DOI:
10.1039/D3MA00472D
(Review Article)
Mater. Adv., 2023,
4, 5003-5017
Recent advances in targeted nanoparticle drug delivery systems for ischaemic stroke
Received
27th July 2023
, Accepted 29th September 2023
First published on 30th September 2023
Abstract
Ischaemic stroke (IS) is the second most common cause of death worldwide. Traditional treatment strategies, including blood flow recanalization therapy and neuroprotective drugs, have reduced safety and effectiveness due to their lack of targeting and inability to penetrate the blood–brain barrier (BBB). Targeted nanoparticles (NPs) can improve BBB penetration and brain targeting by changing their composition and structure, which can integrate a variety of treatment components to match the complex pathological process of IS. In this review, we describe recent advances in IS therapy, specifically in the prevention, detection, and treatment of IS, by using targeted NPs, presenting basic knowledge and possible therapeutic targets to highlight their potential for early treatment in the clinic.
1. Introduction
Ischaemic stroke (IS) is one of the leading causes of death and disability and poses a serious threat to human health.1–3 It creates an enormous burden on the family and society.4,5 IS is caused by emboli, which result in a sudden decrease in blood flow to the brain.6 Ischaemia triggers energy exhaustion and a series of biochemical events, including ionic imbalance and excitotoxicity, oxidative stress, cell death, the complement system, inflammation, and the initiation of immune responses. This eventually leads to brain tissue damage.7–9 Existing therapeutic strategies include antithrombotic and neuroprotective strategies.10 (1) Antithrombotic: drug thrombolysis and surgical thrombolysis are the standard treatments for acute IS.11 However, there is a narrow treatment window12 and a risk of haemorrhagic transformation (HT).13–15 Moreover, reperfusion injury occurs after blood flow recanalization.16–19 Ischaemia–reperfusion injury is a pathological condition in which tissue damage and inflammation are exacerbated after restoration of blood flow and oxygenation.20 (2) Neuroprotective drugs: Neuroprotective drugs can relieve ischaemia–reperfusion injury by fighting inflammation and stress and save the brain tissue of the penumbra around the infarct core area. Although many neuroprotective drugs have been developed, the vast majority have failed to show efficacy because of their poor ability to cross the BBB and target infarcts.8,21 Moreover, the limited regenerative ability of brain tissue and the microenvironment that inhibits regeneration after stroke require brain tissue regeneration therapy.22 Brain tissue regeneration can be effectively promoted through the input of cytokines and exogenous stem cells that stimulate endogenous regeneration.23 Recently, targeted NPs have shown advantages in the treatment of IS.24–26 Targeted NPs can be designed to change their structure and composition so that they can bind to ischaemic brain tissue and ultimately enrich the therapeutic component to achieve a therapeutic effect at a low dose.27 This approach reduces the side effects of systemic high doses. The increased targeting allows the drug to reach therapeutic concentrations in the brain without having to cross the BBB in large quantities to exert a therapeutic effect. It can also increase BBB transport and permeability through the intercellular or cell-mediated pathway,28 which increases the amount of drug in the whole brain and can also have therapeutic effects.23 In addition, targeted NPs can integrate multiple therapeutic components and participate in different pathological processes of IS.42 Although ideal targeting NPs with all the above advantages have not yet been developed, targeted NPs have potential for these advantages and therefore have promising prospects. In this review, we first analyse the shortcomings of conventional treatment strategies and conclude that one of the reasons is the lack of targeting and then focus on the application of three types of targeting NPs in the prevention, diagnosis, and treatment of IS by targeting thrombi, ischaemic brain tissue, and the BBB.
2. Conventional treatment strategies
Conventional treatment strategies for IS mainly include antithrombotic and neuroprotective strategies. Antithrombotic therapy includes antiplatelet drugs and anticoagulant drugs to prevent thrombosis30,43,44 and thrombolytic drugs to dissolve already formed thrombosis.32,45,46 Neuroprotective agents mainly inhibit glutamate-mediated excitotoxicity,40 oxidative stress,47,48 and inflammation.49,50 The types of conventional treatment strategies are summarized in Table 1. The disadvantages of conventional therapy are the short retention time of the drug in the blood, the lack of targeting, and the need for repeated systemic administration of large doses to achieve effective therapeutic concentrations at the lesion site, which increases the side effects of the drug. Therefore, targeting NPs have been developed.
Table 1 Types of conventional treatment strategies and representative therapeutic agents
Treatment |
Therapeutic agent |
Mechanism of action |
Ref. |
Antiplatelet therapy |
Aspirin |
Inhibits COX-1 involved in platelet adhesion |
29 and 30
|
Anticoagulants |
Heparin |
Deactivate thrombin and inhibit clotting factors |
31 and 32
|
|
Warfarin |
Vitamin K antagonist, reduces the production of activated clotting factors |
32–34
|
|
Argatroban |
Directly inhibits the thrombin |
35
|
Thrombolysis |
rtPA, Urokinase |
Activate the plasminogen to form plasmin |
36
|
Neuroprotectants |
Nimodipine |
Calcium channel blocker |
37 and 38
|
|
Edaravone |
Free radical scavenger |
39
|
|
Memantine |
NMDAR antagonist |
40 and 41
|
3. Strategies of targeted NPs in the treatment of IS
The progress of nanotechnology has brought new opportunities for targeted NPs to treat IS. There are three main targeting strategies: passive targeting, active targeting, and stimulus-responsive targeting. Passive targeting can increase retention in blood by modifying nanocarriers. However, due to the lack of specificity, it is difficult for the vector to reach a specific tissue and be released;51 therefore, active targeting strategies have been proposed. Active targeting has cell or tissue specificity and is achieved by binding active ligands to NPs. This active ligand could bind to a specific ligand that is present in certain cells or tissues, such as P-glycoprotein (P-gp) on microvascular endothelial cells that make up the BBB and an ATP-binding cassette (ABC) that is significantly expressed at the BBB.52 Another targeting strategy is stimulus-responsive targeting. Such NPs can release therapeutic components in response to a specific stimulus in the environment.53,54 Things like magnetism, light, sound, a certain range of pH, oxidative stress, enzymes. The clinical application of nanotechnology is mainly reflected in diagnosis and treatment.55,56 In the following section, we summarize the targeted NPs acting on thrombi, the BBB, and ischaemic brain tissue during the development of IS to achieve early detection and diagnosis of thrombus. At the same time, the effective therapeutic components of the drug cross the BBB and reach the lesion site of IS to exert a therapeutic effect. Although there are no targeted NPs that can meet the above comprehensive therapeutic effects, with the progress of science and technology, the improvement of manufacturing level, and more in-depth and continuous research on the pathological mechanism of IS, targeted NPs have broad prospects in the treatment of IS (Fig. 1). Table 2 summarizes the strategies of targeted NPs in the treatment of IS.
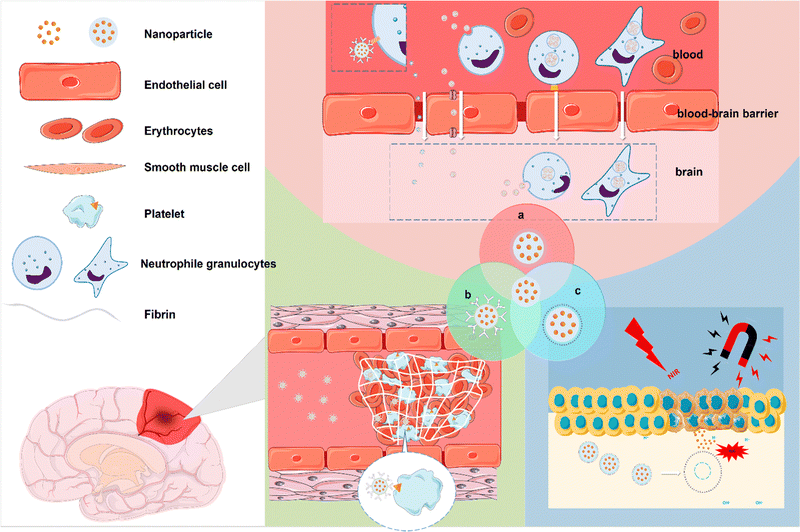 |
| Fig. 1 Schematic images of strategies of targeted NPs in the treatment of IS. (a) Targeted NPs to overcome the BBB. (b) Targeted NPs for the diagnosis and treatment of thrombosis. (c) NPs targeting ischaemic tissue. | |
Table 2 Strategies of targeted NPs in the treatment of IS
Type of targeting |
Location |
NPs |
Target |
Achievement |
Ref. |
Active targeting |
Thrombus |
(PFC) NPs |
αvβ3 integrins |
Detecting and monitoring atherosclerotic plaques |
57
|
Active targeting |
Thrombus |
(FePt) NPs |
Iba-1 |
Monitoring the dynamic development of neuroinflammation |
58
|
Stimulus-responsive targeting |
Thrombus |
pPMNP-rtPA |
|
Reduce the clot lysis time |
59
|
Stimulus-responsive targeting |
Thrombus |
tPA-MRs |
|
Increased clot interaction and penetration of tPA |
60
|
Active targeting |
Thrombus |
Fe3O4-PLGA-PFH-CREKA NPs |
Fibrin |
The early noninvasive diagnosis and treatment of arterial thrombosis |
61
|
Passive targeting |
|
CDNPs |
|
The retention time of CD is extended, ultrasound imaging |
27
|
Stimulus-responsive targeting |
Ischaemia region |
IR-LnNPs |
|
Early assessment of IS |
62
|
Stimulus-responsive targeting |
Ischaemia region |
t-PA@iRNP |
pH-sensitive |
Extended the in vivo half-life of t-PA in systemic circulation |
63
|
Stimulus-responsive targeting |
Ischaemia region |
Ca-MOF@miR-124 |
pH-sensitive |
Enhance the efficacy of NSCs |
64
|
Stimulus-responsive targeting |
Ischaemia region |
SHp-RBC-NP/NR2B9C |
ROS-sensitive |
Reduce ischaemic brain damage |
42
|
Stimulus-responsive targeting |
Ischaemia region |
B-PDEA |
ROS-sensitive |
Mediates efficient gene transfection of NSCs |
65
|
Stimulus-responsive targeting |
Ischaemia region |
DGA |
ROS-sensitive |
Suppressing the translocation of nuclear HMGB1 |
66
|
Stimulus-responsive targeting |
Ischaemia region |
CyBA/PFM NPs |
H2O2-sensitive, platelet-targeted |
Remove ROS, remove clots |
67
|
Stimulus-responsive targeting |
Ischaemia region |
ASNP |
MMP-9 |
Reducing the side effects of systemic gliburide administration |
68
|
Active targeting |
BBB |
MSNPs |
LDLR |
Enhance their transcytosis across the BBB |
28
|
Active targeting |
BBB |
cl PGP-PEG-DGL/CAT-Aco system |
neutrophils |
Enhance their transcytosis across the BBB |
23
|
4. Targeted NPs for the diagnosis and treatment of thrombosis
Thrombosis is a major cause of IS; therefore, NPs targeting thrombosis could be a therapeutic strategy to treat IS.
