DOI:
10.1039/D2PY01470J
(Paper)
Polym. Chem., 2023,
14, 492-499
Synthesis of fluorescent poly(silyl indole)s via borane-catalyzed C–H silylation of indoles†
Received
23rd November 2022
, Accepted 16th December 2022
First published on 21st December 2022
Abstract
The development of a new polymerization strategy is essential for the design and synthesis of polymers with new frameworks, since it would effectively expand the diversity of the structures and properties of polymers. Here we report a metal-free B(C6F5)3-catalyzed step-growth polymerization of indoles and hydrosilanes through regioselective dehydrogenation silylation of indoles. A series of indole and silyl-based monomers were designed and synthesized for the preparation of linear poly(silyl indole)s with good solubility through AA + BB-type or AB-type polymerization. In addition, we employed trisilane and biindole as building blocks to construct hyperbranched polymers with rigid structures. All the obtained polymers were fully structurally characterized and observed to fluorescently emit strong bright blue-violet light in both solution and in the solid state under UV light. These poly(silyl indole)s with their novel type of structure demonstrated very promising application potentials in organic optoelectronic materials.
Introduction
Introducing small-molecule reactions to polymer synthesis has been a long-standing research topic in polymer science,1–6 as the incorporated heteroatom7–12 or heterocycle13–19 can provide the obtained polymer not only with a new framework but also useful properties.20–22 In recent years, the family of polymers with silicon atoms in their main chains, such as polysiloxanes, polycarbosilanes and polysilanes, has emerged as one of the most important families of functionalized polymeric materials. They are widely used as oils, rubbers, silicone resins, heat-resistant materials and optoelectronic materials, because of their excellent thermal, electronic and optical properties.23–26 Progress has been made towards the application of organocatalytic step-growth polymerizations in the synthesis of organosilicon polymers, with such reactions including dehydrogenative coupling,27–32 hydrosilylation of silanes with ketones, alkenes or alkynes,33–37 the Piers–Rubinsztajn reaction38,39 and others.40,41 Using the indole skeleton to construct polymeric materials has also attracted intense attention. It has done so due to the wide applications of polyindoles and their derivatives in sensors, supercapacitors and electrocatalysts.42–44 Electropolymerization and chemical oxidation in the presence of metal catalysts are common strategies for the synthesis of such polyheterocycles.45–47 So far, there are only a few reports on the preparation of polyindoles from organic reactions.48–50
Recently, by using B(C6F5)3 as a catalyst, our group realized the convergent disproportionation of indoles into a series of C3-silylated (or borylated) indoles in up to 99% yields.51,52 Being a powerful metal-free catalyst, B(C6F5)3 has been shown to exhibit a long-life catalytic performance, as evidenced by the observation that both catalytic activity and excellent product yields can be maintained even after 10 sequential additions of starting materials. Furthermore, B(C6F5)3 has been employed to achieve highly regioselective C-3 functionalization of indoles and complete reduction of quinoline at room temperature (RT). Mechanistic studies suggested that by sharing ammonium hydridoborate, C-3 functionalization of an indole and complete reduction of a quinoline would each consume a product generated from the other reaction such that they can mutually promote each other, thus representing an example of mutualism applied in organic synthetic chemistry.53 Moreover, the employment of Al(C6F5)3, a congener of B(C6F5)3 but with stronger Lewis acidity, enabled the switchable C–H silylation of indoles through an Al(C6F5)3-based thermally induced frustrated Lewis pair (FLP). Note that all these methods produced C3-silylated indoles in excellent yields, specifically up to 99%, which used to be challenging to accomplish.54
The introduction of indole groups to silicon-containing polymeric frameworks is expected to produce more reactive chemical bonds, which can be post-functionalized with suitable reagents. Furthermore, they may be made luminescent due to the presence of silicon and indole-containing functional groups alternating within the polymer backbones. It is envisioned that the polymers containing both indole and silicon may exhibit properties superior to those of the existing polyindoles or silicon-containing polymers. In combination with the advantages offered by Lewis-acid-catalyzed reactions, such as high catalytic efficiency, mild reaction conditions and no requirement of additives, we expected that such Lewis-acid-catalyzed reactions can be employed as template reactions for post-polymerization modification55 and to construct poly(silyl indole)s. Therefore, we focused our research efforts on the application of silylation of indoles to polymer synthesis. In this context, a series of biindoles, bifunctional monomers bearing both silyl and indole groups, and trisilyl monomers were successfully designed and synthesized. B(C6F5)3-catalyzed dehydrosilylation of these monomers afforded a wide variety of linear and cyclic model compounds and poly(silyl indole)s. To the best of our knowledge, this was the first time that both indole and silicon-containing groups were simultaneously introduced to the backbone of a polymer. The obtained polymers were structurally characterized using Fourier-transform infrared spectroscopy (FT-IR), nuclear magnetic resonance spectroscopy (NMR) and gel permeation chromatography (GPC). Thermogravimetry analysis (TGA) revealed the high thermal stability levels of the poly(silyl indole)s. Furthermore, these poly(silyl indole)s were observed to emit blue violet light upon being photoexcited, and their photoluminescence behaviors were investigated by carrying out UV-Vis and fluorescence spectroscopy analyses as well.
