DOI:
10.1039/D3NR01299A
(Paper)
Nanoscale, 2023,
15, 11482-11490
Multi-heteroatom-doping promotes molecular oxygen activation on polymeric carbon nitride for simultaneous generation of H2O2 and degradation of oxcarbazepine†
Received
21st March 2023
, Accepted 17th May 2023
First published on 24th May 2023
Abstract
Simultaneously realizing the efficient generation of H2O2 and degradation of pollutants is of great significance for environmental remediation. However, most polymeric semiconductors only show moderate performance in molecular oxygen (O2) activation due to the sluggish electron–hole pair dissociation and charge transfer dynamics. Herein, we develop a simple thermal shrinkage strategy to construct multi-heteroatom-doped polymeric carbon nitride (K, P, O-CNx). The resultant K, P, O-CNx not only improves the separation efficiency of charge carriers, but also improves the adsorption/activation capacity of O2. K, P, O-CNx significantly increases the production of H2O2 and the degradation activity of oxcarbazepine (OXC) under visible light. K, P, O-CN5 shows a high H2O2 production rate (1858 μM h−1 g−1) in water under visible light, far surpassing that of pure PCN. The apparent rate constant for OXC degradation by K, P, O-CN5 increases to 0.0491 min−1, which is 8.47 times that of PCN. Density functional theory (DFT) calculations show that the adsorption energy of O2 near phosphorus atoms in K, P, O-CNx is the highest. This work provides a new idea for the efficient degradation of pollutants and generation of H2O2 at the same time.
1. Introduction
Oxcarbazepine (OXC), as a kind of refractory organic pollutant in wastewater, is strongly toxic and highly persistent, posing a serious threat to the water environment.1–4 Thus, it has become urgently necessary to find solutions all over the world to reduce the toxicity of refractory organic wastewater throughout the process of degradation.5 Recently, several studies have concentrated on the use of advanced oxidation technologies (AOPs) to quickly remove organic contaminants from water.6–8 Nevertheless, to promote the catalytic oxidation process and form reactive oxygen species (ROS), such as hydroxyl radicals (˙OH), superoxide radicals (˙O2−) and singlet oxygen (1O2),9,10 it is often essential to add a strong oxidant (e.g., H2O2 or persulfate) or input energy (e.g., light or electricity) in most AOP techniques.11,12 Although these methods promote the generation of a large number of ROS and effectively degrade organic pollutants, the price is higher energy use and environmental pollution.13 Therefore, there is an increasingly urgent need to develop an environmentally friendly strategyy that has simple operation and mild reaction conditions to solve the problem of water pollution.
Molecular oxygen (O2), as a non-toxic, pollution-free and cheap oxidant, has many advantages over other oxidants, playing an important role in the field of refractory organic wastewater treatment.14 The activated O2 can stimulate the production of ROS, including 1O2, ˙OH, ˙O2− and hydrogen peroxide (H2O2), which are the main active substances in the degradation process of organic pollutants. It is worth noting that polymeric carbon nitride (PCN) has attracted extensive attention from many researchers in O2 activation (O2 → ˙O2− → H2O2 → ˙OH).15–22 Among them, the in situ formation of ˙OH is of great significance for environmental remediation, which is due to its strong oxidation capacity.23–25 However, a one-step single-electron oxygen reduction reaction (ORR) is easy to achieve, resulting in ˙O2− radicals as the main ROS. Considering that the photocatalytic properties of these charge transfer processes are related to the lifetime and concentration of the carriers that can reach the catalyst surface, great efforts have been made in the separation of photoinduced electron–hole pairs, especially in nanostructure design and band structure specifications.26
In particular, elemental doping27 and defect control28 are the most widely used methods to improve the photocatalytic activity of PCN. For instance, PCN that has been doped with alkali metals (K+ or Na+) displays a wide spectrum of light absorption and high separation efficiency of charge. As another example, nonmetallic dopants such as O or S are used to improve the selectivity of PCN for H2O2 formation.29–33 In addition, there are also many relevant literature reports stating that the separation efficiency of electron–hole pairs can be improved by introducing pollutants as hole traps, thus accelerating the ORR.34–36 Given that both dopants and defects may change the electronic structure, adding flaws while doping foreign elements might improve light absorption and encourage charge separation. The narrowing of the band gap and the improvement of light absorption allow for the production of additional photoelectrons and holes. In addition, by facilitating charge separation, more available electrons can be captured and participate in surface reactions.37–39 Thus, the degradation of OXC with simultaneous H2O2 generation is of particular interest for the development of wastewater treatment since H2O2 is an environmentally friendly oxidizing agent that under certain conditions can generate the highly reactive ˙OH.
Herein, multi-heteroatom-doped PCN (K, P, O-CN) was prepared by a simple thermal shrinkage method. The obtained K, P, O-CNx not only greatly improved the charge separation efficiency but also significantly enhanced the O2 activation. The results showed that K, P, O-CN5 had the highest photocatalytic activity to produce H2O2, and the apparent rate constant of OXC removal was 8.47 times higher than that of the pure PCN. Quenching experiments and electron spin resonance (ESR) analysis confirmed that the multi-heteroatom doped PCN effectively promotes the activation of O2, thus realizing the efficient generation of H2O2 and degradation of OXC.
2. Experimental section
2.1. Preparation of PCN and K, P, O-CNx
Normally, 10 g of urea and a series of varying quantities of potassium dihydrogen phosphate (KH2PO4) are added to the crucible to be fully ground into a powder. Then, the ground powder is calcined in a Muffle furnace under 550 °C for 4 h at a heating rate of 5 °C min−1. Then the resulting solid is ground again into a powder. The quantities of KH2PO4 were 0.1 g, 0.3 g, 0.5 g, and 1.5 g, respectively, and the photocatalysts were named K, P, O-CN1, K, P, O-CN3, K, P, O-CN5, and K, P, O-CN15. The preparation process of PCN was also the same as that for K, P, O-CNx, except for not adding potassium dihydrogen phosphate.