4.1 Magnetic NPs
Magnetic nanoparticles, especially iron oxide nanoparticles, have been widely used in biomedicine over the past two decades.69 Iron oxide nanoparticles (IONPs) are potential magnetic resonance imaging (MRI) contrast agents that can increase the contrast between diseased and normal tissues.70 Superparamagnetic properties allow them to move under an applied magnetic field, and the nanoparticles also have the ability to load drug components. Magnetic iron oxide nanoparticles (MIONPs) have emerged as a promising therapeutic nanoplatform for brain imaging and drug delivery due to their unique physical properties and nontoxic, biodegradable properties. Atherosclerosis is one of the most important causes of stroke. There is no doubt that the early detection of problem vessels and early intervention are very important. Angiogenic biomarkers, such as αvβ3, are highly induced in early diseased vessels and have become our targets for detecting early diseased vessels. Winter et al. developed a paramagnetic nanoparticle contrast agent that targets αvβ3 integrins of angiogenesis in atherosclerotic plaques.57 It can detect early diseased vessels and is noninvasive. This experiment was performed at a field strength of 1.5 Tesla, and the enhanced signal on the rabbit aortic wall could be observed, but the layer of the aorta could not be distinguished. With the higher field strength of the application of the MR scanner, higher resolution images can be obtained, which will improve the early patches of positioning and promote clinical risk assessment. In addition, magnetic NPs can be used to track cells and monitor stroke inflammation.71,72 Sillerud et al. developed novel anti-IBA-1 targeting superparamagnetic iron–platinum (FePt) NPs, which target microglia. The development of neuroinflammation after ischaemic stroke is understood by monitoring the spatiotemporal distribution of microglial/macrophage activation in vivo by magnetic resonance imaging.58 Magnetic NPs capable of achieving high resolution and high sensitivity have also been reported.73,74 Magnetic field-responsive NPs can be controlled to accumulate NPs in diseased tissue by simply using an external magnetic field. Hu et al. developed a kind of pPMNP-rtPA that integrates rtPA, fibrin-avid peptide and the magnetic nanoparticle PMNP. This magnetic nanoparticle targets fibrin and can be guided by a magnetic field, thus achieving thrombolysis at a low concentration.60 By incorporating tPA into porous magnetic iron oxide (Fe3O4)-microrods (tPA-MRS), the thrombolytic drug tPA can be targeted to the site of IS caused by distal occlusion of the middle cerebral artery. This NP is rod-like, as observed by scanning electron microscopy (SEM), scanning transmission electron microscopy (STEM) and transmission electron microscopy (TEM). Studies have shown that the intra-arterial injection of tPA-MRS can be targeted to the embolized site in the brain, and tPA is subsequently released under the guidance of an external magnet (Fig. 2). Under the action of an external rotating magnetic field, tPA-MRS can increase the distribution of tPA at the embolization site and can also mechanically destroy the blood clot, which further increases the penetration of tPA. This treatment strategy significantly reduced the time to recanalization of blood flow compared to the tPA treatment group. The downsides of this material are the possibility of endothelial damage and the possibility that a clot that is not destroyed may form another clot in the finer blood vessels downstream. Therefore, further research is necessary. In other reports, L-arginine magnetic NPs wrapped by platelet membranes can be guided by an external magnetic field and targeted by platelet membranes to the characteristics of the injured tissue, and they can aggregate in ischaemic brain tissue by releasing the vasodilator NO.75 In brief, high-resolution imaging depends on magnetic nanoparticles with high magnetic field sensitivity and the field strength provided by the MR scanner, and IONPS as a potential MRI contrast agent needs further investigation. This also limits its application and promotion to some extent.
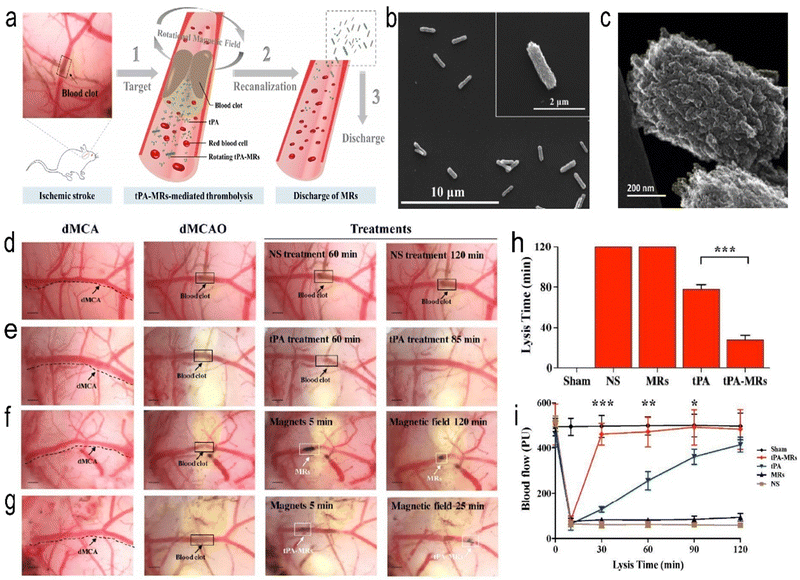 |
| Fig. 2 (a) Schematic representation of the tPA-MR thrombolysis process. (b) SEM images of the Fe3O4-C MRs. (c) TEM image of the edge of an Fe3O4-C MR. (d) Representative images of thrombolysis in dMCAO mice treated with normal saline (NS). (e) After treatment with tPA, the blood clot was lysed. (f) Representative images of thrombolysis in dMCAO mice. (g) In the tPA-MR solution group, thrombi could be lysed. (h) Plot of lysis time for recanalization in the dMCAO mouse model. (i) Laser Doppler test of dMCA blood flow in sham-operated mice and before and after the formation of thrombi in the dMCA. Reprinted (adapted) with permission from ref. 60. Copyright 2018, American Chemical Society. | |
4.2 Ultrasound-responsive NPs
Ultrasound is considered a nondrug strategy for the treatment of cerebral ischaemia,61 which is based on the principle that ultrasound generates microbubbles to mechanically destroy thrombi. The created magnetic microvesicles are guided with the help of an external magnetic field to the thrombus area, where ultrasound-mediated tPA release and rupture of the microvesicles lead to thrombolysis. Zhong et al. synthesized Fe3O4-PLGA-PFH-CREKA NPs. CREKA (Cys-Arg-Glu-Lys-Ala) specifically targets fibrin, and perfluorohexane (PFH) is a functional reagent that causes a liquid–gas phase transition (PT) (Fig. 3).61 This NP is shown to be spherical by TEM. Fe and fluorine (F) were confirmed to be present in the NPs by elemental line-scan mapping. The highly biocompatible compound PLGA was used as the carrier. This NP is also responsive to ultrasound and magnetic fields and targets thrombi. It can be used for the early noninvasive diagnosis and treatment of arterial thrombosis and magnetic resonance (MR) and photoacoustic (PA) imaging. This treatment strategy was shown to significantly reduce thrombus volume in a Sprague Dawley (SD) rat abdominal aortic thrombosis model. However, this therapeutic strategy acts by activating the fibrinolytic system rather than inhibiting platelet aggregation, as confirmed by D-dimer, which reflects the degree of fibrinolysis, and P-selectin, which reflects the degree of platelet aggregation. Atherosclerosis is an important cause of IS.76,77 Mehta et al. developed a system that could deliver 2-hydroxypropyl-beta-cyclodextrin (CD, which dissolves cholesterol and reduces the size of atherosclerotic plaques) NPs (CDNPs) via air-trapped polybutylcyanoacrylate NPs (with diameters of 388 ± 34 nm), which significantly increased CD uptake in mouse cells. The contrast agent can be used for ultrasound and near-infrared (NIR) imaging, and ultrasound can also enhance the uptake of NPs by cells.27 Focused ultrasound can increase the entry of therapeutic NPs into brain tissue by increasing the permeability of the BBB. Nance et al. tested the increased ability of NPs to penetrate the BBB after temporary and reversible local disruption of the barrier using focused ultrasound (FUS) and intravascular microbubbles (MBs) following MR localization.78 Targeted ultrasonic microbubble destruction (UTMD) is a minimally invasive method to open the BBB.79 Zhao et al. developed PS-containing microbubbles (PS-MBs) that open the BBB through UTMD and then convert to PS-NPs, which are ingested by activated microglia/macrophages (M/M). MBs can be used as a drug carrier-loaded therapy component in the treatment of IS. Safety is still needed to consider problems, including damage to the surrounding tissue and blood clots after fracture potential microthrombus again for distal vascular embolization. Other imaging detection methods need continuous improvement.
