycled per day in the industry, inert atmosphere processing to neutralize the cells may reach a slower pace of the overall recycling route.
Dismantle and dissever
It must be dismantled first to access all inner parts of end-of-life (EOL) batteries.62,63 The dismantling strategy directly affects the quality of recovered materials. The route that can ensure the recovery of all components is preferred over others. Procedures have been developed at the lab scale for dismantling small cells used in portable electronics. But very few have gained industrial acceptance for large systems. EV companies design the configuration of battery packs as a compromise among several factors that need to be taken care of for long service life, rider safety, superior performance, available space for battery installation, and market price.64 Depending on the technical characteristics, the diverse nature of interior and exterior design for EV battery packs are commercially available. Hence, it is not easy to establish an optimized disassembly line for all types of LIBs. Reported dismantling techniques can be broadly divided into two categories: (1) comminution-sieving and (2) sequential segregation. Some common steps in EV battery disassembly are as follows: (a) unscrew and remove all casings and coverings, (b) detachment of hardware such as connecting wires, stack holders, and cable ties, (c) uninstallation of powered electronics, i.e., gas venting, BMS, thermal sensors, and master board.65 Waste electric and electronic equipment (WEEE) can be reused directly or handed over to WEEE recycling industry. Recovered Cu finds its uses in road construction, ballast, cement, and concrete industry,66 whereas waste Al-foil can be remodelled into value-added commercial products.67,68
Comminution-sieving
Comminution (dry, wet, and cryogenic).
Mechanical crushing is suitable at the industry level as a large number of packs can be processed in a short time with high throughput. It is cost-effective as well because the basic structure of the crushing machine is more or less the same for all the applications. In simple steps, grinding facilitates the liberation of materials encapsulated in electrodes, separators, plastic cases, and other battery components. However, high-energy-containing EV battery packs are rarely crushed in a single step. Firstly, batteries are teared down into smaller fragments in the shear crushing step. Secondly, impact crushing is used where the blades of the crusher comminute the feed in an air-isolated chamber. Mechanical crushing uses the difference of ductility among different parts that comprise a battery pack. Metal foils and battery casing materials having higher ductility are challenging to break into smaller pieces, whereas active electrode materials produce finer particles on granulation.69,70 Crushing in a dry state is most effective for selective grinding as it uses ductility difference to its whole potential but is associated with the toxic gas release. To hinder the negative side effects of gas evolution, wet crushing using water or brine solution is reported. However, due to the scouring action of water, the difference in the mechanical crushing property diminishes, and metal foils end up in a mixed state with electrode-active materials, making further purification troublesome.39 Hence, cryogenic grinding under an inert atmosphere is the best alternative.
Based on the instrumental setup and type of forces applied, comminution can be classified into 5 categories—shear, cropping, striking, impact, and pressure. In shear comminution, the batteries are cut into two or more parts using moving blades. The size of the resulting product depends on the speed of the blades. It is the most common comminution process and currently used in most recycling industries. In the cropping comminution process, the batteries are disjointed using two tools that move in opposite directions. In the striking comminution process, the batteries are smashed by a heavy object. Impact comminution is the technique where the batteries are crashed against a moving wall or mutually collide. The batteries are pressurized within two movable walls in pressure comminution and crushed. These are the basic definitions of the types of crushing. But industrial comminution units are made using two or more comminution types. The entire setup of the comminution device is customized based on the industrial target and varies largely from one another. For example, Akkuser uses mainly shear crushing, whereas all types of comminutions are utilized in some parts of the Dussenfeld process.36
Sieving.
The distribution of particle size in crushed bulk depends on the speed of crusher blades, time of contact, and efficiency of energy transfer from the crusher blades to the feed. Then, the shredded material is sieved into separate fractions through selective apertures in the equipment. Broadly, it can be differentiated into three size fractions: a coarse fraction that contains battery casing, outer shells, current collectors, plastics, etc., a finer fraction of electrode active materials, and a middle fraction where active materials along with current collectors and separators are present in the agglomerated form and need further grinding before subsequent treatments.71,72 The next step is to sequester components from each fraction based on physical properties, such as electrical conductivity, specific gravity, density, magnetism, and surface hydrophobicity.
Separating components from coarse and midfraction.
Magnetic separation is conducted to distinguish magnetic and nonmagnetic portions. Low-intensity hand magnets are strong enough to separate ferromagnetic steel from the rest of the coarse fraction. High-intensity rare-earth magnetic rolls can concentrate paramagnetic aluminium away from mildly-responsive copper.73 The magnetic process is mentioned in the patents of Akkuser, Recupyl, and Accurec as the steel casing remover.61,74 The technique can also be utilized to separate the components of active materials based on the difference in their magnetic susceptibility. The ReCell project applied a wet magnetic technique to separate the mixture of virgin cathode materials with a >90% efficiency.75 Although the initial results are promising, the cost-effectiveness of the approach at a large scale is unexplored. Density-based classifiers single out materials based on their movements in response to the field of gravity. Generally, larger particles are more affected than smaller ones. Therefore, the sizes should be uniformized to make relative movements more dependent on specific gravity. Lightweight plastics can be easily separated from heavy metals such as copper because of the pronounced gap in densities between copper (8.96 g cm−3) and plastics (<1 g cm−3).76,77 The process of Akkuser includes the separation of evolved gases and low-density plastics (papers and separators) by a cyclonic air remover.74 Casing materials and high-density plastics (pouch cover) remain unaffected. The use of a zig-zag separator is mentioned in the processes of Accurec and Dussenfeld, while the Retriev process uses a shaker table.60,78–80 The main components that can be targeted to separate by the density separation technique are electrode foils (Cu and Al), separators, and casing material. Spouted bed elutriation is an inexpensive option to separate crushed fractions by specific mass and particle size differences.81 Electrical properties are another set of parameters based on which separation can be performed. After extracting ferrous metals using a magnet, nonferrous metals are dragged out from nonmetals by an eddy current separator.73 The eddy current rotor at the end of the conveyor belt exerts repulsive forces in electrically conductive metals due to the interaction between an alternative magnetic field and eddy current induced by the magnetic field. It throws nonferrous metals in a particular direction, while nonmetals fall off due to gravity. Based on the difference in electrical conductivity between metals and nonmetals, the shredded fraction can be divided into conductive and nonconductive zone via corona electrostatic separation. Al and Cu can be recovered from coarse and midfractions. On the other hand, nonconducting plastics may be pulled out of coarse fractions through triboelectric separation.82 Corona electrostatic and triboelectric methods are mainly applied for electrical and electronic waste management but possess great potential to be exploited in the case of LIB waste.
Separation of components from the fine fraction.
