DOI:
10.1039/D3NR02208K
(Paper)
Nanoscale, 2023,
15, 13628-13634
All-inorganic Mn2+-doped metal halide perovskite crystals for the late-time detection of X-ray afterglow imaging†
Received
12th May 2023
, Accepted 20th July 2023
First published on 21st July 2023
Abstract
All-inorganic metal halide perovskite (MHP) materials have been widely studied because of their unique optoelectronic properties, whereas there has been little research reported on their X-ray afterglow imaging properties. Herein, we report the design and synthesis of Mn2+-doped hexagonal CsCdCl3 MHP crystals with excellent X-ray scintillation and X-ray induced afterglow. The orange emission from Mn2+ shows a red shift due to the strong interaction of the Mn2+–Mn2+ dimers formed at higher doping concentrations. The high-energy X-rays with higher electron filling capacity to feed the shallow (0.71 eV) and deep (0.90–1.08 eV) traps enable a long orange afterglow for more than 300 min. The afterglow emission can be rejuvenated effectively by 870 nm stimulus or heating even after 72 h of decay. Finally, we demonstrate the proof-of-concept applications of the fabricated flexible scintillator films for real-time online X-ray imaging with a spatial resolution of 12.2 lp mm−1, as well as time-lapse X-ray imaging recorded by a cell phone, which shows promise for being able to do offline late-time detection of X-ray afterglow imaging in the future.
1. Introduction
Scintillators, defined as a type of material with the ability to absorb high-energy ionising radiation and then convert it into low-energy visible photons via radioluminescence (RL), have gained significant interest due to their ability to facilitate the detection and measurement of radiation, making them invaluable in widespread applications, such as non-destructive inspection, medical radiography and diagnosis, radiation safety monitoring, and nuclear cameras.1–3 Traditional scintillators, such as Tl+-doped Cs(Na)I, Bi4Ge3O12, and Ce3+-doped YAlO3, have demonstrated remarkable scintillation properties.4–7 However, the high-temperature manufacturing process of these materials presents significant cost and implementation challenges. Moreover, the resultant powdered scintillator is prone to severe aggregation, impeding its seamless integration into device architectures. Thus, the development of low-cost, solution-processable scintillators with high X-ray sensitivity, prepared via low-temperature methods, has become increasingly necessary.
Metal halide perovskites (MHPs) are known as a class of superstar semiconductors, and have become a prime candidate for use as scintillators in recent years, because of their advantages of exceptional stopping power, high sensitivity to X-rays, large light yield, and easy preparation. Typically, some organic–inorganic hybrid and all-inorganic MHPs, such as (DIET)3Cu3X3,8 (BA)2CsAgBiBr7,9 [TPPen]2MnBr4,10 TPP2MnBr4,11 CsPbX3,12–14 Rb2CuBr3,15 Cs3Cu2X5,16,17 Cs2AgI3:Cu,18 and Cs2ZrCl6,19 have been reported and demonstrated for use in ultrasensitive X-ray detectors and low-dose X-ray imaging devices. Despite tremendous efforts, the vast majority of studies have focused on real-time X-ray imaging for flat objects by virtue of their outstanding scintillation properties, while ignoring time-lapse X-ray imaging due to the lack of efficient afterglow MHPs.20–22 Afterglow, a captivating phenomenon where a material can store light energy by capturing the electrons and/or holes, and then releasing them continuously at room temperature (RT) in the form of a self-sustained photon emission,23–28 is commonly considered to be detrimental to a high-quality scintillator and needs to be avoided in practical applications. However, the utilization of afterglow in MHP scintillators offers incomparable merits, particularly for time-lapse offline X-ray imaging of curved objects, and long-term storage X-ray imaging in some harsh environments.29,30 Fortunately, Zheng et al. made a pioneering contribution to the synthesis and study of all-inorganic lead-free MHPs with excellent afterglow properties, such as Mn2+-doped double perovskite alloys of Cs2NaxAg1−xInCl6 showing a red afterglow,31 and a Tb3+-doped double perovskite of Cs2NaScCl6 exhibiting an intense green afterglow for up to 12 h.32 These compelling results inspired us to further explore the all-inorganic lead-free afterglow MHPs and extend their applications to time-lapse offline X-ray imaging.
In this work, we reported on the Mn2+-doped all-inorganic metal halide crystals that combined scintillation and long afterglow properties. Hexagonal cadmium-based MHP crystals were prepared and deliberate doping with Mn2+ ions not only boosted the original photoluminescent (PL) intensity up to 11.3 times, but also prolonged the X-ray induced orange afterglow for up to 300 min. The structure (including crystal structure and defects)-property relationships (including PL, afterglow, optically stimulated luminescence (OSL), and scintillation properties) and electron dynamics were systematically studied using the steady-state and transient fluorescence, thermoluminescence, and radioluminescence. Because of the exceptional X-ray absorption capacity and long afterglow characteristics, the resulting phosphors were utilized to produce flexible scintillator films, demonstrating real-time online X-ray imaging with spatial resolution of 12.2 lp mm−1, together with the extension of the application for offline time-lapse X-ray imaging.