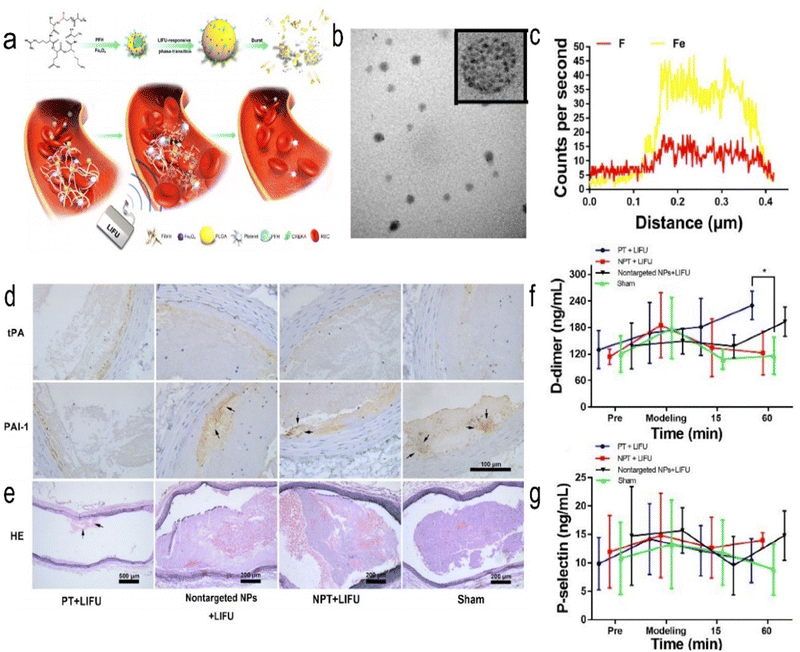 |
| Fig. 3 (a) Schematic representation of the PT thrombolysis process, wherein LIFU pulses are applied remotely from outside the blood vessel. (b) Representative TEM images of Fe3O4-PLGA-PFH-CREKA. (c) Elemental line-scan mapping of Fe3O4-PLGA-PFH-CREKA NPs. (d) Representative immunohistochemistry images of tPA and PAI-1 expression. (e) Representative H&E staining images of long-axis abdominal aorta thrombosis in SD rats. (f) Changes in plasma D-dimer levels. (g) Changes in plasma P-selectin levels. Reprinted (adapted) with permission from ref. 61. Copyright 2019, American Chemical Society. | |
5. NPs targeting ischaemic tissue
Pathological processes of IS (such as inflammation, hypoxia, oxidative stress, acidosis, etc.) are used as targets for the development of stimulus-responsive biomaterials. These biomaterials can “read” microenvironmental changes in ischaemic tissue and respond in predetermined ways to help alleviate, suppress, and sometimes even reverse symptoms of the disease. How these properties can be used as stimuli to develop smart NPs targeting ischaemic tissue in IS is described next.
5.1 Light-sensitive NPs
Near-infrared light (NIR) can penetrate scalp, skull and brain tissue,80–82 and various NPs can be developed to detect and treat IS through the photobiostimulation effect generated by such irradiation.83,84 Zhang et al. developed a highly reactive oxygen species (HROS) response ratio NIR-II nanoprobe based on the dye sensitization system between IR-783 dyes and lanthanide-doped NPs (IR-LnNPs) (Fig. 4). The probe can distinguish the oxidative stress classification of different regions of the lesion site by responding to HROS. Moreover, IR-II can show ischaemic areas 30 minutes after cerebral ischaemia, long before brain damage can be detected by CT or MRI. The same results were confirmed by staining of tissue sections. Thus, early assessment of IS has been achieved.62 Shao et al. used the photothermal effect to create erythrocyte membrane-cloaked Janus polymeric motors (EM-JPMs), which were propelled by NIR laser irradiation and successfully applied in thrombus ablation. Adjusting the power of NIR light to control the temperature increases the temperature of photosensitive materials, resulting in the ablation of blood clots. However, NIR irradiation may also damage adjacent tissues.85 NPs have also been developed that integrate multiple functions of targeting, thrombolysis, and imaging. Urokinase-type plasminogen activator (Uk) is a kind of thrombolytic drug, but due to its nonspecific distribution, it must be used in large doses to increase the concentration at the thrombus to play a role in thrombolysis. However, it also leads to a risk of bleeding. Chang et al. developed a multifunctional nanoparticle in which fucoidan (Fu), an algale-derived anticoagulant, specifically binds to P-selectin, giving the nanoparticle the ability to target the activated platelet thrombus site. The nanoparticles also contained indocyanine green (ICG), a near-infrared (NIR) fluorescence imaging contrast agent, which was incorporated into arginine peptide-functionalized mesoporous silicon-coated gold nanorods (photothermal Si-AuNRs). This nanocomposite has multiple functions, including thrombolytic targeted drug delivery, photothermal thrombolysis, and near-infrared fluorescence imaging, and is therefore an advanced thrombolytic therapy platform to reduce bleeding risk and improve imaging/thrombolysis efficiency. These composite nanomaterials have a variety of functions, including the ability to target activated platelets at the site of the thrombus for rapid removal and prevention of thrombus reformation, while imaging capabilities allow them to monitor the efficacy of thrombolysis, making them promising targeted NPs.86 Although near-infrared light penetration is good, it is still limited, and the deeper tissue effect is poorer. Near-infrared light irradiation in vitro is only an approximate range that cannot be accurately achieved, so photosensitive nanoparticle targeting is particularly important; otherwise, it will damage adjacent tissues, and a more controllable thermal effect of the material needs to be developed.
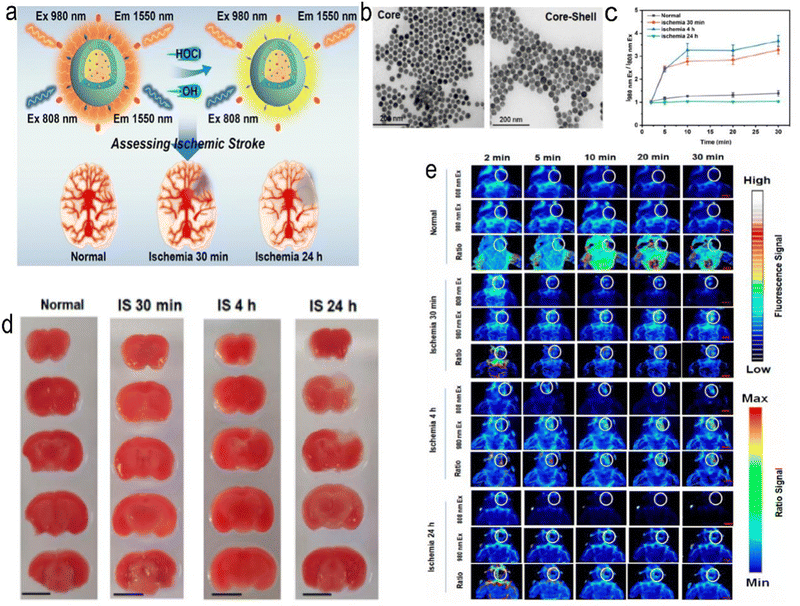 |
| Fig. 4 (a) Schematic representation of ratiometric NIR-II luminescent nanoprobes for the treatment and assessment of IS. (b) TEM images of core (NaYbF4:5%Er,5%Ce) and core–shell structured (NaYbF4:5%Er,5%Ce@NaYF4:20%Nd) LnNPs. (c) Ratio value at the lesion site for normal mice and mice with IS. (d) 2,3,5-Triphenyl tetrazolium hydrochloride (TTC) staining results for normal mice and mice with cerebral ischaemia at different times. (e) In vivo NIR-II luminescence imaging of normal mice and mice with cerebral ischaemia. Reprinted (adapted) with permission from ref. 62. Copyright 2021, American Chemical Society. | |
5.2 pH-responsive NPs
Low pH in ischaemic brain tissue can be the target of targeted NPs. In IS, the lack of oxygen supply at the ischaemic site causes tissue metabolism to switch from aerobic to anaerobic, resulting in lactic acid build-up and a decrease in pH that can be used to guide pH-targeting NPs. Yao et al. prepared a pH-responsive fluorescent liposome probe (BOD@Lip) that combines the benefits of near-infrared fluorescence (NIRF) and targeting ischaemic tissue. It can enable the observation of ischaemic brain tissue in real time with high sensitivity and high resolution, providing a basis for clinicians to adopt timely and accurate treatment.87 Cheng et al. designed a pH-sensitive RAPA NP system that enhances magnetic resonance imaging (MRI) and NIRF imaging signals, and the NPs can be loaded with rapamycin for neuroprotective effects.88 Zhang et al. designed pH-sensitive NPs loaded with betulinic acid (BA), a natural antioxidant component.89 NPs that combine multiple therapeutic components have also been developed. Mei et al. designed pH-sensitive t-PA-coated antioxidation self-assembled polyion-complex NPs (t-PA@iRNP). It targets ischaemic tissue and releases thrombolytic drugs and antioxidants.63 Brain tissue can be protected from further damage by blood flow recirculation therapy and neuroprotective therapy, but due to the limited regenerative ability of brain tissue itself, necrotic brain tissue cannot be completely regenerated.90 Therefore, exogenous treatment will be needed. Neural stem cells (NSCs) can regenerate and differentiate and have great potential in the treatment of IS, but their efficacy is limited in the harsh ischaemic microenvironment rich in reactive oxygen species (ROS).65 Yang et al. developed a Ca-MOF@miR-124 nanoparticle, a pH-responsive nanoparticle that encounters the low pH of neural stem cell lysozyme and releases miR-124, a miRNA that promotes neural stem cells to differentiate into mature neurons (Fig. 5).64 It prevents the rapid degradation of miR-124 during systemic administration and increases the internalization of miR-124. The NPs were found to enhance the efficacy of NSCs in the treatment of IS, reducing ischaemic infarct size to almost normal levels by day 7.
