DOI:
10.1039/C8MT00221E
(Tutorial Review)
Metallomics, 2019,
11, 85-110
Metal-dependent hormone function: the emerging interdisciplinary field of metalloendocrinology
Received
31st July 2018
, Accepted 24th September 2018
First published on 24th September 2018
Abstract
For over 100 years, there has been an incredible amount of knowledge amassed concerning hormones in the endocrine system and their central role in human health. Hormones represent a diverse group of biomolecules that are released by glands, communicate signals to their target tissue, and are regulated by feedback loops to maintain organism health. Many disease states, such as diabetes and reproductive disorders, stem from misregulation or dysfunction of hormones. Increasing research is illuminating the intricate roles of metal ions in the endocrine system where they may act advantageously in concert with hormones or deleteriously catalyze hormone-associated disease states. As the critical role of metal ions in the endocrine system becomes more apparent, it is increasingly important to untangle the complex mechanisms underlying the connections between inorganic biochemistry and hormone function to understand and control endocrinological phenomena. This tutorial review harmonizes the interdisciplinary fields of endocrinology and inorganic chemistry in the newly-termed field of “metalloendocrinology”. We describe examples linking metals to both normal and aberrant hormone function with a focus on highlighting insight to molecular mechanisms. Hormone activities related to both essential metal micronutrients, such as copper, iron, zinc, and calcium, and disruptive nonessential metals, such as lead and cadmium are discussed.
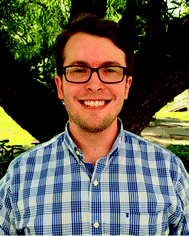
Michael J. Stevenson
| Michael Stevenson obtained a BS in Biochemistry from the University of Washington in 2009 and a PhD in Chemistry from Dartmouth College in 2016 with Dean Wilcox studying the thermodynamics of metal-protein interactions. He then trained for a year with Hannah Shafaat at the Ohio State University studying light-driven hydrogen evolution. He is currently a postdoctoral scholar in the Heffern Lab at the University of California, Davis. His current focus is to study the role of metal ions in regulating and modulating peptide hormones. |
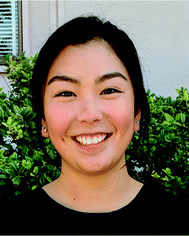
Kylie S. Uyeda
| Kylie Uyeda, originally from San Francisco, obtained a BS in Pharmaceutical Chemistry from the University of California, Davis in 2018. During her undergraduate career, she began working with Dr Marie Heffern to elucidate the effects of metal ions on the structure and function of peptide hormones. She is now continuing her work with the Heffern Lab as a Junior Research Specialist. |
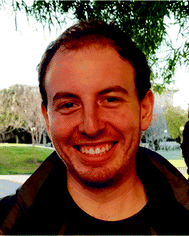
Nathaniel H. O. Harder
| Nathaniel Harder graduated with a BS in Biochemistry and Molecular Biology from the University of California, Davis in 2015. After two years working in Immunology and Rheumatology at the University of California, San Diego with Dr Nunzio Bottini and Dr Stephanie Stanford, he returned to Davis for graduate school to work for Marie Heffern. In the Heffern lab, his work is concentrated on elucidating the role of transition metal homeostasis in obesity related diseases. |
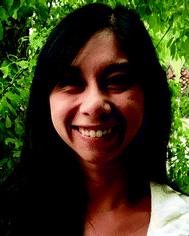
Marie C. Heffern
| Marie Heffern is an assistant professor of chemistry at the University of California, Davis, where she started in August 2017. She obtained her bachelor's degree from the University of Southern California where she researched synthetic routes to perovskite nanocrystals with Richard Brutchey. She earned her PhD at Northwestern University with Thomas Meade investigating metal complexes as protein inhibitors. She subsequently trained with Christopher Chang at the University of California, Berkeley, researching in vivo imaging probes for redox-active metals. Her research group focuses on interdisciplinary approaches within the field of bioinorganic chemistry with a particular emphasis in metals in medicine. |
1. Introduction
The endocrine system comprises a network of glands and specialized cells that secrete chemical messengers into the bloodstream or extracellular fluid. These chemical messengers, known as hormones, act on specific receptors in often distant target tissues to stimulate signaling pathways that bring about physiological changes.1 This chemical network drives both biochemical actions initiated by internal stimuli as well responses from external ones to maintain healthy homeostasis and biological rhythms.
Since Ernest Starling coined the word “hormone” in 1905, the field of endocrinology has seen impressive advances facilitated by interdisciplinary contributions from an array of fields including physiology, biochemistry, analytical chemistry, veterinary medicine, and nutrition.2 The impact of the field is reflected in the copious Nobel Prizes awarded to research in endocrinology – at least 17 in Physiology and Medicine and three in Chemistry. Some prizes reflect basic discoveries of new hormone classes such as Butenandt's discovery of sex hormones (1939) or Guillemin and Schally's shared prize for neuroendocrine hormones (1977). Other prizes have been awarded to mechanistic studies including Sutherland's prize for elucidating the mechanism of action of hormones (1971) and Gillman and Rodbell's award for understanding the function of G-proteins (1994), the predominant class of extracellular hormone receptors. Chemical advances that facilitate studies and applications of hormone biology, such as the synthesis of peptide hormones like oxytocin by du Vigneaud (1955) and Yalow's development of radioimmunoassays for studying peptide hormones (1977), have also been awarded. Finally, Nobel recognition has been given to clinical translations of hormone research, most recently to Edwards for his development of in vitro fertilization (2010).
Over a century of endocrine research has illuminated the centrality of hormone function in human health. A number of diseases have been attributed to dysfunctions in hormone regulation. One of the most prevalent examples is the connection of insulin misregulation to diabetes. Prior to the discovery of insulin, type I diabetes was a death sentence with children typically living no more than two years after diagnosis.3 The study of insulin and its therapeutic applications has drastically improved disease prognosis, prolonging life-spans with many pediatric patients surviving through adulthood. While diabetes remains a leading clinical challenge, the transformative impact of insulin exemplifies the potential value that can be gained from interrogating and dissecting hormone biology in normal physiology and diseases.
The interest in metals within endocrinology first arose from investigations of chemical agents that interfere with hormone function. Earlier work focused on heavy metals such as cadmium, lead, aluminum, and mercury as environmental toxins both in nutritional investigations and human occupational studies. Consequently, much of the research on metal–endocrine interactions has centered around their roles as endocrine disrupting chemicals.4,5 Initially, nutritional metals like zinc, selenium, and copper were classified together with toxic metals; however, mechanistic, biochemical, and structural studies have illuminated the essential roles these metals play in normal hormone function. Indeed, essential metals for life, also called metal micronutrients, consist of bulk biological elements like calcium and trace elements like zinc, iron, and copper. Metal micronutrients are required in small amounts to maintain the health of the organism and can range from milligram to gram per kilogram of body weight.6 These metals must be dietarily obtained in sufficient amounts and are tightly regulated for proper function and utilization, as their misregulation can cause adverse effects in nutrition and health.7 On the other hand, heavy metals which are also called nonessential metals and classified by the absence of deficiency symptoms in dose–response curves, such as those found in periods five and six of the periodic table, are toxic in small amounts.6,8 There is little to no evidence that these heavy metals serve a role in biological systems, and environmental exposure to these metals may interfere with normal metabolic processes by disrupting interactions with metal micronutrients.9,10 While a large volume of research has studied the toxicity of both nutritionally essential and nonessential metals in the environment, emerging work is uncovering their critical roles in regulating a number of biological processes from brain function to immune responses.
Pathologies of genetically-induced overloads have informed on detrimental effects associated with metal miscompartmentalization or overload. For instance, in thalassemia, a genetic blood disorder that induces iron overload, the leading cause for morbidity is endocrine dysfunction manifesting as hypogonadism, hypothyroidism, diabetes, growth failure, or pubertal delay.11 In many of these cases, excess iron tissue deposition precedes endocrine failure by several years. Patients of Wilson's disease, which results in excess copper in the liver, suffer from metabolic dysfunction, including hypoglycemia and fatty livers.12 In some cases, metals like copper and iron can also be accumulated in the mitochondria, resulting in disease states predominantly through generation of reactive oxygen species.13 Yet it was through another inherited iron disorder, hereditary hemochromatosis, that hepcidin, an iron-regulatory hormone, was discovered.14
Micronutrient deficiencies of essential metals have shed light on the necessity of metals in hormone-associated processes. Studies with copper-deficient diets have uncovered the metal-dependence of carboxypeptidases involved in peptide hormone maturation.15 Nutritional zinc deficiencies have long been linked with adverse effects on growth and development.16 Copper and iron deficiencies have been investigated for connections to thyroid hormone function and temperature regulation.17,18
A wealth of opportunities is available in bridging interdisciplinary endocrine research with bioinorganic perspectives, particularly in connecting molecular understanding with physiological studies. Our group is seeking to establish an area of research that brings these two areas together, which we term “metalloendocrinology.” The goal of this tutorial review is to harmonize the diverse, yet in some cases, disparate, literature reports to illuminate growing connections between metal biology and endocrinology. In this review we highlight works that have elucidated direct interactions of hormones with metal micronutrients and their effects on biological activity, as well as studies that have untangled indirect influences of metal micronutrients on hormone function and regulation. Despite the long history since the initial discovery of peptide hormones, connections at the molecular level between metal ions and the endocrine system remain in its infancy. Fig. 1 describes the timeline of advances in endocrinology and metal micronutrient interactions with hormones as covered in this review. The discussion herein is limited to metals regulated by mammalian hormones, and while there is an increasing amount of research into plant and amphibian metal regulating hormones,19–22 the complexity of the different systems leads us to restrict the scope of this tutorial review. We have selected examples that spotlight how the interplay of different research areas can provide understanding or hypotheses for molecular-level pictures of hormone–metal interactions.
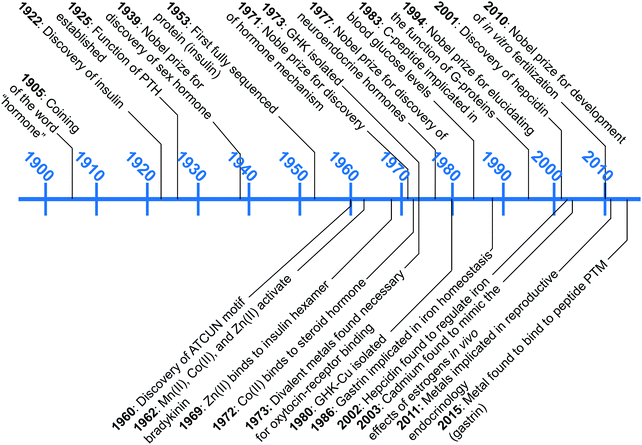 |
| Fig. 1 A snapshot of major endocrinological discoveries (top) and metal–hormone interactions (bottom) over the past 100 years as detailed in this review. | |
2. Overview of hormone biology and metalloendocrinology
At the early stages of endocrinology, researchers studied hormone biochemistry primarily through isolated organ systems.2 Consequently, hormones were first defined by the glands from which they were secreted. However, as research in this area has advanced, it has now become clear that distinct hormones can be secreted by organs and can even elicit different functions based on their tissue targets. An alternative classification of hormones has since been implemented that is based on their chemical characterization.23 Based on this classification, there are three different structural categories of hormones: peptide/protein hormones derived from amino acids, steroid hormones derived from cholesterol, and phospholipid hormones derived from fatty acids. To tie structure to function, hormones may be further classified based on their synthesis, axis (i.e.ref. 5, 9, 10 for digestive, reproductive, etc.), or method and location of storage and release. Functional classification, however, can itself be limited, as many hormones have been implicated in multiple axes, are released from various cell types, act on multiple tissue types, and interact with other hormones and signaling molecules.
Given the diversity and complex nature of hormones, we have classified hormones in this review by functions that highlight commonalities in their interactions with metal micronutrients; but it should be noted that many of the hormones described here participate in functions beyond this categorization. The examples that we have provided widely focus on the interactions of metals with peptide and protein hormones, as mechanistic investigations of their bioinorganic components are most prevalent in literature. Table 1 summarizes the reported metal interactions with peptide/protein hormones that are detailed in this review. Metal micronutrients have the potential to interact with the peptide hormones at many phases during their maturation. Fig. 2 depicts the biosynthesis, secretion, and action of a typical peptide/protein hormone,23,24 and demonstrates the various stages at which metals have been speculated or reported to directly interact in their biology. Peptide and protein hormones are first transcribed as longer sequences called preprohormones, which are then targeted to the endoplasmic reticulum. The signaling sequence is cleaved off upon entering the endoplasmic reticulum yielding the prohormone. There, the prohormone is directed to the Golgi apparatus for processing by peptidases that refine the prohormone into the mature hormone. We would be remiss not to mention the post-translational modifications that the peptide may undergo. The peptide hormone may be subjected to post-translational modifications from a wide range of peptidases including but not limited to peptidylglycine alpha amidating monooxygenase, a copper dependent peptidase, and other metal-dependent carboxypeptidases.25–29 Post-translational modifications are essential for the function of peptides and offer additional complexity to the possible binding interactions between metals and peptides, such as has been observed with the gut hormone gastrin.30 After processing in the Golgi apparatus, the hormone is packaged into secretory vesicles for export from the cell, a process called exocytosis. Upon receiving the signal for secretion, the hormone is released into the gastrointestinal or vascular system. The hormone travels throughout the system to specific target tissue, binds to an extracellular receptor, and initiates a signaling cascade. Throughout synthesis, maturation, secretion, and receptor binding, metals may potentially influence hormone activity. Initially, certain metals may bind DNA or transcription factors and facilitate or inhibit transcription.31 Based on published literature about peptide–metal interactions, it is speculated that upon translation, or upon entering the endoplasmic reticulum, Golgi apparatus, or secretory vesicles, metals may directly bind to hormones impacting their stability and processing.32 Metal binding may also influence hormone conformation after secretion, enabling or inhibiting biological activity.33 Finally, metals may play a role in binding to the hormone receptor by facilitating the hormone–receptor interaction.34 Even through the lens of the established paradigm of polypeptide hormone synthesis and activity, metal binding adds new levels of complexity to our understanding of the endocrine system.
Table 1 Peptide/protein hormones that exhibit direct and/or indirect hormone–metal interactions, as detailed in this review. Hormone is classified as the mature form. Sequence is in single letter amino acid abbreviations. Underlined residues indicate known or potential metal binding sites. Asterisks indicate hypothesized metals and targets
Classification |
Hormone |
Sequence |
Direct metal interaction |
Indirect metal effects |
Origin |
Target |
Mineral regulation |
PTH |
SVSEIQLMHNLGKHLNSMERVEWLRKKLQDVHNF |
Zn(II) |
Ca(II) |
Parathyroid gland |
Kidney, bone, intestines |
Hepcidin |
![[D with combining low line]](https://www.rsc.org/images/entities/char_0044_0332.gif) ![[T with combining low line]](https://www.rsc.org/images/entities/char_0054_0332.gif) FPICIFCCGCCHRSKCGMCCKT |
Cu(II), Ni(II), Zn(II) |
Fe(III) |
Liver, adipose tissue, macrophages |
Liver, duodenum, macrophages |
|
Metabolic regulation |
Insulin A-chain |
GIVEQCCTSICSLYQLENYCN |
Zn(II), Ca(II) |
Unknown |
Pancreas |
Whole organism |
Insulin B-chain |
FVNQHLCGS LV ALYLVCGERGFFYTPKT |
C-Peptide |
EAEDLQVGQVELGGGPGAGSLQPLALEGSLQ |
Zn(II)*, Fe(II)*, Cr(III)* |
Unknown |
Pancreas |
Whole organism* |
Amylin |
KCNTATCATQRLANFLV![[H with combining low line]](https://www.rsc.org/images/entities/char_0048_0332.gif) ![[S with combining low line]](https://www.rsc.org/images/entities/char_0053_0332.gif) NNFGAILSSTNVGSNTY |
Cu(II), Zn(II) |
Unknown |
Pancreas |
Whole organism |
Glucagon |
HSQGTFTSDYSKYLDSRRAQDFVQWLMNT |
Unknown |
Ca(II), Zn(II) |
Pancreas |
Liver, pancreas |
Little gastrin |
QGPWLE![[E with combining low line]](https://www.rsc.org/images/entities/char_0045_0332.gif) ![[E with combining low line]](https://www.rsc.org/images/entities/char_0045_0332.gif) EAYGWMDF |
Fe(III), Ca(II) Bs(III) |
Zn(II) |
Stomach, duodenum, pancreas |
Stomach, intestines |
CCK |
D MGWMDF |
Fe(III), Ca(II) |
Unknown |
Stomach, duodenum, pancreas |
Gallbladder, unknown central nervous system |
|
Reproductive hormones |
LH α-subunit |
VQDCPECTLQENPFFSQPGAPILQCMGCCFSRAYPTPLRSKKTMLVQKNVTSESTCCVAKSYNRVTVMGGFKVENHTACCSTCYYHLS |
Cu(II), Ni(II) |
Mn(II), Cu(II), Cd(II) |
Anterior pituitary |
Gonad |
LH β-subunit |
GLLLLLLLSMGGAWASREPLRPWCHPINAILAVEKEGCPVCITVNTTICAGYPTMMRVLQAVLPPLPQVVCTYRDVRFESIRLPGCPRGVDPVVSFPVALSCRCGPCRRSTSDCGGPKDHPLTCDHPQLSGLLFL |
Oxytocin |
![[C with combining low line]](https://www.rsc.org/images/entities/char_0043_0332.gif) ![[Y with combining low line]](https://www.rsc.org/images/entities/char_0059_0332.gif) ![[I with combining low line]](https://www.rsc.org/images/entities/char_0049_0332.gif) N P![[L with combining low line]](https://www.rsc.org/images/entities/char_004c_0332.gif) ![[G with combining low line]](https://www.rsc.org/images/entities/char_0047_0332.gif) |
Zn(II), Cu(II), Mg(II), Ni(II), Mn(II), Co(II) |
Unknown |
Hypothalumus |
Smooth muscle in mammary gland and uterus |
|
Growth, wound healing, inflammation |
hGH |
FPTIPLSRLFDNAMLRA RL QLAFDTYQEFEEAYIPKEQKYSFLQNPQTSLCFSESIPTPSNREETQQKSNLELLRISLLLIQSWLEPVQFLRSVFANSLVYGASDSNVYDLLKDLEEGIQTLMGRLEDGSPRTGQIFKQTYSKFDTNSHND DALLKNYGLLYCFRKDM KVETFLRIVQCRSVEGSCGF |
Zn(II) |
Unknown |
Anterior pituitary |
Whole organism |
GHK |
![[G with combining low line]](https://www.rsc.org/images/entities/char_0047_0332.gif) ![[H with combining low line]](https://www.rsc.org/images/entities/char_0048_0332.gif) ![[K with combining low line]](https://www.rsc.org/images/entities/char_004b_0332.gif) |
Cu(II) |
Fe(III), Fe(II), Zn(II) |
Collagen |
Whole organism |
Bradykinin |
RPPGFSPFR |
Cu(II), Zn(II) |
Mn(II), Co(II), Zn(II) |
Unknown |
Vascular endothelial cells |
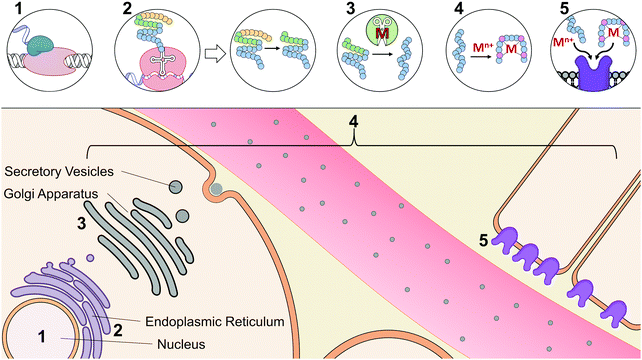 |
| Fig. 2 The central dogma of a metal binding peptide hormone: synthesis, maturation and action of protein and peptide hormones and proposed interactions of metals in this pathway. (1) First, the hormone associated gene is transcribed. (2) The preprohormone is translated in the ER and the signaling sequence (yellow) is cleaved yielding a prohormone. (3) The prohormone is further cleaved to arrive at the mature hormone (blue). (4) Metals can bind the hormone and impact structure, bioactivity, and circulation lifetime and stability. This interaction can occur throughout the maturation of a metal binding peptide from as early as translation to the binding of the peptide with its target. (5) Hormones bind their target receptor to initiate signaling cascades. Metal binding can change the conformation of a hormone to affect the affinity for its receptor or directly facilitate hormone–receptor complex formation. | |
3. Hormones that regulate metals
As metals are not biologically synthesized, they must be acquired, compartmentalized, distributed, and regulated for proper utilization.35 At a cellular level, research has revealed metal importers, exporters, chaperones, and storage proteins that help maintain mineral homeostasis and mobilize them for use when needed.36 Yet, organ–organ communication and control of these metals offer a number of open questions. Understanding the factors that regulate circulating and tissue-specific levels of metals is an ongoing challenge on account of the dearth of strategies to both monitor and control metal metabolism in tissue-specific ways. Despite these complexities, two hormones have been found to directly control metal metabolism: parathyroid hormone, which regulates calcium, and more recently, hepcidin, which regulates iron. These examples offer possible approaches and strategies that could facilitate the discovery of other metal effectors.