2.2. Characterization
The structure and morphology of the photocatalysts were analyzed by X-ray diffraction (XRD, Rigaku, SmartLab). Ultraviolet spectrophotometry (UV-3600, Shimadzu, Japan) with standard BaSO4 powder as a reference was carried out to study the UV-vis diffuse reflectance spectra (DRS) of the prepared samples. What is more, the chemical structure of the photocatalysts was characterized using X-ray photoelectron spectroscopy (XPS; Thermo Scientific K-Alpha+, USA) and Fourier transform infrared (FTIR, Tensor 27, Bruker Optics, German) spectroscopy. Photoluminescence spectroscopy (PL) was performed on a fluorescence spectrophotometer F-7000 (Hitachi, Japan) with a laser excitation source of 360 nm. Electron spin resonance (ESR, EOL JES-FA200 EER) spectroscopy with 5,5-dimethy1-1 pyrroline N-oxide (DMPO) and 2,2,6,6-tetramethyl-L-piperidine-N-oxyl (TEMPO) as spin label molecules was used to detect the active species in the sample. Temperature-programmed desorption (TPD, AutoChem1 II 2920) was used to measure the O2 adsorption on the samples. Additionally, the Brunauer–Emmett–Teller (BET) method was applied using a Micromeritics ASAP 2460 3.01 in order to determine the precise surface areas of samples.
2.3. Photoelectrochemical performance measurement
On an electrochemical workstation (CHI660D, Chenhua, China) with the standard three electrodes, the electrochemical impedance spectra (EIS), the photoelectrochemical properties comprising the photocurrent response (PCR), and the Mott–Schottky plot were measured. The electrochemical workstation consists of the standard Ag/AgCl, Pt plate and working electrode. The sample was prepared as follows: 40 mg of the prepared sample was mixed with 100 μL of Nafion, 500 μL of ethylene glycol, and 500 μL of ethanol in a 2 mL centrifuge tube, and then ultrasonic stirring was performed until the solution was uniform. Then 100 μL of the obtained solution was evenly spread on an indium tin oxide (ITO) glass conductive surface, which had an effective surface area of 1 cm2. In addition, the photocurrent response (PCR) and electrochemical impedance spectra (EIS) of the samples were tested in 0.5 M Na2SO4 aqueous solution and 5 mM Fe [(CN)6]3−/4− solution, respectively.
In addition, on a Chenhua CHI 760E electrochemical workstation with the standard three electrodes, the rotating disk electrode (RDE) was run. The Ag/AgCl electrode and Pt plate were the same as above. The working electrode was prepared as follows: continuous ultrasonic treatment was used to equally distribute 5 mg of catalysts in 1 mL of an ethanol solution containing 100 μL of Nafion. A clean glassy carbon electrode received 5 mL of the produced sample in an even layer, and it was allowed to air dry there at room temperature. In an oxygen-saturated phosphate buffer solution with a pH of 7, the linear sweep voltammetry (LSV) was studied. The rotational speeds are 400 rpm, 800 rpm, 1200 rpm, 1600 rpm, and 2500 rpm, respectively. The LSV curves were produced at a scan rate of 5 mV s−1.
2.4. Photocatalytic activity of H2O2 generation
Photocatalytic activity evaluation was performed under visible light with a 300 W Xe lamp (λ > 420 nm) and the experimental temperature was kept at 25 °C by condensed water device. The experimental steps for the photocatalytic production of H2O2 were as follows: 25 mg of catalyst and 50 mL of deionized water solution were put into a beaker. After 5 min of ultrasonic stirring, the solution was uniformly oxygenated in the dark and stirring continued for 30 min. Before starting the photocatalytic process, the lamp needed to be warmed for 30 minutes. During the photocatalytic reaction, 2 mL of suspension was collected every 10 min. The obtained suspension was centrifuged to remove the precipitate and take the supernatant. The supernatant was chromogenically reacted with horseradish peroxidase (HRP) and N,N-diethyl-p-phenylenediamine sulfate (DPD) to determine the H2O2 concentration with an absorption peak at 551 nm.
2.5. Photocatalytic degradation activities
The ability of the as-prepared samples to degrade OXC was determined in a quartz glass vessel, and the visible light source was a 300 W Xe lamp (λ > 420 nm). Condensed water device was used to maintain the experimental temperature at 293 K. The reaction solution was prepared as follows: 25 mg of photocatalyst was dispersed in 50 mL of OXC solution at 50 ppm. After 5 min of ultrasonic stirring, the mixture was evenly oxygenated in the dark while being stirred for another 30 min. Then, every 10 min during the 60-minute-long degradation process, 1.5 mL of the reaction solution was drawn into the centrifuge tube and centrifuged. The upper clarification fluid was measured using liquid chromatography (HPLC). The mobile phases were composed of acetonitrile and water with a volume ratio of 30
:
70. Furthermore, the flow rate, injection volume, and testing cycle were 1 mL min−1, 10 μL and 7.1 min, respectively. In addition, according to the formula ln(C0/Ct) = Kappt, the photocatalytic degradation rate constant can be evaluated by the pseudo first-order kinetics. Ethylenediamine tetra-acetic acid disodium salt (EDTA-2Na) and argon (Ar) gas were used as scavengers for holes (h+) and superoxide radicals (˙O2−), respectively, and the contributions of h+ and ˙O2− were estimated by the following formula: | 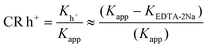 | (1) |
| 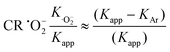 | (2) |
where Ct and C0 are the concentration of oxcarbazepine at time t and initial time. Kapp denotes the apparent rate constant. Furthermore, in the presence of the respective radical scavengers, KAr and KEDTA-2Na are the apparent rate constants throughout the OXC degradation process.