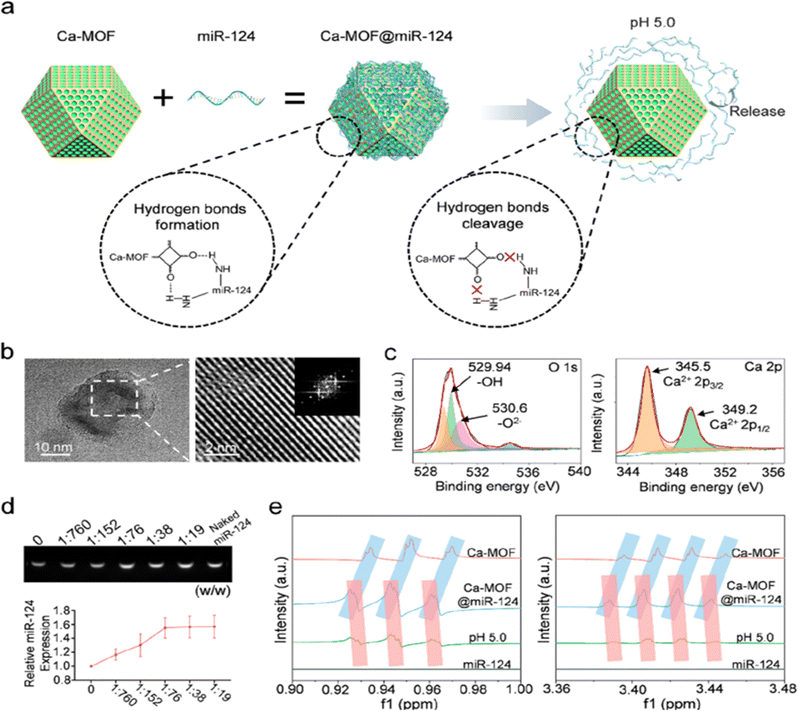 |
| Fig. 5 Design and characterization of Ca-MOF@miR-124 NPs. (a) Diagram of the effective loading of miR-124 onto the surface of Ca-MOF. (b) TEM image and HRTEM image of the Ca-MOF NPs in the supernatant after ultrasonication. (c) XPS analysis of Ca-MOF showing its elemental composition. (d) Agarose gel electrophoresis analysis of miR-124 in Ca-MOF@miR-124 NPs. (e) 1H NMR spectra of miR-124, Ca-MOF NPs, and Ca-MOF@miR-124 NPs. Reprinted (adapted) with permission from ref. 64. Copyright 2022, American Chemical Society. | |
However, due to the high complexity of biological systems, these methods suffer from off-target effects. To overcome these barriers, more stimulus response platforms can be designed to identify multiple IS characteristics and relieve off-target effects. In addition, the combination of passive targeting with active targeting would have additional advantages during drug delivery.
5.3 ROS-responsive NPs
When IS occurs, ROS in brain tissue are upregulated,91 and during ischaemia–reperfusion, ROS are explosively released,92 further damaging pathological tissue. Therefore, the use of antioxidant drugs is necessary,8,93 and ROS can also be the target of targeted NPs, which have great application potential.94,95
Mitochondrial volume accounts for 25% of nerve cell volume, and mitochondrial dysfunction plays a central role in IS.96 The brain is particularly vulnerable to ROS attacks97 because the brain is a highly oxygen-consuming organ (accounting for 20% of total body consumption), but its protective antioxidant mechanism is relatively weak.98 Studies have shown that increased levels of oxidative stress occur in the early stage of IS and are associated with subsequent brain injury.99,100 Therefore, oxidative stress levels can reflect the severity of IS. Lv et al. developed bioengineered and ROS-responsive nanocarriers for the targeted delivery and release of the stroke-specific neuroprotective agent NR2B9C to combat ischaemic brain injury (Fig. 6).42 Boroester is a smart ROS-responsive biomaterial that reacts with the hydroxyl group of dextran, a glucose polymer with good water solubility and biocompatibility, and self-assembles into nanoparticles. This nanoparticle encapsulates NR2B9C, a neuroprotective agent, and forms the “core” of SHp-RBC-NP.101
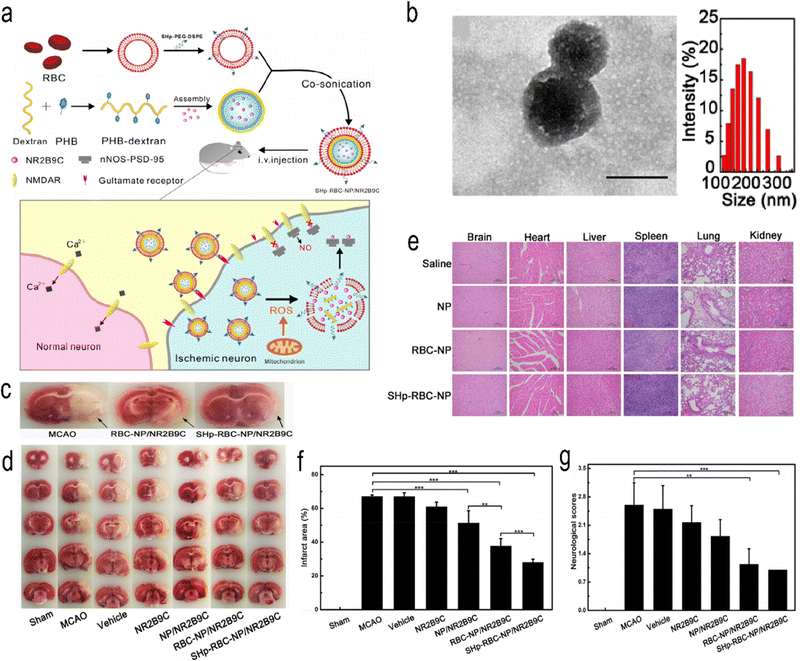 |
| Fig. 6 (a) Schematic design of SHp-RBC-NP/NR2B9C. (b) TEM images and hydrodynamic size distribution of RBC-coated NPs. (c) Representative tissue slices showing that the RBC-NP/NR2B9C and SHp-RBC-NP/NR2B9C groups had significantly reduced infarct volumes. (d) Representative TTC-stained brain sections. (e) Histochemistry analysis of brain, heart, liver, spleen, lung, and kidney tissue sections stained with haematoxylin–eosin. (f) Quantification of brain infarct volume at 24 h after MCAO in rats. (g) Neuroscores of rats after cerebral ischaemia. Reprinted (adapted) with permission from ref. 42. Copyright 2018, American Chemical Society. | |
The erythrocyte membrane has the characteristics of ultrahigh biocompatibility, immune evasion ability and long blood retention time. In the erythrocyte membrane, a stroke-homing peptide (SHp, CLEVSRKNC) has been shown to selectively target brain ischaemic sites and can be colocalized in some apoptotic neurons in the penumbra area of ischaemic brain tissue to form the shell of SHp-RBC-NPs.102 When these NPs are fabricated to target ischaemic brain tissue, these NPs can control the release of NR2B9C from ischaemic neurons triggered by high intracellular ROS. A rat model of middle cerebral artery occlusion (MCAO) was established. The results of in vitro experiments showed that SHp-RBC-NPs antagonized glutamate-induced cytotoxicity in PC-12 cells. The same results were obtained in vivo, in which the retention time of NR2B9C in the systemic circulation was increased, which enhanced the active targeting of the ischaemic region in MCAO rats. The results showed that the NPs significantly reduced infarct size and improved neurological scores. Meanwhile, the NPs also showed good biocompatibility in the safety evaluation. Endogenous NSCs have the potential to differentiate into other neural cells.103 Endogenous neural stem cells (NSCs) can migrate to the lesion site after IS.104,105 It has functions such as cell replacement, neurotrophic effects and immune regulation.106 Jiang et al. developed a ROS-responsive charge-reversal poly[(2-acryloyl) ethyl (p-boronic acid benzyl) diethylammonium bromide] (B-PDEA) nanoparticle, which transfected brain-derived neurotrophic factor (BDNF) into endogenous NSCs, thereby sustaining the expression of BDNF and providing neuroprotective effects.65 Jin et al. synthesized ROS-responsive GA-conjugated diethylaminoethylene (DEAE)-dextran NPs (DGA) to inhibit microglial polarization manipulated by high mobility group Box 1 (HMGB1) to improve the efficacy of cerebral ischaemia therapy.66 Activated platelets are the building blocks of thrombosis.107,108 Thrombosis is often accompanied by elevated ROS.109 The increase in ROS can be used as a target for the development of ROS-responsive NPs, and scavenging ROS is a potential therapeutic tool for thrombus removal.110,111 Wang et al.67 developed platelet-targeted theranostic NPs (CyBA/PFM NPs). Amphiphilic phenylboronated fucoidan/maltodextrin (PHB-Fuco/Malto) polymers were synthesized by introducing hydrophobic phenylboronic branched chains into hydrophilic fucoidan/maltodextrin hybrid polysaccharides. The NPs were then self-assembled with the H2O2-sensitive “switching signal” near-infrared (NIR, 650–900 nm) probe CyBA. The NPs could remove ROS, remove clots, turn on the acousto-optic signal by short laser pulses with a length of several nanoseconds,112 and then use multispectral photoacoustic tomography (MSOT) to detect vessels at a depth of 1–5 cm in a noninvasive manner with high resolution and high contrast.112–114 The neuroprotective mechanisms of these antioxidants need further understanding. In addition, mitochondria are the key organelles for ROS production, and a better understanding of the mechanism of ROS production and more precise targeting of mitochondria may yield just the right therapeutic effect. In addition, the production and concentration of ROS in vivo are also dynamically changing. It is also a challenge to distinguish between normal levels of ROS and pathological sites. Considering clinical translation, biocompatibility, nontoxicity, and immunogenicity should all be considered.
5.4 Enzyme-responsive NPs
Guo et al. reported brain-targeting AMD3100-conjugated, size shrinkable NPs (ASNPs) that respond to proteases to improve stroke treatment.68 The NPs respond to thrombin or matrix metalloproteinase 9 (MMP-9). Brain targeting is mediated by the interaction of AMD3100 with CXCR4, which is abundant in ischaemic brain tissue. ASNPs significantly improved the efficiency of gliburide penetrating cerebral ischaemia and reduced the side effects of systemic gliburide administration (Fig. 7). The experimental results show that the NPs can effectively penetrate the BBB and have strong specificity for ischaemic tissue. At the same time, it has high safety and low toxicity. To achieve clinical translation, higher biosafety and targeting are necessary. Additionally, further clarification of the biological role of MMPs in vivo is the foundation of designing efficient targeted nanoparticles.
