DOI:
10.1039/D3NA00218G
(Review Article)
Nanoscale Adv., 2023,
5, 4311-4336
Fluorescent nano- and microparticles for sensing cellular microenvironment: past, present and future applications
Received
5th April 2023
, Accepted 13th June 2023
First published on 10th July 2023
Abstract
The tumor microenvironment (TME) demonstrates distinct hallmarks, including acidosis, hypoxia, reactive oxygen species (ROS) generation, and altered ion fluxes, which are crucial targets for early cancer biomarker detection, tumor diagnosis, and therapeutic strategies. Various imaging and sensing techniques have been developed and employed in both research and clinical settings to visualize and monitor cellular and TME dynamics. Among these, ratiometric fluorescence-based sensors have emerged as powerful analytical tools, providing precise and sensitive insights into TME and enabling real-time detection and tracking of dynamic changes. In this comprehensive review, we discuss the latest advancements in ratiometric fluorescent probes designed for the optical mapping of pH, oxygen, ROS, ions, and biomarkers within the TME. We elucidate their structural designs and sensing mechanisms as well as their applications in in vitro and in vivo detection. Furthermore, we explore integrated sensing platforms that reveal the spatiotemporal behavior of complex tumor cultures, highlighting the potential of high-resolution imaging techniques combined with computational methods. This review aims to provide a solid foundation for understanding the current state of the art and the future potential of fluorescent nano- and microparticles in the field of cellular microenvironment sensing.
1. Introduction
Cancer is one of the leading causes of death globally and has become more widespread over the last several decades owing to unhealthy lifestyles and environmental factors, causing more mutations to happen and increasing the likelihood of cancer development.1 According to statistical studies recently published by the American Cancer Society, 16.2 million cancer-related deaths and 28 million new cancer cases are projected to be the global burden by 2040.2,3 Moreover, an event that worsened cancer incidence in the last three years has been the coronavirus disease 2019 (COVID-19) pandemic, which has increased the number of the advanced stage of tumor cases, thereby increasing mortality.4
One of the major steps to prevent cancer-associated mortality and increase the chances of successful treatment is the early detection of tumors. To this end, the gold standard methods employed to detect a tumor site are mainly based on medical imaging techniques, where the most common methods are magnetic resonance imaging (MRI),5 positron emission tomography (PET)6 coupled to computed tomography (CT),7 ultrasound scanning,8 and photoacoustic endoscopy.9 However, these techniques present some limitations, such as poor compliance from the patient's viewpoint, lack of long-term stability of contrast agents and tracers, and requirement of highly qualified personnel. Together with oncologists, scientists are directing significant resources and efforts towards the study of biological features of tumors that can promote early detection. In this context, the complex TME, in which cancer cells and rich stroma interact with each other releasing growth factors, proteins and membrane-derived vesicles, provides new avenues for early diagnosis.10–15 Different strategies targeting TME have emerged because of its importance in influencing therapeutic outcomes.16,17 Notably, TME is highly heterogeneous and dynamic, and it is characterized by the establishment of pH and oxygen gradients resulting from the increased cellular metabolic activity and altered blood perfusion. This marked heterogeneity significantly affects the efficacy of anticancer treatments.18 Dynamic mapping of TME's parameters, such as pH and oxygen, is crucial for understanding their role in cellular and subcellular processes because it can help to better comprehend the link between pH/oxygen distribution, cell morphology, and cell functions. In this context, numerous sensing systems have been developed to facilitate the prompt detection of key analytes in the TME and to obtain a valid metabolic read out for cancer diagnosis and treatment.19,20 Among these, ratiometric fluorescence-based nano- and micro-sensors stand out as valid and non-invasive approaches for characterizing cellular microenvironments and sub-cellular compartments with high precision over time and space. These technologies pave the way for the powerful era of modern precision and personalized medicine.
1.1 Deep insight into TME
The genesis and the development of cancer diseases involve multistep processes that start with genetic or epigenetic changes in tumor cells,21–23 followed by dynamic crosstalk, leading to the rearrangement of a tumor-supportive and highly reactive microenvironment (TME) that surrounds the tumor (Fig. 1).24–26 It is now widely recognized that TME plays a crucial role in cancer initiation,27 progression28 and metastasis.29
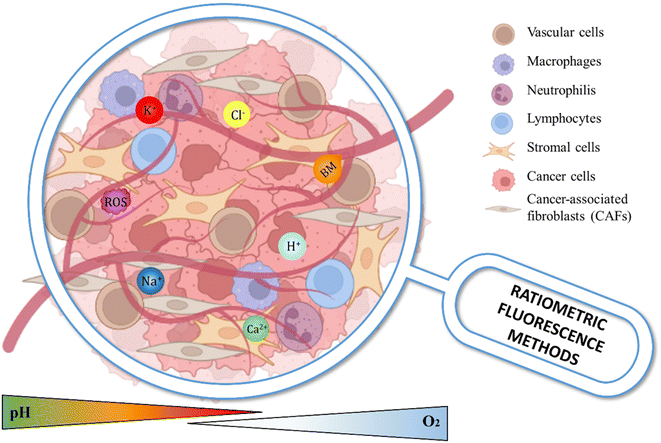 |
| Fig. 1 A close-up look at the different characteristics and analytes having a biological significance within the TME, which can be precisely examined by ratiometric fluorescence methods. The heterogeneity of the TME is mainly due to the complex ecosystem created by the interactions among tumor, stromal and immune cells, all of which are immersed in a dense and dysregulated ECM. Poor blood flow and crowded glycolytic tumor cells form niches characterized by reduced oxygenation, pH acidity, reduced nutrient loading, collection of anti-inflammatory cytokines and chemokine, and storage of metabolic by-products, such as lactate. | |
The main constituents of the TME are cancer cells30,31 and accessory cells, including cancer-associated fibroblasts (CAFs),32,33 immune and inflammatory cells,34,35 all of which are embedded in a dense stroma of extracellular matrix (ECM) components, such as collagen type I,36,37 fibronectin,38 hyaluronic acid39 and growth factors.40 ECM not only functions as a support for tumor cells but also regulates and promotes cell–cell and cell–matrix interactions.41 Additionally, the ECM is involved in signalling pathways that regulate cell behaviour and differentiation; therefore, changes in the ECM can disrupt normal cellular processes, leading to disease development and progression.42 Thus, the interplay between cancer cells and ECM contributes to the increase in tumor heterogeneity, which is considered the major cause of treatment failure in current therapies.43 This feature is not only a consequence of clonal outgrowth of cells with genetic alterations but also of epigenetic alterations promoted by several physical and biochemical signals from the TME.44 The unlimited multiplication of tumor cells is a phenomenon strictly related to their ability to elude growth suppressors45 and apoptotic signals.46 As a result, the TME promotes a sequence of physical (acidosis, hypoxia, temperature and stiffness)47,48 and biochemical (adhesion proteins, glycoproteins and proteoglycan, secreted factors, growth factors, and matrix degradation enzymes)49 adaptations that promote angiogenesis,50 invasion51 and metastasis.52 Moreover, to survive in a hostile microenvironment, which is characterized by high deprivation of oxygen and nutrients, and to maintain a high proliferative rate, some tumor cells are known to adjust their metabolism,53,54 from the oxidative phosphorylation towards the aerobic glycolysis, the so-called “Warburg effect”,55 which was first observed by Otto H. Warburg in the early twentieth century.56 In normal cells with adequate oxygen levels, the pyruvate produced by the breakdown of glucose during the glycolysis process and could enter into the tricarboxylic acid (TCA) cycle to generate energy.57 Tumor cells instead exhibit increased glycolysis activity regardless of the amount of oxygen and produce lactate by activating lactate dehydrogenase and inhibiting mitochondrial metabolism.55 The resulting acidosis effect is a direct consequence of lowering of extracellular pH from physiological pH 7.4 to values up to 5.0, whereas the intracellular pH is increased compared to that of normal cells. Consequently, the acidity of the interstitial space and the high intracellular pH affect the dynamic and functional cell–cell or cell–matrix crosstalk.58 The low extracellular pH is an important factor for inducing more aggressive cancer phenotypes, increasing cell motility, extracellular matrix degradation and modifying cellular and intercellular signaling.59,60 Furthermore, the accumulation of protons (H+) in the extracellular environment is spatially and temporally heterogeneous and influences the efficacy of chemotherapeutic treatments.61 Additionally, it has been well established that also intracellular pH is dysregulated in cancer.62,63 Although many biological mechanisms contribute to intracellular pH dynamics, the main regulators are the plasma membrane ion exchangers, such as Na+/H+ exchanger 1 (NHE1), and plasma membrane ion transport proteins, including V-ATPases and the monocarboxylate transporters (MCTs). Changes in their expression and activity to facilitate H+ efflux contribute to maintaining alkaline intracellular pH and acidic extracellular pH in tumor cells.64
Furthermore, the higher intracellular pH promotes many cancer behaviors, such as increased proliferation, migration, epithelial plasticity and the oncogenic and tumor suppressor functions of mutated proteins.65 Notably, in solid tumors, the low vascularization due to impaired vascular network with the formation of abnormal blood vessels and the reduced perfusion of oxygen within the TME equally contribute to the metabolic switch of the cancer cells, promoting the acidification of the TME.66 This phenomenon known as hypoxia is mainly induced by hypoxia-inducible factors (HIFs), which are recognized as master regulators of cell metabolism.67 The reduction of oxygen levels in the TME is associated with angiogenesis activation and increasing tumor survival, invasiveness, and metastatic potential and hampers the therapeutic response.68
The hypoxic microenvironment also induces the expression of genes that sustain tumor progression and the generation of reactive oxygen species (ROS).69 ‘Reactive oxygen species’ term groups together two classes of molecular oxygen products derived from reduction–oxidation reactions or electronic excitation during aerobic cellular respiration, which are namely non-radical and free radical species. Examples of ROS are hydrogen peroxide (H2O2), its reduction product hydroxyl radical (˙OH), and superoxide anion radical (O2˙−).70–72 These side products derived from cellular respiration and carried out in mitochondria under physiological conditions are kept under control by detoxification mechanisms.73,74 The maintenance of their concentration within physiological levels (10−8 M for H2O2 and 10−11 M for O2˙−) is called oxidative eustress.75 In this phenomenon, more than 40 enzymes, particularly NADPH oxidase enzymes, are involved in the redox signalling pathways that promote proliferation, differentiation, migration and angiogenesis.76 In contrast, elevated levels of ROS in the cellular microenvironment determine oxidative distress, a condition in which unspecific protein oxidation leads to reversible or irreversible damages of biomolecules, causing pathological states that include inflammation, tumor growth, metastasis and cell death.77 In the cancer field, the study of ROS has attracted increasing interest in the last twenty years because it is now generally recognized that the regulation of oxidative stress represents a key factor of tumor development and its responses to anticancer therapies. Gorrini's group78 remarked that moderate concentrations of ROS may contribute to tumor progression because they act as signalling molecules and promote the mutation of nuclear DNA and mitochondrial DNA (mt-DNA). Moreover, concomitant conditions in the TME, such as hypoxia, metabolic defects inducing the Warburg effect,79 endoplasmic reticulum (ER) stress, and activation of oncogenes, cooperate to produce a significant increase in ROS concentration.
In healthy cells, inorganic ions can play different roles in the homeostasis of the human body. For instance, inorganic metal cations, such as magnesium (Mg2+), zinc (Zn2+), iron (Fe2+) and copper (Cu2+), are essential in enzymatic reactions by acting as cofactors, while the cations calcium (Ca2+), sodium (Na+) and potassium (K+), and the anion chloride (Cl−) is involved in electrophysiological events.80 During the malignant transformation of healthy cells into cancer cells, mutations of genes also affect those encoding for plasma membrane ion channels, thus resulting in the alteration of the ion fluxes, cell membrane potentials (Vmem), and, consequently, modifications in the intracellular signalling pathways.81–83 The disruption of ion homeostasis, about the transport of ions through channels and their concentration within the TME, yields biophysical phenomena, such as elevated pressure, increased stiffness, and mechanical stress. Subsequently, these alterations lead to the activation or attenuation of molecular signaling pathways implicated in cancer initiation, promotion, and invasion processes.84 One indicator of normal cells transitioning into cancerous and proliferative tissues is the more positively charged or depolarized membrane, with a Vmem increasing from −60 mV to −10/−30 mV in more undifferentiated cancer stem-cells.85,86 The cytosolic Ca2+ levels are important for the integrin-signalling pathway, which is activated to facilitate cell–cell and cell–ECM communication. The cytosolic Ca2+ levels are important for the integrin-signalling pathway, which is activated to allow cell–cell and cell–ECM communication. The dysregulation of calcium ions within cells contributes to cancer-related processes. In particular, intracellular Ca2+ concentration plays a crucial role in regulating cytoskeletal dynamics, which are involved in extracellular matrix (ECM) degradation and the initiation of the metastatic process. This occurs through the activation of epithelial–mesenchymal transition (EMT) pathways and the enzymatic activity of metalloproteases.87,88 Strictly linked to Ca2+ functions are the fluxes of Na+ cations, which are involved in the synergistic activity of Na+/Ca2+ exchangers.89 Furthermore, alterations in intracellular Na+ concentrations lead to a reduction in H+ in the vicinity of cancer cells, resulting in the formation of integrin-mediated focal adhesion contacts that promotes cell adhesion.90 Concomitant with alterations in the membrane potential of cancer cells, K+ fluxes are linked to proliferation, as fluctuations in K+ levels interact with extracellular signal-regulated kinase (ERK 1/2) and c-Jun N-terminal kinase (JNK) signalling pathways.91,92 Additionally, the Cl− anion, which typically facilitates the transport of cations, such as Ca2+, Na+, and K+, can promote migration and metastasis by modulating cell volume.93
1.2 Fluorescent ratiometric sensors for TME investigation and mapping
Understanding the mechanisms underlying cell–cell interactions is essential for mapping the tumor microenvironment (TME), which is currently a critical aspect of improving prognosis, diagnosis, and therapies. In this context, nanotechnologies aimed at precision medicine pave the way for a ground-breaking approach to combating cancer. This is due to their extensive applications in detecting signature biomarkers, which are crucial steps toward early diagnosis and targeted therapeutic drug delivery.94 Optical biosensors, particularly those based on fluorescence (FL), are becoming increasingly important in cancer research owing to their enhanced detection capabilities. They represent valuable tools for detecting and analysing a wide range of biomolecules, making them advantageous for studying cancer-related processes.95 Fluorescence microscopy is a critical tool for bio-imaging and optical sensing of specific analyte concentrations in tissues and cancer models,96 where the most commonly used fluorescence microscopy techniques include fluorescence lifetime imaging microscopy (FLIM),97 phosphorescence lifetime imaging microscopy (PLIM),98 and near-infrared (NIR) microscopy.99 The methods mentioned earlier require specialized and sophisticated equipment, but the introduction of ratiometric fluorescence (FL) measurements has significantly improved the performance of fluorescence microscopy applications.
Ratiometric FL enables the precise measurement of analyte concentrations within the TME, making it an increasingly valuable tool in cancer research. Many research groups have focused on the achievement of responsive FL molecular probes to detect single targets within the TME. For example, Anderson et al. developed a ratiometric pH-sensitive fluorescent dye based on a seminaphtharhodafluor (SNARF) core to compare the surface cell pH of cancer cells grown either in spheroids, mouse tumor models or in excised tumors.100 In a different approach, Zheng et al. employed an iridium-based hypoxia-activated optical molecular probe to produce an oxygen nanosensor suitable to perform hypoxia imaging in mice bearing hepatoma cells, H22.101,102 Recently, the possibility of detecting two cancer parameters at the same time within the TME has provided the opportunity to deeper correlate cancer hallmarks with each other. In the same research group, Zheng and collaborators designed and synthesized an ultrasensitive molecular probe based on a poly(ethylene glycol)-conjugated iridium(III) complex (Ir-Im-PEG). The imaging of tumor acidity and hypoxia, carried out simultaneously, was studied and performed both in vitro using the HeLa cell line and in vivo by implanting H22 tumors subcutaneously in mice.103 In a different study, Yeh and co-workers successfully employed commercially available probes, SNARF-1 and Rhod-5N, to map and quantify protons and calcium concentrations using an imaging approach. The data obtained allowed the correlation of pH and calcium levels in the intravascular and interstitial space of bone marrow in the mouse calvarium.104 Thus, sensing FL analytical platforms are among the most used tools because they permit the investigation of the physiological and pathological processes of living organisms.105–107 Many of them have been developed and act as indicators for monitoring and quantifying specific analytes and clinically relevant metabolites.108,109
Despite advancements in fluorescence-based sensor technology, designing sensors for in vitro and in vivo applications remains a challenging task because of numerous factors to consider, such as sensor selectivity, sensitivity, biocompatibility, and stability.110 The vast potential of particle-based systems in elucidating the intricacies of the TME is due to the infinite possibilities of tuning materials, size, shape, surface charge, and functionalities, coupled with their ease of preparation and, in some cases, intrinsic biocompatibility. Silica (SiO2), polystyrene (PS), and poly(methyl)methacrylate (PMMA) nanoparticles are particularly noteworthy owing to their large specific surface area, stability properties, and viability. These features make them attractive candidates for in vitro and in vivo applications in cancer research.111,112 Additionally, the coupling of fluorescence (FL) molecular probes to structured micro- and nano-systems has garnered significant interest owing to their high analyte sensitivity, low cost, and rapid spatiotemporal resolved measurements. The unique features of nanoparticles make them ideal for studying various cancer-related processes, such as bio-imaging and optical sensing of oxygen concentration in tissues and cancer models using fluorescence microscopy, particularly FLIM. Organic dyes with specific excitation and emission wavelengths can serve as probes to reliably respond to specific targets in the TME, making them valuable tools for cancer research. The FL behaviour of the molecular probe is determined by structural changes in the fluorophores resulting from the formation and/or breakdown of responsive functional groups. These changes can cause FL quenching (off) or FL enhancement (on) effects,113 which are the results of Förster Resonance Energy Transfer (FRET), photoinduced energy transfer (PET), internal charge transfer (ICT), and self-quenching phenomena. Understanding these phenomena is crucial for developing effective molecular probes for various applications in cancer research. Although single dye-doped sensors are widely used and easily manipulated, they often lack accuracy owing to instrumental, operational, and environmental variations that can interfere during analyses. To address this issue, single-signal sensors have been replaced with ratiometric FL sensors. These sensors incorporate a second FL target-insensitive signal that serves as a reference signal, reducing the possibility of errors through self-calibration and refining the detection limits of the sensors. This advancement has significantly improved the accuracy and reliability of molecular probes used in cancer research.114,115 Another strategy to build a ratiometric FL-sensor is the employment of a dual-emissive probe, which presents two reversible detection signals that are strictly interrelated (Fig. 2). Thus, as a general rule, ratiometric analysis is calculated by plotting the ratio of two FL signals. Moreover, the encapsulation of dyes within the matrix of biosensors can improve the performance of the developed analytical platforms in terms of target selectivity. There are still some aspects that represent current challenges regarding environmental perturbations over long-time experiments, photobleaching, and light scattering phenomena. Within the vast world of nanosized-photoluminescent particles, it is possible to identify two main categories: quantum dots and metallic nanoclusters.116 The multiple advantages observed in the field of fluorescence imaging over the last few years mainly stem from the discovery and the development of brilliant nanoparticles, which are synthetically obtained using elements from groups II–VI, IV–VI and III–V of the periodic table via different techniques.117–119 These nanoparticles, with quantum confinement effects, are defined as semiconductor nanocrystals or simply quantum dots (QDs). Single properties, such as size-dependent emission, narrow emission peaks, and resistance to photobleaching, promote the achievement of optical sensing systems based on QDs compared with the use of organic dyes.120 However, biosensing systems exploiting QD potentials must overpass their cytotoxicity in biological systems121 by surface modifications and/or coating with biocompatible materials.122–124 Moreover, metallic nanoclusters (MNCs), metal-centred nanoparticles that can be stabilized by protective groups, usually biological molecules, represent powerful alternative platforms for fluorescence sensing in vitro and in vivo, and their investigation has been growing over the years. Among their properties, it is important to note intrinsic water solubility and biocompatibility.125,126
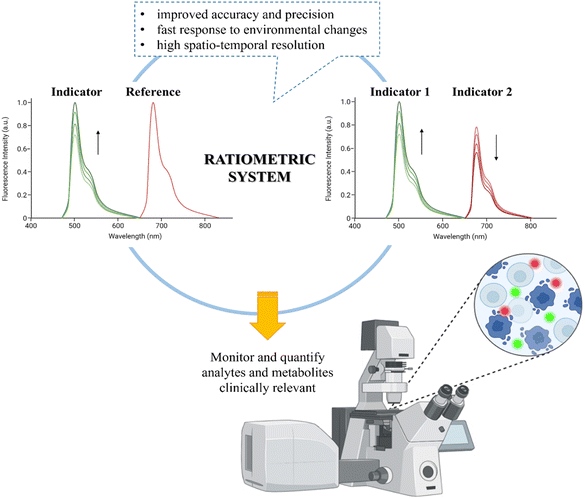 |
| Fig. 2 Sketch of the ratiometric optical methods with their advantages and applications in sensing TME in vitro and in vivo. | |
In this comprehensive review, we delve into cutting-edge developments in the detection and monitoring of critical TME parameters, including pH, O2, ROS, and inorganic ions, along with crucial tumor biomarkers. Through the implementation of nano- and microparticle-based ratiometric fluorescence sensors, we present an in-depth analysis of their latest integrations in two- and three-dimensional architectures. Our review includes a detailed description of the intricate mechanisms behind these sensors and showcases their impressive in vitro and in vivo applications while providing an insightful analysis of the strengths and limitations of each system.
