DOI:
10.1039/D2TC03100K
(Paper)
J. Mater. Chem. C, 2022,
10, 15386-15393
Two-dimensional black phosphorus-modified Cs2AgBiBr6 with efficient charge separation for enhanced visible-light photocatalytic H2 evolution†
Received
23rd July 2022
, Accepted 15th September 2022
First published on 15th September 2022
Abstract
Lead-free double halide perovskite (Cs2AgBiBr6) is considered to be a potential candidate material for photocatalytic hydrogen production due to its excellent visible light capture capability. However, the rate of the hydrogen evolution reaction (HER) taking into account these catalysts is still insufficient. In this study, two-dimensional black phosphorus (BP) anchored on Cs2AgBiBr6 (CABB) by electrostatic coupling was prepared. The resulting catalyst BP/CABB was quite stable in a HBr solution during the entire light reaction and reached a hydrogen production rate of 104.6 μmol h−1 g−1 under visible light, which was higher than that of CABB alone. Significantly enhanced HER activity was explored by optical/photochemical measurements, indicating that BP can be used as an electronic accelerator, transmitted by a Z-scheme heterojunction in the interface of the catalyst to transfer electrons, and further produce hydrogen. This has been instrumental in the high-efficiency photocatalyst by anchoring the nonmetallic active sites on CABB.
1. Introduction
Energy and the environment are currently the two most important issues in society. With the development of technology and the varied demands of the world, the global energy crisis and various environmental issues have become increasingly severe.1–3 Photocatalytic hydrogenation technology involving various semiconductor materials has attracted extensive attention as a clean and sustainable solar–hydrogen energy conversion technology.4–6 Many efforts regarding photocatalytic hydrogen production technology are committed to utilizing low-cost and efficient photocatalysts. Although huge progress has been achieved, better candidates will always be sought. Among the various photocatalytic systems, the ideal photocatalyst should have a suitable optical bandgap and the ability to capture visible light and convert the absorbed solar energy into hydrogen energy.7
In recent years, due to their unique light absorption capacity and efficient charge separation properties, lead-based halide perovskites have become promising photocatalysts and have developed rapidly in the fields of H2 evolution, pollutant degradation, organic reactions, and carbon dioxide reduction.8–10 However, the instability and ecological toxicity of lead-based perovskites hinder their practical applications. In recent years, lead-free double perovskites have been studied gradually deepen, for example, Cs3Bi2X9 (X = Cl, Br, I), (MA)3Bi2I9, DMASnBr3, and Cs2AgBiBr6 (CABB).11–13 Bi-based double perovskite CABB has shown potential in photocatalytic hydrogen production because of its good stability and suitable light absorption coefficient.14–16 In 2018, Kuang et al. prepared CABB nanocrystals via a simple heat injection method and used them for photocatalytic CO2 reduction.17 Zhao et al. constructed a Cs2AgBiBr6/rGO photocatalyst for H2 evolution by an in situ photoreduction method, reporting a photocatalytic system for the construction of a lead-free halide perovskite/semiconductor heterojunction.18 Most recently, N–C,19 Cu–RGO,20 g-C3N4,21 MoS2,22 and NiCoP23 have been exploited as costructures, and the obtained composites showed an obvious enhancement of photocatalytic activity compared to pristine CABB.
Two-dimensional black phosphorus (2D BP) with a large surface area, abundant active sites and adjustable band gaps (0.3–2.0 eV) is widely studied as a photocatalytic semiconductor catalyst.24,25 In particular, BP exhibited excellent characteristics as a potential high-performance cocatalyst: (1) the large surface area has rich active sites, and the photocatalyst is structurally coupled electronically; (2) it has good carrier mobility; (3) the tunable bandgap provides a huge opportunity for a good structure. Shi's group26 prepared black phosphorus quantum dot/CdS (BPQDS/CDS) composites with tight interfacial contact for efficient photocatalytic H2 evolution, benefiting from the strong interfacial interaction between CdS and BPQDS for efficient charge separation. Zhu and colleagues27 anchored inorganic perovskite (CsPbBr3) on BP to obtain a new effective CsPbBr3/BP photocatalyst for CO2 reduction. The introduction of BP gave more active sites to promote the activation of CO2 reduction and capture excitation electrons of CsPbBr3 with high charge separation efficiency. Therefore, the use of BP as a metal-free costructure for photocatalytic H2 production is promising and challenging.
In this work, two-dimensional black phosphorus was first coupled with the CABB double perovskite applied for hydrobromic acid (HBr) splitting under visible light irradiation. Layered BP with good electron transfer properties and acid stability was introduced to further improve the H2 evolution. The H2 evolution rate of BP/CABB can reach 104.6 μmol h−1 g−1, which is 6 times higher than that of pristine CABB. Excitingly, the BP/CABB composites are quite stable in a saturated HBr solution, and their activity does not significantly decrease during the entire light reaction process. BP/CABB heterogeneous structures have good stability, providing new examples for the design and preparation of high-efficiency lead-free double perovskite catalysts.