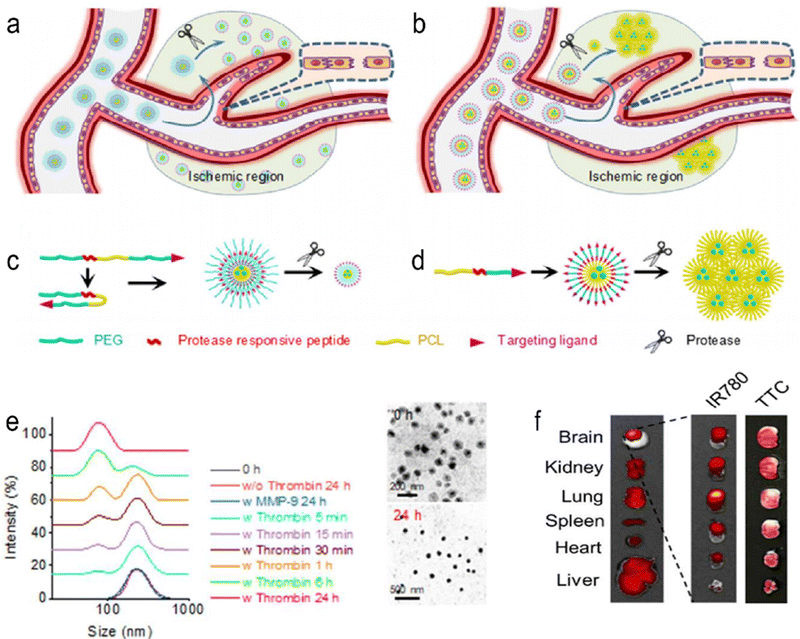 |
| Fig. 7 Schematic diagrams of shrinkable (a) and (c) and expandable (b) and (d) micellar NPs in response to proteases enriched in the ischaemic microenvironment. (e) Dynamic light scattering (DLS) (left) and TEM (right) analyses of PEGoPCLoToPEG NPs in PBS with or without thrombin/MMP-9. (f) Representative images of brain slices with TTC staining (left) and fluorescence imaging (right). Reprinted (adapted) with permission from ref. 68. Copyright 2018, American Chemical Society. | |
6. Targeted NPs to overcome the blood–brain barrier
The BBB has numerous functions and important roles.115,116 The BBB is mainly composed of a physical barrier and a selective metabolic drive barrier. The physical barrier consists of infiltrated brain microvascular endothelial cells and the tight junctions between them. In addition, tight junction proteins and adherens junction proteins expressed by brain microvascular cells contribute to high transendothelial electrical resistance. These structures limit immune surveillance cells (macrophages), transport of xenobiotic and endogenous compounds, and paracellular access of water and solutes. The selective metabolic drive barrier primarily reflects the expression and function of several receptors, ion channels, and inflow/outflow transporters that are prominently expressed at the BBB.117 This is also an important reason why it is difficult to translate central nervous system treatment into clinical practice. Although there is a brief opening of the BBB after IS, it is not enough to allow drugs to enter the brain tissue at an effective therapeutic concentration.118 Most neuroprotective drugs do not cross the BBB.119,120 Traditional methods to overcome the BBB include invasive drug injection,121 which increases the chance of drugs crossing the BBB by improving the permeability of the local BBB and the drug residence time. However, there is a risk of causing brain tissue damage. Intranasal drug administration is also an effective way to bypass the BBB. However, studies have shown that this method of delivery is inefficient.122,123 Mechanisms for noninvasive crossing of the BBB include passive diffusion, vector-mediated transport, and endocytic/transcellular pathways.124 Physical barriers restrict the access of hydrophilic substances and macromolecules to the BBB. Selective metabolic drive barriers actively expel certain foreign molecules from the brain.125 Endocytic/transcellular actions allow internalization, sorting, and transport of many plasma macromolecules.126 Electrostatic adsorption and receptor mediation are involved in this process. Targeted NPs can enter cells through tight junctions between endothelial cells in the BBB,127 through endocytosis, or through receptor-mediated entry. Currently, the most effective strategy for crossing the BBB is to bind to receptors on the BBB by promoting receptor transport. Shen et al. developed a nanoparticle that binds to low-density lipoprotein receptors (LDLR), a resveratrol (RSV) delivery vector based on polylactic acid (PLA)-coated mesoporous silica NPs (MSNPs) (Fig. 8). In an in vitro BBB model of rat brain microvascular endothelial cells (RBECs) cocultured with microglia established in a Transwell chamber, the migration of NPs through the monomolecular layer of rat brain microvascular endothelial cells (RBECs) was significantly enhanced by coupling with LDLR.28 ROS production leads to the aggregation of inflammatory cells at the lesion site,128 which provides opportunities for therapeutic macromolecules that cannot cross the BBB.129 Zhang et al. developed a cl PGP-PEG-DGL/CAT-Aco system. that binds neutrophils, crosses the BBB with neutrophils, reaches the site of injury and is finally released to play a role in antioxidant therapy.23
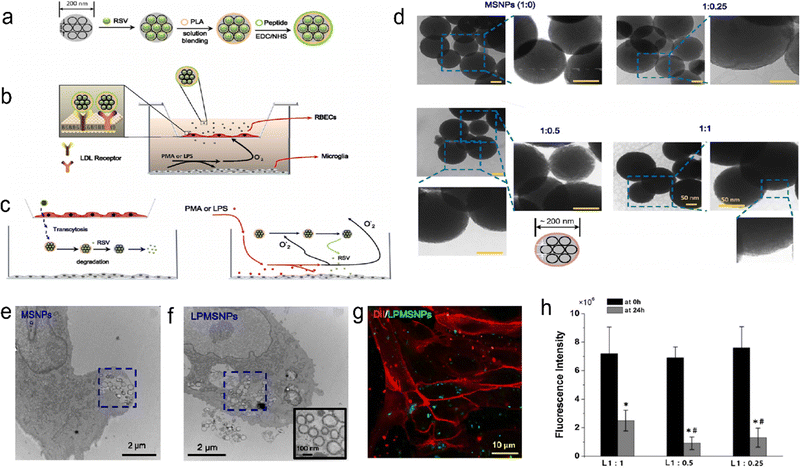 |
| Fig. 8 Schematic of the MSNP delivery system. (a) Design of RSV-loaded MSNPs with a peptide-bound PLA coating. (b) An in vitro BBB model with RBECs cultured on semipermeable membranes of a Transwell chamber. (c) PMA- or LPS-stimulated microglia produce superoxide or nitric oxide. (d) TEM images of PLA-coated MSNPs with different mass ratios. (e) TEM image of MSNPs internalized by an RBEC. (f) TEM image of LDL peptide-decorated PMSNPs internalized by an RBEC. (g) Fluorescence images showing RBECs cultured with LPMSNPs. (h) The fluorescence intensity of FTIC-labelled LPMSNPs in the upper Transwell chamber. Reprinted (adapted) with permission from ref. 28. Copyright 2018, The Author(s). | |
7. Conclusions and outlook
Ischaemic stroke (IS) is a serious threat to human health with high morbidity, mortality, and disability rates. Although reperfusion therapy has been widely used and proven effective, most patients are still left with long-term neurological impairment due to the narrow treatment time window and ischaemia–reperfusion injury. Although many drugs have been developed to treat ischaemic stroke, they are not effective because they do not effectively penetrate the BBB and target ischaemic tissue in the brain. New treatment strategies need to be developed. Targeted NPs show advantages in penetrating the BBB and targeting diseased tissues by changing their composition and structure. This paper reviews the therapeutic strategies and advances of using targeted NPs in the treatment of IS. Although great progress has been made, there are still many challenges. First, the targeted NPs use an endogenous substance at the site of the lesion as a target, but the concentration of the substance needs to be high enough to distinguish the lesion from normal tissue. In addition, due to the complex pathological mechanism, the endogenous substance concentration as the target often has temporal and spatial dynamic changes. Therefore, the developed targeted NPs should also have corresponding mechanisms. Second, targeted NPs will still miss the target. Therefore, active targeting, passive targeting and multiple targeting are often combined to improve targeting, but this also increases the difficulty of manufacturing NPs. Third, we should also consider the biocompatibility and degradability of this exogenous synthetic material. Fourth, the implementation of clinical transformation, including biological stability, effectiveness and safety evaluation, mass production, consistency, evaluation standards, storage stability, etc.25,130 Finally, the pathophysiological mechanism of IS needs further investigation, which is the basis for our development of responsive nanoparticle targets. Although many challenges remain, targeted NPs have proven to be effective in the prevention, detection, and treatment of IS and show great potential. With advances in nanotechnology, more complex processes can be applied to the preparation of NPs, and with advances in medicine, the understanding of the pathological mechanism of IS is deepened. More NPs with precise targeting ability, multiple targeting, high permeability to the BBB, high bioavailability, histocompatibility, and low toxicity will be developed. Targeted NPs are expected to become an ideal drug for the treatment of IS with broad prospects. In this review, we summarize the role of targeting NPs in the prevention, detection, and treatment of IS, providing innovative insights and clues for future developments.
Conflicts of interest
The authors declare that they have no known competing financial interests or personal relationships that could have appeared to influence the work reported in this paper.
Acknowledgements
This work was financially supported by National Natural Science Foundation of China (22372001), the Key Research Project of the Natural Science Foundation of Anhui Provincial Universities (KJ2023AH050997), the Anhui Provincial Health Commission (AHWJ2022b021, GCCRC2022019), Anhui Provincial Natural Science Foundation (2308085MH259), Natural Science Research Project of Anhui Educational Committee (2022AH040169, 2022AH051219, 2023AH051776), Yijishan Hospital Talent Special Research Fund (YR20220204), Science and Technology Project of Wuhu City (2022jc63), Open Project of Key Laboratory of Noncoding RNA Transformation Research of Anhui Higher Education Institution (RNA202201), Starting Fund for Scientific Research of High-Level Talents, Anhui Agricultural University (rc382108), Clinical Medical Research Transformation Project of Anhui Province (202304295107020002) and Research on Active Biomacromolecules at the School Level Open Project of Anhui Provincial Key Laboratory (LAB202203).