2. Microenvironment parameters under study
2.1 pH
The real-time monitoring of pH in the biological environment is a challenging task127 that cannot be fulfilled by standard methods. In lab practice, the pH-meter electrodes used are cheap and reliable tools for bulk pH measurements, but the difficult miniaturization of these devices makes them less suitable for in vitro and in vivo studies. From a physiological viewpoint, proton (H+) concentration varies from one cellular compartment to another. For instance, pH in the cytosol has a value in the range of 7.0–7.4, and it is among 7.2 in the endoplasmic plasmatic reticulum (ER), while it is slightly acidic in the organelles, as it is 6.4 in the Golgi apparatus, 5.0 in lysosomes, 5.4 in secretory granules, 6.2 in early endosomes, 5.3 in late endosomes, and 8.0 in mitochondria.84,128–130 The metabolic switch induced in cancer growth breaks the balance between the compartments of cells, determining pH fluctuations not only in the intracellular environment but also in the extracellular surrounding. Therefore, the need of monitoring and mapping pH inside the cells and in the space between cells constitutes a crucial topic of research interest. Consequently, FL small molecules and nanoprobes have been intensively developed and studied for sensing pH.131 Optical pH measurement is based on the significant change in absorption or fluorescence of suitable pH indicators after protonation/deprotonation at different pH values. Today, many fluorescent pH indicators are commercially available (e.g., fluorescein, semi-naphtharhodafluor 1 (SNARF1), 8-hydroxypyrene-1,3,6-trisulfonic acid trisodium salt (HPTS), and Nile Blue A), and most of them have been successfully employed in non-invasive and real-time imaging of pH in several physio-pathological processes.132 However, one of the main challenges of pH-sensing molecules is their limited sensitivity range and, for some of them, lack of solubility in water solutions, as well as toxicity. Therefore, the encapsulation of FL pH-sensing molecules into nano-structured and biocompatible matrices improves the final analytical platform in terms of photostability, solubility and cell viability, thereby enhancing accuracy. Striking examples are the polyelectrolyte multilayer capsules obtained via the layer-by-layer (LbL) method, which in the past two decades have irrupted the scene of nanotechnology as a straightforward and versatile technique.133–135 In 2011, del Mercato employed the dual-emission ratiometric SNARF1-dextrane derivate to prepare permeable calcium carbonate (CaCO3)-based capsules using the LbL technique.136 The ratiometric SNARF1 dye has the unique property of displaying two emission peaks, depending on pH: the excitation at 543 nm determines, at acidic pH, an emission peak with a maximum value at 594 nm, while, in basic pH, a spectral emissive band at 640 nm is recorded. The FL characterization, employing spectroscopy and FL microscopy, carried out on the pH sensing capsules, confirmed the ability of the labelled and encapsulated amino-dextran SNARF1 (λex = 543 nm, λem1 = 594 nm; λem2 = 640 nm) to efficiently sense H+ concentrations with the same sensitivity as the free dye. Later, the same capsules were applied to measure the intracellular pH in MCF-7 breast cancer cells. The cellular uptake of capsules was tracked to monitor pH in the endosomes and lysosomes.137,138 In a different approach, multilayer pH-sensing capsules, based on SNARF probe, were successfully applied to map the pH microenvironment of human mesenchymal stromal cells (hMSCs) seeded in 3D additive manufactured scaffolds.139 A fully automated computational approach for precisely measuring organelle acidification in cancer cells was set up by Chandra and colleagues.140 The authors developed micrometer-sized silica (SiO2) particles that were functionalized with fluorescein 5-isothiocyanate (FITC) (λex = 492 nm; λem = 518 nm), as pH probe, and rhodamine B isothiocyanate (RBITC) (λex = 570 nm; λem = 595 nm), as the reference dye. Furthermore, to ensure internalization in the cytosolic comportment, the MPs were decorated with a net positive external charge that allowed cell uptake and the finest acidic sensing property because of the lowered pKa (6.30 ± 0.09). The tracking and mapping experiments were performed by applying CLSM time-lapse using tumor models MDA-MB-231 and MCF-7 breast adenocarcinoma cell lines (Fig. 3a). The innovation of the entire method depended on the automated computational approach, which simplified and enriched the interpretation of data derived from image acquisitions through the creation of ad hoc algorithms.
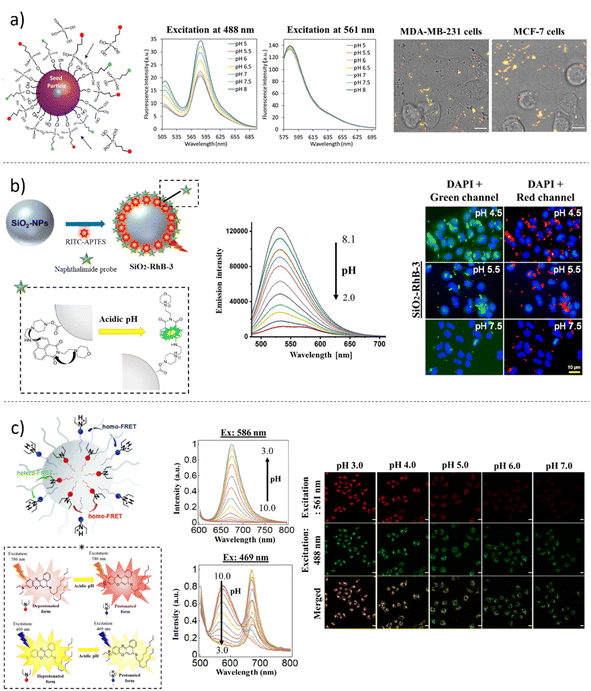 |
| Fig. 3 Examples of pH sensing ratiometric nano-platforms. (a) (Left): Schematic illustration of the ratiometric SiO2 MPs functionalization with FITC and RBITC dyes using a modified Stöber method; (middle): pH-dependent fluorescence of the MPs; (right): fluorescence micrographs showing the color changes in the ratiometric pH-responsive MP sensors added to MDA-MB-231 cells and MCF-7 cells after incubation for 24 hours. Scale bars: 10 μm. Adapted with permission from Chandra et al., ACS Appl. Mater. Interfaces 2022, 14, 18133–18149; figure licensed under CC-BY 4.0, https://creativecommons.org/licenses/by/4.0/. (b) (Left): Schematic representation of the protocol used to synthesize pH-sensing SiO2-NPs; (middle): pH-dependent fluorescence of probe 3 on NPs; (right): epifluorescence images of fixed A549 cells incubated with solutions of pH nanosensors SiO2–RhB-3, monitoring with emission filters set to λem = 470 nm (green channel) and to λem = 560 nm (red channel). Scale bar: 10 μm. Adapted with permission from Srivastava et al., Sci. Rep., 13, 1321, 2023; figure licensed under CC-BY 4.0, https://creativecommons.org/licenses/by/4.0/. (c) (Left): Schematic illustration and working principle of the protonation of Ch3 at the surface of the NPs, turning the color from red to blue; (middle): fluorescence emission spectra (excitation at 586 nm) of the nanosensors containing Ch3, PS-PEO, and NPOE in universal buffer solutions at different pH values from 10 to 3, upon excitation at 586 and 469 nm; (right): CLSM images for the cellular pH calibrations of the nanosensors from pH 3.0 to 7.0. Scale bar: 20 μm. Reprinted with permission from Chen et al., Nano Res., 2022, 15(4): 3471–3478. Copyright © 2021, Tsinghua University Press and Springer-Verlag GmbH Germany, part of Springer Nature. | |
Over the last decade, many groups have developed new FL probes with a wider range of sensitivity towards pH values. This is the case developed by Srivastava and collaborators141 who recently synthesized a novel pH-responsive green naphtalimide-based dye (λex = 405 nm; λem = 525 nm) (Fig. 3b). The dye was obtained by covalently linking two functional moieties: a selective lysosomal targeting part, represented by a morpholine unit bound to 4-bromo-1,8-naphthalic anhydride, and a piperazine ring, which improves the solubility of the dye in water. The working mechanism of the pH-sensing dye was studied over all pH ranges. In detail, the protonation of the morpholine and piperazine amine groups determined the switch-on in an acidic environment of the green FL signal, which therefore was PET-induced quenched gradually passing across neutral and basic pH, thus characterizing the probe selectivity ranging from 2.0 to 8.0 pH values. The significance of this study depends on the fabrication of ratiometric SiO2 NPs, coupling the reference RBITC dye (λex = 570 nm; λem = 595 nm) to the green synthesized pH-indicator dye, and their subsequent in vitro application for mapping lysosomal uptake and pH fluctuations in the human lung cancer A549 cell line using CLSM. Although the developed analytical platform has demonstrated its suitability for this purpose, the authors acknowledge the need for deeper live-cell imaging studies, specifically regarding the co-localization of the NPs in the endosomes and lysosomes. These studies are crucial for further validating the effectiveness of this innovative approach in cancer research.
Although pH-sensitive fluorescent nanosensors based on hydrophobic indicators are largely unexplored, boron-dipyrromethene (BODIPY) and boron-azadipyrromethene (aza-BODIPY)-based dyes are noteworthy examples. The introduction of hydrophilic moieties to the BODIPY core has significantly enhanced their water solubility while retaining their fluorescence properties.142 Currently, near infrared (NIR) emissive aza-BODIPY pH-indicator compounds were synthesized by Strobl et al. and were presented as novel dyes covering the pH scale ranging from 1.5 to 13.143 The great advantages of using such types of dyes in long-wavelength spectral regions are the enhanced photostability, less scattering background and deep light penetration. Despite several in vitro and in vivo studies of BODIPY dyes,144 the biotechnological development of ratiometric pH platforms and their further applications in cell sensing are not present in the literature. However, the successful engagement of hydrophobic pH-sensing dye chromoionophore III (Ch3 or ETH 5053) for exploring lysosomal pH was published by Chen and collaborators in 2022 (Fig. 3c).145 The authors reported the encapsulation of hydrophobic Nile Blue A-derivate Ch3 into the polymeric matrix of poly(styrene)-graft-poly(ethylene oxide) (PS-PEO) NPs. Ch3 is a ratiometric pH sensing probe characterized by two excitation wavelengths and emissive spectra in the far-red region (λex1 = 586 nm and λem1 = 675 nm; λex2 = 469 nm and λem2 = 575 nm), corresponding to the protonated and deprotonated forms, respectively. The mechanism of FL emission is regulated by the FRET phenomenon: moving from pH 10 to 3, the emission peak at 575 nm gradually decreases upon excitation at 469 nm, whereas vice versa, the emission peak at 675 nm gradually increases. The linear regression recorded by plotting the FL intensity at 675 nm versus the FL intensity at 575 nm enabled the authors to test the pH nanosensors, followed by incubation with HeLa cells. The Ch3-NPs were endocytosed by HeLa cells, and subcellular pH monitoring was carried out using time-lapse CLSM acquisitions. After proper pH calibration of the sensors, carried out in the cell medium, the pH of the organelles was determined to be approximately 4.7. The sensor system proposed by Chen and colleagues145 greatly summarized the potential of ratiometric NP engineering, highlighting the easy preparation through dye embedding in a biocompatible matrix, thus making the system suitable for future in vitro and in vivo applications.
2.2 Oxygen
The hypoxia switch-on represents a hallmark within the TME that leads towards concomitant events, such as the metabolic switch, acidosis and ECM rearrangement phenomena, which in turn are involved in the progression and MDR of cancer.146 Therefore, considering the variations of the partial pressure of oxygen (ppO2) appears as the main strategy to be monitored, both in vitro and in vivo.147 Nowadays, several methods for the detection of dissolved oxygen concentration are widely published in the literature, and they are based mainly on electrochemical (amperometry, potentiometry, or conductometry) and chemical (Winkler titration) techniques.148 Although various methods for sensing TME parameters exist, they often lack dynamic real-time and spatiotemporal resolution at a single-cell scale. However, in recent decades, optical and ratiometric FL probes have become the preferred methodology for bioimaging applications, and the field has exhibited increasing advancements. The principle behind oxygen sensing relies on the quenching of the luminescence intensity of an indicator probe by molecular oxygen (O2), a phenomenon governed by energy or electron transfer mechanisms that are well described in the Stern–Volmer equation. By utilizing such probes, researchers may gain valuable insights into oxygen levels within the TME, which may have important implications for the development of novel diagnostic and therapeutic approaches in cancer research.149 Moreover, another important parameter to consider is the diffusion coefficient (D) of O2 throughout the matrix in which the indicator is immersed. Thus, the combination of the choice of the optimal oxygen sensing indicator, together with an unquenchable and photostable reference dye, and the selection of the suitable, inert and gas-permeable/ion-impermeable matrix enable the fabrication of O2 ratiometric FL sensing devices. In this portrait, various oxygen-permeable materials (silicon polymers, organic glassy polymers, fluoropolymers and cellulose derivate polymers)150,151 and reversibly quenched oxygen indicator probes (e.g. transition metal polypyridyl complexes and metalloporphyrins)152 are commercially available.
Among all the transition metal dyes, ruthenium (Ru(II))-based polypyridyl complexes are extensively adopted in bioimaging applications owing to their properties, such as large Stokes' shift, excitation and emission band in the visible region, good photostability and high brightness.153 Despite these characteristics, the excitation and the emission peaks remain quite broad, which can be interpreted positively, having more possibility of choice for the laser excitation, or negatively, because of the difficulties in isolating the emission band during multi-analyte measurements.152 However, (Ru(II))-based polypyridyl complexes remain the most used O2-molecular probes. For instance, Xu et al. reported in 2001 the first sol–gel-based ratiometric FL PEBBLEs for the real-time measurements of oxygen in rat C6 glioma cell line.154 The nanosensor was built-up by encapsulation within the matrix of silica particles, obtained by a modified Stöber method, of the indicator and reference dyes, which were ruthenium(II)–tris(4,7-diphenyl-1,10-phenanthroline) dichloride ([Ru(dpp)3]2+) (λex = 543 nm; λem = 610 nm) and Oregon Green 488-dextran (λex = 488 nm; λem = 525 nm), respectively. The ratiometric linear regression plot, obtained from the calibration curve at different rates of O2, allowed the authors to inject the ratiometric FL nanoprobes into rat C6 glioma cells and collect preliminary information regarding intracellular oxygen through CLSM image acquisition. At that time, the novelty of the work published was the first development and application of a ratiometric O2 sensing system; however, the platform possessed a great limitation, which was the absolute need to control pH within the cell medium because Oregon Green is a pH-sensitive dye for pH values below 6.0.