Results and discussion
Monomer synthesis
Various types of monomers, including biindoles, bifunctional monomers bearing both silyl and indole groups, and trisilanes, were designed and prepared in good to high yields (see ESI† for more details about the syntheses). The biindole monomers (1a–1c) were prepared by carrying out Suzuki–Miyaura coupling reactions between bromo-substituted N-methylindole and indolylboronic acids (Scheme 1A).56,57 AB-type monomers bearing both silyl and indole groups (2a–2d) were synthesized by carrying out Suzuki–Miyaura cross-coupling reactions between indolylboronic acids and 1-bromo-4-iodobenzene (or 1-bromo-3-iodobenzene) followed by lithiation of indoles bearing the 4-bromophenyl (or 3-bromophenyl) group and then alkylation with Me2SiClH (Scheme 1B). Similarly, the trisilane 3b was prepared from 1,3,5-tris(4-bromophenyl)benzene and 1,4-bis(dimethylsilyl)benzene by first subjecting these starting materials to lithiation and then subjecting the resulting species to alkylation (Scheme 1C).
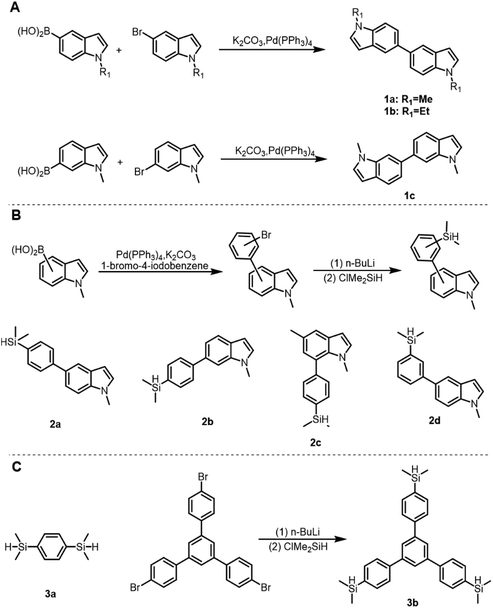 |
| Scheme 1 Structures of and synthetic routes to (A) biindoles, (B) bifunctional monomers and (C) trisilane. | |
Model reaction and polymerization
We also set out to investigate the possibility of achieving a C–H silylation of N-methylindole in a polymer synthesis in the presence of 1 mol% B(C6F5)3. Reacting 1,1′-diethyl-5,5′-biindole (1a) with PhMe2SiH in a 1
:
2 ratio at 120 °C produced model compound 1 (MC1) as a white solid in an 84% yield, under the previously reported optimal conditions51 (Scheme 2A). The molecular structure of the product was confirmed using single-crystal X-ray diffraction (Fig. 1A). Under the same reaction conditions, the C–H silylation of 1-methyl-5-phenyl-indole and 1,4-bis(dimethylsilyl)benzene (3a) furnished model compound 2 (MC2) in 82% yield (Scheme 2B).