References
- C. W. Tsao, A. W. Aday, Z. I. Almarzooq, A. Alonso, A. Z. Beaton, M. S. Bittencourt, A. K. Boehme, A. E. Buxton, A. P. Carson, Y. Commodore-Mensah, M. S. V. Elkind, K. R. Evenson, C. Eze-Nliam, J. F. Ferguson, G. Generoso, J. E. Ho, R. Kalani, S. S. Khan, B. M. Kissela, K. L. Knutson, D. A. Levine, T. T. Lewis, J. Liu, M. S. Loop, J. Ma, M. E. Mussolino, S. D. Navaneethan, A. M. Perak, R. Poudel, M. Rezk-Hanna, G. A. Roth, E. B. Schroeder, S. H. Shah, E. L. Thacker, L. B. VanWagner, S. S. Virani, J. H. Voecks, N. Y. Wang, K. Yaffe and S. S. Martin, Circulation, 2022, 145, e153–e639 CrossRef PubMed.
- Z. Wang, S. Hu, S. Sang, L. Luo and C. Yu, Stroke, 2017, 48, 271–275 CrossRef PubMed.
- K. L. Furie and M. V. Jayaraman, Stroke, 2018, 49, 509–510 CrossRef PubMed.
- P. Gervois, E. Wolfs, J. Ratajczak, Y. Dillen, T. Vangansewinkel, P. Hilkens, A. Bronckaers, I. Lambrichts and T. Struys, Med. Res. Rev., 2016, 36, 1080–1126 CrossRef PubMed.
- S. S. Virani, A. Alonso, E. J. Benjamin, M. S. Bittencourt, C. W. Callaway, A. P. Carson, A. M. Chamberlain, A. R. Chang, S. Cheng, F. N. Delling, L. Djousse, M. S. V. Elkind, J. F. Ferguson, M. Fornage, S. S. Khan, B. M. Kissela, K. L. Knutson, T. W. Kwan, D. T. Lackland, T. T. Lewis, J. H. Lichtman, C. T. Longenecker, M. S. Loop, P. L. Lutsey, S. S. Martin, K. Matsushita, A. E. Moran, M. E. Mussolino, A. M. Perak, W. D. Rosamond, G. A. Roth, U. K. A. Sampson, G. M. Satou, E. B. Schroeder, S. H. Shah, C. M. Shay, N. L. Spartano, A. Stokes, D. L. Tirschwell, L. B. VanWagner and C. W. Tsao, Circulation, 2020, 141, e139–e596 Search PubMed.
- S. J. Mendelson and S. Prabhakaran, JAMA, 2021, 325, 1088–1098 CrossRef CAS PubMed.
- J. Castillo, M. I. Loza, D. Mirelman, J. Brea, M. Blanco, T. Sobrino and F. Campos, J. Cereb. Blood Flow Metab., 2015, 36, 292–301 CrossRef PubMed.
- A. Chamorro, U. Dirnagl, X. Urra and A. M. Planas, Lancet Neurol., 2016, 15, 869–881 CrossRef CAS PubMed.
- C. Iadecola and J. Anrather, Nat. Med., 2011, 17, 796–808 CrossRef CAS PubMed.
- S. Parvez, M. Kaushik, M. Ali, M. M. Alam, J. Ali, H. Tabassum and P. Kaushik, Theranostics, 2022, 12, 689–719 CrossRef CAS PubMed.
- G. A. Donnan, S. M. Davis, M. W. Parsons, H. Ma, H. M. Dewey and D. W. Howells, Nat. Rev. Neurol., 2011, 7, 400–409 CrossRef CAS PubMed.
- L. H. Schwamm, S. F. Ali, M. J. Reeves, E. E. Smith, J. L. Saver, S. Messe, D. L. Bhatt, M. V. Grau-Sepulveda, E. D. Peterson and G. C. Fonarow, Circ.: Cardiovasc. Qual. Outcomes, 2013, 6, 543–549 Search PubMed.
- I. Gravanis and S. E. Tsirka, Expert Opin. Ther. Targets, 2008, 12, 159–170 CrossRef CAS PubMed.
- B. Frank, J. C. Grotta, A. V. Alexandrov, E. Bluhmki, P. Lyden, A. Meretoja, N. K. Mishra, A. Shuaib, N. G. Wahlgren, C. Weimar and K. R. Lees, Stroke, 2013, 44, 727–733 CrossRef CAS PubMed.
- W. Hacke, G. Donnan, C. Fieschi, M. Kaste, R. von Kummer, J. P. Broderick, T. Brott, M. Frankel, J. C. Grotta, E. C. Haley, Jr., T. Kwiatkowski, S. R. Levine, C. Lewandowski, M. Lu, P. Lyden, J. R. Marler, S. Patel, B. C. Tilley, G. Albers, E. Bluhmki, M. Wilhelm and S. Hamilton, Lancet, 2004, 363, 768–774 CrossRef PubMed.
- S. Cadenas, Free Radic. Biol. Med., 2018, 117, 76–89 CrossRef CAS PubMed.
- C. L. Allen and U. Bayraktutan, Int. J. Stroke, 2009, 4, 461–470 CrossRef CAS PubMed.
- C. G. Kevil, T. Oshima, B. Alexander, L. L. Coe and J. S. Alexander, Am. J. Physiol.: Cell Physiol., 2000, 279, C21–C30 CrossRef CAS PubMed.
- P. J. Kelly, J. D. Morrow, M. Ning, W. Koroshetz, E. H. Lo, E. Terry, G. L. Milne, J. Hubbard, H. Lee, E. Stevenson, M. Lederer and K. L. Furie, Stroke, 2008, 39, 100–104 CrossRef CAS PubMed.
- H. K. Eltzschig and T. Eckle, Nat. Med., 2011, 17, 1391–1401 CrossRef CAS PubMed.
- V. E. O'Collins, M. R. Macleod, G. A. Donnan, L. L. Horky, B. H. van der Worp and D. W. Howells, Ann. Neurol., 2006, 59, 467–477 CrossRef PubMed.
- M. V. Sofroniew and H. V. Vinters, Acta Neuropathol., 2010, 119, 7–35 CrossRef PubMed.
- C. Zhang, C. L. Ling, L. Pang, Q. Wang, J. X. Liu, B. S. Wang, J. M. Liang, Y. Z. Guo, J. Qin and J. X. Wang, Theranostics, 2017, 7, 3260–3275 CrossRef CAS PubMed.
- H. Wu, T. Zhang, N. Li and J. Gao, J. Controlled Release, 2023, 360, 169–184 CrossRef CAS PubMed.
- B. Choi, W. Park, S.-B. Park, W.-K. Rhim and D. K. Han, Methods, 2020, 177, 2–14 CrossRef CAS PubMed.
- S. Zhang, Y. Zhou, R. Li, Z. Chen and X. Fan, J. Controlled Release, 2022, 344, 173–201 CrossRef CAS PubMed.
- S. Mehta, V. Bongcaron, T. K. Nguyen, Y. Jirwanka, A. Maluenda, A. P. G. Walsh, J. Palasubramaniam, M. D. Hulett, R. Srivastava, A. Bobik, X. Wang and K. Peter, Small, 2022, 18, e2200967 CrossRef PubMed.
- Y. Shen, B. Cao, N. R. Snyder, K. M. Woeppel, J. R. Eles and X. T. Cui, J. Nanobiotechnol., 2018, 16, 13 CrossRef PubMed.
- B. C. V. Campbell, D. A. De Silva, M. R. Macleod, S. B. Coutts, L. H. Schwamm, S. M. Davis and G. A. Donnan, Nat. Rev. Dis. Primers, 2019, 5, 70 CrossRef PubMed.
- J. L. Mega and T. Simon, Lancet, 2015, 386, 281–291 CrossRef CAS PubMed.
- X. Wang, M. Ouyang, J. Yang, L. Song, M. Yang and C. S. Anderson, Cochrane Database Syst. Rev., 2021, 10, CD000024 Search PubMed.
- P. A. Sandercock, C. Counsell and E. J. Kane, Cochrane Database Syst. Rev., 2015, 2015, CD000024 Search PubMed.
- J. Zhu, M. Ma, Y. Guo, M. Zhou, J. Guo and L. He, BMC Neurol., 2018, 18, 194 CrossRef CAS PubMed.
- K. H. Choi, J. H. Kim, C. Lee, J. M. Kim, K. W. Kang, J. T. Kim, S. M. Choi, M. S. Park and K. H. Cho, Stroke, 2020, 51, 3514–3522 CrossRef CAS PubMed.
- L. Chen, S. Cao and J. Yang, Neurol. Res., 2018, 40, 862–867 CrossRef CAS PubMed.
- P. Tanswell, N. Modi, D. Combs and T. Danays, Clin. Pharmacokinet., 2002, 41, 1229–1245 CrossRef CAS PubMed.
- J. Horn, R. J. de Haan, M. Vermeulen, P. G. Luiten and M. Limburg, Stroke, 2001, 32, 2433–2438 CrossRef CAS PubMed.
- H. Zheng, Y. Wang, A. Wang, H. Li, D. Wang, X. Zhao, P. Wang, H. Shen, L. Zuo, Y. Pan, Z. Li, X. Meng, X. Wang, W. Shi, Y. Ju, L. Liu, K. Dong, C. Wang, R. Sui, R. Xue, X. Pan, X. Niu, B. Luo, Y. Sui, H. Wang, T. Feng and Y. Wang, Sci. Bull., 2019, 64, 101–107 CrossRef CAS PubMed.
