DOI:
10.1039/D3CC00513E
(Feature Article)
Chem. Commun., 2023,
59, 6437-6452
Insights into desalination battery concepts: current challenges and future perspectives
Received
6th February 2023
, Accepted 8th March 2023
First published on 3rd May 2023
Abstract
Water plays an essential role in the development of society. However, the worldwide supply of drinking water is becoming a challenge that needs to be addressed in the future. In this review we focus on new electrochemical technologies based on the concept of desalination batteries (DBs) and which feature different desalination approaches based on battery-like technologies reported to date. Here, we use the state-of-the-art knowledge and the current developments in materials and electrochemical engineering to promote an innovative approach in the search of strategies for increasing ion removal from salty electrolytes and energy storage capability. The motivation behind the present review is to reinforce the knowledge of each group of DB-based methods focusing on their figures of merit (FOM). Accordingly, it aims to address DBs as a promising technology to face water remediation at low energy consumption using the following key-aspects: (1) DB basis/concept, history and comparison to other electrochemical-based technologies; (2) DB-based concepts proposed in the literature, focusing on providing their FOM as the core of this review; (3) limitations and future challenges and opportunities. Moreover, discussions regarding charging–discharging mechanisms, cell designs and current issues on operational modes are also provided.
Introduction
Energy and water resources are closely related and essential in all aspects of life. Water, in addition to being an irreplaceable daily requirement for people at all levels of human activity, specifically allows good economic development of a country if there is efficient management. Drinking water production processes and the management of irrigation systems play a fundamental role in society, but they also present problems of intensive energy consumption.1–3 In addition, water is required in more than 98% of the phases of energy production and electricity generation.4 The energy–water nexus has a considerable impact on two of the most alarming problems that human beings face in the 21st century: growing environmental issues (climate change) and the worldwide shortage of drinking water due to the increasing global water demand.4 By the year 2050, around 51% of the world's population (ca. of 5 billion people) could experience water scarcity, with 33% already under stress conditions, according to the Intergovernmental Panel on Climate Change (IPCC) and to the International Desalination Association (IDA).5–7 In the last 100 years, the consumption of drinking water in the world has increased six-fold, while the population has only tripled.8,9 One of the clearest examples of the water–energy nexus mentioned above arises: in most energy production processes, water consumption is necessary. Furthermore, water is required not only in the energy sector but also in other sectors such as food and in the production of everyday goods.4 Accordingly, better energy and water resource management along with the development of water treatment technologies with low environmental impact and energy consumption has become more essential than ever.1
In this context, desalinated water is an important water resource and thus, water scarcity is compensated by means of desalination.10 Currently, there are some 22
000 desalination plants worldwide and the cumulative installed desalination capacity has reached ca. 100 million m3 per day (35 billion m3 annually).11 The different desalination processes are usually classified according to the energy form required in their performance: (1) thermal energy (e.g. Multi-Effect Distillation, Thermal Vapor Compression or Multi-Stage Flash Evaporation); (2) mechanical energy (e.g. Reverse Osmosis, RO); and (3) electric power (e.g. Electrodialysis, ED). It should be noted that RO alone contributes 69% of the daily production of desalinated water in the world.10,12 However, RO presents a number of environmental drawbacks such as effluents produced associated with the chemical regeneration of membranes.13 In order to slow down surface scaling and biological fouling, RO membranes are periodically treated with chlorine, which is then released in the seawater.14 Because of their high amount of energy consumption, brackish and seawater desalination through such technologies has a high energy footprint and a consequent emission of greenhouse gases. Hence, there is a strong necessity for a secure and sustainable water supply, which requires the development of green technologies for water treatment.
Based on this, it is clear that novel approaches to classic desalination technologies (such as RO or MFD) are needed. In contrast to widespread desalination technologies that require high operating pressures and temperatures, electrochemical-based desalination technologies are based on the movement of ions (minority phase) with respect to the aqueous electrolyte (majority phase). Thus, electrochemical technologies are theoretically superior in terms of operation flexibility (e.g. they can also be applied to water softening, heavy metal removal or disinfestation)15 and maintenance costs to other traditional desalination methods.14 Consequently, different electrochemical based desalination techniques have been proposed:
- Electrodialysis, ED. ED is based on the migration of ions (Na+ and Cl−) to maintain the charge balance of the cell due to the presence of redox reactions (i.e., H2, O2 or Cl2 evolution) taking place at the electrodes. The higher the salinity of the feed, the larger the energy needed to produce freshwater. Therefore, ED is applied to brackish water desalination. Furthermore, it requires the use of ion exchange membranes (IEMs) and thus, the cell presents several chambers.16–19
- Electrochemically Mediated Seawater Desalination, EMD. EMD relies on Ion Concentration Polarization techniques20,21i.e. in the creation of a depletion zone at the junction of a branched microchannel with a bipolar electrode (local electric field gradient) that allows the separation of a seawater feed into brine and desalted water streams. The voltage difference permits the oxidation of Cl− into Cl2.22,23
- Capacitive Deionization, CDI. In CDI, desalination happens thanks to the formation of an electrical double layer (EDL) under the influence of an applied potential. Hence, it relies on the same energy storage/release basis as that of electrochemical double-layer capacitors (EDLCs).24–31 There are a number of variants of CDI technology aiming to promote the efficiency and desalination performance such as (1) Membrane CDI (MCDI) that includes the use of anionic (AEM) and cationic (CEM) exchange membranes in the CDI devices;32–36 (2) hybrid CDI systems (HCDI) in which one of the electrodes captures ions by battery-like materials;29,37–40 and (3) inverted CDI (iCDI) that provides highly selective separations, given by the surface modification.41
- Desalination Batteries, DB. The pioneering approach was based on two battery-like electrodes for capturing ions and desalting feedwater by a 4-step process, consequently resulting in simultaneous salt withdrawal and energy storage.42 Besides the first DB system, several concepts have been proposed in the literature recently: rocking-chair desalination,43–45 Dual Ion Desalination (DIDB) and faradaic Deionization (FDI),46–50 redox-flow DBs,51–53 and metal–air DBs.54–56 Moreover, DB-based systems designed not only to desalinate, but also to synthesise by-products have been also described.57,58 Their working principle is based on seawater batteries (SWB) in which desalination, and acid-alkali production57 or carbon capture58 occur as well. In the following sections, we will focus on the past and current scenario of Desalination Battery concepts reported in the literature.
What is a desalination battery?
Background on DBs.
In 2009 Brogioli proposed an innovative methodology for extracting and capturing the free energy created from the mixing of two streams with different salt contents (e.g. seawater and river water).59 His novel approach was based on entropic energy extraction and then storing this energy in an electrochemical-double layer (EDL) at the surface of electrodes. The entropy variation upon mixing two water streams of different salinity provides the foundation for the so-called capacitive mixing (CAPMIX) methods.60–65 In order to improve and overcome the challenges associated with the use of the electrode/electrolyte interface for energy harvesting, La Mantia et al. demonstrated energy extraction from a difference in salinity and its storage as electrochemical energy within the bulk crystal structure of the electrode's material (i.e. battery/faradaic electrodes in which the energy is held in chemical bonds).66 This approach of generating electrical energy was named Mixing Entropy Battery, also known as Battery Mixing (BattMix).61 Batteries for energy extraction due to water salinity differences established the basis for developing a new technology for water desalination. In 2012, the first concept of a Desalination Battery (DB) for the desalination of seawater was introduced and demonstrated.42 Instead of accumulating the salt at the electrode's interface, as in CAPMIX or CDI technologies, DBs store the charged ions in the bulk phase, thus allowing higher specific desalination rates and lower energy consumption, as well as a more stable desalination operation.
The original DB concept consists of a closed cycle based on four steps (see Fig. 1). Firstly, the electrodes in their fully charged state (i.e. without Na+ or Cl− within the bulk crystal structure) are immersed in seawater and discharged by applying a constant current. This promotes the ion's removal from the bulk solution and leads to energy release. Once the deionization/discharge step (Step 1) is completed, the freshwater solution is extracted and replaced with additional seawater. This water exchange increases the battery cell voltage (Step 2). At this point, electrodes are then charged and ions are released. Accordingly, the regeneration of electrodes/charging (Step 3) is related to the generation of a brine solution (concentrated solution). Finally, brine is replaced with seawater (Step 4) leaving the system ready for the next deionization cycle. The original design of this desalination device consisted of a cationic sodium insertion electrode (Na2−xMn5O10) and a chloride capturing anionic electrode (Ag/AgCl).42 It is also essential to highlight that the net energy consumption of DB is given by the integral along the cycle of the battery cell voltage (ΔE) with respect to the charge (q) (eqn (1)).
|  | (1) |
Hence, the net energy consumption is conditioned by the charging and discharging capacity of the electrodes and the variation in the potential difference involved in the electrolyte-exchanging steps (
i.e. by the electrolyte composition).
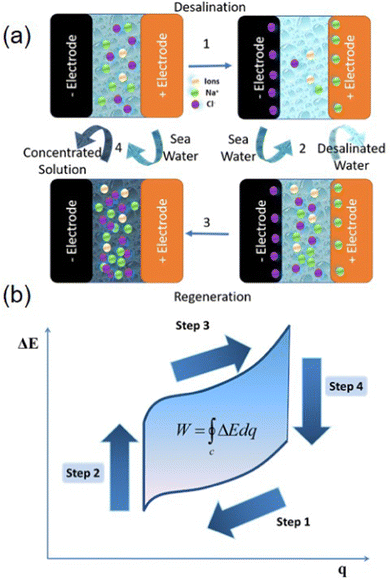 |
| Fig. 1 First Desalination Battery (DB) Concept. (a) Schematic representation of the working principle behind a complete cycle. (Step 1) desalination; (step 2) removal of the desalinated water and inlet of sea water; (step 3) discharge of Na+ and Cl− in seawater; (step 4) exchange to new seawater. (b) Typical form of a cycle of battery cell voltage (ΔE) vs. charge (q) in the DB, demonstrating the energy consumed. Adapted with permission.42 Copyright 2012, Copyright © 2012 American Chemical Society, published by American Chemical Society. | |
Having no need for high operating pressures or thermal energy inputs, most DBs are superior in terms of operation and maintenance costs compared to the other desalination technologies such as ED or RO. Furthermore, their higher electrode capacities in comparison to the CDI system opens the possibility to treat solutions with relatively high salinity and therefore, to go beyond brackish water desalination. For these reasons, DBs are strongly considered as versatile and promising technologies for seawater desalination as they can act as a multi-utility system,57,58 in which the energy storage phase can also be coupled to renewable energy sources.67–69
Latest research: next generation of DBs and performance evaluation.
However, some years passed between the 1st DB study and the next generation of DB concepts (Fig. 2). Recent progress in active materials, novel cell configuration and design development has boosted a renewed interest of the scientific community to overcome challenges concerning the progress of the DB.70–74 At this point, it is important to provide a definition for the desalination battery and answer the question what does the term ‘desalination battery’ entail?