2.6. DFT calculations
DFT calculations were performed using the Gaussian 16, Revision C.01 software package (Frisch, 2019).40,41 Calculations of frequencies were carried out to make sure the stability configuration did not have any imaginary frequencies. The self-consistent reaction field (SCRF) based on the implicit solvation model density (SMD) model was used to account for the solvent impact of H2O.42 The frontier molecular orbital, density of states (DOS), interaction region indicator (IRI),43 hole–electron analysis44 and interfragment charge transfer (IFCT) were calculated with Multiwfn 3.8(dev).45 VMD 1.9.3 was used to display all images of structures and isosurfaces.46 The def2-TZVPP basis set based on ORCA 4.2.1 was used in conjunction with PWPB95-D3(BJ) double-hybrid functionals to calculate the single-point energy.47 The adsorption energy (Eads) of O2 was calculated as follows: | Eads(O2) = E(*O2) − E(*) − E(O2) | (3) |
where E(*O2), E(*) and E(O2) are the total energy of samples with O2 adsorbates on the surface, the energy of pristine samples surface and O2, respectively.
3. Results and discussion
3.1. Structure and morphology analysis
To clarify the material structure of the as-synthesized catalysts, a series of characterization analyses were executed including XRD patterns, FT-IR spectra and XPS spectra. As shown in Fig. 1a, both PCN and K, P, O-CN5 exhibit a similar crystalline structure, showing that multi-heteroatom doping does not change the structure of PCN.
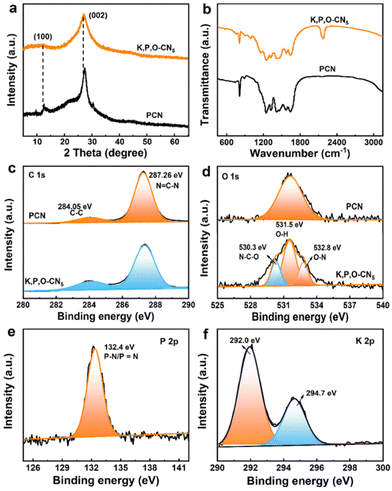 |
| Fig. 1 (a) XRD patterns, (b) FT-IR spectra of PCN and K, P, O-CN5. (c and d) The C 1s and N 1s high-resolution XPS spectra of PCN and K, P, O-CN5. The (e) P 2p and (f) K 2p high-resolution XPS spectra of PCN and K, P, O-CN5. | |
It is worth noting that there are two obvious diffraction peaks at 12.9° (100) and 27.8° (002) in PCN which could be ascribed to the planar ordered arrangement of tri-s-triazine and interlayer superposition of conjugated aromatic hydrocarbons.48–51 Specifically, compared with PCN, the (002) peak of K, P, O-CN5 has shifted significantly, which indirectly proves that K, P and O elements are introduced into the PCN structure. In addition, the FT-IR spectra were obtained to further make out the interior structure of catalysts (Fig. 1b). In detail, the peaks of PCN and K, P, O-CN5 located at 810 cm−1 and 1200–1600 cm−1, respectively, could be put down to the triazine unit and aromatic C–N heterocyclic units. Notably, a new characteristic peak appears at 2200 cm−1, which belongs to the cyano group (–C
N). To get insight into the local electronic structure, the XPS spectra of the as-prepared samples were obtained. From the C 1s XPS spectra in Fig. 1c, the typical peaks at 284.50 eV and 287.26 eV are attributed to the C–C/C
C bonds and N
C–N bonds, respectively.52,53 What is more, the XPS spectra in Fig. S1† for N 1s XPS spectra show characteristic CN
C, N-[C]3 and C-NHx peaks at 397.8 eV, 399.4 eV and 400.4 eV, respectively. Analogously, for O 1s XPS spectra in Fig. 1d, compared to PCN, two new peaks at 530.3 eV and 532.8 eV are attributed to the N–C–O and O–N bonds, demonstrating the successful doping of the O element. What is more, the P 2p XPS spectra of PCN and K, P, O-CN5 can be resolved into one main peak at 132.4 eV (Fig. 1e), which can be assigned to P–N/P
N. Further, the main K1/2p and K3/2p peaks of K 2p are located at 294.7 and 292.0 eV, respectively (Fig. 1f), which are consistent with the binding energy of the K–N bond. In brief, the above characterization studies all demonstrate the successful doping of K, P and O elements into PCN. Furthermore, the morphology of PCN and K, P, O-CN5 was tested by TEM. The TEM images of PCN and K, P, O-CN5 display the analogous nanosheet structure, suggesting that K, P and O co-doping did not change the morphology of PCN (Fig. S2a and b†). The elemental mapping images of K, P, O-CN5 show a uniform distribution of C, N, O, P and K elements (Fig. S2c†), which further confirms the above experimental results.
3.2. Photocatalytic H2O2 production activity of as-prepared samples
The performance of PCN and K, P, O-CNx samples for photocatalytic production of H2O2 was investigated. Fig. 2a shows that the H2O2 yield of K, P, O-CN is improved to some degree compared with that of pure PCN. Obviously, with the increase in the concentration of K2HPO4, the H2O2 yield is also increased. Compared to PCN, the H2O2 yield of K, P, O-CN5 is the highest, which reaches 46.46 μM in 1 hour under pure water. However, the H2O2 yield of K, P, O-CN15 decreases (Fig. S3–S7†), possibly because the concentration of K2HPO4 is too high, which destroys the structure of PCN. As shown in Fig. 2b, it is found that the H2O2 generation of K, P, O-CN5 with an ultrahigh rate of 1858 μM h−1 g−1 is about 1574.91 times that for pure PCN. The improvement of H2O2 production performance of K, P, O-CN5 may be attributed to multi-heteroatom-doped PCN promoting O2 activation. In addition, the conditions of H2O2 production are studied (Fig. 2c and d). It is found that there is no H2O2 detected in the absence of light or catalyst or in a vacuum, indicating that H2O2 is produced by the photocatalytic ORR.