2. Experimental section
2.1. Materials
The raw materials of caesium chloride (CsCl, Aladdin, 99.99%), cadmium chloride (CdCl2, Macklin, 99.99%), manganese carbonate (MnCO3, Aladdin, 99.95%), hydrochloric acid (HCl, Guangzhou Chemical Reagent Factory, China, 37 wt% in water), and anhydrous ethanol (CH3CH2OH, Guangzhou Chemical Reagent Factory, China, AR) were used as received without any further purification.
2.2. Synthesis of CsCdCl3:Mn2+ crystal
The Mn2+-doped CsCdCl3 perovskite (CCCP) crystals were synthesized using a hydrothermal method with a stoichiometric ratio of CCCP:x%Mn2+. In a typical synthesis, 1 mmol of CsCl, 1 − x mmol of CdCl2, and x mmol of MnCO3 were dissolved in 5 ml of HCl and then transferred to a 25 ml Teflon autoclave. The mixture was heated up to 180 °C, held for 24 h, and then slowly cooled to RT over 5 h. Finally, the as-synthesized sample was washed three times with anhydrous CH3CH2OH and then dried at 60 °C overnight before further measurements were carried out.
2.3. Fabrication of the CCCP:Mn2+ films
The gel precursor was prepared by mixing PDMS with the curing agent (10
:
1 by mass). Then, the as-obtained CCCP:Mn2+ powder was added into the gel precursor (1
:
7 by mass) with vigorously stirring. Subsequently, the mixture was poured into a mould made from a circular aluminium alloy plate. The resulting composites were finally heated at 80 °C for 4 h. After cooling down to RT, the as-fabricated film with a thickness of ∼1.5 mm was peeled from the mould and used for the X-ray imaging.
2.4. Measurement and characterization
The powder X-ray diffraction (XRD) spectra of the crystals were collected using a Bruker D8 ADVANCE diffractometer equipped with a CuKα X-ray tube operating at 50 kV and 60 mA. The morphology and energy dispersive X-ray spectroscopy characterization were measured using SEM with a Tescan LYRA3 instrument, and energy-dispersive X-ray spectroscopy mapping (EDS-mapping) was carried out at an acceleration voltage of 10 kV with a charge coupled device camera. Diffuse reflectance spectra were obtained using UV-vis-NIR spectrophotometry with a Shimadzu UV-3600 plus. Photoluminescence excitation (PLE), PL spectra, fluorescence decay curves, and afterglow decay profiles were collected using fluorescence spectrometry with Edinburgh Instruments FLS980, with a continuous 450 W xenon lamp (Osram). Photoluminescence quantum efficiency (PLQY) was measured by a quantum yield measurement system with a Hamamatsu Quantaurus-QY Plus C13534-31. The thermoluminescence (TL) and OSL were collected using thermoluminescence dosimetry with a Risø TL/OSL-DA-20 instrument from RT to 400 °C with a heating rate of 3 °C s−1. A commercially available X-ray tube, Moxtek, TUB00154-9I-W06 with maximum power of 12 W was utilized for the measurements of radioluminescence (RL) and time-lapse X-ray imaging. The X-ray tube voltage and working current were set at 60 kV and 150 μA, respectively. The X-ray images were recorded using a general digital camera or a 12 megapixel rear camera on an iPhone 12 after being reflected by a reflector without an extra attenuator.
3. Results and discussion
As shown in Fig. 1a, the XRD patterns of the samples of CCCP:x%Mn (x = 0–30) match well with the standard XRD pattern (PDF#70-1615) without any impurity phase, indicating the good phase purity of the products before and after Mn2+ doping. Fig. 1b shows the crystal structure of CCCP, which indicates a hexagonal system with a P63/mmc space group. Apparently, there are two kinds of Cd sites in the 3D crystal structure. One is coordinated by six Cl atoms, forming [Cd1Cl6] octahedrons. Two Cd2 are coordinated with nine Cl to form a [Cd2Cl9] subunit, which is constructed from face-sharing [Cd2Cl6] octahedrons. The [Cd2Cl9] dimers corner-shared with the [Cd1Cl6] octahedrons. Consequently, the Mn2+ ions enter either into Cd1 or Cd2 sites, and the Mn2+–Mn2+ dimers are prone to be formed because of the superexchange coupled energy levels of two adjacent Mn2+ ions in [Cd2Cl9] with a distance of 3.33 Å (<5 Å).33 To reveal this, we focussed on the shift of the strongest (110) and the second strongest (204) XRD peaks. As shown in Fig. 1c, as the Mn2+ doping concentration increases, the diffraction peaks shift continuously and slightly towards higher angles. According to Bragg's law of 2d
sin
θ = nλ and the similarity-intermiscibility theory, this change is attributed to the substitution of smaller Mn2+ (r = 0.83 Å, CN = 6) for Cd2+ (r = 0.95 Å, CN = 6) rather than Cs+ (r = 1.67 Å, CN = 6),34 which further verifies the successful incorporation of Mn2+ into the CCCP host lattice. Remarkably, the XRD peak of the (110) plane presents a much faster angle shift rate than that of the (204) plane at a low Mn2+ doping concentration (x < 1) and a similar angle shift rate at 1 < x < 5. When x is larger than 5, the XRD peak of the (110) plane remains almost invariant whereas the (204) plane further shifts, notably to higher angle until x = 20. From the insets of Fig. 1c, it can be seen that the (110) and (204) planes are mainly related to Cd1 and Cd2, respectively. Thus, it can be concluded that Mn2+ preferentially enters into the Cd1 sites at a low doping concentration, and then into the Cd2 sites at a higher doping concentration. The SEM images and elemental mapping of the CCCP:5%Mn sample shown in Fig. 1d show the hexagonal bulk crystals with the uniformly distributed Cd, Cl, Cs, and Mn elements.