2. Experiment
2.1 Photocatalyst synthesis
2.1.1 Preparation of CABB.
CABB was synthesized from aqueous solution according to a previous report.14 A total of 1.0 mmol BiBr3, 1.0 mmol AgBr, and 2.0 mmol CsBr were dissolved in 10 mL of a 48% HBr solution, and then the mixture was heated to 110 °C for 2 h under continuous magnetic stirring in an oil bath. After cooling to room temperature naturally, the precipitates were collected and dried in a vacuum oven at 60 °C for 12 h, and the obtained orange sample was named CABB. As shown in Fig. 1, the saturated HBr solution (saturated with CABB power) for photocatalytic H2 evolution was prepared by mixing HBr and H3PO2 at a volume ratio of 5
:
1.
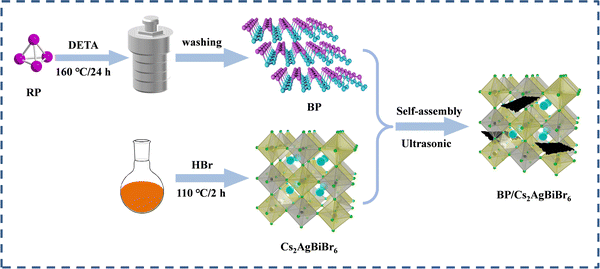 |
| Fig. 1 Schematic diagram of the BP/CABB composite fabrication process. | |
2.1.2 Preparation of BP.
BP was prepared with red phosphorus as the raw material and diethylenetriamine (DETA) as the solvent. In a typical synthesis process, red phosphorus (2.0 g, 0.06 mol) was added to an agate mortar and ground. Then, the mixture with 40 mL deionized water (DI) was transferred to a 100 mL Teflon-lined stainless steel autoclave and heated at 200 °C for 12 h. After cooling to room temperature, the mixture was washed, centrifuged, and dried overnight at 60 °C, and the sample was labeled RP. Next, the prepared RP (0.90 g) was added to 60 mL of a DETA solution and was stirred for 30 min; the mixture was then heated at 160 °C in a 100 mL Teflon-lined stainless steel autoclave for 24 h. After cooling to room temperature, the mixture was washed and centrifuged, and the product was collected and named BP.
2.1.3 Preparation of BP/CABB composites.
We adjusted the mass ratio of the samples to achieve the optimum photocatalytic activity. Therefore, we conducted a series of experiments measuring the hydrogen evolution of the BP/CABB composite materials. Specifically, the prepared 50 mg CABB was first added to a saturated HBr aqueous solution, and then different BP mass ratios were added to the above solution and ultrasonicated for 20 min. During the precipitated crystalline cycle, the BP/CABB photocatalysts were obtained directly for photocatalytic hydrogenation or rinsed and dried to carry out further characterization. Samples were labeled as x% BP/CABB (x = 5, 10, 15, 20), where x is the mass percentage of BP and CABB. And the actual content of the catalyst 10% BP/CABB was also quantified by EDX testing (Table S1 and Fig. S1, ESI†).
2.2 Characterization
Powder X-ray diffraction (XRD) analysis was performed on a PANalytical X’pertProAlpha-1 X-ray diffractometer with Cu Kα radiation to explore the crystalline structure. The zeta potentials of the samples were measured on a Malvern zeta analyzer (Zetasizer Nano-S 90, Malvern Instrument, UK). Transmission electron microscopy (TEM) and high-resolution transmission electron microscopy (HRTEM) images were obtained on a JEOL JEM-2100 F electron microscope operated at an accelerating voltage of 200 kV. The UV-vis diffuse reflectance absorption spectrum (DRS) was measured using an ultraviolet-visible spectrophotometer (UV-3600, Shimadzu) with BaSO4 as a reference sample; X-ray photoelectron spectroscopy (XPS) was performed on a Thermo Scientific ESCALAB Xi +, the excitation source was monochromatic Al Kα, and C 1s = 284.6 eV was used for reference calibration. Raman spectra were recorded on an Alpha 300 Access Raman microscope (Witec, Germany) with a 532 nm laser as an excitation source at room temperature.
2.3 Photocatalytic experiments
Photocatalytic H2 production experiments were performed in an instrument equipped with a quartz vessel under the illumination of a 300 W Xe lamp (λ ≥ 420 nm). The BP/CABB photocatalyst (0.05 g) was added to 10 mL of CABB-saturated HBr/H3PO2 solution, and the temperature of this mixture was maintained at 15 °C through a cooling water circulation system. The suspension extracted dissolved air before the reaction by a vacuum pump. After that, the reaction solution was irradiated to produce H2 under continuous stirring. The amount of H2 produced was automatically removed and analyzed at given time intervals with a CEL-SPH2N fully automatic online catalytic system (Beijing China Education Au-light Co., Ltd).