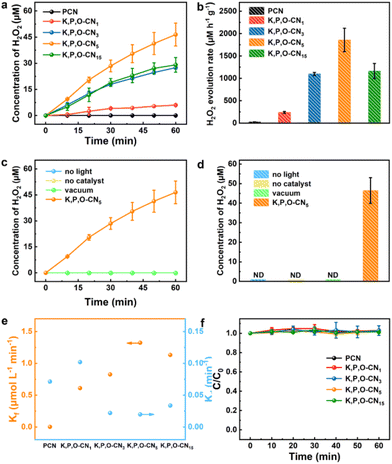 |
| Fig. 2 (a) Photocatalytic H2O2 production, (b) H2O2 production rate of as-prepared samples. (c and d) Photocatalytic H2O2 production activity of K, P, O-CN5 under different conditions. (e) Formation rate constant (Kf) and decomposition rate constant (Kd) for H2O2 production. (f) The photocatalytic decomposition of H2O2 (1 mM) under visible light irradiation. | |
Usually, the final concentration of H2O2 is determined by the amount of H2O2 produced and H2O2 decomposition. By assuming the corresponding zero-order kinetics for H2O2 formation and first-order kinetics for H2O2 decomposition, the concentration of H2O2 accumulated can be well described by the kinetic model, using the equation:
| 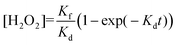 | (4) |
K
f and Kd are the rate constants of H2O2 formation (μM min−1) and decomposition (min−1), respectively. The values of Kf and Kd are estimated using the fitting data in Fig. 2a and eqn (1). As shown in Fig. 2e, the Kf value of K, P, O-CN5 reaches a maximum (1.32 μM min−1) that is 806 times that of PCN (0.00164 μM min−1). Furthermore, K, P, O-CN5 has the lowest Kd (0.0198 min−1), showing that H2O2 generated over K, P, O-CN5 has excellent stability. Therefore, under a certain amount of H2O2 (initial concentration of H2O2 [C0] = 1 mM), the decomposition performance of H2O2 under visible-light radiation was further conducted. Apparently, H2O2 almost never breaks down in 1 h, which leads to excellent photocatalytic H2O2 production.
3.3. Photocatalytic degradation activity of as-prepared samples
To demonstrate that OXC can be used as a sacrificial reagent to promote O2 activation to produce H2O2, photocatalytic degradation of OXC was performed. As shown in Fig. 3a, the yield of K, P, O-CN5 photocatalytic production of H2O2 in a pure aqueous solution was substantially higher compared to PCN, reaching 46.5 μM. What is more, the yield of H2O2 (109.7 μM) for K, P, O-CN5 was obviously enhanced in the OXC solution at a concentration of 50 ppm. Most importantly, as displayed in Fig. 3b, PCN degraded 43.6% of OXC within 60 min, while K, P, O-CN5 degraded 97.0%. Correspondingly, the apparent rate constant of K, P, O-CN5 for OXC removal is 8.47 times higher than that of the pure PCN (Fig. S8†). In addition, the photocatalytic production of H2O2 and degradation activity at different concentrations of OXC are shown in Fig. S9 and S10.† Evidently, multi-heteroatom-doped PCN promotes O2 activation for the in situ generation of H2O2 and the degradation of OXC. In order to verify the contribution of different free radicals to the degradation of OXC by K, P, O-CN5, quenching experiments were performed. During the degradation process, ethylenediamine tetraacetic acid disodium salt (EDTA-2Na) and argon (Ar) are responsible for scavenging holes (h+) and superoxide radicals (˙O2−), respectively. The degradation efficiency of K, P, O-CN5 was found to decrease significantly after the addition of EDTA. The contribution rate of h+ in the photocatalytic degradation reaction was calculated to be 87.5% (Fig. 3c and d), indicating that h+ plays a dominant role in the degradation. The above experimental results also prove that OXC as a hole trapping agent not only accelerates the separation of carriers but also promotes the activation of O2 to produce H2O2. However, it is noteworthy that when isopropanol (IPA) is added to the solution, the photocatalytic degradation performance of OXC is significantly improved. The ability to improve the above performance is attributed to the in situ formation of more ˙OH. Obviously, the electron spin resonance (ESR) spectra directly show the existence of more h+ and ˙OH in K, P, O-CN5 than in the PCN system (Fig. 3e and f), matching well the higher photocatalytic activity of K, P, O-CN5 as compared to PCN.
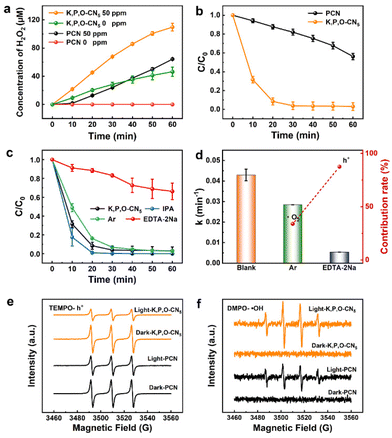 |
| Fig. 3 (a) Photocatalytic H2O2 production of PCN and K, P, O-CN5 with OXC solution at different concentrations under visible light irradiation. (b) The photocatalytic decomposition curves of OXC with PCN and K, P, O-CN5 under visible light irradiation. (c) The decomposition curves of OXC on K, P, O-CN5 with different radical scavengers. (d) The first-order reaction kinetic constants and free radical contribution of OXC degradation on K, P, O-CN5. ESR spectra of the (e) TEMPO-h+ and (f) DMPO-˙OH. | |
3.4. Mechanism analysis of photocatalytic performance improvement
To further investigate the mechanism of photocatalytic H2O2 production, the nitrogen adsorption–desorption isotherms of PCN and K, P, O-CN5 were tested. Compared to PCN, K, P, O-CN5 has a larger specific surface area (Fig. 4a), which indicates that there are more active sites on the surface of K, P, O-CN5 for photocatalytic H2O2 production.54,55 As shown in Fig. 4b, K, P, O-CN5 has stronger O2-TPD signals, unraveling that the oxygen adsorption capacity of K, P, O-CN5 is stronger than that of PCN, thus favoring the occurrence of the ORR. In addition, according to the electron spin-resonance (ESR), the DMPO-˙O2− signal of K, P, O-CN5 is stronger than that of PCN (Fig. 4c), illustrating that H2O2 is likely to be produced by a sequential two-step ORR. As we all know, O2 can accept one electron to form ˙O2−, and then the ˙O2− takes another electron to generate H2O2 through a sequential two-step one electron ORR.56 To further gain insight into the electron transfer in H2O2 production, the rotating disk electrode (RDE) was used. Fig. 4d and e shows linear sweep voltammetry (LSV) curves at different rotational speeds. It is found that the strength of LSV increases with the increase of the rotational speed, and the average numbers of transferred electrons (n) fitted by the Koutecky–Levich plots are calculated to be 2.28 and 0.91 for K, P, O-CN5 and PCN, respectively (Fig. 4f). Therefore, compared with PCN, it is easier for K, P, O-CN5 to produce H2O2 through the 2e− ORR.