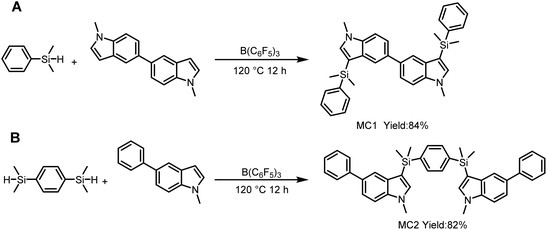 |
| Scheme 2 Synthetic routes to (A) MC1 and (B) MC2. | |
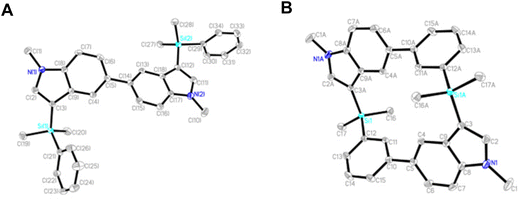 |
| Fig. 1 Depictions of X-ray crystal structures of (A) MC1 and (B) MC3, with hydrogen atoms omitted for clarity and ellipsoids drawn at 50% probability. | |
Next, we employed this metal-free catalyst system and 3a as a common comonomer to expand the scope of biindoles produced. Taking into consideration the characteristics of both the polymerization and organic reaction, we further optimized the reaction conditions for the polymerization. Using 5 mol% catalyst and a prolonged reaction time (72 h), polymerization of 1a and 3a in toluene at 120 °C produced polymer 1 (P1) with a weight-average molecular weight (Mw) of 7.1 kg mol−1 in 82% yield (Table 1, entry 1). Switching to biindole 1b or 1c, polymerization still proceeded smoothly, furnishing polymer 2 (P2) (5.7 kg mol−1) or polymer 3 (P3) (3.9 kg mol−1), respectively (Table 1, entries 2 and 3). In general, step-growth polymerization of monomers bearing bifunctional groups (AB-polymerization) provides a powerful strategy for AA + BB polymerization. AA + BB polymerization, however, is generally notoriously sensitive to monomer purity and causes a stoichiometric imbalance. A monomer composed of both indole and silyl groups was expected to eliminate the requirement of stoichiometric balance and simplify the polymerization procedures. The employment of indoles with the 4-(dimethylsilyl)phenyl group at the C5, C6 or C7 position (2a–2c) yielded the corresponding polymers (P4, 3.9 kg mol−1; P5, 4.8 kg mol−1; P6, 80%), respectively (Table 1, entries 4–6). When 5-(3-(dimethylsilyl)phenyl)-1-methyl-indole (2d) was used, near-quantitative conversion of monomers to only oligomers occurred (Table 1, entry 7). Interestingly, after purification and precipitation, model compound 3 (MC3), a cyclic dimer, was identified to be the major product, which was structurally characterized from NMR and high-resolution mass spectrometry (HRMS) analyses. Its solid-state structure was confirmed from a single-crystal X-ray diffraction study (Fig. 1B). The concentration of 2d was found to have a significant impact on the condensation (Table S1†). When the concentration of 2d was increased from 0.1 to 0.2, 0.4 and 0.6 M, the yield of MC3 gradually decreased from 75% to 70%, 56% and 34%, respectively. Further increasing the concentration of 2d resulted in precipitation, further highlighting the difficulty in synthesizing the polymers. Note that employment of monomers with certain structures and at low concentration tended to result in cyclization rather than intermolecular polymerization. As shown in Table S1,† when polymerization was performed using a monomer concentration of 0.1 M, we obtained cyclic dimer in up to 75% yield, demonstrating that we may easily obtain diverse products through such condensation.
Table 1 Structures of the obtained polymers and polymerization results.a
Hyperbranched polymers, constituting an important class of functional polymeric materials, are widely applied in various areas such as coatings, drug delivery carriers, biomaterials, nanomaterials and so on.58–61 In order to obtain silicon-containing polymers displaying diverse topologies, trisilane 3b was synthesized and copolymerized with biindole 1a for the synthesis of hyperbranched polymers. The polymerization was performed by reacting 1a (0.5 mmol) with 3b (0.33 mmol) in the presence of 5 mol% B(C6F5)3 catalyst at 120 °C, and furnished P7 with a moderate Mw value of 8.1 kg mol−1 in 82% yield. Due to its rigid structure, the obtained transparent block solid (P7) was partially soluble in common organic solvents such as tetrahydrofuran, dichloromethane, chloroform, benzene, toluene, and N,N-dimethylformamide.
Structural characterization and thermal stability
Considering the similar structures of these polymers, P1 was selected as an example and structurally characterized using NMR spectroscopy. Based on a comparison of the 1H NMR spectra (shown in Fig. 2) of P1 (Fig. 2A), model compound MC2 (Fig. 2B), monomers 1a (Fig. 2C) and 3a (Fig. 2D), the characteristic peak centered at δ 4.46 ppm was assigned to the hydrosilane protons of monomer 3a; this peak disappeared in the spectra of MC2 and P1. The doublet centered at δ 0.38 ppm and attributed to the silyl methyl group protons of 3a became a singlet and shifted to δ 0.64 for MC2 and 0.66 ppm for P1. The peak at δ 6.57 ppm, corresponding to the protons at the C3 position of the indole ring in 1a, completely disappeared after production of MC2 and that of P1. Meanwhile, the characteristic [N-CH3] resonance peak at δ 3.86 ppm in 1a shifted toward high field, reaching 3.83 ppm for MC2 and 3.71 ppm for P1. In combination with the polymeric nature as revealed by the typically broad peaks observed for P1, these results clearly demonstrated that successful copolymerization occurred between 1a and 3a.