Froth flotation is a valuable technique to separate cathode and anode active materials in the fine fraction based on their surface hydrophobicity differences. It also preserves the functional integrity of materials. The contact angle between the water droplet and the material surface dictates the surface wettability properties. A smaller angle means higher hydrophilicity, whereas a larger angle reveals higher hydrophobicity. Therefore, the higher the angle difference, the more significant the wettability gap, and consequently, the separation via froth flotation will be easier. Pure graphite is a naturally hydrophobic material having a contact angle of ∼80°.83 But water can easily seep through the metal oxide layer in LiMO2, causing the surface to be hydrophilic. Thus, graphite is floated with froth during the procedure leaving the metal oxide separated in the concentrate. However, the difference in contact angle is not remarkable for spent battery materials because metal oxides are composited with hydrophobic carbon additives and polymer binders. At the same time, graphite layers develop hydrophilic functional groups due to surface oxidation with ageing.84 Fenton reagent-assisted froth flotation is reported as a modified strategy. Hydroxyl radical (˙OH), generated from the decomposition of H2O2 by Fe2+, breaks the polymer binder apart into small molecules and oxidizes the organic layer to form CO2 and H2O. Unfortunately, only 5% increased efficiency is obtained than direct flotation.85 The main reason behind that is the rapid increase of pH during the action of hydroxyl radical that precipitates Fe(OH)3 and its subsequent adsorption on the electrode material surface levels up the wettability property, resulting in poor recovery rate. In a study, HCl is used as a pH buffer to maintain a highly acidic pH that further improves the efficiency rate.86 Another method is grinding flotation, where mechanical attrition wipes off secondary layers to expose primary surfaces and enhances original wettability. The horizontal shearing force of grinding disintegrates the internal lamellar structure of graphite but only partly smoothens the cathode surface due to the inherent hardness of metal oxides. In contrast, the vertical rolling pressure of grinding causes the surface adhesion of graphite with metal oxides, thus worsening the scenario.87 Hence, the optimization of grinding time, the ratio between the weight of steel or zirconia balls and waste solid, shape of grinding medium, and absence of any external contaminants is critical for better efficiency. Thermal treatment is also a way that evaporates all organic impurities away and increases the contact angle difference. In the roasting flotation experiment, the highest value of angle difference is obtained at ∼400 °C. Temperature higher than 550 °C is avoided to prevent the ignition loss of graphite.88 In pyrolysis-enhanced flotation, heating is done within an enclosed chamber to capture toxic gases from the binder and electrolyte decomposition. This method is more environment friendly than roasting pyrolysis.89 At conditions standardized for flotation, the reported concentrate grades for cathode material (LiCoO2) obtained from direct flotation, Fenton flotation, HCl-modified Fenton flotation, roasting flotation, and pyrolysis-enhanced flotation are 55.63%, 60.03%, 75%, 89.64%, and 98%, respectively.86 Despite demonstrating several promises, issues related to the discharge of metal-contaminated flotation wastewater should be mitigated before the large-scale applications of froth flotation.
Mechanical crushing as pretreatment is industrially viable. Fig. 6 represents a flowchart of the separation methods of the cell components via crushing and deep screening based on particle size fraction.90 The elemental distribution plot (Fig. 7) shows that the cathode (Li, Ni, Mn, Co) and anode (C) are mostly concentrated in a −0.2 mm size fraction, whereas Al and Cu are of a +1.4 mm size fraction.89 However, some electrode materials are hard to separate from the current collectors due to binders that reduce the recycling efficiency. But it has several drawbacks. A lot of energy is wasted in crushing, sieving, and other separation steps. During crushing, micro short-circuits occur between cathode and anode fragments, inducing Joule heating that may evaporate the electrolyte. Evolved gas and a vast amount of dust contain toxic substances and cannot be released into the atmosphere.60 Besides, thick crushing byproduct layers accumulate on the desired material surface. High-resolution X-ray photoelectron spectroscopy reveals 75% organic product, 5% metal oxide, 6% metal fluorides, and 2% phosphates in the surface composition of LiCoO2 and graphite. Therefore, additional costs to manage post-processing contamination may outweigh the beneficial effects.91
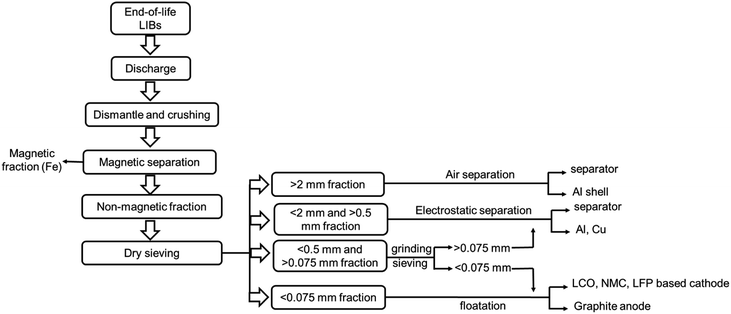 |
| Fig. 6 A flowchart representing the physical separation of the cell components from end-of-life LIBs based on particle size classification. | |
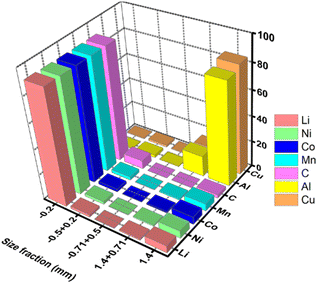 |
| Fig. 7 Elemental distribution of the electrode components after the sieving process in different size fractions. | |
Sequential segregation
The dismantling of cells to sequentially segregate inner parts is performed manually with proper protection kits such as safety glasses, gloves, gas masks, and within fume hood that absorbs toxic gases. A manual procedure for dismantling small LIBs is reported. Firstly, plastic cases are removed by a small knife and screwdriver. The battery is immersed in liquid nitrogen for 4 min and fixed in a lathe. The metallic shell is cut by a saw and removed gradually, starting from the terminal. After cutting along the longitudinal line, the inner parts are disengaged using pliers. Anode and cathode rolls are uncurled and dried at 60 °C for 24 h for the slow evaporation of the immobilized electrolyte.54
Automated segregation.
The manual dismantling of gigantic battery packs in the industry is a challenging and impractical task. Hence, automation is earnestly required from an economic standpoint. It can secure faster disassembly, winnow out the lifetime risk of human operators, enhance the purity of the recovered material, simplify downstream separation, and make sequential segregation applicable in the recycling industry. A robotic gripper is reported that identifies battery types, adjusts itself according to cell geometry, grips tightly, and displaces the cell smoothly.92 An automated assembly line for Z-folded cell type has also been developed, which trims the sealed edges and metal tabs, peels off Al-laminated housing, recovers electrode-separator system and sorts of cathode, anode, and separator, respectively.93 But the exact degree of robotic implementation is subject to question. Robots carry out iterative tasks in the engineered environment and even the simplest robotic movements are driven by arduous computer programming. Unluckily, versatile battery pack designs by manufacturers to meet customers' demand, lack of standardization in module fabrication, different approaches to dismantle cylindrical or pouch or prismatic cells, insufficient numbers of trained employees for managing the automated setup, and the exponential increase of battery-run gadgets over past few years present a massive challenge to artificial intelligence experts. It entails a human-robot collaboration to overcome the automated disassembly shortcomings.94 Literature reports are available on developing robotic parts that accomplish unscrewing and gripping in a shared space with humans.65,95 In the manufacturing plants of Volkswagen, BMW, Audi, etc., robots are already used to assist human workers in handling coolant expansion tanks, actuators, sensors, door sealing, cylinder head assembly, etc.96 But the fields of application are mainly confined to noncognitive tasks. One of the biggest concerns of the hybrid workstation is the collision injuries for human operators as safety cannot be negotiated at any cost. Robotic sensors are being processed that can detect the proximity of obstacles and change the velocity accordingly to avoid accidents. By implementing these visual, capacitive, and motion sensors, a dynamic workspace for robots can be created where humans and robots collaborate without overlapping each other's specified working zones.97,98 Nevertheless, the safety regulations of hybrid workplaces slow the progress rate, and it lacks productivity compared to conventional workstations. Therefore, semiautomated sequential segregation is anticipated soon but demands advancement in robotics research to push the biomechanical limits.