2.4 PEC measurements
The photocurrents and electrochemical impedance spectroscopy (EIS) profiles of the catalysts were evaluated using a standard three-electrode system (CHI760E), where a Pt electrode was used as the counter electrode, an Ag/AgCl electrode was used as the reference electrode, photocatalysts were coated on FTO (1 cm × 1 cm) as the working electrode, and 0.1 M tetrabutylammonium hexafluorophosphate (TBAPF6) in dichloromethane (CH2Cl2) was the electrolyte. The working electrode was prepared as follows: 10 mg samples were added to a mixture of 500 μL absolute ethanol and 10 μL Nafion and then sonicated for 60 min. Finally, a certain amount of the mixture was dripped onto FTO to prepare the working electrode.
3. Results and discussion
3.1 Structure, composition, and optical properties of the photocatalyst
To achieve large-scale and low-cost BP production, BP was prepared from bulk red phosphorus using a solvent thermal reaction. After the solvent thermal reaction, the resulting solution was observed to become black, and a black product was obtained after washing. The X-ray diffraction pattern of the prepared BP powder sample showed the main features of orthogonal BP (PDF#76-1967); diffraction peaks located at 17.6°, 26.8°, 35.2°, and 57.2° correspond to the (020), (021), (040), and (151) crystalline planes, respectively (Fig. S2a, ESI†).28 It is worth noting that the synthetic BP had an additional peak at 10.2° in the XRD pattern, which was due to a stacking breaking layer or periodic distortion in the c-axis direction.29 Raman spectroscopy also confirmed the successful preparation of BP and composites. In Fig. S2b (ESI†), we recorded the Raman spectrum of BP and obtained three typical Raman peaks: Ag1 (outdoor patterns), B2g and Ag2 (in-plane mode), and the wavenumbers were ∼361, ∼437, and ∼460 cm−1, respectively.30 These results suggest that this bottom-up solvothermal method is a reliable method for preparing large-scale and low-cost BP.
To verify the structural stability of the BP, we tested the XRD of the prepared BP after 12 h of stirring in a HBr solution. As shown in Fig. S3a (ESI†), the structure of BP did not change significantly after HBr treatment, but the intensity of the diffraction peak was reduced, which is possibly due to BP oxidation by the environment. As seen in Fig. S3b (ESI†), CABB and BP had zeta potentials of −16.4 mV and 8.98 mV, respectively. The opposite potentials generate strong electrostatic attraction between CABB and BP, which increases interfacial contacts. We used the ultrasonic electrostatic self-assembly method to prepare a series of x% BP/CABB composites. As seen in Fig. 2a, the XRD of the BP/CABB composites showed all the characteristic peaks of CABB with a good cubic structure. The characteristic diffraction peaks were located at 13°, 15.9°, 22.3°, 26.1°, 27.4°, 31.8°, 35.7°, 39.2° and 45.5° belonging to the (111), (002), (022), (113), (222), (004), (024), (224) and (440) planes, respectively (JCPDS # 01-084-8699).20 No additional diffraction peaks attributed to BP were observed even at higher addition amounts, probably due to the high dispersion of BP and the high crystallinity of CABB.31–33 The presence of BP in the composites was also confirmed by comparing the UV-visible diffuse spectra (DRS) of the original BP, CABB and BP/CABB composites. As shown in Fig. 2b, the light absorption of the BP/CABB composite was enhanced between 600 and 750 nm relative to the original CABB due to the black effect of the BP. With the increase of black phosphine loading, the optical absorption intensity of the composite material also increased, but the increase amount changed little.
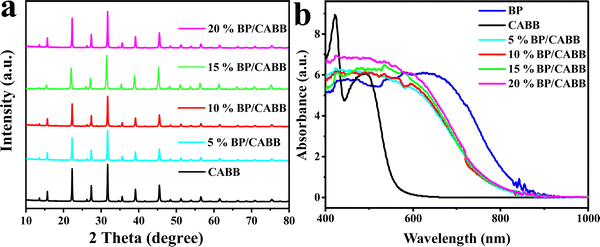 |
| Fig. 2 (a) XRD and (b) DRS patterns of the obtained CABB, BP and BP/CABB. | |
To clarify the interface interactions in BP/CABB, TEM and SEM images were obtained, and the corresponding elemental mapping analysis was performed. The original CABB particles had a relatively smooth and large bulk, and BP showed a laminate stacked structure (Fig. S43a and b, ESI†). The surface of the BP/CABB composite becomes rougher due to the coupling of layered BP (Fig. 3a). Thus, more contact sites in the reaction are provided. The TEM image of composite BP/CABB showed small, layered BP in close contact with large CABB particles (Fig. 3b), and the size of the BP was approximately 500 nm. The EDS mappings (Fig. 3c) of composite BP/CABB confirmed the uniform distribution of Cs, Ag, Bi, Br, and P elements on the catalyst surface. In the high-resolution TEM image (Fig. 3d), two different lattice fringes of 0.33 nm and 0.23 nm were present, corresponding to the (021) plane of BP and the (004) plane of CABB, respectively. The TEM images show that the composites are tightly bound together; in particular, the high-resolution TEM images (Fig. S4c and d, ESI†) show that BP and CABB are embedded together rather than physically adsorbed. These results revealed the successful coupling of CABB with BP.