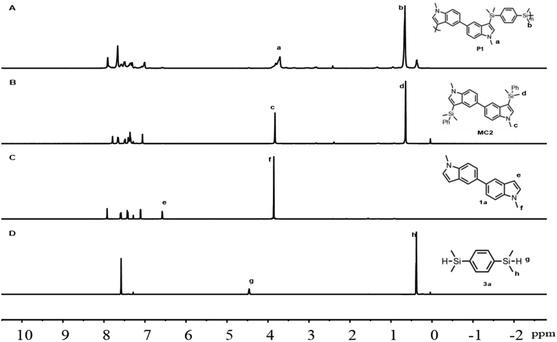 |
| Fig. 2
1H NMR spectra of (A) P1, (B) MC2, (C) 1a and (D) 3a in CDCl3. | |
We also analyzed the FT-IR spectrum of P1 and compared it with those of monomers 1a and 3a (Fig. 3A–C). As seen in Fig. 3A, the characteristic peak of 3a associated with Si–H stretching vibration was observed at 2121 cm−1; this peak almost disappeared in the spectrum of P1 (Fig. 3B), indicating the consumption of the Si–H group of the monomers and occurrence of polymerization. Similar results were observed in the FT-IR spectrum of P4 (Fig. S2†). Furthermore, thermogravimetric analysis (TGA) of the obtained silicon-containing polymers showed the linear polymers displaying high thermal stability (Fig. 3D). There was only a less than 5% weight loss in nitrogen (Td5) below 205 °C for P1–P6. Note that P1, P2 and P3, composed of AA + BB monomers, each exhibited two stages in the thermal decomposition process. In sharp contrast, P4, P5 and P6 derived from AB-polymerization each showed only one step in the thermal decomposition process and the major weight loss occurred in the range 350–550 °C. P7 underwent a more complex thermal decomposition process, exhibiting a relatively low Td5 value of 171 °C but high residual mass of 47% at 800 °C.
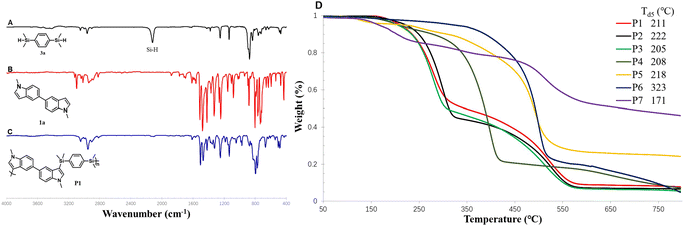 |
| Fig. 3 FT-IR spectra of (A) 3a, (B) 1a and (C) P1. (D) Thermogravimetric analysis (TGA) results for the obtained polymers P1–P7. | |
Photophysical properties
Due to the application of the indole derivatives as fluorescent materials, we further investigated the optical properties of the obtained polymers. These polymers were all purple blue solids, and able to fluorescently emit strong bright blue-violet light both in solution and in the solid state when exposed to UV light (Fig. 4A). To get more information about the fluorescence properties of this conjugated alternating copolymer, we compared the UV-Vis absorption spectra of diluted P1–P7 THF solutions (Fig. 4A). It turned out that they all showed strong absorption tailing to 400 nm due to their conjugated units. Fluorescence spectra shown in Fig. 4B revealed that the maximum emission wavelength (λmax) values of the polymers were between 360 and 380 nm, attributed to the intramolecular charge transfer between indole donor and silane acceptor units in the polymeric backbone of poly(silyl indole)s. All these results demonstrated the very promising application prospects of these polymers with novel polymeric frameworks in organic optoelectronic materials.
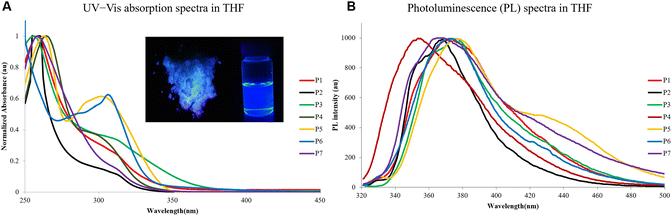 |
| Fig. 4 (A) UV-Vis absorption spectra of diluted P1–P7 THF solutions. (B) Photoluminescence (PL) spectra of diluted P1–P7 THF solutions. Inset: photographs of fluorescent P1 in the solid state (left) and in THF solution (right) taken under 365 nm-wavelength UV irradiation. | |
Conclusions
In summary, we developed a strategy involving metal-free B(C6F5)3-catalyzed step-growth polymerization of indoles and hydrosilanes through highly regioselective silylation of indoles. A broad variety of biindoles and silyl substituted indoles were designed, synthesized and utilized as monomers. In model reactions, both linear and cyclic oligomers can be obtained in high yields by selecting monomers with appropriate structures and adjusting the monomer concentration. Linear poly(silyl indole)s with excellent solubility in common organic solvents can be obtained, specifically through AA + BB polymerization for P1–P3 and AB polymerization for P4–P6. Hyperbranched polymers can also be prepared from copolymerization of trisilane and biindole. These polymers were fully structurally characterized from the results of FT-IR spectroscopy, NMR spectroscopy, GPC and TGA studies. Moreover, the obtained poly(silyl indole)s all exhibited strong bright blue-violet fluorescence in both solution and solid states. Our strategy provided a simple and efficient way to synthesize poly (silyl indole)s with a novel polymeric framework showing promising application prospects in the field of organic optoelectronic materials. Further investigation of the practical applications of these poly(silyl indole)s is underway.
