DOI:
10.1039/D2NR05850B
(Communication)
Nanoscale, 2023,
15, 2036-2043
Piezoelectricity-modulated optical recombination dynamics of monolayer-MoS2/GaN-film heterostructures†
Received
21st October 2022
, Accepted 3rd December 2022
First published on 5th December 2022
Abstract
Dynamic manipulation of optoelectronic responses by mechanical stimuli is promising for developing wearable electronics and human–machine interfacing. Although 2D–3D hybrid heterostructures can bring advancements in optoelectronics, their dynamic optical responses to external strains remain rarely studied. Here, we demonstrate the strain-tuned recombination dynamics of monolayer-MoS2 and thin-film-GaN heterostructures. We find that optical excitons in the heterostructures, apart from trions, can be markedly modulated by strains. We argue that MoS2 piezoelectric dipoles across the interfaces lead to curved band diagrams, in which optical excitons dissociate into spatially separated quasi-particles and concurrently relocate to the maxima of valence bands and the minima of conduction bands. With the increase in tensile strains, the photoluminescence (PL) intensity of the heterostructures shows quenched responses. Noticeably, the change in PL spectra strongly depends on the directions of the applied strains because of the lateral piezoelectric periodicity of MoS2 flakes. This work not only helps in understanding the underlying physics of the decreased PL intensities upon applying strains but also demonstrates a feasible way (i.e., strains) to manipulate the PL efficiency of 2D-material-based optoelectronics.
Two-dimensional (2D) materials, including ultrathin MoS2, exhibit remarkable physical properties, unlike those of the bulk. For example, monolayer MoS2 pertains to a D3h space group and lacks inversion symmetry;1,2 the Coulomb interaction between electrons and holes is largely enhanced in monolayer MoS2.3 Furthermore, a direct bandgap of ∼1.85 eV, which lies in the spectral range of visible light, endows monolayer MoS2 with intriguing optical properties.4–8 A broad range of ultrathin MoS2 optoelectronics have been developed, including photodetectors,9–15 photovoltaics,16–19 LEDs,20–24 and photoelectrochemical cells.25,26 Monolayer MoS2 features a hexagonal crystal structure and it is non-centrosymmetric, thus demonstrating strong piezoelectricity along the e11 direction.27 Unfortunately, piezoelectricity emerges only from odd-numbered layers of MoS2 crystals but disappears in even-numbered layers.28,29 Moreover, piezoelectric-MoS2-based self-adaptive piezophototronic devices offer a possible way to develop flexible nano-opto-electromechanical systems.30,31 The inherent piezoelectricity (i.e., piezoelectric field), triggered by a high-frequency acoustic wave, has been used to tune the photoluminescence (PL) efficiency of monolayer MoS2 grown on a sapphire substrate.32
2D-material-based heterostructures provide a new platform for exploring rich physics and device applications. Vertical stacking of ultrathin MoS2 semiconductors with traditional bulk materials has received considerable attention due to the combination of both 2D and 3D merits.33–35 The 2D–3D assembly can not only help us to realize high-performance electronics/optoelectronics but also overcome technological challenges in silicon scaling.20,36,37 Using the piezoelectricity of ZnO thin films, the photocurrent in a MoS2–ZnO 2D–3D heterojunction photodiode has been enhanced four times by the piezophototronic effect.38 However, to date, whether the strain-induced piezoelectric field in heterostructures such as monolayer MoS2 could regulate photo-excited dynamic responses in these vertically integrated 2D–3D heterostructures remains rarely studied.
Monolayer MoS2 shows a small lattice mismatch with GaN films,39 and the hybrid of these two materials is promising for high-performance optoelectronics. Here, using the vertically stacked monolayer-MoS2/GaN-film heterostructures as a model system, we study the piezoelectricity-modulated carrier recombination dynamics. Compared to separate monolayer MoS2, the PL spectra of the heterostructures show a remarkably decreased weight of trion X− and exciton B, which is associated with charge transfer over the interfaces. When tensile strains are applied to the heterostructures, the 2S-Mo piezoelectric dipoles lead to a bent energy band. The excitons dissociate into spatially separated quasi-particles and are stored in the maxima and minima of valence and conduction bands. Accordingly, with the increase of in-plane strain magnitude, the PL peaks related to MoS2 distinctly decrease and the variation strongly depends on the in-plane directions of the applied strain due to the periodic in-plane MoS2 piezoelectricity. This work helps us to illustrate the underlying physical mechanisms of strain-dependent quenching of PL spectra for 2D heterostructure optoelectronics.