Parathyroid hormone (PTH)
Parathyroid hormone (PTH) was the first hormone discovered to regulate metal metabolism. The 84-amino acid hormone was first uncovered in the context of trying to understand the physiological role of the parathyroid gland.37 It was found that removal of parathyroid glands resulted in death by tetany.38 MacCallum and various coworkers demonstrated that parathyroid glands control blood calcium levels, as oral administration of large doses of calcium lactate could prevent tetany.39,40 Specifically, hypocalcemia was documented as the true explanation for tetany.38 Still, the role of PTH was highly debated until a definitive study in 1925 established the parathyroid as an endocrine gland which secretes PTH,41 leading researchers to begin studying PTH pathophysiology. Clinical investigations revealed that, in addition to PTH deficiency, PTH resistance (a.k.a. pseudohypoparathyroidism), could cause hypocalcemia, a disease in which bones are demineralized. On the other hand, enlargement of parathyroid glands and excess secretion of PTH were associated with osteomalacia, another disease in which the bones are demineralized.42 In the 1970s a handful of groups43–45 determined the structure and biologically-active portion of bovine, porcine, and human PTH, making a highly purified, active synthetic PTH polypeptide available for molecular and cellular biology assays. The successful cloning of the PTH receptor in 199146,47 lead to deeper understanding of the physiological role of PTH in multiple target tissues both in vitro and in vivo.
PTH regulates calcium levels in the blood via a negative feedback loop through its actions on the kidneys, bones, and intestines. In the kidney, PTH increases vitamin D synthesis and calcium absorption, regulates renal calcium and phosphate transport, and lowers the amount of urinary calcium excreted.47–50 PTH also acts on various specialized bone cells to mobilize calcium stores. Most recently, PTH has been studied and applied as a bone mass restoration therapy for patients with osteoporosis.37,51
Abnormalities in levels and metabolism of metal micronutrients beyond calcium may be correlated to PTH levels, particularly in relation to children's growth in stature,52 as well as the occurrence of some chronic diseases, such as chronic renal failure (CRF)53 and bone fragility.54 Of interest has been the relationship of PTH specifically with zinc. Research supports a significant negative correlation between zinc and serum levels of PTH.53 It has also been suggested that zinc deficiency increases serum concentrations of PTH through a decrease in serum calcium in rats.54 To further assess the influence of zinc on PTH activity, Carter et al. installed a metal-binding motif at position 10 of rat PTH. This modification resulted in a dramatic eight-fold increase in the zinc-induced potency as compared to the activity of the derivative in the absence of metal, suggesting the formation of a ternary complex between PTH, Zn(II), and the extracellular loop/transmembrane domain region of the PTH-1 receptor.55 Most recently, PTH has been studied for its therapeutic potential in treating bone diseases.56
In addition, heavy metals, specifically lead or cadmium, interact with the metabolism of metal micronutrients such as calcium, zinc, and iron, which, in turn, may be correlated to PTH levels. Costume jewelers who were regularly exposed to high levels of lead showed significantly increased PTH levels, suggesting that lead exposure affects calcium metabolism and impairs the synthesis of calcitrol.57 Lead and cadmium exposure have also been shown to stunt bone growth in children, most likely by causing kidney and osteoblast/osteoclast dysfunction.52 Emerging work is uncovering effects of toxic elements on vitamin D and essential minerals, which are essential for bone growth and maintenance.58
Calcium regulation is further facilitated by calcitonin, a hormone that opposes the action of PTH.59 Calcitonin reduces blood calcium levels by inhibition of bone-degrading osteoclasts, as well as by decreasing resorption of calcium in the kidneys. The importance of its role remains unclear, as patients with abnormal calcitonin levels show no adverse effects. Research remains active in understanding its structure, biosynthesis, physiology, pathophysiology, and pharmacology.60
Hepcidin
Hepcidin is considered the master regulatory hormone for iron metabolism and tissue distribution.61,62 To the best of our knowledge, hepcidin is the only peptide hormone known to directly regulate a transition metal. It was independently discovered in the early 2000's by Krause et al.63 and Park et al.64 in studies of antimicrobial peptides in bodily fluids. Its name stemmed from an abbreviation of hep(ato)- to indicate its production in the liver, and -cidin referring to its natural antibiotic properties. Though of initial interest as an antimicrobial agent, researchers linked HEPC, the gene that codes for hepcidin, to iron overload disorders,65,66 illuminating hepcidin as the signaling molecule for iron homeostasis. Since then, research has surged in this area, offering expanding insight into its role in iron metabolism, connection to acute processes like inflammation,67 and potentially as a ‘real time’ indicator of iron need.68
Hepcidin is a highly conserved 25-residue peptide that is rarely found with random mutations and lacks iron-related gene sequence motifs, which most likely explains why it was historically elusive.67 It contains eight cysteines which are oxidized when isolated from the body and form a distorted β-sheet between residues 7 and 23 while the N-terminus remains flexible.69 It is unclear if the fully oxidized, fully reduced, or mixed species elicits its biological function. Its molecular target is ferroportin, the only vertebrate iron exporter identified to date.70 Hepcidin is expressed and released into the bloodstream in response to changes in plasma iron and liver iron stores,71 erythropoiesis,72 inflammation,73 infection,74 and hypoxia.75 In the case of inflammation, production of hepcidin increases which drastically alters iron homeostasis and decreases serum iron levels. This decrease in serum iron, or hypoferremia, may aid the host in resisting infection.67,73,76,77 While primarily produced in the liver, accumulating data suggests that smaller amounts may be secreted by other cell types such as adipose tissue and macrophages.78 It impairs iron export at a number of tissue targets including duodenal enterocytes, macrophages, and hepatocytes.78,79 At the target tissues, hepcidin binds to ferroportin and promotes internalization of the complex and subsequent degradation. Consequently, iron export is decreased, increasing iron retention in the cell and lowering overall plasma iron levels. The established roles and clinical relevance of the hepcidin–ferroportin axis in iron homeostasis and utilization has been extensively studied and reviewed.73,80–83 Interestingly, it was recently found that leptin, a peptide hormone that is secreted by adipose tissue and responsible for regulating appetite, energy metabolism, and cardiovascular functions, has a direct effect on serum hepcidin levels by upregulating hepcidin expression and, by extension, iron metabolism.84 Current research actively seeks to elucidate the unclear molecular mechanisms underpinning hepcidin regulation of inflammation, erythropoiesis, sex hormones, and growth factors, as well as iron sensing mechanisms that govern hepcidin stimulation.79
Variants of the full hepcidin (hepcidin-25) have been isolated from biological fluids with N-terminal truncations: hepcidin-24, -23, -22, and -20. The biological functions and origins of these isoforms are unknown, but they may have pathological significance. It has been shown that these isoforms exhibit decreased activity in ferroportin internalization85 and increased levels of these isoforms along with hepcidin-25 have been observed in chronic kidney disease and sepsis.85–88 In a structure–function study, Nemeth and coworkers found that the five N-terminal residues are essential for activity.89 The authors systematically modified hepcidin with truncations and additions at both termini and removal of various disulfide bonds. By monitoring ferroportin internalization and serum iron levels, they correlated progressive N-terminal truncation to decreased activity. Addition of an N-terminal Ala, changes to the C-terminus, and alteration of the disulfide bond pattern did not substantially affect bioactivity, pointing to the crucial role of the N-terminal residues. Interestingly, shortened derivatives containing the first three or six amino acids alone did not induce internalization or decrease serum iron levels nor compete with native hepcidin activity. These results indicate that the five N-terminal residues are essential and require attachment to the remaining distorted β-sheet core for its ferroportin-associated activity.89
Located within this N-terminal region of hepcidin is a consensus sequence at residues 1–3 (Asp1-Thr2-His3) corresponding to an amino-terminal Cu(II) and Ni(II) (ATCUN) binding motif.90 N-Terminal truncations that eliminate function also remove this ATCUN motif, supporting the requirement of this motif for proper hormone function. Mass spectrometry and spectroscopic studies showed that this site exhibited high affinity for Cu(II) and suggests coordination to the N-terminal amine of Asp1, two backbone amides, and the π-nitrogen of His3 in a distorted square planar geometry, resembling the binding mode of human serum albumin (HSA) (Fig. 3A and B).91–93 Tselepis et al. characterized hepcidin complexes with Cu(II), Ni(II), and Zn(II) by electrospray ionization mass spectrometry (ESI-MS) and estimated the association constant of Cu(II)–hepcidin ≫ 106,93 which correlates with other ATCUN motifs. Interestingly, despite its major role in iron regulation, there is little data to suggest that Fe(II) or Fe(III) bind directly to hepcidin, and iron sensing mechanisms related to its stimulation remain unclear.93
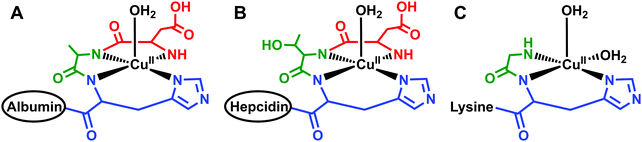 |
| Fig. 3 Schematic models of equatorial Cu(II) bound to protein or peptides with similar geometries. (A) Cu(II) bound to the N-terminus of human serum albumin;249 (B) Cu(II) bound to the N-terminus of hepcidin;92 and (C) Cu(II) bound to the N-terminus of glycine, backbone amide, and histine of the tripeptide GHK where the lysine does not participate in binding at physiological pH.242 Only the first three residues of human serum albumin and hepcidin are shown and the remaining structure is simplified to the ovals. The structures for human serum albumin, hepcidin, and GHK were modeled from Hureau et al., Kulprachakarn et al., and Pickart et al., respectively. | |
The presence of the ATCUN motif among other observations may suggest interplay between iron homeostasis and systemic regulation of other metals. Brasse-Lagnel et al. suggest that increased levels of hepcidin signals degradation of the divalent metal transporter 1 (DMT1),94 which exhibits a broad substrate profile beyond iron.14 There is also evidence to suggest hepcidin regulates zinc levels where incubation of Caco-2 cells with hepcidin resulted in upregulation of metallothionein and the zinc importer ZIP14 along with downregulation of the zinc exporter ZnT1.95 Crosstalk of metal homeostasis is not surprising given other hypotheses of metal homeostasis interplay (e.g. Cu(II) and Zn(II) with Atox1).96 Elucidation of interactions between hepcidin, iron, and other metals will provide the missing links within the complexities of global metal homeostasis.
4. Hormones in metabolic regulation
The gastrointestinal tract utilizes a network of hormones secreted by the enteroendocrine cells of the stomach, pancreas, and small intestine to facilitate digestion upon food intake. Through complex feedback loops, these hormones regulate one another and stimulate target cells to metabolize and balance nutrients, promoting uptake, storage, and energy utilization (Fig. 4). The enteroendocrine hormones also synergize to respond to ingested toxins by initiating appropriate immune responses.97 With the rise of diet-related metabolic disorders such as liver disease and diabetes, there has been considerable interest in understanding and controlling the enteroendocrine system for the treatment and diagnosis of these health problems. Moreover, emerging connections between neural regulation and digestion have sparked studies on how these hormones contribute to interconnectivities of appetite control, nutrient sensing, emotions, and stress.98
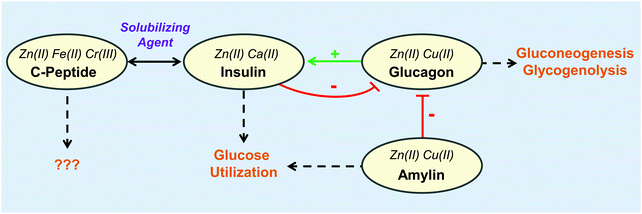 |
| Fig. 4 The interplay of metal binding peptides in the pancreas. Metals shown to impact each peptide are indicated in italics. The metals further increase the complexity of the interplay between peptides and metals. Physiological responses shown by dashed arrows. For more information regarding the role of the peptide and its corresponding metals, refer to Table 1. | |
Of the organs in the gastrointestinal system, the role of zinc in the pancreas is perhaps the most well-studied within the area of metalloendocrinology on account of the high concentrations of these ions in secretory granules.99,100 As such, the majority of this section describes the interactions of metals with pancreatic hormones (Fig. 4). While less studied, it is no less intriguing to consider the role metals might play in the function of other digestive hormones. Upon ingestion, metals, like other nutrients, first encounter the enteroendocrine cells. A large portion of these metal micronutrients are transported to the liver, which regulates the trafficking and distribution of metal micronutrients to the rest of the body.101 As might be expected, hepatic and enteroendocrine cells exhibit metal micronutrient levels that are up to four-fold higher than in most other organs as in the case of zinc, iron, and copper.100,102–104 Dietary metal deficiencies and misregulations have been associated with a number of metabolic disorders.9 In addition to the direct metal interactions with hormones, metals themselves have been implicated in regulatory roles. Vanadium complexes, for instance, have received notable attention for their antidiabetic properties and their ability to mimic insulin.105–107 Copper has also been found to modulate brain–gut interactions by regulating the secondary messenger cyclic AMP thereby affecting lipolysis108 and norepinephrine–noradreniline signaling.109 However, the focus of this review is on metal micronutrients and their interactions with peptide hormones. This prompts speculations that metals may be associated with the function of other digestive hormones, and further investigations in this area may offer novel mechanisms for micronutrient-associated pathologies and therapies.
Insulin
Insulin is one of the most well-studied peptide hormones whose discovery dates to 1922 by a Toronto group of Frederick Banting, Charles Best, James Collip and John Macleod.110 Within months of its discovery and isolation, insulin was administered to its first patients. The three decades following saw intensive research on understanding the physiochemical and pharmacological properties of the hormone. During this time, insulin was successfully crystallized, and disulfide bonds were identified that linked the A- and B-chains to form a monomer.110 Later, four papers were published between 1951 and 1953 by Sanger and colleagues determining the primary sequence of insulin, making it the first protein to have its full sequence determined.110–114 The next two decades led to the discovery of the insulin receptor (IR) and conformational changes associated the insulin–IR interaction.110
Insulin is released from pancreatic β-cells into the bloodstream when blood glucose levels, in part, are elevated, subsequently signaling other tissues to absorb or utilize glucose for glycogen synthesis or fat storage.115 Destruction or misregulation of the glucose–insulin pathway causes either type 1 or type 2 diabetes.116 In type 1 diabetes, little insulin is produced because pancreatic β-cells are destroyed by the autoimmune system.117 On the other hand, type 2 diabetes is initiated as resistance to insulin in target tissues.118,119 Purified insulin is administered as a treatment for diabetic patients to counteract this pathology. With the increasing prevalence of diabetes, insulin is one of the most-prescribed peptide therapeutics.110
Perhaps one of the quintessential examples of interactions between metals and hormones is that between Zn(II) and insulin. Earlier studies found that insulin formulations that were stabilized with Zn(II) and protamine exhibited slower effects while still exerting the same action.120 When the three-dimensional structure of the insulin hexamer was determined in 1969 by Dorothy Hodgkin and coworkers, two Zn(II) ions were found stabilizing the hexamer formation, each was found to bind to three monomeric B-chain His10.121 Additional studies with Cd(II), a Zn(II) surrogate, indicated up to three equivalents of Cd(II) could bind to the insulin hexamer.122 Further studies by Sudmeier et al. used 113Cd(II) nuclear magnetic resonance (NMR) to determine that the third Cd(II) was binding in a glutamic acid core formed by six B-chain Glu13 residues, and addition of Ca(II), which is in high concentrations in pancreatic β-cells, could displace the Cd(II) from that site.123 Within the secretory vesicles of pancreatic β-cells, insulin, Zn(II), and Ca(II) are at millimolar concentrations.124–127 It is now well-agreed that the hexamer biologically forms with two His-bound Zn(II) sites and one Glu-bound Ca(II).99
Researchers have sought to investigate the dynamics of metal-mediated insulin maturation at the molecular level. Insulin can self-associate into dimers at micromolar concentrations independently of metal binding. Coffman and Dunn126 and Kadima and coworkers128 showed that the monomeric and dimeric insulin do not have high affinities for Zn(II) or Ca(II) at the B-chain His10 or Glu13 positions, respectively. As insulin concentration increases, the distribution of species further diversifies to include tetramers, or dimer of dimers, whose association constant is ≈200 μM. Given the millimolar concentration of insulin in secretory vesicles, the tetramer would become physiologically relevant and upon its formation, Zn(II) and Ca(II) binding have affinities in the low micromolar range.126 Addition of the third dimer creates two Zn(II) binding sites composed of three His10 residues at each end of the hexamer and one Ca(II)-binding site from the six-glutamate core. The role of Zn(II) in insulin hexamerization in the secretory vesicles is further supported by identification of ZnT8 on the membranes of the vesicles. Zn(II)-specific imaging agents have shown that ZnT8 can pump Zn(II) from the cytosol into the vesicles to achieve millimolar concentrations of Zn(II).110
Since Zn(II) and Ca(II) are spectroscopically silent, many studies have used spectroscopically-active metal ions as Zn(II) analogues, such as Co(II) for UV-Vis spectroscopy and Cd(II) for 113Cd(II) NMR spectroscopy, to probe the metal binding site.99,123,126,128–130 Quantification of binding parameters have been complicated by the complexity of the system which has its native primary, secondary, and tertiary structures, in addition to a Zn(II)-mediated quaternary structure. Recently, Carpenter and Wilcox131 performed the first quantitative thermodynamic analysis of the metal-mediated quaternary system. They determined that the insulin hexamer requires 2.5 protons for assembly and suggest a mechanism for hexamer stabilization in the acidic secretory vesicles and destabilization upon insulin release into the bloodstream where the pH increases to 7.4. In addition, Pertusa et al.132 have recently shown that serum albumin may chelate Zn(II) away from the insulin hexamer upon release from the pancreas thereby expediting its dissociation into monomer form.