Conflicts of interest
There are no conflicts to declare.
Acknowledgements
This work was supported by the National Natural Science Foundation of China (Grant no. 21871107, 22225104, 22071077 and 21975102).
References
- A. Bossion, K. V. Heifferon, L. Meabe, N. Zivic, D. Taton, J. L. Hedrick, T. E. Long and H. Sardon, Opportunities for organocatalysis in polymer synthesis via step-growth methods, Prog. Polym. Sci., 2019, 90, 164–210 CrossRef CAS.
- Y. Liu, A. Qin and B. Z. Tang, Polymerizations based on triple-bond building blocks, Prog. Polym. Sci., 2018, 78, 92–138 CrossRef CAS.
- B. Carsten, F. He, H. J. Son, T. Xu and L. Yu, Stille polycondensation for synthesis of functional materials, Chem. Rev., 2011, 111, 1493–1528 CrossRef CAS PubMed.
- A. Qin, J. W. Y. Lam and B. Z. Tang, Click polymerization, Chem. Soc. Rev., 2010, 39, 2522–2544 RSC.
- M. K. Kiesewetter, E. J. Shin, J. L. Hedrick and R. M. Waymouth, Organocatalysis: opportunities and challenges for polymer synthesis, Macromolecules, 2010, 43, 2093–2107 CrossRef CAS.
- J. Sakamoto, M. Rehahn, G. Wegner and A. D. Schlueter, Suzuki Polycondensation: Polyarylenes ála Carte, Macromol. Rapid Commun., 2009, 30, 653–687 CrossRef CAS.
- J. Zhang, Q. Zang, F. Yang, H. Zhang, J. Z. Sun and B. Tang, Sulfur conversion to multifunctional poly(O-thiocarbamate)s through multicomponent polymerizations of sulfur, diols, and diisocyanides, J. Am. Chem. Soc., 2021, 143, 3944–3950 CrossRef CAS.
- O. Daglar, E. Cakmakci, U. S. Gunay, G. Hizal, U. Tunca and H. Durmaz, A straightforward method for fluorinated polythioether synthesis, Macromolecules, 2020, 53, 2965–2975 CrossRef CAS.
- M. Uchiyama, M. Osumi, K. Satoh and M. Kamigaito, Thiol–ene cationic and radical reactions: cyclization, step–growth, and concurrent polymerizations for thioacetal and thioether units, Angew. Chem., Int. Ed., 2020, 59, 6832–6838 CrossRef CAS.
- J. Wang, Y. Chen, C. Ye, A. Qin and B. Tang, C(sp3)–H polyamination of internal alkynes toward regio- and stereoregular functional poly(allylic tertiary amine)s, Macromolecules, 2020, 53, 3358–3369 CrossRef CAS.
- Y. J. Jang, S. H. Hwang and T. L. Choi, Iridium-catalyzed direct C-H amidation polymerization: step-growth polymerization by C-N bond formation via C-H activationto give fluorescent polysulfonamides, Angew. Chem., Int. Ed., 2017, 56, 14474–14478 CrossRef CAS.
- W. J. Chung, J. J. Griebel, E. T. Kim, H. Yoon, A. G. Simmonds, H. J. Ji, P. T. Dirlam, R. S. Glass, J. J. Wie, N. A. Nguyen, B. W. Guralnick, J. Park, Á. Somogyi, P. Theato, M. E. Mackay, Y.-E. Sung, K. Char and J. Pyun, The use of elemental sulfur as an alternative feedstock for polymeric materials, Nat. Chem., 2013, 5, 518–524 CrossRef CAS PubMed.