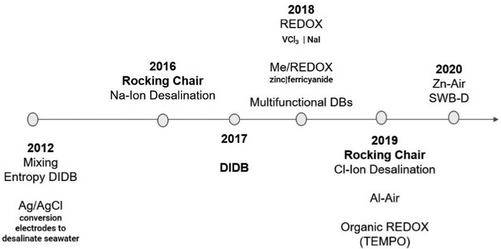 |
| Fig. 2 Timeline of the different DB-based methods. | |
A DB can be defined as a rechargeable battery based on the simultaneous transfer of cations (Na+) and/or anions (Cl−) from the electrolyte to the electrodes implying the (de)salination and the concurrent charging/discharging steps. Therefore, the main objective of a DB is to produce deionized water at a minimum net energy consumption. However, the desalination could be an energy demanding or a spontaneous process depending on the potential of the chosen electrodes/redox electrolytes.72 Considering that the Cl− uptake electrode is the anionic electrode and the one capturing Na is the cationic one, if the anionic electrode (chlorination process) has a higher potential than the cationic electrode (sodiation process), then the desalination step consumes energy and the salination produces energy. Conversely, if the cationic electrode is the positive one then the desalination releases energy and the salination is an energy demanding process. Keeping the redox potential difference at its minimum guarantees a low net energy consumption. For further details on the discussion of DBs versus desalination cells, we refer the reader to the perspective written by Nam et al.72
Electrochemical desalination metrics and indicators
Considering that several DB concepts have emerged, numerous performance metrics and indicators have been reported. Therefore, it is important to propose and use a unified definition of parameters that allow the standardization of figures of merit (FOM) for DB-based systems. The following publications discuss in detail how to evaluate accurately desalination performance metrics of different electrochemical desalination cells.74,75 Some considerations regarding the analysis of desalination metrics found in literature are briefly discussed below.
Salt removal performance metrics.
The salt concentration reduction (% of salt removal) is one of the most used metrics to indicate the desalinization performance. However, this parameter needs complementary data to provide insightful information of the electrode and/or device performance. For instance, operational parameters such as feedwater concentration, flow rate conditions and single-pass or batch operation mode or electrode configuration (active material mass) are conditions that greatly affect the amount of salt removed.
The analysis of the performance of electrochemical deionization devices is mostly based on the ion retention capacity in the electrodes. In CDI literature, it is called the salt adsorption capacity. Here, we prefer the general term desalination capacity or salt uptake capacity (SUC) that it is independent from the ion's removal mechanism. SUC is expressed as milligrams of salt removed (mostly, NaCl) divided by the mass of the electrodes (mgNaCl gT−1). It is of importance to report this indicator when the system is in equilibrium and to consider the total mass of both electrodes. For any particular cases, in which the aim is to report and discuss a specific ion removal by a novel electrode material, it might be useful to report the amount of the ion removed divided by the mass of the active material76 or by the electrode's volume.77 Moreover, this indicator could also be used to express the removal of cations or anions, instead of giving the total salt removal.11 It is therefore essential to strictly present the uptake capacity in such a clear and detailed way that facilitates the evaluation and comparison of the result to other electrochemical desalination systems.
Another widely used parameter is the average salt uptake rate (ASUR). Whereas the SUC gives information on the amount of salt removed by the electrodes, the ASUR refers to the salt removal rate (mg g−1 s−1). It is recommended to report this metric by considering the total cycle time (ΔtCycle): both the charge and the discharge times.27
It is worth mentioning that all these parameters are directly related to the measurement of ion removal. An appropriate tracking of ion concentration variation upon operation allows the precise evaluation of desalination performance. In most of the desalination studies, the ion concentration is determined with a conductivity-meter at the outlet of the desalination device. However, these measurements are greatly affected by any minor pH modification (e.g. charge unbalanced, secondary reactions, local pH changes). If measured conductivity values are not corrected, this would create a misguiding evaluation of the phenomena taking place in the system. Other techniques to measure ion concentration are Inductively Coupled Plasma (ICP) based techniques,11,42 Ion Chromatography (IC)78 or the use selective Na-ion and/or Cl-ion electrodes.79
Energy and electrochemical performance evaluation.
One of the distinguishing features of most electrochemical-based deionization approaches is the possibility to recover a fraction of the energy invested in the process. It then becomes necessary to indicate accurately if the energy consumption reported is the net value (i.e. through the whole cycle) or if it is calculated for the (de)salination step alone. The energy is normalized by the amount of salt removed or volume of water treated.
Regarding the electrochemical performance, the most common reported parameters are the charging and discharging specific capacities (mA h g−1). These parameters should not be directly interpreted as the ion removal capacity, but they might contribute to a preliminary evaluation of the potential desalination performance of a certain type of electrode material.
Direct correlation parameters between the charge and the ion removal.
The desalination efficiency (Λ) is a useful metric to assess the selectivity of the materials or devices towards the ions in solution. It is defined as the ratio between the charge associated with the variation in concentration measured and the charge involved in the process (eqn (2)). | 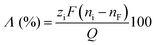 | (2) |
where zi is the valence state of the ion, F is the Faraday constant (96
485 C mol−1), ni and nF are the initial and final number of moles in the electrolyte, respectively, and Q is the total charge transferred during the desalination, including the one coming from parasitic reactions. In the literature, it is also reported as dynamic charge efficiency, current efficiency or charge efficiency. A comparable concept introduced in DB literature is the faradaic efficiencies for ion capturing that compares the charges passed to the electrode during ion removal and the actual change in ion concentration in the salt solution.76,79
In this feature article, we will discuss the different DB concepts and gather their main performance parameters previously described.† Accordingly, here we intend to place special emphasis on the figures of merit (FOM) of each group of techniques.
Different desalination battery concepts
As mentioned earlier, in the literature desalination battery (DB) means a device which reduces the salt content of the electrolyte using typical charge–discharge mechanisms and/or chemistries similar to those of batteries. Accordingly, the DB concepts can be classified into four main groups (Fig. 3): (i) Na-Ion Desalination (NID) and Chloride-Ion Desalination (CID) based on the rocking-chair storage mechanisms43,45,80–93 (Fig. 3(a and b)); (ii) the Dual-ion DB (DIDB) or faradaic Deionization (FDI) technologies concerning those electrochemical cells in which both Na+ and Cl− are simultaneously captured in battery-like electrodes42,47,49,50,76,78,79,94–101 (Fig. 3(c)); (iii) Redox-flow DB systems (RF-DB)51–53,67,68,102–106 (Fig. 3(d and e); and (iv) Metal Air DBs54–56,107,108 (Fig. 3(f and g)). Within the following paragraphs, we intend to explore their fundamentals, their development and highlight their strengths.
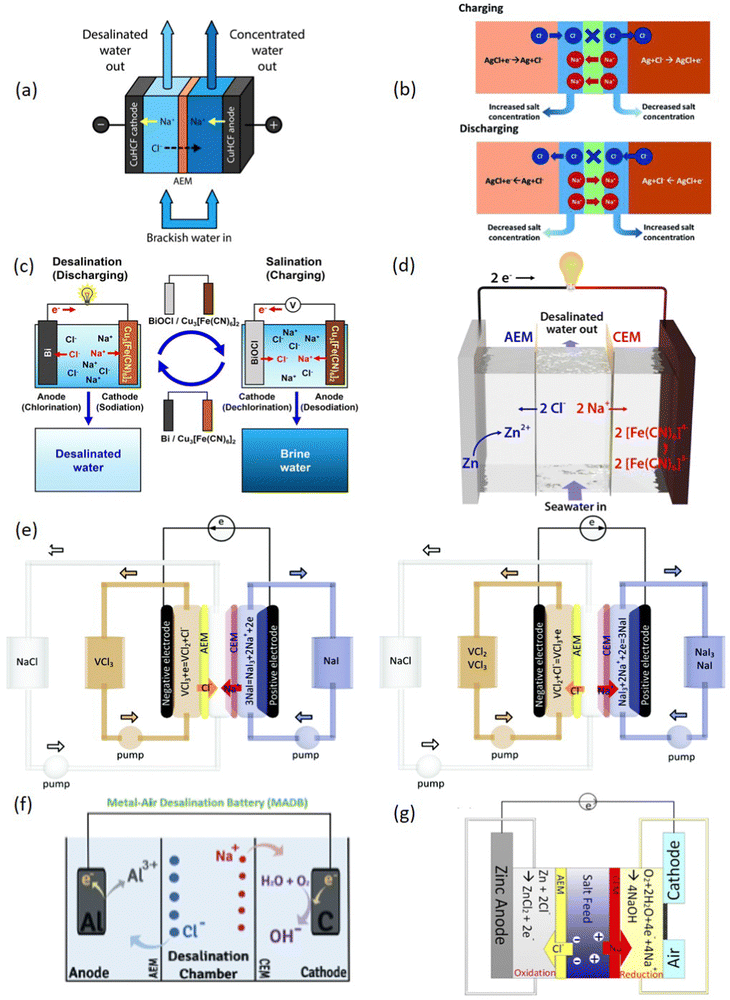 |
| Fig. 3 Different desalination batteries (DB) concepts classified according to their ion removal mechanism. Rocking-chair configuration: (a) Na-Ion Desalination. Reproduced with permission.87 Copyright © 2017 American Chemical Society, published by American Chemical Society; (b) Cl-Ion Desalination. Reproduced under the terms of the CC-BY license.47 Copyright 2018, The Authors, published by RSC. (c) Dual-Ion DB. Reproduced with permission.76 Copyright © 2019 American Chemical Society, published by American Chemical Society. Redox-based DBs: (d) Schematic illustration of a DB based on Zn/[Fe(CN)6]4−/3− Reproduced with permission.53 Copyright © 2019 American Chemical Society, published by American Chemical Society; (e) Schematic illustration of a DB based on VCl3/NaI. Reproduced with permission.51 Copyright 2018, RSC. Metal–air DBs: (f) Al–air DB. Reproduced with permission.54 Copyright 2019, published by Elsevier, and (g) Zn–air DB. Reproduced with permission.55 Copyright © 2020 American Chemical Society, published by American Chemical Society. | |
Rocking chair configuration: Na-ion Desalination (NID) and Cl-ion desalination (CID)
Thanks to the growing interest in Na-batteries as a promising alternative to Li-based batteries due to their electrochemical similarities (similar intercalation chemistry to Li) and the current limitations associated with Li ions (scarcity, limited distribution and thus, high cost of lithium resources),109 there has been an enormous development of active materials capable of storing Na+ electrochemically.110,111 There are three main groups of electrode materials for the (de)insertion of Na+ in aqueous electrolytes:112 (1) Metal oxides (e.g. MnO2 and Na0.44MnO2, V2O5),113–121 (2) Prussian Blue Analogues, PBA (e.g. copper and nickel hexacyanoferrates)122–128 and (3) alkali/transition metal phosphates (e.g. NaFePO4, Na2FeP2O7, or the NASICON-type, NaTi2(PO4)3).129–133 Between 2014–2016, metal-free hybrid seawater fuel cells and Na-air seawater batteries were also studied.134–138 The use of NaCl as a catholyte was explored to develop this kind of rechargeable aqueous Na batteries. The operation mode was based on a battery charging process in which Na+ ions, from seawater, were transfered to the negative electrode. The aim of these studies was therefore to explore the energy storage/release of seawater batteries (SWB) but not their desalination performance.