Upon stimulation of insulin release, insulin is believed to dissociate from the metal ions and adopt monomeric form, and Zn(II) and Ca(II) are co-secreted in their ionic forms. Until recently, the role of this secreted Zn(II) was neglected. A renewed focus, however, has been placed on the role of Zn(II) on insulin activity outside of the pancreas with possible evidence suggesting that Zn(II) can serve as suppressor of IR-induced insulin uptake at target tissues.33 Tamaki et al. showed that mice with ZnT8 knocked out (thus reducing concentrations of Zn(II) in pancreatic secretory vesicles) exhibited reduced endocytosis of insulin in the liver,133 one of the target organs of insulin secretion. This was further corroborated in cell culture studies wherein incubation of hepatocytes with Zn(II) inhibited insulin uptake. IR inhibition is unknown, but literature suggests two possible mechanisms.33 In one scenario, increased concentrations of Zn(II) can stabilize the insulin hexamer in plasma thereby preventing the insulin monomer from interacting with the IR. In the other, Zn(II) may bind directly to the His710 residue and either Glu or Asp in the IR in a tetrahedral coordination and directly compete with insulin.33
Many questions still remain regarding the extrapancreatic roles of secreted Zn(II) and have been detailed by O’Halloran et al.33 Further insight is needed to understand the role of Zn(II) in the IR binding and possible regulation of the insulin–IR complex. This knowledge will produce a more detailed picture of how insulin and Zn(II) work synergistically in the glucose–insulin pathway.
C-Peptide
As the prohormone proinsulin matures in the secretory vesicle it is cleaved into C-peptide and the A- and B-chains of insulin. Initially thought to be biologically inert,134 strong evidence suggests a physiological role for C-peptide as a solubilizing agent for insulin.135,136 Additional hormone-like roles have been suggested for C-peptide after its secretion, including blood flow regulation possibly via stimulation of ATP release from erythrocytes,137–139 glucose transport,140,141 activation of MAPK-dependent pathways,142,143 and stimulation of Na+,K+-ATPase and eNOS activities.143,144 While mechanisms for these activities are not known, prevailing hypotheses suggest C-peptide either binds to an achiral receptor145 or G-protein coupled receptor (GPCR),146 or is internalized by the cell.147 The transportation of C-peptide through blood may be facilitated by Zn(II) and HSA.148
Studies have suggested that complexation of the peptide with a metal ion, particularly Zn(II), Fe(II), or Cr(III) is necessary for activity. The Spence lab has used ESI-MS to show that Zn(II), Fe(II), and Cr(III) bind to C-peptide and that Zn(II) binding at four conserved Glu residues is necessary, but mutation of one Glu to Ala decreases, but does not obliterate, Zn(II) binding. C-peptide adducts of Fe(II) and Cr(III) have been shown to increase the release of ATP from erythrocytes with concomitant increase of glucose transport through GLUT1, whereas apo C-peptide has no effect on ATP release,139,140 where Cr(III) may have additional insulinomimetic effects as in the case of chromodulin.149,150 Meyer et al. interestingly found that Zn(II) also stimulates ATP release when bound to C-peptide in healthy erythrocytes, but erythrocytes harvested from type 2 diabetic rats had a resistance to Zn(II)–C-peptide.151 When the Zn(II)–C-peptide complex was administered to erythrocytes in conjunction with metformin, an insulin sensitizer, it led to restoration of ATP production and release. Based on these observations, the authors suggested that Zn(II)–C-peptide acts independently of the insulin pathway.151 C-Peptide treated with Ca(II) and Zn(II) has also been shown to stimulate the direct and indirect production of nitric oxide in endothelial cells through activation of the eNOS pathway and possibly through purinergic signaling, respectively.144,152 In addition, C-peptide can activate Na+,K+-ATPase in the presence of Ca(II) which, in combination with enzymes in the eNOS pathway, are deficient in tissues of individuals with type 1 diabetes.143,144 Taken together, growing evidence suggests that C-peptide may function as a peptide hormone that is targeted, specific, and requires metal ions for activation. Understanding the structure of C-peptide complexed with the activating metal ions and cellular receptors could positively impact the therapeutic potential of this peptide.
Amylin
Amylin, or islet amyloid polypeptide (IAPP), is a 37-residue amidated peptide hormone co-expressed and co-released from pancreatic β-cells with insulin in a 1
:
10–100 amylin
:
insulin ratio.153–155 It functions as a synergistic partner to insulin in glucose regulation by suppressing glucagon secretion from pancreatic α-cells, slowing gastric emptying, and promoting appetite suppression via interactions with calcitonin receptors that are modified by receptor activity modifying proteins (RAMPs).156,157 Human amylin comprises three distinct regions: the membrane disrupting region (residues 1–19), amyloidogenic region (20–29), and the C-terminal region believed to enhance its amyloidogenicity.158 The amyloidogenic region drastically increases the propensity of amylin fibril formation in β-cells, which is linked to oxidative stress and cell death.159 These fibrils are observed in approximately 95% of patients with type 2 diabetes, making its understanding a pressing issue in therapeutic and diagnostic approaches for the disease.160,161 Of note is the difference between human and rat amylin sequences. Rat amylin is non-amyloidogenic because it has proline variations in the amyloidogenic region which causes kinks in the secondary structure. These kinks are thought to disrupt β-sheet aggregation.162
In a similar fashion to the proline residues of rat amylin, metal ion coordination is proposed to disrupt amyloidogenicity of amylin.163–165 Inside of the secretory vesicles where amylin is stored, metal ions are posited to play protective roles by stabilizing the peptide and preventing amyloidogenesis.32,163,166–168 In addition to amylin fibril formation, the β-cells in patients with type 2 diabetes exhibit metal ion dyshomeostasis, suggesting a possible connection between the two phenomena.160,161 One such metal that has altered cellular concentrations is Zn(II). In solution, low Zn(II) concentrations increase the lag-time of amylin fibrillization and decrease the rate of monomer addition to preformed fibrils, whereas high concentrations have the opposite effect.163 On the one hand, several studies have attributed the increased lag-time to Zn(II) binding to His18, which can introduce a kink in secondary structure near the amyloidogenic region similar to the effect of proline in rat amylin.163–165 Moreover, Zn(II) binding introduces cationic character to a largely hydrophobic portion of the peptide, potentially disrupting fibrillization.163 On the other hand, Zn(II)-induced fibrillization at high concentrations has been attributed to formation of a prefibrillar intermediate through coordination of His18 and the N-terminal amine of Lys1.158 It is worth noting that these studies were performed at pH ≈ 7 which has about 30-fold fewer protons than in the secretory vesicle. Within the secretory vesicle where pH ≈ 5.5, His18 would already be partially protonated and introduce a partial positive charge to amylin that may disrupt fibrillization leading to a reduced role for Zn(II) to prevent amyloidogenesis.163 The few studies with Zn(II) that have been performed at the secretory pH of ≈5.5 show different results. Brender et al. found that the addition of Zn(II) to amylin at pH 5.5 decreases fibrillization lag-time and accelerates monomer addition to fibrils indicating a pH-dependent mechanism.163 Their proposed mechanism describes a process by which amylin fibrillization is inhibited by low pH in the secretory vesicle, but, after exocytosis and concomitant pH increase, Zn(II) can then act to prevent fibrillization in the extracellular space.163 This is further substantiated by a computational study that has identified a putative Zn(II) binding site.166
Several studies have shown that Cu(II), like Zn(II), can bind amylin, but whether it elicits harmful or beneficial effects remains controversial.167–172 Some studies have suggested that Cu(II) can bind to amylin monomer and prevent incorporation into fibrils.32,167,168,171,173 However, other work has shown that Cu(II) can promote the formation of smaller oligomers with possibly higher toxicity than fibrils.169,170,172 Other studies have attributed toxic effects of Cu(II) to the formation of reactive oxygen species (ROS).174 However, Yu et al. showed that Ni(II), a surrogate to Cu(II) that does not produce ROS, could mimic the aggregative and toxic effects observed with Cu(II)-mediated oligomer formation, implying a structural rather than oxidative basis for apoptotic induction.169 Lee et al. have proposed that amylin may protect rather than harm pancreatic cells from Cu(II)-mediated ROS formation.32 Incubation of Cu(II) with reducing agents exhibited substantial decrease H2O2 formation in the presence of amylin, suggesting that amylin may act to sequester Cu(II).
Researchers have sought to characterize the Cu(II)–amylin binding interaction in an effort to understand Cu(II)-mediated effects. The Quintanar group has provided strong spectroscopic evidence that at pH 5.5 Cu(II) anchors itself to the imidazole of His18 and binds to backbone amides towards the C-terminus and an oxygen, presumably from Ser20, to yield an equatorial 3N1O coordination geometry.175 This binding mode could induce a more compact and rigid structure to the mostly random coil structure of apo-amylin. The electron paramagnetic resonance (EPR) signal of the Cu(II)-adduct with amylin monomer differs from that of the Cu(II)–fibril adduct, suggesting that the Cu(II)-monomer conformer would compete with the formation of Cu(II)-bound fibrils.175 Challenges yet remain in understanding metal interactions with amylin given the complex structural behavior of the peptide (as is the case with many amyloidogenic peptides). The fate of these metal–amylin adducts upon release from secretory vesicles presents a more elusive question.
Glucagon
Glucagon is a 29-residue peptide that is secreted by pancreatic α-cells and is regarded as the main catabolic peptide hormone. At low plasma glucose levels, glucagon is released and travels through the blood to stimulate gluconeogenesis and glycogenolysis in hepatic cells. In turn, these activated pathways increase glucose concentration in plasma.24 Not surprisingly, glucagon and insulin have interwoven regulations. Glucagon is secreted from α-cells at low insulin blood levels and retained at high levels,176 while insulin secretion is enhanced by the presence of glucagon.133 In addition to the dynamic interplay between glucagon and insulin regulation, metal ions have also been implicated in glucagon secretion and is the focus of intense research.
One metal signal that affects exocytosis of stored glucagon is increased levels of Ca(II) in α-cells. In order for the concentration of Ca(II) to increase, the α-cells must become excited. As detailed by Briant et al.,177 both pancreatic α- and β-cells are excitable electrically through ion flux. At low plasma glucose, Na+,K+-ATPases increase the action potential of the cells, in turn causing voltage-gated Ca(II) channels to import extracellular Ca(II) into the cytosol. The Ca(II) influx facilitates exocytosis of glucagon into the bloodstream, signaling for production and release of glucose in the liver.
In addition to Ca(II), it is speculated that Zn(II) can affect glucagon release. It was initially thought that Zn(II), with its high levels at the surface of β-cells upon co-secretion with insulin, was a paracrine factor and suppressed glucagon release.178,179 Recent work, however, does not support this paracrine role. Both Tamaki et al.133 and Hardy et al.180 used ZnT8 knockout mice to assess the effects of granular Zn(II) on glucagon release. ZnT8 is responsible for transporting Zn(II) from the cytoplasm into secretory vesicles and a knockout of ZnT8 would drastically lower the overall concentration of Zn(II) in β-cell secretory vesicles. Under these reduced granular Zn(II) concentrations, Tamaki et al.133 and Hardy et al.180 found a negligible effect on glucagon secretion. These studies were further substantiated by a one year study that showed Zn(II) supplementation in humans did not affect glucagon response, although the authors suggest further research is needed on patients with more advanced disease states.181
While increasing evidence negates the hypothesis that Zn(II) secreted from β-cells have little or no effect on glucagon release, Zn(II) in α-cells may be involved in glucagon regulation. Solomou et al. produced α-cell ZnT8 mouse knockouts and discovered that with decrease in cytosolic and secretory Zn(II) levels, glucagon release increased during low glucose blood levels.182 Of note is that the concentration of Ca(II) remained unchanged indicating a specific and independent role for Zn(II) in α-cells.182 Glucagon is a prime example of the challenges faced when studying metal ion interactions in vivo in light of cell-specific actions even within the same organ. The emerging work connecting Zn(II) to glucagon regulation in α-cells demonstrates the importance of considering different cell types in studying the metal biology of hormone function.
Gastrin
Gastrin is a peptide hormone that regulates digestion through stimulating gastric acid release and promoting gastric mucosa proliferation.183–187 Transcribed as an 80-amino acid prohormone, it undergoes an array of post-translational modifications to generate biologically active species.188 Gastrin is commonly found in three major isoforms: gastrin-34 (big gastrin), gastrin-17 (little gastrin), and gastrin-14 (minigastrin). These isoforms have been found with further modifications, including Gly extension, C-terminal amidation, and side-chain sulfation and phosphorylation, each of which modulate their function and localization.188
In the late 1980's Graham Baldwin from the University of Melbourne illuminated an association between little gastrin and transferrin, and hypothesized that gastrin may play a role in the uptake of dietary iron.189 In subsequent work Baldwin and coworkers observed significantly higher levels of little gastrin in plasma, serum, and gastric mucosal extracts from mouse models of hemochromatosis, a genetic iron overload, as compared to their wild-type counterparts, further connecting gastrin to systemic iron homeostasis.190 To further understand the relationship between iron and gastrin, they initiated investigations focused on the ability of gastrin to bind iron ions. Through UV-Vis spectroscopy, tyrosine fluorescence quenching, and EPR spectroscopy, they determined that the glycine-extended analog of little gastrin (Ggly) can bind two Fe(III) ions.191 Peptide mutagenesis studies alongside NMR studies identified the glutamate-rich region as the metal binding site.192,193 In particular, NMR peak broadening was observed at Glu7 with one molar equivalent of Fe(III) followed by broadening of the Glu8 and Glu9 upon addition of a second equivalent.193 Quantitative parameters of the binding interaction as well as iron coordination geometry have yet to be determined.
The Fe(III)-dependent effects on gastrin were further extended to Ggly. Gly has been shown to direct gastrin activity towards stimulation of cell proliferation.183–185 Radioligand binding assays of Ggly to its receptor, CCK2R showed hormone binding in the presence of Fe(III), which could be eradicated by addition of the iron chelator desferoxamine (DFO).193 The iron-dependent hormone–receptor interaction was substantiated in cell proliferation assays wherein Ggly promoted IMGE-5 cell growth only in the presence of iron.193 This is in contrast to the C-terminal amidated analog of little gastrin that has been shown to bind a single Fe(III) in the glutamate-rich region which appears not to be involved in activity.192 However, little is understood about the exact molecular interaction between Ggly and CCK2R, complicating mechanistic elucidations of this iron-dependence. Recent evidence has shown that zinc and bismuth may be involved in gastrin regulation through upregulation and receptor binding inhibition, respectively, opening the possibility of other metal interactions.194,195
Tyrosine sulfation and phosphorylation of little gastrin has also been shown to increase the affinity of Fe(III) for gastrin, but effects on receptor binding were not reported.30,196 Nonetheless, this effect presents the possibility that post-translational modifications could influence metal interactions on peptide hormone function.
In the same family as gastrin is the cholecystokinin hormone (CCK). CCK shares a receptor and C-terminal pentapeptide amide with gastrin. The eight amino acid fragment, CCK-8, plays a role in gallbladder contractions as well as an unknown role in the central nervous system.197 Given its high sequence conservation to the postulated Fe(III) binding sites of gastrin, Baldwin investigated the metal-binding properties of CCK-8.198 CCK-8 was found to bind one Fe(III) or one Ca(II) ion, phosphorylation and sulfation of the Tyr residue added an additional site for binding of a second Fe(III).198 While the biological implications are not yet clear, these findings add intrigue to the question as to how post-translational modifications can influence metal-dependent endocrine activity.
5. Reproductive hormones
Significant energy of the endocrine system is devoted to reproductive processes. In humans, reproductive hormones are primarily regulated by the hypothalamus–pituitary axis.199 The hypothalamus monitors and controls the release of reproductive hormones via secretion of gonadotropin-releasing hormone (GnRH, also known as luteinizing hormone releasing hormone, LHRH). GnRH stimulates the anterior pituitary gland to synthesize and release luteinizing hormone (LH) and follicle-stimulating hormone (FSH). In turn, these pituitary outputs target cells in the gonads (testes in males and ovaries in females) to initiate and regulate gameto- and steroidogenesis. Moreover, LH and FSH can stimulate production of steroids, like estradiol and progesterone, and peptides, such as activin and inhibin, which serve regulatory functions in both positive and negative feedback loops.199 These hormones can further interface with neuroendocrine hormones, which in turn can drive social behaviors that facilitate reproductive success.200
The earliest reports on metals in relation to reproductive endocrinology focused on their possible link to infertility, spontaneous abortions, and infant mortality under high-exposure conditions. Such human occupational studies arose alongside plant and factory exposure to toxic metals including lead, cadmium and mercury. Although initial studies focused on female reproduction,201 studies emerged linking adverse reproductive effects in males to high acute and chronic exposure to heavy metals.202 Active research seeks to elucidate molecular mechanisms of acute and heavy metal toxicity to reproductive success as well as metal-induced oncogenic processes related to cancers in reproductive organs. Interestingly, studies are emerging that point to essential roles of nutritional metals like zinc and copper to proper reproductive function. A highlight of these efforts is work by Woodruff, O’Halloran, and coworkers, who have revealed an association between zinc regulation and mammalian egg fertilization.203–205 Upon fertilization, an incredible release of labile or transient zinc (∼200 μM) precipitates the progression of the fertilized egg to leave metaphase arrestation and begin the rapid divisions of embryonic development. The characteristic flashes of zinc that were visualized via a labile zinc responsive fluorescent probe were accordingly coined “zinc sparks.” These studies among others warrant additional efforts to disentangle the reproductive toxicity of metals from their necessity.