Evolution of the Raman and PL spectra at different temperatures
As shown in Fig. 1a, exfoliated n-type MoS2 flakes on silica substrates were transferred to p-type GaN films, forming vertically stacked p–n 3D–2D heterostructures. Temperature-dependent Raman spectra were used to study the evolution of heterostructure photon vibration. In Fig. 1b, typical Raman signals of p-type GaN films at room temperature are presented to help identify the origin of the whole heterostructure spectra. Two strong Raman peaks, located at 570 cm−1 and 735 cm−1, are observed because of the contributions from E2 and A1 longitudinal optical modes in GaN films.40 Moreover, a small peak is detected at 418 cm−1, which originates from the sapphire substrate as previously reported.41Fig. 1c shows the temperature-dependent Raman spectra for monolayer-MoS2/GaN heterostructures. It is seen that Raman responses from the heterostructures are superimposed by those from MoS2 flakes and GaN films. Raman scattering intensities of in-plane E2g1 and out-of-plane A1g1 modes associated with MoS2 flakes are relatively weak and the width between those active modes remains 19 cm−1, which is in agreement with previous works.42,43 With the increase in temperature, all Raman peaks exhibit a redshift and the width for E2g1 and A1g1 remains constant as shown in Fig. 1c and S1.† The temperature-induced variation at the Raman peak position is mainly attributed to thermal expansion.44 To systematically examine the variation of photon vibration for heterostructures with different layered MoS2, the temperature-dependent Raman spectra acquired for four-layer-MoS2/GaN and bulk-MoS2/GaN heterostructures are shown in Fig. 1d and e. As highlighted in Fig. 1d and e, the widths of two significant MoS2 peaks are 23 cm−1 and 25 cm−1 for four-layer and bulk heterostructures, respectively. When the temperatures are dramatically increased, the redshift trends for the two heterostructures coincide with that for monolayer-MoS2/GaN heterostructures. It is noted that compared to the Raman spectra shown in Fig. 1c, the emission peaks related to MoS2 are largely intensified, while the peaks related to GaN film substrate weaken. This is caused by the increased thickness of MoS2 and agrees with the variation of separate MoS2.44
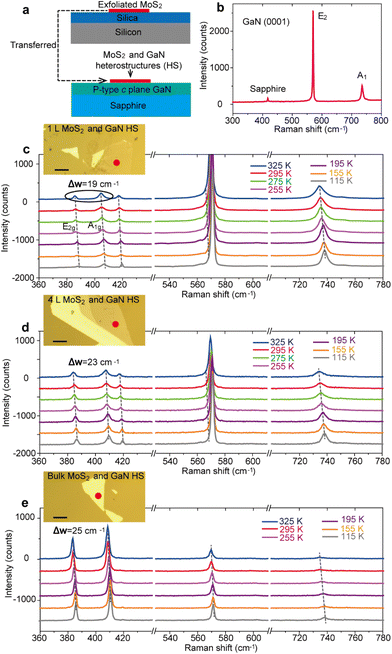 |
| Fig. 1 Raman spectra of MoS2/GaN heterostructures. (a) Schematics of the fabrication of vertically stacked MoS2/GaN heterostructures. Exfoliated MoS2 flakes on silica were transferred to the GaN film by a wet chemical method. (b) Typical Raman spectra for GaN films with the c plane at room temperature. Temperature-dependent Raman spectra obtained for the heterostructures consisting of (c) 1-layer, (d) 4-layer, and (e) bulk MoS2. With the increase in temperature, all Raman active peaks exhibit a red shift. Raman spectra were excited by a 532 nm laser and the red dots in the insets of (c)–(e) represent the photoexcited spots. Scale bar: 10 μm. | |
Next, we turn to the characterization of photoexcited dynamics of MoS2/GaN heterostructures by PL spectra. For comparison, Fig. 2a shows the PL responses of separate monolayer MoS2 on silica substrates. Due to the strong spin–orbit coupling in monolayer MoS2, valence bands at the K points of the Brillouin zone can split, giving rise to two prominent direct-bandgap transitions (i.e., excitons A and B) as shown in the inset of Fig. 2a.2 Experimentally, the PL spectra of monolayer MoS2 exhibit two significant peaks (A and B). However, reduced dielectric screening in a monolayer crystal facilitates enhanced Coulomb interaction between holes and electrons. Thus, tightly bound excitons or trions with high binding energy easily form,45 which dominate the optical properties of ultrathin MoS2 flakes3 and play an important role in the photo-excited processes as described below. Fig. 2b shows PL emission peaks between 1.7 and 2.1 eV from monolayer-MoS2/GaN heterostructures, while the inset exhibits the schematic of exciton (X) and trion (X−) creation in MoS2 crystals. As indicated by the orange arrow, a sharp and strong PL signal at 1.78 eV denotes the sapphire-substrate-related emission peak (Fig. S2†). When the n-type monolayer MoS2 and p-type GaN films are assembled, charge transfer inevitably occurs,46 leading to a decreased PL emission (Fig. 2b). A small PL blueshift (∼22 meV) can be detected, possibly arising from the doping effect from the GaN substrate.47 To give a deep insight into the spectral changes shown in Fig. 2a and b, the emission peaks are decomposed into a negative trion (X−), neutral exciton (X), and high-energy exciton peaks (B) through the Lorentzian function.4 Compared to the PL spectra of separate monolayer MoS2, it is apparent that the spectral weights of the negative trion X− and exciton B are greatly reduced (the weight of exciton B nearly becomes zero), while that of exciton X is increased. This confirms the presence of charge transfer between the n-type MoS2 flake and the p-type GaN film.