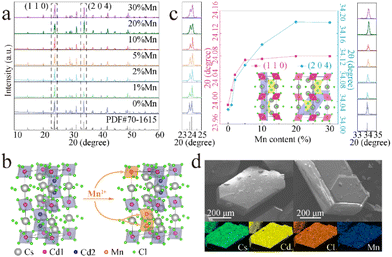 |
| Fig. 1 (a) The XRD patterns of CCCP:x%Mn crystals (left) and magnified images of the (204) diffraction peaks (right). (b) The crystal structure of CCCP and the doping of Mn2+ into two different Cd sites. (c) The XRD peaks corresponding to the (110) and (204) planes of the Mn2+-doped CsCdCl3 perovskite (CCCP) and angle shifts as the function of Mn2+ doping concentration. (d) The SEM images and EDS elemental mapping of the CCCP:5%Mn2+ crystals. | |
Fig. 2a shows the UV-vis diffuse reflectance spectra of the CCCP:x%Mn (x = 0–20) crystals. The reflectivity of the CCCP host shows a plateau in the wavelength range of 270–800 nm and starts to decrease sharply at 270 nm which is caused by host absorption. In contrast, a notable drop in the wavelength range of 240–330 nm and multiple absorption peaks in the wavelength range of 340–500 nm appear for the Mn2+-doped ones, which are typically caused by charge transfer and d–d transitions of Mn2+ ions, respectively.35 In addition, the Kubelka–Munk function and the Tauc relationship were used to estimate the optical bandgap according to the following function:36
| (F(R)hν)1/n = A(hν − Eg) | (1) |
where
F(
R) is the reflectance coefficient,
hν is the photon energy,
A is the proportional constant, and
Eg is the optical bandgap. Herein,
n = 1/2 is adopted for the estimations because of the direct bandgap of CCCP.
37 The optical bandgap of CCCP was calculated to be 4.94 eV. Fig. S1 (ESI)
† shows the PLE and PL spectra of the non-doped CCCP crystals. The PLE spectrum shows a narrow peak with the maximum at 255 nm. Upon 255 nm excitation, it exhibits orange emission with a broad band centred at 598 nm in the wavelength range of 530–750 nm. Such a large Stokes’ shift of the broadband emission for CCCP was consistent with the characteristics of self-trapped exciton (STE) emission.
38,39 As shown in
Fig. 2b, the Mn
2+-doped CCCP crystals exhibit a significantly different PLE spectrum and a much brighter PL emission with the peak maximum at 605 nm. The PLQY was found to be about 80%. Compared with the non-doped one, the PLE spectra of the Mn
2+-doped CCCP showed a noticeable red shift to 290 nm (Fig. S2, ESI
†), which was attributed to the overlap of the STE excitation and the charge transfer transitions. In addition, according to the Tanabe–Sugano diagram shown in Fig. S3 (ESI),
† some new PLE peaks within 340–450 nm were ascribed to characteristic d–d transitions of the Mn
2+ ions,
i.e., from
6A
1g to
4E
g (D) (335 nm),
4T
2g (D) (355 nm),
4A
1g,
4E
g (D) (369 nm),
4T
2g (G) (419 nm), and
4T
1g (G) (442 nm). Meanwhile, the PL spectra present a slight red shift (Fig. S2, ESI
†) due to the
4T
1g–
6A
1g energy level transitions of Mn
2+.
40–42 As the Mn
2+ doping concentration increased, it showed further red shifting caused by the formation of the Mn
2+–Mn
2+ dimers, and resulted in stronger interactions between them.
43,44 Moreover, the orange emission intensity reached the maximum which was about 11.3 times higher than the undoped one at
x = 10% and then decreased due to concentration quenching.