Platinum (Pt(II))- and palladium (Pd(II))-based metalloporphyrins constitute another category of optical O2 sensors having strong phosphorescence, good molar absorption coefficients and large Stokes' shifts.155 In the work reported by Wu and collaborators, platinum(II)-octaethyl porphyrin (PtOEP) (λex = 580 nm; λem = 650 nm) was employed as oxygen sensitive dye and entrapped using particle precipitation technique into the matrix of polyfluorene derivates poly(9,9-dihexylfluorene) (PDHF) (λex = 350 nm; λem = 420 nm) and poly(9,9-dioctylfluorene) (PFO) (λex = 350 nm; λem = 420 nm), which in turn acted as reference signals in the ratiometric system and as hydrophobic, glassy and gas-permeable polymers.156 The working mechanism of the probes was based on the FRET phenomenon, in which the PDHF and PFO were the donor units, whereas PtOEP was the acceptor one. In this study, π-conjugated polymer nanoparticles (CPdots) exhibited peculiar properties: under excitation, the polymer matrices were capable of transferring energy to the phosphorescent PtOEP, thus enhancing its sensitive ability to respond at different concentrations of oxygen; additionally, the confinement inside the polymeric matrices determined augmented photostability. Therefore, the fluorescence emission of PtOEP was linearly quenched in the presence of increasing concentrations of O2, while PDHF and PFO did not change their fluorescence signals. Following the characterization of the sensors, the authors tracked the cellular uptake operated by the macrophage-like murine J774A1 cell line using differential interference contrast (DIC) images and phosphorescence images of the nanoparticle-labelled cells, demonstrating the possibility of detecting subcellular O2 concentrations. The overall results indicated that CPdots possessed great potential for the quantification of oxygen in in vitro experiments dictated by their unique qualities, such as brightness, ratiometric emission, small size and, consequently, cellular uptake. Considering the advantage of these findings, in 2012, in Wang's lab,157 PtOEP was used to prepare a ratiometric FL probe, together with the O2-insensitive dye coumarin 6 (C6) (λex = 381 nm; λem = 510 nm), fabricated by the precipitation and encapsulation of the dyes within the matrix of PMMA nanoparticles, whose surface was functionalized with poly-L-lysine to facilitate cellular uptake and thus intracellular oxygen imaging visualization (Fig. 4a). The linear regression obtained by the Stern–Volmer plot, and the characterizations performed, allowed the authors to apply the nanoprobes in cellular cultures of the HepG2 human hepatocellular liver carcinoma cell line. CLSM images were obtained after 12 hours of incubation of the NPs with cells in the culture medium. Assuming vesicular cellular uptake, the NPs were found to be localized in the intracellular space of HepG2 cells. By subjecting the cell cultures to hypoxia and normoxia conditions, Wang and collaborators were able to evaluate the goodness of the developed ratiometric FL O2 analytical platform, confirming the potential of such a tool to sense intracellular oxygen concentrations.157
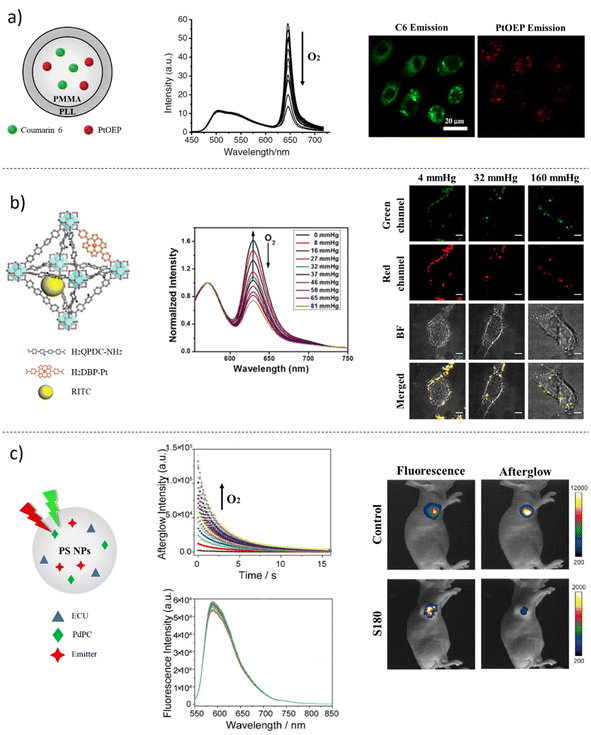 |
| Fig. 4 Examples of O2 sensing nano-platforms. (a) (Left) Schematic representation of the oxygen ratiometric PMMA-NPs; (middle): emission spectra of the sensor NPs at various concentrations of oxygen upon excitation at 381 nm excitation; (right): CLSM images of HepG2 cells loaded with the oxygen sensing NPs under normoxia conditions; the green fluorescence of C6 of ratiometric NPs was recorded using a 560 nm emission band-pass filter with a 405 nm excitation, while the red fluorescence of PtOEP using a 750 nm emission band-pass filter with a 543 nm excitation wavelength. Scale Bar: 20 μm. Reprinted with permission from Wang et al., Microchim Acta, 2012, 178, 147–152; Copyright © 2012, Springer-Verlag. (b) (Left): Schematic representation of R-UiO NMOF based on Pt(II)-porphyrin ligand as an O2-sensitive probe and a rhodamine-B isothiocyanate ligand as an O2-insensitive reference probe; (middle) emission spectra (λex = 514 nm) of R-UiO in HBSS buffer under various oxygen partial pressures; (right): CLSM images of CT26 cells under hypoxia (4 mmHg), normoxia (32 mmHg), and aerated conditions (160 mmHg) after incubation with R-UiO-2. Scale bar: 5 μm. Reprinted with permission from Xu et al., J. Am. Chem. Soc., 2016, 138, 2158–2161. Copyright © 2016, American Chemical Society. (c) (Left): Schematic illustration of the working principle of afterglow/fluorescence dual-emissive ratiometric O2 probe; (middle): afterglow decay curves of AGNPs and fluorescence spectra of AGNPs in different oxygen concentrations; (right): fluorescence and afterglow images of mice with the subcutaneous implantation of AGNPs in the mouse bearing no tumor and of mice with the intratumor injection of AGNPs in the mouse bearing the tumor, with the corresponding fluorescence and afterglow intensity. Reprinted with permission from Wen et al., Anal. Chem., 2023, 95, 4, 2478–2486. Copyright © 2023, American Chemical Society. | |
Based on the need to improve the existing O2-sensing probes, Xu et al. developed a ratiometric metal–organic framework (MOF) for sensing intracellular oxygen (Fig. 4b).158 MOFs are nanomaterials synthesized by bridging metal ions or clusters using organic ligands, which can be molecular sensing probes, thus preparing a final unit with a specific analytical scope.159 For this reason, in recent years, researchers have explored MOFs as promising devices because of their intrinsic characteristics: first, encapsulated dyes and therapeutic drugs can diffuse easily from the core owing to the high porosity of the material; second, the encapsulation of sensing molecules can enhance the photostability and reduce the self-quenching phenomenon; lastly, the covalent bounds between the metal and organic linkers prevent unforeseen leaching.160,161 Thus, exploiting the challenging properties of MOF, Xu and co-workers prepared a nanomaterial R-UiO-based having Pt-5,15-di(p-benzoato)porphyrin (H2DBP-Pt) (λex = 570 nm; λem = 595 nm) as bridging ligand, sensible to changes in O2 concentration, and RBITC-conjugated quaterphenyldicarboxylate (λex = 570 nm; λem = 595 nm), as reference fluorophore.158 The authors recorded linear Stern–Volmer regression when the R-UiO was calibrated in the presence of different concentrations of O2. The mouse colon carcinoma CT26 cell line was chosen as a cancer model, and Hank's balanced salt solution (HBSS) was used as a buffer medium for measuring O2 intracellular levels. Through CLSM imaging, efficient cellular uptake was tracked and captured with image acquisitions after 2 hours of incubation of R-UiO MOFs with CT26 cells. The authors carried out the experiments using three O2 concentrations: hypoxic (4 mmHg), normoxic (32 mmHg) and aerated (160 mmHg) conditions. The ratiometric calculations, obtained via the image analysis of the internalized R-UiO, corresponded to 5.1 ± 2.5, 27.3 ± 3.1, and 158 ± 11 mmHg; these data matched the theoretical O2 values, demonstrating the accuracy of the proposed method. A step forward in imaging oxygen at tissue levels has been performed in a reported work of early 2023 published by Wen et al., in which an afterglow/fluorescence dual-emissive ratiometric O2 sensor was engineered (Fig. 4c).162 In particular, the probe was based on a photochemical reaction-based afterglow system: the afterglow palladium(II) 1,4,8,11,15,18,22,25-octabutoxyphthalocyanine (PdPc) (λex = 730 nm; λem = 600 nm) was the indicator dye sensitive to variations in O2 concentration, while Nile Red (NR) (λex = 582 nm; λem = 600 nm) represented the unquenchable O2 dye. The reaction based on the ratiometric FL system occurred as follows: in the presence of O2, the FL intensity of the afterglow gradually increased because of the formation of singlet oxygen species, whereas the reference NR maintained its FL peak unperturbed. The encapsulation of the fluorophores within polystyrene particles (AGNPs) using the swelling method facilitated dyes confinement, oxygen penetration, good solubility and viability.162 The study by Wen and collaborators is significant because it is among the first studies on coupling afterglow/fluorescence sensing procedures for in vitro and in vivo experiments to explore the hypoxia environment in solid tumors. To this end, a mouse sarcoma S180 cell line was employed to test the cytotoxicity of the nanoparticles using a 3-(4,5-dimethylthiazol-2-yl)-2,5-diphenyltetrazolium bromide (MTT) assay. Finally, female athymic nude mice bearing mouse sarcoma cell-derived S180 and female athymic nude mice bearing no tumor were treated with AGNPs. After two weeks, the mice were imaged, showing a remarkable afterglow enhancement in mice with no tumor masses, while the afterglow signal was quite off in tumor bearing mice. In this way, the quantification of the O2 concentration, using the ratio of the afterglow signal versus fluorescence intensity, in solid tumors in the area of injection of the AGNPs showed a 4.94-fold lower intensity compared to the ratio obtained in normal tissues. The study by Wen et al. proposed to the scientific community a stable and accurate ratiometric sensor for O2 quantification in vitro and in vivo, but some efforts are still needed to improve the quantification of oxygen in deeper solid tumors.
2.3 Reactive oxygen species (ROS)
As mentioned previously, the most important ROS are hydrogen peroxide (H2O2), its reduction product hydroxyl radical (˙OH), and superoxide anion radical (O2˙−).70–72 In the complex landscape of TME, nanotechnologies play a fundamental role in ROS detection and monitoring. Consequently, in the last two decades, many efforts have been devoted to developing fluorescent probes for ROS sensing.163,164 Two mechanisms exist by which non-fluorescent ROS-sensitive dyes are activated: H2O2 selective cleavage and oxidation. In 2008, Srikun and co-workers165 published an internal charge transfer (ICT)-based approach to detect H2O2 in living cells using Peroxy Lucifer-1 (PL1) as a ratiometric fluorescent reporter. PL1 presents a 1,8-naphtalimide core structure with a 4-boronate-based carbamate protecting group that, once excited in the absence of H2O2, displays a blue emission peak (λex = 375 nm; λem = 475 nm). In the presence of H2O2, PL1 loses the boronate-based carbamate protecting group by chemoselective cleavage, returning the green fluorescent aminonaphthalimide (λex = 435 nm; λem = 540 nm). The biocompatible and ratiometric reactive dye PL1 was used to detect the endogenous concentration of H2O2 in RAW264.7 macrophages and HEK 293 T cells. Later on, Kim's group166 prepared silica nanoparticles decorated with PL1. The aim was to develop a new scaffold as a promising tool for monitoring hydrogen peroxide. Currently, neither in vitro nor in vivo applications of PL1-SiO2 particles have been reported in the literature. The most used probe for the detection of ROS and oxidative stress in cellular systems is the 2′,7′-dichlorofluorescein (DCF) dye. The enzymatic activity of esterases in the cytosol can convert the non-fluorescent diacetate form of 2′,7′-dichlorofluorescein (DCF-DA) in its hydrophilic form, generating a strong fluorescence response (λex = 488 nm; λem = 525 nm) deriving, in a particular manner, from the oxidation procured by H2O2 among other ROS. Kim and collaborators167 developed a ratiometric nanoPEBBLE sensor to quantitatively estimate the H2O2 generation from stimulated RAW264.7 macrophages in vitro. After DCF-DA encapsulation into the ormosil nanoparticle matrix, the nanoprobe surface was functionalized with a reference dye, Alexa568 N-succinimidyl ester, and a membrane penetrating peptide, cysteine-terminated TAT peptide, which guided the delivery of the sensing PEBBLEs directly into the cytosol. In the lab of Kazakova,168 the amphiphilic dye dihydrorhodamine 123 (DHR123) was employed as a sensing H2O2 unit in the fabrication of novel lactate microcapsule sensors. DHR123 is a non-emitting molecule, but in the presence of H2O2, it undergoes oxidation by generating the green emitting rhodamine 123 (λex = 488 nm; λem = 550 nm). The novelty reported by Kazakova is involved in the concept of enzyme-assisted substrate sensing. In fact, the encapsulation of lactate oxidase (LOx, an enzyme that catalyses the transformation of L-lactate into pyruvate and H2O2)169,170 coupled with the embedding of the amphiphilic ROS-sensitive fluorescent dye DHR123 onto the surface of calcium carbonate (CaCO3) capsules, via the LbL deposition of oppositely charged polyelectrolytes, enabled to monitor H2O2 over time using an optical approach. The data reported in this study confirmed that the increased fluorescence of the DHR123 fluorophore (λex = 488 nm; λem = 550 nm) is linearly correlated with the enzymatic activity of LOx and lactate concentration in the millimolar range. The results obtained here depicted the possibility of indirectly measuring lactate in the physio-pathological ranges and, by the further implementation of the following technique, paved the way for future monitoring of metabolites in vitro or in vivo.
Hydroxyl radicals (˙OH) are recognized as the most dangerous free radicals among ROS because they come from H2O2 reduction in metal-catalysed Fenton chemistry, involving free iron (Fe2+) ions. Liu et al.171 developed a dye-doped-ratiometric fluorescent probe coupling the fluorescence response of coumarin-3-carboxylic acid (CCA), as indicator dye for ˙OH detection and quantification, and 6-carboxy-X-rhodamine N-succinimidyl ester (ROX-SE), as a reference fluorophore (Fig. 5a). The blue-enhanced fluorescence emission of CCA is strictly linked to its reaction with ˙OH, which transforms CCA into 7-hydroxy coumarin 3-carboxylic acid product. The engineered ratiometric silica nanoparticles displayed a dual-emission fluorescent spectrum by employing a single excitation wavelength (λex = 395 nm; λem = 555–620 nm). After the analytical method was validated, the authors monitored the nanoparticle uptake and detected ˙OH levels in HeLa cells by live imaging. Importantly, cells incubated over time with 100 μM of ˙OH, in the presence of the sensing probe, displayed an increased blue fluorescent signal of CCA, whereas no changes in the red fluorescence of ROX were observed. Fluorescent sensing dyes, used as reporter and imaging agents, can be classified as FRET-based molecules when changes in the electronic interactions between a donor and an acceptor occur.117 In analytical chemistry and live imaging applications, this concept is significantly being considered in the scientific literature because it permits the selective detection of target analytes of particular biological importance, such as in the case of ROS. The diselenide bond (Se–Se) represents a highly reactive linker with specific selectivity towards ROS because it is oxidized into the selenic acid. Deepagan et al.172 successfully prepared an H2O2 on–off nanoprobe for an in vitro live imaging application (Fig. 5b). This nanosensor was based on the ability of gold nanoparticles to inhibit, in a distance-dependent manner, the fluorescence of surface-bound fluorescein isothiocyanate (FITC) dye via FRET in the absence of H2O2 (“off” state). When H2O2 concentration increased, the diselenide bond broke down selectively, releasing the free dye that could enhance its fluorescence (“on” state). The fluorescence characterization, performed in the presence of H2O2, demonstrated that the probe enhancement linearly depended on the concentration of hydrogen peroxide and allowed the authors to test the sensors in activated RAW264.7 macrophages to explore intracellular H2O2 changes over time. Other reactive groups, i.e. thiochetals,173 phenylboronic acids and thioethers,174 have been engaged as FRET-based sensors because they represent a nucleophilic anchor and a cleavable site for H2O2 species. An example of H2O2 sensitive FRET-based biosensor was developed by Feng's group.175 The authors indirectly detected the glucose concentration produced by measuring the enzyme-catalysed H2O2 production carried out by glucose oxidase (GOx). For the fabrication of their sensing system, a self-assembly technique was employed using two functionalized lipophilic polymers: 4-carboxy-3-fluorophenylboronic acid (FPBA)-modified DSPE-PEG (DSPE-PEG-FPBA) and 7-hydroxycoumarin (HC)-conjugated DSPE-PEG (DSPE-PEG-HC). The addiction of Alizarin Red S (ARS) to the probe unit represents novelty. ARS is a non-fluorescent molecule, but its ability to create adducts with boronic acids makes possible the generation of a fluorescent signal. In this way, the detection approach developed by Feng et al. was based on the selective cleavage of ARS from FPBA caused by increased concentrations of H2O2. When no ARS was present in the polymeric micelles, the nanoprobe displayed a fluorescence peak in the blue region of the spectrum due to HC (λex = 405 nm; λem = 450 nm). The subsequent addiction of ARS to the polymeric probe determined conjugation with FPBA and, consequently, the FRET phenomenon between the ARS-FPBA adduct and HC, which generated a new peak in the red region of the spectrum (λex = 405 nm; λem = 600 nm). A ratiometric fluorescence response could then be recorded in the presence of H2O2. Under this condition, ARS was decoupled from the probe, resulting in a decrease in the emission peak at λem = 600 nm and an increase in fluorescence for the peak at λem = 450 nm. From a future perspective, the intrinsic potential of the multifunctional polymeric fluorescent probe proposed by Feng and collaborators can contribute to biochemical studies of the cell microenvironment.