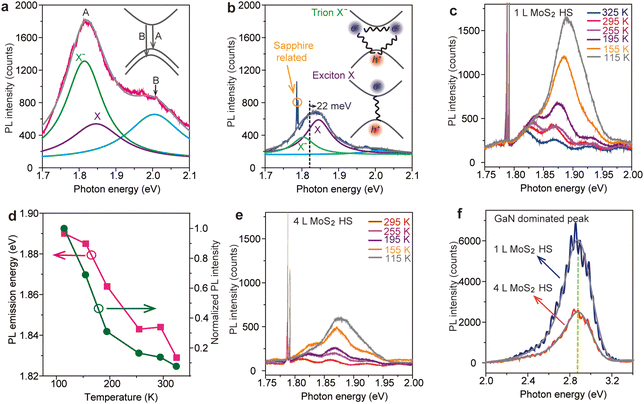 |
| Fig. 2 PL spectra of typical MoS2/GaN heterostructures. PL responses at ∼1.8 eV, which were collected from (a) separate monolayer MoS2 on silica substrates and (b) monolayer-MoS2/GaN heterostructures using a 100× objective lens at room temperature. The inset in (a) shows A and B excitons generated by valence band splitting in monolayer MoS2, whereas the schematics of excitons and trions are shown in the inset of (b). PL spectra were deconvoluted to show the change in neutral excitons (X), charged trions (X−), and neutral excitons (B) using Lorentzian functions. Temperature-dependent PL spectra (c) and the relevant derived features (d), such as peak energy and peak height of the 1-layer-MoS2/GaN heterostructure. The oscillating PL signals described here were collected through a 50× objective lens in the measurement system. (e) PL spectra of the 4-layer-MoS2/GaN heterostructures at different temperatures. (f) GaN-dominated PL spectra for the vertical heterostructures at room temperature. The fitting curves are indicated by the grey lines. The MoS2-related emission peaks are excited by a 532 nm laser, while the GaN-related emission peak is excited by a 325 nm laser. | |
To explore the evolution of exciton and trion recombination, the temperature-dependent PL spectra of monolayer-MoS2/GaN heterostructures were collected (Fig. 2c). Note that during the measurement a long-focus lens (50× objective) was used to leave a large space for applying strains. PL signal oscillation, especially at high temperatures, possibly stems from the heat effect and the interference of light at the MoS2/GaN interface. When the temperature is decreased, PL spectra exhibit a blueshift from 1.83 eV to 1.89 eV (Fig. 2d), which is associated with the lattice shrinking effect. The peak intensity is markedly increased owing to the enhanced exciton recombination as quantitatively presented in Fig. 2d (the data are derived from the fitting lines of Fig. 2c). Fig. S3† shows the fitting curves of the temperature-dependent PL spectra using the Lorentzian function. To discuss the MoS2-thickness-dependent PL emission from MoS2/GaN heterostructures, 4-layer-MoS2 was adopted. It is observed that the PL intensity of 4-layer-MoS2/GaN heterostructures at different temperatures (Fig. 2e) is greatly decreased compared with that of the monolayer MoS2 counterparts (Fig. 2c). Furthermore, Fig. 2f and S4† show another PL emission peak between 2.0 and 3.3 eV for different-layer-MoS2/GaN heterostructures, and this peak corresponds to GaN-dominated responses. The PL intensity is expectedly weakened, which contrasts with separate GaN samples without top MoS2 layers and verifies charge transfer across the vertical heterostructures. Note that the peak oscillation stems from the Fabry–Perot optical interference within the GaN film.48
External strain-tuned recombination dynamics
Next, we examine strain-dependent PL responses for monolayer-MoS2/GaN heterostructures. In the experiment, one edge of the substrate was tightly clamped on a right-angle bracket using a hexagonal nut (Fig. 3a); heterostructure samples were placed just above the edge of the bracket to ensure that they could receive the maximum strain. A 3D stage with a resolution of 10 μm was used to drive another right-angle bracket to apply tensile strains on the samples. From the stage, the largest displacement distance (Dmax) can be obtained to estimate the magnitude of the applied strains. Fig. 3b shows the optical image of our practical testing set-up. The strain direction orientation along one edge of the trigonal MoS2 flake is defined as 0°, as indicated by the grey dashed arrow highlighted in the optical image of the monolayer-MoS2/GaN heterostructure (the right inset shown in Fig. 3b). Fig. 3c plots PL responses with different tensile strains and strain directions (e.g., 0° and 30°; the scenario of 45° is presented in Fig. S5†). As the strain magnitude increases, PL signals show a quenching behavior and a slight red shift induced by the piezoresistance effect. Note that the strain magnitude described here is much smaller than that applied to the flexible substrate, which is because 2D materials have a good contact with the rigid sapphire and the strain can be well transmitted to the sample. To quantify photoexcited dynamics upon the application of strains, it is necessary to fit the raw PL spectra in Fig. 3c with smooth curves using the Lorentzian function (Fig. S6†) and then de-convolute those fitting curves to demonstrate the variations of optically induced excitons and trions (Fig. 3d). As for the strain direction of 0°, when the strain magnitude is increased, the peak intensity of trion X− shows no obvious changing trends as the grey fitting line indicates, yet the peak intensity of exciton X rapidly decreases in a linear relationship with strains. In the case of 30°, the variation in trions also shows no obvious change, while that of exciton X first decreases and then reaches saturation.