GnRH and LH
Initial studies investigating metal effects on reproductive hormones assessed levels of LH and FSH. Data supported correlations between high metal exposure to impaired LH levels. For instance, in healthy, premenopausal women, environmentally relevant levels of metals including cadmium, lead, and mercury were associated with changes in reproductive hormone levels, including LH.206 Higher exposure to metals such as lead, cadmium, and molybdenum in male petrol workers were correlated to lower LH levels.207 Pine and coworkers performed studies in rats to elucidate factors that initiated adverse reproductive effects, and found that Mn(II) can stimulate specific puberty-related hormones, including LH, and suggested that Mn(II) may facilitate a normal onset of puberty.208
In order to examine the mechanism underlying metal-induced alterations in LH levels, researchers have investigated the upstream LH effector, the 10-mer peptide GnRH. Ex vivo tissue culture models have been implemental in understanding metal influences on GnRH-mediated LH release. The administration of Cu(II) was shown to stimulate the release of GnRH from isolated hypothalamic granules and median eminence explants, and increases levels of GnRH in the pituitary stalk of rabbits.209,210In vitro studies with anterior pituitary fragments isolated from rats demonstrated that LH release is unaffected by Ni(II) and Zn(II), while Cd(II) cause an increase in baseline LH secretion.211
Given that the concentration of copper in the hypothalamic axonal terminals is 1–2 orders of magnitude greater than in the plasma, researchers have suggested that Cu(II) may serve as a natural stimulating factor of GnRH in vivo.212–214 Cu(II) has been shown to form a stable complex with GnRH by coordinating three nitrogen donors. Ni(II) has also been shown to form a stable complex with GnRH that is different than the Cu(II)–GnRH complex by coordinating four nitrogen donors.209 While these findings suggest that GnRH and LH levels are influenced by metal treatments, it has been difficult to distinguish whether GnRH, LH, both, or another upstream factor serve as the molecular targets of cationic metals; even less is understood regarding how various metals may elicit different effects.
Oxytocin
While the hypothalamus–pituitary–gonad axis serves as the driving pathway for healthy reproduction, this works in concert with hormones that affect social behavior. Among these is oxytocin, a peptide that has received attention with respect to its bioinorganic properties. Oxytocin is produced by the paraventricular nucleus of the hypothalamus, amidated by PAM, and released by the posterior pituitary into the bloodstream as a hormone.215 This dynamic nonamer is often referred to as ‘the love hormone’ or the ‘cuddle chemical’ on account of its critical role in complex relationship establishment between mammals; for example, mothers and babies tend to show elevated levels of oxytocin when they are together. Upon binding of oxytocin to its receptor, it regulates a diverse set of processes ranging from parturition to lactation. Oxytocin was the first peptide hormone to be sequenced and synthesized, and it is extensively used in clinical therapies.200
The presence of certain divalent metal ions was first reported by Soloff and Swartz to be essential for oxytocin receptor binding in rat mammary tissue membranes, with receptor binding modulated in increasing order by Zn(II), Mg(II), Ni(II), Mn(II), and Co(II); after the subsequent addition of the chelator EDTA, receptor binding was negligible.216 Soloff and Pearlmutter further postulated two distinct metal-binding regions of the oxytocin receptor.34 Metal binding to one region increases binding sites without affecting affinity for oxytocin, whereas metal binding to the other increases affinity for oxytocin; binding of metal to both sites may result in a receptor of high affinity with full availability. However, there is uncertainty whether the metal ions primarily interact with the receptor, oxytocin, or both. More recently, while no specific mechanisms are reported, Kleszczewski et al. reported that Cu(II) complexation does not affect oxytocin action in vitro.217
While the exact mechanism of metal-mediated receptor binding remains unclear, the use of electrochemical and spectroscopic methods has been employed to elucidate the effects of divalent metals on the structure of the isolated or synthesized hormone. Using potentiometric techniques, researchers concluded that oxytocin forms very stable complexes with Cu(II) by coordination of the terminal amino and three amide nitrogen donor atoms,218,219 but no information on the specific metal binding site or conformational changes upon Cu(II) binding were reported. Using cross-sectional MS and hydration energy MS in addition to density functional theory (DFT) and Chemistry at Harvard Macromolecular Mechanics (CHARMM) calculations, the Bowers group proposed a Zn(II)-bound structure wherein the Zn(II) forms a near-perfect octahedral complex with six of the backbone carbonyl oxygens of oxytocin. The authors hypothesized that this Zn(II) coordination creates a near planar surface ideal for receptor interaction, in addition to freeing the N-terminus.220 In subsequent work, Bowers and coworkers applied MS and ion mobility spectrometry (IMS) in combination with molecular dynamics (MD) and DFT calculations to further characterize oxytocin interactions with divalent metal ions.221 They suggested several structures of an [OT-3H + Cu(II)]− complex (Fig. 5). They also suggested quasi-octahedral and quasi-trigonal-bipyramidal structures of an [OT + Zn(II)]2+ complex. Joly et al. further studied the influence of Cu(II) on the structural and optical properties of oxytocin with IMS and UV photodissociation experiments in combination with DFT calculations.222 They concluded that copper complexation drastically changes the structure of oxytocin, specifically predicting that the Cu(II) is chelated by the carbonyl oxygen of the oxytocin ring; thus leading to a contraction of the oxytocin ring and orientating the Tyr2 side chain away from the ring. Indeed, the proposed structure of Zn(II)-bound oxytocin and its receptor-binding ability differs from Cu(II)-bound oxytocin.
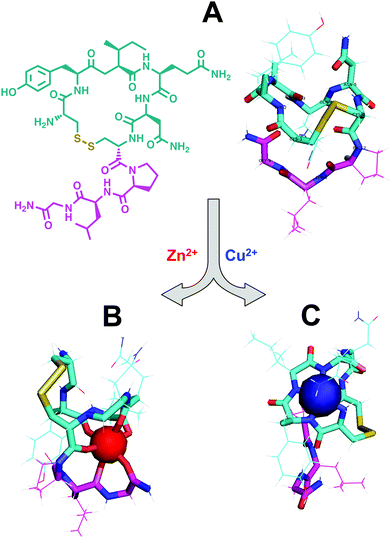 |
| Fig. 5 Proposed structures of apo-oxytocin and oxytocin with copper or zinc by the Bowers group.172,173 Amino acid residues 1–6 form a 20 membered ring (cyan) closed by a disulfide bond between Cys1 and Cys6 (yellow). Residues 7–9 shown in pink. (A) Left: Chemical structure of biologically active apo-oxytocin. Right: Calculated structure of biologically active apo-oxytocin. (B) Calculated structure of [OT + Zn]2+ showing Zn(II) octahedral coordination to six backbone carbonyl oxygens of residues 2, 3, 4, 6, 8, and 9. Zinc binding may potentiate receptor binding. (C) Lowest energy structure of [OT-3H + Cu]− showing Cu(II) coordination to backbone amide nitrogens of residues 1, 2, 3, and 4. Copper binding neither increases nor decreases oxytocin receptor binding. | |
6. Metal interactions with hormones in growth, wound healing, and inflammation
Controlled growth and tissue repair is essential for all living organisms and the endocrine system invests much energy and resources to ensure that it is properly regulated. In its simplest definition, growth is the proliferation of cells. In another instance of cell proliferation, after an injury or infection, tissues must regenerate themselves to repair the damages. The presence of such a traumatic event like injury or infection often leads to inflammation which is characterized by an immunological response to damaged tissues.223–225 Cell proliferation and controlled inflammation are necessary processes for proper growth and repair, and may be further implicated in aging and various disease states. The exact mechanisms behind the control of inflammation and cell proliferation are yet to be uncovered; however, research has contributed to our understanding of hormonal control of these processes.
While the nutritional importance of metals such as zinc, copper, and iron has been recognized for a long time, their importance in immune responses is becoming more apparent. Zn(II) regulates immune responses by tightly regulating its availability, and a disturbance in this mechanism may adversely alter cell survival, proliferation, differentiation, and maturation.226 Cu(II) serves as an important catalytic cofactor in redox chemistry for proteins that are involved in growth and development, and its misregulation has been linked to several growth- and immune-related disease states.227 Perhaps the most well-studied metal in relation to the inflammatory response is iron. As discussed earlier in this review, hepcidin, the master regulatory hormone for iron, is intimately tied to resisting infection.67,73,76,77,228 Interestingly, a chronic state of low serum iron levels can lead to anemia of inflammation, a chronic disease in which the host organism has a perpetually reduced serum iron level but, as a result, a decrease in inflammation.229 In attempts to untangle the complex mechanisms behind various inflammatory diseases, the following examples in this section showcase works elucidating interactions of metal micronutrients with hormones involved in growth, healing, and inflammation.
Human growth hormone (hGH)
As its name would indicate, human growth hormone (hGH) is the primary regulator of somatic growth.230,231 The 191-residue α-helical protein is biosynthesized in the pituitary gland and stored in somatotrophic granules where Zn(II) concentrations exceed 1 mM.232–234
Two hypotheses have been put forth as to how hGH is stored. In the first hypothesis, Zn(II) mediates the formation of an inactive homodimer for tight-packing and storage (Fig. 6A).234 In this regime, His18, His21, or Glu174 from each unit tetrahedrally coordinate to the metal center with a pseudo-mirror plane of symmetry.234 Studies have quantified a dissociation constant of 2.6 μM for this Zn(II)–homodimer complex, making this conformation feasible given the millimolar Zn(II) concentrations in the granules.232–234 The Zn(II)-mediated homodimer complex has also been found to be more stable to guanidine–HCl denaturation than the protein monomer, indicating Zn(II)–homodimerization could prolong hGH lifetime in storage. This stabilization could further prevent premature hGH signaling near the pituitary gland at release until hGH is diluted sufficiently in circulation.234 At this point the dimer would dissociate into the active monomer for receptor binding at the target tissue.
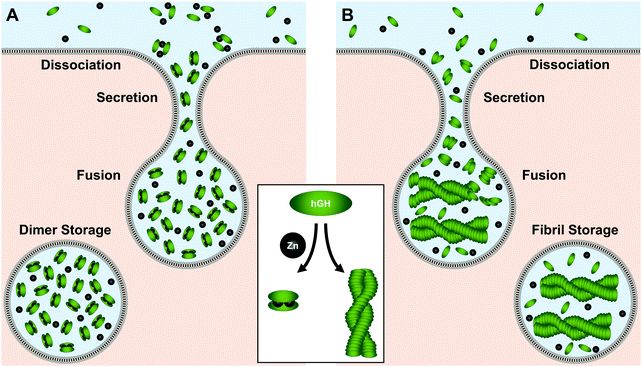 |
| Fig. 6 Model of the two proposed pathways for hGH storage and secretion by (A) Zn(II)-mediated homodimer, and (B) Zn(II)-induced fibrillization of hGH is stored in the secretory vesicle; however, it is unknown if, or how, Zn(II) coordinates to the fibril. Upon stimulation of release, fusion of the secretory vesicle occur at the membrane. At secretion, the dimers or fibrils are diluted to monomers in a concentration-dependent manner. Active monomer then diffuses to the target tissue for receptor binding. Green oval represents hGH monomer and black circle represents Zn(II) ion. | |
In the second storage hypothesis, Zn(II) induces amyloid fibril formation of hGH (Fig. 6B).235 Similar models have been postulated for storage of other proteins in secretory vesicles.236,237 In recent work supporting this hypothesis, Jacob et al. observed a colocalization of growth hormone and amyloid immunostains as well as with FluoZin-3, a Zn(II)-detecting fluorophore.235 With sequence analysis, they determined that hGH has high potential to form amyloid fibrils and speculated that Zn(II) could initiate fibrillization. Moreover, they found that isolated fibrils formed in the presence of Zn(II) could release the monomers upon dilution, offering a possible mechanism for protein release from Zn(II)-stabilized storage.235 Despite the differences between the storage modes, both hypotheses support a crucial role for Zn(II) in mediating hGH secretion.
Zinc dependence of hGH activity has also been observed in receptor binding at the target tissues. hGH is known to bind the hGH receptor (hGHr) and the human prolactin receptor (hPRLr) at a 1
:
2 and 1
:
1 ratio of hormone to receptor.231,238,239 While the hGH:hGHr ternary complex relies on electrostatics to form a salt bridge, the interaction of hGH with hPRLr occurs through bridging via tetrahedral Zn(II) coordination of a Glu and His from hGH and Asp and His from hPRLr.231 In a study by Voorhees et al.,240 tyrosine Förster resonance energy transfer (FRET) was used to demonstrate the ability of Zn(II) to induce a conformational change in hGH that would allow for hPRLr binding. While many questions remain to fully understand the influence of Zn(II) on hGH, increasing evidence supports the importance of its role in hGH function. Elucidating these interactions can impact therapeutic approaches to growth hormone dysfunction.
GHK–Cu
The tripeptide GHK was first isolated by Loren Pickart in the early 1970s and described as a liver growth factor.241 Initially GHK was isolated in its metal-free form, but, in 1980, Pickart et al. isolated GHK in the presence of Cu(II) and noticed that the resulting complex, GHK–Cu, was more active for copper and iron uptake in hepatoma (HTC4) cells.242 Since then, GHK–Cu has been isolated in plasma, saliva, and urine and is implicated in a variety of advantageous physiological processes.243–247
GHK is naturally produced as part of the collagen synthesis pathway and is cleaved after collagen injury or tissue degradation.248 The tripeptide binds Cu(II) at the N-terminal amine, backbone amide nitrogen of Gly, and the π-nitrogen of the imidazole ring on His. With this binding mode, one axial and one equatorial site are available to bind water or carbonyl oxygen at physiological pH.249–251 Raising the pH facilitates concomitant Lys deprotonation and displacement of an oxygen ligand at one of these sites with the Lys amine.252,253 The coordination ligands and binding geometry at physiological pH is reminiscent of the ATCUN binding motif found on HSA (Fig. 3A and C), the most abundant protein in blood and a main transporter of copper throughout the body.249 The binding affinity of GHK and Cu(II) is log
K = 16.4, while the affinity of HSA and Cu(II) is log
K = 16.2.244,252 Although these affinities are comparable, their equatorial binding modes differ: Cu(II) is bound by four strongly coordinating equatorial ligands in HSA resulting in slow exchange rates, whereas one of the equatorial ligands in GHK–Cu is weakly coordinated, permitting fast exchange at this position.249 This has led to the hypothesis that GHK–Cu may act as a local delivery system from blood to tissues. Cu(II) could be exchanged from HSA to GHK, given their similar affinities, but GHK–Cu would be more suitable and non-toxic for copper delivery and utilization by tissues.252
Beyond its role in directly regulating copper metabolism, GHK–Cu has numerous biological benefits that include wound healing, increased antioxidant capabilities, and regulating iron release from ferritin.243–247,254 A postulated way that GHK–Cu promotes wound healing is through altering gene expression. Initial studies by Pickart et al. found that GHK–Cu potentially upregulates the expression of matrix metalloproteinases and other metal-dependent enzymes associated with remodeling and regeneration.244,247 They later showed that GHK–Cu affects more than matrix metalloproteinase genes, but also genes associated with growth, antioxidant control, and iron homeostasis.255 For instance, insulin-like 6 (INSL6) protein, which is responsible for sperm development and fertilization, is upregulated by almost two-fold. On the other hand, insulin-like growth factor 1 (IGF1) is responsible for inducing growth and is downregulated by five-fold. These two results suggest that GHK–Cu may be able to increase longevity and inhibit ageing. TLE1, a gene that inhibits NF-κB, is upregulated almost eight-fold. Upregulation of NF-κB results in oxidative conditions within the cell and has been implicated in cancer and inflammatory diseases when upregulated. In this case, GHK–Cu may serve as an antioxidant inhibitor.
GHK–Cu was also found to upregulate HEPH,255 a gene that is responsible for increasing iron efflux, lowering cellular iron concentrations, and decreasing the propensity of ROS. Other studies have corroborated connections between GHK–Cu and iron metabolism apart from HEPH upregulation. In the blood, GHK–Cu blocks iron release from ferritin by up to 87%.246 The prevailing hypothesis for this suppression is that the GHK–Cu complex may block iron pores of ferritin,246 but further evidence is required to validate this proposal. Since its discovery, GHK–Cu research has opened up a wealth of possible actions for the hormone, and insight on which of these directly contribute to its health benefits and clarity on tissue targets will propel its therapeutic applications.
Bradykinin
Bradykinin (BK) is a nonapeptide hormone involved in inflammatory response. It elicits its proinflammatory effect by stimulating vascular endothelial cells to release prostacyclin, nitric oxide, and endothelium-derived hyperpolarizing factor, resulting in vasodilation.211 While its role as an inflammatory mediator has been established, it has emerging roles in neuromodulation, blood pressure regulation, allergy response, and renal function, which are the subject of active research.256 BK has been found to synergize with a number of different metabolites, hormones, and enzymes, many of which are associated with biological metals.
BK stability is affected by a number of metalloenzymes. In 1962, Aarsen and Kemp found that Mn(II), Co(II), and Zn(II) could reactivate a deactivated kininase to degrade bradykinin.257 It is now known that the catalytic activity of this enzyme, kininase II, which is also known as angiotensin-converting enzyme (ACE), contains a Zn(II)-dependent active site.258 BK was later found to be cleaved by another Zn(II)-dependent metallopeptidase, neprilysin.259 The inhibition of ACE and neprilysin degradation of BK as well as other signaling peptides involved with vascular biology and natriures are of therapeutic interest, particularly in treating heart failure.260–262
Zn(II) obtained from diet has, in fact, been linked to blood pressure regulation, with Zn(II) deficiency reducing the vasodilation response of BK.263 While this may be partially attributed to the Zn(II)-dependence of BK-degrading enzymes, researchers have explored the possibility that metals may directly interact with BK. Studies such as those by Cerda et al. have employed MS and spectrophotometric methods to illustrate the ability of metals like Zn(II) and Cu(II) to bind BK.264–268 In recent work, Grasso and coworkers investigated the influence of Cu(II) and Zn(II) on BK conformation.266 This interest was in light of growing controversial evidence that BK may be related to Alzheimer's disease pathology on account of its roles in both inflammation and neuromodulation and Cu(II) and Zn(II) were selected due to their high concentrations in the brain. The authors performed the first detailed study of Cu(II)- and Zn(II)-conformational modulation of BK using MS, circular dichroism (CD), and computational methods, as well as biochemical analyses. While both metals were found to bind BK, their resulting effect on conformation differed. While oligomers could be detected in the presence of Zn(II), Cu(II) appeared to hinder oligomerization. Moreover, Cu(II) reduced the inflammatory stimulation of BK in monocytic cell culture, whereas Zn(II) showed little effect.266 While most of the focus on metal effects on BK-associated pathways have been at the enzymatic level, attention may be warranted towards direct interactions of metals with BK itself.