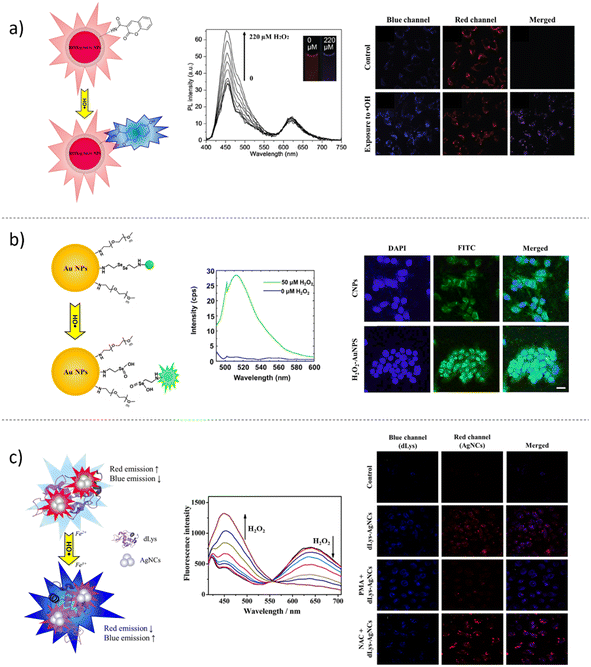 |
| Fig. 5 Examples of ROS sensing nano-platforms. (a) (Left): Schematic illustration of the dual-emission probe synthesis procedure and the working principle for ratiometric fluorescence detection of ˙OH; (middle): fluorescence spectra of the ratiometric probe solution upon the exposure to different concentrations of ˙OH at various H2O2 concentrations; (right): confocal fluorescence images of HeLa cells after being incubated with the dual-emission probe in the absence and presence of ˙OH. The images were collected at 410–520 nm (blue channel) and 580–680 nm (red channel) upon excitation at 405 nm. Scale bar: 20 μm. Reprinted with permission of Royal Society of Chemistry, from Liu et al., Analyst, 2016, 141, 7, 2296–2302; permission conveyed through Copyright Clearance Center, Inc. (b) (Left): Schematic illustration of the H2O2-sensitive on-off H2O2-AuNPs; (middle): H2O2 responsive fluorescence spectra of H2O2 sensitive-AuNPs; (right): in vitro confocal microscopic images of activated RAW264.7 cells incubated with the CNPs and H2O2 –AuNPs for 3 hours at pH 7.4. Scale bar: 20 μm. Reprinted from Deepagan et al., Macromol. Res., 2018, 26(7), 577–580. Copyright © 2018, The Polymer Society of Korea and Springer Science Business Media B.V., part of Springer Nature. (c) (Left): Schematic illustration of the fluorescent responding mechanism of dLys-AgNCs to Fenton Reagents; (middle): fluorescence spectra of the ratiometric NPs towards different H2O2 concentrations; (right): fluorescence confocal images of PC-3 cells alone (first raw), PC-3 cells treated with dLy-AgNC probe (second raw), PC-3 cells incubated with PMA (third raw) and NAC (forth raw) prior to treatment with dLys-AgNCs. Adopted from ref. Liu et al., Anal. Chem., 2016, 88, 21, 10631–10638. Copyright © 2016 American Chemical Society. | |
QDs have also been successfully employed for H2O2 detection. For instance, Zhou et al.176 described the preparation and application of TGA-capped Si–CdTe dual-emissive QDs for the selective monitoring of H2O2 in the intracellular space of HeLa cells. The coupling of Si-QSDs with CdTe QDs represented an innovation not only because of the addiction of a nanosized fluorescent unit but also because it skips the cytotoxicity problem using a silicon-based material, which has excellent solubility in water, great stability, and is easy to obtain. The FRET-sensing system reported by Zhou's group was based on the fluorescence intensity of blue Si-QDs (λex = 370 nm; λem = 442 nm) and red CdTe QDs (λex = 370 nm; λem = 562 nm). By gradually increasing the H2O2 concentration, the TGA-capping broke down; consequently, CdTe QDs fluorescence was reduced, whereas the Si-QDs emission became stronger in a linear relationship. By CLSM analyses of HeLa cells cultured with Si–CdTe QDs, the authors established a valid method to monitor H2O2 levels following nanoparticle uptake. Metallic nanoclusters (MNCs) are metal-centred nanoparticles stabilized by protective groups, usually biological molecules; for this reason, their employment as novel platforms for fluorescence sensing in vitro and in vivo has been increasing over the years. Among their properties, it is important to remark water-solubility and biocompatibility.125,126 Liu et al. fabricated a dual-emissive fluorescent ratiometric probe for H2O2 and ˙OH sensing based on lysozyme-capped silver nanoclusters (dLys-AgNCs) (Fig. 5c).177 The fluorescent ratio, obtained between the quenching of the red peak at 640 nm and the enhancement at 450 nm due to ˙OH-induced oxidation of the tyrosine residue present in lysozyme, was linearly correlated with the H2O2 concentration. Live imaging experiments, performed using PC-3 cells after incubation with dLys-AgNCs, confirmed the ratiometric measurements. As a proof of concept, to the sensing system composed of PC-3 cells and dLy-AgNC probe, the authors added phorbol-12-myristate-13-acetate (PMA) and N-acetyl-cysteine (NAC), an ˙OH generation stimulator and a free radical scavenger, respectively. The fluorescence confocal analysis strengthened the ability of the dLys-AgNCs to detect and monitor in vitro changing levels in ˙OH.
2.4 Inorganic cations and anions (Ca2+, Na+, K+, and Cl−)
The leading phenomena that characterize the TME, such as acidosis, hypoxia and ROS generation, and drag with them also the dysregulation of ion fluxes, contributing to the enhancement of the cancer disorder and strong perturbations inside cells and in their surroundings. Involved in cancer processes, such as proliferation, invasion and metastasis, ions Ca2+, Na+, K+ and Cl− are the main players of cell–cell interactions and ECM digestions favouring the activation of specific signalling pathways.178
Calcium cations, Ca2+, are involved in many cellular processes and signalling pathways; in particular, it is essential in muscle contraction, osteogenesis and neurotransmission.179 Its concentration is kept constant in body fluids, which is around 100 nM in the cytosol and way higher in blood and interstitial fluids, around 2 mM.180 These concentration differences should be maintained while designing intracellular or extracellular sensors. There are different FL probes for calcium cation imaging, and some of them are commercially available, such as fura, indo and fluo dyes. All these compounds contain multiple carboxylic functions that act as chelators for Ca2+, in an “EDTA-like” motif, with different selectivities for other bivalent cations.80,181 Si et al. reported the development of PEBBLE (Photonic Explorers for Bioanalysis with Biologically Localized Embedding) ratiometric nanosensors for Ca2+ imaging. The nanosensor was obtained by embedding the Ca2+ sensitive dye, Rhod-2, in polyacrylamide nanogels. To obtain a ratiometric probe, the Ca2+ insensitive dye Hilyte™ 647 was covalently conjugated to the nanogel surface. These PEBBLEs showed a Kd of 500–600 nM and were tested on PC-3 human prostate cancer cells for intracellular live imaging.182 Lin et al. reported the synthesis and characterization of fluorescent Ca2+ nanosensors based on CDs. Fluorescent CDs were synthesized by the pyrolysis of citric acid, purified by applying dialysis and covered with a Ca2+ binding peptide (Fig. 6a). The peptide sequence was based on the EF-Hand domain of human calmodulin. The binding of Ca2+ cations to the nanosensors was able to quench the CD fluorescence, showing a sensitivity range in the micromolar range of concentration.183 The toxicity of the nanosensors was evaluated on SH-SYS5 human neuroblastoma cells through MTT assay, proving the low toxicity of the nanoprobes. Schulz et al. reported the synthesis of Ca2+ cation nanosensors based on hybrid silica-dextran nanoparticles.184 Silica nanoparticles were obtained by employing a modified Stöber method, doped with aminopropyl triethoxysilane (APTES) and covalently linked to RBITC. Fluo-4-modified aminodextran was used to form the outer shell around the silica. The conjugation was performed using disuccinimidyl carbonate as a linker between aminodextran and amino-modified nanoparticles. The ratiometric sensor showed a sensitivity range from 0 to 39.7 μM of Ca2+, with a kd of 780 nM, which can be suitable for intracellular Ca2+ imaging.
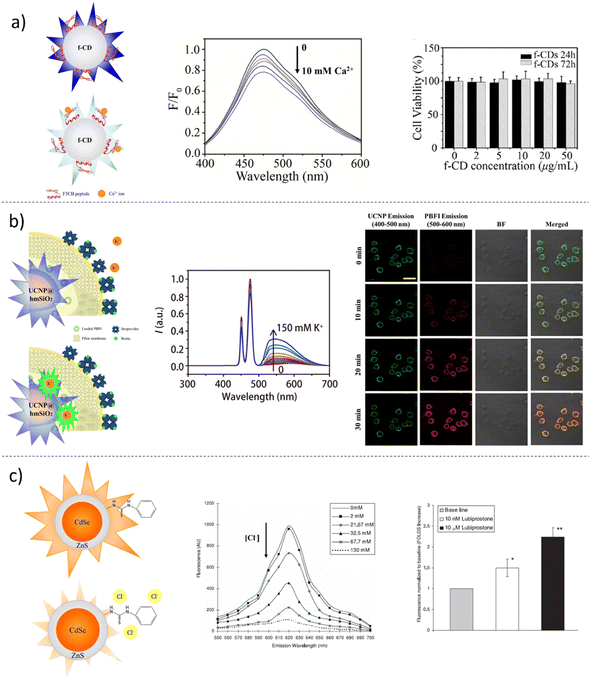 |
| Fig. 6 Examples of ion ratiometric sensing platforms. (a) (Left): Schematic illustration of the peptide-functionalized carbon dots (f-CDs) that operate as Ca2+ nanosensors; (middle): fluorescence emission spectra of f-CDs at various concentrations of Ca2+ (λex = 350 nm); the binding of calcium cations can quench the fluorescence emission of f-CDs; (right): cell viability assay on SH-SY5Y cells incubated at various concentrations of f-CDs for 24 and 72 hours. Reprinted with permission from Lin et al., Sens. Actuators, B, 2018, 273, 1654–1659. Copyright © 2018 Elsevier B.V. All rights reserved. (b) (Left): To the right: Schematic illustration of the K+ nanosensors; the upconverting nanoparticles (UCNPs) are coated with PBFI-loaded silica and an outer shell of K+ permeable film; (middle): fluorescence emission spectra of the nanosensors at various K+ concentrations (λex = 808 nm); (right): CLSM micrographs of HEK 293 cells labelled with K+ nanosensors, showing fluorescence emission at 400–500 nm and 500–600 nm. The potassium cation efflux, after the treatment with 5 μM nigericin, 5 μM bumetanide, and 10 μM ouabain, is verified by the fluorescence enhancement of the PBFI. Reprinted with permission of ref. (Liu et al., Sci. Adv., 6, eaax9757, 2020); figure licensed under a Creative Commons Attribution NonCommercial License 4.0 (CC BY-NC). (c) (Left): Schematic illustration of the Cl− nanosensors; CdSe/ZnS quantum dots are capped with the chloride sensitive thiourea; (middle): fluorescence emission spectra of the Cl− nanosensors at increasing concentration of chloride (λex = 425 nm); (right): fluorescence emission of T84 cells incubated with the nanosensors and treated with Lubiprostone, showing the efflux of chloride anions from cells. Reproduced with permission from ref. Wang et al., Nanotechnology, 2010, 21, 055101. Copyright © 2010 IOP Publishing. All rights reserved. | |
Sodium cations are the most abundant inorganic cations in the human body. They play a fundamental role in physiology, being involved in neurotransmission, muscle contraction and blood volume regulation.179 The Na+ concentration in bodily fluids can significantly vary. The typical concentration in blood is 135–150 mM, in the interstitial fluids is around 142 mM and in the cytosol is always maintained low at around 5–30 mM by the activity of ion pumps (such as Na+/K+ ATPase).185 Fluorescent probes for Na+ and K+ imaging chemically differ from the Ca2+ ones. Instead of having polycarboxylic moieties, they have a typical ether crown, that can bind monovalent cations, and a fluorescent aromatic core.80 One of the most used molecular probes for Na+ cation imaging is SBFI (Sodium-Binding Benzofuran Isophthalate), which is 20-fold more selective for Na+ compared to K+, with a Kd of 20 mM, making SBFI useful for intracellular imaging. Sodium Green is another Na+ sensitive fluorophore, with higher selectivity over potassium (41-fold more selective) and a lower Kd of 8.4 mM, which facilitates the detection of even smaller variations in sodium concentration. Other Na+ sensitive fluorophores are CoroNa (with a Kd of 80 mM), ANa1 and ANa2.185 Dietrich et al. reported the synthesis of Na+ sensitive ratiometric nanosensors based on gold nanoparticles.186 Sodium Green was used as the sensitive dye, while Texas red was used as the reference dye and both were embedded in the nanosensors by covering gold nanoparticles with layers of poly(vinylalcohol) and polyacetal. Nanosensors were incubated with CHO cells (Chinese hamster ovary cells), and Na+ intracellular variations were monitored during the treatment with ionophores nigericin, gramicidin and monesin. Wang et al. reported the synthesis of ionophore-based fluorescent ratiometric nanosensors for Na+ and K+, by exploiting graphene quantum dots (G-QDs).187 G-QDs were modified with propargyl bromide, and then a Cu2+-catalysed Huisgen addition was used to coat the G-QDs with polyoxyethylene bis(azide). Sodium sensitive nanosensors were obtained by mixing PEG-GQDs with sodium ionophore X (NaX), sodium tetrakis-[3,5-bis(trifluoromethyl)-phenyl] borate (TFPB), bis(2-ethylhexyl) sebacate (DOS) and the oxazinoindolines (OX-R).188 To obtain K+ nanosensors, valinomycin was used instead of NaX. Both nanosensors displayed a sensitivity range between 0.1 mM and 1 M. One of the main limits in the use of this chromoionophore system is the interference of pH values in the readout of the sensors. However, Na+ sensors were tested using HeLa cells to evaluate toxicity, showing good biocompatibility. To assess whether the nanosensors can detect alteration in the intracellular environment, HeLa cells were incubated with nanosensors and treated with gramicidin189 and carbonyl cyanide 3-chlorophenylhydrazone (CCCP),190 which act as ionophores on cell membranes. Fluctuation in fluorescence emission was monitored by applying CLSM, showing a decrease in intracellular Na+ levels.
Potassium cations (K+) are the most abundant cations in the intracellular compartment, with a mean concentration range of 140–150 mM, while in blood and extracellular fluids, it is lower at 3.5–5 mM.191 K+ cations play a crucial role in neurotransmission, muscle contraction, and insulin release and are also involved in pathological events, such as epilepsy, cardiac arrhythmia and cancer.191 There are different fluorescent molecular probes for potassium cation imaging. The most widely employed probe is Potassium-Binding Benzofuran Isophthalate (PBFI), which suffers from poor selectivity between Na+ and K+, and an excitation peak in the far UV, with low penetration capability and potentially harmful for living cells.192 The Asante potassium green (APG or IPG) family of fluorophores is more selective compared to PBFI, with an excitation peak in visible light and with different Kd.192 To overcome the limitation of PBFI as sensitive probes, Liu et al. reported the synthesis of K+ nanosensors based on upconverting nanoparticles.193 These nanoparticles display the ability to convert two or more photons into one photon with higher energy.194 In this case, NaYF4:Yb/Tm nanoparticles were coated with silica, and then PBFI was embedded in an ion-selective polymer. This nanosensor design allowed for the excitation of PBFI with a near-infrared wavelength at 800 nm, increasing the tissue penetration capability. Moreover, the low selectivity of PBFI was improved by the ion-selective polymer. The shielded sensors displayed a sensitivity range between 2.8 μM and 150 mM of K+. Nanosensors were tested on HEK 293 cell to assess their ability to monitor fluctuations in the extracellular environment using nigericin and bumetanide (the first as an ionophore and the latter as an inhibitor of the Na–K–Cl co-transporter).195 Liu et al. reported the synthesis of K+ nanosensors based on silica nanoparticles.196 The silica nanoparticles were covered with an ion-selective polymer to trap the Asante potassium green 2 (APG-2) (Fig. 6b). This nanosensor showed a sensitivity range from 1.3 μM to 150 mM and was used in both ex vivo and in vivo models of murine epilepsy to monitor K+ variations. Ruckh et al. reported the synthesis of a ratiometric K+ nanosensor based on QDs.197 The system comprised two QD species with non-overlapping emission spectra: a non-fluorescent chromoionophore and an ionophore. The protonation state of the chromoionophore affected its absorption spectra, with a consequent effect on the QD emission spectra. The final readout was derived from the ratio of the two QD species. The nanoparticles based on this system and embedded with a plasticizer displayed a sensitivity range of 2–120 mM. The nanoprobes were tested on HEK 293 cells to assess their ability to monitor K+ fluctuations in the extracellular environment.
The chloride anion (Cl−) is the most abundant anion in the human body and the most important in electrophysiological regulation. It plays a crucial role in neurotransmission and is also involved in pathological conditions, such as cystic fibrosis.80 The chloride concentration inside cytosol can vary significantly in different cell lines, while in plasma and in interstitial, the concentration is maintained constant by kidney filtration at approximately 100 mM.198 Most electrophysiological studies conducted on the Cl− role in physio-pathological processes are performed with patch clamps, ion-selective electrodes and chloride radioisotope. However, different fluorescent molecular probes are commercially available for Cl−, such as lucigenin (bis-N-methylacridinium nitrate), SPQ (6-methoxy-N-(3-sulfopropyl)quinolinium), MEQ (6-methoxy-N-ethylquinolinium), and BAC (10,10′ bis[3-carboxypropyl]-9,9′-biacridinium dinitrate). Ruedas-Rama et al. reported the synthesis of Cl− nanosensors based on semiconductor QDs and lucigenin.199 Hexadecylamine-capped CdSe/ZnS QDs were modified with 3-mercaptopropionic acid (MPA), and lucigenin was bound to nanoparticles by simple electrostatic interactions between the positively charged acridine and negatively charged MPA. The lucigenin fluorescence was quenched by Cl− owing to a charge transfer mechanism. However, the QD–lucigenin conjugate showed increasing fluorescence emission in the QD spectrum, related to the competing action of FRET between QDs and lucigenin, and charge transfer between lucigenin and Cl−. The calibration showed how the nanosensors could be used through FLIM or with the ratio between QD and lucigenin emissions. The system proved to be selective toward other anions and sensitive in the Cl− concentration range of 0.5 mM to 50 mM. Wang et al. reported the synthesis of Cl− nanosensors based on semiconductor quantum dots and a novel thiourea moiety. The 1-(2-mercapto-ethyl)-3-phenyl-thiourea was synthesized and used for the capping of CdSe/ZnS QDs (Fig. 6c).200 The nanosensors displayed sensitivity in the range of Cl− concentration from 2 mM to 130 mM. A comparison between the MEQ and nanosensors was performed using CF-PAC human epithelial cells. The two probes were embedded into liposomes and administered to CF-PAC cells treated with glibenclamide (as a chloride channel inhibitor). The nanosensors exhibited better sensitivity in monitoring intracellular Cl− fluctuations, compared to MEQ by employing FLIM analyses.