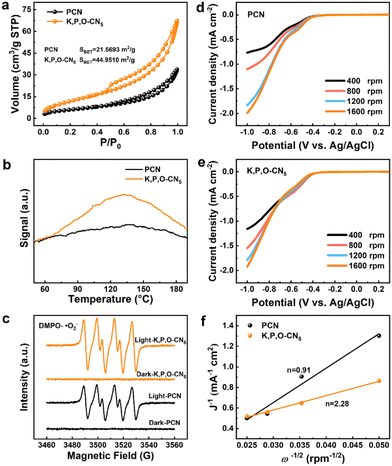 |
| Fig. 4 (a) N2 adsorption/desorption isotherms and (b) O2-TPD spectra of K, P, O-CN5 and PCN. (c) ESR spectra of DMPO-˙O2−adduct of K, P, O-CN5 and PCN. (d) PCN and (e) K, P, O-CN5 measured on RDE at different rotating speeds. (f) Koutecky–Levich plots of data obtained by RDE analysis of PCN and K, P, O-CN5. | |
The UV-vis DRS and the corresponding band structure of PCN and K, P, O-CN5 were explored to further clarify the intrinsic structural merits for photocatalytic activity.57 Apparently, Fig. 5a shows that K, P, O-CN5 has a broader range of light absorption compared to PCN and the fitted band gap energies (Eg) of PCN and K, P, O-CN5 are 2.95 eV and 2.76 eV. What is more, the flat band potentials (EFB) of PCN and K, P, O-CN5 are −1.35 V and −0.91 V, respectively, measured by Mott–Schottky analysis (Fig. S11 and S12†), which are converted to −1.15 V and −0.71 V (vs. NHE) in accordance with the relationship between EFB and conduction band CB over an n-type semiconductor. Integrated with the equation Eg = EVB − ECB, the calculated valence band (VB) positions of PCN and K, P, O-CN5 are 1.8 V and 2.05 V, respectively. Therefore, the corresponding band structures of PCN and K, P, O-CN5 were obtained, as shown in Fig. 5b. It can be seen that both PCN and K, P, O-CN5 satisfy the needs of O2 reduction E (O2/H2O2 = 0.68 V vs. NHE) and H2O oxidation E (H2O/H2O2 = 0.68 V vs. NHE).58 Specifically, the narrower band gap and more positive VB means more efficient carrier separation and greater oxidizability. In addition to the light absorption capacity and band structure, the electrochemical properties of as-prepared samples also have a great influence on the catalytic performance. As shown in Fig. 5c, the K, P, O-CN5 possesses a lower photoluminescence (PL) peak, which is due to the K, P, O element doping changing the efficiency of carrier separation within the catalysts. Analogously, the higher photocurrent response (PCR) density and smaller EIS of K, P, O-CN5 than PCN mean that K, P, O-CN5 is endowed with a stronger and more available charge separation efficiency (Fig. 5d and e).59,60 Moreover, excellent photoelectric performance was also mapped by the LSV response and EPR (Fig. 5f and Fig. S13†).
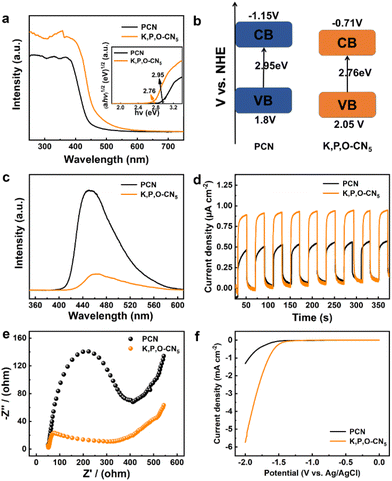 |
| Fig. 5 (a) UV-vis DRS (inset: Tauc plots of as-prepared catalysts) and (b) corresponding band structures of PCN and K, P, O-CN5. (c) PL, (d) PCR, (e) EIS and (f) LSV curves of PCN and K, P, O-CN5. | |
3.5. Possible degradation pathways
The main intermediates produced during the degradation of OXC were revealed by high-performance liquid chromatography–mass spectrometry (HPLC-MS) analysis, as shown in Fig. 6a, Fig. S14 and Table S1.† OXC was attacked by ˙OH to produce P1 (m/z = 268), after which there were two degradation pathways. The first pathway was attack by –CONH2 to produce P2 (m/z = 225), then P2 was degraded to P3 (m/z = 207), and P3 was converted to P4 (m/z = 179). The second pathway is that P1 continues to be attacked by ˙OH, which eventually transforms into P8 (m/z = 282). In addition, there is another pathway in the system. OXC may also react with ˙OH and –CONH2 to form P9 (m/z = 223), and P9 undergoes benzilic acid rearrangement to form P10 (m/z = 223). P10 rearranges and reacts with –CO to form P12 (m/z = 195). After the rearrangement of P12, it reacts with ˙OH to eventually convert to P14 (m/z = 211). In addition, the interfacial interaction between OXC and catalyst was first investigated by theoretical calculations based on DFT. As shown in Fig. 6b–d, the interaction region index (IRI) demonstrates the weak contact (π–π) between OXC and PCN as well as K, P, O-CN5, respectively.61 In addition, the weak interactions between OXC and PCN as well as K, P, O-CN5, respectively, were examined by lowering the density gradient (RDG). As shown in Fig. 6c–e, blue, green and red represent strong gravitational, van der Waals and repulsive forces, respectively. The results of RDG show that there are van der Waals forces between OXC and K, P, O-CN5, which are greater than those between OXC and PCN.