Challenges in cell vs. battery pack disassembly
The mechanical crushing of the collected battery packs is the industrially viable route to recover the metals easily using a hammer mill. However, this results in a mixed waste stream of electrode materials, plastic scraps, steel casing, etc. Proper sieving and separating components from coarse and midfraction methodology might compromise the purity during several metal recovery steps. Therefore, disassembling the battery pack module to the cell level is the only choice to segregate the high-graded metals in their purest form. It has been observed that different battery manufacturers use different cathode materials of lithium transition metal oxides or phosphates (NMC, LMO, NCA, LCO, and LFP). Besides, the manufacturers adopt different cell configurations such as cylindrical for Tesla model S 85 Mk1 kW h battery pack (Panasonic), prismatic for BMW i3 Mk1 22 kW h battery pack (Samsung/SDI), and pouch for Nissan Leaf Mk1 22 kW h battery pack (AESC).36 It requires unique strategies to disassemble the battery pack, causing a severe challenge to automation. An automated system is reported that classifies batteries according to cell chemistries by recognizing machine-accessible features such as QR codes, labels, or tags. But improvements are required before rigorously implementing it at the industry level. Assigning labels from the manufacturer side on the outer cover of batteries indicate that active material chemistry will be a massive push toward automated segregation. The World Economic Forum and Global Battery Alliance also suggested a common data-sharing platform for EV batteries in their report. Recently, the concept of the Battery Identity Global Passport (BIGP) that passes information on battery chemistry to the recycling industry has been proposed.99 But the challenging aspect is to bring all the manufacturers to the same ground and attune them to share proprietary information.
Recovery of black mass
Unlike mechanical comminution, the recovered material is not obtained as a commingled mass in this process. Therefore, higher purity is ascertained for all parts. However, segregated cathode and anode rolls need further treatments for the delamination of black mass from aluminium and copper foil. A blend of redox-active electrode material, carbon as a conductive matrix, and organic polymer as a binder is known as a ‘Black Mass’. The binder keeps the active material attached to the current collector. PVDF is industrially most used as the binder for the cathode other than PTFE. Styrene butadiene rubber (SBR) and sodium carboxy methyl cellulose (SCMC) are used for the anode. But the adhesive force of binders degrades to a certain extent after prolonged cycling due to two reasons, mainly (a) the generated mechanical stress as a result of volume change during the lithiation–delithiation process lowers the adhesive nature, (b) the dissolution of the polymer by organic carbonates in electrolyte misbalances the ratio of active material, carbon, and binder to reduce the integrity of black mass.100 These phenomena that provoke cell failure ease the process of delamination. Moreover, copper, being more malleable, is easy to separate from graphite. Thus, the reported literature in active material delamination can be divided into four categories: (a) dissolution of the polymer binder using solvents, (b) alkaline medium to remove Al as the soluble salt, (c) thermal treatments to evaporate the binder, and (d) depolymerization of PVDF.
Solvent dissolution
This method uses organic solvents to dissolve binders and detach the black mass from the current collector. NMP is mainly used as a solvent because it can dissolve more than 200 g PVDF in 1 L. In this process, the cathode roll is dipped inside NMP at a 10
:
1 ratio of liquid:solid and heated to about 80–100 °C for 3–4 h.101 The ultrasonic effect can catalyze the dissolution process. Ultrasound produces acoustic cavitation in liquids. These cavitation bubbles grow rapidly as the dissolved gas inside the solvent enters them. Then, its implosion within no time due to increased adiabatic compression pressure releases high-energy jets toward a solid surface. The associated shockwaves with the jets create ultrasmall hotspots when they hit a solid mass. It causes the black mass to peel off from the metal foil. The dissolution of PVDF by NMP from the black mass becomes more straightforward. Thus, ultrasonication provides enough activation energy to decrease the applied temperature and facilitates the process in lesser time. The peel-off efficiency is reported to be increased by 6% than normal dissolution for all solvents when a 240 W ultrasonic bath is used under optimized conditions.102 According to the literature report, a specially designed high-power ultrasonic (maximum power intensity = 70 W cm−2) equipment ruptures the adhesive force between the current collector and active material within 0.5 s.103 The principle behind the solvent dissolution process lies in ‘like dissolves like’. PVDF, being more polar due to the presence of the –CF2 group adjacent to the –CH2 group, gets dissolved better in polar solvents such as NMP, DMAC, DMF, and DMSO than the less polar acetone, CCl4, and ethanol. The roughly estimated relative efficiency order of different solvents for PVDF runs as NMP > DMAC > DMF > DMSO > H2O > DCM > CCl4 > acetone > ethanol.103–105 Conversely, tetrafluoroacetic acid (TFA) is a better choice for the more polar PTFE binder.106 Due to the higher cost and hazardous nature of NMP, other solvents such as DMSO and DMF are also exploited as cheaper and safer alternatives.105 Triethyl phosphate (TEP) is not carcinogenic, teratogenic, or mutagenic and performs equally well as NMP.107 After the treatment, the whole liquid media is filtered. The accumulated mass in the residue is heated to burn off carbon, whereas the filtrate is heated at a temperature higher than the boiling point of NMP (202 °C). NMP can be distilled as PVDF does not decompose below ∼350 °C and can be reused. Al-foil is washed using dilute H2SO4 to remove trace impurities. The advantages of this process include the recovery of Al-foil in the metallic form, PVDF in its compound form, and reusable solvent. However, further experiments on the purity of the recovered active materials suggest that residual PVDF agglomerates increase the average particle size and decrease the product quality.108 Therefore, additional heating to liberate the active material from residual PVDF and carbon and a certain amount of toxicity of the used solvents need to be overcome.
Removal of Al-foil
Al is amphoteric in nature. If not removed before the extraction step of valuable metals, Al3+ will be deposited along with Cu, Co3+, and Li+ to change the pH of the medium. Al is solubilized in aqueous alkali to avoid unwanted contamination as a pretreatment. NaOH can dissolve Al and the surface protection layer, i.e., Al2O3 as Na[Al(OH)4]. 10 wt% (w/w) of its aqueous solution at a 1
:
10 (solid
:
liquid) ratio is reported to recover Al with 98% efficiency at room temperature within 5 h.45 The Pourbaix diagram of the Al–Na–H2O system depicts increased Al leaching efficiency with an increase in the NaOH concentration. But 10 wt% NaOH solution is the most suitable to eliminate the possibility of precipitation.109 The disadvantages of this process are the generation of a huge volume of environmentally harmful alkali wastewater and the recovery of Al foil in nonreusable ionic form.110 Furthermore, extra efforts to liberate the active material from black mass offset the advantages.
Thermal treatment
Thermal treatment involves simple operational procedures and is associated with high throughput. The PVDF and carbon black decomposition temperatures are ∼350 °C and ∼600 °C, respectively. A temperature of about 600 °C is perfect as at the decomposition temperature. At temperature higher than 600 °C, Al-foils become fragile. The evaporation of binder and conductive carbon decreases the adhesive force between the current collector and black mass and cohesive force among cathode active material particles. The ‘Adhesion Neutralization Via Incineration and Impact Liberation’ (ANVIL) method uses a specially designed setup in which the cathode rolls are heated at 550 °C under an oxygen atmosphere and high-speed air jet to leave the impact stress on the agglomerates. But the incineration of electrodes in the aerial atmosphere releases corrosive gaseous byproducts, such as HCN, HF, HCHO, COF2, HNCO, CO, and CO2. Trapping these gases is necessary instead of releasing them into the environment.100 The vacuum pyrolysis method is exploited as a solution. It functions at 600 °C and a cooling chamber is attached, which condenses small fragmented gaseous molecules from the electrolyte or PVDF, leaving a solid metal oxide in the main product chamber.111 In reducing thermal treatment, acetylene black from the black mass is utilized as a reducing agent to generate more soluble low-valent metals from metal oxide. It executes a clean separation of Al and Cu foils that can be reused after a water wash.112,113 A molten salt mixture drops down the high applied temperature. AlCl3–NaCl demonstrates the best performance with a 99.8% peeling-off efficiency. This salt mixture began to absorb heat at about 125 °C, underwent a thermodynamic phase transition at 160 °C, and dissociated into ions, which act as a high-temperature reaction medium. The released heat can melt PVDF at 160 °C within a contact time of just 20 min.114 Ionic liquids have also been demonstrated as benign delaminating agents. N-substituted imidazole, [BMIM] [BF4], works as a rapid heat conduction medium to dissolve PVDF at only 180 °C with a peel-off efficiency of 99%. Water soluble ionic liquid can be recovered and reused.115 Therefore, thermal treatments are compatible at the industry level, but low-cost sustainable catalyzing methods are needed to be scaled up to decrease the temperature.