2.5 Biomarkers
Macromolecules, such as nucleic acids, proteins, metabolites, isoenzymes and hormones, are well recognized as characteristic signatures of cancer onset and progression.201 Biomarkers are molecules or substances that can indicate the presence or progression of a disease, and they are classified into three main categories in clinical practice: (i) diagnostic biomarkers, which are used for disease detection; (ii) prognostic biomarkers, which provide information about the likelihood of disease recurrence; and (iii) predictive biomarkers, which can help determine the patient's response to cancer treatment.201 The most used methods to detect and quantify biomarkers are based on enzyme-linked immunosorbent assay (ELISA)202 and polymerase-chain-reaction (PCR)-based protocols.203 Although they are widely accepted as crucial procedures in cancer diagnosis and treatment, these technologies are not without limitations. One significant challenge is the slow reaction mechanism of detection, which can delay diagnosis and treatment initiation. Additionally, the exorbitant cost of reagents required for these techniques can result in high expenses for patients, limiting their accessibility and affordability.202,203 Currently, there are only few published studies on ratiometric fluorescence-based nano-systems for cancer biomarkers.204
A strong correlation between extracellular pH acidification and the increased expression of tumor-related proteases is associated with the invasion and dissemination of cancer cells in other organs.205,206 The quantification of matrix metalloproteases-2 (MMP-2) in the blood is still a great challenge because of the complexity of the biological fluid. In this context, Wang et al.207 engineered an upconversion FRET-based biosensor to target MMP-2 particularly. The authors recorded a linear and proportional relationship between MMP-2 concentration and fluorescence recovery of the sensing system in the range of 10–500 pg mL−1. The validation of the bioanalytical sensors was carried out by collecting human plasma and whole blood samples. In the last 20 years, several efforts have been made to construct sensing platforms that can track the activity of proteases, exploiting their detection for the early diagnosis of cancer. An example is represented by the FL-gold nanoparticle activatable probes.208–210 In 2008 and 2009, Lee and collaborators developed a NIR-FL gold nanoparticle that was selective for matrix metalloproteases-2 (MMP-2). Owing to its specificity, the developed probe facilitated the simple monitoring of the activities of MMP-2, both in vitro, using HepG2 cell line, and in vivo, adopting mice bearing SCC7 (squamous cell carcinoma) tumors.211,212 In another work by Yin et al.,213 an MMP-2 activatable probe was prepared by covalently coupling a near infrared dye (Cy5), a quencher (QSY21), a tumor targeting peptide (cyclic Arg-Gly-Asp) and a radionucleotide 125I-labeled peptide substrate. The developed probe, which light-up upon proteolytic cleavage operated by active MMP-2, was employed for accurate detection via NIRF and single-photon emission computed tomography (SPECT) imaging techniques of the metastatic lymph nodes (MLNs) in mice bearing murine breast carcinoma cell line 4T1 before and during treatment with an MMP-2 inhibitor. The above-reported examples represent a fundamental application of highly selective and sensitive FL-based platforms and represent milestones in the field of ratiometric probes. In 2018, Ma et al. created an FL ratiometric probe to track matrix metallopeptidase-9 (MMP-9) activity and extracellular pH, both in vitro and in vivo (Fig. 7a).214 The sensing platform was architected as follows: biocompatible-PEGylated iron oxide (Fe3O4) magnetic NPs were chosen as the sensor support material; the sensing units were represented by the pH-sensitive naphthalimide dye ANNA (λex = 455 nm; λem = 510 nm) and labelled with a peptide substrate of MMP-9 (GGKGPLGLPG), and the reference dye Cy5.5 (λex = 675 nm; λem = 695 nm) were covalently linked to PEGylated Fe3O4 NPs. The FRET-based mechanism determined the FL “off” state when the pH fluorophore ANNA was bound onto the surface of Fe3O4 NPs. The cleavage of the peptide substrate, operated by MMP-9, contributed to the FL enhancement of ANNA dye, which transitioned to the “on” state because of its protonation. Employing time-lapse CLSM, the ratiometric linear regression obtained by plotting IANNA/ICy5.5 allowed the authors to detect and quantify MMP-9, following the incubation of the ratiometric sensing probe in human colorectal cancer cell line LS180, which is known to overexpress MMP-9. To further validate the analytical platforms, the authors injected a ratiometric probe via the rat vein of tumor-bearing mice. The results showed that the nanoprobes were activated in the tumor site 4 hours post-injections, and the pH mapping and MMP-9 quantification, executed by image analysis, confirmed the possibility of real-time monitoring of multiple TME targets.
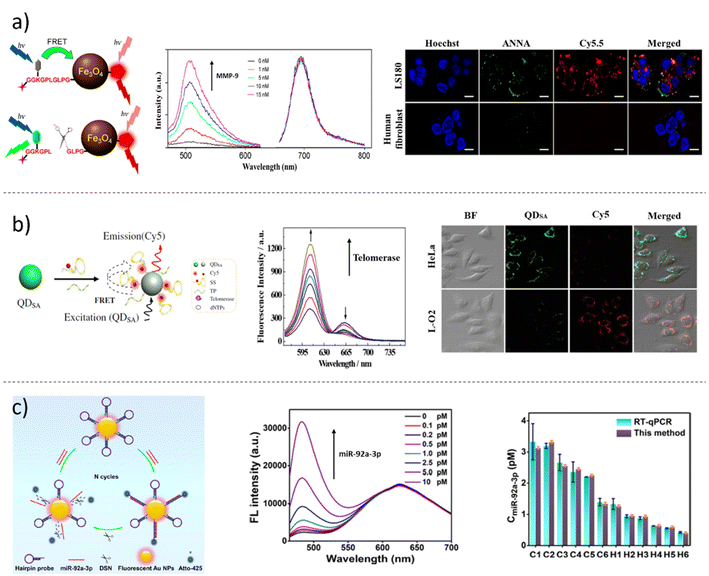 |
| Fig. 7 Examples of biomarker sensing nano-platforms. (a) (Left): Schematic illustration of the working mechanism of Fe3O4 MMP-9 activity sensing NPs; (middle): fluorescence spectra recorded after the nanoprobes were incubated with different concentrations of activated MMP-9; (right): confocal microscopy images of LS180 cells (top row) and human fibroblast control cells (bottom row) obtained after incubation with the nanoprobe for 6 h and then imaged through different channels according to the dye emissions (cell nuclei were stained with Hoechst, and the scale bar corresponds to 10 μm). Reprinted with permission from Ma et al., J. Am. Chem. Soc., 2018, 140, 1, 211–218. Copyright © 2018, American Chemical Society. (b) (left): The QDsSA@DNA nanoprobe for monitoring of telomerase activity in situ; (middle): fluorescence emission spectra of the designed QDsSA@DNA nanoprobe (100 nM) in response to telomerase extraction from different numbers of HeLa cells; (right): confocal fluorescence microscopy imaging of HeLa and L-O2 cells incubated with the QDsSA@DNA nanoprobe for 4 h. The concentration of the added QDsSA@DNA nanoprobe was 100 nM. Scale bar: 25 μm. Reprinted with permission from Ma et al., Anal. Bioanal. Chem., 414, 1891–1898 (2022). Copyright © 2022, Springer-Verlag GmbH Germany, part of Springer Nature. (c) (left): Schematic of the ratiometric fluorescent detection of miR-92a-3p based on fluorescent Au-NP and DSN-assisted signal amplification; (middle): fluorescence spectra of the biosensor under different concentrations of miR-92a-3p; (right) comparison of the exosomal miR-92a-3p concentrations of CRC patients and healthy controls detected by RT-qPCR and this method (n = 3, mean ± s day). C1–C6 represents CRC patients; H1–H6 represents healthy controls. Reprinted with permission from Sun et. al., Bioconjugate Chem., 2022, 33, 9, 1698–1706. Copyright © 2022, American Chemical Society. | |
Another interesting target in clinical routine is telomerase, a transcriptase responsible for unlimited cancer proliferation.215,216 For this reason, today, it is considered a diagnostic and prognostic biomarker. A QD-based ratiometric FL sensor, with an FRET mechanism, was developed by Ma et al. to specifically target intracellular telomerase activity (Fig. 7b).217 The nanoprobe comprised a core–shell streptavidin-modified cadmium selenide/zinc sulphide core–shell (CdSe/ZnS) QD (QDsSA) (λex = 235 nm; λem = 600 nm), which was a functionalized with a telomerase primer (TP) and a signal switching sequence (SS), which was designed to form a hairpin filament complementary to telomerase. To obtain a sensing system, the SS was labelled at its 5′ end with cyanine 5 (Cy5) (λex = 630 nm; λem = 665 nm) that acted as an FL acceptor. Thus, once assembled, the nanoprobe exhibited only the FL of Cy5 because of the FRET-phenomenon; in contrast, in the presence of telomerase, the TP was recognized and elongated by telomerase, hybridized with SS to form a double-strain and disassembled from the nanoprobe by providing the switch-on of QDsSA and amplification of the FL signal, yielding a significant ratiometric FL change (FQDsSA/FCy5). After optimization under the best sensing conditions, the nanoprobes were incubated with different interfering biomolecules, such as bovine serum albumin (BSA), immunoglobulin G (IgG), lysozyme, thrombin, trypsin, ATP, RNA, and Bst DNA polymerases. For none of these, the probe showed significant FL changes, thus presenting strong selectivity for telomerases. To map telomerase activity in vitro, the authors employed two types of cells: cervical carcinoma HeLa cells and human hepatocyte cells (L-O2). As expected, in cancerous HeLa cells, the green fluorescence of QDsSA was visible, reflecting high telomerase activity, whereas the opposite result was obtained in healthy L-O2 cells. These results confirmed that intracellular telomerase can now be detected and monitored in a spatio-temporal manner owing to ratiometric FL platforms to be used, in the near future, as promising tools for diagnostic cancer screening and telomerase-targeted anticancer drugs.
With the advent/irruption of cancer liquid biopsy, the possibility of screening and detecting a high number of circulating tumor biomarkers, such as extracellular vesicles, proteins, nucleic acids, and microRNAs (miRNA), using simply a blood sample has become a reality.218,219 A smart example of sensing colorectal cancer (CRC)-associated exosomal miRNA (miR-92a-3p), by developing ratiometric FL nanoparticles, was described in the work by Sun and collaborators (Fig. 7c).220 The nanoprobe was composed of a hairpin DNA, labelled with sulfhydryl Atto-425 (λex = 443 nm; λem = 482 nm) on the 5′ end and 3′ end, and conjugated to the surface of a fluorescent gold NPs (Au-NPs) (λex = 320 nm; λem = 625 nm). In this way, the FL emissive peak of Atto-425 was quenched using a FRET mechanism by the FL of the Au-NPs. In contrast, in the presence of miR-92a-3p, Atto-425 was unbound from the surface of the Au-NPs, recovering its fluorescence. The detachment of Atto-425 was coordinated by the activity of a duplex-specific nuclease (DSN), which cleaved the DNA filament in a miR-92a-3p/DNA heteroduplex, keeping the RNA fragment intact. This process drove to signal amplification because the free miR-92a-3p could open another hairpin DNA, repeating the sensing cycle in this way. The ratiometric calibration curve, carried out plotting the IAtto425/IAu-NPs, in a concentration range of 0.1–10 pM miR-92a-3p presented a linear regression fit of 0.995 and a limit of detection of 45 fM, thus improving the sensitivity in miRNA detection. After the lysis of exosomes, exosomal miR-92a-3p was extracted from the sera clinical samples of 3 CRC patients and 6 healthy controls. The results in the detection and quantification of miR-92a-3p extracted from patient and control samples using the ratiometric FL nanosensors agreed with those obtained by adopting real-time quantitative PCR (RT-qPCT). Thus, the biotechnological analytical platform can be considered a promising device and can be adopted as a potential tool in clinical diagnosis.
3. Hybrid materials/systems including fluorescent nano-microparticles for biomedical applications
Nowadays, monitoring the metabolic variations in TME in toto and simultaneously providing real-time detection at a single-cell scale are still challenging cues in cancer, tissue engineering and regenerative medicine.221 The combination of diverse nanotechnologies has recently led to the development of integrated sensing devices that can reveal the spatio-temporal behaviour of cells using high-resolution and computational methods. Therefore, particle-reinforced biocomposites are treated, in which the dispersed phase is represented by smart fluorescent nano- and microparticles for medical therapeutic and/or diagnostic purposes. The manipulation of parameters such as the volume ratios of the components and matrix type, including particle size and nature, geometry, orientation and distribution, offers wide design flexibility. Matrix materials of biomedical interest are represented by fibrous matrices based on biopolymers because they replicate the organization and biological behaviour of the extracellular matrix.222 ECM-like fiber mats can be produced by electrospinning, which is a cost-effective method to fabricate fibers with diameters ranging from nanometers to microns.223 Electrospun fibers exhibit many advantages, including tunable composition and size, tunable alignment, and the possibility of being loaded with drugs and stimuli-responsive nanomaterials to enable controlled and sustained release via physiological or physical stimuli.224 Because of their size and surface features, nanofibers show a high surface-to-volume ratio and porosity, which favour the transport of small molecules as ions, making them a particularly attractive platform for the development of ultrasensitive sensors. Thus, nano- and microparticle-based sensors can be dispersed in the polymer solution and entrapped by electrified jets within the lumen of the electrospun fibers, forming functional optical regions within the mats. The use of electrospinning to produce sensing matrices has been successfully reported by many groups.225,226 For example, pH-sensing electrospun fiber scaffolds were produced by embedding pH sensors for the ratiometric measurement of local proton concentration, with high spatial resolution and in a fast and non-invasive manner.227,228 The fluorescence changes in the functional regions were correlated with H+ concentration during the spatio-temporal measurements of the extracellular acidity of pancreatic tumors and stromal co-cultures. Single-cell fermentation flux analysis, conducted via constraint-based inverse modelling, demonstrated that H+ trafficking was strongly heterogeneous with just few cells showing high activity, and, therefore, responsible for a large fraction of the pH gradient in the cell culture (Fig. 8a).228 Nano- and microparticles have also been incorporated in biopolymer-based hydrogels, which is a widely used class of materials in tissue engineering, ophthalmic, wound healing and drug delivery owing to their properties, such as biocompatibility and biodegradability.229 Particle sensors can be easily dispersed within hydrogels, and because of their macromolecular polymer network structure and hydration, the mobility of small ions does not decrease significantly compared to their diffusion in aqueous solutions.230,231 Among hydrogels, alginate is a naturally occurring biopolymer that has many applications owing to its biocompatibility, low cost, ease of gelation and optical transparency, which make it highly suitable even for microscopy applications. Very recently, alginate-based three-dimensional microgels were produced using an electrostatic droplet encapsulation method to embed FITC-RBITC SiO2 pH sensors together with pancreatic tumor and/or stromal cells (Fig. 8b).232 The method involved the use of high voltage to obtain droplets of an average diameter of around 200 μm, which were crosslinked in a solution of calcium chloride. Extracellular pH metabolic variations were monitored by applying 4D (x, y, z, t) live-cell imaging, showing that the pH was cell line-specific and time-dependent. In addition, differences in acidification were measured in 3D mono-against 3D cell co-cultures, suggesting the existence of cancer-stromal cell crosstalk resulting from metabolic cell reprogramming to a glycolytic phenotype.233 The same droplet encapsulation method with alginate has also been used to incorporate pH-sensitive carbon nanoparticles for measuring pH during bacterial cultures. Ratiometric detection was assessed by calculating the ratio 550 nm/450 nm of fluorescence emissions that was plotted against the incubation time to obtain the growth rate of bacteria. The results showed that the emission ratio increased, and therefore the pH decreased over time, reflecting the growth of bacteria.234 Luminescent amphiphilic carbon dots (CDs) nanoparticles were also embedded within an ascorbic acid derivative hydrogel to detect reactive oxygen species (ROS). ROS induced the oxidation of the ascorbic acid units with consequent collapse of the hydrogel, the aggregation of the CDs and therefore quenching of their luminescence, monitored under ultraviolet (UV) excitation. CD-hydrogel was applied as a sensing platform to detect in vitro the presence of ROS from HeLa cells, whether exposed to 5-fluorouracil (5-FU) or not. 5-FU is a chemotherapeutic known for generating intracellular ROS, leading to apoptosis of cancer cells.235 Therefore, quenching of the CDs-hydrogel revealed the efficacy of the treatment, demonstrating the potential of the system for drug screening applications.235 Recently, Delic et al. developed another fluorescent composite material from CDs. To preserve the intrinsic fluorescence in the dried solid state and in aqueous solution over a broad pH range (pH = 3–12), nanoparticles were dispersed and embedded throughout silica particles. Owing to hydrothermal treatment at low temperatures in the presence of urea and citric acid, a final fluorescent macroporous hollow structure, ideal for drug storage and delivery systems, was obtained.236 Another ratiometric fluorescent microgel was produced by Li et al. from polyurethane (PU), a material widely used in medicine owing to its stability and biocompatibility. pH-sensing nanoparticles were loaded within the PU to obtain a final particle size distribution of around 75 μm and spherical morphology. Nanoparticles were synthesized by cross-linking denatured pH-sensitive bovine serum albumin proteins and pH-insensitive Nile Red as a reference and exhibited linear reversible fluorescence in response to pH ranging from 6 to 10. The system was used to study, through colorimetric maps, local extracellular pH during biomaterial degradation because this phenomenon could significantly affect the surrounding cells; for example, it could affect the balance between bone formation and resorption.237 Fluorescent microparticles were also included in a polymer layer to create an integrated sensor for monitoring the oxygen gradients of hypoxic tumors. In particular, silica microparticles were first absorbed with oxygen-sensitive (Ru(dpp)3Cl2) and insensitive (Nile Blue chloride) fluorophores and then mixed with polydimethylsiloxane (PDMS). The mixture was then poured onto the pillars of a microfluidic device to recreate insulated oxygen conditions on the two sides of a monolayer of human breast cancer cell MCF7 and, therefore, hypoxic levels in the monolayer. After 24 hours of cell culture, enhanced fluorescence of ruthenium was observed at the center of the pillar, which decreased radially. The ratiometric ruthenium by Nile Blue intensity was plotted against the radial distance; molecules and proteins regulated by hypoxia were immunostained and correlated with the oxygen gradient.238 To monitor oxygen concentration within large 3D scaffolds, Wilson et al. reported the synthesis via organic-in-oil suspension of fluorescent hydrogel MPs containing palladium(II) meso(tetracarboxyphenyl)-porphine as an oxygen-sensitive fluorophore and Alexa Fluor 633 carboxylic acid tris(triethylammonium) salt as reference fluorophore. MPs were encapsulated into a cellularized hydrogel scaffold, and oxygen gradients were measured via ratiometric imaging, showing that the signals were robustly photostable and unaffected by hydrogel thicknesses of over 2 mm.239 Another approach for monitoring oxygen gradients within a hydrogel environment, providing real-time information for ensuring efficient cell functions, involved the development of functional fluorescence-based nano-oxygen particles (FNOPs). In particular, pluronic F127-grafted polystyrene beads (PSBs) were linked with the commercially available oxygen-sensitive fluorescent molecule Ru(dpp)3Cl2 through hydrophobic interactions. FNOPs were applied to measure the oxygen concentration of RIN-m5F/HeLa cell lines in hydrogel spheres of 700–1000 μm in diameter generated by the electrospray technique for more than 5 days.239 Oxygen concentration can also be probed using a confocal phosphorescence life-time microscope