Inspired by these SWBs, a new desalination concept named Na-Ion Desalination (NID) was proposed in 2016 to desalinate seawater using symmetric Na-ion intercalation electrodes separated by anion-selective membranes (AEM) to isolate electrodes in the device (Fig. 3(a)).86 In short, this concept involves the same effect observed in rocking-chair Li-ion batteries: the salt depletion effect that implies the simultaneous accumulation and depletion of electrolyte-phase salt ions in opposing electrodes.139–143 The NID device operates during the charge process supplying Na+ ions from the cathode electrode (deintercalation) and capturing them in the anode (e.g.eqn (3)).
| Na2Ni[Fe2+(CN)] ↔ NaNi[Fe3+(CN)6] + Na+ + e− | (3) |
Due to the charge-imbalance, Cl
− ions migrate from the anode compartment to the cathodic one through the permselective membrane. Accordingly, the cell is divided into two compartments where there is the simultaneous production of freshwater and a concentrated solution. During the discharge, these processes are reversed. Therefore, there is a continuous production of freshwater. It is worth mentioning that, if both electrodes are based on the same active material (symmetrical cells), there is no energy stored in these devices but there is an energy input independent of the direction of the Na
+ movement.
72 To the best of our knowledge, considering both simulation and experimental studies, the following pairs of electrodes have been reported in the literature:
Na0,26MO2/Na0,44MO2,86 NaTi2(PO4)3/Na3Ti2(PO4)3,86
NaxNiHCF/Na1+xFeHCF,43,85 NaCuHCF/Na1+xCuHCF,87,88,144
NaNiHCF/Na2NiHCF,44,89,91–93,145 and NaTiV(PO4)3/Na2TiV(PO4)3.80
NID devices set the basis to explore the chloride-ion rocking-chair desalination mechanism. To accomplish the overall desalination, Cl− ions shuttle from one electrode to the other whereas the Na+ ions diffuse across a cation-exchange membrane (CEM) to maintain the charge-balancing of the aqueous media (Fig. 3(b)). In the same way as NID cells, the CID device is divided into two compartments with the resulting diluted and concentrated streams. However, in comparison to NID, there are less electroactive materials capable of removing Cl− ions selectively and reversely from aqueous media. To date, most conversion reactions based on Ag/AgCl45,81 or Bi-based systems82,83 have been reported (eqn (4) and (5)).
| Bi + Bi2O3 + 3Cl− ↔ 3BiOCl + 3e− | (5) |
There are several advantages that are the reason why Ag/AgCl conversion reaction (
eqn (4)) is explored as a favourable process to Cl
− capturing: their stability in aqueous electrolytes, the low solubility and their stability under potential among others. But silver-based electrodes present some substantial disadvantages of using Ag/AgCl systems: the considerable high price of Ag, the toxicity of nanoparticles of Ag and dissolved Ag
+,
146 the limited electronic conductivity of the AgCl and the kinetic limitation of the subsurface AgCl conversion to Ag,
147,148 concurrent with the formation of the silver oxide (side reaction).
149 It was also highlighted that due to the insulating nature of AgCl, an overpotential was identified during the Cl
− uptake that was taking place by the Ag. Additionally, a percentage of Ag was found in the AgCl after cycling, as an indication of the incomplete AgCl/Ag reaction conversion. Therefore, ions tend to react just at the surface of the AgCl, reducing the experimental capacity recorded. Additionally, the Ag foil becomes porous due to the conversion reaction changing its mechanical properties.
Despite the drawbacks presented in the electrodes based on Ag/AgCl, this conversion reaction has stood out as the most used for Cl− capture/release through the years, representing most of the Cl− capturing active material reported in desalination studies. The use of Ag was justified due to the lack of stable and insoluble electrode materials in aqueous media, the reasonable reversibility of the Ag/AgCl conversion reaction and the significant theoretical specific capacity of the system (250 mA h g−1). It was not until 2017 when an alternative conversion reaction was proposed to uptake Cl−: Bi/BiOCl (eqn (5)). Chen et al. introduced an aqueous rechargeable Chloride Ion Battery (CIB) comprising commercial BiOCl and silver active materials for the anode and cathode, respectively, in an aqueous 1 M NaCl electrolyte solution.150 This promising early work on aqueous CIB paved the way for the study of metal oxychlorides as battery-like electrodes for selective Cl− uptake in desalination devices.47 Recently, these Bi-based electrodes have been used to configure Cl-ion rocking chair devices.82,83 Despite the high theoretical capacity (385 mA h g−1), Bi-based desalination cells also suffer from irreversibility of the reactions involved and from significant morphology changes in the microstructure of the electrode (volume expansion, pulverization and grain coarsening) that imply a considerable degradation of the cell performance.
Fig. 4 gathers FOM of rocking-chair desalination cells. Both NID and CID present an increase in the ASUR at high SUR values (Fig. 4(a)). CID shows a better uptake performance (>80 mgNaCl gT−1 and >0.05 mgNaCl gT−1 s−1) probably due to the higher specific capacity of the Cl− capturing electrodes. In these systems, desalination efficiency results are coupled to higher SUC (Fig. 4(b)). While such results demonstrate the importance of guaranteeing the energy efficiency of the cell, the salt removal results reveal reasonably high values (>50%) but in most of the cases, at the cost of net energy consumption (Fig. 4(c)). As expected, thanks to the improvement of diffusion phenomena, the energy demand is reduced significantly when the feed concentration increases from 10 to 100 mM reaching an average value of ca. 0.07 W h gNaCl−1 for seawater and brine salinities (Fig. 4(d)).
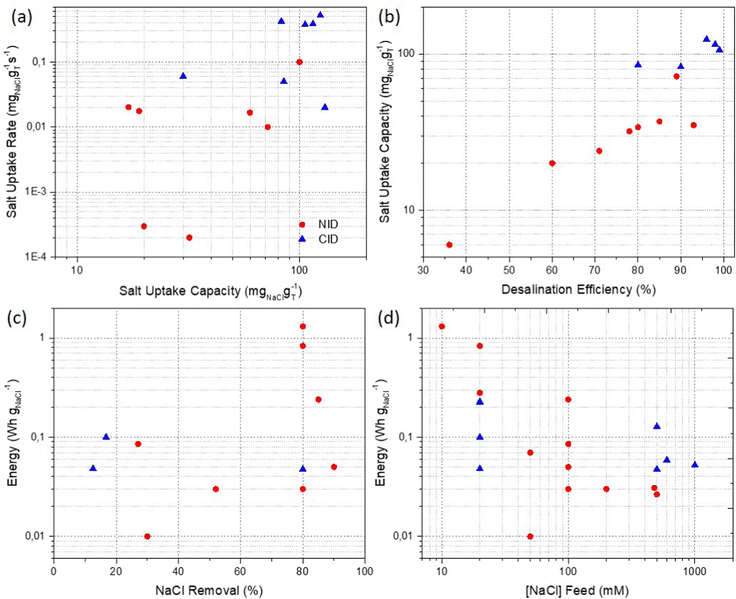 |
| Fig. 4 FOM of NID (red circles) and CID (blue triangles) methods. (a) SUR vs. SUC; energy consumption (b) vs. NaCl concentration of the feed solution and (c) vs. NaCl removal; (d) SUC vs. desalination efficiency of the feed solution and (c) vs. final Li concentration of the recovery. | |
Dual-ion desalination batteries (DIDB)
When the former two groups of electrodes (i.e., Na+ selective electrodes and the Cl− conversion electrodes) are paired together in a device, the working principle is similar to the one in a dual-ion battery (Fig. 3(c)). Accordingly, simultaneous battery-like capture/release of both Na+ and Cl− in the electrodes producing the (de)salination of the feed is proposed. In the literature, these dual-ion desalination batteries (DIDB) can be found as Dual-ion Deionization electrochemical deionization (DEDI) and faradaic Deionization (FDI).46–50 The starting point of DIDBs is to tackle the limitations of CDI technology related to its inherent electrosorption mechanism based on EDLCs: low ion removal and intensive energy consumption when treating high-concentration solutions.28
As described above, cationic electrodes have been developed to enhance energy storing properties thanks to the increasing interest in Na-Ion batteries. Compared with the variety of cation insertion materials, relatively few materials have been identified which incorporate anions under applied potential. To the best of our knowledge, the following DIDBs have been reported to date: NaxMnO2/Ag,42,46,78,100 Na3V2(PO4)2F3/Ag,151 NaTi2(PO4)3/Ag,49,94,101 NaTi2(PO4)3/Bi,79,152 Cu3HCF/Bi,76 NaxMnO2/BiOCl,47 symmetric 2D layered MoS2,50 NiHCF/Ag,95,96 NiHCF/redox-polymer,97 CuHCF/PPy,153 and NaxMnO2/PPyr-Ti.98
Most DIDB cell designs include the use of ion exchange membranes that increase the ion selectivity.78,80,86 Another important strategy to improve the cell performance is to provide an adequate mass balance in these devices. In this regard, it must be considered not only the different theoretical capacity of the electrodes in aqueous electrolyte (e.g. NMO 40–45 mA h g−1 and BiOCl 92 mA h g−1), but also the limited sub-surface conversion reaction in Cl− uptake electrodes. It is estimated that ca. 50% of the Bi is electrochemically active in 0.6 M NaCl.79 Furthermore, the expansion/contraction of the conversion electrodes as a result of the phase transformation must be analysed for an optimal design of the cell.
An alternative type of electrodes that may overcome the former drawbacks are those based on insertion materials such as transition metal carbides (MXenes) or transition metal dichalcogenides (TMDCs) and they have also been studied in DIDBs. MXenes are 2D nanomaterials with the general formula Mn+1XnTx where M is the transition metal, X is carbon and/or nitrogen and Tx is the surface functional group (e.g. Ti3C2Tx). Shifting their point of zero charge, these materials could be used not only for Na+ removal but also, to capture Cl−via intercalation within their 2D layered structure.154–156
TMDCs are also 2D materials typically with a MX2 (X–M–X) stoichiometry in a hexagonal lattice, where M is the transition metal and X is the chalcogen (e.g. MoS2).157–159 Additionally, TMDs show a molecular sensing capability due to their high surface volume ratio and availability of reactive sites. Among these properties, one of the main characteristics of TMDs is their interlayer spacing for energy storage application.160–162 In the field of blue energy and electrochemically assisted water desalination, there has been increasing interest in these layered materials.50,163 It is important to note that these materials show enhanced desalination properties via Na+/Cl− removal depending on their surface modification for example, the presence of negative charges due to the creation of defects on the surface increases the electrostatic attraction of Na+ ions.164,165 Keeping in mind that these materials present a relatively low specific surface area (ca. 20 m2 g−1), it is reasonable to assume that the ion removal mechanism occurs via faradic intercalation where both capacitance surface and diffusion-controlled processes play an important role.50 In summary, MoS2-based electrodes appeared promising in anion intercalation materials, but their chemical surface charge must be controlled.
Lastly, redox polymers have been paired with cation intercalation electrodes97,98,153 or with surface modified carbons.166 In this configuration, the Cl− ion is captured by a charge compensation mechanism.