- Y. B. Hu, N. Yan, X. L. Liu, L. Y. Pei, C. B. Ye, W. X. Wang, W. Y. L. Jacky and B. Z. Tang, In Situ Generation of N-Heteroaromatic Polymers: Metal-Free Multicomponent Polymerization for Photopatterning, Morphological Imaging, and Cr(VI) Sensing, CCS Chem., 2022, 4, 2308–2320 CrossRef CAS.
- Y. S. Wu, M. M. Ding, J. Wang, B. Zhao, Z. Q. Wu, P. Zhao, D. L. Tian, Y. Ding and A. G. Hu, Controlled Step-Growth Polymerization, CCS Chem., 2020, 2, 64–70 CrossRef.
- R. Dong, Q. Chen, X. Cai, Q. Zhang and Z. Liu, Synthesis of fused conjugated polymers containing imidazo[2,1-b]thiazole units by multicomponent one-pot polymerization, Polym. Chem., 2020, 11, 5200–5206 RSC.
- Z. Zhang, D. Yuan, X. Liu, M. J. Kim, A. Nashchadin, V. Sharapov and L. Yu, BODIPY-Containing polymers with ultralow band gaps and ambipolar charge mobilities, Macromolecules, 2020, 53, 2014–2020 CrossRef CAS.
- T. Han, H. Deng, Z. Qiu, Z. Zhao, H. Zhang, H. Zou, N. L. C. Leung, G. Shan, M. R. J. Elsegood, J. W. Y. Lam and B. Tang, Facile multicomponent polymerizations toward unconventional luminescent polymers with readily openable small heterocycles, J. Am. Chem. Soc., 2018, 140, 5588–5598 CrossRef CAS.
- W. Fu, L. Dong, J. Shi, B. Tong, Z. Cai, J. Zhi and Y. Dong, Synthesis of polyquinolines via one-pot polymerization of alkyne, aldehyde, and aniline under metal-free catalysis and their properties, Macromolecules, 2018, 51, 3254–3263 CrossRef CAS.
- K. Kawashima, T. Fukuhara, Y. Suda, Y. Suzuki, T. Koganezawa, H. Yoshida, H. Ohkita, I. Osaka and K. Takimiya, Implication of fluorine atom on electronic properties, ordering structures, and photovoltaic performance in naphthobisthiadiazole-based semiconducting polymers, J. Am. Chem. Soc., 2016, 138, 10265–10275 CrossRef CAS.
- X. Yin, J. Liu and F. Jakle, Electron-Deficient Conjugated Materials via p–p* Conjugation with Boron: Extending Monomers to Oligomers, Macrocycles, and Polymers, Chem. – Eur. J., 2021, 27, 2973–2986 CrossRef CAS.
- A. M. Priegert, B. W. Rawe, S. C. Serin and D. P. Gates, Polymers and the p-block elements, Chem. Soc. Rev., 2016, 45, 922–953 RSC.
- X. Wang, G. Sun, P. Routh, D.-H. Kim, W. Huang and P. Chen, Heteroatom-doped graphene materials: syntheses, properties and applications, Chem. Soc. Rev., 2014, 43, 7067–7098 RSC.
-
A. Feigl, A. Bockholt, J. Weis and B. Rieger, Adv. Polym. Sci., in Silicon Polymers, ed. A. M. Muzafarov, Springer, Berlin/Heidelberg, 2011, vol. 235, pp. 1–31 Search PubMed.
-
R. G. Jones, W. Ando and J. Chojnowski, Silicon-Containing polymers, Kluwer, Dordrecht, 2000 Search PubMed.
-
M. A. Brook, Silicon in organic, organometallic, and polymer chemistry, Wiley-Interscience, New York, 2000 Search PubMed.
- M. Birot, J.-P. Pillot and J. Dunogues, Comprehensive chemistry of polycarbosilanes, polysilazanes, and polycarbosilazanes as precursors of ceramics, Chem. Rev., 1995, 95, 1443–1477 CrossRef CAS.
- H. Fouilloux, M. N. Rager, P. Ríos, S. Conejero and C. M. Thomas, Highly efficient synthesis of poly(silylether)s: Access to degradable polymers from renewable resources, Angew. Chem., Int. Ed., 2022, 134(7), e202113443, DOI:10.1002/anie.202113443.
- C. Li, L. Wang, M. Wang, B. Liu, X. Liu and D. Cui, Step-Growth coordination polymerization of 5-hydroxymethyl furfural with dihydrosilanes: Synergistic catalysis using heteroscopionate zinc hydride and B(C6F5)3, Angew. Chem., Int. Ed., 2019, 58, 11434–11438 CrossRef CAS.
- L. J. Morris, G. R. Whittell, J. C. Eloi, M. F. Mahon, F. Marken, I. Manners and M. S. Hill, Ferrocene-Containing polycarbosilazanes via the alkaline-earth-catalyzed dehydrocoupling of Silanes and Amines, Organometallics, 2019, 38, 3629–3648 CrossRef CAS.