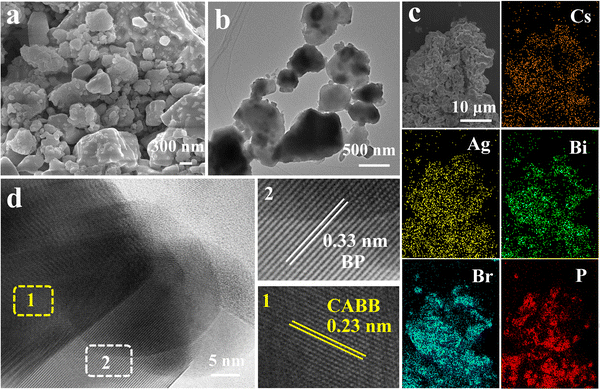 |
| Fig. 3 (a) SEM image, (b) TEM image, (c) elemental mapping of Cs, Ag, Bi, Br and P and (d) HRTEM images of the 10% BP/CABB composite. | |
X-Ray photoelectron spectroscopy (XPS) was further used to explore the chemical state of the resulting BP, CABB and CABB/CABB composites. The XPS survey spectra (Fig. 4a) confirmed the presence of BP in the BP/CABB composites. As shown in Fig. 4b, Cs 3d can be divided into two peaks at 723.9 eV and 739.9 eV, corresponding to Cs 3d5/2 and Cs 3d3/2, respectively. Ag 3d can be divided into peaks located at 367.5 eV and 373.6 eV, corresponding to Ag 3d5/2 and Ag 3d3/2, respectively (Fig. 4c). As shown in Fig. 4d, Bi 4f can be divided into two peaks located at 158.7 eV and 164.1 eV, corresponding to Bi 4f7/2 and Bi 4f5/2, respectively. Br 3d can be divided into two peaks located at 67.2 eV and 68.3 eV, corresponding to Br 3d5/2 and Br 3d3/2, respectively (Fig. 4e). Interestingly, Bi 4f is shifted to a lower binding energy (0.3 eV) (Fig. 4d), while the P 2p peaks migrate to a higher binding energy (0.5 eV) (Fig. 4f). The study shows that the binding energy migration is closely related to the electron density outside the nucleus. When the binding energy is shifted to a high field, the electron density decreases.34 This is consistent with the results reported in the literature.21,35,36 The binding energy migration of P and Bi greatly confirms the strong electronic coupling between BP and CABB, which is in accordance with the Raman spectroscopy results. It is worth noting that a small P oxide species is apparent at 134 eV due to the inevitable local oxidation during the preparation process. All the results show that the prepared BP/CABB composite has a strong interfacial electron coupling rather than simple physical mixing, which is very beneficial to the efficient migration of interfacial carriers.
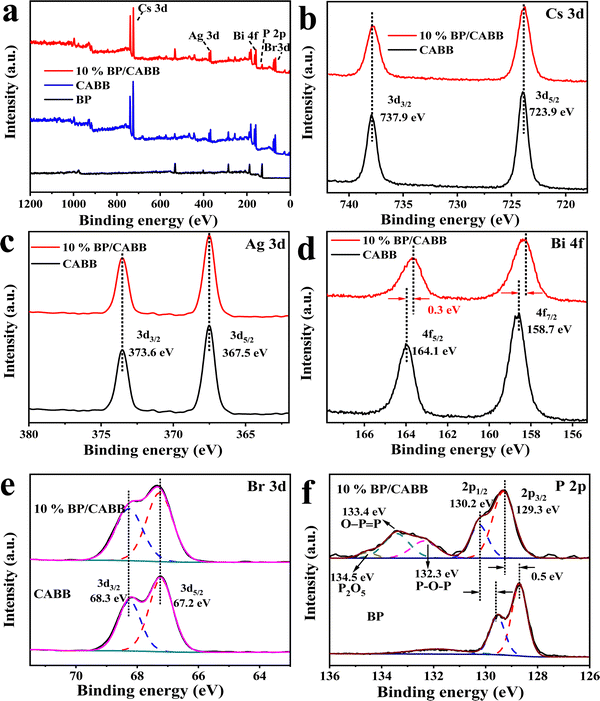 |
| Fig. 4 (a) Survey XPS spectra of CABB, BP and the 10% BP/CABB composite; high-resolution XPS spectrum of (b) Cs 3d, (c) Ag 3d, (d) Br 3d, (e) Bi 4f and (f) P 2p. | |
3.2 Photocatalytic hydrogenation activity
The photocatalyst was evolved in a saturated HBr/H3PO2 mixed solution (v/v = 5
:
1) under visible light irradiation, and the photocatalytic H2 evolution activity of the different catalysts is shown in Fig. 5a. Bare BP (50 mg) showed little H2 evolution activity, while CABB (50 mg) showed an H2 evolution rate of 17.7 μmol g−1 h−1, which was extremely low due to the rapid recombination of photogenerated electron–hole pairs. As the amount of BP added to the BP/CABB increased, the hydrogen production rate was further improved, and the catalyst 10% BP/CABB composite gave the best rate of 104.6 μmol h−1 g−1, which was 6 times higher than that of pristine CABB. When the BP addition further increased to 15%, the incident light was blocked by excess BP, resulting in a decrease in photocatalytic activity. The results showed that BP can show photocatalytic H2 evolution under visible light as a highly active cocatalyst of CABB.