- T. Wada, H. Yasunaga, R. Inokuchi, H. Horiguchi, K. Fushimi, T. Matsubara, S. Nakajima and N. Yahagi, J. Neurol. Sci., 2014, 345, 106–111 CrossRef CAS PubMed.
- M. Trotman, P. Vermehren, C. L. Gibson and R. Fern, J. Cereb. Blood Flow Metab., 2015, 35, 230–239 CrossRef CAS PubMed.
- S. M. Seyedsaadat and D. F. Kallmes, Rev. Neurosci., 2019, 30, 203–220 CAS.
- W. Lv, J. Xu, X. Wang, X. Li, Q. Xu and H. Xin, ACS Nano, 2018, 12, 5417–5426 CrossRef CAS PubMed.
- T. Huang, N. Li and J. Gao, Asian J. Pharm. Sci., 2019, 14, 233–247 CrossRef PubMed.
- N. Mackman, Nature, 2008, 451, 914–918 CrossRef CAS PubMed.
- B. C. V. Campbell, P. J. Mitchell, L. Churilov, N. Yassi, T. J. Kleinig, R. J. Dowling, B. Yan, S. J. Bush, H. M. Dewey, V. Thijs, R. Scroop, M. Simpson, M. Brooks, H. Asadi, T. Y. Wu, D. G. Shah, T. Wijeratne, T. Ang, F. Miteff, C. R. Levi, E. Rodrigues, H. Zhao, P. Salvaris, C. Garcia-Esperon, P. Bailey, H. Rice, L. de Villiers, H. Brown, K. Redmond, D. Leggett, J. N. Fink, W. Collecutt, A. A. Wong, C. Muller, A. Coulthard, K. Mitchell, J. Clouston, K. Mahady, D. Field, H. Ma, T. G. Phan, W. Chong, R. V. Chandra, L. A. Slater, M. Krause, T. J. Harrington, K. C. Faulder, B. S. Steinfort, C. F. Bladin, G. Sharma, P. M. Desmond, M. W. Parsons, G. A. Donnan and S. M. Davis, N. Engl. J. Med., 2018, 378, 1573–1582 CrossRef CAS PubMed.
- R. L. Medcalf, Br. J. Pharmacol., 2012, 165, 75–89 CrossRef CAS PubMed.
- S. Amemiya, T. Kamiya, C. Nito, T. Inaba, K. Kato, M. Ueda, K. Shimazaki and Y. Katayama, Eur. J. Pharmacol., 2005, 516, 125–130 CrossRef CAS PubMed.
- G. Edaravone Acute Infarction Study, Cerebrovasc. Dis., 2003, 15, 222–229 CrossRef PubMed.
- Y. Fu, N. Zhang, L. Ren, Y. Yan, N. Sun, Y. J. Li, W. Han, R. Xue, Q. Liu, J. Hao, C. Yu and F. D. Shi, Proc. Natl. Acad. Sci. U. S. A., 2014, 111, 18315–18320 CrossRef CAS PubMed.
- J. Huang, T. F. Choudhri, C. J. Winfree, R. A. McTaggart, S. Kiss, J. Mocco, L. J. Kim, T. S. Protopsaltis, Y. Zhang, D. J. Pinsky and E. S. Connolly, Jr., Stroke, 2000, 31, 3047–3053 CrossRef CAS PubMed.
- J. Xiang, R. Zhao, B. Wang, X. Sun, X. Guo, S. Tan and W. Liu, Front. Oncol., 2021, 11, 758143 CrossRef CAS PubMed.
- R. Bendayan, G. Lee and M. Bendayan, Microsc. Res. Tech., 2002, 57, 365–380 CrossRef CAS PubMed.
- J. Zhu, W. Chen, Y. Sun, X. Huang, R. Chu, R. Wang, D. Zhou and S. Ye, Mater. Adv., 2022, 3, 7687–7708 RSC.
- J. Zhu, R. Hou, M. Liu, L. Wang, W. Chen, Y. Sun, W. Wei and S. Ye, Mater. Today Sustainability, 2022, 18, 100125 CrossRef.
- H. L. Wong, X. Y. Wu and R. Bendayan, Adv. Drug Delivery Rev., 2012, 64, 686–700 CrossRef CAS PubMed.
- R. Zhong, R. Chu, J. Zhu, J. Ling, L. Zhang, Y. Zhou, M. Yin, Z. Hao, C. Liang, S. Cao, T. Xu, S. Ye and S. Fan, Mater. Today Sustainability, 2023, 21, 100323 CrossRef.
- P. M. Winter, A. M. Morawski, S. D. Caruthers, R. W. Fuhrhop, H. Zhang, T. A. Williams, J. S. Allen, E. K. Lacy, J. D. Robertson, G. M. Lanza and S. A. Wickline, Circulation, 2003, 108, 2270–2274 CrossRef CAS PubMed.
- L. O. Sillerud, Y. Yang, L. Y. Yang, K. B. Duval, J. Thompson and Y. Yang, J. Cereb. Blood Flow Metab., 2020, 40, S117–S133 CrossRef CAS PubMed.
- H. A. Chen, Y. H. Ma, T. Y. Hsu and J. P. Chen, Int. J. Mol. Sci., 2020, 21, 2690 CrossRef CAS PubMed.
- J. Hu, S. Huang, L. Zhu, W. Huang, Y. Zhao, K. Jin and Q. ZhuGe, ACS Appl. Mater. Interfaces, 2018, 10, 32988–32997 CrossRef CAS PubMed.
- Y. Zhong, Y. Zhang, J. Xu, J. Zhou, J. Liu, M. Ye, L. Zhang, B. Qiao, Z. G. Wang, H. T. Ran and D. Guo, ACS Nano, 2019, 13, 3387–3403 CrossRef CAS PubMed.
- M. Zhang, Z. Wang, C. Wang, Y. Wu, Z. Li and Z. Liu, ACS Nano, 2021, 15, 11940–11952 CrossRef CAS PubMed.
- T. Mei, A. Kim, L. B. Vong, A. Marushima, S. Puentes, Y. Matsumaru, A. Matsumura and Y. Nagasaki, Biomaterials, 2019, 215, 119209 CrossRef CAS PubMed.
- H. Yang, M. Han, J. Li, H. Ke, Y. Kong, W. Wang, L. Wang, W. Ma, J. Qiu, X. Wang, T. Xin and H. Liu, ACS Nano, 2022, 16, 14503–14516 CrossRef CAS PubMed.
- X. C. Jiang, J. J. Xiang, H. H. Wu, T. Y. Zhang, D. P. Zhang, Q. H. Xu, X. L. Huang, X. L. Kong, J. H. Sun, Y. L. Hu, K. Li, Y. Tabata, Y. Q. Shen and J. Q. Gao, Adv. Mater., 2019, 31, e1807591 CrossRef PubMed.
- L. Jin, Z. Zhu, L. Hong, Z. Qian, F. Wang and Z. Mao, Bioact. Mater., 2023, 19, 38–49 CrossRef CAS PubMed.
- Y. Wang, C. Jian, Y. Long, X. Xu, Y. Song and Z. Yin, Acta Biomater., 2023, 158, 769–781 CrossRef CAS PubMed.
- X. Guo, G. Deng, J. Liu, P. Zou, F. Du, F. Liu, A. T. Chen, R. Hu, M. Li, S. Zhang, Z. Tang, L. Han, J. Liu, K. N. Sheth, Q. Chen, X. Gou and J. Zhou, ACS Nano, 2018, 12, 8723–8732 CrossRef CAS PubMed.
- L. L. Israel, A. Galstyan, E. Holler and J. Y. Ljubimova, J. Controlled Release, 2020, 320, 45–62 CrossRef CAS PubMed.
- M. Jeon, M. V. Halbert, Z. R. Stephen and M. Zhang, Adv. Mater., 2020, 33, e1906539 CrossRef PubMed.
- J. W. Bulte and D. L. Kraitchman, Curr. Pharm. Biotechnol., 2004, 5, 567–584 CAS.
- M. Wiart, N. Davoust, J. B. Pialat, V. Desestret, S. Moucharrafie, T. H. Cho, M. Mutin, J. B. Langlois, O. Beuf, J. Honnorat, N. Nighoghossian and Y. Berthezène, Stroke, 2007, 38, 131–137 CrossRef PubMed.
- Z. W. Tay, S. Savliwala, D. W. Hensley, K. L. B. Fung, C. Colson, B. D. Fellows, X. Zhou, Q. Huynh, Y. Lu, B. Zheng, P. Chandrasekharan, S. M. Rivera-Jimenez, C. M. Rinaldi-Ramos and S. M. Conolly, Small Methods, 2021, 5, e2100796 CrossRef PubMed.
- N. Nighoghossian, M. Wiart, S. Cakmak, Y. Berthezène, L. Derex, T. H. Cho, C. Nemoz, F. Chapuis, G. L. Tisserand, J. B. Pialat, P. Trouillas, J. C. Froment and M. Hermier, Stroke, 2007, 38, 303–307 CrossRef PubMed.
- M. Li, J. Li, J. Chen, Y. Liu, X. Cheng, F. Yang and N. Gu, ACS Nano, 2020, 14, 2024–2035 CrossRef CAS PubMed.
- Y. C. Chen, A. L. Huang, T. S. Kyaw, A. Bobik and K. Peter, Arterioscler., Thromb., Vasc. Biol., 2016, 36, e63–e72 CAS.
- X. Wang and K. Peter, Arterioscler., Thromb., Vasc. Biol., 2017, 37, 1029–1040 CrossRef CAS PubMed.
- E. Nance, K. Timbie, G. W. Miller, J. Song, C. Louttit, A. L. Klibanov, T. Y. Shih, G. Swaminathan, R. J. Tamargo, G. F. Woodworth, J. Hanes and R. J. Price, J. Controlled Release, 2014, 189, 123–132 CrossRef CAS PubMed.
- Y. Kung, C. Lan, M.-Y. Hsiao, M.-K. Sun, Y.-H. Hsu, A. P. H. Huang, W.-H. Liao, H.-L. Liu, C. Inserra and W.-S. Chen, Sci. Rep., 2018, 8, 2218 CrossRef PubMed.