- X. Y. Zhai, S. B. Hu, L. Shi and Y. G. Zhou, Synthesis of poly (silyl ethers) via iridium-catalyzed dehydrocoupling polymerization, Organometallics, 2018, 37, 2342–2347 CrossRef CAS.
- C. Bellini, C. Orione, J. F. Carpentier and Y. Sarazin, Tailored cyclic and linear polycarbosilazanes by Barium-Catalyzed N−H/H−Si dehydrocoupling reactions, Angew. Chem., Int. Ed., 2016, 55, 3744–3748 CrossRef CAS.
- M. Itazaki, K. Ueda and H. Nakazawa, Iron-Catalyzed dehydrogenative coupling of tertiary silanes, Angew. Chem., Int. Ed., 2009, 48, 3313–3316 CrossRef CAS PubMed.
- Z. Huang, Z. Chen, Y. Jiang, N. Li, S. Yang, G. Wang and X. Pan, Metal-Free hydrosilylation polymerization by merging photoredox and hydrogen atom transfer catalysis, J. Am. Chem. Soc., 2021, 143, 19167–19177 CrossRef CAS PubMed.
- W. Chen, X. Liu, J. Kong, J. Li, Y. Chen and C. Cui, Rare-Earth-Catalyzed selective synthesis of linear hydridopolycarbosilanes and their functionalization, Macromolecules, 2021, 54, 673–678 CrossRef CAS.
- X. Wang, X. Zhai, B. Wu, Y. Bai and Y. Zhou, Synthesis of chiral poly(silyl ether)s via CuH-catalyzed asymmetric hydrosilylation polymerization of diketones with silanes, ACS Macro Lett., 2020, 9, 969–973 CrossRef CAS.
- D. W. Kim, S. Joung, J. G. Kim and S. Chang, Metal-Free hydrosilylation polymerization by borane catalyst, Angew. Chem., Int. Ed., 2015, 54, 14805–14809 CrossRef CAS.
- P. Lu, J. W. Y. Lam, J. Liu, C. K. W. Jim, W. Yuan, C. Y. K. Chan, N. Xie, Q. Hu, K. K. L. Cheuk and B. Tang, Regioselective alkyne polyhydrosilylation: synthesis and photonic properties of poly(silylenevinylene)s, Macromolecules, 2011, 44, 5977–5986 CrossRef CAS.
- C. S. Sample, S. H. Lee, M. W. Bates, J. M. Ren, J. Lawrence, V. Lensch, J. A. Gerbec, C. M. Bates, S. Li and C. J. Hawke, Metal-Free synthesis of poly(silyl ether)s under ambient conditions, Macromolecules, 2019, 52, 1993–1999 CrossRef CAS.
- M. A. Brook, New control over silicone synthesis using SiH chemistry: The Piers–Rubinsztajn reaction, Chem. – Eur. J., 2018, 24, 8458–8469 CrossRef CAS PubMed.
- X. Y. Zhai, X. Q. Wang, B. Wu and Y. G. Zhou, Copper-Catalyzed Si−H bond insertion polymerization for synthesis of optically active polyesters containing silicon, Chin. J. Chem., 2021, 40, 21–27 CrossRef.
- S. Ikeda, Y. Hanamura, H. Tada and R. Shintani, Rhodium-Catalyzed stitching polymerization of alkynylsilylacetylenes, J. Am. Chem. Soc., 2021, 143, 19559–19566 CrossRef CAS.
- M. Ifra, Y. Wang and M. Tebyetekerwa, Polyindole batteries and supercapacitors, Energy Storage Mater., 2020, 33, 336–359 CrossRef.
- R. B. Choudhary, S. Ansari and B. Purty, Robust electrochemical performance of polypyrrole (PPy) and polyindole (PIn) based hybrid electrode materials for supercapacitor application: A review, J. Energy Storage, 2020, 29, 101302 CrossRef.
- W. Zhou and J. Xu, Progress in conjugated polyindoles: synthesis, polymerization mechanisms, properties, and applications, Polym. Rev., 2017, 57, 248–275 CrossRef CAS.
- S. Li, Z. Chen, H. Du, M. Z. hang, J. Yin, J. Zheng, K. Deng and X. Zhang, Facile preparation of poly(indole/thiophene) for energy storage and sensor applications, Electrochim. Acta, 2020, 358, 136919 CrossRef CAS.