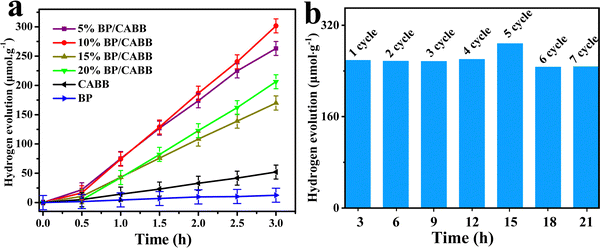 |
| Fig. 5 (a) Yield of H2 reduction by CABB, BP and the 10% BP/CABB composite after 3 h of photochemical reaction, error bar: ±0.03; and (b) recycling test of the 10% BP/CABB composite. | |
Cyclic performance is an important index for evaluating heterogeneous catalysts. In this catalytic system, no significant decline in H2 evolution was observed after 7 cycles of 3 h of continuous photoreaction (Fig. 5b). After being reused, the catalyst showed no crystallographic phase change in the XRD pattern (Fig. S5, ESI†). The good structural stability of BP/CABB composites can be rationalized, and CABB surfaces can constantly resume sustained oxidation of Br−–Br3− due to the perfect dynamic equilibrium of the CABB crystal in a saturated aqueous solution. Therefore, both CABB and BP in the composite material were highly stable due to the strong interaction. BP, as a photosensitizer and H2 evolution cocatalyst, also enhanced the efficient and sustainable photocatalytic H2 evolution.
3.3 Activation mechanism
We studied the photocatalytic mechanism of the BP/CABB system by optical absorption and valence band (VB) XPS. According to the Tauc plot method, (αhv)1/n = A (hv − Eg), where the direct bandgap semiconductor is n = 1/2 and the indirect bandgap semiconductor is n = 2. BP is a direct band gap semiconductor, CABB is an indirect bandgap semiconductor, and the Eg values of BP and CABB were calculated to be 1.57 V and 2.15 V, respectively (Fig. S6a and b, ESI†). The calculated value of BP and CABB from the intercept between the tangent of the onset and the baseline of the spectra is 0.50 V and 1.69 V, respectively (Fig. S6c and d, ESI†). This is consistent with the reported valence band position (EVB).21,37 Based on the relationships between ECB and EVB (ECB = EVB − Eg), the calculated conduction band positions (ECB) for BP and CABB were −1.07 V and −0.46 V.26 Based on the above results, a photocatalytic mechanism was proposed (Fig. 6). According to the H2 evolution “band matching” theory, the ECB of BP is more negative than CABB, and the EVB of CABB is more positive than BP. When both BP and CABB were excited under visible light irradiation, the photogenerated electrons in the CB of CABB quickly combined with the photogenerated holes in the VB of BP and then combined with the photogenerated electrons of BP for the reduction reaction. The photogenerated holes in the VB of CABB are used for the oxidation reaction. These band structures suggest the possibility of constructing the Z-scheme photocatalysis feature to split HBr into H2 and Br3−, where Br3− is then converted to Br− by H3PO2.
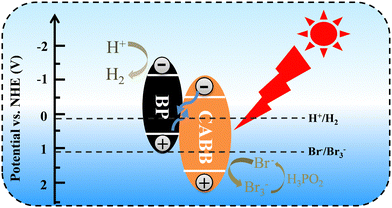 |
| Fig. 6 Schematic illustration of the H2 evolution of the BP/CABB composites. | |
Photoelectric chemistry (PEC) analysis is widely used to provide effective evidence for the separation and metastasis of photogenerated electron–hole pairs. To explore the mechanism of BP effect on the photocatalytic activity on CABB, we performed a series of PEC measurements. BP/CABB exhibited a higher photocurrent response than bare CABB (Fig. 7a), and the photocurrent responses of the samples were highly repeatable upon on/off light cycling. This indicated the efficient separation of carriers in BP/CABB. Electrochemical impedance spectroscopy (EIS) measurements (Fig. 7b) indicated that BP/CABB had a smaller charge transfer resistor than the bare CABB, indicating that the coupled BP provides a rapid electron transfer pathway to improve charge separation efficiency. All the results demonstrated that coupling with BP can significantly increase the carrier separation efficiency of CABB, which greatly increases the photocatalytic efficiency of BP/CABB.