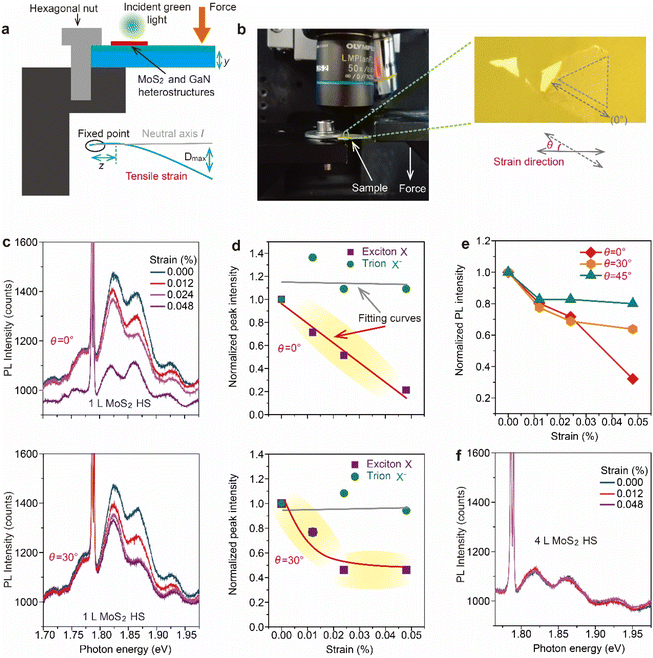 |
| Fig. 3 PL intensity of monolayer-MoS2/GaN heterostructures as a function of tensile strain. (a) Schematic cross-sectional view and (b) practical microscopy of our testing set-up. A 3D stage was employed to apply external forces on the substrates for introducing tensile strain on the heterostructure as the red arrow indicates. One edge of monolayer MoS2 is defined as 0° for the tensile strains (the inset of (b)). (c) PL responses to a function of the applied strains on monolayer-MoS2/GaN heterostructures with the strain directions of 0° and 30°, respectively. As the strain increases, both the PL intensities remarkably decrease. (d) The changes in optical excitons and trions with respect to the applied strains. (e) Quantitative comparison of the relationships between PL peak intensities and tensile strains in different strain directions. The PL intensities at the directions of 0°, 30°, and 45° are normalized by the corresponding peaks without tensile strain. (f) PL spectra related to the applied strains for 4-layer-MoS2/GaN heterostructures. Owing to the absence of the piezoelectric effect on 4-layer MoS2, there is no obvious change in the PL responses. | |
To sum up, for vertically stacked heterostructures, when suffering tensile strains, the weight of excitons shows remarkable reduction but the weight of trions remains unchanged. Fig. 3e systematically depicts the normalized PL intensities as a function of the applied strain directions for monolayer-MoS2/GaN heterostructures. Upon applying a small strain, the peak variation at different strain directions is almost identical. However, upon applying a large strain, the variation exhibits discrepancies arising from the angle-dependent piezoelectric field in monolayer MoS2 (see Fig. 5 for more details): PL intensity at 0° rapidly decreases but the intensities at 30° and 45° slowly drop with different rates. In contrast, 4-layer-MoS2/GaN heterostructures (Fig. 3f) demonstrate weak PL signals and no dependence on strains.
With regard to another emission peak (∼2.84 eV, dominated by GaN films) from monolayer-MoS2/GaN heterostructures (Fig. 4a) and separate GaN films (Fig. S7†), the applied strains have no obvious impact on the relevant PL spectra. This validates that the strain-induced piezoelectric field in the GaN film would not affect optical recombination. As discussed in Fig. 3, once MoS2/GaN stacking forms, photoexcited dynamic responses at 1.75–1.95 eV are predominately determined by MoS2 flakes. Therefore, considering the angle dependence of the quenched PL responses in monolayer-MoS2/GaN stacking and the almost unchanged PL signals in the four-layer-MoS2/GaN stacking, we attribute this quenched behavior to intrinsic MoS2 piezoelectricity. Furthermore, we also obtained the suppressed PL intensity from flexible MoS2 devices, in which monolayer MoS2 was directly transferred on the PET substrates. Fig. 4b shows a typical optical microscopic image of monolayer MoS2 and the inset displays a pertinent practical flexible device. Fig. 4c presents the PL spectra of a monolayer MoS2 flake on the flexible PET substrate with respect to tensile strains. With the increase in the strain magnitude, PL spectra exhibit quenching responses and a slight red shift, suggesting that without heterostructure stacking (the piezoelectricity in the GaN film) the observed behaviors still exist. The documented work with SU8 as a flexible substrate reported that, as the strains were applied, monolayer MoS2 showed a significant decrease in the PL intensity while the PL intensity of the bilayer was indeed strain independent.49 The authors attribute this quenching phenomenon to strain-induced transformation between direct and indirect optical band gaps in monolayer MoS2. Nonetheless, a key question arises: how do the applied strains affect the optical-bandgap transformation? Here, as for either flexible monolayer MoS2 devices or our MoS2/GaN heterostructures, we propose that piezoelectricity in monolayer MoS2 regulates the transformation (i.e., decreased PL behaviors).