- Y. Lampl, J. A. Zivin, M. Fisher, R. Lew, L. Welin, B. Dahlof, P. Borenstein, B. Andersson, J. Perez, C. Caparo, S. Ilic and U. Oron, Stroke, 2007, 38, 1843–1849 CrossRef PubMed.
- P. A. Lapchak, J. Wei and J. A. Zivin, Stroke, 2004, 35, 1985–1988 CrossRef PubMed.
- W. Chen, R. Chu, H. Li, T. Hua, H. Chen, R. Li, D. Zhou, S. Cao, S. Ye and H. Li, Mater. Adv., 2023, 4, 2918 RSC.
- J. Klohs, A. Wunder and K. Licha, Basic Res. Cardiol., 2008, 103, 144–151 CrossRef CAS PubMed.
- P. A. Lapchak, Ann. Med., 2010, 42, 576–586 CrossRef PubMed.
- J. Shao, M. Abdelghani, G. Shen, S. Cao, D. S. Williams and J. C. M. van Hest, ACS Nano, 2018, 12, 4877–4885 CrossRef CAS PubMed.
- L. H. Chang, E. Y. Chuang, T. M. Cheng, C. Lin, C. M. Shih, A. T. Wu, P. R. Jheng, H. Y. Lu, C. C. Shih and F. L. Mi, Acta Biomater., 2021, 134, 686–701 CrossRef CAS PubMed.
- S. Yao, C. He, P. Yuan, X. Xu, X. Zhou, L. Shen, Q. Hu, N. K. H. Slater, W. Sun, Y. Shen, H. Hu and J. Tang, Adv. Healthcare Mater., 2022, e2201981 Search PubMed.
- Y. Cheng, A. Cheng, Y. Jia, L. Yang, Y. Ning, L. Xu, Y. Zhong, Z. Zhuang, J. Guan, X. Zhang, Y. Lin, T. Zhou, X. Fan, J. Li, P. Liu, G. Yan and R. Wu, ACS Appl. Mater. Interfaces, 2021, 13, 56909–56922 CrossRef CAS PubMed.
- S. Zhang, B. Peng, Z. Chen, J. Yu, G. Deng, Y. Bao, C. Ma, F. Du, W. C. Sheu, W. T. Kimberly, J. M. Simard, D. Coman, Q. Chen, F. Hyder, J. Zhou and K. N. Sheth, Bioact. Mater., 2022, 16, 57–65 CrossRef CAS PubMed.
- R. Lemmens and G. K. Steinberg, Curr. Opin. Neurol., 2013, 26, 617–625 CrossRef CAS PubMed.
- Z. Ren, S. Sun, R. Sun, G. Cui, L. Hong, B. Rao, A. Li, Z. Yu, Q. Kan and Z. Mao, Adv. Mater., 2020, 32, e1906024 CrossRef PubMed.
- E. T. Chouchani, V. R. Pell, E. Gaude, D. Aksentijević, S. Y. Sundier, E. L. Robb, A. Logan, S. M. Nadtochiy, E. N. J. Ord, A. C. Smith, F. Eyassu, R. Shirley, C. H. Hu, A. J. Dare, A. M. James, S. Rogatti, R. C. Hartley, S. Eaton, A. S. H. Costa, P. S. Brookes, S. M. Davidson, M. R. Duchen, K. Saeb-Parsy, M. J. Shattock, A. J. Robinson, L. M. Work, C. Frezza, T. Krieg and M. P. Murphy, Nature, 2014, 515, 431–435 CrossRef CAS PubMed.
- M. Petro, H. Jaffer, J. Yang, S. Kabu, V. B. Morris and V. Labhasetwar, Biomaterials, 2016, 81, 169–180 CrossRef CAS PubMed.
- T. Zhao, W. Wu, L. Sui, Q. Huang, Y. Nan, J. Liu and K. Ai, Bioact. Mater., 2022, 7, 47–72 CrossRef CAS PubMed.
- L. Hu, Z. Cao, L. Ma, Z. Liu, G. Liao, J. Wang, S. Shen, D. Li and X. Yang, Biomaterials, 2019, 223, 119469 CrossRef CAS PubMed.
- N. K. Mondal, J. Behera, K. E. Kelly, A. K. George, P. K. Tyagi and N. Tyagi, Neurochem. Int., 2019, 122, 120–138 CrossRef CAS PubMed.
- A. Popa-Wagner, S. Mitran, S. Sivanesan, E. Chang and A. M. Buga, Oxid. Med. Cell. Longevity, 2013, 2013, 963520 Search PubMed.
- W. Chen and D. Li, Front. Chem., 2020, 8, 732 CrossRef CAS PubMed.
- E. H. Lo, T. Dalkara and M. A. Moskowitz, Nat. Rev. Neurosci., 2003, 4, 399–415 CrossRef CAS PubMed.
- M. A. Moskowitz, E. H. Lo and C. Iadecola, Neuron, 2010, 67, 181–198 CrossRef CAS PubMed.
- K. E. Broaders, S. Grandhe and J. M. J. Fréchet, J. Am. Chem. Soc., 2010, 133, 756–758 CrossRef PubMed.
- H.-Y. Hong, J. S. Choi, Y. J. Kim, H. Y. Lee, W. Kwak, J. Yoo, J.-T. Lee, T.-H. Kwon, I.-S. Kim, H.-S. Han and B.-H. Lee, J. Controlled Release, 2008, 131, 167–172 CrossRef CAS PubMed.
- J.
T. Gonçalves, S. T. Schafer and F. H. Gage, Cell, 2016, 167, 897–914 CrossRef PubMed.
- M. Faiz, N. Sachewsky, S. Gascon, K. W. Bang, C. M. Morshead and A. Nagy, Cell Stem Cell, 2015, 17, 624–634 CrossRef CAS PubMed.
- I. Kazanis, N. Gorenkova, J.-W. Zhao, R. J. M. Franklin, M. Modo and C. ffrench-Constant, Exp. Neurol., 2013, 248, 387–397 CrossRef PubMed.
- T. Zhao, T. Zhu, L. Xie, Y. Li, R. Xie, F. Xu, H. Tang and J. Zhu, Transl. Stroke Res., 2022, 13, 665–675 CrossRef PubMed.
- B. Furie and B. C. Furie, N. Engl. J. Med., 2008, 359, 938–949 CrossRef CAS PubMed.
- G. K. Hansson, N. Engl. J. Med., 2005, 352, 1685–1695 CrossRef CAS PubMed.
- J. Loscalzo, Pathophysiol. Haemostasis Thromb., 2002, 32, 359–360 CrossRef CAS PubMed.
- J. H. Park, D. Dehaini, J. Zhou, M. Holay, R. H. Fang and L. Zhang, Nanoscale Horiz., 2020, 5, 25–42 RSC.
- L. J. Rich and M. Seshadri, Radiology, 2015, 275, 110–118 CrossRef PubMed.
- K. Jansen, G. van Soest and A. F. van der Steen, Ultrasound Med. Biol., 2014, 40, 1037–1048 CrossRef PubMed.
- B. L. Chung, M. J. Toth, N. Kamaly, Y. J. Sei, J. Becraft, W. J. Mulder, Z. A. Fayad, O. C. Farokhzad, Y. Kim and R. Langer, Nano Today, 2015, 10, 759–776 CrossRef PubMed.
- A. Hannah, G. Luke, K. Wilson, K. Homan and S. Emelianov, ACS Nano, 2014, 8, 250–259 CrossRef CAS PubMed.
- N. J. Abbott, L. Ronnback and E. Hansson, Nat. Rev. Neurosci., 2006, 7, 41–53 CrossRef CAS PubMed.
- W. M. Pardridge, Mol. Interventions, 2003, 3, 90–105 CrossRef CAS PubMed , 190–151.
- S. Ohtsuki and T. Terasaki, Pharm. Res., 2007, 24, 1745–1758 CrossRef CAS PubMed.
- R. Jin, G. Yang and G. Li, Neurobiol. Dis., 2010, 38, 376–385 CrossRef CAS PubMed.
- P. M. George and G. K. Steinberg, Neuron, 2015, 87, 297–309 CrossRef CAS PubMed.
- X. Dong, Theranostics, 2018, 8, 1481–1493 CrossRef CAS PubMed.
- M. F. Bennewitz and W. M. Saltzman, Neurotherapeutics, 2009, 6, 323–336 CrossRef CAS PubMed.
- D. S. Jones and D. Q. M. Craig, J. Pharm. Pharmacol., 2004, 56, 1–2 CrossRef.
- A. Mistry, S. Stolnik and L. Illum, Int. J. Pharm., 2009, 379, 146–157 CrossRef CAS PubMed.
- B. V. Zlokovic, Neuron, 2008, 57, 178–201 CrossRef CAS PubMed.
- O. Kis, K. Robillard, G. N. Chan and R. Bendayan, Trends Pharmacol. Sci., 2010, 31, 22–35 CrossRef CAS PubMed.
- M. Simionescu, A. Gafencu and F. Antohe, Microsc. Res. Tech., 2002, 57, 269–288 CrossRef CAS PubMed.
- L. Crawford, J. Rosch and D. Putnam, J. Controlled Release, 2016, 240, 251–266 CrossRef CAS PubMed.
- R. S. Pandya, L. Mao, H. Zhou, S. Zhou, J. Zeng, A. J. Popp and X. Wang, Cent. Nerv. Syst. Agents Med. Chem., 2011, 11, 81–97 CrossRef CAS PubMed.
- I. Brasnjevic, H. W. Steinbusch, C. Schmitz and P. Martinez-Martinez, Prog. Neurobiol., 2009, 87, 212–251 CrossRef CAS PubMed.
- I. L. Colao, R. Corteling, D. Bracewell and I. Wall, Trends Mol. Med., 2018, 24, 242–256 CrossRef CAS PubMed.
Footnote |
† These authors contribute equally to this work. |
|
This journal is © The Royal Society of Chemistry 2023 |