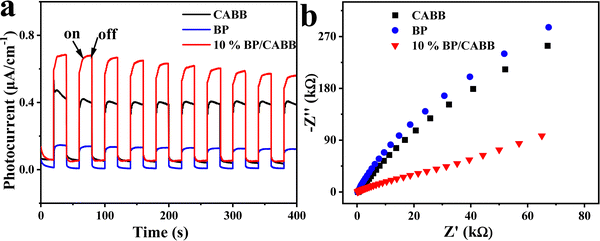 |
| Fig. 7 (a) Chopped light amperometric I–t curves and (b) EIS Nyquist plots of CABB, BP and the 10% BP/CABB composite. | |
4. Conclusions
In summary, we successfully fabricated 2D BP/CABB composites with a high-quality interface via a facile electrostatic self-assembly method. The H2 evolution rate of the most effective BP/CABB composite was 6 times higher than that of the original CABB and had excellent H2 evolution stability. In the BP/CABB composites, the charge induction between BP and CABB made the interface interaction strong, which can realize efficient charge separation and improve the photocatalytic H2 evolution activity. This work demonstrates a simple and effective strategy for the synthesis of BP/CABB composites with high-quality interactions. This study also provides new insight into the design of highly active photocatalysts based on lead-free double halides.
Author contributions
Kunpeng Song: guidance of experimental projects, revision and submission of papers; Jiaojiao Gou: experiment and the writing of the draft; Lin Wu: experiment; Chunmei Zeng: design of the experimental projects.
Conflicts of interest
The authors declare that they have no known competing financial interests or personal relationships that could have appeared to influence the work reported in this paper.
Acknowledgements
This work was supported by the Key Program of Sichuan Provincial Department of Education (16ZA0176) and the Fundamental Research Funds of China West Normal University (17C037 and 15E005).
References
- J. Ran, J. Zhang, J. Yu, M. Jaroniec and S. Z. Qiao, Earth-abundant cocatalysts for semiconductor-based photocatalytic water splitting, Chem. Soc. Rev., 2014, 43, 7787–7812 RSC.
- X. Zou and Y. Zhang, Noble metal-free hydrogen evolution catalysts for water splitting, Chem. Soc. Rev., 2015, 44, 5148–5180 RSC.
- R. Schlögl, Chemical energy storage enables the transformation of fossil energy systems to sustainability, Green Chem., 2021, 23, 1584–1593 RSC.
- Z. Abdin, A. Zafaranloo, A. Rafiee, W. Mérida, W. Lipiński and K. R. Khalilpour, Hydrogen as an energy vector, Renewable Sustainable Energy Rev., 2020, 120, 109620 CrossRef CAS.
- F. Dawood, M. Anda and G. M. Shafiullah, Hydrogen production for energy: An overview, Int. J. Hydrogen Energy, 2020, 45, 3847–3869 CrossRef CAS.
- S. Liu, M. W. Heindl, N. Fehn, S. Caicedo-Davila, L. Eyre, S. M. Kronawitter, J. Zerhoch, S. Bodnar, A. Shcherbakov, A. Stadlbauer, G. Kieslich, I. D. Sharp, D. A. Egger, A. Kartouzian and F. Deschler, Optically Induced Long-Lived Chirality Memory in the Color-Tunable Chiral Lead-Free Semiconductor (R)/(S)-CHEA4Bi2BrxI10-x (x = 0-10), J. Am. Chem. Soc., 2022, 144, 14079–14089 CrossRef CAS PubMed.
- C. Xia, H. Wang, J. K. Kim and J. Wang, Rational Design of Metal Oxide-Based Heterostructure for Efficient Photocatalytic and Photoelectrochemical Systems, Adv. Funct. Mater., 2021, 31, 2008247 CrossRef CAS.
- W. Song, Y. Wang, C. Wang, B. Wang, J. Feng, W. Luo, C. Wu, Y. Yao and Z. Zou, Photocatalytic Hydrogen Production by Stable CsPbBr3@PANI Nanoparticles in Aqueous Solution, ChemCatChem, 2021, 13, 1711–1716 CrossRef CAS.
- Z. Zhang, M. Shu, Y. Jiang and J. Xu, Fullerene modified CsPbBr3 perovskite nanocrystals for efficient charge separation and photocatalytic CO2 reduction, Chem. Eng. J., 2021, 414, 128889 CrossRef CAS.