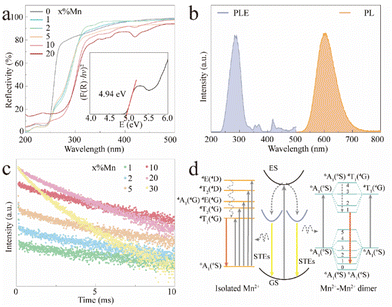 |
| Fig. 2 (a) Diffuse reflectance spectra of CCCP:x%Mn crystals and the plot of (F(R)·hν)2versus the light energy hν (inset). (b) Excitation and emission spectra of the CCCP:10%Mn crystals. (c) The fluorescence decay curve of the CCCP:x%Mn crystals (λex = 290 nm, λem = 605 nm). (d) Schematic diagram for the photophysical processes in CCCP:Mn crystals. | |
To further explore the fluorescence dynamics of the different emission centres, the fluorescence decay behaviour at RT was investigated. All the luminescence decay curves were well fitted with the following bi-exponential decay function (2):45,46
| 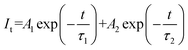 | (2) |
where
It is the luminescence intensity,
t is time,
A1 and
A2 are two constants, and
τ1 and
τ2 are the lifetimes for the two exponential components. The average lifetime (
τ) can be calculated by the formula:

. As shown in Fig. S4 (ESI),
† the average lifetime of undoped CCCP was calculated to be 4.0 μs. In contrast, as shown in
Fig. 2c, the average lifetimes of the CCCP:
x%Mn
2+ (
x = 1–30) crystals, equalling 10.22, 7.92, 5.43, 5.07, 3.08 and 1.47 ms, were much slower due to the spin-forbidden 3d–3d transition nature of the Mn
2+ ions. The monotonous decrease of lifetime with the increase of Mn
2+ doping content might be ascribed to the increasing energy-transfer probability among the Mn
2+ ions. The high temperature-dependent PL spectra of CCCP:10%Mn are shown in Fig. S5a (ESI).
† The PL intensity monotonously decreases due to thermal-quenching. However, it still maintains 61.3% of the initial intensity at 523 K, indicating the good luminescence thermal stability of Mn
2+ in the CCCP crystals. Meanwhile, the emission peak appears as a notable blue shift, and the full width at half maximum (FWHM) is broadened significantly. These results were caused by the intensified electron–phonon coupling effect with the increasing of the temperature. To quantify the electron–phonon interaction, temperature-dependent FWHM is usually fitted by the following
eqn (3):
47 | 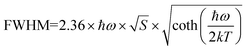 | (3) |
where
ħω is the phonon frequency,
S is Huang–Rhys factor, and
k is the Bolzmann constant. As the fitted results in Fig. S5b (ESI)
† show, the
ħω and
S were determined to be 36.3 meV and 6, respectively. The large
S implied that there was a relatively strong electron–phonon coupling effect. Based on the results presented previously, a schematic energy level diagram was constructed to illustrate the photophysical mechanism occurring in the CCCP:Mn crystals (see
Fig. 2d). Upon UV light excitation, electrons can be pumped to the excited states of the CCCP host and then relax to the self-trapped states
via non-radiative transitions, followed by the STEs emission with a large Stokes’ shift. As the Mn
2+ ions were incorporated into the CCCP host lattice, the isolated Mn
2+ ions located in the [MnCl
6]
4− octahedrons can act as exciton acceptors, in addition to the self-excitation to higher energy levels, and further as the emission centres to give the strong orange PL
via the
4T
1g to
6A
1g energy level transitions. Further doping of Mn
2+ ions leads to the formation of the Mn
2+–Mn
2+ dimers, causing the exchange coupling of the energy levels. Specifically, the ground state [
6A
1(
6S)
6A
1(
6S)] was formed by exchange coupling of the ground states of the two Mn
2+ ions in a Mn
2+–Mn
2+ dimer and the lowest excited state arose from the exchange coupling between the first excited state of one Mn
2+ [
4T
1(
4G)] and the ground state of another Mn
2+ [
6A
1(
6S)] in a Mn
2+–Mn
2+ dimer. Consequently, the exchange coupling resulted in a red-shifted orange emission from the Mn
2+–Mn
2+ dimers.
Apart from the orange emission, more interesting is the observation of a long orange afterglow in both the pristine and Mn2+-doped CCCP crystals after UV irradiation (Fig. 3a and ESI†). From the afterglow decay curves shown in Fig. 3b, it can be seen that the afterglow was greatly enhanced by Mn2+ doping and approached its optimum at x = 2. To determine the trap effect on afterglow, the TL curves of CCCP:x%Mn crystals were measured at a heating rate of 2 °C s−1 after 254 nm UV light irradiation. As shown in Fig. 3c, the CCCP:x%Mn crystals exhibited TL curves with a broad band from 30 to 300 °C with apparent two peaks, which indicating a complex trap distribution. As the Mn2+ doping concentration increased, the TL intensity increased initially, reaching a maximum at x = 2, and then decreased, which indicated that the incorporation of Mn2+ induced the enrichment of the traps. To reveal the contributions of different traps to the orange afterglow, the TL glow curves were recorded at