(PLIM).98 Commercially available oxygen microsensor beads were mixed with the cell suspension in the presence or absence of gelatin methacryloyl (GelMA) as a photocrosslinkable hydrogel matrix, prior to seeding in static 2D, 3D cultures or in single or 8-channel array perfusion chips. To map and predict oxygen distributions for different cell densities, media and cultured cells, oxygen-dependent phosphorescence decay profiles of the microsensors can be converted through a calibration curve to oxygen concentration (Fig. 8c).240 Finally, hybrid systems, including pH-fluorescent sensors, were developed by combining different technologies to probe 3D cell growth and tissue regeneration.241 Capsules based on SNARF-1 were included in a 3D additive-manufactured scaffold with controlled geometry and porosity by providing a real-time detection of the acidification of human mesenchymal stromal cells. pH in the cell microenvironment showed a reduction after 7 days, which was more prominent at the edges of the 3D scaffolds, demonstrating the importance of considering the position of the sensors within a scaffold to detect smaller pH gradients that exist spatially around the cells.242
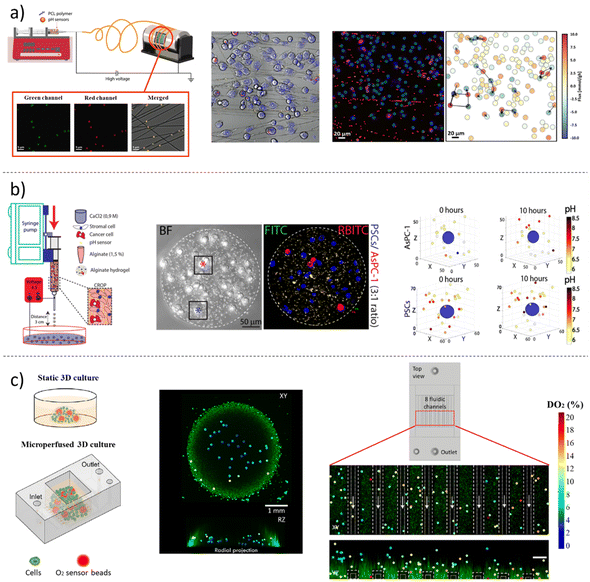 |
| Fig. 8 Examples of hybrid materials/systems including fluorescent nano-microparticles for biomedical applications. (a) (left): Sketch showing the fabrication of electrospun polycaprolactone (PCL) fibers embedding ratiometric SiO2-based microparticle sensors and representative CLSM micrographs showing PCL nanofibers embedding pH sensors (deposition time = 30 s). FITC (green channel), RBITC (red channel), and overlay with bright-field (BF, gray channel) are shown. Scale bar: 5 μm; (middle): representative CLSM image showing cells co-cultured on pH-sensing fibers and analyzed by CLSM time-lapse imaging (x, y, z, t; t = 6 h) (nuclei are shown in blue, and cell membranes are shown in magenta for tumor cells). (Right): Results of the segmentation show the detection of the single pH sensors (red circles), AsPC-1 cells (green circles), and CAF cells (yellow circles), corresponding to the reconstruction of the cell fluxes through physically constrained statistical inference, with a relative colormap. Scale bar: 20 μm. Reprinted with permission from Onesto et al., ACS Nano, 2023, 17, 3313–3323; figure licensed under CC-BY 4.0 https://creativecommons.org/licenses/by/4.0. (b) (Left): Schematic illustration of the microencapsulation system for the generation of 3D spherical hydrogel embedding pH sensors, tumor and stromal cells; (middle): maximum intensity projection of 3D time-lapse CLSM acquisitions of alginate hydrogel, including FITC/RBITC pH sensors (yellow), tumor cells (magenta) and stromal cells (blue). Bright-field (BF, grey). Scale bar: 50 μm. (Right): 3D scatter plots of the pH sensors around a selected tumor and cancer cells at times 0 and 10 h, with relative pH colour-maps. Reprinted with permission from Rizzo et al., Biosensors and Bioelectronics, 2022, 212, 114401; figure licensed under CC-BY 4.0 https://creativecommons.org/licenses/by/4.0. Copyright © 2022, The Authors. Published by Elsevier B.V. (c) (Left): The cell mixture is dispensed into the targeted 3D static culture, and 3D dynamic culture within a chip supporting microperfusion (perfusion pathway illustrated by arrows); (middle): CLSM PLIM imaging of the embedded oxygen sensor beads and subsequent conversion to the corresponding local oxygen concentration is shown as colour-coded concentrations overlaid with green fluorescence from calcein AM staining of metabolically active cells in lateral (XY) and radial (RZ) projections. HepG2 cells at 20 × 106 cells per mL embedded in a hydrogel of 7.5% w/v GelMA in a medium; (right): oxygenation in a 3D tissue model with an array of 8 perfused microfluidic channels (inner dimensions of 140 × 140 μm2) and projected bottom view of sensor beads at all elevations overlaid on a projected confocal fluorescence micrograph of live-stained cells. Reproduced from Wesseler et al., Lab Chip, 2022, 22, 4167 with permission from the Royal Society of Chemistry. | |
4. Challenges and future perspectives of ratiometric FL sensors in oncology
The feasibility and versatility of accurate and sensitive ratiometric FL sensing systems are explored in this review with a particular focus on their application for in vitro and in vivo spatiotemporal mapping of TME parameters, highlighting their potential for applications in cancer diagnostics and therapeutics. Among the many milestones achieved in this era, the possibility of adopting FL ratiometric sensors in clinical practice has become a reality and is now of great impact in surgery.243 In fact, intraoperative FL-guided surgery can today discriminate a healthy tissue from a cancerous one, resulting in more accurate resection of the diseased area.244–247 The main advantage coming from such fine surgical resection is the complete eradication of the tumor. Therefore, this procedure is strictly linked to the reduction of tumor metastasis circulation from a primary lesion to other organs. In addition to this, several other reports in the literature use ratiometric FL probes for mapping tumor's margins in biopsy tissues248 or during surgery.249 Frequently, surgery is accomplished by pharmacological therapies; therefore, another benefit that could be added to the clinical routine is the use of ratiometric FL sensing systems that can locally monitor drug release.250 An example is represented by a recently developed ratiometric drug delivery system comprising a target-specific antibody for selective delivery to cancerous cells linked to a “drug-switchable dye” conjugate and a reference dye for the ratiometric fluorescence monitoring of drug release.251–254 Using this system, the authors showed intensity-based monitoring of drug distribution and accumulation in vitro and in vivo as well as ratiometric measurements of drug release in vitro. Possible future developments could also include the fabrication and application of barcode ratiometric sensors for in vitro/in vivo multiplex spatiotemporal analysis of key metabolic TME parameters (e.g., pH and oxygen) or metal ion concentrations (e.g., calcium). Multiplexed non-invasive ratiometric sensing allows for the continuous and online recording of multiple cellular input signals influencing the physiological and pathological states of the body, thus improving our understanding of cell–cell interactions and cell response to therapies.
5. Conclusions
Fluorescence-based sensing and imaging have emerged as cutting-edge technologies for ratiometric fluorescence devices, providing accurate in vitro and in vivo detection and monitoring of TME parameters and related cancer biomarkers. The ability to capture cell–cell interactions and single-cell behaviour at different time points, qualitatively and quantitatively, allows for a deeper understanding of the complex world of cancer. This approach provides researchers and clinicians with precise and informative tools to fight against this widespread disease.
The design of smart ratiometric FL probes in the form of nano- and microparticles may seem straightforward at first glance. However, the working principles and effectiveness of a developed sensor platform depend on overcoming various obstacles that must be considered when imaging is the ultimate goal. Some of them are photostability, leaching, and auto-fluorescence generated by macromolecules in living organisms, as well as perturbations caused by operator, instrumental and environmental conditions, which can inevitably occur during the whole experiments. The strength of the ratiometric FL method depends on its self-calibration, achieved by employing an indicator signal and a reference signal, or two reversible signals, knocking down errors and enhancing accuracy and precision. In addition, the ongoing research and development of novel sensing probes spanning various options, including organic dyes, quantum dots, nanoclusters, and metal–organic frameworks, coupled with diverse synthetic methodologies, such as encapsulation and layer-by-layer (LbL) deposition of sensing units, the ability to customize particles with biocompatible molecules, and the ability to tune their size, shape, charge, and matrix support. These advancements have made ratiometric FL tools versatile platforms for sensing TME both in vitro and in vivo.
Today, the main players of the TME, including both cellular and non-cellular components, have been widely studied; even though their leading functions in cancerogenesis and progression have been interpreted, the entire tumor pool still remains a constant-evolving topic. Phenomena such as acidosis, hypoxia, ROS generation, and ion fluxes variations are known to be strictly interconnected with each other, and all of them represent targets that are selectively hit by therapeutic protocols. Moreover, in the literature, it is possible to find many ratiometric FL tools for the detection of the intracellular and extracellular pH of cancer cells. More forces need to be engaged to develop ratiometric FL sensors for monitoring and real-time measurements of tumor hypoxia. However, intrinsically fluorescent nanomaterials, such as semiconductor quantum dots and metal nanoclusters, have emerged as promising candidates for the design of highly sensitive and photostable ratiometric FL systems. These nanomaterials have been successfully employed to monitor physio-pathological changes in pH, dissolved oxygen, ROS, and ion levels in tumor cells, and they have greatly assisted in the development of analytical platforms that are being used as point-of-care devices for the screening and targeting of diagnostic, prognostic, and predictive biomarkers. To overcome the challenge of mimicking the complex TME and capturing its metabolic dynamics in a spatiotemporal manner, ratiometric FL sensors have been successfully integrated into various biocompatible and easily handled polymer matrices to create 2D or 3D sensing platforms. This innovative approach offers the potential for high-resolution and reliable real-time measurements of cell metabolism during drug treatment, facilitating a more comprehensive understanding of TME and cell metabolic dynamics.
The future prospective is called “precision medicine” owing to the irruption of the nanotechnologies, which in turn are advancing daily toward more sophisticated and ultrasensitive platforms for boosting and gaining the finest and trustworthy quantification of the TME in its complexity. Owing to its related molecular biomarkers, cancer treatments will soon progress towards targeted therapies, the new revolutionary frontier.
Conflicts of interest
There are no conflicts to declare.
Acknowledgements
The authors acknowledge the funding from the European Research Council (ERC) under the European Union's Horizon 2020 research and innovation program ERC Starting Grant “INTERCELLMED” (contract number 759959), the European Union's Horizon 2020 research and innovation programme under grant agreement No. 953121 (FLAMIN-GO), the Associazione Italiana per la Ricerca contro il Cancro (AIRC) (MFAG-2019, contract number 22902), the “Tecnopolo per la medicina di precisione” (TecnoMed Puglia) – Regione Puglia: DGR n.2117 of 21/11/2018, CUP: B84I18000540002. The Italian Ministry of Research (MUR) under the complementary actions to the NRRP (PNC0000007) “Fit4MedRob-Fit for Medical Robotics” Grant (contract number CUP B53C22006960001) and the MUR NRRP “National Center for Gene Therapy and Drugs based on RNA Technology” (Project no. CN00000041 CN3 RNA). AC also acknowledges the Jain University, Bangalore (India) for its support through the provision of seed fund assistance.
References
- P. Vineis and C. P. Wild, Lancet, 2014, 383, 549–557 CrossRef PubMed.
-
American Cancer Society, Cancer Facts & Figures, 2022 Search PubMed.
- R. L. Siegel, K. D. Miller, N. S. Wagle, A. Jemal and C. A. Cancer, J. Clin., 2023, 73, 17–48 Search PubMed.
- K. R. Yabroff, X.-C. Wu, S. Negoita, J. Stevens, L. Coyle, J. Zhao, B. J. Mumphrey, A. Jemal and K. C. Ward, JNCI, J. Natl. Cancer Inst., 2022, 114, 907–909 CrossRef PubMed.
- G. Schmidt, D. Dinter, M. F. Reiser and S. O. Schoenberg, Dtsch. Ärztebl. Int., 2010, 107, 383–389 Search PubMed.
- J. J. Vaquero and P. Kinahan, Annu. Rev. Biomed. Eng., 2015, 17, 385–414 CrossRef CAS PubMed.
- C. J. Garvey and R. Hanlon, Br. Med. J., 2002, 324, 1077–1080 CrossRef PubMed.
- K. Christensen-Jeffries, O. Couture, P. A. Dayton, Y. C. Eldar, K. Hynynen, F. Kiessling, M. O'Reilly, G. F. Pinton, G. Schmitz, M. X. Tang, M. Tanter and R. J. G. van Sloun, Ultrasound Med. Biol., 2020, 46, 865–891 CrossRef PubMed.
- T.-J. Yoon, World J. Gastrointest. Endosc., 2013, 5, 534 CrossRef PubMed.
- J. A. Joyce, Cancer Cell, 2005, 7, 513–520 CrossRef CAS PubMed.
- P. A. Kenny, G. Y. Lee and M. J. Bissell, Front. Biosci., 2007, 12, 3468–3474 CrossRef CAS PubMed.
- N. E. Sounni and A. Noel, Clin. Chem., 2013, 59, 85–93 CrossRef CAS PubMed.
- C. Roma-Rodrigues, R. Mendes, P. Baptista and A. Fernandes, Int. J. Mol. Sci., 2019, 20, 840 CrossRef CAS PubMed.
- Y. Xiao and D. Yu, Pharmacol. Ther., 2021, 221, 107753 CrossRef CAS PubMed.
- L. Bejarano, M. J. C. Jordāo and J. A. Joyce, Cancer Discovery, 2021, 11, 933–959 CrossRef CAS PubMed.
- C. Belli, G. Antonarelli, M. Repetto, M. Boscolo Bielo, E. Crimini and G. Curigliano, Cancers, 2022, 14, 4278 CrossRef CAS PubMed.
- E. Henke, R. Nandigama and S. Ergün, Front. Mol. Biosci., 2020, 6, 1–24 Search PubMed.
- O. Tredan, C. M. Galmarini, K. Patel and I. F. Tannock, JNCI, J. Natl. Cancer Inst., 2007, 99, 1441–1454 CrossRef CAS PubMed.
- Y. Xu, X. Hu, S. Kundu, A. Nag, N. Afsarimanesh, S. Sapra, S. C. Mukhopadhya and T. Han, Sensors, 2019, 19, 2908 CrossRef CAS PubMed.
- R. Jenjob, T. Phakkeeree and D. Crespy, Biomater. Sci., 2020, 8, 2756–2770 RSC.
- W. A. Flavahan, E. Gaskell and B. E. Bernstein, Science, 2017, 357, 1–20 CrossRef PubMed.
-
K. Pruitt, in Progress in Molecular Biology and Translational Science, Elsevier Inc., 1st edn, 2016, vol. 144, pp. 3–47 Search PubMed.
- I. P. Pogribny, Exp. Oncol., 2010, 32, 132–136 CAS.
- S. T. Boyle, M. Zahied Johan and M. S. Samuel, J. Cell Sci., 2021, 133, 1–9 Search PubMed.
- I. Elia and M. C. Haigis, Nat. Metab., 2021, 3, 21–32 CrossRef PubMed.
- J. Winkler, A. Abisoye-Ogunniyan, K. J. Metcalf and Z. Werb, Nat. Commun., 2020, 11, 1–19 CrossRef PubMed.
- M. Wang, J. Zhao, L. Zhang, F. Wei, Y. Lian, Y. Wu, Z. Gong, S. Zhang, J. Zhou, K. Cao, X. Li, W. Xiong, G. Li, Z. Zeng and C. Guo, J. Cancer, 2017, 8, 761–773 CrossRef CAS PubMed.
- H. F. Dvorak, V. M. Weaver, T. D. Tlsty and G. Bergers, J. Surg. Oncol., 2011, 103, 468–474 CrossRef CAS PubMed.
- H. A. Goubran, R. R. Kotb, J. Stakiw, M. E. Emara and T. Burnouf, Cancer Growth Metastasis, 2014, 7, CGM.S11285 CrossRef PubMed.
- S. Balani, L. V. Nguyen and C. J. Eaves, Nat. Commun., 2017, 8, 1–10 CrossRef PubMed.
- D. P. Tabassum and K. Polyak, Nat. Rev. Cancer, 2015, 15, 473–483 CrossRef CAS PubMed.
- T. D. Tlsty and P. W. Hein, Curr. Opin. Genet. Dev., 2001, 11, 54–59 CrossRef CAS PubMed.
- O. De Wever and M. Mareel, J. Pathol., 2003, 200, 429–447 CrossRef CAS PubMed.
- X. Mao, J. Xu, W. Wang, C. Liang, J. Hua, J. Liu, B. Zhang, Q. Meng, X. Yu and S. Shi, Mol. Cancer, 2021, 20, 1–30 Search PubMed.
- Y. An, F. Liu, Y. Chen and Q. Yang, J. Cell. Mol. Med., 2020, 24, 13–24 CrossRef PubMed.
- B. Sun, Cell Rep. Phys. Sci., 2021, 2, 100515 CrossRef CAS PubMed.
- S. Xu, H. Xu, W. Wang, S. Li, H. Li, T. Li, W. Zhang, X. Yu and L. Liu, J. Transl. Med., 2019, 17, 1–22 CAS.
- S. Spada, A. Tocci, F. Di Modugno and P. Nisticò, J. Exp. Clin. Cancer Res., 2021, 40, 1–14 CrossRef PubMed.
- M. Liu, C. Tolg and E. Turley, Front. Immunol., 2019, 10, 1–9 CrossRef PubMed.
- X. Zhang, D. Nie and S. Chakrabarty, Front. Biosci., 2010, 15, 151–165 CrossRef CAS PubMed.
- V. Poltavets, M. Kochetkova, S. M. Pitson and M. S. Samuel, Front. Oncol., 2018, 8, 1–19 CrossRef PubMed.
- G. Maguire, Neural Regener. Res., 2018, 13, 1185–1186 CrossRef PubMed.
- F. D. S. E. Melo, L. Vermeulen, E. Fessler and J. P. Medema, EMBO Rep., 2013, 14, 686–695 CrossRef CAS PubMed.
- R. Baghban, L. Roshangar, R. Jahanban-Esfahlan, K. Seidi, A. Ebrahimi-Kalan, M. Jaymand, S. Kolahian, T. Javaheri and P. Zare, Cell Commun. Signaling, 2020, 18, 1–19 CrossRef PubMed.
- R. G. Jones and C. B. Thompson, Genes Dev., 2009, 23, 537–548 CrossRef CAS PubMed.
- K. Fernald and M. Kurokawa, Trends Cell Biol., 2013, 23, 620–633 CrossRef PubMed.
- F. Spill, D. S. Reynolds, R. D. Kamm and M. H. Zaman, Curr. Opin. Biotechnol., 2016, 40, 41–48 CrossRef CAS PubMed.
- M. J. Oudin and V. M. Weaver, Cold Spring Harbor Symp. Quant. Biol., 2016, 81, 189–205 CrossRef PubMed.
- T. R. Cox, Nat. Rev. Cancer, 2021, 21, 217–238 CrossRef CAS PubMed.