7. Steroid hormones
While mechanistic studies in metalloendocrinology are more prevalent with peptide and protein hormones, interactions of metals with steroid hormones have been a topic of interest albeit with less molecular understanding. In fact, metal influences on steroids have been reported as early as 1972, when corticosteroids were observed to form metal complexes with Co(II).269 A handful of steroid hormones have since been reported to be noticeably impacted by metals, including the neurosteroid pregnenolone, the sex steroids estrogen, progesterone, and androgen, as well as the corticosteroid glucocorticoid.270–273 In some cases, metals have been hypothesized to influence steroidogenesis. For instance, Ca(II) uptake by adrenal mitochondria can stimulate formation of pregnenolone, a neurosteroid and precursor to various other steroid hormones, by increasing the interaction of mitochondrial cytochrome P-450 with endogenous substrate.274 In other cases, metals have been assessed as disruptors to normal endocrine action.275 Increasing levels of heavy metal pollution paired with a rise in the occurrence of hormone-related cancers has influenced recent studies focused on investigating the mechanisms and applications of metalloestrogens, a class of inorganic xenoestrogens which can affect the gene expression of estrogen-responsive cells. Many metalloestrogens have demonstrated the capability to bind cellular estrogen receptors, mimicking the actions of natural estrogens.273,276 Of particular interest is the heavy metal Cd(II), which has been suggested as an environmental endocrine disruptor due to its ability to mimic the effects of estrogen and activate the estrogen receptor.277–280 Alongside studies of toxic metal effects, altered levels in naturally-abundant biological metal micronutrients have been linked to disturbances in steroid function. For example, significantly lower Zn(II) was observed in the testes of rats with testicular feminization, suggesting a relationship between Zn(II) and androgen production or action.281,282 These reports indicate that metals are non-innocent with respect to steroid-driven processes, yet the exact factors that direct metal–hormone action remain an open question. Further molecular understanding is required to disentangle whether metals elicit beneficial or disruptive effects to steroid actions and which points of the steroid pathways are directly impacted.
8. Conclusion
The endocrine system is a sophisticated network coordinated at the physiological, biological, and chemical level. At the same time, increasing research is introducing new paradigms in metal homeostasis and control. The perspective from which these areas in combination are approached can influence the mechanisms that are uncovered. In examining global and clinical phenomena, connecting symptomatic observations to alterations in metal metabolism can put a spotlight on specific axes and pathways that may be influenced by bioinorganic functions. At the cellular level, controlling biomolecules that function as bioinorganic regulators may uncover the molecular pieces influenced by metals. At the chemical level, understanding structure and pinpointing coordination modes can link the activity of the metal to its influence on hormone function. While interest in hormones and metals may have started several decades ago, the field of metalloendocrinology can immensely benefit from the surge in technological advances in new genetic manipulation strategies, expanding chemical biology tools for probing bioinorganic chemistry in vivo, and innovative analytical techniques for studying metal interactions with biological molecules.
This review has focused on over 100 years of research concerning the endocrine system, specifically revolving around advances in the understanding of the direct influences of metal micronutrients on hormone structure and activities, as well as the indirect influences of both metal micronutrients and toxic metals on hormone levels and functions. In this light, the review harmonizes inorganic chemistry and endocrinology to a newly-termed field of “metalloendocrinology,” suggesting the necessity of future research in this interdisciplinary field. More is needed to understand metal micronutrient modulation of endocrine function; either through structure–function relationship or by mediating a hormone–receptor complex. It may be interesting to note that, beyond the research described in this review, several other areas of study can improve understanding and application of bioinorganic chemistry to hormone biology. Metals may impact the function of steroids and their receptors; and impact the synthesis of phospholipid derived eicosanoids. In addition to direct metal interactions with hormones, metals themselves can mimic hormones as in the case of vanadium complexes and insulin or Cd(II) and estrogen. Finally, beyond the canonical endocrine network, other tissues are being found to serve as possible endocrine glands, with much recent attention focused on adipose tissue. This opens up new possibilities and systems wherein metal biology may be investigated.
The benefits of understanding hormone biology are clear from the clinical applications ushered in by interdisciplinary research in the area. Placing focus on the impacts of metals in these systems can provide missing links to elusive mechanisms and offer innovative solutions for emerging health concerns. Research in metalloendocrinology offers the potential to significantly improve human health by informing nutritional recommendations, contributing to new diagnostic approaches, and transforming hormone-based therapeutic strategies.
Conflicts of interest
The authors declare no competing financial interests.
Acknowledgements
Financial support was provided by the University of California, Davis. M. C. H. would like to thank the University of California Office of the President and the UC Davis Advance CAMPOS Faculty Scholar program for additional support. This work was supported in part by gift funds from the UC Davis Comprehensive Cancer Center.
References
- W. W. de Herder, Heroes in endocrinology: Nobel Prizes, Endocr. Connect., 2014, 3, R94–R104 Search PubMed.
- J. R. Tata, One hundred years of hormones, EMBO Rep., 2005, 6, 490–496 CrossRef CAS.
- M. Patlak, New Weapons to Combat an Ancient Disease: Treating Diabetes, FASEB J., 2002, 16, 1853e–1853e CrossRef.
- WHO|Endocrine Disrupting Chemicals (EDCs), http://www.who.int/ceh/risks/cehemerging2/en/, accessed 30 July 2018.
- I. Iavicoli, L. Fontana and A. Bergamaschi, The Effects of Metals as Endocrine Disruptors, J. Toxicol. Environ. Health, Part B, 2009, 12, 206–223 CAS.
- W. Maret and M. Copsey, Metallomics: whence and whither, Metallomics, 2012, 4, 1017 RSC.
- M. R. Bleackley and R. T. A. MacGillivray, Transition metal homeostasis: from yeast to human disease, Biometals, 2011, 24, 785–809 CrossRef CAS PubMed.
- D. Templeton, Speciation in Metal Toxicity and Metal-Based Therapeutics, Toxics, 2015, 3, 170–186 CrossRef CAS.
- R. A. Goyer, Toxic and Essential Metal Interactions, Annu. Rev. Nutr., 1997, 17, 37–50 CrossRef CAS.
- S. V. S. Rana, Perspectives in Endocrine Toxicity of Heavy Metals—A Review, Biol. Trace Elem. Res., 2014, 160, 1–14 CrossRef CAS.
- J. C. Wood, L. Noetzl, A. Hyderi, M. Joukar, T. Coates and S. Mittelman, Predicting pituitary iron and endocrine dysfunction, Ann. N. Y. Acad. Sci., 2010, 1202, 123–128 CrossRef CAS.
- D. Huster and S. Lutsenko, Wilson disease: not just a copper disorder. Analysis of a Wilson disease model demonstrates the link between copper and lipid metabolism, Mol. BioSyst., 2007, 3, 816 RSC.
- W. Xu, T. Barrientos and N. C. Andrews, Iron and copper in mitochondrial diseases, Cell Metab., 2013, 17, 319–328 CrossRef CAS.
- O. Loréal, T. Cavey, E. Bardou-Jacquet, P. Guggenbuhl, M. Ropert and P. Brissot, Iron, hepcidin, and the metal connection, Front. Pharmacol., 2014, 5, 1–10 Search PubMed.
- S. M. Wazir and I. Ghobrial, Copper deficiency, a new triad: anemia, leucopenia, and myeloneuropathy, J. Community Hosp. Intern. Med. Perspect., 2017, 7, 265–268 CrossRef.
- M. M. Black, Zinc deficiency and child development, Am. J. Clin. Nutr., 1998, 68, 464S–469S CrossRef CAS.
- H. C. Lukaski, C. B. Hall and M. J. Marchello, Body temperature and thyroid hormone metabolism of copper-deficient rats, J. Nutr. Biochem., 1995, 6, 445–451 CrossRef CAS.
- J. L. Beard, M. J. Borel and J. Derr, Impaired thermoregulation and thyroid function in iron-deficiency anemia, Am. J. Clin. Nutr., 1990, 52, 813–819 CrossRef CAS.
- S. Y. Kim, F. Zhang, W. Gong, K. Chen, K. Xia, F. Liu, R. A. Gross, J. M. Wang, R. J. Linhardt and M. L. Cotten, Copper regulates the interactions of antimicrobial piscidin peptides from fish mast cells with formyl peptide receptors and heparin, J. Biol. Chem., 2018 DOI:10.1074/jbc.RA118.001904.
- J. Shi and A. C. Camus, Hepcidins in amphibians and fishes: Antimicrobial peptides or iron-regulatory hormones?, Dev. Comp. Immunol., 2006, 30, 746–755 CrossRef CAS.
- A. S. N. Reddy, G. S. Ali, H. Celesnik and I. S. Day, Coping with Stresses: Roles of Calcium- and Calcium/Calmodulin-Regulated Gene Expression, Plant Cell, 2011, 23, 2010–2032 CrossRef CAS.
- M. C. Udechukwu, S. A. Collins and C. C. Udenigwe, Prospects of enhancing dietary zinc bioavailability with food-derived zinc-chelating peptides, Food Funct., 2016, 7, 4137–4144 RSC.
-
S. Nussey and S. Whitehead, Endocrinology: An Integrated Approach, BIOS Scientific Publishers, 2001 Search PubMed.
-
D. Voet and J. G. Voet, Biochemistry, Wiley, New York, 4th edn, 2011 Search PubMed.
- C. D. Kline and N. J. Blackburn, Substrate-Induced Carbon Monoxide Reactivity Suggests Multiple Enzyme Conformations at the Catalytic Copper M-Center of Peptidylglycine Monooxygenase, Biochemistry, 2016, 55, 6652–6661 CrossRef CAS.
- A. Ozawa, Y. Cai and I. Lindberg, Production of bioactive peptides in an in vitro system, Anal. Biochem., 2007, 366, 182–189 CrossRef CAS PubMed.
- A. Mizutani, H. Inoko and M. Tanaka, Carboxypeptidase E, Identified As a Direct Interactor of Growth Hormone, Is Important for Efficient Secretion of the Hormone, Mol. Cells, 2016, 39, 756–761 CrossRef CAS PubMed.
- C. W. Liew, A. Assmann, A. T. Templin, J. C. Raum, K. L. Lipson, S. Rajan, G. Qiang, J. Hu, D. Kawamori, I. Lindberg, L. H. Philipson, N. Sonenberg, A. B. Goldfine, D. A. Stoffers, R. G. Mirmira, F. Urano and R. N. Kulkarni, Insulin regulates carboxypeptidase E by modulating translation initiation scaffolding protein eIF4G1 in pancreatic cells, Proc. Natl. Acad. Sci. U. S. A., 2014, 111, E2319–E2328 CrossRef CAS.
- M. R. Sapio and L. D. Fricker, Carboxypeptidases in disease: Insights from peptidomic studies, Proteomics: Clin. Appl., 2014, 8, 327–337 CAS.
- G. S. Baldwin and I. Sims, Tyrosine modification increases the affinity of gastrin for ferric ions, SpringerPlus, 2015, 4, 815 CrossRef.
- S. A. Lambert, A. Jolma, L. F. Campitelli, P. K. Das, Y. Yin, M. Albu, X. Chen, J. Taipale, T. R. Hughes and M. T. Weirauch, The Human Transcription Factors, Cell, 2018, 172, 650–665 CrossRef CAS.
- E. C. Lee, E. Ha, S. Singh, L. Legesse, S. Ahmad, E. Karnaukhova, R. P. Donaldson and A. M. Jeremic, Copper(II)–human amylin complex protects pancreatic cells from amylin toxicity, Phys. Chem. Chem. Phys., 2013, 15, 12558 RSC.
- T. V. O’Halloran, M. Kebede, S. J. Philips and A. D. Attie, Zinc, insulin, and the liver: a ménage à trois, J. Clin. Invest., 2013, 123, 4136–4139 CrossRef.
- A. F. Pearlmutter and M. S. Soloff, Characterization of the metal ion requirement for oxytocin–receptor interaction in rat mammary gland membranes, J. Biol. Chem., 1979, 254, 3899–3906 CAS.
- C. J. Chang, Searching for harmony in transition-metal signaling, Nat. Chem. Biol., 2015, 11, 744–747 CrossRef CAS.
- T. V. O’Halloran and V. C. Culotta, Metallochaperones, an Intracellular Shuttle Service for Metal Ions, J. Biol. Chem., 2000, 275, 25057–25060 CrossRef.
- J. T. Potts, Parathyroid hormone: past and present, J. Endocrinol., 2005, 187, 311–325 CAS.
- W. M. Boothby, The Parathyroid Glands A Review of the Literature, Endocrinology, 1921, 5, 403–440 CrossRef.
- W. G. Maccallum and C. Voegtlin, On the Relation of Tetany to the Parathyroid Glands and to Calcium Metabolism, J. Exp. Med., 1909, 11, 118–151 CrossRef CAS.
- W. G. Maccallum and K. M. Vogel, Further Experimental Studies in Tetany, J. Exp. Med., 1913, 18, 618–650 CrossRef CAS.
- J. Collip, The Extraction of a Parathyroid Hormone Which Will Prevent or Control Parathyroid Tetany And Which Regulates the Level of Blood Calcium, J. Biol. Chem., 1925, 63, 395–438 CAS.
- F. Albright and E. C. Recfenstein, The Parathyroid Glands and Metabolic Bone Disease, Ulster Med. J., 1950, 19, 130–131 Search PubMed.
- H. B. Brewer, T. Fairwell, R. Ronan, G. W. Sizemore and C. D. Arnaud, Human parathyroid hormone: amino-acid sequence of the amino-terminal residues 1–34, Proc. Natl. Acad. Sci. U. S. A., 1972, 69, 3585–3588 CrossRef CAS.
- H. D. Niall, R. T. Sauer, J. W. Jacobs, H. T. Keutmann, G. V. Segre, J. L. O’Riordan, G. D. Aurbach and J. T. Potts, The amino-acid sequence of the amino-terminal 37 residues of human parathyroid hormone, Proc. Natl. Acad. Sci. U. S. A., 1974, 71, 384–388 CrossRef CAS.
- H. T. Keutmann, M. M. Sauer, G. N. Hendy, L. H. O’Riordan and J. T. Potts, Complete amino acid sequence of human parathyroid hormone, Biochemistry, 1978, 17, 5723–5729 CrossRef CAS.
- H. Jüppner, A. B. Abou-Samra, M. Freeman, X. F. Kong, E. Schipani, J. Richards, L. F. Kolakowski, J. Hock, J. T. Potts, H. M. Kronenberg and G. V. Segre, A G protein-linked receptor for parathyroid hormone and parathyroid hormone-related peptide, Science, 1991, 254, 1024–1026 CrossRef.
- C. Bergwitz, T. J. Gardella, M. R. Flannery, J. T. Potts, H. M. Kronenberg, S. R. Goldring and H. Jüppner, Full activation of chimeric receptors by hybrids between parathyroid hormone and calcitonin. Evidence for a common pattern of ligand–receptor interaction, J. Biol. Chem., 1996, 271, 26469–26472 CrossRef CAS.
-
T. J. Gardella, H. Jüppner, E. M. Brown, H. M. Kronenberg and J. T. Potts, Endocrinology: Adult and Pediatric, Elsevier, 7th edn, 2016, pp. 969–990 Search PubMed.
- A. Iida-Klein, J. Guo, M. Takemura, M. T. Drake, J. T. Potts, A. Abou-Samra, F. R. Bringhurst and G. V. Segre, Mutations in the second cytoplasmic loop of the rat parathyroid hormone (PTH)/PTH-related protein receptor result in selective loss of PTH-stimulated phospholipase C activity, J. Biol. Chem., 1997, 272, 6882–6889 CrossRef CAS.
- M. Shimizu, P. H. Carter, A. Khatri, J. T. Potts and T. J. Gardella, Enhanced Activity in Parathyroid Hormone-(1–14) and -(1–11): Novel Peptides for Probing Ligand–Receptor Interactions 1, Endocrinology, 2001, 142, 3068–3074 CrossRef CAS.
- J. T. Potts and T. J. Gardella, Progress, paradox, and potential: parathyroid hormone research over five decades, Ann. N. Y. Acad. Sci., 2007, 1117, 196–208 CrossRef CAS.
- M. Berglund, A. Akesson, P. Bjellerup and M. Vahter, Metal–bone interactions, Toxicol. Lett., 2000, 112–113, 219–225 CrossRef CAS.
- A. A. El Tayeb, N. A. Abd El-Mottaleb and E. A. Abdel Aziz, Relationship between serum parathyroid hormone and trace elements (serum zinc and magnesium) in hemodialyzed chronic renal failure children, Biol. Trace Elem. Res., 2009, 128, 128–134 CrossRef.
- T. Suzuki, Y. Kajita, S. Katsumata, H. Matsuzaki and K. Suzuki, Zinc Deficiency Increases Serum Concentrations of Parathyroid Hormone through a Decrease in Serum Calcium and Induces Bone Fragility in Rats, J. Nutr. Sci. Vitaminol., 2015, 61, 382–390 CrossRef CAS.
- P. H. Carter and T. J. Gardella, Zinc(II)-mediated enhancement of the agonist activity of histidine-substituted parathyroid hormone(1–14) analogues, Biochim. Biophys. Acta, Mol. Cell Res., 2001, 1538, 290–304 CrossRef CAS.
- L. Osagie-Clouard, A. Sanghani, M. Coathup, T. Briggs, M. Bostrom and G. Blunn, Parathyroid hormone 1–34 and skeletal anabolic action, Bone Joint Res., 2017, 6, 14–21 CrossRef CAS.
- I. Mazumdar, K. Goswami and M. S. Ali, Status of Serum Calcium, Vitamin D and Parathyroid Hormone and Hematological Indices Among Lead Exposed Jewelry Workers in Dhaka, Bangladesh, Indian J. Clin. Biochem., 2017, 32, 110–116 CrossRef CAS.
- G. K. Schwalfenberg and S. J. Genuis, Vitamin D, Essential Minerals, and Toxic Elements: Exploring Interactions between Nutrients and Toxicants in Clinical Medicine, Sci. World J., 2015, 2015, 318595 Search PubMed.
- D. H. Copp, Calcitonin: discovery, development, and clinical application, Clin. Invest. Med., 1994, 17, 268–277 CAS.
- J.-J. Body, Calcitonin for the long-term prevention and treatment of postmenopausal osteoporosis, Bone, 2002, 30, 75S–79S CrossRef CAS.
- T. Ganz, Hepcidin, a
key regulator of iron metabolism and mediator of anemia of inflammation, Blood, 2012, 102, 783–788 CrossRef.
- V. Sangkhae and E. Nemeth, Regulation of the Iron Homeostatic Hormone Hepcidin, Adv. Nutr., 2017, 8, 126–136 CrossRef CAS.
- A. Krause, S. Neitz, H.-J. Mägert, A. Schulz, W. Forssmann, P. Schulz-Knappe and K. Adermann, LEAP-1, a novel highly disulfide-bonded human peptide, exhibits antimicrobial activity, FEBS Lett., 2000, 480, 147–150 CrossRef CAS.
- C. H. Park, E. V. Valore, A. J. Waring and T. Ganz, Hepcidin, a Urinary Antimicrobial Peptide Synthesized in the Liver, J. Biol. Chem., 2001, 276, 7806–7810 CrossRef CAS.