Our FOM analysis for DIDBs (Fig. 5) shows that the ASUC is kept at a significant value (>0.03 mgNaCl gT−1 s−1) without compromising the SUC of this system (>40 mgNaCl gT−1). It is important to note that better desalination performance results are obtained when the cell comprises the use of IEMs (Fig. 5(a)). In general, an increase in the salt removal capacity was observed with the increment in the salt concentration feed water (Fig. 5(b)), though mostly results from brackish water studies have been reported. We have seen that this dependence is greatly affected by the pair of electrodes chosen and the operational modes selected. On the one hand, there is a direct correlation between the SUC and the desalination efficiency: increasing from 20 to 70 mgNaCl gT−1 for desalination efficiencies higher than 90%. As predicted, this exponential increase is at the cost of a larger energy input (Fig. 5(c)). On the other hand, in the conversion reactions used in DIDBs, releasing the Cl− is kinetically limited and requires an overpotential. High salinities of the feed favours kinetics lowering the net energy consumption and enhancing the desalination efficiency. As expected, higher net energy consumption is needed when the feed solution is below 100 mM (Fig. 5(d)).
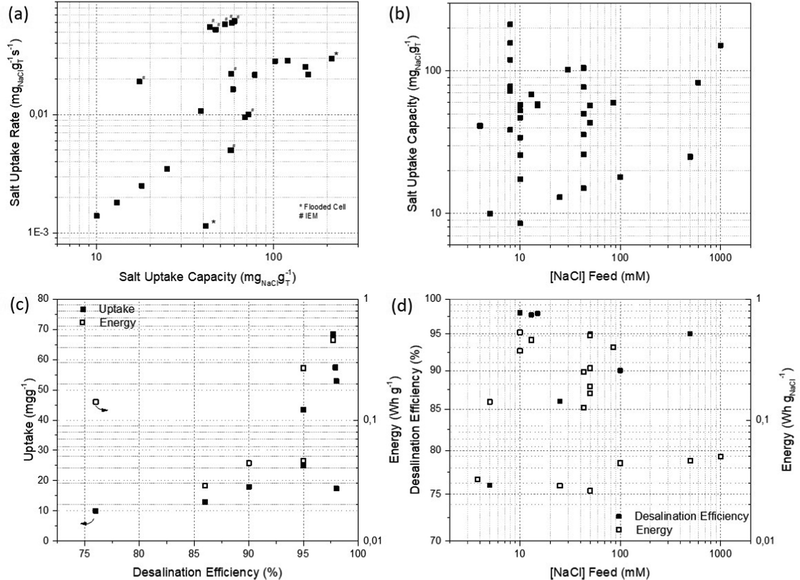 |
| Fig. 5 FOM of DIDB methods. (a) SUR vs. SUC; (b) SUC vs. NaCl concentration of the feed solution, (c) SUC and energy consumption vs. Desalination efficiency; (d) desalination efficiency and energy consumption vs. NaCl concentration of the feed solution. | |
Redox-based desalination batteries (ReDB)
Redox flow batteries (RFBs) have gained increasing attention due to their ability to store large amounts of electrical energy by using redox couples dissolved in electrolytes. In short, the RFB cell requires two electrolytes (catholyte or anolyte where the active cathode and anode materials are dissolved, respectively) and a separator. The energy is delivered by these electrolytes and stored in separated tanks thus, for charging/discharging the electrolytes are pumped into a stack, therefore, enabling the power and capacity of the battery to be scaled independently from each other.167–170 Redox-active compounds could be also a promising DB approach to decouple the desalination performance from the electrode's capacity. In the literature, redox mediators have been also demonstrated for electrochemically-mediated desalination. However, their implementation as desalination technologies requires several considerations such as electrode concept redefinition and different cell design concepts (Fig. 3(d and e)).
The desalination mechanism in these redox-based DBs (ReDB) relies on the transport of Na and Cl ions from the feed channel through IEMs to compensate the redox reactions taking place at the catholyte and anolyte compartments. The selection of the redox couple implies that the desalination could be an energy consuming or releasing process. In this fashion, the simplest ReDB cell design requires the use of three compartments: two for the electrolytes (i.e. anolyte and catholyte) and a central chamber where the salt solution to be desalted flows. And thus, the cell needs the incorporation of an AEM and a CEM to perform. It is worth mentioning here that ReDBs can also be understood as ED desalination cells in which there is energy storage thanks to the use of different redox reactions than O2 and H2 evolution. Accordingly, ReDBs studies are generally described as electrochemical redox desalination methods in the literature.51,53,67,104,106
By seeking the widespread use of RFBs into DBs systems to enhance their performance and versatility, some studies have been published recently. To the best of our knowledge, the first publications where redox electrolytes were used to desalinate were published in 2018. Hou et al. proposed a proof-of-concept of a redox flow battery desalination using VCl3/NaI aqueous electrolytes (eqn (6), overall reaction)51 and Desai et al. demonstrated a hybrid flow ReDB based on Zn/ferricyanide (eqn (7) overall reaction).53
| VCl3 + 3NaI ↔ 2VCl2 + 2NaCl | (6) |
| Zn + 2K3[Fe(CN)6] + 2NaCl + 2e− ↔ ZnCl2 + 2K3Na[Fe(CN)6] | (7) |
We note that organic redox flow DBs are the most widely studied. To the best of our knowledge, these are the redox molecules studied in ReDBs: Ferricyanide, 1,1'-bis[3-(trimethylammonio)propyl]ferrocene dichloride (BTMAP-Fc), 4-hydroxy-2,2,6,6-tetramethylpiperidin-1-oxyl (TEMPO), 4-hydroxy-TEMPO (TEMPOL), riboflavin-5′-phosphate sodium salt dihydrate (FMN-Na) and methyl viologen (MV). These are the redox couples used for electrochemical desalination concepts:
VCl3/NaI,51 Zn/[Fe(CN)6]4−/3−,53 Zn/Br(3)−,171 Zn/I(3)−,69 TEMPO/TEMPO+,105 TEMPOL/TEMPOL+,67 MV+/MV2+,106
[Fe(CN)6]4−/[Fe(CN)6]3−,68,102,103,172,173 [Fe(CN)6]4−/3/[BTMAP-Fc]2+/3+,104 and FMN-Na2/FMN-Na.174
Recently, a continuous desalination cell based on a dual-Zn device was demonstrated.84 The desalination mechanism relies on Cl− diffusion to compensate the charge-unbalance of the cell due to the oxidation/reduction of Zn in ZnCl2. The cell design of this method comprised the use of two AEMs and one CEM thus, the cell was divided into four compartments: one for the feed stream, one of the desalted one and two more for the ZnCl2 electrolyte.
When reviewing the literature, the salt removal showed by ReDBs configurations is significantly high, being >80% at concentrations typical for brackish to even brine solutions (Fig. 6(a)). This is likely due to the fact that the desalination performance of this device is independent of the capacity of the electrodes. Complementary, the impact of the salt concentration feed on the overall cell performance must be considered. The increment in concentration might induce polarization problems thus, it is essential to control the salt concentration level of the multiple compartments during the operation. For most of the feed salinities, the energy demand is <1 W h gNaCl−1 and in most cases the desalination efficiency is >90% (Fig. 6(b)). However, in ReDB devices, a significant polarization effect has been reported as the current density increases. This effect leads to a salt removal and desalination efficiency decrease and to the increase of energy consumption (Fig. 6(c)). Lastly, an overall increase in the energy input is required to increase the productivity (Fig. 6(d)). Note that productivity is the general term used in the literature for the amount of salt removed per unit area of the membrane in the unit time.
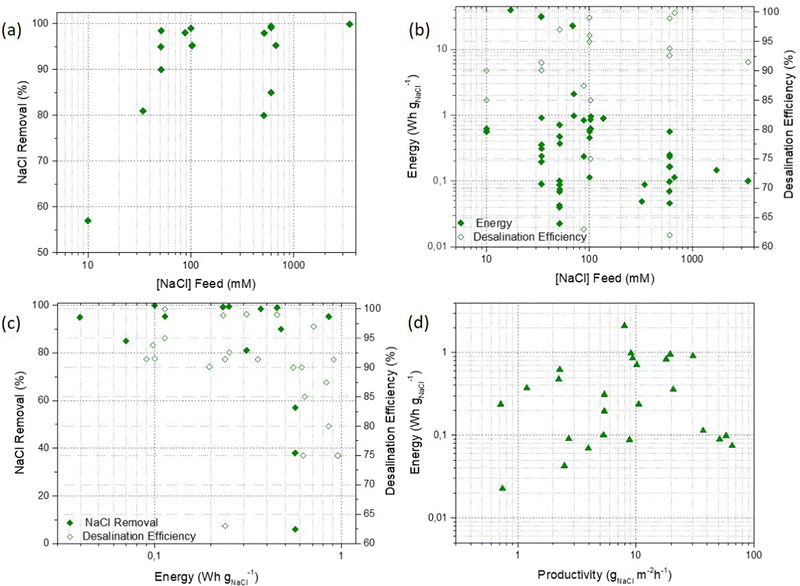 |
| Fig. 6 FOM of ReDB methods. (a) NaCl removal. (b) Energy consumption and desalination efficiency vs. NaCl concentration of the feed solution. (c) NaCl removal and desalination efficiency vs. Energy consumption. (d) Energy consumption vs. productivity. | |
To conclude, we want to mention here the desalination based on seawater batteries (SWB) as their mechanism also relies on the transport of Na and Cl ions from the feed channel through IEMs to compensate the redox reactions taking place at the catholyte and anolyte compartments (Fig. 7). As stated in the Na-ion desalination discussion, the original use of seawater batteries had the purpose of harvesting energy.134–138 However, since 2020, their desalination performance has been also explored in the so-called seawater desalination concept (SWB-D).175–180 For further details, we refer the reader to the latest work of Arnold et al. in which both the energy storage and desalination performance of seawater batteries are recently reviewed.181
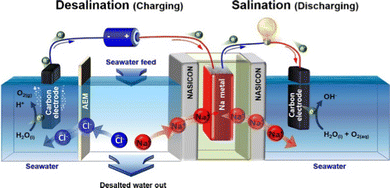 |
| Fig. 7 Seawater battery desalination (SWB-D). Reproduced with permission.176 Copyright 2020, published by Elsevier. | |
Metal–air desalination (MeDB)
One of the most novel electrochemical desalination concepts that appeared in the literature is based on the metal–air battery mechanism (Fig. 3(f and g)).182–186
The combination of metal–air batteries and desalination (MeDB) has been explored using Al–air DB54,107 and Zn–air concepts DB (eqn (8)and (9) overall reaction).55,56,108
| Zn + 2Cl− → ZnCl2 + 2e− | (8) |
| O2 + 2H2O + 4e− → 4OH− | (9) |
MeDB designs require at least the use of three chambers: metal/anolyte chamber, central chamber with the feed stream and the air cathode compartment. Thus, both CEM and AEM membranes are used in MeDBs.
In Fig. 8, preliminary FOMs based on the studies reported are shown. It seems that the energy consumption remains on average around 0.5 W h gNaCl−1 for brackish and seawater salinities and for different salt removal values and several desalination efficiencies. The latter parameter shows a decrease with the feed concentration. Metal–air batteries have gained global attention in recent years. Nevertheless, the FOM here discussed must be regarded as preliminary as they gather data from 5 publications.