- P. Mayuri, S. T. Huang, V. Mani and A. S. Kumar, A new organic redox species-indole tetraone trapped MWCNT modified electrode prepared by in-situ electrochemical oxidation of indole for a bifunctional electrocatalysis and simultaneous flow injection electroanalysis of hydrazine and hydrogen peroxide, Electrochim. Acta, 2018, 268, 150–162 CrossRef CAS.
- Y. A. Udum, M. Düdükcü and F. Köleli, Electrochemical polymerization and spectroscopic investigation of 2-methylindole, React. Funct. Polym., 2008, 68, 861–867 CrossRef CAS.
- A. G. Mustafin, L. R. Latypova, A. N. Andriianova, S. M. Salikhov, A. F. Sattarova, I. N. Mullagaliev, R. B. Salikhov and I. B. Abdrakhmanov, Synthesis and physicochemical properties of poly(2-ethyl-3-methylindole), Macromolecules, 2020, 53, 8050–8059 CrossRef CAS.
- G. Chang, C. Wang, M. Du, S. Liu and Y. Yang, Metal-coordination crosslinked N-polyindoles as recyclable high-performance thermosets and nondestructive detection for their tensile strength and glass transition temperature, Chem. Commun., 2018, 54, 2906–2909 RSC.
- G. Chang, L. Yang, S. Liu, X. Luo, R. Lin and L. Zhang, Synthesis of indole-based functional polymers with well-defined structures via a catalyst-free C−N coupling reaction, RSC Adv., 2014, 4, 30630–30637 RSC.
- Y. X. Han, S. T. Zhang, J. H. He and Y. T. Zhang, B(C6F5)3-catalyzed (convergent) disproportionation reaction of indoles, J. Am. Chem. Soc., 2017, 139, 7399–7407 CrossRef CAS.
- S. T. Zhang, Y. X. Han, J. H. He and Y. T. Zhang, B(C6F5)3-catalyzed C3-selective C−H borylation of indoles: Synthesis, intermediates, and reaction mechanism, J. Org. Chem., 2018, 83, 1377–1386 CrossRef CAS PubMed.
- S. T. Zhang, H. Xu, J. H. He and Y. T. Zhang, Application of Mutualism in Organic Synthetic Chemistry: Mutually Promoted C−H Functionalization of Indole and Reduction of Quinoline, Adv. Synth. Catal., 2021, 363, 5319–5329 CrossRef CAS.
- Y. X. Han, S. T. Zhang, J. H. He and Y. T. Zhang, Switchable C–H silylation of indoles catalyzed by a thermally induced frustrated Lewis pair, ACS Catal., 2018, 8, 8765–8773 CrossRef CAS.
- S. T. Zhang, H. Xu, J. H. He and Y. T. Zhang, Synthesis and Post-polymerization Modification of Polystyrene Containing Indole Side Group, Acta Polym. Sin., 2020, 51, 1147–1152 CAS.
- A. F. G. Maier, S. Tussing, T. Schneider, U. Flörke, Z. W. Qu, S. Grimme and J. Paradies, Frustrated Lewis Pair catalyzed dehydrogenative oxidation of indolines and other heterocycles, Angew. Chem., Int. Ed., 2016, 55, 12219–12223 CrossRef CAS PubMed.
- W. Wu, R. Tang, Q. Li and Z. Li, Functional hyperbranched polymers with advanced optical, electrical and magnetic properties, Chem. Soc. Rev., 2015, 44, 3997–4022 RSC.
- Y. Zheng, S. Li, Z. Weng and C. Gao, Hyperbranched polymers: Advances from synthesis to applications, Chem. Soc. Rev., 2015, 44, 4091–4130 RSC.
- D. Wang, T. Zhao, X. Zhu, D. Yan and W. Wang, Bioapplications of hyperbranched polymers, Chem. Soc. Rev., 2015, 44, 4023–4071 RSC.
- F. Sun, X. Luo, L. Kang, X. Peng and C. Lu, Synthesis of hyperbranched polymers and their applications in analytical chemistry, Polym. Chem., 2015, 6, 1214–1225 RSC.
- F. Xie, H. Zhao, D. Li, H. Chen, H. Quan, X. Shi, L. Lou and Y. Hu, Synthesis and biological evaluation of 2,4,5-substituted pyrimidines as a new class of tubulin polymerization inhibitors, J. Med. Chem., 2011, 54, 3200–3205 CrossRef CAS PubMed.
Footnote |
† Electronic supplementary information (ESI) available: Experimental details, NMR spectra, crystal data, bond lengths and angles, and further tabular data. CCDC 2167809 and 2167804. For ESI and crystallographic data in CIF or other electronic format see DOI: https://doi.org/10.1039/d2py01470j |
|
This journal is © The Royal Society of Chemistry 2023 |