Depolymerization of PVDF
Polyvinylidene difluoride (PVDF) is a highly nonreactive fluoropolymer. Its adhesive nature depends on its polymeric integrity. But, the strongly electron-withdrawing –CF2 group renders the hydrogen of the adjacent –CH2 group susceptible to base attack. This chemical sensitivity is utilized deliberately to depolymerize PVDF. Deep eutectic solvent, i.e., a mixture of choline chloride and glycerol at a 2.3
:
1 ratio, delaminates 99.8 wt% black mass from cathode scraps at 190 °C with 15 min of contact time. The produced hydroxyl group (–OH) in the solvent mixture converts PVDF into an enol monomer and deactivates it.116 Solid phase alkaline CaO bed also acts as a versatile defluorinating agent via the same mechanism. It converts PVDF into unsaturated hydrocarbon, adsorbs toxic CO2 and HF to generate environment-friendly CaCO3 and CaF2, and simultaneously acts as a heat sink. At 300 °C, it shows >95% efficiency for all types of cathode materials (LCO, NMC, and LFP).117 These processes incorporate cheaper sources, take less time, and can be used in the industry as a greener alternative.
Table 1 represents the pretreatment protocol of a few selected recycling industries followed for the black mass recovery from spent LIBs containing LCO, NMC, LFP-based cathode, and a graphite anode.
Table 1 A summary of industrial black mass recovery processes and beyond
Company name |
Summary of the process |
Subsequent recycling method and recovered products |
Onto Technology118 |
Discharge – cell case perforation – inner cell components separation in supercritical conditions – sieving – magnetic separation |
Cu, Al foils, steel casing, electrolyte, black mass |
Sony-Sumitomo119,120 |
Sorting – dismantling – incinerated >1000 °C |
Acid leaching – Ash contains organics, lithium, fluorides – steel, Cu recovered by physical separation and Co recovered by hydrometallurgy |
Umicore119,120 |
Dismantle |
Pyrometallurgy – Co, Ni, Cu form alloys and Li, Al, Fe, Mn are found in slag – hydrometallurgy – precipitation of metals |
Retriev Technologies (Toxco)69,119 |
Short-circuit in cooled atm −196 °C – shredding – sieving to separate metals |
Metals recovered by hydrometallurgy and lithium as Li2CO3 |
Recupyl Valibat120 |
Shear in the inert atmosphere – screening and magnetic separation |
Li3PO4, Li2CO3 and Co(OH)2, Co metal by leaching |
JX Nippon Mining and Metals120 |
Incinerated >1000 °C – screening – acid leaching – selective metal precipitation |
Li2CO3, MnCO3, Co, Ni metals |
Duesenfeld37 |
Shredding in inert atmosphere CO2-assisted electrolyte extraction – sieving magnetic separation to eliminate separator and cell-case |
Thermal treatment (400–600 °C) to separate black mass from current collector hydrometallurgy for metal recovery |
In summary, an effective collection methodology with national or supranational legislation, storage, and sorting for retired batteries is essential in sustainable e-waste management. The crucial step is recovering the black mass and high value-added metals after carefully dismantling and removing the polymer binder and carbon black. The mechanochemical process for metal extraction with a low level of contamination is only possible via different cathode material-based sorting and initial shredding processes with sequential segregation. Besides, the recycling efficiency will be enhanced if the battery module pack is torn down to the cell level. Furthermore, recycling high cobalt-content LCO-type batteries must be prominent to reinforce the scarcity of cobalt. Therefore, an industry-compatible low-cost pathway for material separation on different scales from spent LIBs is a global research hotspot for resource sustainability and economic prospects.
Industrial trends in black mass recovery – current status and future outlook
The logistics of battery waste are not well organized yet. Industries receive LIBs in bulk mixtures with other types of primary and secondary batteries. As the number of retired EV battery packs is much lesser in the status quo, industries are not aiming toward the direct reuse of battery packs, modules, or cells. Therefore, SOH-based sorting is not needed now. On the other hand, cathode material-based sorting is not possible as the retiring batteries do not have any labels or tags containing information about the active material. The entire process of labeling the batteries is in progress. Countries are bringing new legislation on ‘battery identification tags (barcodes, QR codes, id numbers, etc.),’ and industries will adopt that soon. This is why batteries retiring around 2030 or beyond will be suitable for active material-based sorting. Thus, the waste logistics, collection, transport, storage, and diagnosis techniques described in this article will be in effect in the future. Currently, LIB packs are manually sorted from the mixed stream of battery waste using a moving conveyor belt.
The use of the overdischarge method in the neutralization step is not adopted in the industry. The use of electronic machines for solid media discharge is only useful when there are plans to store and reuse the contained energy of spent battery packs. The liquid media discharge is apt for a lab-to-pilot scale only. The excessive volume of wastewater adds an extra burden to the large-scale recycling industry. For neutralization, industries are more prone to use inert atmosphere processing (Recupyl, Onto, LithoRec, etc.) and cryogenic conditioning (Retriev, Dussenfeld, etc.). In the next step, industries mainly comminute the battery packs in their custom-built device with a constant supply of inert gas or very low temperatures. Dussenfeld uses a deactivation powder to cease the spontaneous chemical combustion of active materials. However, the details of these are not publicly disclosed. In the sieving process, magnetic and density-based classifiers are used by all industries in some form. The shape, size, and style of classifiers are uniquely designed based on the other processes in the recycling line.
The process of active material recovery from the electrode rolls is divided here into several types to incorporate all previous literature reports. Undoubtedly, these techniques will be highly used in the future when the recovery of all components is aimed. But the current industrial scenario can be generalized in the following way. Typically, a mixture of strong acids or alkalis with reducing agents is used for leaching black mass from current collectors, followed by a thermal treatment to extract the active material.
In short, the current industrial trend of black mass recovery can be summarized as follows. An unorganized bulk of spent batteries are manually sorted. The sorted LIBs are comminuted under the controlled setup, i.e., the application of inert gases and low temperatures. The useful black mass is sieved by density, gravity, and magnetic classifiers. After the acid or alkali leaching, active materials are extracted from the black mass using thermal treatments. However, considering the aim of a closed-loop battery economy and the necessity of recovering all components, other techniques mentioned in this article will be adopted in the industry very soon. In particular, artificial intelligence-based automation can revolutionize the recycling process and is earnestly required to control the huge amount of LIB waste.
Conclusion
The booming market of LIBs might result in an inevitable amount of spent LIBs in no time. A European Union (EU) statistic predicted the generation of 11 million metric tonnes of discarded LIBs at the end of 2030. From a qualitative perspective, recycling spent LIBs is worth consideration due to its high recovery value of metal-enriched functional components. Despite growing attention and the development of several recycling strategies, only 3% of spent LIBs are being recycled today. Apart from recycling the batteries, echelon utilization has an alternative importance, where the battery is reused for second-life applications to other energy storage devices such as communication base stations. Proper policy incentives by government and legislative pressure might encourage the collection rate and recycling efficiency, reducing the environmental and economic burden. In addition to the high material recovery rate, high-grade material recovery and electrode resynthesis are challenging for battery manufacturers or other industrial applications. The waste chemicals, solvents, and byproducts during the leaching steps of cathode recovery must be treated appropriately with minimized ecotoxicity. Redesigning the existing technologies with better sorting methods, segregation, sustainable separating technology for electrode materials, effective architecture of EOL battery recycling, and manufacturer standardization can potentially benefit the closed-loop recycling project. Furthermore, automation in the dismantling step with artificial intelligence-based hybrid robotic setups can diminish the risk to human workers. However, the physical dismantling process is in the infancy stage and limited to the laboratory scale; more insight is still needed for pilot-scale industrial applications that hold the key to an unprecedented growth trajectory in the portable electronics and automotive industries in the near future.