- C. Pigeon, G. Ilyin, B. Courselaud, P. Leroyer, B. Turlin, P. Brissot and O. Loréal, A New Mouse Liver-specific Gene, Encoding a Protein Homologous to Human Antimicrobial Peptide Hepcidin, Is Overexpressed during Iron Overload, J. Biol. Chem., 2001, 276, 7811–7819 CrossRef CAS.
- G. Nicolas, M. Bennoun, I. Devaux, C. Beaumont, B. Grandchamp, A. Kahn and S. Vaulont, Lack of hepcidin gene expression and severe tissue iron overload in upstream stimulatory factor 2 (USF2) knockout mice, Proc. Natl. Acad. Sci. U. S. A., 2001, 98, 8780–8785 CrossRef CAS.
- T. Ganz, Hepcidin and iron regulation, 10 years later, Blood, 2011, 117, 4425–4433 CrossRef CAS.
- D. J. Hare, Hepcidin: a real-time biomarker of iron need, Metallomics, 2017, 9, 606–618 RSC.
- H. N. Hunter, D. Bruce Fulton, T. Ganz and H. J. Vogel, The solution structure of human hepcidin, a peptide hormone with antimicrobial activity that is involved in iron uptake and hereditary hemochromatosis, J. Biol. Chem., 2002, 277, 37597–37603 CrossRef CAS.
- E. Nemeth, M. S. Tuttle, J. Powelson, M. B. Vaughn, A. Donovan, D. M. Ward, T. Ganz and J. Kaplan, Hepcidin Regulates Cellular Iron Efflux by Binding to Ferroportin and Inducing Its Internalization, Science, 2004, 306, 2090–2093 CrossRef CAS.
- A. Pietrangelo, Pathogens, Metabolic Adaptation, and Human Diseases – An Iron-Thrifty Genetic Model, Gastroenterology, 2015, 149, 838 Search PubMed.
- S. B. Keel, R. Doty, L. Liu, E. Nemeth, S. Cherian, T. Ganz and J. L. Abkowitz, Evidence that the expression of transferrin receptor 1 on erythroid marrow cells mediates hepcidin suppression in the liver, Exp. Hematol., 2015, 43, 469–478 CrossRef CAS.
- E. Nemeth, M. S. Tuttle, J. Powelson, M. B. Vaughn, A. Donovan, D. M. Ward, T. Ganz and J. Kaplan, Hepcidin Regulates Cellular Iron Efflux by Binding to Ferroportin and Inducing Its Internalization, Science, 2004, 306, 2090–2093 CrossRef CAS.
- J. Arezes, G. Jung, V. Gabayan, E. Valore, P. Ruchala, P. A. Gulig, T. Ganz, E. Nemeth and Y. Bulut, Hepcidin-induced hypoferremia is a critical host defense mechanism against the siderophilic bacterium vibrio vulnificus, Cell Host Microbe, 2015, 17, 47–57 CrossRef CAS.
- G. Nicolas, C. Chauvet, L. Viatte, J. L. Danan, X. Bigard, I. Devaux, C. Beaumont, A. Kahn and S. Vaulont, The gene encoding the iron regulatory peptide hepcidin is regulated by anemia, hypoxia, and inflammation, J. Clin. Invest., 2002, 110, 1037–1044 CrossRef CAS.
- T. Ganz, Iron and infection, Int. J. Hematol., 2018, 107, 7–15 CrossRef CAS.
- M. R. Hanudel, M. Rappaport, V. Gabayan, G. Jung, I. B. Salusky, E. Nemeth, T. Ganz and J. Zaritsky, Increased serum hepcidin contributes to the anemia of chronic kidney disease in a murine model, Haematologica, 2017, 102, e85–e88 CrossRef CAS.
- M. J. Hubler, K. R. Peterson and A. H. Hasty, Iron homeostasis: A new job for macrophages in adipose tissue?, Trends Endocrinol. Metab., 2015, 26, 101–109 CrossRef CAS.
- V. Sangkhae and E. Nemeth, Regulation of the Iron Homeostatic Hormone Hepcidin, Adv. Nutr., 2017, 8, 126–136 CrossRef CAS.
- P. Ruchala and E. Nemeth, The pathophysiology and pharmacology of hepcidin, Trends Pharmacol. Sci., 2014, 35, 155–161 CrossRef CAS.
- T. Ganz, Cellular iron: Ferroportin is the only way out, Cell Metab., 2005, 1, 155–157 CrossRef CAS.
- B. Qiao, P. Sugianto, E. Fung, A. Del-Castillo-Rueda, M. J. Moran-Jimenez, T. Ganz and E. Nemeth, Hepcidin-induced endocytosis of ferroportin is dependent on ferroportin ubiquitination, Cell Metab., 2012, 15, 918–924 CrossRef CAS.
- A. Donovan, C. A. Lima, J. L. Pinkus, G. S. Pinkus, L. I. Zon, S. Robine and N. C. Andrews, The iron exporter ferroportin/Slc40a1 is essential for iron homeostasis, Cell Metab., 2005, 1, 191–200 CrossRef CAS.
- K. Yamamoto, T. Kuragano, T. Kimura, M. Nanami, Y. Hasuike and T. Nakanishi, Interplay of adipocyte and hepatocyte: Leptin upregulates hepcidin, Biochem. Biophys. Res. Commun., 2018, 495, 1548–1554 CrossRef CAS.
- C. M. M. Laarakkers, E. T. Wiegerinck, S. Klaver, M. Kolodziejczyk, H. Gille, A. M. Hohlbaum, H. Tjalsma and D. W. Swinkels, Improved Mass Spectrometry Assay For Plasma Hepcidin: Detection and Characterization of a Novel Hepcidin Isoform, PLoS One, 2013, 8, e75518 CrossRef CAS.
- N. Campostrini, M. Traglia, N. Martinelli, M. Corbella, M. Cocca, D. Manna, A. Castagna, C. Masciullo, L. Silvestri, O. Olivieri, D. Toniolo, C. Camaschella and D. Girelli, Serum levels of the hepcidin-20 isoform in a large general population: The Val Borbera study, J. Proteomics, 2012, 76, 28–35 CrossRef CAS PubMed.
- Y. Hasegawa, K. Miura, K. Furuya, K. I. Yoshiura and H. Masuzaki, Identification of complete hydatidiform mole pregnancy-associated MicroRNAs in plasma, Clin. Chem., 2013, 59, 1410–1412 CrossRef CAS.
- N. Tomosugi, H. Kawabata, R. Wakatabe, M. Higuchi, H. Yamaya, H. Umehara and I. Ishikawa, Detection of serum hepcidin in renal failure and inflammation by using ProteinChip System, Blood, 2006, 108, 1381–1387 CrossRef CAS.
- E. Nemeth, G. C. Preza, C. L. Jung, J. Kaplan, A. J. Waring and T. Ganz, The N-terminus of hepcidin is essential for its interaction with ferroportin: Structure-function study, Blood, 2006, 107, 328–333 CrossRef CAS.
- T. Peters, Interaction of one mole of copper with the alpha amino group of bovine serum albumin, Biochim. Biophys. Acta, 1960, 39, 546–547 CrossRef CAS.
- S. Melino, L. Garlando, M. Patamia, M. Paci and R. Petruzzelli, A metal-binding site is present in the amino terminal region of the bioactive iron regulator hepcidin-25, J. Pept. Res., 2005, 66, 65–71 CrossRef.
- K. Kulprachakarn, Y. L. Chen, X. Kong, M. C. Arno, R. C. Hider, S. Srichairatanakool and S. S. Bansal, Copper(II) binding properties of hepcidin, J. Biol. Inorg. Chem., 2016, 21, 329–338 CrossRef CAS.
- C. Tselepis, S. J. Ford, A. T. McKie, W. Vogel, H. Zoller, R. J. Simpson, J. Diaz Castro, T. H. Iqbal and D. G. Ward, Characterization of the transition-metal-binding properties of hepcidin, Biochem. J., 2010, 427, 289–296 CrossRef CAS.
- C. Brasse-Lagnel, Z. Karim, P. Letteron, S. Bekri, A. Bado and C. Beaumont, Intestinal DMT1 cotransporter is down-regulated by hepcidin via proteasome internalization and degradation, Gastroenterology, 2011, 140, 1261–1271 CrossRef CAS.
- S. R. Hennigar and J. P. McClung, Hepcidin Attenuates Zinc Efflux in Caco-2 Cells, J. Nutr., 2016, 146, 2167–2173 CrossRef CAS.
- A. Badarau, A. Basle, S. J. Firbank and C. Dennison, Crosstalk between Cu(I) and Zn(II) homeostasis via Atx1 and cognate domains, Chem. Commun., 2013, 49, 8000–8002 RSC.
- J. J. Worthington, The intestinal immunoendocrine axis: novel cross-talk between enteroendocrine cells and the immune system during infection and inflammatory disease, Biochem. Soc. Trans., 2015, 43, 727–733 CrossRef CAS.
- M. Carabotti, A. Scirocco, M. A. Maselli and C. Severi, The gut-brain axis: interactions between enteric microbiota, central and enteric nervous systems, Ann. Gastroenterol., 2015, 28, 203–209 Search PubMed.
- M. F. Dunn, Zinc-ligand interactions modulate assembly and stability of the insulin hexamer – a review, Biometals, 2005, 18, 295–303 CrossRef CAS PubMed.
- I. Sekler, S. L. Sensi, M. Hershfinkel and W. F. Silverman, Mechanism and regulation of cellular zinc transport, Mol. Med., 2007, 13, 337–343 CAS.
-
The Liver in Systemic Diseases, ed. H. Ohira, Springer Japan, Tokyo, 2016 Search PubMed.
- G. E. Griesmann, A. C. Hartmann and F. F. Farris, Concentrations and correlations for eight metals in human liver, Int. J. Environ. Health Res., 2009, 19, 231–238 CrossRef CAS.
- W. Maret, Analyzing free zinc(II) ion concentrations in cell biology with fluorescent chelating molecules, Metallomics, 2015, 7, 202–211 RSC.
- M. Yaman, Comprehensive Comparison of Trace Metal Concentrations in Cancerous and Non-Cancerous Human Tissues, Curr. Med. Chem., 2006, 13, 2513–2525 CrossRef CAS.
- B. Brannick, M. Kocak and S. Solomon, Vanadium in Glucose Metabolism: Past, Present and Future, J. Toxicol. Pharmacol., 2017, 1, 11 Search PubMed.
- K. H. Thompson, J. H. McNeill and C. Orvig, Vanadium Compounds as Insulin Mimics, Chem. Rev., 1999, 99, 2561–2572 CrossRef CAS.
- X. F. Meng, Q. F. Liu, J. L. Liu, M. H. Sun and J. J. Ma, Synthesis, crystal structure, and insulin-like activity of [N′-(2-hydroxy-3-methoxybenzylidene)-2-methoxybenzohydrazonato](1,10-phenanthroline)oxovanadium(IV) methanol solvate, Inorg. Nano-Met. Chem., 2017, 47, 1585–1589 CrossRef CAS.
- L. Krishnamoorthy, J. A. Cotruvo, J. Chan, H. Kaluarachchi, A. Muchenditsi, V. S. Pendyala, S. Jia, A. T. Aron, C. M. Ackerman, M. N. V. Wal, T. Guan, L. P. Smaga, S. L. Farhi, E. J. New, S. Lutsenko and C. J. Chang, Copper regulates cyclic-AMP-dependent lipolysis, Nat. Chem. Biol., 2016, 12, 586–592 CrossRef CAS PubMed.
- T. Xiao, C. M. Ackerman, E. C. Carroll, S. Jia, A. Hoagland, J. Chan, B. Thai, C. S. Liu, E. Y. Isacoff and C. J. Chang, Copper regulates rest-activity cycles through the locus coeruleus-norepinephrine system, Nat. Chem. Biol., 2018, 14, 655–663 CrossRef CAS.
- C. W. Ward and M. C. Lawrence, Landmarks in insulin research, Front. Endocrinol., 2011, 2, 1–11 Search PubMed.
- F. Sanger and H. Tuppy, The Amino-acid Sequence in the Glycyl Chain of Insulin, Biochem. J., 1953, 49, 463–481 CrossRef.
- F. Sanger and E. O. P. Thompson, The Amino-acid Sequence in the Glycyl Chain of Insulin 1. The Identification of Lower Peptides from Partial Hydrolysates, Biochem. J., 1953, 53, 353 CrossRef CAS.
- F. Sanger and H. Tuppy, The amino-acid sequence in the phenylalanyl chain
of insulin. I. The identification of lower peptides from partial hydrolysates, Biochem. J., 1951, 49, 463–481 CrossRef CAS.
- F. Sanger and H. Tuppy, The Amino-acid Sequence in the Phenylalanyl Chain of Insulin 2. The investigation of peptides from enzymic hydrolysates, Biochem. J., 1951, 49, 481–490 CrossRef CAS.
- G. Wilcox, Insulin and insulin resistance, Clin. Biochem. Rev., 2005, 26, 19–39 Search PubMed.
- A. S. Prasad, Discovery of Human Zinc Deficiency: Its Impact on Human Health and Disease, Adv. Nutr., 2013, 4, 176–190 CrossRef CAS.
- Z. Xie, C. Chang and Z. Zhou, Molecular Mechanisms in Autoimmune Type 1 Diabetes: a Critical Review, Clin. Rev. Allergy Immunol., 2014, 47, 174–192 CrossRef CAS.
- J. L. Leahy, Pathogenesis of type 2 diabetes mellitus, Arch. Med. Res., 2005, 36, 197–209 CrossRef CAS.
- A. Khan and F. Awan, Metals in the pathogenesis of type 2 diabetes, J. Diabetes Metab. Disord., 2014, 13, 16 CrossRef.
- G. Harry and M. D. Jacobi, Protamine Insulin in the Treatment of Diabetes, Am. J. Dig. Dis. Nutr., 1936, 3, 908–915 CrossRef.
- M. J. Adams, T. L. Blundell, E. J. Dodson, G. G. Dodson, M. Vijayan, E. N. Baker, M. M. Harding, D. C. Hodgkin, B. Rimmer and S. Sheat, Structure of rhombohedral 2 zinc insulin crystals, Nature, 1969, 224, 491–495 CrossRef CAS.
- T. Blundell, G. Dodson, D. Hodgkin and D. Mercola, Insulin: the structure in the crystal and its reflection in chemistry and biology, Adv. Protein Chem., 1972, 26, 279–402 CrossRef CAS.
- A. J. L. Sudmeier, S. J. Bell, M. C. Storm, M. F. Dunn, S. Science, N. Series, N. May and J. Maher, Cadmium-113 Nuclear Magnetic Resonance Studies of Bovine Insulin: Two-Zinc Insulin Hexamer Specifically Binds Calcium Cadmium-113 Nuclear Magnetic Resonance Studies of Bovine Insulin: Two-Zinc Insulin Hexamer Specifically Binds Calcium, Science, 1981, 212, 560–562 CrossRef.
- T. Izumi, K. Kasai and H. Gomi, Secretory vesicle docking to the plasma membrane: molecular mechanism and functional significance, Diabetes, Obes. Metab., 2007, 9, 109–117 CrossRef CAS.
- J. D. Castle, R. S. Cameron, P. Arvan, M. von Zastrow and G. Rudnick, Similarities and differences among neuroendocrine, exocrine, and endocytic vesicles, Ann. N. Y. Acad. Sci., 1987, 493, 448–460 CrossRef CAS.
- F. D. Coffman and M. F. Dunn, Insulin—Metal Ion Interactions: The Binding of Divalent Cations to Insulin Hexamers and Tetrarners and the Assembly of Insulin Hexamers, Biochemistry, 1988, 27, 6179–6187 CrossRef CAS.
- W. Maret, Zinc in pancreatic islet biology, insulin sensitivity, and diabetes, Prev. Nutr. Food Sci., 2017, 22, 1–8 CrossRef.
- W. Kadima, M. Roy, R. W. K. Lee, N. C. Kaarsholm and M. F. Dunn, Studies of the association and conformational properties of metal-free insulin in alkaline sodium chloride solutions by one- and two-dimensional 1H NMR, J. Biol. Chem., 1992, 267, 8963–8970 CAS.
- M. L. Brader, N. C. Kaarsholm, S. E. Harnung and M. F. Dunn, Ligand Perturbation Effects on a Pseudotetrahedral Co(II)(His)3-Ligand Site, J. Biol. Chem., 1997, 272, 1088–1094 CrossRef CAS.
- M. L. Brader, Zinc coordination, asymmetry, and allostery of the human insulin hexamer, J. Am. Chem. Soc., 1997, 119, 7603–7604 CrossRef CAS.
- M. C. Carpenter and D. E. Wilcox, Thermodynamics of formation of the insulin hexamer: metal-stabilized proton-coupled assembly of quaternary structure, Biochemistry, 2014, 53, 1296–1301 CrossRef CAS.
- J. A. G. Pertusa, T. León-Quinto, G. Berná, J. R. Tejedo, A. Hmadcha, F. J. Bedoya, F. Martín and B. Soria, Zn(II) chelation by serum albumin improves hexameric Zn(II)-insulin dissociation into monomers after exocytosis, PLoS One, 2017, 12, e0187547 CrossRef PubMed.
- M. Tamaki, Y. Fujitani, A. Hara, T. Uchida, Y. Tamura, K. Takeno, M. Kawaguchi, T. Watanabe, T. Ogihara, A. Fukunaka, T. Shimizu, T. Mita, A. Kanazawa, M. O. Imaizumi and T. Abe, The diabetes-susceptible gene SLC30A8/ZnT8 regulates hepatic insulin clearance. Supplemental Material, J. Clin. Invest., 2013, 123, 1–11 CrossRef.
- T. Kunt, T. Forst, A. Pfützner, J. Beyer and J. Wahren, The physiological impact of proinsulin C-peptide, Pathophysiology, 1999, 5, 257–262 CrossRef CAS.
- J. Shafqat, E. Melles, K. Sigmundsson, B. L. Johansson, K. Ekberg, G. Alvelius, M. Henriksson, J. Johansson, J. Wahren and H. Jörnvall, Proinsulin C-peptide elicits disaggregation of insulin resulting in enhanced physiological insulin effects, Cell. Mol. Life Sci., 2006, 63, 1805–1811 CrossRef CAS.
- C. G. Frankær, P. Sønderby, M. B. Bang, R. V. Mateiu, M. Groenning, J. Bukrinski and P. Harris, Insulin fibrillation: the influence and coordination of Zn(II), J. Struct. Biol., 2017, 199, 27–38 CrossRef.
- T. Forst and T. Kunt, Effects of C-peptide on microvascular blood flow and blood hemorheology, Exp. Diabesity Res., 2004, 5, 51–64 CrossRef CAS.
- T. Forst, T. Kunt, T. Pohlmann, K. Goitom, M. Engelbach, J. Beyer and A. Pfützner, Biological activity of C-peptide on the skin microcirculation in patients with insulin-dependent diabetes mellitus, J. Clin. Invest., 1998, 101, 2036–2041 CrossRef CAS PubMed.