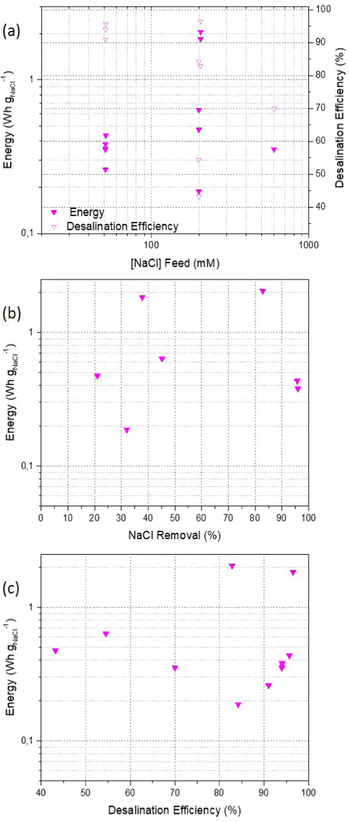 |
| Fig. 8 FOM of MeDB methods. (a) Energy consumption and desalination efficiency vs. NaCl concentration of the feed solution; (b) energy consumption vs. NaCl removal; (c) energy consumption vs. desalination efficiency. | |
In summary, the desalination performance results strongly depend on separation conditions such as cell design, feedwater concentration, electrode configuration among others. Thus, there is a compelling need of providing comparable descriptors/metrics of energy-water performance along with environmental information as detailed as possible. Considering reported results not only on DBs studies but also on other electrochemical-based water treatment technologies such as CDI, we refer the reader to the following publications for more comprehensive discussion on desalination performance metrics.27,75 In Table 1 we summarized experimental conditions and the main results of publications from different DB-methods.
Table 1 Summary of the main performance parameters of desalination battery techniques reported in the literature
Technology |
Electrodes |
Reactor typea Op. mode |
Membranesb cell design |
Feed salinity (mM NaCl) |
Salt removal (%) |
NaCl uptake capacity (mg NaClgT−1) |
NaCl uptake rate (mg NaCl gT−1 s−1) |
Desalination efficiency (%) |
Energy (W h gNaCl−1) |
Reactor type: flooded cell (FC); flow-by (FB); flow-through (FT) operational mode: constant voltage (CV); constant current (CC).
Ionic Exchange Membranes (IEMs); anionic exchange membranes (AEMs); cationic exchange membranes (CEMs).
Symm.-: symmetric electrodes (i.e., same electrode used as the cathode/anode or catholyte/anolyte).
g m−2 h−1. N.A. Not available. N.D. Not enough data reported on this parameter. |
Rocking NID |
Na0,26MO2/Na0,44MO286 |
FB44,87 FT88,187 CC |
AEM multichannel |
10–500 |
27–85 |
20–40 |
0.010–0.020 |
60–85 |
0.02–0.80 |
NaTi2(PO4)3/Na3Ti2(PO4)386 |
NaxNiHCF/Na1+xFeHCF43,85 |
NaCuHCF/Na1+xCuHCF87,88,144 |
NaNiHCF/Na2NiHCF44,89–93,145 |
NaTiV(PO4)3/Na2TiV(PO4)380 |
Rocking CID |
Ag/AgCl45,81 Bi-based systems82,83 |
FB45,83 FT82 CC |
CEM multichannel |
20 |
17–80 |
50–110 |
0.050–0.400 |
>80 |
0.05–0.30 |
500–1000 |
Dual-ion DB |
NaxMnO2/Ag42,78,99,100 NaTi2(PO4)3/Bi79,152 |
FC76,79 FB46,49,95 FT100 CC, CV98,100 |
Not required with IEMs,78,80,86 multichannel95,96 |
<10 15–100 < 1000 |
N.D. |
15–150 |
0.002–0.055 |
>75 |
0.05–0.40 |
Cu3HCF/Bi76 NaxMnO2/BiOCl47 |
Symm.-c MoS250 NaTi2(PO4)3/Ag49,94,101 |
Na3V2(PO4)2F3/Ag151 NiHCF/Ag95,96 |
NiHCF/redox-polymer97 CuHCF/PPy,153 |
NaxMnO2/PPy-Ti98 CuHCF/PPy153 |
Redox-DB |
VCl3/NaI.51 Zn/[Fe(CN)6]4−/3−53 Zn/Br(3)−171 |
FB CC & CV102,187 |
IEMs multichannel |
30–100 300–600 |
50–95 |
N.A. |
5–50d |
85–95 |
0.04–1.00 |
Zn/I(3)−69 |
Symm.-[Fe(CN)6]4−[Fe(CN)6]3–68,102,103,172,173,188 |
[Fe(CN)6]4−/3−/[BTMAP-Fc]2+/3+104 |
Symm.-TEMPOL/TEMPOL+:67 |
Symm.-TEMPO/TEMPO+.105 |
Symm.-FMN-Na2/FMN-Na174 |
Symm.-MV+/MV2+106 |
Dual Zn battery84 |
SWB-D57,58,175–180 |
Me-Air DB |
Al–air54,107 Zn–air55,56,108 |
FB CC |
IEMs multichannel |
50, 200 |
30–50 |
N.A. |
5–20d |
70–90 |
0.20–0.70 |
Challenges and perspectives
We have identified three main key-points to understand the past and current limitations for an extensive application of DB-based concepts.
Electrode materials synthesis and cell designs
Novel electrochemical-based desalination devices such as DBs need to be designed to show a relatively low energy consumption using not only Na+ insertion electrodes, but also developing innovative materials for their use in anionic electrodes. We have perceived that there is a lack of development on anion capturing electrodes to fully exploit novel desalination methods based on electrochemistry. Therefore, the research and development of Cl− insertion electrodes are ongoing scientific challenges. Our literature assessment on Cl-capturing electrodes has shown that most articles are focused on Ag/AgCl electrodes, probably due to the increasing concern of developing novel active materials and cell configurations to capture Cl ions over the last few years. In studies in which a novel Na+ capturing electrode is proposed and evaluated, the Cl− electrode is usually based on Ag/AgCl conversion reaction. The targeted chemical and physical features of the DBs electrodes, working in a sea water concentration range, are: (i) desalination through selective Na+/Cl− capturing electrode, (ii) high cyclability (reversible ion removal/release, physicochemical stability), and (iii) an accurate selection of synthesis routes and an optimal cell design configuration.
It is worth mentioning that this feature article does not aim to fully discuss active electrode materials used in DB-based methods. For further details on charge-transfer materials and redox electrolytes, we refer the reader to the review of Srimuk et al.74 However, here we stress the importance of their electrochemical stability, electronic conductivity, and electrolyte/electrode compatibility. The electrolyte/electrode interface of these materials might be a key-parameter to enhance the desalination performance in terms of ion removal providing larger interfacial areas of the porous structure. This facilitates the ion diffusion maximizing the conversion rate. The nanoparticulated morphology of the active materials might also be critical to increase the interface electrode/electrolyte and cycling stability by preventing the agglomeration.
Concerning the cell design, except for the DIDB cell, the other three DB concepts intrinsically require the use of IEMs. Indeed, this fact gives an idea of the importance of membranes in the further development if DB concepts. IEMs allow the desalination performance to increase by means of favouring the ion's removal and even more importantly, they open the possibility to tap new and promising DBs concepts such as the ones based on redox couples. In all DB concepts, there is no need for high operating pressures such as the ones used in RO. However, the incorporation of membranes might involve other drawbacks such as higher costs and maintenance (i.e. membranes are prone to fouling problems and surface scaling), a larger pressure drop in flow-through configurations, an increase in the probability of leakages from the pumping system or the loss of performance due to their chemical decomposition or the electrolyte crossover through the membranes. Hence, distinct advantages could be achieved by the DIDB method: (i) reduction of ohmic drop and cross-over effects because no membranes are strictly required; (ii) simplicity of the system that presents one compartment thanks to the use of a single electrolyte (seawater to be desalinated).
Energy-intensive consumption and operational modes
In the field of electrochemical-based desalination, the focus of the development in battery-based electrodes is to broaden the salinity range of feed water without sacrificing energy efficiency or desalination performance. Electrochemical desalination technologies such as ED or CDI present constraints in desalting highly concentrated solutions at a low energy consumption.28 This is not the case for DB-based methods. However, to promote the widespread implementation of DBs, there is a compelling need to explore different operational modes to optimize the energy consumption and energy efficiency. It is needed to minimize the electrical resistances to improve the energy performance of these electrochemical-based desalination technologies. As for the research on the presence of side reactions and their influence in the energy efficiency of this group of desalination techniques, to the best of our knowledge, there is no study addressing this topic.
Regarding flow configurations, most DB device designs are based in flow-by (i.e. where the electrolyte flows in the electrode's gap) and little to none are based on flow-through conditions. Additionally, in ReDBs configuration, it might be needed to shed light into the performance loss described in the literature. It is required to increase the current density range in which these systems operate, to further explore the impact of variables such as flow rates and the optimization of the composition of the electrolytes (the anolyte, catholyte and feedwater), and how the efficiency is affected by the ions removed accumulation during the recirculation of the electrolytes. At this point, it is important to highlight that in those cell designs including several compartments (i.e. rocking DB, ReDBs, MeDB), the efficiency loss due to backward diffusion depends on the difference between the bulk concentration of desalination and electrolyte chambers. The concentration in the redox reservoirs should be wisely chosen in order to avoid ion diffusion to the desalination chamber and thus, minimizing polarization problems.
Moreover, the energy consumption associated with the pumping requirements of DBs might be considered in the total energy input to perform the desalination.
Scaling-up viability and niche market scenarios
DB based concepts are currently at the laboratory scale and thus, there is a broad range of possibilities to explore and advance both the design and the scaling-up viability of DB devices. Accordingly, it might be expected that in the coming years this technology will face challenges associated with the development towards the pilot scale and to the scalability processes: electrode's scaling up and their configuration, the increment in the volume of salt water treated, control system tool. In ReDBs and MeDBs, particular attention should be paid to challenges such as the volumes of catholyte and anolyte required, their chemical decomposition, the electrolyte crossover through the membrane(s) or the leakage from the pumping system upon cycling.
Additionally, it is interesting to consider applying the DB basis to the selective removal of ions of interest such as Li+.
Conclusions
Over the last 10 years, interest in electrochemical-based desalination devices has noticeably grown. DBs are strongly considered as a versatile technology for brackish and seawater desalination and could act also as multifunctional systems. In the literature, past years have witnessed a considerable increase of studies on different DB approaches. Lately, the concept of a DB device has been connected to different battery-like ion storage. This feature article proposes an analysis of the figures of merit (FOM) gathering main results reported from the following DB-based methods: Dual-Ion DBs (DIDB), rocking chair DBs (both NID and CID), redox-based DBs (ReDB) and metal–air DBs (MeDB).