Author contributions
Madhushri Bhar: conceptualization, methodology, writing-review & editing. Shuvajit Ghosh: conceptualization, methodology, writing-review & editing. Satheesh Krishnamurthy: project administration, funding acquisition. Y. Kaliprasad: resources, validation, industry coordinator. Surendra K. Martha: supervision, conceptualization, writing-review & editing, project administration, funding acquisition, communication.
Conflicts of interest
There are no conflicts to declare.
Acknowledgements
MB acknowledges DST-INSPIRE (code: IF180708) Ministry of Science and Technology, Govt. of India for fellowship. SG acknowledges CSIR, Govt. of India (File No: 09/1001(0067)/2019-EMR-I) for fellowship. SKM acknowledges the UKIERI program under grant no. DST/INT/UK/P-173/2017, Govt. of India, for the funding. SKM and SK further acknowledge partial funds from the Royal Academy of Engineering (Academic reference IAPP1R2\100125) for initial support of this work.
References
-
Three new lithium ion batteries, https://www.maritimejournal.com/news101/power-and-propulsion/three-new-lithium-ion-batteries Search PubMed.
- T. Kim, W. Song, D.-Y. Son, L. K. Ono and Y. Qi, Lithium-ion batteries: outlook on present, future, and hybridized technologies, J. Mater. Chem. A, 2019, 7, 2942–2964 RSC.
- X. Ma, M. Chen, B. Chen, Z. Meng and Y. Wang, High-performance graphite recovered from spent lithium-ion batteries, ACS Sustainable Chem. Eng., 2019, 7, 19732–19738 CrossRef CAS.
-
N. Bullard, Electric Car Price Tag Shrinks Along With Battery Cost, Bloom. Opin., 2019, https://www.bloomberg.com/opinion/articles/2019-04-12/electric-vehicle-battery-shrinks-and-so-does-the-total-cost Search PubMed.
- R. J. Brodd and C. Helou, Cost comparison of producing high-performance Li-ion batteries in the US and in China, J. Power Sources, 2013, 231, 293–300 CrossRef CAS.
- G. Berckmans, M. Messagie, J. Smekens, N. Omar, L. Vanhaverbeke and J. Van Mierlo, Cost projection of state of the art lithium-ion batteries for electric vehicles up to 2030, Energies, 2017, 10, 1314 CrossRef.
- B. K. Sovacool, The precarious political economy of cobalt: Balancing prosperity, poverty, and brutality in artisanal and industrial mining in the Democratic Republic of the Congo, Extr. Ind. Soc., 2019, 6, 915–939 Search PubMed.
- S. DeCarlo and D. Matthews, More than a pretty color: the renaissance of the cobalt industry, Int. J. Economics Commerce Manag., 2019, 1 Search PubMed.
- Y. Li, W. Lv, H. Huang, W. Yan, X. Li, P. Ning, H. Cao and Z. Sun, Recycling of spent lithium-ion batteries in view of green chemistry, Green Chem., 2021, 23, 6139–6171 RSC.
- Y. Jiang, X. Chen, S. Yan, Y. Ou and T. Zhou, Mechanochemistry-induced recycling of spent lithium-ion batteries for synergistic treatment of mixed cathode powders, Green Chem., 2022, 24, 5987–5997 RSC.
- X. Zhang, L. Li, E. Fan, Q. Xue, Y. Bian, F. Wu and R. Chen, Toward sustainable and systematic recycling of spent rechargeable batteries, Chem. Soc. Rev., 2018, 47, 7239–7302 RSC.
- J. Mao, C. Ye, S. Zhang, F. Xie, R. Zeng, K. Davey, Z. Guo and S. Qiao, Toward practical lithium-ion battery recycling: adding value, tackling circularity and recycling-oriented design, Energy Environ. Sci., 2022, 15, 2732–2752 RSC.
- J. Hou, X. Ma, J. Fu, P. Vanaphuti, Z. Yao, Y. Liu, Z. Yang and Y. Wang, A green closed-loop process for selective recycling of lithium from spent lithium-ion batteries, Green Chem., 2022, 24, 7049–7060 RSC.
-
Industrial Chemistry Library, ed. G. Pistoia, J.-P. Wiaux and S. P. Wolsky, Elsevier, 2001, vol. 10, pp. 341–367 Search PubMed.
-
T. Tasaki, The Recycling Scheme for Compact Rechargeable Batteries in Japan – under the Act on the Promotion of Effective Utilization of Resources, 2014, https://www.oecd.org/environment/waste/EPR_Japan_battery.pdf Search PubMed.
- A. D. Ballantyne, J. P. Hallett, D. J. Riley, N. Shah and D. J. Payne, Lead acid battery recycling for the twenty-first century, R. Soc. Open Sci., 2018, 5, 171368 CrossRef.
-
How China Is Cornering the Lithium-Ion Cell Recycling Market, https://www.greentechmedia.com/articles/read/how-china-is-cornering-the-lithium-ion-cell-recycling-market, accessed 6 March 2023 Search PubMed.
-
M. Jacoby, It's Time to Get Serious about Recycling Lithium-Ion Batteries, Chemical & Engineering News, 2019, https://cen.acs.org/materials/energy-storage/time-serious-recycling-lithium/97/i28 Search PubMed.
- D. Werner, U. A. Peuker and T. Mütze, Recycling Chain for Spent Lithium-Ion Batteries, Metals, 2020, 10, 316 CrossRef CAS.
-
T. Tasaki, The Recycling Scheme for Compact Rechargeable Batteries in Japan – under the Act on the Promotion of Effective Utilization of Resources, 2014, https://www.oecd.org/environment/waste/EPR_Japan_battery.pdf Search PubMed.
-
zai, How to Store Lithium Batteries|Lithium Battery Storage Buildings, https://www.uschemicalstorage.com/how-to-store-lithium-batteries/ Search PubMed.
- O. Velázquez-Martínez, J. Valio, A. Santasalo-Aarnio, M. Reuter and R. Serna-Guerrero, A critical review of lithium-ion battery recycling processes from a circular economy perspective, Batteries, 2019, 5, 68 CrossRef.
- L. Gaines, The future of automotive lithium-ion battery recycling: Charting a sustainable course, Sustainable Mater. Technol., 2014, 1, 2–7 CrossRef.
-
H. E. Melin, WEF: World Economic Forum, 2018 Search PubMed.
- Q. Liao, M. Mu, S. Zhao, L. Zhang, T. Jiang, J. Ye, X. Shen and G. Zhou, Performance assessment and classification of retired lithium ion battery from electric vehicles for energy storage, Int. J. Hydrogen Energy, 2017, 42, 18817–18823 CrossRef CAS.
- L. Canals Casals, M. Rodríguez, C. Corchero and R. E. Carrillo, Evaluation of the end-of-life of electric vehicle batteries according to the state-of-health, World Electr. Veh. J., 2019, 10, 63 CrossRef.