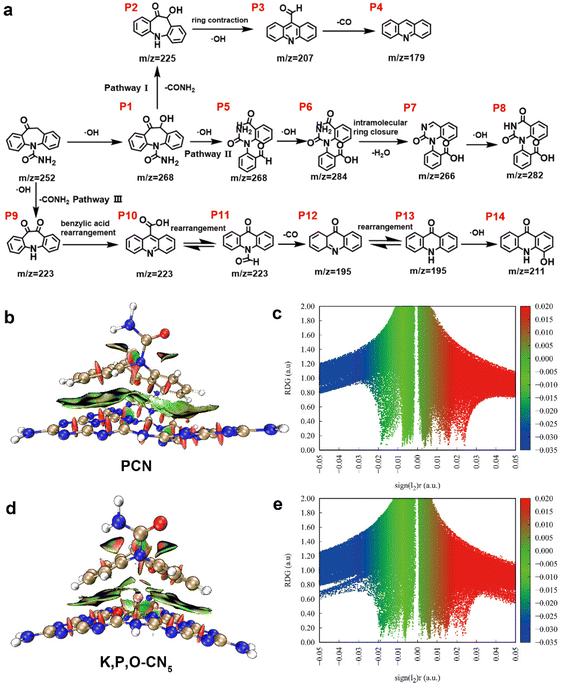 |
| Fig. 6 (a) Presumed OXC degradation pathways in K, P, O-CN5. (b) Isosurface map of IRI and (c) the plots of RDG versus electron density (ρ) multiplied by the sign of the second Hessian eigenvalue (λ2) of PCN. (d) Isosurface map of IRI and (e) the plots of RDG versus electron density (ρ) multiplied by the sign of the second Hessian eigenvalue (λ2) of K, P, O-CN5. | |
3.6. DFT calculations
In order to further analyze the catalytic mechanism, PCN and K, P, O-CN5 conjugated polymer system models were established based on DFT. As shown in Fig. 7e, the adsorption energy of O2 adsorption at different sites of PCN is roughly the same. In contrast, the adsorption energy of O2 adsorption for K, P, O-CN5 is improved to a certain extent, and the adsorption energy near the phosphorus atom is the largest (−0.179 eV), as shown in Fig. 7d. DFT was also used to investigate the density of states (DOS), aiming to show how the band structure had changed. As shown in Fig. 7a and b, the K, P, O-CN5 conjugated polymer has small bandgaps in comparison with PCN. Notably, as seen in Fig. 7c, the nitrogen ρz orbitals and C–N orbitals are primarily responsible for PCN's highest occupied molecular orbit (HOMO) and lowest occupied molecular orbit (LUMO).62 The HOMO and LUMO of K, P, O-CN5 conjugated polymers, on the other hand, are scattered on various structural units, showing a spatial separation of HOMO and LUMO. The above results prove that K, P, O-CN5 has higher O2 adsorption efficiency and better photocatalytic performance.
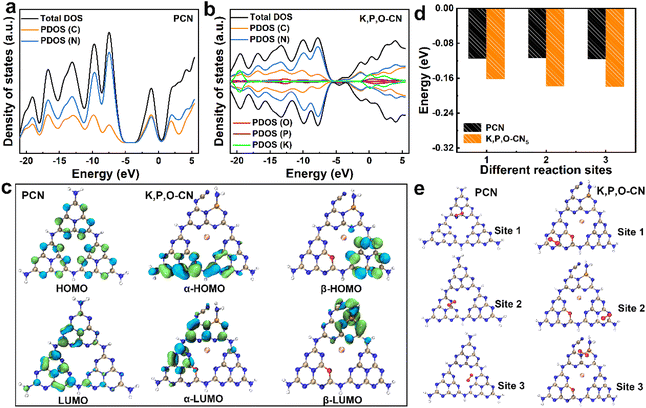 |
| Fig. 7 (a and b) DOS of PCN and K, P, O-CN5. (c) Electronic structure of the optimized HOMO and LUMO of pure PCN and K, P, O-CN5. (d) The adsorption energy of O2 for PCN and K, P, O-CN5 at different sites. (e) The structures after O2 adsorption at different sites of PCN and K, P, O-CN5. Green and blue isosurfaces represent electron and hole distributions, respectively. The isosurface value is 0.002 e Å−3. Gray, blue, red, white, pink and golden represent C, N, O, H, K and P, respectively. | |
4. Conclusions
In conclusion, multi-heteroatom-doped PCN was prepared by a simple thermal shrinkage method. The K, P, O-CN5 exhibits the highest photocatalytic activity for H2O2 production (1858 μM h−1 g−1), which is about 1574.91 times that for pure PCN. In addition, the apparent rate constant of OXC degradation in K, P, O-CN5 reaches 0.0491 min−1, which is 8.47 times that of photocatalysis over pure PCN. The doping of multiple heteroatoms in PCN not only speeds up the efficiency of carrier separation but also increases the adsorption/activation capacity of O2, thus improving the 2e− ORR of H2O2 synthesis. DFT calculation further proved that K, P, O-CN5 showed better O2 adsorption capacity. This work provides a new vision for PCN modification and water environmental treatment.
Conflicts of interest
There are no conflicts to declare.