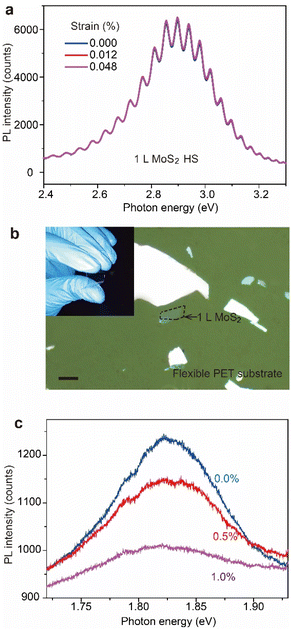 |
| Fig. 4 Contrastive experiments to confirm that the quenched responses result from the MoS2 piezoelectricity in heterostructures. (a) PL spectra dominated by GaN films under different applied strains for monolayer-MoS2/GaN heterostructures. As the magnitude of the external strain increases, the related emission peaks show no obvious change. (b) Optical microscopy of monolayer MoS2 on a flexible PET substrate. The inset shows a typical flexible device. Scale bar: 10 μm. (c) PL spectra with respect to different applied tensile strains for the mentioned flexible device in (b). The quenched response is also observed, manifesting that the piezoelectricity in the MoS2 flake governs the photo-excited process. | |
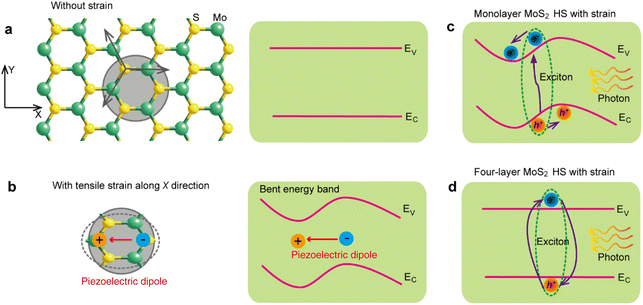 |
| Fig. 5 Crystal structure and band diagrams to interpret the underlying physics for the quenched PL responses. (a) Hexagonal crystal structure of monolayer MoS2 viewing from the top without strain, and the relevant energy band. The directions of x and y denote ‘armchair’ and ‘zigzag’ edges of MoS2, respectively. The piezoelectric field exists along the direction pointing from the S site to the Mo site, namely, the e11 direction as indicated by grey arrows. (b) As the semiconductor is elongated, the tensile stress in the x direction and the compressive stress in the y direction are created, simultaneously resulting in the displacement of the Mo and S atoms and producing a net piezoelectric dipole as indicated by the red arrow. The immobile positive and negative ions among the piezoelectric dipole will make the bands bend downward and upward. Superposition of the generated net Mo–S piezoelectric dipoles along the X direction gives rise to a strong piezoelectric field. (c) Due to the inherent piezoelectric field, optical excitons will dissociate into spatially separated e–h quasi-particles and be stored in the bent energy band. (d) No modulation under applied strains in the absence of the piezoelectricity for four-layer MoS2 heterostructures. | |
Interpretation of the underlying physics
Fig. 5 elucidates how the piezoelectricity in MoS2 flakes influences the optical recombination dynamics of monolayer-MoS2/GaN heterostructures or separate monolayer MoS2. The hexagonal crystal structure of monolayer MoS2 and the relevant simplified energy band structure are shown in Fig. 5a. We define the x and y directions along the ‘armchair’ and ‘zigzag’ edges of monolayer MoS2, respectively. In principle, as the solid grey arrows indicated, the produced piezoelectric fields point from S to Mo sites in the xy plane, namely, the e11 direction.28,29 A hexagonal structural unit is chosen for analysis, as indicated by the big grey circle highlighted in Fig. 5a. When applying a tensile strain along the x direction, the unit suffers a deformation: tensile stress in x and compressive stress in y are created. Meanwhile, Mo and S atoms produce relative displacements and piezoelectric dipoles.50 These dipoles along the x direction are superimposed, whereas those along other directions offset each other. Therefore, net piezoelectric dipoles can be generated, which orient from the negative S site to the positive Mo site, as shown in the left-hand side image of Fig. 5b.
In the right-hand side image of Fig. 5b, within a piezoelectric dipole, the immobile positive ion attracts the free electrons across the monolayer MoS2 to aggregate around it, leading to a decrease in the electrical potential energy and bandgap; in contrast, the negative ion compels the free electrons to move away from the dipole, resulting in an increase in the electrical potential energy and bandgap. Additionally, a strong piezoelectric field introduced by the net piezoelectric dipole moment along the direction of the applied tensile strain (x direction) can appear, which constitutes a driving force for the dissociation of tightly bound excitons. When a ray of laser is applied on the strained monolayer-MoS2/GaN heterostructures (or MoS2 flexible devices), a majority of optically induced excitons will dissociate, spatially separate, and be stored in the maxima and minima of valence and conduction bands, as displayed in Fig. 5c. This can explain the observed decrease in PL intensity. Because of the periodic piezoelectric field as indicated in Fig. 5a, the samples exhibit different strain tuning effects at different orientations, as shown in Fig. 3e. However, when removing the applied strains, this piezoelectric modulation effect disappears (Fig. S8†), clearly demonstrating that temporarily separated electron–hole pairs (quasi-particles) exist and will recover to recombination excitons. Because of the lack of piezoelectricity in the four-layer MoS2, the applied strain does not have any influence on the energy band of MoS2 (Fig. 5d), and thus the PL responses for the four-layer-MoS2/GaN heterostructures.
In conclusion, we have investigated piezoelectricity-tuned optical dynamics of atom-thick-MoS2 and thin-film-GaN heterostructures. With the increase in temperatures, all Raman active modes and PL spectra show a red shift, yet the PL intensities show a decrease due to the thermal expansion. As the magnitude of the applied tensile strain increases, the corresponding PL spectra show quenched responses mainly associated with the markedly decreased weight of exciton X. We ascribe this phenomenon to the piezoelectricity in monolayer MoS2. Within strain-induced piezoelectric dipoles, the positive and negative immobile ions can render MoS2 energy bands bending downward and upward, respectively. Consequently, a majority of optically induced excitons will spatially separate and then store in the maxima and minima of valence and conduction bands, thereby resulting in our observed behaviors. In addition, because of the lateral periodic piezoelectricity in MoS2 flakes, the changing trend of PL emission peaks can be determined by strain directions.