-
E. W. Hedegor, R. L. Oliver and S. Sawahara, Battery tester and sorting apparatus, US Pat., 6781344, 2004 Search PubMed.
-
M. Li, G. Zhang, S. Nidamarthi, T. Wang and A. Srivastava, Batteries testing and sorting system and the method thereof, US Pat., 8912459, 2014 Search PubMed.
- H. Chen and J. Shen, A degradation-based sorting method for lithium-ion battery reuse, PLoS One, 2017, 12, e0185922 CrossRef PubMed.
-
The EV battery chemistry debate just got more complicated, https://www.fastmarkets.com/muthu-krishna, https://www.fastmarkets.com/insights/the-ev-battery-chemistry-debate-just-got-more-complicated Search PubMed.
- Y. Miao, P. Hynan, A. Von Jouanne and A. Yokochi, Current Li-ion battery technologies in electric vehicles and opportunities for advancements, Energies, 2019, 12, 1074 CrossRef CAS.
-
F. Lambert, All-electric Iridium E Mobil motorhome is coming to market, https://electrek.co/2018/12/10/electric-motorhome-iridium-e-mobil/ Search PubMed.
-
C. Pillot, The Rechargeable Battery Market and Main Trends 2011–2020, 2013 Search PubMed.
- H. Maleki and J. N. Howard, Internal short circuit in Li-ion cells, J. Power Sources, 2009, 191, 568–574 CrossRef CAS.
- G. Harper, R. Sommerville, E. Kendrick, L. Driscoll, P. Slater, R. Stolkin, A. Walton, P. Christensen, O. Heidrich and S. Lambert, Recycling lithium-ion batteries from electric vehicles, Nature, 2019, 575, 75–86 CrossRef CAS PubMed.
-
J. Diekmann and A. Kwade, Recycling of Lithium-Ion Batteries: the LithoRec Way, Springer, 2018 Search PubMed.
- P. Millet, R. Ngameni, S. A. Grigoriev, N. Mbemba, F. Brisset, A. Ranjbari and C. Etiévant, PEM water electrolyzers: From electrocatalysis to stack development, Int. J. Hydrogen Energy, 2010, 35, 5043–5052 CrossRef CAS.
- T. Zhang, Y. He, L. Ge, R. Fu, X. Zhang and Y. Huang, Characteristics of wet and dry crushing methods in the recycling process of spent lithium-ion batteries, J. Power Sources, 2013, 240, 766–771 CrossRef CAS.
- H. Nie, L. Xu, D. Song, J. Song, X. Shi, X. Wang, L. Zhang and Z. Yuan, LiCoO2: recycling from spent batteries and regeneration with solid state synthesis, Green Chem., 2015, 17, 1276–1280 RSC.
- J. Kang, J. Sohn, H. Chang, G. Senanayake and S. M. Shin, Preparation of cobalt oxide from concentrated cathode material of spent lithium ion batteries by hydrometallurgical method, Adv. Powder Technol., 2010, 21, 175–179 CrossRef CAS.
- J. Shaw-Stewart, A. Alvarez-Reguera, A. Greszta, J. Marco, M. Masood, R. Sommerville and E. Kendrick, Aqueous solution discharge of cylindrical lithium-ion cells, Sustainable Mater. Technol., 2019, 22, e00110 CrossRef CAS.
- M. Lu, H. Zhang, B. Wang, X. Zheng and C. Dai, The re-synthesis of LiCoO2 from spent lithium ion batteries separated by vacuum-assisted heat-treating method, Int. J. Electrochem. Sci., 2013, 8, 8201–8209 CrossRef CAS.
- C. Xu, M. Ouyang, L. Lu, X. Liu, S. Wang and X. Feng, Preliminary study on the mechanism of lithium ion battery pack under water immersion, ECS Trans., 2017, 77, 209 CrossRef CAS.
- J. Nan, D. Han and X. Zuo, Recovery of metal values from spent lithium-ion batteries with chemical deposition and solvent extraction, J. Power Sources, 2005, 152, 278–284 CrossRef CAS.
- S. Ojanen, M. Lundström, A. Santasalo-Aarnio and R. Serna-Guerrero, Challenging the concept of electrochemical discharge using salt solutions for lithium-ion batteries recycling, Waste Manage., 2018, 76, 242–249 CrossRef CAS PubMed.
- J. Li, G. Wang and Z. Xu, Generation and detection of metal ions and volatile organic compounds (VOCs) emissions from the pretreatment processes for recycling spent lithium-ion batteries, Waste Manage., 2016, 52, 221–227 CrossRef CAS.
- L. P. Yao, Q. Zeng, T. Qi and J. Li, An environmentally friendly discharge technology to pretreat spent lithium-ion batteries, J. Cleaner Prod., 2020, 245, 118820 CrossRef CAS.
- M. A. Roscher, O. Bohlen and J. Vetter, OCV hysteresis in Li-ion batteries including two-phase transition materials, Int. J. Electrochem., 2011, 2011, 984320 Search PubMed.
- R. Guo, L. Lu, M. Ouyang and X. Feng, Mechanism of the entire overdischarge process and overdischarge-induced internal short circuit in lithium-ion batteries, Sci. Rep., 2016, 6, 1–9 CrossRef PubMed.
- H. Maleki and J. N. Howard, Effects of overdischarge on performance and thermal stability of a Li-ion cell, J. Power Sources, 2006, 160, 1395–1402 CrossRef CAS.
- J. Shu, M. Shui, D. Xu, D. Wang, Y. Ren and S. Gao, A comparative study of overdischarge behaviors of cathode materials for lithium-ion batteries, J. Solid State Electrochem., 2012, 16, 819–824 CrossRef CAS.
-
F. Cardarelli, J. Dube and L. P. Avestor, Method for recycling spent lithium metal polymer rechargeable batteries and related materials, US Pat., 7192564, 2007 Search PubMed.
- G. Dorella and M. B. Mansur, A study of the separation of cobalt from spent Li-ion battery residues, J. Power Sources, 2007, 170, 210–215 CrossRef CAS.
-
T. Tanii, S. Tsuzuki, S. Honmura, T. Kamimura, K. Sasaki, M. Yabuki and K. Nishida, Method for crushing cell, US Pat., 6524737, 2003 Search PubMed.
- D. Ouyang, M. Chen, Q. Huang, J. Weng, Z. Wang and J. Wang, A review on the thermal hazards of the lithium-ion battery and the corresponding countermeasures, Appl. Sci., 2019, 9, 2483 CrossRef CAS.
- H. Pinegar and Y. R. Smith, Recycling of end-of-life lithium-ion batteries, part II: laboratory-scale research developments in mechanical, thermal, and leaching treatments, J. Sustain. Metall., 2020, 6, 142–160 CrossRef.
-
A. Vezzini, in Lithium-Ion Batteries, Elsevier, 2014, pp. 529–551 Search PubMed.
- T. Träger, B. Friedrich and R. Weyhe, Recovery concept of value metals from automotive lithium-ion batteries, Chem. Ing. Tech., 2015, 87, 1550–1557 CrossRef.
- J. Diekmann, C. Hanisch, L. Froböse, G. Schälicke, T. Loellhoeffel, A.-S. Fölster and A. Kwade, Ecological recycling of lithium-ion batteries from electric vehicles with focus on mechanical processes, J. Electrochem. Soc., 2016, 164, A6184 CrossRef.
-
F. Tedjar and J. C. Foudraz, Method for the mixed recycling of lithium-based anode batteries and cells, US Pat., 7820317, 2010 Search PubMed.
- M. Bhar, S. Ghosh, S. Krishnamurthy, K. Yalamanchili and S. K. Martha, Electrochemical Compatibility of Graphite Anode from Spent Li-Ion Batteries: Recycled via a Greener and Sustainable Approach, ACS Sustainable Chem. Eng., 2022, 10, 7515–7525 CrossRef CAS.