different delay times and these are shown in Fig. 3d. It was evident that the intensity of the low-temperature TL declined rapidly and almost disappeared after 6 h but for the high temperature TL one this was very slow (more than 50% intensity remained after 72 h decay), which indicated that the observable orange afterglow was mainly dominated by the easier release of electrons from shallow traps with a smaller energy barrier. The deep traps with a much higher energy barrier meant that it was difficult to release the trapped electrons, resulting in the almost unnoticeable afterglow at room temperature. When the thermal field was applied, the nearly frozen electrons were endowed with a higher energy and were then impelled promptly to escape out of the deep traps, causing a bright orange emission. As shown in Fig. 3e, to further extract the trap distribution in the Mn2+-doped CCCP crystals, the TL curves were measured using a thermal cleaning method devised by McKeever.48,49 As expected, the low-temperature TL peak drops sharply and the high-temperature TL peak shifts gradually to the higher temperature side as the preheating temperature is increased from 30 to 250 °C, which arose from the thermally stimulated electrons from the traps to the emission centres. From these results, the relationship of Tmax−Tstop (Tmax: the temperature of the TL peak maximum at the low-temperature side, Tstop: the preheating temperature) was drawn and is shown in Fig. 3f. The Tmax − Tstop plot exhibits three distinct intervals: two flat platforms at about 79.6 ± 2 and 179.2 ± 5 °C at the lower Tstop ranges, and an oblique stage at the higher Tstop. The two flat platforms were independent of the preheating temperature which implied the presence of two individual discrete trap energy levels. The gradual increase of Tmax with the increasing preheating temperatures in the oblique stage indicated the existence of a continuous trap distribution. Thus, it can be concluded that the low-temperature TL peak is mainly attributed to a single discrete trap which dominates the observable orange afterglow, whereas the high-temperature TL peak is overlapped with a single discrete trap and continuously distributed traps. The trap depth (Etrap) is usually estimated by the following formula:31
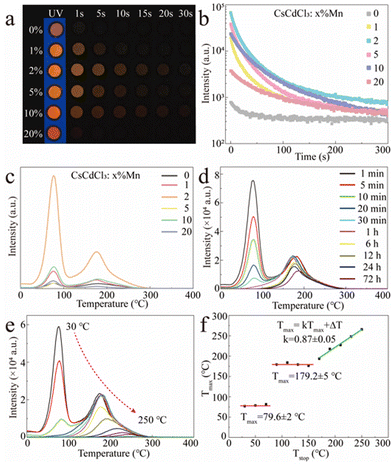 |
| Fig. 3 (a) Afterglow photographs of CCCP:x%Mn (x = 0–20) crystals taken under UV excitation and at different times after the removal of the UV light. (b) Afterglow decay curves, and (c) TL curves of CCCP:x%Mn (x = 0–20). (d) The TL curves of CCCP:2%Mn obtained at various delay times from 1 min to 24 h. (e) The TL curves of CCCP:2%Mn recorded with preheating at various temperatures. (f) The Tmax − Tstop plot of CCCP:2%Mn. | |
Accordingly, the trap depths were estimated to be 0.71 and 0.90 eV for the two individual discrete traps, as well as 0.90–1.08 eV for the continuously distributed traps.
Although the excited orange afterglow was observed in Mn2+-doped CCC MHP crystals, the afterglow performance still needed to be improved due to the limited filling ability (the traps seemingly reached saturation just by using UV irradiation at 254 nm for 30 s as shown in Fig. S6 (ESI)†) and the application is to be expanded. Keeping this in mind, we attempted to use X-rays alone as the excitation source. The TL intensity was much stronger than the UV irradiated one and the traps reached a saturation until the X-ray irradiation for 1 min occurred (Fig. S7, ESI†). As shown in Fig. 4a, the orange afterglow of CCCP:2%Mn2+ is greatly prolonged from ∼70 min by UV excitation to ∼300 min by X-ray irradiation, which is caused by the stronger filling capability of high-energy X-ray. As shown in Fig. 4b, the overall TL intensity of CCCP:2%Mn2+ with irradiation was much stronger than that with UV light irradiation, confirming that the X-rays may be a powerful tool, inducing more effective trap filling, thus triggering a better afterglow performance. In addition to the self-release of the trapped electrons at RT, and the thermally-facilitated electron release at high temperature, the localized electrons can also be stimulated from traps to Mn2+ emission centres, causing OSL.50 As shown in Fig. 4c, repeated OSL curves were obtained at different decay times (1, 24, and 72 h) using a 870 nm NIR light stimulus. After 72 h of decay after the stoppage of X-ray irradiation, the orange afterglow which had almost disappeared could still can be rejuvenated several times to a strong intensity and it showed very slight attenuation of the light intensity after 20 cycles of testing. It allows the as-obtained CCCP:Mn scintillator to be used for the on-demand and late-time detection of X-ray images.