We have found that depending on the desalination concept, there are some performance metrics that are not provided in the discussion. Moreover, the experimental conditions are often not specified in detail, hindering a proper evaluation of the metrics and reproducibility of the experiments. This complicates the assessment and evaluation among the different DB concepts found in the literature and traditional desalination methods such as RO or MFD. Furthermore, parameters such as water recovery or the selectivity towards Na+ and/or Cl− are not fully discussed in DB literature. The latter is mainly because there are no studies working with multi-ion solutions as the feed stream. There is also a lack of data providing information about cell resistance, cell dead volumes evaluation or pumping energy. Results regarding the production are still not reliable due to the low technology readiness level (TRL below 3) of these concepts. In this context, in order to assess if and which of the DB technologies will be able to perform sea water desalination at an industrial scale, a higher TRL should be reached. This requires further work, in particular at the pilot scale. Currently, considering TRL < 5, the redox-based DBs appear to have superior desalination capacities; however they require membranes. Nevertheless, thanks to their synergies with electrochemical devices with higher TRLs, DB-based methods could experience a further and fast development in a short-term scenario. It is likely that the economic evaluation on large industrial-scale production needs to compare the fabrication costs of materials (e.g., electrode active materials, membranes and redox electrolytes) and devices as well as the operational costs (e.g., electrolytes pumping, maintenance, net energy consumption) to the desalination performance output (e.g., %NaCl removed, desalination rate and efficiency).
Encouragingly, DB devices have shown promising desalination results not only in terms of desalination capacity (>70 mg NaCl gT−1), but also regarding their efficiency (Λ > 85%) and low net energy consumptions (<0.1 W h gNaCl−1) even in seawater (>500 mM NaCl). In this context, the scientific community moves towards the full development of DB methodologies. Fostering the development of both the electrodes (active materials and the redox couples) and the membranes is a priority to face the challenges associated with the evolution/progress on most DBs.
Author contributions
C. Santos: conceptualization, funding acquisition, investigation, visualization, writing-original draft. F. La Mantia: conceptualization, funding acquisition, supervision, writing-review & editing.
Conflicts of interest
There are no conflicts to declare.
Acknowledgements
C. S. acknowledges funding from the European Union's Horizon 2020 research and innovation program under the Marie Skłodowska-Curie Individual Fellowship. Grant Agreement 84062 (REDEBA Project).
Notes and references
- UNESCO, The United Nations world water development report 2019: leaving no one behind, 2019.
- E. Global Water Institute. Hameeteman, ns Global Water Institute Future Water (In)Security: Facts, Figures, and Predictions.
-
P. Quentin Grafton,R. Wyrwoll, C. White and D. Allendes, Global Water: Issues and Insights.
-
UNESCO, The United Nations world water development report 2020: water and climate change, 2020 Search PubMed.
- United Nations, World Population Prospects 2019.
- The Intergovernmental Panel on Climate Change, IPCC Reports.
- International Desalination Association, The IDA Water Security Handbook 2019–2020.
- J. Chenoweth, Desalination, 2008, 229, 245–256 CrossRef CAS.
- Q. Schiermeier, Nature, 2008, 452, 260–261 CrossRef CAS PubMed.
-
UNESCO, United Nations: The United Nations World Water Development Report 2021: Valuing Water, 2021.
- International Desalination Association, Desalination by the numbers, https://idadesal.org/(accessed: January 2023).
- E. Jones, M. Qadir, M. T. H. Van Vliet, V. Smakhtin and S. Kang, Sci. Total Environ, 2019, 657, 1343–1356 CrossRef CAS PubMed.
- M. Qin, A. Deshmukh, R. Epsztein, S. K. Patel, O. M. Owoseni, W. S. Walker and M. Elimelech, Desalination, 2019, 455, 100–114 CrossRef CAS.
- A. Subramani and J. G. Jacangelo, Water Res., 2015, 75, 164–187 CrossRef CAS PubMed.
- J. Choi, P. Dorji, H. K. Shon and S. Hong, Desalination, 2019, 449, 118–130 CrossRef CAS.
- D. Nam and K. Choi, ACS Sustain. Chem. Eng., 2018, 6, 15455–15462 CrossRef CAS.
- R. K. McGovern, A. M. Weiner, L. Sun, C. G. Chambers, S. M. Zubair and J. H. Lienhard, Appl. Energy, 2014, 136, 649–661 CrossRef.
-
B. Van der Bruggen, Advances in electrodialysis for water treatment, Elsevier Ltd, 2015 Search PubMed.
- S. Al-Amshawee, M. Y. B. M. Yunus, A. A. M. Azoddein, D. G. Hassell, I. H. Dakhil and H. A. Hasan, Chem. Eng. J., 2020, 380, 122231 CrossRef CAS.
- S. J. Kim, S. H. Ko, K. H. Kang and J. Han, Nat. Nanotechnol., 2010, 5, 297–301 CrossRef CAS PubMed.
- T. A. Zangle, A. Mani and J. G. Santiago, Chem. Soc. Rev., 2010, 39, 1014–1035 RSC.
- K. N. Knust, D. Hlushkou, R. K. Anand, U. Tallarek and R. M. Crooks, Angew. Chem., Int. Ed., 2013, 52, 8107–8110 CrossRef CAS PubMed.
- K. N. Knust, D. Hlushkou, U. Tallarek and R. M. Crooks, ChemElectroChem, 2014, 1, 850–857 CrossRef CAS.
- Y. Oren, Desalination, 2008, 228, 10–29 CrossRef CAS.
- M. A. Anderson, A. L. Cudero and J. Palma, Electrochim. Acta, 2010, 55, 3845–3856 CrossRef CAS.
- S. Porada, R. Zhao, A. Van Der Wal, V. Presser and P. M. Biesheuvel, Prog. Mater. Sci., 2013, 58, 1388–1442 CrossRef CAS.
- M. E. Suss, S. Porada, X. Sun, P. M. Biesheuvel, J. Yoon and V. Presser, Energy Environ. Sci., 2015, 8, 2296–2319 RSC.
- M. E. Suss and V. Presser, Joule, 2018, 2, 10–15 CrossRef.
- W. Tang, J. Liang, D. He, J. Gong, L. Tang, Z. Liu, D. Wang and G. Zeng, Water Res., 2019, 150, 225–251 CrossRef CAS PubMed.
- A. V. Delgado, M. L. Jiménez, G. R. Iglesias and S. Ahualli, Curr. Opin. Colloid Interface Sci., 2019, 44, 72–84 CrossRef CAS.
- J. Landon, X. Gao, A. Omosebi and K. Liu, Curr. Opin. Chem. Eng., 2019, 25, 1–8 CrossRef.
- P. Długołecki and A. Van Der Wal, Environ. Sci. Technol., 2013, 47, 4904–4910 CrossRef PubMed.
- J. Kang, T. Kim, H. Shin, J. Lee, J. I. Ha and J. Yoon, Desalination, 2016, 398, 144–150 CrossRef CAS.
- R. Zhao, S. Porada, P. M. Biesheuvel and A. Van der Wal, Desalination, 2013, 330, 35–41 CrossRef CAS.
- J. Zhang, K. B. Hatzell and M. C. Hatzell, Environ. Sci. Technol. Lett., 2017, 4, 470–474 CrossRef CAS.
- P. M. Biesheuvel, R. Zhao, S. Porada and A. van der Wal, J. Colloid Interface Sci., 2011, 360, 239–248 CrossRef CAS PubMed.
- J. Lee, S. Kim, C. Kim and J. Yoon, Energy Environ. Sci., 2014, 7, 3683–3689 RSC.
- S. Wang, G. Wang, T. Wu, C. Li, Y. Wang, X. Pan, F. Zhan, Y. Zhang, S. Wang and J. Qiu, Environ. Sci. Technol., 2019, 53, 6292–6301 CrossRef CAS PubMed.
- H. Yoon, J. Lee, S. Kim and J. Yoon, Desalination, 2017, 422, 42–48 CrossRef CAS.
- S. Choi, B. Chang, S. Kim, J. Lee, J. Yoon and J. W. Choi, Adv. Funct. Mater., 2018, 28, 1–9 Search PubMed.
- X. Gao, A. Omosebi, J. Landon and K. Liu, Energy Environ. Sci., 2015, 8, 897–909 RSC.
- M. Pasta, C. D. Wessells, Y. Cui and F. La Mantia, Nano Lett., 2012, 23–27 Search PubMed.
- J. Lee, S. Kim and J. Yoon, ACS Omega, 2017, 2, 1653–1659 CrossRef CAS PubMed.
- S. Porada, A. Shrivastava, P. Bukowska, P. M. Biesheuvel and K. C. Smith, Electrochim. Acta, 2017, 255, 369–378 CrossRef CAS.
- P. Srimuk, S. Husmann and V. Presser, RSC Adv., 2019, 9, 14849–14858 RSC.
- F. Chen, Y. Huang, L. Guo, M. Ding and H. Y. Yang, Nanoscale, 2017, 9, 10101–10108 RSC.
- F. Chen, Y. Huang, L. Guo, L. Sun, Y. Wang and H. Y. Yang, Energy Environ. Sci., 2017, 10, 2081–2089 RSC.
- W. Zhao, L. Guo, M. Ding, Y. Huang and H. Y. Yang, ACS Appl. Mater. Interfaces, 2018, 10, 40540–40548 CrossRef CAS PubMed.
- Y. Huang, F. Chen, L. Guo, J. Zhang, T. Chen and H. Ying, Desalination, 2019, 451, 241–247 CrossRef CAS.
- P. Srimuk, J. Lee, S. Fleischmann, S. Choudhury, N. Jäckel, M. Zeiger, C. Kim, M. Aslan and V. Presser, J. Mater. Chem. A, 2017, 5, 15640–15649 RSC.
- X. Hou, Q. Liang, X. Hu, Y. Zhou, Q. Ru, F. Chen and S. Hu, Nanoscale, 2018, 10, 12308–12314 RSC.
- Q. Liang, F. Chen, S. Wang, Q. Ru, Q. He, X. Hou, C. Su and Y. Shi, Energy Storage Mater., 2019, 20, 203–207 CrossRef.
- D. Desai, E. S. Beh, S. Sahu, V. Vedharathinam, Q. Van Overmeere, C. F. De Lannoy, A. P. Jose, A. R. Völkel and J. B. Rivest, ACS Energy Lett., 2018, 3, 375–379 CrossRef CAS.
- M. Ghahari and H. Bemana, J. Power Sources, 2019, 412, 197–203 CrossRef CAS.
- J. Dai, N. Lar, W. Pyae, F. Chen, M. Liang, S. Wang, K. Ramalingam, S. Zhai, C. Su, Y. Shi, S. C. Tan, L. Zhang and Y. Chen, ACS Appl. Mater. Interfaces, 2020, 12, 25728–25735 CrossRef CAS PubMed.
- P. Srimuk, L. Wang, Ö. Budak and V. Presser, Electrochem. Commun., 2020, 115, 106713 CrossRef CAS.
- Y. Zhang, T. Senthilkumar, J. Park and J. Park, Batter. Supercaps Commun., 2018, 1, 6–10 CrossRef.
- H. Bae, J. Park, S. T. Senthilkumar, S. Min and Y. Kim, J. Power Sources, 2019, 410–411, 99–105 CrossRef CAS.
- D. Brogioli, Phys. Rev. Lett., 2009, 103, 058501 CrossRef PubMed.
- D. Brogioli, R. Ziano, R. A. Rica, D. Salerno, O. Kozynchenko, H. V. M. Hamelers and F. Mantegazza, Energy Environ. Sci., 2012, 5, 9870 RSC.
- N. Y. Yip, D. Brogioli, H. V. M. Hamelers and K. Nijmeijer, Environ. Sci. Technol., 2016, 50, 12072–12094 CrossRef CAS PubMed.