We believe that strain is a general approach to tune optical recombination dynamics via the piezoelectric effect51 and can be applied to other material systems, such as 2D piezoelectric SnS and ZnO.52,53 This work not only helps in interpreting the underlying mechanism of strain-dependent PL spectra of piezoelectric materials, but also provides a technical route to control the performances of optoelectronics by strain and develop tactile adaptive optoelectronics.
Experimental methods
Preparation of MoS2/GaN heterostructures
MoS2 samples were exfoliated from bulk crystals (bought from SPI) using mechanical methods and then transferred onto silicon substrates with 300 nm silica layers. After identifying the thicknesses by optical microscopy and Raman spectra, the chosen samples were transferred to GaN films grown on a sapphire substrate, using the wet chemical method as follows. First, PMMA (A3) was uniformly coated on the samples and baked at 170 °C for 10 min. Second, the KOH solution with a mass concentration of 10% was heated to 80 °C for boosting the etching rate of the oxide layer. Meanwhile, the samples covered with PMMA were soaked in the prepared KOH solution. Third, when floating on the top surface of deionized water, the PMMA layers, carried with target samples, were slowly picked up and successfully transferred to GaN films. Fourth, the sacrificed PMMA layers were dissolved in acetone. Note that the p-type GaN films, with thicknesses of 4–5 μm, were Mg-doped and grown by the MOCVD method with a carrier density of 6 × 1016 cm−3.
Raman and PL measurements
The temperature testing setup was a HFS600E-PB4 temperature-controlled probe stage brought from Linkam Scientific Instruments. The Raman and PL spectra were measured using a Horiba LABRAM-HR evolution microscope. The experimental data in Fig. 2a and b were collected using a short-focus objective lens (100×) to ensure that the CCD can receive more emission photons from the samples. A UV objective lens (40×) was employed to obtain the data shown in Fig. 2f, and a long-focus objective lens (50×) was used to conduct the rest of the experiments for conveniently applying the variations in temperature and strain. The 532 nm laser with a power of 6 mW was used to perform the Raman characterizations and related PL responses. In addition, a UV laser with a 325 nm wavelength was employed to excite the GaN films as shown in Fig. 2f.
Estimation of the strain induced in MoS2 and GaN heterostructures
Due to the strong van der Waals forces between atomically thin MoS2 and GaN films, the MoS2 flake will follow the deformations of the GaN substrates under the applied strains. The applied tensile strain (ε) can be estimated using the following equation:28
where y, l, z and Dmax are all labeled in Fig. 3a.
Data availability
The data supporting the findings of this study are available within the article and the supplementary files are available from the authors upon reasonable request.
Conflicts of interest
The authors declare no competing interests.
Acknowledgements
This research was supported by the ZJU-Hangzhou Global Scientific and Technological Innovation Center with a startup funding (02170000-K02013012).
References
- R. Ganatra and Q. Zhang, ACS Nano, 2014, 8, 4074–4099 CrossRef CAS PubMed.
- K. F. Mak, C. Lee, J. Hone, J. Shan and T. F. Heinz, Phys. Rev. Lett., 2010, 105, 136805 CrossRef PubMed.
- A. Chernikov, T. C. Berkelbach, H. M. Hill, A. Rigosi, Y. Li, B. Aslan, D. R. Reichman, M. S. Hybertsen and T. F. Heinz, Phys. Rev. Lett., 2014, 113, 076802 CrossRef CAS PubMed.
- S. Mouri, Y. Miyauchi and K. Matsuda, Nano Lett., 2013, 13, 5944–5948 CrossRef CAS PubMed.
- H. Nan, Z. Wang, W. Wang, Z. Liang, Y. Lu, Q. Chen, D. He, P. Tan, F. Miao, X. Wang, J. Wang and Z. Ni, ACS Nano, 2014, 8, 5738–5745 CrossRef CAS PubMed.
- M. Amani, D.-H. Lien, D. Kiriya, J. Xiao, A. Azcatl, J. Noh, S. R. Madhvapathy, R. Addou, K. C. Santosh, M. Dubey, K. Cho, R. M. Wallace, S.-C. Lee, J.-H. He, J. W. Ager III, X. Zhang, E. Yablonovitch and A. Javey, Science, 2015, 350, 1065–1068 CrossRef CAS PubMed.
- D.-H. Lien, S. Z. Uddin, M. Yeh, M. Amani, H. Kim, J. W. Ager III, E. Yablonovitch and A. Javey, Science, 2019, 364, 468–471 CrossRef CAS PubMed.
- Y. Yamada, K. Shinokita, Y. Okajima, S. N. Takeda, Y. Matsushita, K. Takei, T. Yoshimura, A. Ashida, N. Fujimura, K. Matsuda and D. Kiriya, ACS Appl. Mater. Interfaces, 2020, 12, 36496–36504 CrossRef CAS PubMed.
- D.-S. Tsai, K.-K. Liu, D.-H. Lien, M.-L. Tsai, C.-F. Kang, C.-A. Lin, L.-J. Li and J.-H. He, ACS Nano, 2013, 7, 3905–3911 CrossRef CAS PubMed.