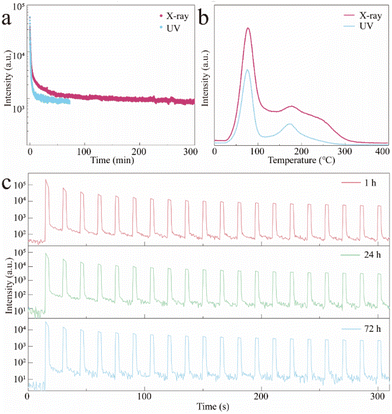 |
| Fig. 4 (a) Afterglow decay curves, and (b) the TL glow curves of CCCP:2%Mn2+ after excitation by 254 nm UV light and X-rays for 10 min. (c) The repeated OSL obtained at different decay times (1, 24, and 72 h) with an 870 nm NIR light stimulus. The stimulation time in each cycle was set as 3 s. | |
As a proof-of-concept, the as-obtained CCCP:2%Mn2+ was prepared in the form a flexible phosphor film with a diameter of 6 cm for the scintillator screen (see the inset of Fig. 5a). The RL spectrum (Fig. 5a) of the scintillator film exhibited the same shape as the emission spectrum (Fig. 2b), which implied the occurrence of orange RL. Fig. 5b shows a schematic diagram of the real-time online and time-lapse offline X-ray imaging systems employing the scintillator film. In the former case, the image can be taken using an ordinary digital camera or a mobile phone after being reflected 45 degrees by a flat mirror, and this effectively avoids the noise signal produced by direct incident X-rays (72.3 mGyair s−1). In the latter case, the images were taken directly above the charged films under the thermal and light fields. To determine the spatial resolution, the modulation transfer function (MTF) calculation was carried out by using slanted-edge image. As shown in Fig. S8 (ESI),† the spatial resolution of 12.2 lp mm−1 was obtained at a MTF of 0.2. As shown in Fig. 5c, a key inside a rubber pig (i), a nut inside a rubber duck (ii), and a plastic toy car (iii) were used for the real-time and time-lapse X-ray imaging. The target objects, including the key, nut, and the internal structure of the toy car, were clearly seen without distortion (see second row in Fig. 5c), which demonstrated high resolution real-time X-ray imaging. The flexible films were able to record the patterns during the X-ray exposure, meaning that there is potential to perform time-lapse X-ray imaging. After the stoppage of the X-ray irradiation, the recorded patterns can also be visualized, and captured by applying the thermal (180 °C) and light (870 nm) fields, which can be seen in the third and fourth rows in Fig. 5c. The previous results demonstrate that the CCCP:Mn2+ may be a potential candidate for both real-time and time-lapse X-ray imaging.
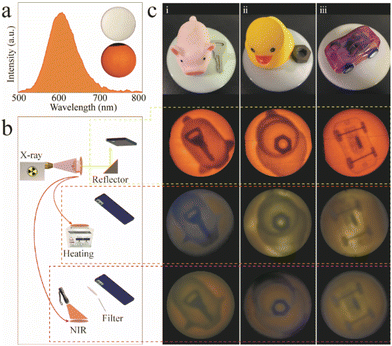 |
| Fig. 5 (a) The RL spectrum of the fabricated phosphor film. Inset: photographs of the film under daylight (top) and X-ray irradiation (bottom). (b) The schematic diagram of the real-time and time-lapse (with external heating and NIR light stimuli) X-ray imaging. (c) The real-time and time-lapse X-ray imaging photographs of various objects, including a key inside a rubber pig (i), a nut inside a rubber duck (ii), and a plastic toy car (iii), under daylight (first row), X-ray irradiation (second row), and then heating (third row), and NIR light stimulus after the removal of X-ray irradiation (fourth row). | |
4. Conclusions
In conclusion, we have synthesized, all-inorganic hexagonal cadmium-based MHP crystals combining the scintillation and afterglow properties via a typical hydrothermal method. The incorporation of Mn2+ resulted in the 4T1g to 6A1g transitions with an intense orange emission, which was 11.3 times stronger than the pristine STE emission from the non-doped CCCP crystals. The red shift of the Mn2+ emission with doping was attributed to the stronger interaction of Mn2+–Mn2+ dimers. The as-obtained Mn2+-doped CCCP crystals could be excited by X-rays and were capable of producing excellent orange long afterglows lasting over 300 min. Moreover, the structure and property relationships, encompassing PL, afterglow, OSL, and scintillation properties, were comprehensively investigated. By virtue of the exceptional X-ray absorption capacity and long afterglow features, flexible scintillator films were manufactured using the as-obtained CCCP crystals to demonstrate not only the real-time online X-ray imaging with a spatial resolution of 12.2 lp mm−1, but also the extended application for offline time-lapse X-ray imaging. The resulting insights will be used to design and optimize new MHPs for advanced offline X-ray imaging applications.
Author contributions
Sijian Wu: conceptualization, methodology, investigation, writing – original draft, writing – review, and writing – editing. Lifang Yuan: conceptualization, investigation, and data curation. Geng Chen: software and validation. Chaoyue Peng: validation and investigation. Yahong Jin: writing – review, writing – editing, supervision, data curation, and funding acquisition.
Conflicts of interest
There are no conflicts to declare.
Acknowledgements
This work was supported by the National Natural Science Foundation of China (Grant No. 51802045) and a Guangzhou Basic and Applied Basic Research Project (Grant No. 202102020871). The authors would also like to thank the staff of the Analysis and Test Center of Guangdong University of Technology for their help with the XRD and diffuse reflectance spectroscopy analyses.
References
- Y. Zhou, J. Chen, O. M. Bakr and O. F. Mohammed, ACS Energy Lett., 2021, 6, 739–768 CrossRef CAS
.
- F. Zhou, Z. Li, W. Lan, Q. Wang, L. Ding and Z. Jin, Small Methods, 2020, 4, 2000506 CrossRef CAS
.