-
F. La Mantia, D. Brogioli and M. Pasta, Capacitive mixing and mixing entropy battery, Elsevier Ltd, 2016 Search PubMed.
- R. A. Rica, D. Brogioli, R. Ziano, D. Salerno and F. Mantegazza, J. Phys. Chem. C, 2012, 116, 16934–16938 CrossRef CAS PubMed.
- R. A. Rica, R. Ziano, D. Salerno, F. Mantegazza, M. Z. Bazant and D. Brogioli, Electrochim. Acta, 2013, 92, 304–314 CrossRef CAS.
- R. A. Rica, R. Ziano, D. Salerno, F. Mantegazza, R. van Roij and D. Brogioli, Entropy, 2013, 15, 1388–1407 CrossRef.
- F. La Mantia, M. Pasta, H. D. Deshazer, B. E. Logan and Y. Cui, Nano Lett., 2011, 11, 1810–1813 CrossRef CAS PubMed.
- D. Nam and K. Choi, ACS Appl. Mater. Interfaces, 2019, 11, 38641–38647 CrossRef CAS PubMed.
- K. Ramalingam, M. Liang, N. Lar, W. Pyae, S. H. Aung, T. Z. Oo, P. Srimuk, J. Ma, V. Presser, F. Chen and T. D. Waite, ACS Appl. Mater. Interfaces, 2020, 12, 32788–32796 CrossRef CAS PubMed.
- K. Ramalingam, Q. Wei, G. Babu, Y. Zhu, M. Han and Y. Xiao, ACS Appl. Mater. Interfaces, 2022, 14, 30907–30913 CrossRef CAS PubMed.
- D. Xu, W. Wang, M. Zhu and C. Li, ACS Appl. Mater. InterfacesCS Appl. Mater. Interfaces, 2020, 12, 57671–57685 CrossRef CAS PubMed.
- L. Wang, Y. Zhang, K. Moh and V. Presser, Curr. Opin. Electrochem., 2021, 100758 CrossRef CAS.
- D. Nam, M. A. Lumley and K. Choi, ACS Energy Lett., 2021, 6, 1034–1044 CrossRef CAS.
-
C. Xu, Z. Yang, X. Zhang, M. Xia, H. Yan and J. Li, Prussian Blue Analogues in Aqueous Batteries and Desalination Batteries, Springer Singapore, 2021, vol. 13 Search PubMed.
- P. Srimuk, X. Su, J. Yoon, D. Aurbach and V. Presser, Nat. Rev. Mater., 2020, 5, 517–538 CrossRef CAS.
- S. A. Hawks, A. Ramachandran, S. Porada, P. G. Campbell, M. E. Suss, P. M. Biesheuvel, J. G. Santiago and M. Stadermann, Water Res., 2019, 152, 126–137 CrossRef CAS PubMed.
- D. Nam, M. A. Lumley and K. Choi, Chem. Mater., 2019, 31, 1460–1468 CrossRef CAS.
- W. Bao, X. Tang, X. Guo, S. Choi, C. Wang, Y. Gogotsi and G. Wang, Joule, 2018, 2, 778–787 CrossRef CAS.
- S. Kim, H. Yoon, D. Shin, J. Lee and J. Yoon, J. Colloid Interface Sci., 2017, 506, 644–648 CrossRef CAS PubMed.
- D. H. Nam and K. S. Choi, J. Am. Chem. Soc., 2017, 139, 11055–11063 CrossRef CAS PubMed.
- A. Shrivastava, V. Q. Do and K. C. Smith, ACS Appl. Mater. Interfaces, 2022, 14, 30672–30682 CrossRef CAS PubMed.
- J. Ahn, J. Lee, S. Kim, C. Kim, J. Lee and P. M. Biesheuvel, Desalination, 2020, 476, 114216 CrossRef CAS.
- Y. Liu, X. Gao, Z. Wang, K. Wang, X. Dou, H. Zhu and X. Yuan, Chem. Eng. J., 2021, 403, 126326 CrossRef CAS.
- Y. Liu, L. Wang, Q. Yao, X. Gao, X. Du, X. Dou, H. Zhu, X. Yuan and J. Xie, Adv. Funct. Mater., 2022, 32, 2110087 CrossRef CAS.
- J. Dai, J. Wang, X. Hou, Q. Ru, Q. He, P. Srimuk, V. Presser and F. Chen, ChemSusChem, 2020, 13, 2792–2798 CrossRef CAS PubMed.
- J. Ahn, S. Kim, S. Jeon, C. Lee, J. Lee and J. Yoon, Desalination, 2021, 500, 114778 CrossRef CAS.
- K. C. Smith, R. Dmello, J. E. Soc, K. C. Smith and R. Dmello, J. e, 2016, 163, A530–A539 CAS.
- T. Kim, C. A. Gorski and B. E. Logan, Environ. Sci. Technol. Lett., 2017, 4, 444–449 CrossRef CAS.
- M. Son, V. Pothanamkandathil, W. Yang, J. S. Vrouwenvelder, C. A. Gorski and B. E. Logan, Environ. Sci. Technol., 2020, 54, 3628–3635 CrossRef CAS PubMed.
- K. C. Smith, Electrochim. Acta, 2017, 230, 333–341 CrossRef CAS.
- S. Porada, A. Shrivastava, P. Bukowska, P. M. Biesheuvel and K. C. Smith, Electrochim. Acta, 2017, 255, 369–378 CrossRef CAS.
- E. R. Reale, L. Regenwetter, A. Agrawal, B. Dardon, N. Dicola, S. Sanagala and K. C. Smith, Water Res. X, 2021, 13, 100116 CrossRef CAS PubMed.
- S. Yao, New J. Chem., 2022, 46, 1516–1532 RSC.
- S. Yao, Z. Wu, R. Liu and Q. Zhang, Ionics (Kiel)., 2022, 1791–1807 CrossRef CAS.
- L. Wang, C. Mu, H. Li and F. Li, Inorg. Chem. Front., 2018, 5, 2522–2526 RSC.
- J. Lee, J. Lee, J. Ahn, K. Jo, S. P. Hong, C. Kim, C. Lee and J. Yoon, ACS Appl. Mater. Interfaces, 2019, 11, 36580–36588 CrossRef CAS PubMed.
- J. Lee, J. Lee, S. Won, C. Kim and J. Yoon, Desalination, 2021, 515, 115188 CrossRef CAS.
- K. Silambarasan and J. Joseph, Energy Technol., 2019, 7, 1800601 CrossRef.
- Y. Tu, Y. Yang and C. Hu, Desalination, 2021, 498, 114807 CrossRef CAS.
- F. Chen, Y. Huang, L. Guo, M. Ding and H. Y. Yang, Nanoscale, 2017, 9, 10101–10108 RSC.
- B. Shapira, I. Cohen, T. R. Penki, E. Avraham and D. Aurbach, J. Power Sources, 2018, 378, 146–152 CrossRef CAS.
- F. Chen, Y. Huang, D. Kong, M. Ding, S. Huang and H. Y. Yang, FlatChem, 2018, 8, 9–16 CrossRef CAS.
- N. Kim, S. P. Hong, J. Lee, C. Kim and J. Yoon, ACS Sustain. Chem. Eng., 2019, 7, 16182–16189 CrossRef CAS.
- F. Chen, J. Wang, C. Feng, J. Ma and T. D. Waite, Chem. Eng. J., 2020, 126111 CrossRef CAS.
- E. S. Beh, M. Benedict, D. Desai and J. B. Rivest, ACS Sustain. Chem. Eng., 2019, 7, 13411–13417 CrossRef CAS.
- J. Wang, Q. Zhang, F. Chen, X. Hou, Z. Tang, Y. Shi, P. Liang, D. Y. W. Yu, Q. He and L. Li, J. Mater. Chem. A, 2019, 7, 13941–13947 RSC.
- F. Chen, J. Wang, Q. Ru, S. H. Aung, T. Z. Oo and B. Chu, J. Electrochem. Soc., 2020, 167, 083503 CrossRef CAS.
- S. M. Shariatpannahi, M. S. Hatamipour, S. Rashid-Nadimi and M. K. Amini, J. Environ. Chem. Eng., 2021, 9, 104802 CrossRef CAS.
- J. Dai, J. Zhang, R. Karthick, M. Liang, Q. Wei, X. Chen, Y. Shi, S. Zhai, G. Wang and F. Chen, J. Mater. Chem. A, 2022, 10, 12213–12224 RSC.
- L. Baudino, C. Santos, C. F. Pirri, F. La Mantia and A. Lamberti, Adv. Sci., 2022, 2201380 CrossRef CAS PubMed.
- H. Kang, Y. Liu, K. Cao, Y. Zhao, L. Jiao, Y. Wang and H. Yuan, J. Mater. Chem. A, 2015, 3, 17899–17913 RSC.
- V. Palomares, M. Casas-Cabanas, E. Castillo-Martínez, M. H. Han and T. Rojo, Energy Environ. Sci., 2013, 6, 2312–2337 RSC.
- Y. You, Z. Sang and J. Liu, Mater. Technol., 2016, 31, 501–509 CrossRef CAS.
- J. F. Whitacre, T. Wiley, S. Shanbhag, Y. Wenzhuo, A. Mohamed, S. E. Chun, E. Weber, D. Blackwood, E. Lynch-Bell, J. Gulakowski, C. Smith and D. Humphreys, J. Power Sources, 2012, 213, 255–264 CrossRef CAS.
- D. A. Tompsett and M. S. Islam, Chem. Mater., 2013, 25, 2515–2526 CrossRef CAS.
- Q. T. Qu, L. L. Liu, Y. P. Wu and R. Holze, Electrochim. Acta, 2013, 96, 8–12 CrossRef CAS.
- Y. Zhang, C. Yuan, K. Ye, X. Jiang, J. Yin, G. Wang and D. Cao, Electrochim. Acta, 2014, 148, 237–243 CrossRef CAS.
- B. Tekin, S. Sevinc, M. Morcrette and R. Demir-Cakan, Energy Technol., 2017, 5, 2182–2188 CrossRef CAS.
- Y. Zhang, Y. An, J. Jiang, S. Dong, L. Wu, R. Fu, H. Dou and X. Zhang, Energy Technol., 2018, 6, 2146–2153 CrossRef CAS.
- Z. Khan, B. Senthilkumar, S. O. Park, S. Park, J. Yang, J. H. Lee, H. K. Song, Y. Kim, S. K. Kwak and H. Ko, J. Mater. Chem. A, 2017, 5, 2037–2044 RSC.
- G. Pang, P. Nie, C. Yuan, L. Shen, X. Zhang, J. Zhu and B. Ding, Energy Technol., 2014, 2, 705–712 CrossRef CAS.
- J. F. Whitacre, A. Tevar and S. Sharma, Electrochem. Commun., 2010, 12, 463–466 CrossRef CAS.
- X. Wu, Y. Luo, M. Sun, J. Qian, Y. Cao, X. Ai and H. Yang, Nano Energy, 2015, 13, 117–123 CrossRef CAS.
- M. Pasta, C. D. Wessells, N. Liu, J. Nelson, M. T. McDowell, R. A. Huggins, M. F. Toney and Y. Cui, Nat. Commun., 2014, 5, 1–9 Search PubMed.