- Z. Keltner, J. A. Meyer, E. M. Johnson, A. M. Palumbo, D. M. Spence and G. E. Reid, Mass spectrometric characterization and activity of zinc-activated proinsulin C-peptide and C-peptide mutants, Analyst, 2010, 135, 278–288 RSC.
- J. A. Meyer, J. M. Froelich, G. E. Reid, W. K. A. Karunarathne and D. M. Spence, Metal-activated C-peptide facilitates glucose clearance and the release of a nitric oxide stimulus via the GLUT1 transporter, Diabetologia, 2008, 51, 175–182 CrossRef CAS.
- C. Wojcikowski, V. Maier, K. Dominiak, R. Fussganger and E. F. Pfeiffer, Effects of Synthetic Rat C-Peptide in Normal and Diabetic Rats, Diabetologia, 1983, 25, 288–290 CrossRef CAS.
- M. Henriksson, E. Nordling, E. Melles, J. Shafqat, M. Ståhlberg, K. Ekberg, B. Persson, T. Bergman, J. Wahren, J. Johansson and H. Jörnvall, Separate functional features of proinsulin C-peptide, Cell. Mol. Life Sci., 2005, 62, 1772–1778 CrossRef CAS.
- J. Wahren, J. Shafqat, J. Johansson, A. Chibalin, K. Ekberg and H. Jörnvall, Molecular and cellular effects of C-peptide–new perspectives on an old peptide, Exp. Diabesity Res., 2004, 5, 15–23 CrossRef CAS.
- T. Wallerath, T. Kunt, T. Forst, E. I. Closs, R. Lehmann, T. Flohr, M. Gabriel, D. Schäfer, A. Göpfert, A. Pfützner, J. Beyer and U. Förstermann, Stimulation of endothelial nitric oxide synthase by proinsulin C-peptide, Nitric Oxide, 2003, 9, 95–102 CrossRef CAS.
- Y. Ido, K. C. Vindigni, L. Stramm, R. Chance, W. F. Heath, R. D. DiMarchi, E. Di Cera and J. R. Williamson, Prevention of vascular and neural dysfunction in diabetic rats by C-peptide, Science, 1997, 277, 563–566 CrossRef CAS.
- G. L. C. Yosten, G. R. Kolar, L. J. Redlinger and W. K. Samson, Evidence for an interaction between proinsulin C-peptide and GPR146, J. Endocrinol., 2013, 218, B1–B8 CAS.
- E. Lindahl, U. Nyman, E. Melles, K. Sigmundsson, M. Ståhlberg, J. Wahren, B. Öbrink, J. Shafqat, B. Joseph and H. Jörnvall, Cellular internalization of proinsulin C-peptide, Cell. Mol. Life Sci., 2007, 64, 479–486 CrossRef CAS.
- Y. Liu, C. Chen, S. Summers, W. Medawala and D. M. Spence, C-peptide and zinc delivery to erythrocytes requires the presence of albumin: implications in diabetes explored with a 3D-printed fluidic device, Integr. Biol., 2015, 7, 534–543 RSC.
- M. Viera and C. M. Davis-McGibony, Isolation and characterization of low-molecular-weight chromium-binding substance (LMWCr) from chicken liver, Protein J., 2008, 27, 371–375 CrossRef CAS.
- L. E. Wu, A. Levina, H. H. Harris, Z. Cai, B. Lai, S. Vogt, D. E. James and P. A. Lay, Carcinogenic Chromium(VI) Compounds Formed by Intracellular Oxidation of Chromium(III) Dietary Supplements by Adipocytes, Angew. Chem., Int. Ed., 2016, 55, 1742–1745 CrossRef CAS.
- J. A. Meyer, W. Subasinghe, A. A. F. Sima, Z. Keltner, G. E. Reid, D. Daleke and D. M. Spence, Zinc-activated C-peptide resistance to the type 2 diabetic erythrocyte is associated with hyperglycemia-induced phosphatidylserine externalization and reversed by metformin, Mol. BioSyst., 2009, 5, 1157–1162 RSC.
- A. W. Giebink, P. A. Vogel, W. Medawala and D. M. Spence, C-peptide-stimulated nitric oxide production in a cultured pulmonary artery endothelium is erythrocyte mediated and requires Zn(II), Diabetes/Metab. Res. Rev., 2013, 29, 44–52 CrossRef CAS.
- G. J. Cooper, A. C. Willis, A. Clark, R. C. Turner, R. B. Sim and K. B. Reid, Purification and characterization of a peptide from amyloid-rich pancreases of type 2 diabetic patients, Proc. Natl. Acad. Sci. U. S. A., 1987, 84, 8628–8632 CrossRef CAS.
- T. Hanabusa, K. Kubo, C. Oki, Y. Nakano, K. Okai, T. Sanke and K. Nanjo, Islet amyloid polypeptide (IAPP) secretion from islet cells and its plasma concentration in patients with non-insulin-dependent diabetes mellitus, Diabetes Res. Clin. Pract., 1992, 15, 89–96 CrossRef CAS.
- X.-X. Zhang, Y.-H. Pan, Y.-M. Huang and H.-L. Zhao, Neuroendocrine hormone amylin in diabetes, World J. Diabetes, 2016, 7, 189 CrossRef.
- C. Martin, The physiology of amylin and insulin: Maintaining the balance between glucose secretion and glucose uptake, Diabetes Educ., 2006, 32, 101–104 Search PubMed.
- R. L. Bower and D. L. Hay, Amylin structure-function relationships and receptor pharmacology: implications for amylin mimetic drug development, Br. J. Pharmacol., 2016, 173, 1883–1898 CrossRef CAS.
- M. Rowińska-Zyrek, Coordination of Zn(II) and Cu(II) to the membrane disrupting fragment of amylin, Dalton Trans., 2016, 45, 8099–8106 RSC.
- P. Cao, P. Marek, H. Noor, V. Patsalo, L. H. Tu, H. Wang, A. Abedini and D. P. Raleigh, Islet amyloid: From fundamental biophysics to mechanisms of cytotoxicity, FEBS Lett., 2013, 587, 1106–1118 CrossRef CAS.
- J. W. M. Höppener and C. J. M. Lips, Role of islet amyloid in type 2 diabetes mellitus, Int. J. Biochem. Cell Biol., 2006, 38, 726–736 CrossRef.
- P. Westermark and E. Wilander, The influence of amyloid deposits on the islet volume in maturity onset diabetes mellitus, Diabetologia, 1978, 15, 417–421 CrossRef CAS.
- A. Abedini, F. Meng and D. P. Raleigh, A single-point mutation converts the highly amyloidogenic human islet amyloid polypeptide into a potent fibrillization inhibitor, J. Am. Chem. Soc., 2007, 129, 11300–11301 CrossRef CAS.
- J. R. Brender, K. Hartman, R. P. R. Nanga, N. Popovych, R. de la Salud Bea, S. Vivekanandan, E. N. G. Marsh and A. Ramamoorthy, Role of zinc in human islet amyloid polypeptide aggregation, J. Am. Chem. Soc., 2010, 132, 8973–8983 CrossRef CAS.
- S. Salamekh, J. R. Brender, S. J. Hyung, R. P. R. Nanga, S. Vivekanandan, B. T. Ruotolo and A. Ramamoorthy, A two-site mechanism for the inhibition of IAPP amyloidogenesis by zinc, J. Mol. Biol., 2011, 410, 294–306 CrossRef CAS.
- J. R. Brender, J. Krishnamoorthy, G. M. L. Messina, A. Deb, S. Vivekanandan, C. La Rosa, J. E. Penner-Hahn and A. Ramamoorthy, Zinc stabilization of prefibrillar oligomers of human islet amyloid polypeptide, Chem. Commun., 2013, 49, 3339–3341 RSC.
- V. Wineman-Fisher and Y. Miller, Insight into a New Binding Site of Zinc Ions in Fibrillar Amylin, ACS Chem. Neurosci., 2017, 8, 2078–2087 CrossRef CAS PubMed.
- C. Kállay, Á. Dávid, S. Timári, E. M. Nagy, D. Sanna, E. Garribba, G. Micera, P. De Bona, G. Pappalardo, E. Rizzarelli and I. Sóvágó, Copper(II) complexes of rat amylin fragments, Dalton Trans., 2011, 40, 9711 RSC.
- B. Ward, K. Walker and C. Exley, Copper(II) inhibits the formation of amylin amyloid in vitro, J. Inorg. Biochem., 2008, 102, 371–375 CrossRef CAS.
- Y.-P. Yu, P. Lei, J. Hu, W.-H. Wu, Y.-F. Zhao and Y.-M. Li, Copper-induced cytotoxicity: reactive oxygen species or islet amyloid polypeptide oligomer formation, Chem. Commun., 2010, 46, 6909 RSC.
- L. Ma, X. Li, Y. Wang, W. Zheng and T. Chen, Cu(II) inhibits hIAPP fibrillation and promotes hIAPP-induced beta cell apoptosis through induction of ROS-mediated mitochondrial dysfunction, J. Inorg. Biochem., 2014, 140, 143–152 CrossRef CAS.
- A. Magrì, D. La Mendola, V. G. Nicoletti, G. Pappalardo and E. Rizzarelli, New Insight in Copper-Ion Binding to Human Islet Amyloid: The Contribution of Metal-Complex Speciation To Reveal the Polypeptide Toxicity, Chem. – Eur. J., 2016, 22, 13287–13300 CrossRef.
- G. Caruso, D. A. Distefano, P. Parlascino, C. G. Fresta, G. Lazzarino, S. M. Lunte and V. G. Nicoletti, Receptor-mediated toxicity of human amylin fragment aggregated by short- and long-term incubations with copper ions, Mol. Cell. Biochem., 2017, 425, 85–93 CrossRef CAS.
- M. Seal and S. G. Dey, Active-Site Environment of Copper-Bound Human Amylin Relevant to Type 2 Diabetes, Inorg. Chem., 2018, 57, 129–138 CrossRef CAS.
- S. J. Stohs and D. Bagchi, Oxidative mechanisms in the toxicity of metal ions, Free Radical Biol. Med., 1995, 18, 321–336 CrossRef CAS.
- L. Rivillas-Acevedo, C. Sánchez-López, C. Amero and L. Quintanar, Structural basis for the inhibition of truncated islet amyloid polypeptide aggregation by Cu(II): insights into the bioinorganic chemistry of type II diabetes, Inorg. Chem., 2015, 54, 3788–3796 CrossRef CAS PubMed.
- B. A. Cooperberg and P. E. Cryer, Insulin reciprocally regulates glucagon secretion in humans, Diabetes, 2010, 59, 2936–2940 CrossRef CAS PubMed.
- L. Briant, A. Salehi, E. Vergari, Q. Zhang and P. Rorsman, Glucagon secretion from pancreatic α-cells, Upsala J. Med. Sci., 2016, 121, 113–119 CrossRef.
- H. Ishihara, P. Maechler, A. Gjinovci, P. L. Herrera and C. B. Wollheim, Islet β-cell secretion determines glucagon release from neigbouring α-cells, Nat. Cell Biol., 2003, 5, 330–335 CrossRef CAS.
- H. Zhou, T. Zhang, J. S. Harmon, J. Bryan and R. P. Robertson, Zinc, not insulin, regulates the rat alpha-cell response to
hypoglycemia in vivo, Diabetes, 2007, 56, 1107–1112 CrossRef CAS.
- A. B. Hardy, A. S. Serino, N. Wijesekara, F. Chimienti and M. B. Wheeler, Regulation of glucagon secretion by zinc: lessons from the β cell-specific Znt8 knockout mouse model, Diabetes, Obes. Metab., 2011, 13, 112–117 CrossRef CAS.
- A. Pérez, P. Rojas, F. Carrasco, K. Basfi-fer, F. Pérez-Bravo, J. Codoceo, J. Inostroza and M. Ruz, Zinc Supplementation Does Not Affect Glucagon Response to Intravenous Glucose and Insulin Infusion in Patients with Well-Controlled Type 2 Diabetes, Biol. Trace Elem. Res., 2018, 185, 255–261 CrossRef.
- A. Solomou, G. Meur, E. Bellomo, D. J. Hodson, A. Tomas, S. M. Li, E. Philippe, P. L. Herrera, C. Magnan and G. A. Rutter, The zinc transporter Slc30a8/ZnT8 is required in a subpopulation of pancreatic α-cells for hypoglycemia-induced glucagon secretion, J. Biol. Chem., 2015, 290, 21432–21442 CrossRef CAS.
- F. Hollande, A. Imdahl, T. Mantamadiotis, G. D. Ciccotosto, A. Shulkes and G. S. Baldwin, Glycine-extended gastrin acts as an autocrine growth factor in a nontransformed colon cell line, Gastroenterology, 1997, 113, 1576–1588 CrossRef CAS.
- C. Seva, C. J. Dickinson and T. Yamada, Growth-promoting effects of glycine-extended progastrin, Science, 1994, 265, 410–412 CrossRef CAS.
- F. Nègre, P. Fagot-Revurat, M. Bouisson, J. F. Rehfeld, N. Vaysse and L. Pradayrol, Autocrine stimulation of AR4-2J rat pancreatic tumor cell growth by glycine-extended gastrin, Int. J. Cancer, 1996, 66, 653–658 CrossRef.
- J. M. Henderson, G. Lidgard, D. H. Osborne, D. C. Carter and R. C. Heading, Lower oesophageal sphincter response to gastrin–pharmacological or physiological?, Gut, 1978, 19, 99–102 CrossRef CAS.
- J. F. Rehfeld, L. Friis-Hansen, J. P. Goetze and T. V. O. Hansen, The Biology of Cholecystokinin and Gastrin Peptides, Curr. Top. Med. Chem., 2007, 7, 1154–1165 CrossRef CAS.
-
A. Shulkes and G. S. Baldwin, Gastrin, Elsevier Inc., 2nd edn, 2013 Search PubMed.
- G. S. Baldwin, R. Chandler and J. Weinstock, Binding of gastrin to gastric transferrin, FEBS Lett., 1986, 205, 147–149 CrossRef CAS.
- K. A. Smith, S. Kovac, G. J. Anderson, A. Shulkes and G. S. Baldwin, Circulating gastrin is increased in hemochromatosis, FEBS Lett., 2006, 580, 6195–6198 CrossRef CAS PubMed.
- G. S. Baldwin, C. C. Curtain and W. H. Sawyer, Selective, high-affinity binding of ferric ions by glycine-extended gastrin, Biochemistry, 2001, 40, 10741–10746 CrossRef CAS.
- J. Pannequin, J. P. Tantiangco, S. Kovac, A. Shulkes and G. S. Baldwin, Divergent roles for ferric ions in the biological activity of amidated and non-amidated gastrins, J. Endocrinol., 2004, 181, 315–325 CrossRef CAS.
- J. Pannequin, K. J. Barnham, F. Hollande, A. Shulkes, R. S. Norton and G. S. Baldwin, Ferric ions are essential for the biological activity of the hormone glycine-extended gastrin, J. Biol. Chem., 2002, 277, 48602–48609 CrossRef CAS PubMed.
- L. Xiao, S. Kovac, M. Chang, A. Shulkes, G. S. Baldwin and O. Patel, Zinc ions upregulate the hormone gastrin via an E-box motif in the proximal gastrin promoter, J. Mol. Endocrinol., 2013, 52, 29–42 Search PubMed.
- S. Kovac, S. W. Loh, S. Lachal, A. Shulkes and G. S. Baldwin, Bismuth ions inhibit the biological activity of non-amidated gastrins in vivo, Biochem. Pharmacol., 2012, 83, 524–530 CrossRef CAS.
- G. J. Dockray, A. Varro, R. Dimaline and T. Wang, The gastrins: their production and biological activities, Annu. Rev. Physiol., 2001, 63, 119–139 CrossRef CAS PubMed.
- K. Miyasaka and A. Funakoshi, Cholecystokinin and cholecystokinin receptors, J. Gastroenterol., 2003, 38, 1–13 CrossRef PubMed.
- G. S. Baldwin, M. F. Bailey, B. P. Shehan, I. Sims and R. S. Norton, Tyrosine Modification Enhances Metal Ion Binding, Biochem. J., 2009, 416, 77–84 CrossRef PubMed.
- T. Dagklis, K. Ravanos, K. Makedou, A. Kourtis and D. Rousso, Common features and differences of the hypothalamic–pituitary–gonadal axis in male and female, Gynecol. Endocrinol., 2015, 31, 14–17 CrossRef CAS PubMed.
- J. G. Veening, T. R. De Jong, M. D. Waldinger, S. M. Korte and B. Olivier, The role of oxytocin in male and female reproductive behavior, Eur. J. Pharmacol., 2015, 753, 209–228 CrossRef CAS PubMed.
- P. Rzymski, K. Tomczyk, P. Rzymski, B. Poniedziałek, T. Opala and M. Wilczak, Impact of heavy metals on the female reproductive system, Ann. Agric. Environ. Med., 2015, 22, 259–264 CrossRef CAS PubMed.
- L. V. Perelomov, I. V. Perelomova and U. L. Venevtseva, The toxic effects of trace elements on male reproductive health, Hum. Physiol., 2016, 42, 454–462 CrossRef CAS.
- A. M. Kim, S. Vogt, T. V. O’Halloran and T. K. Woodruff, Zinc availability regulates exit from meiosis in maturing mammalian oocytes, Nat. Chem. Biol., 2010, 6, 674–681 CrossRef CAS.
- A. M. Kim, M. L. Bernhardt, B. Y. Kong, R. W. Ahn, S. Vogt, T. K. Woodruff and T. V. O’Halloran, Zinc Sparks Are Triggered by Fertilization and Facilitate Cell Cycle Resumption in Mammalian Eggs, ACS Chem. Biol., 2011, 6, 716–723 CrossRef CAS PubMed.
- E. L. Que, R. Bleher, F. E. Duncan, B. Y. Kong, S. C. Gleber, S. Vogt, S. Chen, S. A. Garwin, A. R. Bayer, V. P. Dravid, T. K. Woodruff and T. V. O’Halloran, Quantitative mapping of zinc fluxes in the mammalian egg reveals the origin of fertilization-induced zinc sparks, Nat. Chem., 2015, 7, 130–139 CrossRef CAS PubMed.
- A. Z. Pollack, E. F. Schisterman, L. R. Goldman, S. L. Mumford, P. S. Albert, R. L. Jones and J. Wactawski-Wende, Cadmium, lead, and mercury in relation to reproductive hormones and anovulation in premenopausal women, Environ. Health Perspect., 2011, 119, 1156–1161 CrossRef CAS PubMed.
- J. Jouda, E. K. J. Abdul, A. A. Maktoof, F. A. Shafi, R. T. Al-muswie and A. E. M. Alubadi, Work's Environment Effect on Metal and Male Reproductive Hormones Levels: Circulating Testosterone, LH, and FSH are Positively Associated with Cadmium, Lead, and Molybdenum, J. Global Pharma Technol., 2017, 7, 139–142 Search PubMed.
- M. Pine, B. Lee, R. Dearth, J. K. Hiney and W. Les Dees, Manganese acts centrally to stimulate luteinizing hormone secretion: a potential influence on female pubertal development, Toxicol. Sci, 2005, 85, 880–885 CrossRef CAS PubMed.