- H. Wu, Y. Ge, G. Niu and J. Tang, Matter, 2021, 4, 144–163 CrossRef CAS
.
- M. da C. C. Pereira, T. M. Filho, J. R. Berretta and C. H. de Mesquita, Mater. Sci. Appl., 2018, 9, 268 Search PubMed
.
- W. Mengesha, T. Taulbee, B. Rooney and J. Valentine, IEEE Trans. Nucl. Sci., 1998, 45, 456–461 CAS
.
- K. Takagi, T. Oi, T. Fukazawa, M. Ishii and S. Akiyama, J. Cryst. Growth, 1981, 52, 584–587 CrossRef CAS
.
- J. Mares, M. Nikl, N. Solovieva, C. D'Ambrosio, F. De Notaristefani, K. Blazek, P. Maly, K. Nejezchleb, P. Fabeni and G. Pazzi, Nucl. Instrum. Methods Phys. Res., Sect. A, 2003, 498, 312–327 CrossRef CAS
.
- K. Han, J. Jin, B. Su, J. Qiao and Z. Xia, Adv. Opt. Mater., 2022, 9, 3529–3539 Search PubMed
.
- Z. Xu, X. Liu, Y. Li, X. Liu, T. Yang, C. Ji, S. Han, Y. Xu, J. Luo and Z. Sun, Angew. Chem., Int. Ed., 2019, 58, 15757 CrossRef CAS PubMed
.
- J. Jin, K. Han, Y. Hu and Z. Xia, Adv. Opt. Mater., 2023, 2300330 CrossRef CAS
.
- K. Han, K. Sakhatskyi, J. Jin, Q. Zhang, M. V. Kovalenko and Z. Xia, Adv. Mater., 2022, 34, 2110420 CrossRef CAS PubMed
.
- Q. Chen, J. Wu, X. Ou, B. Huang, J. Almutlaq, A. A. Zhumekenov, X. Guan, S. Han, L. Liang, Z. Yi, J. Li, X. Xie, Y. Wang, Y. Li, D. Fan, D. B. L. Teh, A. H. All, O. F. Mohammed, O. M. Bakr, T. Wu, M. Bettinelli, H. Yang, W. Huang and X. Liu, Nature, 2018, 561, 88–93 CrossRef CAS PubMed
.
- J. H. Heo, D. H. Shin, J. K. Park, D. H. Kim, S. J. Lee and S. H. Im, Adv. Mater., 2018, 30, 1801743 CrossRef PubMed
.
- H. Zhang, Z. Yang, M. Zhou, L. Zhao, T. Jiang, H. Yang, X. Yu, J. Qiu, Y. Yang and X. Xu, Adv. Mater., 2021, 33, 2102529 CrossRef CAS PubMed
.
- L. Han, H. Zhang, Y. Ning, H. Chen, C. Guo, J. Cui, G. Peng, Z. Ci and Z. Jin, Chem. Eng. J., 2022, 430, 132826 CrossRef CAS
.
- Q. Zhou, J. Ren, J. Xiao, L. Lei, F. Liao, H. Di, C. Wang, L. Yang, Q. Chen, X. Yang, Y. Zhao and X. Han, Nanoscale, 2021, 13, 19894–19902 RSC
.
- L. Lian, M. Zheng, W. Zhang, L. Yin, X. Du, P. Zhang, X. Zhang, J. Gao, D. Zhang, L. Gao, G. Niu, H. Song, R. Chen, X. Lan, J. Tang and J. Zhang, Adv. Sci., 2020, 7, 2000195 CrossRef CAS PubMed
.
- T. He, Y. Zhou, X. Wang, J. Yin, L. Gutiérrez-Arzaluz, J.-X. Wang, Y. Zhang, O. M. Bakr and O. F. Mohammed, ACS Energy Lett., 2022, 7, 2753–2760 CrossRef CAS
.
- F. Zhang, Y. Zhou, Z. Chen, M. Wang, Z. Ma, X. Chen, M. Jia, D. Wu, J. Xiao and X. Li, Adv. Mater., 2022, 34, 2204801 CrossRef CAS PubMed
.
- S. He, Q. Qiang, T. Lang, M. Cai, T. Han, H. You, L. Peng, S. Cao, B. Liu and X. Jing, Angew. Chem., 2022, 134, e202208937 Search PubMed
.
- Z. Tang, R. Liu, J. Chen, D. Zheng, P. Zhou, S. Liu, T. Bai, K. Zheng, K. Han and B. Yang, Angew. Chem., Int. Ed., 2022, 61, e202210975 CAS
.
- S. Wu, L. Yuan, G. Chen, Z. Li and Y. Jin, J. Lumin., 2022, 252, 119374 CrossRef CAS
.
- V. Shanker, D. Haranath and G. Swati, Defect Diffus. Forum, 2015, 361, 69–94 Search PubMed
.
- S. Xu, R. Chen, C. Zheng and W. Huang, Adv. Mater., 2016, 28, 9920–9940 CrossRef CAS PubMed
.
- M. Li, Y. Jin, L. Yuan, B. Wang, H. Wu, Y. Hu and F. Wang, ACS Appl. Mater. Interfaces, 2023, 15, 13186–13194 CrossRef CAS PubMed
.