- Y. Zhao, Y. Dai, Q. Wang, Y. Dong, T. Song, A. Mudryi, Q. Chen and Y. Li, Anions-Exchange-Induced Efficient Carrier Transport at CsPbBrxCl3-x/TiO2 Interface for Photocatalytic Activation of C(sp3)-H bond in Toluene Oxidation, ChemCatChem, 2021, 13, 2592–2598 CrossRef CAS.
- L. Malavasi, L. Romani, A. Speltini, F. Ambrosio, E. Mosconi, A. Profumo, S. Margadonna, A. Milella, F. Fracassi, A. Listorti, F. De Angelis and M. Marelli, Water-Stable DMASnBr3 Lead-Free Perovskite for Effective Solar-Driven Photocatalysis, Angew. Chem., Int. Ed., 2020, 133, 3655–3662 Search PubMed.
- L. Romani, A. Speltini, C. N. Dibenedetto, A. Listorti, F. Ambrosio, E. Mosconi, A. Simbula, M. Saba, A. Profumo, P. Quadrelli, F. De Angelis and L. Malavasi, Experimental Strategy and Mechanistic View to Boost the Photocatalytic Activity of Cs3Bi2Br9 Lead-Free Perovskite Derivative by g-C3N4 Composite Engineering, Adv. Funct. Mater., 2021, 31, 2104428 CrossRef CAS.
- Y. Tang, C. H. Mak, R. Liu, Z. Wang, L. Ji, H. Song, C. Tan, F. Barrière and H. Y. Hsu, In Situ Formation of Bismuth-Based Perovskite Heterostructures for High-Performance Cocatalyst-Free Photocatalytic Hydrogen Evolution, Adv. Funct. Mater., 2020, 16(836), 2006919 CrossRef.
- A. H. Slavney, T. Hu, A. M. Lindenberg, H. I. Karunadasa and A. Bismuth-Halide, Double Perovskite with Long Carrier Recombination
Lifetime for Photovoltaic Applications, J. Am. Chem. Soc., 2016, 138, 2138–2141 CrossRef CAS PubMed.
- Z. Xiao, W. Meng, J. Wang and Y. Yan, Thermodynamic Stability and Defect Chemistry of Bismuth-Based Lead-Free Double Perovskites, ChemSusChem, 2016, 9, 2628–2633 CrossRef CAS PubMed.
- N. Hao, Y. Qiu, J. W. Lu, X. Han, Y. Q. Li, J. Qian and K. Wang, Flexibly regulated electrochemiluminescence of all-inorganic perovskite CsPbBr3 quantum dots through electron bridge to across interfaces between polar and non-polar solvents, Chin. Chem. Lett., 2021, 32, 2861–2864 CrossRef CAS.
- L. Zhou, Y. F. Xu, B. X. Chen, D. B. Kuang and C. Y. Su, Synthesis and Photocatalytic Application of Stable Lead-Free Cs2AgBiBr6 Perovskite Nanocrystals, Small, 2018, 14, 1703762 CrossRef.
- T. Wang, D. Yue, X. Li and Y. Zhao, Lead-free double perovskite Cs2AgBiBr6/RGO composite for efficient visible light photocatalytic H2 evolution, Appl. Catal., B, 2020, 268, 118399 CrossRef CAS.
- Y. Jiang, K. Li, X. Wu, M. Zhu, H. Zhang, K. Zhang, Y. Wang, K. P. Loh, Y. Shi and Q.-H. Xu, In Situ Synthesis of Lead-Free Halide Perovskite Cs2AgBiBr6 Supported on Nitrogen-Doped Carbon for Efficient Hydrogen Evolution in Aqueous HBr Solution, ACS Appl. Mater. Interfaces, 2021, 13, 10037–10046 CrossRef CAS PubMed.
- S. Kumar, I. Hassan, M. Regue, S. Gonzalez-Carrero, E. Rattner, M. A. Isaacs and S. Eslava, Mechanochemically synthesized Pb-free halide perovskite-based Cs2AgBiBr6–Cu–RGO nanocomposite for photocatalytic CO2 reduction, J. Mater. Chem. A, 2021, 9, 12179–12187 RSC.
- Y. Wang, H. Huang, Z. Zhang, C. Wang, Y. Yang, Q. Li and D. Xu, Lead-free perovskite Cs2AgBiBr6@g-C3N4 Z-scheme system for improving CH4 production in photocatalytic CO2 reduction, Appl. Catal., B, 2021, 282, 119570 CrossRef CAS.
- Y. Zhang, Z. Sun, Z. Wang, Y. Zang and X. Tao, Efficient and long-term photocatalytic H2 evolution stability enabled by Cs2AgBiBr6/MoS2 in aqueous HBr solution, Int. J. Hydrogen Energy, 2022, 47, 8829–8840 CrossRef CAS.
- Q. Huang, Y. Guo, J. Chen, Y. Lou and Y. Zhao, NiCoP modified lead-free double perovskite Cs2AgBiBr6 for efficient photocatalytic hydrogen generation, New J. Chem., 2022, 46, 7395–7402 RSC.