- D. Ribatti and F. Pezzella, Cells, 2021, 10, 1–13 CrossRef PubMed.
- A. G. Clark and D. M. Vignjevic, Curr. Opin. Cell Biol., 2015, 36, 13–22 CrossRef CAS PubMed.
- M. Castaneda, P. den Hollander, N. A. Kuburich, J. M. Rosen and S. A. Mani, Semin. Cancer Biol., 2022, 87, 17–31 CrossRef CAS PubMed.
- H. S. Chae and S. T. Hong, Int. J. Mol. Sci., 2023, 24, 12–41 CrossRef CAS PubMed.
- S. Aki, R. Nakahara, K. Maeda and T. Osawa, BBA, Biochim. Biophys. Acta, Gen. Subj., 2023, 1867, 130330 CrossRef CAS PubMed.
- R. J. DeBerardinis and N. S. Chandel, Nat. Metab., 2020, 2, 127–129 CrossRef PubMed.
- O. Warburg, Science, 1956, 123, 309–314 CrossRef CAS PubMed.
- S. Y. Lunt and M. G. Vander Heiden, Annu. Rev. Cell Dev. Biol., 2011, 27, 441–464 CrossRef CAS PubMed.
- E. Pérez-Herrero and A. Fernández-Medarde, Acta Pharm. Sin. B, 2021, 11, 2243–2264 CrossRef PubMed.
- E. Boedtkjer and S. F. Pedersen, Annu. Rev. Physiol., 2020, 82, 103–126 CrossRef CAS PubMed.
- M. R. Greco, L. Moro, S. Forciniti, K. Alfarouk, S. Cannone, R. A. Cardone and S. J. Reshkin, Int. J. Mol. Sci., 2021, 22, 1–13 Search PubMed.
- D. E. Korenchan and R. R. Flavell, Cancers, 2019, 11, 1–17 CrossRef PubMed.
- B. A. Webb, M. Chimenti, M. P. Jacobson and D. L. Barber, Nat. Rev. Cancer, 2011, 11, 671–677 CrossRef CAS PubMed.
- P. Swietach, Cancer Metastasis Rev., 2019, 38, 5–15 CrossRef CAS PubMed.
- V. Huber, A. De Milito, S. Harguindey, S. J. Reshkin, M. L. Wahl, C. Rauch, A. Chiesi, J. Pouysségur, R. A. Gatenby, L. Rivoltini and S. Fais, J. Transl. Med., 2010, 8, 57–60 CrossRef PubMed.
- K. A. White, B. K. Grillo-Hill and D. L. Barber, J. Cell Sci., 2017, 130, 663–669 CrossRef CAS PubMed.
- F. Parades, H. C. Williams and A. San Martin, Cancer Lett., 2021, 502, 133–142 CrossRef PubMed.
- A. J. Majmundar, W. J. Wong and M. C. Simon, Mol. Cell, 2010, 40, 294–309 CrossRef CAS PubMed.
- B. Muz, P. de la Puente, F. Azab and A. K. Azab, Hypoxia, 2015, 3, 83–92 CrossRef PubMed.
- R. Missiaen, N. P. Lesner and M. C. Simon, EMBO J., 2023, e112067 CrossRef CAS PubMed.
- M. Assi, Am. J. Physiol.: Regul., Integr. Comp. Physiol., 2017, 313, R646–R653 CrossRef PubMed.
- V. Aggarwal, H. S. Tuli, A. Varol, F. Thakral, M. B. Yerer, K. Sak, M. Varol, A. Jain, M. A. Khan and G. Sethi, Biomolecules, 2019, 9, 79–86 CrossRef PubMed.
- A. Kirtonia, G. Sethi and M. Garg, Cell. Mol. Life Sci., 2020, 77, 4459–4483 CrossRef CAS PubMed.
- F. Colella, G. Scillitani and C. L. Pierri, Toxicology, 2021, 447, 152612 CrossRef CAS PubMed.
- B. Zhang, C. Pan, C. Feng, C. Yan, Y. Yu, Z. Chen, C. Guo and X. Wang, Redox Rep., 2022, 27, 45–52 CrossRef CAS PubMed.
- B. Chance, H. Sies and A. Boveris, Physiol. Rev., 1979, 59, 527–605 CrossRef CAS PubMed.
- H. Sies, Redox Biol., 2017, 11, 613–619 CrossRef CAS PubMed.
- H. Sies and D. P. Jones, Nat. Rev. Mol. Cell Biol., 2020, 21, 363–383 CrossRef CAS PubMed.
- C. Gorrini, I. S. Harris and T. W. Mak, Nat. Rev. Drug Discovery, 2013, 12, 931–947 CrossRef CAS PubMed.
- J. Zheng, Oncol. Lett., 2012, 4, 1151–1157 CrossRef CAS PubMed.
- J. Krämer, R. Kang, L. M. Grimm, L. De Cola, P. Picchetti and F. Biedermann, Chem. Rev., 2022, 122, 3459–3636 CrossRef PubMed.
- N. Prevarskaya, R. Skryma and Y. Shuba, Trends Mol. Med., 2010, 16, 107–121 CrossRef CAS PubMed.
- A. Becchetti, L. Munaron and A. Arcangeli, Front. Physiol., 2013, 4, 1–3 Search PubMed.
- E. Bates, Annu. Rev. Cell Dev. Biol., 2015, 31, 231–247 CrossRef CAS PubMed.
- M. Futai, T. Oka, G. H. Sun-Wada, Y. Moriyama, H. Kanazawa and Y. Wada, J. Exp. Biol., 2000, 203, 107–116 CrossRef CAS PubMed.
- M. Levin, BioEssays, 2012, 34, 205–217 CrossRef CAS PubMed.
- D. S. Adams and M. Levin, Cell Tissue Res., 2013, 352, 1–50 CrossRef PubMed.
- C. A. Jones and L. A. Hazlehurst, Biomedicines, 2021, 9, 1200 CrossRef CAS PubMed.
- T. A. Stewart, K. T. D. S. Yapa and G. R. Monteith, Biochim. Biophys. Acta, Biomembr., 2015, 1848, 2502–2511 CrossRef CAS PubMed.
- B. Chovancova, V. Liskova, P. Babula and O. Krizanova, Biomolecules, 2020, 10, 1–10 CrossRef PubMed.
- T. K. Leslie, A. D. James, F. Zaccagna, J. T. Grist, S. Deen, A. Kennerley, F. Riemer, J. D. Kaggie, F. A. Gallagher, F. J. Gilbert and W. J. Brackenbury, Biochim. Biophys. Acta, Rev. Cancer, 2019, 1872, 1–38 CrossRef PubMed.
- X. Huang and L. Y. Jan, J. Cell Biol., 2014, 206, 151–162 CrossRef CAS PubMed.
- T. Mijatovic, F. Dufrasne and R. Kiss, Pharm. Pat. Anal., 2012, 1, 91–106 CrossRef CAS PubMed.
- H. J. Kim, P. C.-W. Lee and J. H. Hong, Cancers, 2022, 14, 856 CrossRef CAS PubMed.
- J. Shi, P. W. Kantoff, R. Wooster and O. C. Farokhzad, Nat. Rev. Cancer, 2017, 17, 20–37 CrossRef CAS PubMed.
- E. S. Kawasaki and A. Player, Nanomedicine, 2005, 1, 101–109 CrossRef CAS PubMed.
- M. J. Sanderson, I. Smith, I. Parker and M. D. Bootman, Cold Spring Harb. Protoc., 2014, 10, 1–36 Search PubMed.
- R. Datta, T. M. Heaster, J. T. Sharick, A. A. Gillette and M. C. Skala, J. Biomed. Opt., 2020, 25, 1 Search PubMed.
-
P. S. Chelushkin and S. P. Tunik, in Springer Series in Chemical Physics, Springer International Publishing, 2019, vol. 119, pp. 109–128 Search PubMed.
- P. Liu, R. Zhao, H. Li, T. Zhu, Y. Li, H. Wang and X.-D. ZHang, Nano Res., 2023, 16, 692–714 CrossRef CAS.
- M. Anderson, A. Moshnikova, D. M. Engelman, Y. K. Reshetnyak and O. A. Andreev, Proc. Natl. Acad. Sci. U. S. A., 2016, 113, 8177–8181 CrossRef CAS PubMed.
- X. Zheng, H. Tang, C. Xie, J. Zhang, W. Wu and X. Jiang, Angew. Chem., Int. Ed., 2015, 54, 8094–8099 CrossRef CAS PubMed.
- X. Zheng, X. Wang, H. Mao, W. Wu, B. Liu and X. Jiang, Nat. Commun., 2015, 6, 5834 CrossRef CAS PubMed.
- X. Zheng, H. Mao, D. Huo, W. Wu, B. Liu and X. Jiang, Nat. Biomed. Eng., 2017, 1, 1–9 CrossRef.
- S. C. A. Yeh, J. Hou, J. W. Wu, S. Yu, Y. Zhang, K. D. Belfield, F. D. Camargo and C. P. Lin, Nat. Commun., 2022, 13, 1–13 Search PubMed.
- G. Rong, E. E. Tuttle, A. N. Reilly and H. A. Clark, Annu. Rev. Anal. Chem., 2019, 12, 109–128 CrossRef CAS PubMed.
- G. Rong, S. R. Corrie and H. A. Clark, ACS Sens., 2017, 2, 327–338 CrossRef CAS PubMed.
- S. J. Kim, S. J. Choi, J. S. Jang, H. J. Cho and I. D. Kim, Acc. Chem. Res., 2017, 50, 1587–1596 CrossRef CAS PubMed.
-
A. Turner, I. Karube and G. S. Wilson, Biosensors: Fundamentals and Applications, Oxford University Press, New York, Oxford, 1st edn, 1987 Search PubMed.
- S. E. Eklund, D. Taylor, E. Kozlov, A. Prokop and D. E. Cliffel, Anal. Chem., 2004, 76, 519–527 CrossRef CAS PubMed.
- M. A. Boyd, A. M. Davis, N. R. Chambers, P. Tran, A. Prindle and N. P. Kamat, Cell. Mol. Bioeng., 2021, 14, 459–469 CrossRef CAS PubMed.
- X. Huang, X. Teng, D. Chen, F. Tang and J. He, Biomaterials, 2010, 31, 438–448 CrossRef CAS PubMed.
- T. S. Atabaev, G. Urmanova and N. H. Hong, Nano LIFE, 2014, 04, 1441003 CrossRef CAS.
- P. Anger, P. Bharadwaj and L. Novotny, Phys. Rev. Lett., 2006, 96, 3–6 CrossRef PubMed.
- X. Pei, Y. Pan, L. Zhang and Y. Lv, Appl. Spectrosc. Rev., 2020, 1–22 Search PubMed.
- X. Huang, J. Song, B. C. Yung, X. Huang, Y. Xiong and X. Chen, Chem. Soc. Rev., 2018, 47, 2873–2920 RSC.
- H. J. Park, D. J. Shin and J. Yu, J. Chem. Educ., 2021, 98, 703–709 CrossRef CAS.
- L. Wu, C. Huang, B. P. Emery, A. C. Sedgwick, S. D. Bull, X. P. He, H. Tian, J. Yoon, J. L. Sessler and T. D. James, Chem. Soc. Rev., 2020, 49, 5110–5139 RSC.
- S. Kargozar, S. J. Hoseini, P. B. Milan, S. Hooshmand, H. Kim and M. Mozafari, Biotechnol. J., 2020, 15, 2000117 CrossRef CAS PubMed.
- W. A. A. Mohamed, H. Abd El-Gawad, S. Mekkey, H. Galal, H. Handal, H. Mousa and A. Labib, Nanotechnol. Rev., 2021, 10, 1926–1940 CrossRef CAS.
- U. Resch-Genger, M. Grabolle, S. Cavaliere-Jaricot, R. Nitschke and T. Nann, Nat. Methods, 2008, 5, 763–775 CrossRef CAS PubMed.
- A. Valizadeh, H. Mikaeili, M. Samiei, S. M. Farkhani, N. Zarghami, M. Kouhi, A. Akbarzadeh and S. Davaran, Nanoscale Res. Lett., 2012, 7, 19–21 CrossRef PubMed.
- S. Pandey and D. Bodas, Adv. Colloid Interface Sci., 2020, 278, 102137 CrossRef CAS PubMed.
- H. D. Duong and J. Il Rhee, Sensors, 2019, 19, 4977 CrossRef CAS PubMed.
- M. Hu, J. Tian, H. T. Lu, L. X. Weng and L. H. Wang, Talanta, 2010, 82, 997–1002 CrossRef CAS PubMed.
- F. Wen, Y. Dong, L. Feng, S. Wang, S. Zhang and X. Zhang, Anal. Chem., 2011, 83, 1193–1196 CrossRef CAS PubMed.
- R. Shen, P. Liu, Y. Zhang, Z. Yu, X. Chen, L. Zhou, B. Nie, A. Zaczek, J. Chen and J. Liu, Anal. Chem., 2018, 90, 4478–4484 CrossRef CAS PubMed.
- J. Hou, W. X. Ren, K. Li, J. Seo, A. Sharma, X.-Q. Yu and J. S. Kim, Chem. Soc. Rev., 2017, 46, 2076–2090 RSC.
- D. E. Johnson, P. Ostrowski, V. Jaumouillé and S. Grinstein, J. Cell Biol., 2016, 212, 677–692 CrossRef CAS PubMed.
- A. Kurkdjian and J. Guern, Annu. Rev. Plant Physiol. Plant Mol. Biol., 1989, 40, 271–303 CrossRef CAS.
- M. Flinck, S. H. Kramer and S. F. Pedersen, Acta Physiol., 2018, 223, 1–17 Search PubMed.
- J. Han and K. Burgess, Chem. Rev., 2010, 110, 2709–2728 CrossRef CAS PubMed.
- A. Steinegger, O. S. Wolfbeis and S. M. Borisov, Chem. Rev., 2020, 120, 12357–12489 CrossRef CAS PubMed.
- L. L. del Mercato, P. Rivera-gil, A. Z. Abbasi, M. Ochs, C. Ganas, I. Zins, C. Sonnichsen and W. J. Parak, Nanoscale, 2010, 2, 458–467 RSC.
- L. L. del Mercato, M. M. Ferraro, F. Baldassarre, S. Mancarella, V. Greco, R. Rinaldi and S. Leporatti, Adv. Colloid Interface Sci., 2014, 207, 139–154 CrossRef CAS PubMed.
- J. Li, B. V. Parakhonskiy and A. G. Skirtach, Chem. Commun., 2023, 59, 807–835 RSC.
- L. L. del Mercato, A. Z. Abbasi and W. J. Parak, Small, 2011, 3, 351–363 CrossRef PubMed.
- P. R. Gil, M. Nazarenus, S. Ashraf and W. J. Parak, Small, 2012, 8, 943–948 CrossRef PubMed.
- M. De Luca, M. M. Ferraro, R. Hartmann, P. Rivera-Gil, A. Klingl, M. Nazarenus, A. Ramirez, W. J. Parak, C. Bucci, R. Rinaldi and L. L. Del Mercato, ACS Appl. Mater. Interfaces, 2015, 7, 15052–15060 CrossRef CAS PubMed.
- I. L. Moldero, A. Chandra, M. Cavo, C. Mota, D. Kapsokalyvas, G. Gigli, L. Moroni and L. L. del Mercato, Small, 2020, 16, 2002258 CrossRef CAS PubMed.
- A. Chandra, S. Prasad, F. Alemanno, M. De Luca, R. Rizzo, R. Romano, G. Gigli, C. Bucci, A. Barra and L. L. del Mercato, ACS Appl. Mater. Interfaces, 2022, 14, 18133–18149 CrossRef CAS PubMed.
- P. Srivastava, I. Tavernaro, L. Scholtz, C. Genger, P. Welker, F. Schreiber, K. Meyer and U. R. Genger, Sci. Rep., 2023, 13, 1–16 CrossRef PubMed.
- G. Fan, L. Yang and Z. Chen, Front. Chem. Sci. Eng., 2014, 8, 405–417 CrossRef CAS.
- M. Strobl, T. Rappitsch, S. M. Borisov, T. Mayr and I. Klimant, Analyst, 2015, 140, 7150–7153 RSC.
- Y. Ni and J. Wu, Org. Biomol. Chem., 2014, 12, 3774–3791 RSC.
- Q. Chen, J. Zhai, J. Li, Y. Wang and X. Xie, Nano Res., 2022, 15, 3471–3478 CrossRef CAS.
- A. Sebestyén, L. Kopper, T. Dankó and J. Tímár, Pathol. Oncol. Res., 2021, 27, 1–15 Search PubMed.
- I. Godet, S. Doctorman, F. Wu and D. M. Gilkes, Cells, 2022, 11, 686 CrossRef CAS PubMed.
- Y. Wei, Y. Jiao, D. An, D. Li, W. Li and Q. Wei, Sensors, 2019, 19, 3995–4033 CrossRef CAS PubMed.
- M. H. Gehlen, J. Photochem. Photobiol., C, 2020, 42, 100338 CrossRef CAS.
- Y. Amao, Microchim. Acta, 2003, 143, 1–12 CrossRef CAS.
- I. Dalfen and S. M. Borisov, Anal. Bioanal. Chem., 2022, 414, 4311–4330 CrossRef CAS PubMed.
- M. Quaranta, S. M. Borisov and I. Klimant, Bioanal. Rev., 2012, 4, 115–157 CrossRef PubMed.
- M. Mital and Z. Ziora, Coord. Chem. Rev., 2018, 375, 434–458 CrossRef CAS.
- H. Xu, J. W. Aylott, R. Kopelman, T. J. Miller and M. A. Philbert, Anal. Chem., 2001, 73, 4124–4133 CrossRef CAS PubMed.
- Y. Amao and I. Okura, J. Porphyrins Phthalocyanines, 2009, 13, 1111–1122 CrossRef CAS.
- C. Wu, B. Bull, K. Christensen and J. McNeill, Angew. Chem., Int. Ed., 2009, 48, 2741–2745 CrossRef CAS PubMed.
- X.-H. Wang, H. Peng and Z. Chang, Microchim. Acta, 2012, 178, 147–152 CrossRef CAS.
- R. Xu, Y. Wang, X. Duan, K. Lu, D. Micheroni, A. Hu and W. Lin, J. Am. Chem. Soc., 2016, 138, 2158–2161 CrossRef CAS PubMed.
- J. Meng, X. Liu, C. Niu, Q. Pang, J. Li, F. Liu, Z. Liu and L. Mai, Chem. Soc. Rev., 2020, 49, 3142–3186 RSC.
- Z. Luo, S. Fan, C. Gu, W. Liu, J. Chen, B. Li and J. Liu, Curr. Med. Chem., 2018, 26, 3341–3369 CrossRef PubMed.