- X. Wu, M. Sun, S. Guo, J. Qian, Y. Liu, Y. Cao, X. Ai and H. Yang, ChemNanoMat, 2015, 1, 188–193 CrossRef CAS.
- A. J. Fernández-Ropero, M. J. Piernas-Muñoz, E. Castillo-Martínez, T. Rojo and M. Casas-Cabanas, Electrochim. Acta, 2016, 210, 352–357 CrossRef.
- Q. Yang, W. Wang, H. Li, J. Zhang, F. Kang and B. Li, Electrochim. Acta, 2018, 270, 96–103 CrossRef CAS.
- K. Nakamoto, R. Sakamoto, Y. Sawada, M. Ito and S. Okada, Small Methods, 2019, 3, 1–5 Search PubMed.
- C. D. Wessells, S. V. Peddada, R. A. Huggins and Y. Cui, Nano Lett., 2011, 11, 5421–5425 CrossRef CAS PubMed.
- A. J. Fernández-Ropero, D. Saurel, B. Acebedo, T. Rojo and M. Casas-Cabanas, J. Power Sources, 2015, 291, 40–45 CrossRef.
- Y. Mei, Y. Huang and X. Hu, J. Mater. Chem. A, 2016, 4, 12001–12013 RSC.
- A. J. Fernández-Ropero, M. Zarrabeitia, M. Reynaud, T. Rojo and M. Casas-Cabanas, J. Phys. Chem. C, 2018, 122, 133–142 CrossRef.
- Z. Liu, G. Pang, S. Dong, Y. Zhang, C. Mi and X. Zhang, Electrochim. Acta, 2019, 311, 1–7 CrossRef CAS.
- S. Sevinc, B. Tekin, A. Ata, M. Morcrette, H. Perrot, O. Sel and R. Demir-Cakan, J. Power Sources, 2019, 412, 55–62 CrossRef CAS.
- J. K. Kim, F. Mueller, H. Kim, S. Jeong, J. S. Park, S. Passerini and Y. Kim, ChemSusChem, 2016, 9, 42–49 CrossRef CAS PubMed.
- M. Abirami, S. M. Hwang, J. Yang, S. T. Senthilkumar, J. Kim, W. S. Go, B. Senthilkumar, H. K. Song and Y. Kim, ACS Appl. Mater. Interfaces, 2016, 8, 32778–32787 CrossRef CAS PubMed.
- Z. Khan, S. O. Park, J. Yang, S. Park, R. Shanker, H. K. Song, Y. Kim, S. K. Kwak and H. Ko, J. Mater. Chem. A, 2018, 6, 24459–24467 RSC.
- J. K. Kim, F. Mueller, H. Kim, D. Bresser, J. S. Park, D. H. Lim, G. T. Kim, S. Passerini and Y. Kim, NPG Asia Mater., 2014, 6, e144 CrossRef CAS.
- J. Kim, Y. Kim and Y. Kim, J. Mater. Chem. A, 2014, 2, 19584–19588 RSC.
- A. Mauger, C. M. Julien, J. B. Goodenough and K. Zaghib, J. Electrochem. Soc., 2020, 167, 070507 CrossRef CAS.
- Y. Idota, T. Kubota, A. Matsufuji, Y. Maekawa and T. Miyasaka, Science, 1997, 276, 1395–1397 CrossRef CAS.
- J. M. Tarascon and D. Guyomard, Electrochim. Acta, 1993, 38, 1221–1231 CrossRef CAS.
- S. Y. Huang, J. Electrochem. Soc., 1995, 142, L142 CrossRef CAS.
- H. Zhang, C. Li, G. G. Eshetu, S. Laruelle, S. Grugeon, K. Zaghib, C. Julien, A. Mauger, D. Guyomard, T. Rojo, N. Gisbert-Trejo, S. Passerini, X. Huang, Z. Zhou, P. Johansson and M. Forsyth, Angew. Chem., Int. Ed., 2020, 59, 534–538 CrossRef CAS PubMed.
- L. Shi, X. Bi, E. Newcomer, D. M. Hall, C. A. Gorski, A. Galal and B. E. Logan, Chem. Eng. J., 2022, 435, 135001 CrossRef CAS.
- S. Yao, R. Liu, Z. Wu, J. Luo and Q. Zhang, J. Electrochem. Soc., 2022, 169, 123501 CrossRef CAS.
- World Health Organization, WHO: Alternative drinking-water disinfectants: bromine, iodine and silver, World Health Organization, Geneva, 2018 Search PubMed.
- T. Katan, S. Szpak and D. N. Bennion, J. Electrochem. Soc., 1974, 121, 757 CrossRef CAS.
- H. Ha and J. Payer, Electrochim. Acta, 2011, 56, 2781–2791 CrossRef CAS PubMed.
- J. M. M. Droog and F. Huisman, J. Electroanal. Chem., 1980, 115, 211–224 CrossRef CAS.
- F. Chen, Z. Y. Leong and H. Y. Yang, Energy Storage Mater., 2017, 7, 189–194 CrossRef.
- S. Vafakhah, M. Saeedikhani, M. Ding, L. Guo and Y. Von Lim, Desalination, 2022, 528, 115514 CrossRef CAS.
- W. Wei, X. Feng, Z. Chen, R. Wang and H. Chen, ACS Sustain. Chem. Eng., 2022, 10, 9295–9302 CrossRef CAS.
- Y.-H. Yang, Y. Tu, H. Huang and C. Hu, Desalination, 2023, 545, 116160 CrossRef CAS.
- P. Srimuk, J. Halim, J. Lee, Q. Tao, J. Rosen and V. Presser, ACS Sustain. Chem. Eng., 2018, 6, 3739–3747 CrossRef CAS.
- B. Zhang, A. Boretti and S. Castelletto, Chem. Eng. J., 2022, 435, 134959 CrossRef CAS.
- N. Hussain, N. Mujawar and R. Rao, Chemosphere, 2023, 314, 137643 CrossRef PubMed.
- R. A. Klemm, Phys. C, 2015, 514, 86–94 CrossRef CAS.
-
X. Li, L. Wu, L. Hao and Y. Fu, Emerging 2D nanomaterials for supercapacitor applications, Elsevier Inc., 2018 Search PubMed.
-
B. Chakraborty, Electronic structure and theoretical aspects on sensing application of 2D materials, Elsevier Ltd, 2019 Search PubMed.
- M. Pumera, Z. Sofer and A. Ambrosi, J. Mater. Chem. A, 2014, 2, 8981–8987 RSC.
- Q. Yun, L. Li, Z. Hu, Q. Lu, B. Chen and H. Zhang, Adv. Mater., 2020, 32, 1–29 Search PubMed.
- M. A. Bissett, S. D. Worrall, I. A. Kinloch and R. A. W. Dryfe, Electrochim. Acta, 2016, 201, 30–37 CrossRef CAS.
- J. Feng, M. Graf, K. Liu, D. Ovchinnikov, D. Dumcenco, M. Heiranian, V. Nandigana, N. R. Aluru, A. Kis and A. Radenovic, Nature, 2016, 536, 197–200 CrossRef CAS PubMed.
- F. Xing, T. Li, J. Li, H. Zhu, N. Wang and X. Cao, Nano Energy, 2017, 31, 590–595 CrossRef CAS.
- F. Jia, K. Sun, B. Yang, X. Zhang, Q. Wang and S. Song, Desalination, 2018, 446, 21–30 CrossRef CAS.
- W. Wei, X. Gu, R. Wang, X. Feng and H. Chen, Nano Lett., 2022, 22, 7572–7578 CrossRef CAS PubMed.
- J. Winsberg, T. Hagemann, T. Janoschka, M. D. Hager and U. S. Schubert, Angew. Chem., Int. Ed., 2017, 56, 686–711 CrossRef CAS PubMed.
- C. Choi, S. Kim, R. Kim, Y. Choi, S. Kim, H. Young Jung, J. H. Yang and H. T. Kim, Renew. Sustain. Energy Rev., 2017, 69, 263–274 CrossRef CAS.
- X. L. Zhou, T. S. Zhao, L. An, Y. K. Zeng and L. Wei, J. Power Sources, 2017, 339, 1–12 CrossRef CAS.
- A. Z. Weber, M. M. Mench, J. P. Meyers, P. N. Ross, J. T. Gostick and Q. Liu, J. Appl. Electrochem., 2011, 41, 1137–1164 CrossRef CAS.
- S. A. Khalla and M. E. Suss, Desalination, 2019, 467, 257–262 CrossRef.
- C. Cheng, T. Chen, K. Chen, J. Ma and C. Hou, Desalination, 2022, 534, 115783 CrossRef CAS.
- G. Mohandass, W. Chen, S. Krishnan and T. Kim, Environ. Sci. Technol., 2022, 56, 4477–4488 CrossRef CAS PubMed.
- Q. Zhang, S. H. Aung, T. Z. Oo and F. Chen, Mater. Today Commun., 2020, 23, 100921 CrossRef CAS.
- M. Ligaray, N. Kim, S. Park, J. Park, J. Park, Y. Kim and K. Hwa, Chem. Eng. J., 2020, 395, 125082 CrossRef CAS.
- N. Kim, J. Park, A. M. Harzandi, K. Kishor, M. Ligaray, K. H. Cho and Y. Kim, Desalination, 2020, 495, 114666 CrossRef CAS.
- S. Park, M. Ligaray, Y. Kim, K. Chon, M. Son and K. Hwa, Desalination, 2021, 506, 115018 CrossRef CAS.
- S. Park, N. Kim, Y. Kim, M. Son and K. Hwa, J. Clean. Prod., 2022, 333, 130188 CrossRef CAS.
- N. Kim, S. Jeong, W. Go and Y. Kim, Water Res., 2022, 215, 118250 CrossRef CAS PubMed.
- M. Son, J. Shim, S. Park, N. Yoon, K. Jeong and H. Cho, Desalination, 2022, 531, 115713 CrossRef CAS.
- S. Arnold, L. Wang and V. Presser, Small, 2022, 18, 2107913 CrossRef CAS PubMed.
- J. S. Lee, S. T. Kim, R. Cao, N. S. Choi, M. Liu, K. T. Lee and J. Cho, Adv. Energy Mater., 2011, 1, 34–50 CrossRef CAS.
- F. Cheng and J. Chen, Chem. Soc. Rev., 2012, 41, 2172–2192 RSC.
- X. Zhang, X. G. Wang, Z. Xie and Z. Zhou, Green Energy Environ., 2016, 1, 4–17 CrossRef.
- X. Han, X. Li, J. White, C. Zhong, Y. Deng, W. Hu and T. Ma, Adv. Energy Mater., 2018, 8, 1–28 Search PubMed.
- Y. Li and J. Lu, ACS Energy Lett., 2017, 2, 1370–1377 CrossRef CAS.
- E. R. Reale, A. Shrivastava and K. C. Smith, Water Res., 2019, 114995 CrossRef CAS PubMed.
- N. Kim, J. Jeon, J. Elbert, C. Kim and X. Su, Chem. Eng. J., 2022, 428, 131082 CrossRef CAS.
Footnote |
† Most of the desalination parameters here gathered are explicitly reported in the ad hoc publication. However, some parameters were estimated from the info provided in the reference. |
|
This journal is © The Royal Society of Chemistry 2023 |