- M. Bhar, A. Dey, S. Ghosh, M. A. van Spronsen, V. Selvaraj, Y. Kaliprasad, S. Krishnamurthy and S. K. Martha, Plasma jet printing induced high-capacity graphite anodes for sustainable recycling of lithium-ion batteries, Carbon, 2022, 198, 401–410 CrossRef CAS.
-
T. Elwert, F. Römer, K. Schneider, Q. Hua and M. Buchert, Recycling of batteries from electric vehicles, in Behaviour of lithium-ion batteries in electric vehicles: battery health, performance, safety, and cost, 2018, pp. 289–321 Search PubMed.
- K. Wegener, W. H. Chen, F. Dietrich, K. Dröder and S. Kara, Robot assisted disassembly for the recycling of electric vehicle batteries, Procedia CIRP, 2015, 29, 716–721 CrossRef.
- K. Murari, R. Siddique and K. K. Jain, Use of waste copper slag, a sustainable material, J. Mater. Cycles Waste Manage., 2015, 17, 13–26 CrossRef CAS.
- A. I. Osman, J. K. Abu-Dahrieh, M. McLaren, F. Laffir, P. Nockemann and D. Rooney, A facile green synthetic route for the preparation of highly active γ-Al2O3 from aluminum foil waste, Sci. Rep., 2017, 7, 3593 CrossRef PubMed.
- N. A. Ghulam, M. N. Abbas and D. E. Sachit, Preparation of synthetic alumina from aluminium foil waste and investigation of its performance in the removal of RG-19 dye from its aqueous solution, Indian Chem. Eng., 2020, 62, 301–313 CrossRef CAS.
- T. Georgi-Maschler, B. Friedrich, R. Weyhe, H. Heegn and M. Rutz, Development of a recycling process for Li-ion batteries, J. Power Sources, 2012, 207, 173–182 CrossRef CAS.
- G. Granata, F. Pagnanelli, E. Moscardini, Z. Takacova, T. Havlik and L. Toro, Simultaneous recycling of nickel metal hydride, lithium ion and primary lithium batteries: accomplishment of European Guidelines by optimizing mechanical pre-treatment and solvent extraction operations, J. Power Sources, 2012, 212, 205–211 CrossRef CAS.
- X. Wang, G. Gaustad and C. W. Babbitt, Targeting high value metals in lithium-ion battery recycling via shredding and size-based separation, Waste Manage., 2016, 51, 204–213 CrossRef CAS PubMed.
- S. M. Shin, N. H. Kim, J. S. Sohn, D. H. Yang and Y. H. Kim, Development of a metal recovery process from Li-ion battery wastes, Hydrometallurgy, 2005, 79, 172–181 CrossRef CAS.
- D. Marinos and B. Mishra, An Approach to processing of lithium-ion batteries for the zero-waste recovery of materials, J. Sustain. Metall., 2015, 1, 263–274 CrossRef.
-
F. Tedjar and J. C. Foudraz, Method for the mixed recycling of lithium-based anode batteries and cells, US Pat., 7820317, 2010 Search PubMed.
-
ReCell Advanced Battery Recycling Center First Quarter Progress Report 2020 – ReCell Center, https://recellcenter.org/2020/01/01/recell-advanced-battery-recycling-center-first-quarter-progress-report-2020/, accessed 6 March 2023 Search PubMed.
- E. Gratz, Q. Sa, D. Apelian and Y. Wang, A closed loop process for recycling spent lithium ion batteries, J. Power Sources, 2014, 262, 255–262 CrossRef CAS.
- A. J. da Costa, J. F. Matos, A. M. Bernardes and I. L. Müller, Beneficiation of cobalt, copper and aluminum from wasted lithium-ion batteries by mechanical processing, Int. J. Miner. Process., 2015, 145, 77–82 CrossRef.
-
K. Environmental, Research Study on Reuse and Recycling of Batteries Employed in Electric Vehicles: the Technical, Environmental, Economic, Energy and Cost Implications of Reusing and Recycling EV Batteries, Energy API, 2019 Search PubMed.
- L. Lander, T. Cleaver, M. A. Rajaeifar, V. Nguyen-Tien, R. J. Elliott, O. Heidrich, E. Kendrick, J. S. Edge and G. Offer, Financial viability of electric vehicle lithium-ion battery recycling, Iscience, 2021, 24, 102787 CrossRef PubMed.
- R. Sommerville, J. Shaw-Stewart, V. Goodship, N. Rowson and E. Kendrick, A review of physical processes used in the safe recycling of lithium ion batteries, Sustainable Mater. Technol., 2020, 25, e00197 CrossRef CAS.
- D. A. Bertuol, C. Toniasso, B. M. Jiménez, L. Meili, G. L. Dotto, E. H. Tanabe and M. L. Aguiar, Application of spouted bed elutriation in the recycling of lithium ion batteries, J. Power Sources, 2015, 275, 627–632 CrossRef CAS.
- J. Cui and E. Forssberg, Mechanical recycling of waste electric and electronic equipment: a review, J. Hazard. Mater., 2003, 99, 243–263 CrossRef CAS PubMed.
- R. Zhan, Z. Oldenburg and L. Pan, Recovery of active cathode materials from lithium-ion batteries using froth flotation, Sustainable Mater. Technol., 2018, 17, e00062 CrossRef CAS.
- Y. He, T. Zhang, F. Wang, G. Zhang, W. Zhang and J. Wang, Recovery of LiCoO2 and graphite from spent lithium-ion batteries by Fenton reagent-assisted flotation, J. Cleaner Prod., 2017, 143, 319–325 CrossRef CAS.
- J. Liu, H. Wang, T. Hu, X. Bai, S. Wang, W. Xie, J. Hao and Y. He, Recovery of LiCoO2 and graphite from spent lithium-ion batteries by cryogenic grinding and froth flotation, Miner. Eng., 2020, 148, 106223 CrossRef CAS.
- J. Yu, Y. He, H. Li, W. Xie and T. Zhang, Effect of the secondary product of semisolid phase Fenton on the flotability of electrode material from spent lithium-ion battery, Powder Technol., 2017, 315, 139–146 CrossRef CAS.
- J. Yu, Y. He, Z. Ge, H. Li, W. Xie and S. Wang, A promising physical method for recovery of LiCoO2 and graphite from spent lithium-ion batteries: Grinding flotation, Sep. Purif. Technol., 2018, 190, 45–52 CrossRef CAS.
- F. Wang, T. Zhang, Y. He, Y. Zhao, S. Wang, G. Zhang, Y. Zhang and Y. Feng, Recovery of valuable materials from spent lithium-ion batteries by mechanical separation and thermal treatment, J. Cleaner Prod., 2018, 185, 646–652 CrossRef CAS.
- G. Zhang, Z. Du, Y. He, H. Wang, W. Xie and T. Zhang, A sustainable process for the recovery of anode and cathode materials derived from spent lithium-ion batteries, Sustainability, 2019, 11, 2363 CrossRef CAS.
- D. Yu, Z. Huang, B. Makuza, X. Guo and Q. Tian, Pretreatment options for the recycling of spent lithium-ion batteries: A comprehensive review, Miner. Eng., 2021, 173, 107218 CrossRef CAS.
- T. Zhang, Y. He, F. Wang, H. Li, C. Duan and C. Wu, Surface analysis of cobalt-enriched crushed products of spent lithium-ion batteries by X-ray photoelectron spectroscopy, Sep. Purif. Technol., 2014, 138, 21–27 CrossRef CAS.
-
J. Schmitt, H. Haupt, M. Kurrat and A. Raatz, in 2011 15th International Conference on Advanced Robotics (ICAR), IEEE, 2011, pp. 291–297 Search PubMed.