- G. A. Udourioh, M. M. Solomon, C. O. Matthews-Amune, E. I. Epelle, J. A. Okolie, V. E. Agbazue and U. Onyenze, React. Chem. Eng., 2023, 8, 278–310 RSC.
- Y. Wen, S. Zhang, W. Yuan, W. Feng and F. Li, Anal. Chem., 2023, 95, 2478–2486 CrossRef CAS PubMed.
- S. Duanghathaipornsuk, E. J. Farrell, A. C. Alba-Rubio, P. Zelenay and D. S. Kim, Biosensors, 2021, 11, 1–41 CrossRef PubMed.
- H. Guo, H. Aleyasin, B. C. Dickinson, R. E. Haskew-Layton and R. R. Ratan, Cell Biosci., 2014, 4, 1–10 CrossRef PubMed.
- D. Srikun, E. W. Miller, D. W. Domaille and C. J. Chang, J. Am. Chem. Soc., 2008, 130, 4596–4597 CrossRef CAS PubMed.
- Y. Kim, H. S. Kim, Y. Yang, K. Baek, N. Choi and M. H. Park, J. Toxicol. Environ. Health Sci., 2017, 9, 108–115 CrossRef.
- G. Kim, Y. E. L. Koo, H. Xu, M. A. Philbert and R. Kopelman, Anal. Chem., 2010, 82, 2165–2169 CrossRef CAS PubMed.
- L. I. Kazakova, L. I. Shabarchina, S. Anastasova, A. M. Pavlov, P. Vadgama, A. G. Skirtach and G. B. Sukhorukov, Anal. Bioanal. Chem., 2013, 405, 1559–1568 CrossRef CAS PubMed.
- K. G. de la Cruz-López, L. J. Castro-Muñoz, D. O. Reyes-Hernández, A. García-Carrancá and J. Manzo-Merino, Front. Oncol., 2019, 9, 1–21 CrossRef PubMed.
- B. Phypers and J. M. T. Pierce, Contin. Educ. Anaesth., Crit. Care Pain, 2006, 6, 128–132 CrossRef.
- S. Liu, J. Zhao, K. Zhang, L. Yang, M. Sun, H. Yu, Y. Yan, Y. Zhang, L. Wu and S. Wang, Analyst, 2016, 141, 2296–2302 RSC.
- V. G. Deepagan, E. K. Pramod Kumar, Y. D. Suh and J. H. Park, Macromol. Res., 2018, 26, 577–580 CrossRef CAS.
- Q. Pan, X. Deng, W. Gao, J. Chang, Y. Pu and B. He, Colloids Surf., B, 2020, 194, 111223 CrossRef CAS PubMed.
- K. Rajes, K. A. Walker, S. Hadam, F. Zabihi, J. Ibrahim-Bacha, G. Germer, P. Patoka, B. Wassermann, F. Rancan, E. Rühl, A. Vogt and R. Haag, ACS Biomater. Sci. Eng., 2021, 7, 2485–2495 CrossRef CAS PubMed.
- C. Feng, F. Wang, Y. Dang, Z. Xu, H. Yu and W. Zhang, Langmuir, 2017, 33, 3287–3295 CrossRef CAS PubMed.
- J. Zhou, R. Zhao, Y. Du, S. Liu, W. Li, S. Gai, F. He, L. Feng and P. Yang, Adv. Funct. Mater., 2022, 32, 2112083 CrossRef CAS.
- F. Liu, T. Bing, D. Shangguan, M. Zhao and N. Shao, Anal. Chem., 2016, 88, 10631–10638 CrossRef CAS PubMed.
- M. Sheth and L. Esfandiari, Front. Oncol., 2022, 12, 1–15 Search PubMed.
- M. Wei, P. Lin, Y. Chen, J. Y. Lee, L. Zhang, F. Li and D. Ling, Nanomedicine, 2020, 15, 2871–2881 CrossRef CAS PubMed.
- M. L. Valentine, A. E. Cardenas, R. Elber and C. R. Baiz, Biophys. J., 2018, 115, 1541–1551 CrossRef CAS PubMed.
- R. M. Paredes, J. C. Etzler, L. T. Watts, W. Zheng and J. D. Lechleiter, Methods, 2008, 46, 143–151 CrossRef CAS PubMed.
- D. Si, T. Epstein, Y. E. Koo Lee and R. Kopelman, Anal. Chem., 2012, 84, 978–986 CrossRef CAS PubMed.
- Y. Lin, Y. Zheng, Y. Guo, Y. Yang, H. Li, Y. Fang and C. Wang, Sens. Actuators, B, 2018, 273, 1654–1659 CrossRef CAS.
- A. Schulz, R. Woolley, T. Tabarin and C. McDonagh, Analyst, 2011, 136(8), 1722 RSC.
- G. Gao, Y. Cao, W. Liu, D. Li, W. Zhou and J. Liu, Anal. Methods, 2017, 9, 5570–5579 RSC.
-
S. Dietrich, S. E. Stanca, C. G. Cranfield, B. Hoffmann, K. Benndorf and C. Biskup, in Multiphoton Microscopy in the Biomedical Sciences X, ed. A. Periasamy, P. T. C. So and K. König, SPIE BiOS, San Francisco, California, United States, 2010, vol. 756914, p. 756914 Search PubMed.
- R. Wang, X. Du, Y. Wu, J. Zhai and X. Xie, ACS Sens., 2018, 3, 2408–2414 CrossRef CAS PubMed.
- X. Xie, G. A. Crespo and E. Bakker, Anal. Chem., 2013, 85, 7434–7440 CrossRef CAS PubMed.
- J. M. David and A. K. Rajasekaran, J. Kidney Cancer VHL, 2015, 2, 15–24 CrossRef PubMed.
- D. Kwon, E. Park, H. Sesaki and S. Kang, Biochem. Biophys. Res. Commun., 2017, 493, 737–743 CrossRef CAS PubMed.
- M. Zacchia and L. Abategiovanni, Kidney Dis., 2016, 2, 72–79 CrossRef PubMed.
- T. S. Rimmele and J.-Y. Chatton, PLoS One, 2014, 9, 1–9 CrossRef PubMed.
- J. Liu, L. Pan, C. Shang, B. Lu, R. Wu, Y. Feng, W. Chen, R. Zhang, J. Bu, Z. Xiong and W. Bu, Sci. Adv., 2020, 6, 1–11 Search PubMed.
- S. Wilhelm, ACS Nano, 2017, 11, 10644–10653 CrossRef CAS PubMed.
- B. Andersson and V. Janson, Toxicol. In Vitro, 2006, 20, 986–994 CrossRef CAS PubMed.
- J. Liu, F. Li, Y. Wang, L. Pan, P. Lin, B. Zhang, K. Shin, D. Kim, S. Jang, H. J. Chung, H. Tian and Q. Wang, Nat. Nanotechnol., 2020, 15, 321–330 CrossRef CAS PubMed.
- T. T. Ruckh, C. G. Skipwith, W. Chang, A. W. Senko, V. Bulovic, P. O. Anikeeva and H. A. Clark, ACS Nano, 2016, 10, 4020–4030 CrossRef CAS PubMed.
- N. Shcheynikov, A. Son, J. Hee, O. Yamazaki, E. Ohana and I. Kurtz, Proc. Natl. Acad. Sci. U. S. A., 2015, 5, E329–E337 Search PubMed.
- M. J. Ruedas-Rama, A. Orte, E. A. H. Hall, J. M. Alvarez-Pez and E. M. Talavera, Analyst, 2012, 137, 1500–1508 RSC.
- Y. Wang, H. Mao and L. B. Wong, Nanotechnology, 2010, 21, 055101 CrossRef PubMed.
- N. L. Henry and D. F. Hayes, Mol. Oncol., 2012, 6, 140–146 CrossRef CAS PubMed.
-
S. Hosseini, P. Vázquez-Villegas, M. Rito-Palomares and S. O. Martinez-Chapa, Enzyme-linked Immunosorbent Assay (ELISA), Springer Singapore, Singapore, 2018 Search PubMed.
- M. T. Rahman, M. S. Uddin, R. Sultana, A. Moue and M. Setu, Anwer Khan Mod. Med. Coll. J., 2013, 4, 30–36 CrossRef.
- U. Laraib, S. Sargazi, A. Rahdar, M. Khatami and S. Pandey, Int. J. Biol. Macromol., 2022, 195, 356–383 CrossRef CAS PubMed.
- D. A. Murphy and S. A. Courtneidge, Nat. Rev. Mol. Cell Biol., 2011, 12, 413–426 CrossRef CAS PubMed.
- R. Ferrari, G. Martin, O. Tagit, A. Guichard, A. Cambi, R. Voituriez, S. Vassilopoulos and P. Chavrier, Nat. Commun., 2019, 10, 1–15 CrossRef PubMed.
- Y. Wang, P. Shen, C. Li, Y. Wang and Z. Liu, Anal. Chem., 2012, 84, 1466–1473 CrossRef CAS PubMed.
- X. He, J. Gao, S. S. Gambhir and Z. Cheng, Trends Mol. Med., 2010, 16, 574–583 CrossRef CAS PubMed.
- M. Swierczewska, S. Lee and X. Chen, Phys. Chem. Chem. Phys., 2011, 13, 9929–9941 RSC.
- E. Hutter and D. Maysinger, Trends Pharmacol. Sci., 2013, 34, 497–507 CrossRef CAS PubMed.
- S. Lee, E. J. Cha, K. Park, S. Y. Lee, J. K. Hong, I. C. Sun, S. Y. Kim, K. Choi, I. C. Kwon, K. Kim and C. H. Ahn, Angew. Chem., Int. Ed., 2008, 47, 2804–2807 CrossRef CAS PubMed.
- S. Lee, J. H. Ryu, K. Park, A. Lee, S. Y. Lee, I. C. Youn, C. H. Ahn, S. M. Yoon, S. J. Myung, D. H. Moon, X. Chen, K. Choi, I. C. Kwon and K. Kim, Nano Lett., 2009, 9, 4412–4416 CrossRef CAS PubMed.
- L. Yin, H. Sun, M. Zhao, A. Wang, S. Qiu, Y. Gao, J. DIng, S. J. Ji, H. Shi and M. Gao, J. Org. Chem., 2019, 84, 6126–6133 CrossRef CAS PubMed.
- T. Ma, Y. Hou, J. Zeng, C. Liu, P. Zhang, L. Jing, D. Shangguan and M. Gao, J. Am. Chem. Soc., 2018, 140, 211–218 CrossRef CAS PubMed.
- C. D. Belair, T. R. Yeager, P. M. Lopez and C. A. Reznikoff, Proc. Natl. Acad. Sci. U. S. A., 1997, 94, 13677–13682 CrossRef CAS PubMed.
- J. Vinagre, V. Pinto, R. Celestino, M. Reis, H. Pópulo, P. Boaventura, M. Melo, T. Catarino, J. Lima, J. M. Lopes, V. Máximo, M. Sobrinho-Simões and P. Soares, Virchows Arch., 2014, 465, 119–133 CrossRef CAS PubMed.
- D. Ma, H. Bai, J. Li, Y. Li, L. Song, J. Zheng and C. Miao, Anal. Bioanal. Chem., 2022, 414, 1891–1898 CrossRef CAS PubMed.
- K. Clack, N. Soda, S. Kasetsirikul, R. G. Mahmudunnabi, N.-T. Nguyen and M. J. A. Shiddiky, Small, 2023, 1, 2205856 CrossRef PubMed.
- S. Singh, P. S. Podder, M. Russo, C. Henry and S. Cinti, Lab Chip, 2023, 23, 44–61 RSC.
- Z. Sun, J. Li, Y. Yang, Y. Tong, H. Li, C. Wang, L. Du and Y. Jiang, Bioconjugate Chem., 2022, 33, 1698–1706 CrossRef CAS PubMed.
- J. Gao, X. Yu, X. Wang, Y. He and J. Ding, Engineering, 2022, 13, 31–45 CrossRef CAS.
- M. Cavo, F. Serio, N. R. Kale, E. D'Amone, G. Gigli and L. L. del Mercato, Biomater. Sci., 2020, 8, 4887 RSC.
-
A. Accardo, M. Ventre, C. Chiappini, V. Onesto, M. L. Coluccio, P. Netti and F. Gentile, in Neural Regenerative Nanomedicine, Elsevier, 2020, pp. 47–88 Search PubMed.
- F. Serio, N. Silvestri, S. Kumar, G. E. P. Nucci, S. Nitti, V. Onesto, F. Catalano, E. D. Amone, G. Gigli, L. Loretta and T. Pellegrino, J. Colloid Interface Sci., 2022, 607, 34–44 CrossRef CAS PubMed.
- E. Hendrick, M. Frey, E. Herz and U. Wiesner, J. Eng. Fibers Fabr., 2010, 5, 21–30 CAS.
- B. Y. Chen, C. C. Kuo, Y. S. Huang, S. T. Lu, F. C. Liang and D. H. Jiang, ACS Appl. Mater. Interfaces, 2015, 7, 2797–2808 CrossRef CAS PubMed.
- L. L. del Mercato, M. Moffa, R. Rinaldi and D. Pisignano, Small, 2015, 11, 6417–6424 CrossRef CAS PubMed.
- V. Onesto, S. Forciniti, F. Alemanno, K. Narayanankutty, A. Chandra, S. Prasad, A. Azzariti, G. Gigli, A. Barra, A. De Martino, D. De Martino and L. L. del Mercato, ACS Nano, 2023, 17, 3313–3323 CrossRef CAS PubMed.
- A. Mahmood, D. Patel, B. Hickson, J. Desrochers and X. Hu, Int. J. Mol. Sci., 2022, 23, 1415 CrossRef CAS PubMed.
- G. Schuszter, T. Gehèr-Herczegh, A. Szucs, A. Toth and D. Horvath, Phys. Chem. Chem. Phys., 2017, 19, 12136–12143 RSC.
- R. Rizzo, V. Onesto, G. Morello, H. Iuele, F. Scalera, S. Forciniti, G. Gigli, A. Polini, F. Gervaso and L. L. del Mercato, Mater. Today Bio, 2023, 20, 100655 CrossRef CAS PubMed.
- R. Rizzo, V. Onesto, S. Forciniti, A. Chandra, S. Prasad, H. Iuele, F. Colella, G. Gigli and L. L. del Mercato, Biosens. Bioelectron., 2022, 212, 114401 CrossRef CAS PubMed.
- P. Chiarugi and P. Cirri, Cancer Lett., 2016, 380, 272–280 CrossRef CAS PubMed.
- A. Chandra and N. Singh, Chem. Commun., 2018, 54, 1643–1646 RSC.
- D. B. Longley, D. P. Harkin and P. G. Johnston, Nat. Rev. Cancer, 2003, 3, 330–338 CrossRef CAS PubMed.
- A. Delic, E. Mariussen, D. Roede and A. Krivokapic, Chempluschem, 2021, 86, 176–183 CrossRef CAS PubMed.
- F. Li, Y. Liu, Y. Xu, Y. Li, J. Liu, M. Lv, C. Ruan, H. Pan and X. Zhao, ACS Omega, 2020, 5, 19796–19804 CrossRef CAS PubMed.
- Y. Ando, H. P. Ta, D. P. Yen, S. Lee, S. Raola and K. Shen, Sci. Rep., 2017, 7, 15233 CrossRef PubMed.
- R. L. Wilson, J. P. Connell and K. J. Grande-allen, ACS Biomater. Sci. Eng., 2019, 5, 4522–4230 CrossRef CAS PubMed.
- M. F. Wesseler, M. N. Johansen, A. Kızıltay, K. I. Mortensen and N. B. Larsen, Lab Chip, 2022, 22, 4167–4179 RSC.
- A. Fedi, C. Vitale, P. Giannoni, G. Caluori and A. Marrella, Sensors, 2022, 22, 1517 CrossRef CAS PubMed.
- I. L. Moldero, A. Chandra, M. Cavo, C. Mota, D. Kapsokalyvas, G. Gigli, L. Moroni and L. L. del Mercato, Small, 2020, 16, 2002258 CrossRef CAS PubMed.
- S. Hernot, L. van Manen, P. Debie, J. S. D. Mieog and A. L. Vahrmeijer, Lancet Oncol., 2019, 20, e354–e367 CrossRef CAS PubMed.
- M. Koch and V. Ntziachristos, Annu. Rev. Med., 2016, 67, 153–164 CrossRef CAS PubMed.
- J. S. D. Mieog, F. B. Achterberg, A. Zlitni, M. Hutteman, J. Burggraaf, R. J. Swijnenburg, S. Gioux and A. L. Vahrmeijer, Nat. Rev. Clin. Oncol., 2022, 19, 9–22 CrossRef CAS PubMed.
- S. Van Keulen, M. Hom, H. White, E. L. Rosenthal and F. M. Baik, Mol. Imaging Biol., 2023, 25, 36–45 CrossRef CAS PubMed.
- K. Wang, Y. Du, Z. Zhang, K. He, Z. Cheng, L. Yin, D. Dong, C. Li, W. Li, Z. Hu, C. Zhang, H. Hui, C. Chi and J. Tian, Nat. Rev. Bioeng., 2023, 1, 161–179 CrossRef.
- X. Zhou, Y. Liu, Q. Liu, L. Yan, M. Xue, W. Yuan, M. Shi, W. Feng, C. Xu and F. Li, Theranostics, 2019, 9, 4597–4607 CrossRef CAS PubMed.
- J. T. Unkart, S. L. Chen, I. L. Wapnir, J. E. González, A. Harootunian and A. M. Wallace, Ann. Surg. Oncol., 2017, 24, 3167–3173 CrossRef PubMed.
- M. I. Khan, M. I. Hossain, M. K. Hossain, M. H. K. Rubel, K. M. Hossain, A. M. U. B. Mahfuz and M. I. Anik, ACS Appl. Bio Mater., 2022, 5, 971–1012 CrossRef CAS PubMed.
- E. Thankarajan, S. Jadhav, G. Luboshits, G. Gellerman and L. Patsenker, Anal. Chem., 2021, 93, 8265–8272 CrossRef CAS PubMed.
- T. M. Ebaston, A. Rozovsky, A. Zaporozhets, A. Bazylevich, H. Tuchinsky, V. Marks, G. Gellerman and L. D. Patsenker, ChemMedChem, 2019, 14, 1727–1734 CrossRef CAS PubMed.
- L. Patsenker and G. Gellerman, Isr. J. Chem., 2020, 60, 504–518 CrossRef CAS.
- A. Rozovsky, T. M. Ebaston, A. Zaporozhets, A. Bazylevich, H. Tuchinsky, L. Patsenker and G. Gellerman, RSC Adv., 2019, 9, 32656–32664 RSC.
|
This journal is © The Royal Society of Chemistry 2023 |