- N. V. Chien, H. Shin and J. Y. Song, Sn-assisted solid state crystallization of red phosphorus to black phosphorus, Scr. Mater., 2020, 177, 128–131 CrossRef.
- L. Zhang, Z.-Q. Wang, J. Liao, X. Zhang, D. Feng, H. Deng and C. Ge, Infrared-to-visible energy transfer photocatalysis over black phosphorus quantum dots/carbon nitride, Chem. Eng. J., 2022, 431, 133453 CrossRef CAS.
- F. Liu, Z. Wang, Y. Weng, R. Shi, W. Ma and Y. Chen, Black Phosphorus Quantum Dots Modified CdS Nanowires with Efficient Charge Separation for Enhanced Photocatalytic H2 Evolution, ChemCatChem, 2021, 13, 1355–1361 CrossRef CAS.
- X. Wang, J. He, J. Li, G. Lu, F. Dong, T. Majima and M. Zhu, Immobilizing perovskite CsPbBr3 nanocrystals on Black phosphorus nanosheets for boosting charge separation and photocatalytic CO2 reduction, Appl. Catal., B, 2020, 277, 119230 CrossRef CAS.
- Y. Wang, M. He, S. Ma, C. Yang, M. Yu, G. Yin and P. Zuo, Low-Temperature Solution Synthesis of Black Phosphorus from Red Phosphorus: Crystallization Mechanism and Lithium Ion Battery Applications, J. Phys. Chem. Lett., 2020, 11, 2708–2716 CrossRef CAS PubMed.
- A. Ozawa, M. Yamamoto, T. Tanabe, S. Hosokawa and T. Yoshida, Black phosphorus synthesized by solvothermal reaction from red phosphorus and its catalytic activity for water splitting, J. Mater. Chem. A, 2020, 8, 7368–7376 RSC.
- S. Zhu, Q. Liang, Y. Xu, H. Fu and X. Xiao, Facile Solvothermal Synthesis of Black Phosphorus Nanosheets from Red Phosphorus for Efficient Photocatalytic Hydrogen Evolution, Eur. J. Inorg. Chem., 2020, 773–779 CrossRef CAS.
- Y. Wu, P. Wang, X. Zhu, Q. Zhang, Z. Wang, Y. Liu, G. Zou, Y. Dai, M.-H. Whangbo and B. Huang, Composite of CH3NH3PbI3 with Reduced Graphene Oxide as a Highly Efficient and Stable Visible-Light Photocatalyst for Hydrogen Evolution in Aqueous HI Solution, Adv. Mater., 2018, 30, 1704342 CrossRef.
- R. Li, X. Li, J. Wu, X. Lv, Y.-Z. Zheng, Z. Zhao, X. Ding, X. Tao and J.-F. Chen, Few-layer black phosphorus-on-MAPbI3 for superb visible-light photocatalytic hydrogen evolution from HI splitting, Appl. Catal., B, 2019, 259, 118075 CrossRef CAS.
- Z. Zhao, J. Wu, Y.-Z. Zheng, N. Li, X. Li and X. Tao, Ni3C-Decorated MAPbI3 as Visible-Light Photocatalyst for H2 Evolution from HI Splitting, ACS Catal., 2019, 9, 8144–8152 CrossRef CAS.
- M. Zhu, Z. Sun, M. Fujitsuka and T. Majima, Z-Scheme Photocatalytic Water Splitting on a 2D Heterostructure of Black Phosphorus/Bismuth Vanadate Using Visible Light, Angew. Chem., Int. Ed., 2018, 57, 2160–2164 CrossRef CAS PubMed.
- Y. Jiang, H.-Y. Chen, J.-Y. Li, J.-F. Liao, H.-H. Zhang, X.-D. Wang and D.-B. Kuang, Z-Scheme 2D/2D Heterojunction of CsPbBr3/Bi2WO6 for Improved Photocatalytic CO2 Reduction, Adv. Funct. Mater., 2020, 30, 2004293 CrossRef CAS.
- Z. Chen, Y. Hu, J. Wang, Q. Shen, Y. Zhang, C. Ding, Y. Bai, G. Jiang, Z. Li and N. Gaponik, Boosting Photocatalytic CO2 Reduction on CsPbBr3 Perovskite Nanocrystals by Immobilizing Metal Complexes, Chem. Mater., 2020, 32, 1517–1525 CrossRef CAS.
- B. Tian, B. Tian, B. Smith, M. C. Scott, Q. Lei, R. Hua, Y. Tian and Y. Liu, Facile bottom-up synthesis of partially oxidized black phosphorus nanosheets as metal-free photocatalyst for hydrogen evolution, Proc. Natl. Acad. Sci. U. S. A., 2018, 115, 4345–4350 CrossRef CAS.
|
This journal is © The Royal Society of Chemistry 2022 |