- B. Wang, H. Wang, J. Huang, J. Zhou and P. Liu, J. Am. Ceram. Soc., 2019, 103, 315–323 CrossRef
.
- B.-M. Liu, R. Zou, S.-Q. Lou, Y.-F. Gao, L. Ma, K.-L. Wong and J. Wang, Chem. Eng. J., 2021, 404, 127133 CrossRef CAS
.
- T. Lyu, P. Dorenbos, C. Li, S. Li, J. Xu and Z. Wei, Chem. Eng. J., 2022, 435, 135038 CrossRef CAS
.
- X. Zhou, K. Han, Y. Wang, J. Jin, S. Jiang, Q. Zhang and Z. Xia, Adv. Mater., 2023, 35, 2212022 CrossRef CAS PubMed
.
- X. Ou, X. Qin, B. Huang, J. Zan, Q. Wu, Z. Hong, L. Xie, H. Bian, Z. Yi and X. Chen, Nature, 2021, 590, 410–415 CrossRef CAS PubMed
.
- W. Zheng, X. Li, N. Liu, S. Yan, X. Wang, X. Zhang, Y. Liu, Y. Liang, Y. Zhang and H. Liu, Angew. Chem., Int. Ed., 2021, 60, 24450–24455 CrossRef CAS PubMed
.
- X. Wang, X. Zhang, S. Yan, H. Liu and Y. Zhang, Angew. Chem., 2022, 134, e202210853 Search PubMed
.
- A. Vink, M. De Bruin, S. Roke, P. Peijzel and A. Meijerink, J. Electrochem. Soc., 2001, 148, E313 CrossRef CAS
.
- R. D. Shannon, Acta Crystallogr., Sect. A: Cryst. Phys., Diffr., Theor. Gen. Crystallogr., 1976, 32, 751–767 CrossRef
.
- Y. Su, L. Yuan, B. Wang, S. Wu and Y. Jin, J. Colloid Interface Sci., 2022, 624, 725–733 CrossRef CAS PubMed
.
- G. Xiong, L. Yuan, Y. Jin, H. Wu, B. Qu, Z. Li, G. Ju, L. Chen, S. Yang and Y. Hu, J. Mater. Chem. C, 2021, 9, 13474–13483 RSC
.
- C. F. Holder, J. Fanghanel, Y. Xiong, I. Dabo and R. E. Schaak, Inorg. Chem., 2020, 59, 11688–11694 CrossRef CAS PubMed
.
- S. Li, J. Luo, J. Liu and J. Tang, J. Phys. Chem. Lett., 2019, 10, 1999–2007 CrossRef CAS PubMed
.
- P. Han, C. Luo, S. Yang, Y. Yang, W. Deng and K. Han, Angew. Chem., Int. Ed., 2020, 59, 12709–12713 CrossRef CAS PubMed
.
- B. Su, G. Zhou, J. Huang, E. Song, A. Nag and Z. Xia, Laser Photonics Rev., 2021, 15, 2000334 CrossRef CAS
.
- J.-H. Wei, J.-F. Liao, X.-D. Wang, L. Zhou, Y. Jiang and D.-B. Kuang, Matter, 2020, 3, 892–903 CrossRef
.
- Q. Wei, M. Li, Z. Zhang, J. Guo, G. Xing, T. C. Sum and W. Huang, Nano Energy, 2018, 51, 704–710 CrossRef CAS
.
- X. Li, T. Liu, K. Zhang, Z. Hu, H. An, S. Deng, Y. Kong and B. Wang, J. Mater. Chem. C, 2023, 11, 712–721 RSC
.
- E. Song, S. Ye, T. Liu, P. Du, R. Si, X. Jing, S. Ding, M. Peng, Q. Zhang and L. Wondraczek, Adv. Sci., 2015, 2, 1500089 CrossRef PubMed
.
- K. Han, J. Qiao, S. Zhang, B. Su, B. Lou, C. Ma and Z. Xia, Laser Photonics Rev., 2023, 17, 2200458 CrossRef CAS
.
- X. Li, W. Huang, R. Zhang, Y. Guo, H. Yang, X. He, M. Yu, L. Wang and Q. Zhang, Rare Met., 2022, 41, 1230–1238 CrossRef CAS
.
- J. Jin, Y. Peng, Y. Xu, K. Han, A. Zhang, X.-B. Yang and Z. Xia, Chem. Mater., 2022, 34, 5717–5725 CrossRef CAS
.
- S. W. S. McKeever, Phys. Status Solidi A, 1980, 62, 331–340 CrossRef CAS
.
- C. Wang, Y. Jin, J. Zhang, X. Li, H. Wu, R. Zhang, Q. Yao and Y. Hu, Chem. Eng. J., 2023, 453, 139558 CrossRef CAS
.
- L. Yuan, Y. Jin, Y. Su, H. Wu, Y. Hu and S. Yang, Laser Photonics Rev., 2020, 14, 2000123 CrossRef CAS
.
|
This journal is © The Royal Society of Chemistry 2023 |