- K. Gerega, H. Kozlowski, E. Masiukiewicz, L. D. Pettit, S. Pyburn and B. Rzeszotarska, Metal complexes of luteinizing hormone-releasing hormone (LHRH). potentiometric and spectroscopic studies, J. Inorg. Biochem., 1988, 33, 11–18 CrossRef CAS.
- R. C. Tsou, R. A. Dailey, C. S. McLanahan, A. D. Parent, G. T. Tindall and J. D. Neill, Luteinizing hormone releasing hormone (LHRH) levels in pituitary stalk plasma during the preovulatory gonadotropin surge of rabbits, Endocrinology, 1977, 101, 534–539 CrossRef CAS.
- R. L. Cooper, J. M. Goldman, G. L. Rehnberg, W. K. McElroy and J. F. Hein, Effects of metal cations on pituitary hormone secretion in vitro, J. Biochem. Toxicol., 1987, 2, 241–249 CrossRef CAS.
- G. R. Merriam, L. L. Nunnelley, J. W. V. Trish and F. Naftolin, Sex-Related and Cyclic Variation of Trace Elements in Rat Hypothalamus and Pituitary, Brain Res., 1979, 171, 503–510 CrossRef CAS.
- J. Donaldson, D. McGregor and F. LaBella, Determination of Na(I), K(I), Mg(II), Cu(II), Zn(II), and Mn(II) in rat brain regions, Can. J. Biochem., 1973, 51, 87–92 CrossRef CAS.
- K. S. Rajan, R. W. Colburn and J. M. Davis, Distribution of metal ions in the subcellular fractions of several rat brain areas, Life Sci., 1976, 18, 423–431 CrossRef CAS PubMed.
- E. L. Sheldrick and A. P. F. Flint, Post-translational processing of oxytocin-neurophysin prohormone in the ovine corpus luteum: activity of peptidyl glycine α-amidating mono-oxygenase and concentrations of its cofactor, ascorbic acid, J. Endocrinol., 1989, 122, 313–322 CAS.
- S. Soloff, M. S. Soloff and T. L. Swartz, Characterization of a proposed oxytocin receptor in rat mammary gland, J. Biol. Chem., 1973, 248, 6471–6478 Search PubMed.
- T. Kleszczewski, B. Modzelewska, W. Bal, M. Sipowicz, E. Kleszczewska and A. Kostrzewska, Cu(II) complexation does not affect oxytocin action on pregnant human myometrium in vitro, Reprod. Toxicol., 2016, 59, 60–65 CrossRef CAS.
- W. Bal, H. Kozlowski, B. Lammek, K. Rolka and L. D. Pettit, Potentiometric and spectroscopic studies of the Cu(II) complexes of Ala-Arg8-vasopressin and oxytocin: Two vasopressin-like peptides, J. Inorg. Biochem., 1992, 45, 193–202 CrossRef CAS PubMed.
- K. Várnagy, B. Bóka, I. Sóvágó, D. Sanna, P. Marras and G. Micera, Potentiometric and spectroscopic studies on the copper(II) and nickel(II) complexes of tripeptides of methionine, Inorg. Chim. Acta, 1998, 275–276, 440–446 CrossRef.
- D. Liu, A. B. Seuthe, O. T. Ehrler, X. Zhang, T. Wyttenbach, J. F. Hsu and M. T. Bowers, Oxytocin–receptor binding: Why divalent metals are essential, J. Am. Chem. Soc., 2005, 127, 2024–2025 CrossRef CAS PubMed.
- M. T. Bowers, D. Liu and T. Wyttenbach, Interaction of divalent metal ions with the hormone oxytocin: hormone receptor binding, J. Am. Chem. Soc., 2008, 130, 1–19 CrossRef PubMed.
- L. Joly, R. Antoine, F. Albrieux, R. Ballivian, M. Broyer, F. Chirot, J. Lemoine, P. Dugourd, C. Greco and R. Mitric,
et al., Optical and Structural Properties of Copper-Oxytocin Dications in the Gas Phase, J. Phys. Chem. B, 2009, 113, 11293–11300 CrossRef CAS PubMed.
- R. G. Rosique, M. J. Rosique and J. A. Farina, Junior, Curbing Inflammation in Skin Wound Healing: A Review, Int. J. Inflammation, 2015, 2015, 316235 Search PubMed.
- N. M. Ashpole, J. E. Sanders, E. L. Hodges, H. Yan and W. E. Sonntag, Growth hormone, insulin-like growth factor-1 and the aging brain, Exp. Gerontol., 2015, 68, 76–81 CrossRef CAS.
- N. X. Landén, D. Li and M. Ståhle, Transition from inflammation to proliferation: a critical step during wound healing, Cell. Mol. Life Sci., 2016, 73, 3861–3885 CrossRef.
- P. Bonaventura, G. Benedetti, F. Albarède and P. Miossec, Zinc and its role in immunity and inflammation, Autoimmun. Rev., 2015, 14, 277–285 CrossRef CAS PubMed.
- A. Hordyjewska, Ł. Popiołek and J. Kocot, The many ‘faces’ of copper in medicine and treatment, Biometals, 2014, 27, 611–621 CrossRef CAS PubMed.
- M. Wessling-Resnick, Iron Homeostasis and the Inflammatory Response, Annu. Rev. Nutr., 2010, 30, 105–122 CrossRef CAS PubMed.
- P. G. Fraenkel, Anemia of Inflammation: A Review, Med. Clin. North Am., 2017, 101, 285–296 CrossRef PubMed.
- R. K. Chawla, J. S. Parks and D. Rudman, Structural Variants of Human Growth Hormone: Biochemical, Genetic, and Clinical Aspects, Annu. Rev. Med., 1983, 34, 519–545 CrossRef CAS PubMed.
- W. Somers, M. Ultsch, A. M. De Vos and A. A. Kossiakoff, The X-ray structure of a growth hormone-prolactin receptor complex, Nature, 1994, 372, 478–481 CrossRef CAS PubMed.
- O. Thorlacius-Ussing, Zinc in the anterior pituitary of rat: a histochemical and analytical work, Neuroendocrinology, 1987, 45, 233–242 CrossRef CAS PubMed.
-
R. H. Williams, J. D. Wilson and D. W. Foster, Williams Textbook of endocrinology, Saunders, Philadelphia, 7th edn, 1985 Search PubMed.
- B. C. Cunningham, M. G. Mulkerrin and J. A. Wells, Dimerization of Human Growth Hormone by Zinc, Science, 1991, 253, 545–548 CrossRef CAS PubMed.
- R. S. Jacob, S. Das, S. Ghosh, A. Anoop, N. N. Jha, T. Khan, P. Singru, A. Kumar and S. K. Maji, Amyloid formation of growth hormone in presence of zinc: relevance to its storage in secretory granules, Sci. Rep., 2016, 6, 1–18 CrossRef PubMed.
- E. Chanat and W. B. Huttner, Milieu-induced, selective aggregation of regulated secretory proteins in the trans-Golgi network, J. Cell Biol., 1991, 115, 1505–1519 CrossRef CAS PubMed.
- P. Arvan and D. Castle, Sorting and storage during secretory granule biogenesis: looking backward and looking forward, Biochem. J., 1998, 332, 593–610 CrossRef CAS PubMed.
- T. Clackson, M. H. Ultsch, J. A. Wells and A. M. de Vos, Structural and functional analysis of the 1
:
1 growth hormone
:
receptor complex reveals the molecular basis for receptor affinity, J. Mol. Biol., 1998, 277, 1111–1128 CrossRef CAS PubMed.
- J. J. Kopchick and J. M. Andry, Growth hormone (GH), GH receptor, and signal transduction, Mol. Genet. Metab., 2000, 71, 293–314 CrossRef CAS PubMed.
- J. L. Voorhees, G. V. Rao, T. J. Gordon and C. L. Brooks, Zinc binding to human lactogenic hormones and the human prolactin receptor, FEBS Lett., 2011, 585, 1783–1788 CrossRef CAS PubMed.
- L. Pickart and M. M. Thaler, Tripeptide in human serum which prolongs survival of normal liver cells and stimulates growth in neoplastic liver, Nat. New Biol., 1973, 243, 85–87 CAS.
- L. Pickart, J. H. Freedman, W. J. Loker, J. Peisach, C. M. Perkins, R. E. Stenkamp and B. Weinstein, Growth-modulating plasma tripeptide may function by facilitating copper uptake into cells, Nature, 1980, 288, 715–717 CrossRef CAS PubMed.
- A. Siméon, Y. Wegrowski, Y. Bontemps and F. X. Maquart, Expression of glycosaminoglycans and small proteoglycans in wounds: modulation by the tripeptide–copper complex glycyl-L-histidyl-L-lysine-Cu(II), J. Invest. Dermatol., 2000, 115, 962–968 CrossRef PubMed.
- L. Pickart, The human tri-peptide GHK and tissue remodeling, J. Biomater. Sci., Polym. Ed., 2008, 19, 969–988 CrossRef CAS PubMed.
- J. D. Pollard, S. Quan, T. Kang and R. J. Koch, Effects of copper tripeptide on the growth and expression of growth factors by normal and irradiated fibroblasts, Arch. Facial Plast. Surg., 2005, 7, 27–31 CrossRef PubMed.
- D. M. Miller, D. DeSilva, L. Pickart and S. D. Aust, Effects of glycyl-histidyl-lysyl chelated Cu(II) on ferritin dependent lipid peroxidation, Adv. Exp. Med. Biol., 1990, 264, 79–84 CrossRef CAS.
- D. Downey, W. F. Larrabee, V. Voci and L. Pickart, Acceleration of wound healing using glycyl-histidyl-lysyl Cu(II), Surg. Forum, 1985, 36, 573–575 CAS.
- L. Pickart, J. Vasquez-Soltero and A. Margolina, GHK-Cu may Prevent Oxidative Stress in Skin by Regulating Copper and Modifying Expression of Numerous Antioxidant Genes, Cosmetics, 2015, 2, 236–247 CrossRef CAS.
- C. Hureau, H. Eury, R. Guillot, C. Bijani, S. Sayen, P.-L. Solari, E. Guillon, P. Faller and P. Dorlet, X-ray and Solution Structures of Cu(II)GHK and Cu(II)DAHK Complexes: Influence on Their Redox Properties, Chem. – Eur. J., 2011, 17, 10151–10160 CrossRef CAS PubMed.
- M. A. Kremennaya, M. A. Soldatov, Y. S. Podkovyrina, I. A. Dadasheva and A. V. Soldatov, X-ray spectroscopy study of the Cu(II)GHK peptide complex, J. Struct. Chem., 2017, 58, 1261–1267 CrossRef.
- P. Gonzalez, K. Bossak, E. Stefaniak, C. Hureau, L. Raibaut, W. Bal and P. Faller, N-Terminal Cu-Binding Motifs (Xxx-Zzz-His, Xxx-His) and Their Derivatives: Chemistry, Biology and Medicinal Applications, Chem. – Eur. J., 2018, 24, 8029–8041 CrossRef CAS PubMed.
- S. J. Lau and B. Sarkar, The interaction of copper(II) and glycyl-L-histidyl-L-lysine, a growth-modulating tripeptide from plasma, Biochem. J., 1981, 199, 649–656 CrossRef CAS PubMed.
- J. H. Freedman, L. Pickart, B. Weinstein, W. B. Mims and J. Peisach, Structure of the Glycyl-L-histidyl-L-lysine-Copper(II) Complex in Solution, Biochemistry, 1982, 21, 4540–4544 CrossRef CAS PubMed.
- J.-R. Park, H. Lee, S.-I. Kim, S.-R. Yang, J.-R. Park, H. Lee, S.-I. Kim and S.-R. Yang, The tri-peptide GHK-Cu complex ameliorates lipopolysaccharide-induced acute lung injury in mice, Oncotarget, 2016, 5, 58405–58417 Search PubMed.
- L. Pickart, J. M. Vasquez-Soltero and A. Margolina, GHK and DNA: Resetting the Human Genome to Health, BioMed Res. Int., 2014, 2014, 1–10 CrossRef PubMed.
- M. Maurer, M. Bader, M. Bas, F. Bossi, M. Cicardi, M. Cugno, P. Howarth, A. Kaplan, G. Kojda, F. Leeb-Lundberg, J. Lötvall and M. Magerl, New topics in bradykinin research, Allergy, 2011, 66, 1397–1406 CrossRef CAS PubMed.
- P. N. Aarsen and A. Kemp, Influence of metals on the activity of a bradykinin-destroying enzyme kininase, Br. J. Pharmacol. Chemother., 1962, 19, 442–450 CrossRef CAS.
- P. Bünning and J. F. Riordan, The functional role of zinc in angiotensin converting enzyme: implications for the enzyme mechanism, J. Inorg. Biochem., 1985, 24, 183–198 CrossRef.
- J. A. Loo, P. Hu and R. D. Smith, Interaction of angiotensin peptides and zinc metal ions probed by electrospray ionization mass spectrometry, J. Am. Soc. Mass Spectrom, 1994, 5, 959–965 CrossRef CAS PubMed.
- M. Volpe, M. Carnovali and V. Mastromarino, The natriuretic peptides system in the pathophysiology of heart failure: from molecular basis to treatment, Clin. Sci., 2015, 130, 57–77 CrossRef PubMed.
- P. A. Deddish, B. M. Marcic, F. Tan, H. L. Jackman, Z. Chen and E. G. Erdös, Neprilysin inhibitors potentiate effects of bradykinin on B2 receptor, Hypertension, 2002, 39, 619–623 CrossRef CAS.
-
A. J. Turner and N. N. Nalivaeva, Peptide Degradation (Neprilysin and Other Regulatory Peptidases), Elsevier Inc., 2nd edn, 2013 Search PubMed.
- J. D. Browning, P. G. Reeves and B. L. O’Dell, Zinc deficiency in rats reduces the vasodilation response to bradykinin and prostacyclin, J. Nutr., 1987, 117, 490–495 CrossRef CAS PubMed.
- B. A. Cerda, L. Cornett and C. Wesdemiotis, Probing the interaction of alkali and transition metal ions with bradykinin and its des-arginine derivatives via matrix-assisted laser desorption/ionization and postsource decay mass spectrometry, Int. J. Mass Spectrom., 1999, 193, 205–226 CrossRef CAS.
- B. Ivanova and M. Spiteller, Coordination ability of bradykinin with ZnII- and AgI-metal ions – experimental and theoretical study, Inorg. Chim. Acta, 2012, 392, 211–220 CrossRef CAS.
- I. Naletova, V. G. Nicoletti, D. Milardi, A. Pietropaolo and G. Grasso, Copper, differently from zinc, affects the conformation, oligomerization state and activity of bradykinin, Metallomics, 2016, 8, 750–761 RSC.
- M. Prudent and H. H. Girault, On-line Electrogeneration of Copper–Peptide Complexes in Microspray Mass Spectrometry, J. Am. Soc. Mass Spectrom, 2008, 19, 560–568 CrossRef CAS PubMed.
- J. Dong and R. W. Vachet, Coordination Sphere Tuning of the Electron Transfer Dissociation Behavior of Cu(II)-Peptide Complexes, J. Am. Soc. Mass Spectrom, 2012, 23, 321–329 CrossRef CAS PubMed.
- C. H. Eger, C. Yarborough, M. Greiner and D. A. Norton, The Participation of the 17B Side Chain of Corticosteroids in the Formation of Complexes with Co(II), Steroids, 1972, 250, 349–360 CrossRef.
- T. J. Schmidt, C. A. Barnett and G. Litwack, Activation of the glucocorticoid–receptor complex, J. Cell. Biochem., 1982, 20, 15–27 CrossRef CAS PubMed.
- J. Kerkay and U. Westphal, Steroid-protein interactions, Arch. Biochem. Biophys., 1969, 129, 480–489 CrossRef CAS PubMed.
- A. Jackson, J. Davis, R. J. Pither, A. Rodger and M. J. Hannon, Estrogen-derived steroidal metal complexes: Agents for cellular delivery of metal centers to estrogen receptor-positive cells, Inorg. Chem., 2001, 40, 3964–3973 CrossRef CAS PubMed.
- P. D. Darbre, Metalloestrogens: An emerging class of inorganic xenoestrogens with potential to add to the oestrogenic burden of the human breast, J. Appl. Toxicol., 2006, 26, 191–197 CrossRef CAS PubMed.
- E. R. Simpson and D. L. Williams-Smith, Effect of calcium(ion) uptake by rat adrenal mitochondrial on pregnenolone formation and spectral properties of cytochrome P-450, Biochim. Biophys. Acta, Gen. Subj., 1975, 404, 309–320 CrossRef CAS.
- J. K. Kresovich, M. Argos and M. E. Turyk, Associations of lead and cadmium with sex hormones in adult males, Environ. Res., 2015, 142, 25–33 CrossRef CAS PubMed.
- I. S. Butler, A. Vessières and G. Jaouen, Application of Organotransition Metal Carbonyl Complexes as Infrared Markers for Hormonal Steroids in Biological Processes, Comments Inorg. Chem., 1989, 8, 269–286 CrossRef CAS.
- P. Garcia-Morales, M. Saceda, N. Kenney, N. Kim, D. S. Salomon, M. M. Gottardis, H. B. Solomon, P. F. Sholler, V. C. Jordan and M. B. Martin, Effect of cadmium on estrogen receptor levels and estrogen-induced responses in human breast cancer cells, J. Biol. Chem., 1994, 269, 16896–16901 CAS.
- A. Stoica, B. Katzenellenbogen and M. Martin, Activation of estrogen receptor-alpha by the heavy metal cadmium, Mol. Endocrinol., 2000, 14, 545–553 CAS.
- Y. Zang, S. Odwin-DaCosta and J. D. Yager, Effects of cadmium on estrogen receptor mediated signaling and estrogen induced DNA synthesis in T47D human breast cancer cells, Toxicol. Lett., 2009, 184, 134–138 CrossRef CAS PubMed.
- S. A. Ronchetti, E. A. Miler, B. H. Duvilanski and J. P. Cabilla, Cadmium mimics estrogen-driven cell proliferation and prolactin secretion from anterior pituitary cells, PLoS One, 2013, 8, 1–13 CrossRef PubMed.
- W. Y. Chan, K. W. Chung, J. Bates, L. Blomberg and O. M. Rennert, Organ specific zinc deficiency in testicular feminization rats: hormone–metal interaction, Biochem. Biophys. Res. Commun., 1981, 102, 630–635 CrossRef CAS PubMed.
- A. Fallah, A. Mohammad-Hasani and A. H. Colagar, Zinc is an essential element for male fertility: A review of Zn roles in men's health, germination, sperm quality, and fertilization, J. Reprod. Infertil., 2018, 19, 69–81 Search PubMed.
Footnote |
† These authors contributed equally. |
|
This journal is © The Royal Society of Chemistry 2019 |
Click here to see how this site uses Cookies. View our privacy policy here.