- O. Lopez-Sanchez, D. Lembke, M. Kayci, A. Radenovic and A. Kis, Nat. Nanotechnol., 2013, 8, 497–501 CrossRef CAS PubMed.
- Y. Pang, F. Xue, L. Wang, J. Chen, J. Luo, T. Jiang, C. Zhang and Z. L. Wang, Adv. Sci., 2016, 3, 1500419 CrossRef PubMed.
- G. A. Saenz, G. Karapetrov, J. Curtis and A. B. Kaul, Sci. Rep., 2018, 8, 1276 CrossRef PubMed.
- H.-Y. Lan, Y.-H. Hsieh, Z.-Y. Chiao, D. Jariwala, M.-H. Shih, T.-J. Yen, O. Hess and Y.-J. Lu, Nano Lett., 2021, 21, 3083–3091 CrossRef CAS PubMed.
- N. Li, C. He, Q. Wang, J. Tang, Q. Zhang, C. Shen, J. Tang, H. Huang, S. Wang, J. Li, B. Huang, Z. Wei, Y. Guo, J. Yuan, W. Yang, R. Yang, D. Shi and G. Zhang, Nano Res., 2022, 15, 5418–5424 CrossRef CAS.
- Y. Li, L. Li, S. Li, J. Sun, Y. Fang and T. Deng, ACS Omega, 2022, 7, 13615–13621 CrossRef CAS PubMed.
- M.-L. Tsai, S.-H. Su, J.-K. Chang, D.-S. Tsai, C.-H. Chen, C.-I. Wu, L.-J. Li, L.-J. Chen and J.-H. He, ACS Nano, 2014, 8, 8317–8322 CrossRef CAS PubMed.
- S. Wi, H. Kim, M. Chen, H. Nam, L. J. Guo, E. Meyhofer and X. Liang, ACS Nano, 2014, 8, 5270–5281 CrossRef CAS PubMed.
- C.-J. Park, H. J. Park, J. Y. Lee, J. Kim, C.-H. Lee and J. Joo, ACS Appl. Mater. Interfaces, 2018, 10, 29848–29856 CrossRef CAS PubMed.
- K. M. Islam, T. Ismael, C. Luthy, O. Kizilkaya and M. D. Escarra, ACS Appl. Mater. Interfaces, 2022, 14, 24281–24289 CrossRef CAS PubMed.
- Y. Ye, Z. Ye, M. Gharghi, H. Zhu, M. Zhao, Y. Wang, X. Yin and X. Zhang, Appl. Phys. Lett., 2014, 104, 193508 CrossRef.
- M. Choi, Y. J. Park, B. K. Sharma, S.-R. Bae, S. Y. Kim and J.-H. Ahn, Sci. Adv., 2018, 4, eaas8721 CrossRef PubMed.
- Y. Woo, W. Hong, S. Y. Yang, H. J. Kim, J.-H. Cha, J. E. Lee, K. J. Lee, T. Kang and S.-Y. Choi, Adv. Electron. Mater., 2018, 4, 1800251 CrossRef.
- H. Kwon, S. Garg, J. H. Park, Y. Jeong, S. Yu, S. M. Kim, P. Kung and S. Im, npj 2D Mater. Appl., 2019, 3, 9 CrossRef.
- M. Choi, S.-R. Bae, L. Hu, H. Anh Tuan, S. Y. Kim and J.-H. Ahn, Sci. Adv., 2020, 6, eabb5898 CrossRef CAS PubMed.
- A. Alarawi, V. Ramalingam, H. C. Fu, P. Varadhan, R. S. Yang and J. H. He, Opt. Express, 2019, 27, A352–A363 CrossRef CAS PubMed.
- A. Alarawi, V. Ramalingam and J.-H. He, Mater. Today Energy, 2019, 11, 1–23 CrossRef CAS.
- K.-A. N. Duerloo, M. T. Ong and E. J. Reed, J. Phys. Chem. Lett., 2012, 3, 2871–2876 CrossRef CAS.
- W. Wu, L. Wang, Y. Li, F. Zhang, L. Lin, S. Niu, D. Chenet, X. Zhang, Y. Hao, T. F. Heinz, J. Hone and Z. L. Wang, Nature, 2014, 514, 470–474 CrossRef CAS PubMed.
- H. Zhu, Y. Wang, J. Xiao, M. Liu, S. Xiong, Z. J. Wong, Z. Ye, Y. Ye, X. Yin and X. Zhang, Nat. Nanotechnol., 2015, 10, 151–155 CrossRef CAS PubMed.
- W. Wu, L. Wang, R. Yu, Y. Liu, S.-H. Wei, J. Hone and Z. L. Wang, Adv. Mater., 2016, 28, 8463–8468 CrossRef CAS PubMed.
- Y. Zhang, W. Jie, P. Chen, W. Liu and J. Hao, Adv. Mater., 2018, 30, 1707007 CrossRef PubMed.
- A. R. Rezk, B. Carey, A. F. Chrimes, D. W. M. Lau, B. C. Gibson, C. Zheng, M. S. Fuhrer, L. Y. Yeo and K. Kalantar-zadeh, Nano Lett., 2016, 16, 849–855 CrossRef CAS PubMed.
- M. Moun, M. Kumar, M. Garg, R. Pathak and R. Singh, Sci. Rep., 2018, 8, 11799 CrossRef PubMed.