Acknowledgements
We are grateful for the grants from the Natural Science Foundation of China (No. 51979081 and 52100179), the Fundamental Research Funds for the Central Universities (No. B210202052), the China Postdoctoral Science Foundation (No. 2020M680063 and 2021T140176), the Ministry of Education of Singapore (Tier 1: RG4/20 and Tier 2: MOET2EP10120-0002), the Agency for Science, Technology and Research (AME IRG: A20E5c0080) and PAPD.
References
- T. C. Liu, K. Yin, C. B. Liu, J. M. Luo, J. Crittenden, W. Q. Zhang, S. L. Luo, Q. Y. He, Y. X. Deng, H. Liu and D. Y. Zhang, Water Res., 2018, 147, 204–213 CrossRef CAS PubMed.
- L. J. Bu, S. Q. Zhou, Z. Shi, C. Bi, S. M. Zhu and N. Y. Cao, Sep. Purif. Technol., 2017, 178, 66–74 CrossRef CAS.
- H. Fenet, L. Arpin-Pont, A. Vanhoutte-Brunier, D. Munaron, A. Fiandrino, M. M. Bueno, C. Boillot, C. Casellas, O. Mathieu and E. Gomez, Environ. Int., 2014, 68, 177–184 CrossRef CAS PubMed.
- Z. Li, H. Fenet, E. Gomez and S. Chiron, Water Res., 2011, 45, 1587–1596 CrossRef CAS PubMed.
- W. Liu, P. F. Wang, Y. H. Ao, J. Chen, X. Gao, B. H. Jia and T. Y. Ma, Adv. Mater., 2022, 34, 2202508 CrossRef CAS PubMed.
- M. C. V. M. Starling, R. P. de M. Neto, G. F. F. Pires, P. B. Vilela and C. C. Amorim, Sci. Total Environ., 2021, 786, 147448 CrossRef PubMed.
- E. Cako, R. D. C. Soltani, X. Sun and G. Boczkaj, Chem. Eng. J., 2022, 439, 135354 CrossRef CAS.
- L. Ge, K. Moor, B. Zhang, Y. L. He and J. H. Kim, Nanoscale, 2014, 6, 13579–13585 RSC.
- Y. H. Sun, X. X. Chen, L. Liu, F. Xu and X. C. Zhang, Sci. Total Environ., 2021, 770, 145203 CrossRef CAS PubMed.
- C. H. Fang, H. L. Jia, S. Chang, Q. F. Ruan, P. Wang, T. Chen and J. F. Wang, Energy Environ. Sci., 2014, 7, 3431–3438 RSC.
- U. Ushani, X. Q. Lu, J. H. Wang, Z. Y. Zhang, J. J. Dai, Y. J. Tan, S. S. Wang, W. J. Li, C. X. Niu, T. Cai, N. Wang and G. Y. Zhen, Chem. Eng. J., 2020, 402, 126232 CrossRef CAS.
- N. López, S. Plaza, A. Afkhami, P. Marco, J. Giménez and S. Esplugas, Chem. Eng. J., 2017, 318, 112–120 CrossRef.
- J. Lee, U. V. Gunten and J. H. Kim, Environ. Sci. Technol., 2020, 54, 3064–3081 CrossRef CAS PubMed.
- Q. Li and F. T. Li, Chem. Eng. J., 2021, 421, 129915 CrossRef CAS.
- H. N. Che, X. Gao, J. Chen, J. Hou, Y. H. Ao and P. F. Wang, Angew. Chem., Int. Ed., 2021, 60, 25546–25550 CrossRef CAS PubMed.
- B. Zhu, B. Cheng, J. Fan, W. Ho and J. Yu, Small Struct., 2021, 2, 2100086 CrossRef CAS.
- W. Liu, P. F. Wang, J. Chen, X. Gao, H. N. Che, B. Liu and Y. H. Ao, Adv. Funct. Mater., 2022, 32, 2205119 CrossRef CAS.
- L. P. Xu, L. J. Li, L. Yu and J. C. Yu, Chem. Eng. J., 2022, 431, 134241 CrossRef CAS.
- Y. H. Zhao, D. X. Wang, H. N. Che, B. Liu and Y. H. Ao, Environ. Funct. Mater., 2022, 1, 316–324 Search PubMed.
- W. Ong, L. Tan, Y. Ng, S. Yong and S. Chai, Chem. Rev., 2016, 116, 7159–7329 CrossRef CAS PubMed.
- J. J. Liu, C. B. Xiong, S. J. Jiang, X. Wu and S. Q. Song, Appl. Catal., B, 2019, 249, 282–291 CrossRef CAS.
- Q. H. Zhu and J. L. Zhang, Environ. Funct. Mater., 2022, 1, 121–125 Search PubMed.
- S. D. Sohn, Y. H. Kim, S. C. Jung, J. S. Kang, H. J. Han, K. S. Kim, K. Park and H. J. Shin, ACS Catal., 2022, 12, 5990–5996 CrossRef CAS.
- Y. Deng and J. D. Englehardt, Water Res., 2006, 40, 3683–3694 CrossRef CAS PubMed.
- B. J. Dramou, V. Shahb and J. M. Pinto, Energy Environ. Sci., 2008, 1, 395–402 RSC.
- Y. Wu, H. N. Che, B. Liu and Y.H. Ao, Small Struct., 2023, 2200371 CrossRef.
- J. G. Song, H. T. Zhao, R. R. Sun, X. Y. Li and D. J. Sun, Energy Environ. Sci., 2017, 10, 225–235 RSC.
- S. Gao, X. Wang, C. Song, S. Zhou, F. Yang and Y. Kong, Appl. Catal., B, 2021, 295, 120272 CrossRef CAS.
- J. W. Fu, J. G. Yu, C. J. Jiang and B. Cheng, Adv. Energy Mater., 2018, 8, 1701503 CrossRef.
- G. F. Liao, Y. Gong, L. Zhang, H. Y. Gao, G. J. Yang and B. Z. Fang, Energy Environ. Sci., 2019, 12, 2080–2147 RSC.