- L. Li, P. Zheng, T. Yang, R. Sturges, M. W. Ellis and Z. Li, Disassembly automation for recycling end-of-life lithium-ion pouch cells, JOM, 2019, 71, 4457–4464 CrossRef CAS.
- J. R. Duflou, G. Seliger, S. Kara, Y. Umeda, A. Ometto and B. Willems, Efficiency and feasibility of product disassembly: a case-based study, CIRP Ann., 2008, 57, 583–600 CrossRef.
-
C. Herrmann, A. Raatz, S. Andrew and J. Schmitt, in Advanced Materials Research, Trans Tech Publ., 2014, vol. 907, pp. 391–401 Search PubMed.
- R. Gerbers, M. Mücke, F. Dietrich and K. Dröder, Simplifying Robot Tools by Taking Advantage of Sensor Integration in Human Collaboration Robots, Procedia CIRP, 2016, 44, 287–292 CrossRef.
-
A. Hoffmann, A. Schierl, A. Angerer, M. Stüben, M. Vistein and W. Reif, Robot Collision Avoidance Using an Environment Model for Capacitive Sensors, Paper accepted for workshop on planning, control, and sensing for safe human-robot interaction, Seattle, USA, 2015 Search PubMed.
- P. Gil, J. Pomares, S. T. P. C. Diaz, F. Candelas and F. Torres, Flexible multi-sensorial system for automatic disassembly using cooperative robots, Int. J. Comput. Integr. Manuf., 2007, 20, 757–772 Search PubMed.
- Y. Bai, N. Muralidharan, Y.-K. Sun, S. Passerini, M. S. Whittingham and I. Belharouak, Energy and environmental aspects in recycling lithium-ion batteries: concept of battery identity global passport, Mater. Today, 2020, 41, 304–315 CrossRef CAS.
- C. Hanisch, T. Loellhoeffel, J. Diekmann, K. J. Markley, W. Haselrieder and A. Kwade, Recycling of lithium-ion batteries: a novel method to separate coating and foil of electrodes, J. Cleaner Prod., 2015, 108, 301–311 CrossRef CAS.
- M. Contestabile, S. Panero and B. J. Scrosati, A laboratory-scale lithium-ion battery recycling process, J. Power Sources, 2001, 92, 65–69 CrossRef CAS.
- L.-P. He, S.-Y. Sun, X.-F. Song and J.-G. Yu, Recovery of cathode materials and Al from spent lithium-ion batteries by ultrasonic cleaning, Waste Manage., 2015, 46, 523–528 CrossRef CAS PubMed.
- C. Lei, I. Aldous, J. M. Hartley, D. L. Thompson, S. Scott, R. Hanson, P. A. Anderson, E. Kendrick, R. Sommerville and K. S. Ryder, Lithium ion battery recycling using high-intensity ultrasonication, Green Chem., 2021, 23, 4710–4715 RSC.
- L. Yao, Y. Feng and G. Xi, A new method for the synthesis of LiNi1/3Co1/3Mn1/3O2 from waste lithium ion batteries, RSC Adv., 2015, 5, 44107–44114 RSC.
- O. E. Bankole, C. Gong and L. Lei, Battery recycling technologies: recycling waste lithium ion batteries with the impact on the environment in-view, J. Environ. Prot. Ecol., 2013, 10, 14–28 CrossRef.
- X. Zhang, Y. Xie, H. Cao, F. Nawaz and Y. Zhang, A novel process for recycling and resynthesizing LiNi1/3Co1/3Mn1/3O2 from the cathode scraps intended for lithium-ion batteries, Waste Manage., 2014, 34, 1715–1724 CrossRef.
- Y. Bai, R. Essehli, C. J. Jafta, K. M. Livingston and I. Belharouak, Recovery of cathode materials and aluminum foil using a green solvent, ACS Sustainable Chem. Eng., 2021, 9, 6048–6055 CrossRef CAS.
- D. Song, X. Wang, E. Zhou, P. Hou, F. Guo and L. Zhang, Recovery and heat treatment of the Li(Ni1/3Co1/3Mn1/3)O2 cathode scrap material for lithium ion battery, J. Power Sources, 2013, 232, 348–352 CrossRef CAS.
- D. A. Ferreira, L. M. Z. Prados, D. Majuste and M. B. Mansur, Hydrometallurgical separation of aluminium, cobalt, copper and lithium from spent Li-ion batteries, J. Power Sources, 2009, 187, 238–246 CrossRef CAS.
- L. Chen, X. Tang, Y. Zhang, L. Li, Z. Zeng and Y. Zhang, Process for the recovery of cobalt oxalate from spent lithium-ion batteries, Hydrometallurgy, 2011, 108, 80–86 CrossRef CAS.
- L. Sun and K. Qiu, Vacuum pyrolysis and hydrometallurgical process for the recovery of valuable metals from spent lithium-ion batteries, J. Hazard. Mater., 2011, 194, 378–384 CrossRef CAS PubMed.
- Y. Yang, G. Huang, S. Xu, Y. He and X. Liu, Thermal treatment process for the recovery of valuable metals from spent lithium-ion batteries, Hydrometallurgy, 2016, 165, 390–396 CrossRef CAS.
- P. Zhu, E. H. Driscoll, B. Dong, R. Sommerville, A. Zorin, P. R. Slater and E. Kendrick, Direct reuse of aluminium and copper current collectors from spent lithium-ion batteries, Green Chem., 2023, 25(9), 3503–3514 RSC.
- K. Gu, J. Chang, X. Mao, H. Zeng, W. Qin and J. Han, Efficient separation of cathode materials and Al foils from spent lithium batteries with glycerol heating: a green and unconventional way, J. Cleaner Prod., 2022, 369, 133270 CrossRef CAS.
- X. Zeng and J. Li, Innovative application of ionic liquid to separate Al and cathode materials from spent high-power lithium-ion batteries, J. Hazard. Mater., 2014, 271, 50–56 CrossRef CAS PubMed.
- M. Wang, Q. Tan, L. Liu and J. Li, A low-toxicity and high-efficiency deep eutectic solvent for the separation of aluminum foil and cathode materials from spent lithium-ion batteries, J. Hazard. Mater., 2019, 380, 120846 CrossRef CAS PubMed.
- M. Wang, Q. Tan, L. Liu and J. Li, A facile, environmentally friendly, and low-temperature approach for decomposition of polyvinylidene fluoride from the cathode electrode of spent lithium-ion batteries, ACS Sustainable Chem. Eng., 2019, 7, 12799–12806 CrossRef CAS.
- W. Lv, Z. Wang, H. Cao, Y. Sun, Y. Zhang and Z. Sun, A Critical Review and Analysis on the Recycling of Spent Lithium-Ion Batteries, ACS Sustainable Chem. Eng., 2018, 6, 1504–1521 CrossRef CAS.
- A. Sonoc, J. Jeswiet and V. K. Soo, Opportunities to improve recycling of automotive lithium ion batteries, Procedia CIRP, 2015, 29, 752–757 CrossRef.
-
M. N. Akram and W. Abdul-Kader, EV Battery Recycling and its Impact on Society, Proceedings of the 5th NA International Conference on Industrial Engineering and Operations Management, Detroit, Michigan, USA, 2020 Search PubMed.
-
Y. Haga, K. Saito and K. Hatano, in Materials Processing Fundamentals 2018, ed. G. Lambotte, J. Lee, A. Allanore and S. Wagstaff, Springer International Publishing, Cham, 2018, pp. 143–147 Search PubMed.
Footnote |
† Equal contributing authors. |
|
This journal is © The Royal Society of Chemistry 2023 |