- A. Quellmalz, X. Wang, S. Sawallich, B. Uzlu, M. Otto, S. Wagner, Z. Wang, M. Prechtl, O. Hartwig, S. Luo, G. S. Duesberg, M. C. Lemme, K. B. Gylfason, N. Roxhed, G. Stemme and F. Niklaus, Nat. Commun., 2021, 12, 917 CrossRef CAS PubMed.
- P. Wang, C. Jia, Y. Huang and X. Duan, Matter, 2021, 4, 552–581 CrossRef CAS.
- D. Li, R. Cheng, H. Zhou, C. Wang, A. Yin, Y. Chen, N. O. Weiss, Y. Huang and X. Duan, Nat. Commun., 2015, 6, 7509 CrossRef PubMed.
- M. C. Lemme, D. Akinwande, C. Huyghebaert and C. Stampfer, Nat. Commun., 2022, 13, 1392 CrossRef CAS PubMed.
- F. Xue, L. Chen, J. Chen, J. Liu, L. Wang, M. Chen, Y. Pang, X. Yang, G. Gao, J. Zhai and Z. L. Wang, Adv. Mater., 2016, 28, 3391–3398 CrossRef CAS PubMed.
- P. Gupta, A. A. Rahman, S. Subramanian, S. Gupta, A. Thamizhavel, T. Orlova, S. Rouvimov, S. Vishwanath, V. Protasenko, M. R. Laskar, H. G. Xing, D. Jena and A. Bhattacharya, Sci. Rep., 2016, 6, 23708 CrossRef CAS PubMed.
- W. Limmer, W. Ritter, R. Sauer, B. Mensching, C. Liu and B. Rauschenbach, Appl. Phys. Lett., 1998, 72, 2589–2591 CrossRef CAS.
- D. Ruzmetov, K. Zhang, G. Stan, B. Kalanyan, G. R. Bhimanapati, S. M. Eichfeld, R. A. Burke, P. B. Shah, T. P. O'Regan, F. J. Crowne, A. G. Birdwell, J. A. Robinson, A. V. Davydov and T. G. Ivanov, ACS Nano, 2016, 10, 3580–3588 CrossRef CAS PubMed.
- L. Chen, F. Xue, X. Li, X. Huang, L. Wang, J. Kou and Z. L. Wang, ACS Nano, 2016, 10, 1546–1551 CrossRef CAS PubMed.
- F. Xue, L. Chen, L. Wang, Y. Pang, J. Chen, C. Zhang and Z. L. Wang, Adv. Funct. Mater., 2016, 26, 2104–2109 CrossRef CAS.
- S. Sahoo, A. P. S. Gaur, M. Ahmadi, M. J.-F. Guinel and R. S. Katiyar, J. Phys. Chem. C, 2013, 117, 9042–9047 CrossRef CAS.
- K. F. Mak, K. He, C. Lee, G. H. Lee, J. Hone, T. F. Heinz and J. Shan, Nat. Mater., 2013, 12, 207–211 CrossRef CAS PubMed.
- J. Zhang, J. Wang, P. Chen, Y. Sun, S. Wu, Z. Jia, X. Lu, H. Yu, W. Chen, J. Zhu, G. Xie, R. Yang, D. Shi, X. Xu, J. Xiang, K. Liu and G. Zhang, Adv. Mater., 2016, 28, 1950–1956 CrossRef CAS PubMed.
- M. Buscema, G. A. Steele, H. S. J. van der Zant and A. Castellanos-Gomez, Nano Res., 2014, 7, 561–571 CrossRef.
- N. Ben Sedrine, T. C. Esteves, J. Rodrigues, L. Rino, M. R. Correia, M. C. Sequeira, A. J. Neves, E. Alves, M. Bockowski, P. R. Edwards, K. P. O'Donnell, K. Lorenz and T. Monteiro, Sci. Rep., 2015, 5, 13739 CrossRef CAS PubMed.
- H. J. Conley, B. Wang, J. I. Ziegler, R. F. Haglund Jr., S. T. Pantelides and K. I. Bolotin, Nano Lett., 2013, 13, 3626–3630 CrossRef CAS PubMed.
- Z. L. Wang and J. Song, Science, 2006, 312, 242–246 CrossRef CAS PubMed.
- H. Chen, Z. Dong, Y. Zhao, S. Li, X. Du, Z. Wu, W. Liu and Y. Zhang, J. Lumin., 2020, 219, 116914 CrossRef CAS.
- H. Khan, N. Mahmood, A. Zavabeti, A. Elbourne, M. A. Rahman, B. Y. Zhang, V. Krishnamurthi, P. Atkin, M. B. Ghasemian, J. Yang, G. Zheng, A. R. Ravindran, S. Walia, L. Wang, S. P. Russo, T. Daeneke, Y. Li and K. Kalantar-Zadeh, Nat. Commun., 2020, 11, 3449 CrossRef CAS PubMed.
- N. Mahmood, H. Khan, K. Tran, P. Kuppe, A. Zavabeti, P. Atkin, M. B. Ghasemian, J. Yang, C. Xu, S. A. Tawfik, M. J. S. Spencer, J. Z. Ou, K. Khoshmanesh, C. F. McConville, Y. Li and K. Kalantar-Zadeh, Mater. Today, 2021, 44, 69–77 CrossRef CAS.
|
This journal is © The Royal Society of Chemistry 2023 |