- S. Y. Zhang, Y. Yang, Y. P. Zhai, J. Q. Wen, M. Zhang, J. K. Yu and S. Y. Lu, Chin. Chem. Lett., 2023, 34, 107652 CrossRef CAS.
- J. R. Ran, T. Y. Ma, G. P. Gao, X. W. Du and S. Z. Qiao, Energy Environ. Sci., 2015, 8, 3708–3717 RSC.
- R. F. Du, K. Xiao, B. Y. Li, X. Han, C. Q. Zhang, X. Wang, Y. Zuo, P. Guardia, J. S. Li, J. B. Chen, J. Arbiol and A. Cabot, Chem. Eng. J., 2022, 441, 135999 CrossRef CAS.
- M. Xie, J. C. Tang, L. S. Kong, W. H. Lu, V. Natarajan, F. Zhu and J. H. Zhan, Chem. Eng. J., 2019, 360, 1213–1222 CrossRef CAS.
- F. Li, M. Tang, T. Li, L. L. Zhang and C. Hu, Appl. Catal., B, 2020, 268, 118397 CrossRef CAS.
- S. Y. Chen, J. P. Yu, Z. F. Chai, W. Q. Shi and L. Y. Yuan, Chem. Eng. J., 2023, 460, 141742 CrossRef CAS.
- J. N. Li, J. Chen, Y. H. Ao, X. Gao, H. N. Che and P. F. Wang, Sep. Purif. Technol., 2022, 281, 119863 CrossRef CAS.
- X. Y. Wang, L. B. Sang, L. Zhang, G. Yang, Y. H. Guo and Y. X. Yang, J. Alloys Compd., 2023, 941, 168921 CrossRef CAS.
- C. Yang, Y. T. Hou, G. Q. Luo, J. G. Yua and S. W. Cao, Nanoscale, 2022, 14, 11972–11978 RSC.
- C. Adamo and V. Barone, J. Chem. Phys., 1999, 110, 6158–6170 CrossRef CAS.
- S. Grimme, S. Ehrlich and L. Goerigk, J. Comput. Chem., 2011, 32, 1456–1465 CrossRef CAS PubMed.
- F. Weigend and R. Ahlrichs, Phys. Chem. Chem. Phys., 2005, 7, 3297–3305 RSC.
- Z. Liu, T. Lu and Q. Chen, Carbon, 2020, 165, 461–467 CrossRef CAS.
- T. Lu and F. Chen, J. Comput. Chem., 2012, 33, 580–592 CrossRef CAS PubMed.
- W. Humphrey, A. Dalke and K. Schulten, J. Mol. Graphics, 1996, 14, 33–38 CrossRef CAS PubMed.
- L. Goerigk and S. Grimme, J. Chem. Theor. Comput., 2010, 7, 291–309 CrossRef PubMed.
- F. Neese, Science, 2018, 8, 1327 Search PubMed.
- Z. F. Chen, S. C. Lu, Q. L. Wu, F. He, N. Q. Zhao, C. N. He and C. S. Shi, Nanoscale, 2018, 10, 3008–3013 RSC.
- Y. Wu, J. Chen, H. N. Che, X. Gao, Y. H. Ao and P. F. Wang, Appl. Catal., B, 2022, 307, 121185 CrossRef CAS.
- L. H. Lin, C. Wang, W. Ren, H. H. Ou, Y. F. Zhang and X. C. Wang, Chem. Sci., 2017, 8, 5506–5511 RSC.
- Y. Y. Wen, J. Chen, X. Gao, W. Liu, H. N. Che, B. Liu and Y. H. Ao, Nano Energy, 2023, 107, 108173 CrossRef CAS.
- H. N. Che, P. F. Wang, J. Chen, X. Gao, B. Liu and Y. H. Ao, Appl. Catal., B, 2022, 316, 121611 CrossRef CAS.
- Y. J. Zhou, L. X. Zhang, W. M. Huang, Q. L. Kong, X. Q. Fan, M. Wang and J. L. Shi, Carbon, 2016, 99, 111–117 CrossRef CAS.
- Y. Xia, B. Cheng, J. J. Fan, J. G. Yu and G. Liu, Small, 2019, 15, 201902459 CrossRef PubMed.
- W. Yan, Y. Yu, H. H. Zou, X. F. Wang, P. Li, W. Y. Gao, J. Z. Wang, S. M. Wu and K. J. Ding, Sol. RRL, 2018, 2, 1800058 CrossRef.
- G. D. Liu, H. Q. Deng, J. Greeley and Z. H. Zeng, Chin. J. Catal., 2022, 43, 3126–3133 CrossRef CAS.
- Q. Zhang, J. Chen, X. Gao, H. N. Che, P. F. Wang and Y. H. Ao, Appl. Catal., B, 2018, 239, 578–585 CrossRef.
- Q. Zhang, J. Chen, X. Gao, H. N. Che, Y. H. Ao and P. F. Wang, J. Hazard. Mater., 2022, 430, 128386 CrossRef CAS PubMed.
- K. Zhang, L. Y. Wang, X. W. Sheng, M. Ma, M. S. Jung, W. J. Kim, H. Y. Lee and J. H. Park, Adv. Energy Mater., 2016, 6, 1502352 CrossRef.
- S. P. Wan, C. R. Dong, J. Jin, J. Li, Q. Zhong, K. Zhang and J. H. Park, ACS Energy Lett., 2022, 7, 3024 CrossRef CAS.
- Q. Zhang, J. Chen, X. Gao, H. N. Che, P. F. Wang, B. Liu and Y. H. Ao, Sep. Purif. Technol., 2022, 300, 121947 CrossRef CAS.
- H. N. Che, J. Wang, X. Gao, J. Chen, P. F. Wang, B. Liu and Y. H. Ao, J. Colloid Interface Sci., 2022, 627, 739–748 CrossRef CAS PubMed.
|
This journal is © The Royal Society of Chemistry 2023 |